- 1Co-Innovation Center for Sustainable Forestry in Southern China, College of Forestry, Nanjing Forestry University, Nanjing, China
- 2Environmental Sciences, School of Agriculture and Environment, Massey University, Palmerston North, New Zealand
- 3Key Laboratory of Soil and Water Conservation and Ecological Restoration of Jiangsu Province, Nanjing Forestry University, Nanjing, China
Biochar application can improve crop yield, reduce ammonia (NH3) volatilization and nitrous oxide (N2O) emission from farmland. We here conducted a pot experiment to compare the effects of biochar application on rice yield, nitrogen (N) uptake, NH3 and N2O losses in paddy soil with low, medium, and high N inputs at 160 kg/ha, 200 kg/ha and 240 kg/ha, respectively. The results showed that: (1) Biochar significantly increased the rice grain yield at medium (200 kg/ha) and high (240 kg/ha) N inputs by 56.4 and 70.5%, respectively. The way to increase yield was to increase the rice N uptake, rice panicle number per pot and 1,000 grain weight by 78.5–96.5%, 6–16% and 4.4–6.1%, respectively; (2) Under low (160 kg/ha) N input, adding biochar effectively reduced the NH3 volatilization by 31.6% in rice season. The decreases of pH value and NH4+-N content in surface water, and the increases of the abundance of NH4+-N oxidizing archaea and bacteria (AOA and AOB) communities contributed to the reduction of NH3 volatilization following the biochar application; (3) Under same N input levels, the total N2O emission in rice season decreased by 43.3–73.9% after biochar addition. The decreases of nirK and nirS gene abundances but the increases of nosZ gene abundance are the main mechanisms for biochar application to reduce N2O emissions. Based on the results of the current study, adding biochar at medium (200 kg/ha) N level (N200 + BC) is the best treatment to synchronically reduce NH3 and N2O losses, improve grain yield, and reduce fertilizer application in rice production system.
1. Introduction
China is a large rice growing country, with about 3.10 × 107 ha of rice field cultivation area. China rice production accounts for 27% of global rice production and about 35% of China’s total grain production (Ullah et al., 2023). To meet the global population in rice demand, farmers apply inorganic nitrogen (N) fertilizer to increase rice yield (Chen C.R. et al., 2013; Chen T.T. et al., 2013; Chen et al., 2014). However, farmers often apply excessive N fertilizer inputs (more than 300–350 kg/ha/year) in the pursuit of maximum rice yields (Ali et al., 2020). Previous studies have demonstrated that approximately 50% of applied inorganic fertilizer N is lost either through emissions or leaching, which have detrimental effects to the atmosphere and water environment (Cao et al., 2013; Li et al., 2017). Of which, ammonia (NH3) volatilization is one major N loss pathway and account for 10–60% of the total N fertilizer input in the rice season. Moreover, nitrous oxide (N2O) emissions from rice fields can account for 7–11% of N2O emissions from agricultural fields in China (Zou et al., 2007; Cai et al., 2017). The N2O emission can deplete the stratosphere and accelerate climate change. Therefore, ensuring food security while reducing fertilizer N environmental losses by coupling other soil additives is a major ongoing concern in terms of agricultural production and ecological environment in China.
In previous literatures, several management practices have been recommended to reduce N losses in paddy fields such as the use of biochar. Biochar is a type of solid material produced by high-temperature pyrolysis carbonization of biomass under anaerobic conditions. It is characterized with well-developed pore structure, high carbon content and large specific surface area, which results to it having high stability and strong adsorption performance (Zou et al., 2007; Tian et al., 2020). Biochar itself carries micronutrients for crop growth (Pyoungchung et al., 2011) and can also increase soil carbon stocks, promote nutrient cycling and sequestration, and improve crop yields (Gaskin et al., 2008; Novak et al., 2009; Ali et al., 2020). For example, Mcconnell et al. (2007), demonstrated that biochar application with additional N (240 kg/ha) fertilizer increased rice yield by 49.7%, attributing to the increasing effective spike number, spike grain number and 1,000 grain weight. In addition, biochar application in agricultural fields can improve soil quality and reduce nutrient losses, thus increasing crop yields (Steiner et al., 2007). Apart from influencing yields, biochar application has been widely used to reduce pollutants in agricultural production processes (Sun et al., 2019). The amended biochar itself can adsorb NH3 and achieve NH3 volatilization reduction by enhancing soil N fixation capacity and increasing soil nitrification rate (Chen C.R. et al., 2013; Chen T.T. et al., 2013). However, the presence of high salt-based ions such as calcium and magnesium in biochar has been shown to have the potential to exchange with hydrogen and aluminum ions in the soil, etc., and this effect usually raises soil pH and leads to increased NH3 volatilization (Wang et al., 2011; Clough et al., 2013). Meanwhile, the effect of biochar on N2O emissions from rice fields has been inconsistently reported. Soil NH4+ content is a key factor affecting N2O emission rate, and biochar addition can slow down the denitrification process and reduce soil NH4+ content, thus reducing the N2O emission rate (Wang et al., 2011; Yang et al., 2020). However, some studies have also found no effect of biochar on soil N2O emission, or even a promoting effect (Clough et al., 2010; Wang et al., 2011). Several studies have demonstrated that the effects of biochar addition on NH3 volatilization and N2O emissions is highly influenced by environmental factors, biochar application rate, soil type and cropping system (Sha et al., 2019). However, limited studies investigated the comprehensive effects of biochar on NH3 volatilization and N2O emission in a whole crop grow cycle. Therefore, there is a need to undertake studies that cover a major share of the gaseous N loss pathways in rice production.
The integrated evaluation of crop yield, soil NH3 volatilization and N2O emission in response to N fertilizer application and exogenous substance addition has been a key area of research in the evaluation of the effects of on-farm management practices. However, most of the published recent studies have evaluated the effect of biochar application to agricultural fields based on a single level of N application rate (Ma et al., 2013). However, there is no clear evidence on the application of biochar with different rates of N fertilizer. This could help to underpin the best combination of biochar and N fertilizer with less N losses and without reducing rice yields. Hence, this study evaluated the effects of biochar addition on rice yield, NH3 volatilization and N2O emission at different levels of N supply. Further, explain the mechanistic effect of biochar on N losses based on N uptake, field water pH and ammonium N (NH4+-N), soil NH4+-N, and nitrate (NO3−-N) contents and functional microbial gene abundance by a pot experiment. The results and conclusions of the study can provide technical support and theoretical basis for the mutual interaction of N fertilizer and biochar to achieve N fertilizer reduction and biochar resource conservation, crop yield stabilization and environmental protection in rice production.
2. Materials and methods
2.1. Background information and soil column set-up
Test soil for this study was collected at depth of 0–20 cm from an approximately 30 ha paddy field in Zhoutie town of Yixing city (31° 28′ N, 119° 59′ E), Jiangsu Province, which is located at the Taihu Lake region of China. This region has a subtropical monsoon climate, with an annual mean air temperature and rainfall of 15.7°C and 1,177 mm, respectively. The soil was mixed, air-dried for approximately 10 days, ground and sieved through a 2-mm nylon sieve, and repacked layer-wise (0–10 and 10–20 cm) into soil columns (inner diameter 35 cm, height 28 cm) at similar bulk density (1.3 g/cm3) as in the field. About 20 kg soil was filled into each soil column according to the volume of soil column and the soil bulk density. The selected properties of 0–20 cm topsoil was as follows: pH 6.38 (soil: water ratio 1: 5), soil organic matter 29.2 g/kg, total N 1.72 g/kg, available P 23.1 mg/kg, available K 159.3 mg/kg, and CEC 22.6 cmol/kg. In this experiment, biochar was produced using wheat straw that had been heated to 500°C. The reactor was heated by a stepwise procedure under oxygen-limited conditions. For pyrolysis, the temperature was raised to 500°C at a rate of 5°C/min and held constant for 8 h. The measured properties of the biochar were pH 9.80, total N 0.81%, total C 37.5%, and BET surface area 32.0 m2/g. At the initiation of the experiment, at the same time of repacking soil into column/pot, biochar was mixed with the top layer (0–20 cm) soil.
2.2. Experimental treatments and rice management
This current experiment constituted seven treatments; urea only at low, medium, and higher rates of N with 160, 200, and 240 kg/ha, named NN160N200, andN240, respectively; N160, N200, and N240 plus biochar at 5 t/ha, named N160 + BC, N200 + BC, and N240 + BC, respectively; Meanwhile a and control treatment without urea and biochar was tested. The N fertilizer rates used on this study were based on deficiency, sufficiency, and over application. Each treatment was replicated three times, therefore there were totally 21 soil columns, Biochar was evenly incorporated into each soil column into the soil at a depth of 15 cm at the initiation of the experiment.
Rice (Oryza sativa L., var. Nangeng 46) seedlings (28 days old) were transplanted into the soil columns (three holes in each column and two plants per hole) on July 1, 2021. The total fertilizer N application was split into a basal dressing at transplanting, and two top-dressing during the season in the ratio of 40–30–30%, respectively. Application periods were July 1, July 16, and August 14 in 20,218. Calcium superphosphate and potassium chloride were applied to all treatments including the CK at the rates of 90 kg/ha (P2O5) and 150 kg/ha (K2O), respectively, at the time of transplanting as basal fertilizer. The floodwater was drained at a mid-season drainage period from July 31 to August 7, 2021 to control invalid tillering and improve the rice root development. During other times, a 3–5 cm depth floodwater level was maintained with tap water. Weeds and pests were controlled according to the local farmers’ traditional practices. The rice shoots (including straw and grain) were manually harvested on November 8, 2021.
2.3. Sampling and measurements
2.3.1. Crop
Rice plants were harvested at the physiological maturity stage to determine grain yield and its components. Before harvesting, the plant height was measured using a ruler, and the yield-related agronomic traits (number of panicles, number of grains per panicle, and 1,000 seed weight) were also recorded. Rice straw and grain were oven-dried at 105°C for 30 min, and then dried at 80°C until constant weight. The dried plant samples were ground into powder using a high-speed crusher (DS-YM-001), passed through a 0.2 mm nylon sieve. Ground plant samples were kept in sealed containers until digestion. Sub-sample (0.25 g) of ground plant samples were digested in a mixture of H2SO4 and H2O2 and used for determination of total N content using the Kjeldahl method detailed in Sun et al. (2015). Rice NUE was calculated using Equation 1 outlined by Dong et al. (2015):
(1)
Where and denote the N uptake as measured at harvest in the fertilizer applied and the control treatments (kg/ha), respectively, while denotes the N fertilizer added rate (160, 200, and 240 kg/ha in the current work).
To reflect the leaf chlorophyll content, SPAD values of rice leaves were measured using the chlorophyll content meter (SPAD-502Plus, Japan) at the tillering, earing, and maturation stage, respectively. This was done by selecting three rice plants from each soil column and three leaves of each rice plant were measured. Therefore, the SPAD values presented in this study represented average SPAD value of the three plants in each replicate (Li et al., 2019).
2.3.2. NH3 volatilization
The daily NH3 volatilization rates were measured at three N fertilizer applications, using the sponge absorption method (Rochette et al., 2013). The gas-capturing device was made of polyvinylchloride (PVC) plastic tube with an inner diameter of 15 cm and a height of 15 cm. Two sponges with a thickness of 2 cm and a diameter of 16 cm were dipped in 15 mL of phosphoglycerol (50 mL of phosphoric acid plus 40 mL of glycerol and then diluted to 1,000 mL with deionized water) and placed in a plastic tube. The lower sponge was 5 cm from the bottom of the tube and the upper sponge was at the top of the tube.
During sampling, the lower sponge was taken out (8: 00 am), immediately sealed in a bag, and replaced in the gas-capturing device with a new sponge also beforehand dipped in phosphoglycerol. The upper sponge was replaced once every 2 days. The sampled sponge was placed into a 500 mL plastic bottle, submerged in 300 mL of 1 M KCl, and shook at 180 r per minute for 1 h. The NH4+-N concentration in the extract was determined by an autoanalyzer (SKALAR San++ System, Netherlands). The NH3 volatilization was calculated using Equation 2 outlined below:
ω = ×10−3(2)
where, ω: NH3 content in a single collection device (mg); : NH4+-N concentration (mg/L); : the volume of solution used to measure absorbance after constant volume (mL); : the KCl solution volume for extracting ammonium from sponge (mL); : the volume of extracting solution used for measurement (mL). The NH3 emission factor and yield-scale NH3 volatilization were calculated according to that introduced in our previous work (Min et al., 2021).
2.3.3. N2O emission
The gas samples for N2O determination were collected using the modified closed chamber method as described in Min et al. (2021). The chamber was a transparent Plexiglas cylinder with 100 cm height and 36 cm inner diameter (adjusted for the height of rice plant and the pot size), and covered with Al foil to exclude light. It was fitted into a grove at the bottom (for sealing by tap water in the grove) and had a small fan at the top to properly mix gas before sampling.
Gas samples were collected using a plastic syringe at 15 min intervals. We took the gas samples on the 2nd, 4th, 6th, and 8th day after each N fertilization application and during water drainage period. Thereafter, sampling was done every 10 days until harvest. The sponge absorption device was temporarily moved out during the N2O measurement, to avoid any disturbance that may occur. When calculated the NH3 flux, we adjusted the cover time according to the fact. Gas sample collection was done between 6:00–8:00 a.m. Meanwhile, air temperature in each collection device was recorded at collection. Four gas samples were collected using a 50-mL medical syringe at 0, 15, 30, and 45 min after the collection device was sealed. The gas samples were then injected into pre-evacuated 50 ml vacuum bottles fitted with butyl rubber lids for laboratory analysis. The N2O concentrations were determined using a gas chromatograph (Agilent 7890B, Agilent Technologies, United States) at 350°C equipped with an electron capture detector (ECD). Total N2O emission was calculated from the individual fluxes and the interval times (Sun et al., 2022).
2.3.4. NH4+-N, NO3−-N concentrations and pH in overlying water
Overlying water samples were collected using a syringe on the same day and time as NH3 volatilization samples collection. Collected water samples were filtered through a 0.45 μm membrane, then analyzed for pH using a combined reference electrode (Ф255 pH/temp/mV meter, Coulter Bechman Co., United States). A sub-sample of 50 ml filtered water was stored in clean plastic bottles at −20°C for further analysis. The concentrations of NH4+-N and NO3−-N in overlying water were determined by an autoanalyzer (SKALAR San++ System, Netherlands).
2.3.5. Soil properties
At the end of the experimental period after rice harvest, three soil cores in each pot were randomly sampled at 0–20 cm depth using a soil drill (50 mm in diameter), top layer soil was sampled at selected points. Soil samples were composited, mixed manually, placed in self-sealing bags, and brought back to the laboratory in collar box with ice. Soil samples were divided into two parts: one was stored at −80°C for molecular analysis and another at −20°C for analysis of other properties. The soil pH was measured in the 1:2.5 (w/v) soil: water suspension using a combined reference electrode (Ф255 pH/temp/mV meter). Soil samples were extracted with 2.0 M KCl (1:5 soil: extractant, w/v) for NH4+-N and NO3−-N determination. Soil extracts were filtered through a 0.45 μm membrane filter and the NH4+-N and NO3−-N concentrations were determined using an autoanalyzer (SKALAR San++ System, Netherlands). The gene copy numbers of AOA and AOB amoA, nirK, nirS, and nosZ of soil samples were determined by Shanghai Majorbio Biomedical Co., Ltd. according to the procedures detailed in Chu et al. (2020) and Ye et al. (2021).
2.4. Data analysis
A statistical analysis was performed using SPSS 22.0, and one-way analysis of variance (ANOVA) was used to determine the significance of the difference between treatments. The level of significance was measured using Duncan’s multiple-comparison test (p < 0.05).
3. Results
3.1. Rice yield and nitrogen uptake
3.1.1. Rice yield and its component factors
The results in Table 1 showed that N200 and N240 treatments significantly (p < 0.05) increased the straw biomass by 54.0 and 77.0% relative to the control treatment. Both N160 and N160 + BC had no difference in straw biomass compared to the control. The combinations of BC with N200 and N240 significantly (p < 0.05) increased rice straw biomass by 79.5 and 42.3% compared to N200 and N240 alone, respectively. However, there was no difference in straw biomass between N160 + BC and N160. Interestingly, the grain yield in N added treatments (70.53–145.50 g/pot) were significantly (p < 0.05) higher than the no N added control treatment (42 g/pot). Moreover, N200 + BC and N240 + BC treatments significantly (p < 0.05) promoted the rice grain yield by 31.9 and 70.6% relative to N200 and N240 treatments, respectively. Nevertheless, amendment of biochar induced no difference in rice grain yield at low N input level (160 kg/ha).
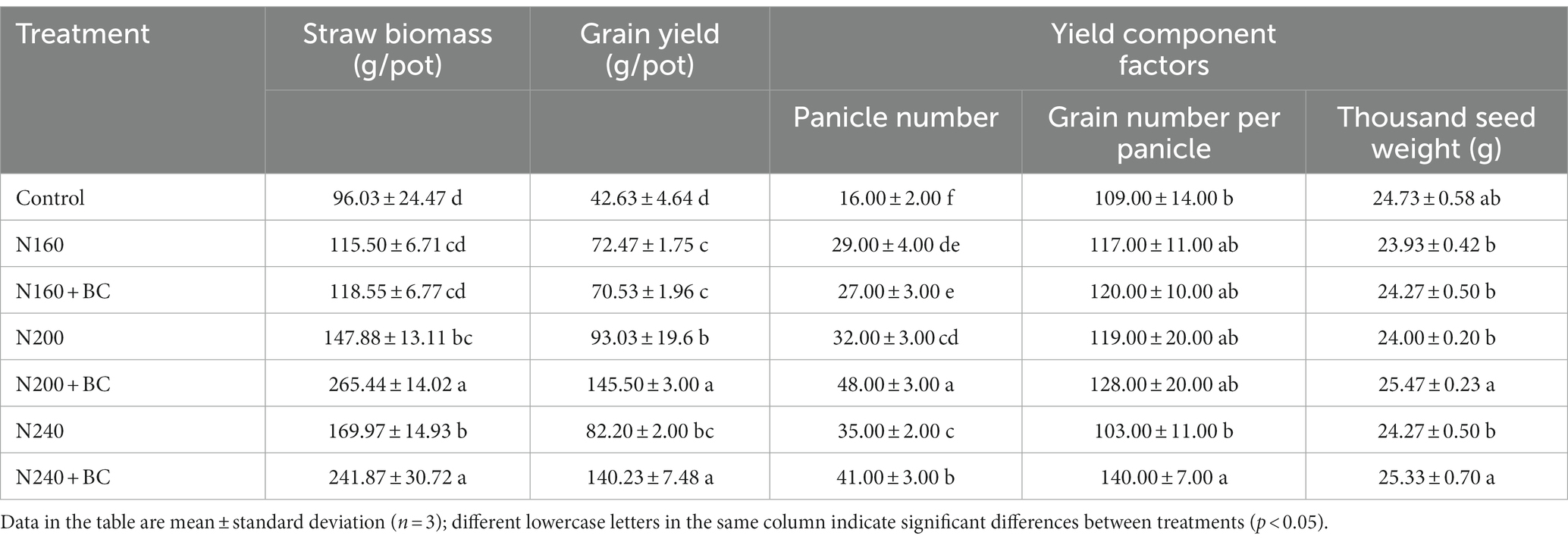
Table 1. Effects of biochar application and N fertilizer reduction on rice straw biomass, grain yield and its component factors.
Moreover, the number of panicles and 1,000 grain weight of rice in N200 + BC treatment were significantly (p < 0.05) 16 panicles/pot and 6.1% higher than N200, respectively. Compared with N240 treatment, however, N240 + BC treatment significantly (p < 0.05) increased the number of panicles, grains per panicle and 1,000 grain weight of rice by 6 panicles/pot, 35.9, and 4.4%, respectively (Table 1).
3.1.2. Rice plant height and leaf SPAD
At the tillering stage, either N fertilizer reduction or biochar addition had no effect on rice plant height (Figure 1A), but the SPAD value of rice leaf in N240 + BC treatment was significantly increased by 8.6% (p < 0.05) compared with N240 treatment (Figure 1B). At earing and maturation stages, rice plant height of rice was significantly (p < 0.05) increased by 5.0–11.0% and 7.1–19.5%, and rice leaf SPAD value was significantly (p < 0.05) increased by 9.9–13.4% and 17.6–29.9% (p < 0.05) when biochar was applied at medium (200 kg/ha) and high N (240 kg/ha) application levels. Among the different treatments at both early and maturing stage, the N200 + BC treatment had the highest values in terms of rice plant height and leaf SPAD value (Figure 1).
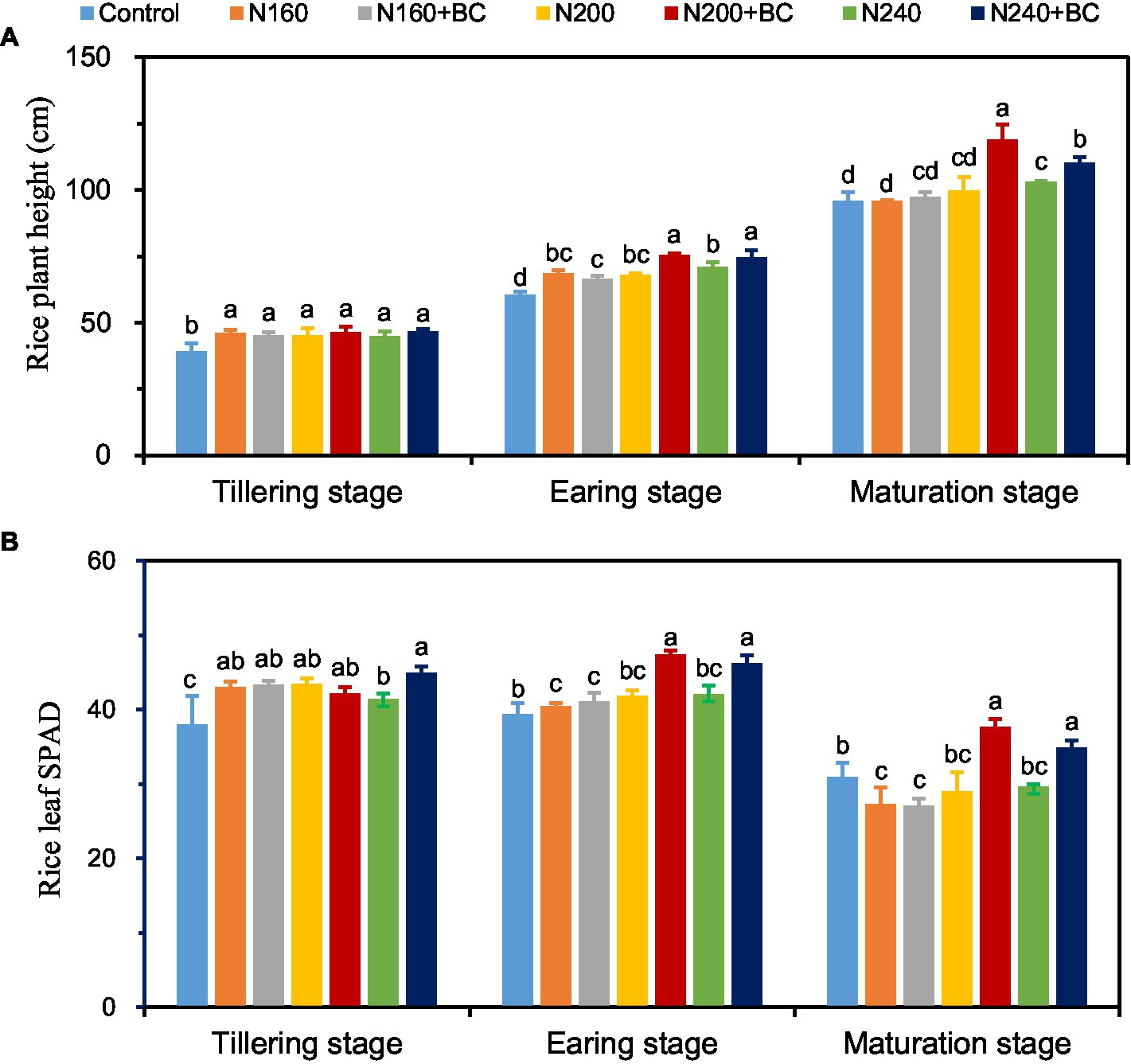
Figure 1. Effects of biochar application and N fertilizer reduction on rice plant height (A) and flag leaf SPAD (B) at different growth stages. Different lowercase letters above the columns indicate significant differences between treatments (p < 0.05).
3.1.3. N content and uptake of rice straw and seeds
No significant difference in N content and uptake by rice straw among the treatments with N fertilizer but without biochar (Table 2). However, the seed N content and uptake increased when N addition increased from 160 kg/ha to 200 kg/ha, but decreased when N addition further increased to 240 kg/ha The N200 treatment showed a significant (p < 0.05) increase of 9.7 and 40.7% in seed N content and uptake, compared with N160 treatment, respectively. Biochar addition significantly (p < 0.05) increased rice straw N content by 39.8–75.7% and N uptake by 148–152% at medium and high N supply. Overall, biochar addition exerted no effect on seed N content of rice receiving same N fertilizer, while biochar addition significantly (p < 0.05) increased seed N uptake by 54.7–73.0% at middle (200 kg/hm2) and high (240 kg/hm2) N supply. Meanwhile, the total N uptake (straw + seeds) in N200 + BC and N240 + BC treatments was 1.8 and 2.0 times higher compared to N200 and N240 treatments, respectively (Table 2).
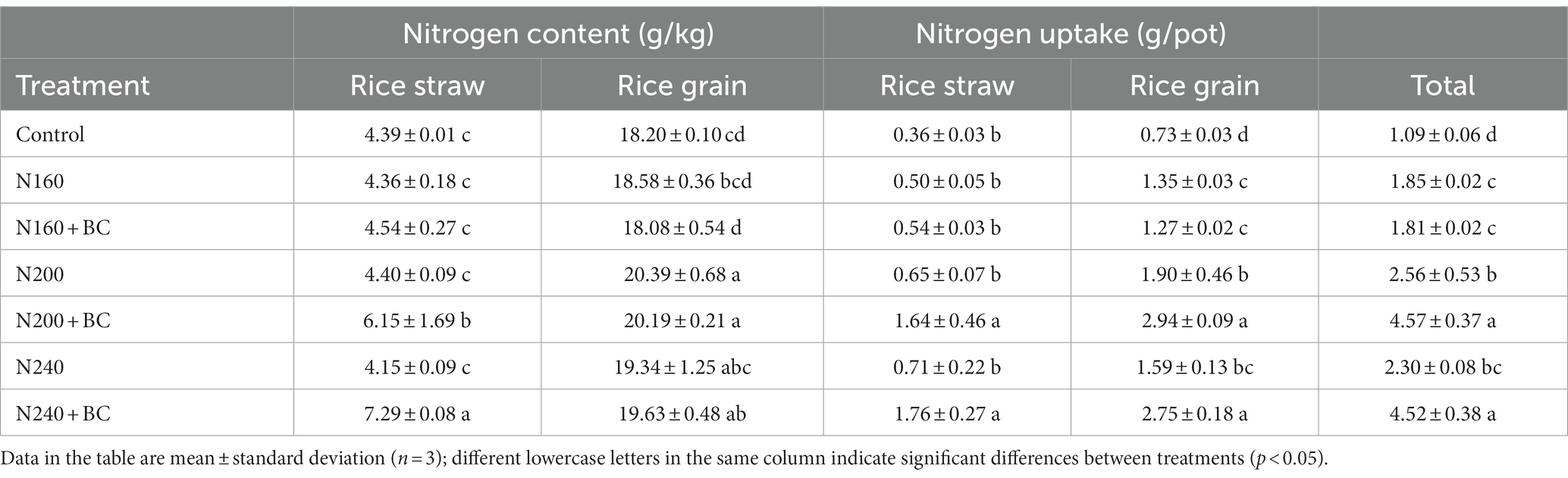
Table 2. Effect of biochar addition and N fertilizer reduction on total N content and uptake of rice straw and grain.
3.2. NH3 volatilization, emission factor and yield-scale NH3 volatilization
As shown in Supplementary Figure 1, during BF observation, the peak NH3 volatilization rate (24.95–67.03 mg/pot/d) was observed on the third days after BF, while the peak NH3 volatilization rate (29.48–110.63 mg/pot/d) was observed on the first day after SF2. Except for the N240 treatment, NH3 volatilization could reach its peak (41.33–74.84 mg/pot/d) within the first 3 days after SF1. The NH3 volatilization rate in all N fertilizer applied treatments dropped to a low level as that in the control treatment within 7 days after N each fertilizer application conducted.
The cumulative NH3 volatilizations in the rice season under N applied treatments were 0.20–0.53 g/pot, accounting for 10.5–28.7% of the fertilizer N input into the rice paddy. The NH3 losses after BF, SF1 and SF2 were 0.06–0.18 g/pot, 0.09–0.23 g/pot, and 0.05–0.20 g/pot, respectively (Table 3). N160 + BC treatment reduced the NH3 volatilization at both SF1 (38.9%) and SF2 (37.2%) observations, compared with N160 treatment, and this effect resulted in an overall significant (p < 0.05) reduction in cumulative amount of NH3 volatilization by 31.6%. Moreover, the application of biochar at N160 level significantly (p < 0.05) reduced the NH3 emission factor by 37.2%. Nevertheless, the total NH3 losses and emission factor in the rice season were not influenced by biochar at 200 kg/ha and 240 kg/ha applications. Interestingly, biochar addition significantly (p < 0.05) reduced yield-scale NH3 volatilization at all three N application levels by 29.7, 52.7, and 46.3% relative to N160, N200, and N240 kg/ha supply, respectively (Table 3).
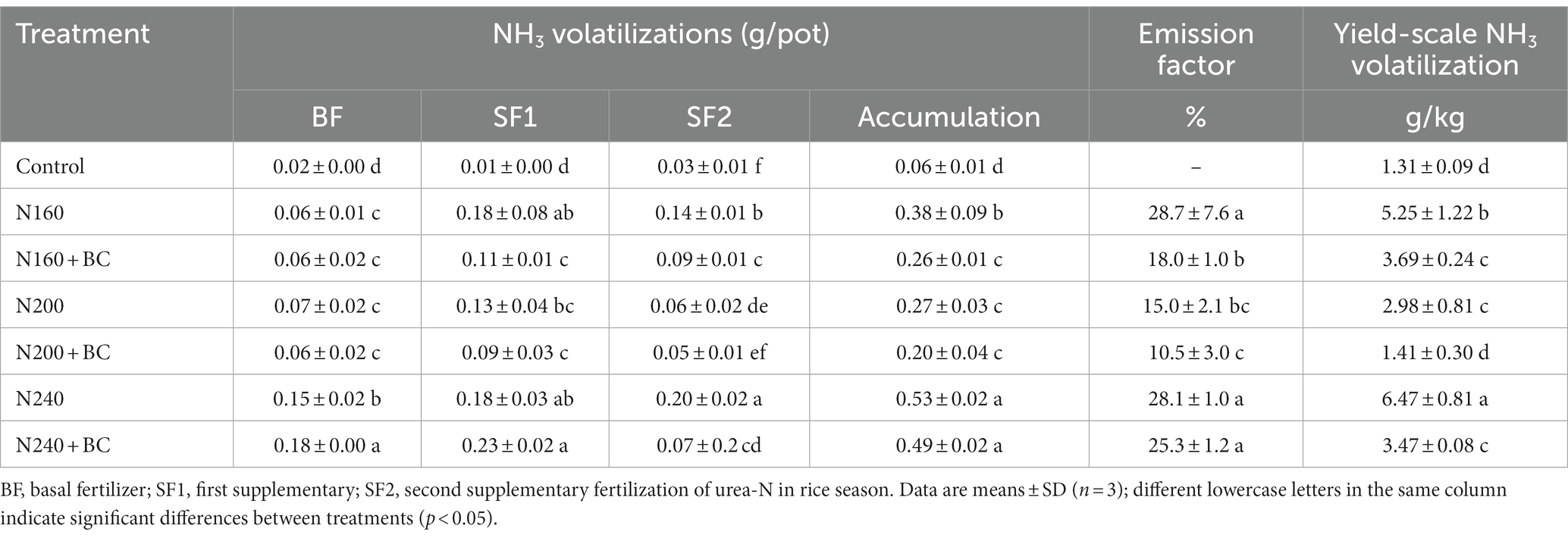
Table 3. Effect of biochar application and N fertilizer reduction on the accumulation of NH3 volatilization, emission factor and yield-scale NH3 volatilization at different fertilization periods and the whole reproductive period of rice.
3.3. N2O emission
Under no biochar additions, N2O emissions in the rice season increased significantly (p < 0.05) with the increasing N application (Figure 2). Biochar addition significantly (p < 0.05) reduced the cumulative N2O emissions in rice season by 54.1, 43.3, and 73.9% relative to N160, N200, and N240, respectively. Meanwhile, results in Figure 2 show that the cumulative N2O emissions of N200 + BC and N240 + BC treatments in rice season can be reduced to the N160 level, and the N2O emissions of N160 + BC treatment can be reduced to the control treatment level.
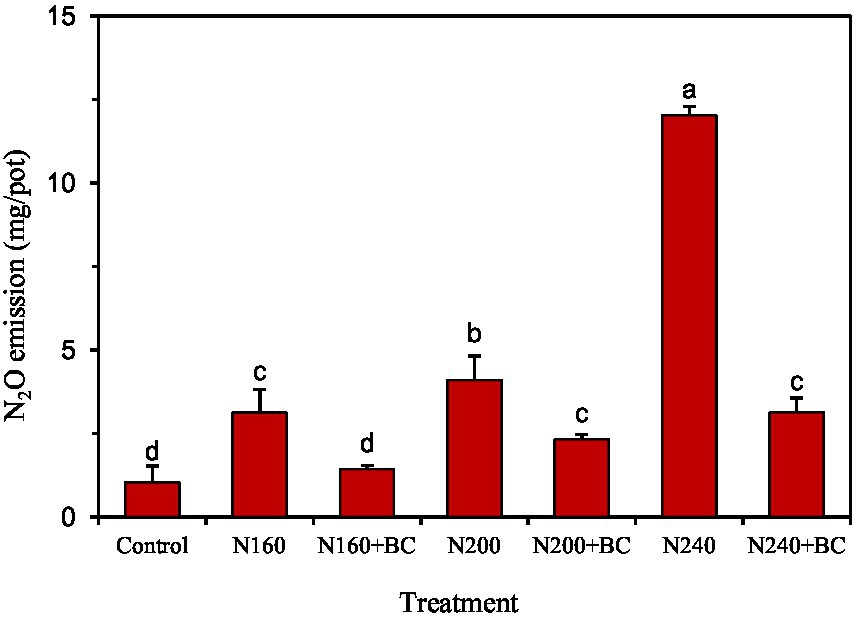
Figure 2. Effects of biochar application and N fertilizer reduction on cumulative N2O emission from rice paddy. Different lowercase letters above the columns indicate significant differences between treatments (p < 0.05).
3.4. NH4+-N and NO3−-N concentrations in overlying water
3.4.1. NH4+-N concentration in overlying water
The peak NH4+-N concentrations in the overlying water of each treatment during the BF occurred on the 2–5 days (16.1–29.0 mg/L) after N fertilizer application (Figure 3A). Except for the N240 treatment, on the first day after SF1 and SF2 applied, the NH4+-N concentrations of overlying water came to the peak with 27.8–77.5 mg/L and 71.7–172.4 mg/L, respectively (Figures 3B,C). After reaching the peak, the NH4+-N concentration in the overlying water of each treatment decreased rapidly to no significant difference among all treatments (Figure 3).
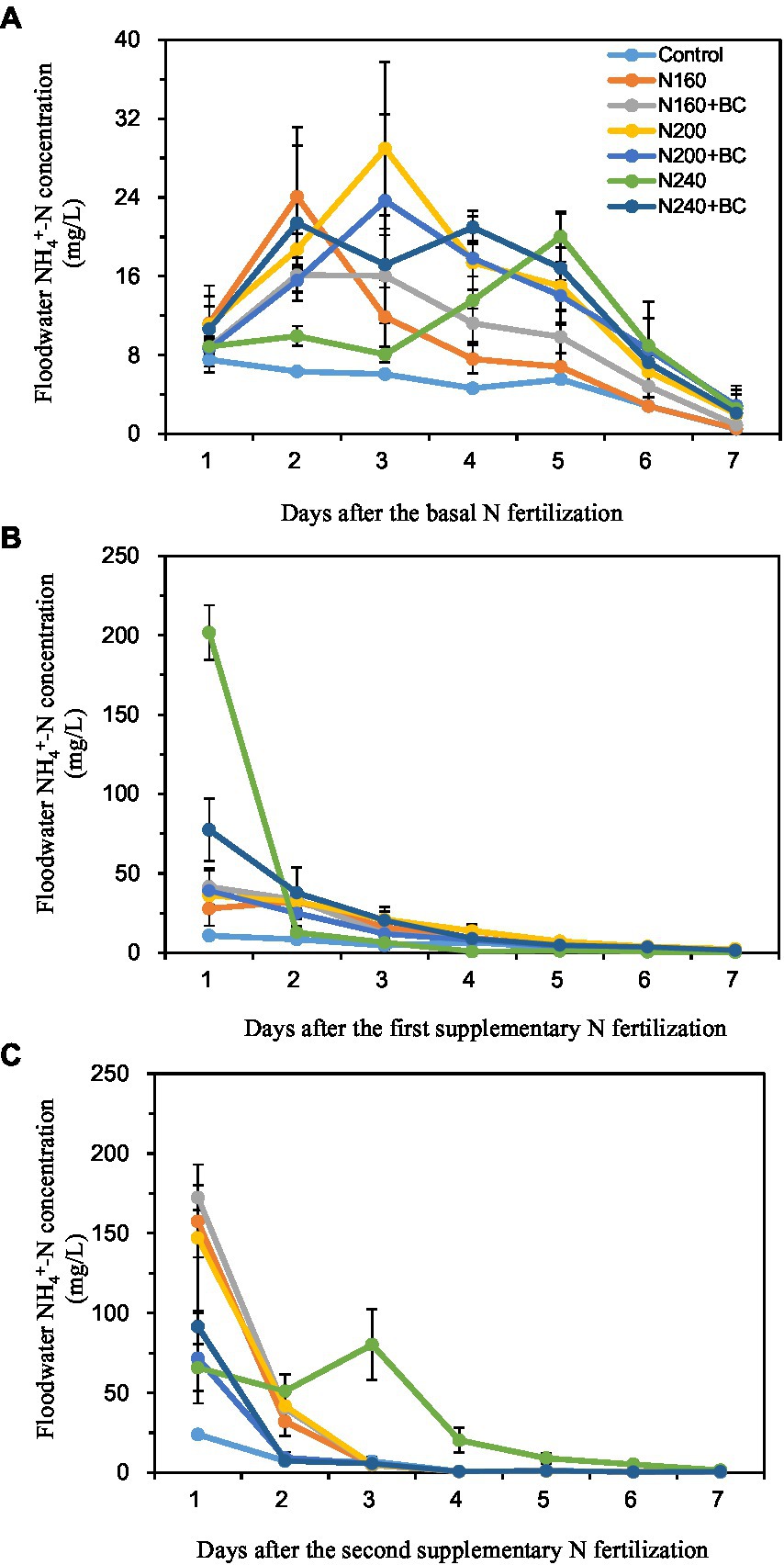
Figure 3. Effect of biochar application and N fertilizer reduction on ammonium nitrogen (NH4+-N) concentration in overlying water after applications of basal fertilizer (BF, A), first supplementary fertilizer (SF1, B) and second supplementary fertilizer (SF2, C).
At the BF observation, compared with N200, the average NH4+-N concentration of overlying water in N200 + BC decreased by 8.5%. However, biochar addition into low (160 kg/ha) and high (240 kg/ha) N supplied treatments increased the average NH4+-N concentration of overlying water by 4.4 and 33.9%, respectively. After the SF1 and SF2 applied, the addition of biochar at low (160 kg/ha) N supply level increased the average NH4+-N concentration of overlying water by 10.0 and 11.8%, respectively. Nevertheless, biochar addition at medium (200 kg/ha) and high (240 kg/ha) N supply levels reduced the mean overlying water NH4+-N concentrations by 20.3–54.5% and 31.0–53.7%, respectively, during the same periods.
3.4.2. NO3−-N concentration in overlying water
During the BF, the addition of biochar at all three levels of N supply increased the mean NO3−-N concentration in the overlying water by 16.2, 3.8, and 11.0%, respectively (Figure 4A). During the SF1, the addition of biochar at the medium (200 kg/ha) N level increased the mean NO3−-N concentration in the overlying water by 66.0%, but at the high (240 kg/ha) N level it decreased the mean NO3−-N concentration in the overlying water by 41.3%. During the SF2, the average NO3−-N concentration in the overlying water of N200 + BC and N240 + BC treatments was 3.5 and 1.2 times higher than that of N200 and N240 treatments, respectively (Figures 4B,C).
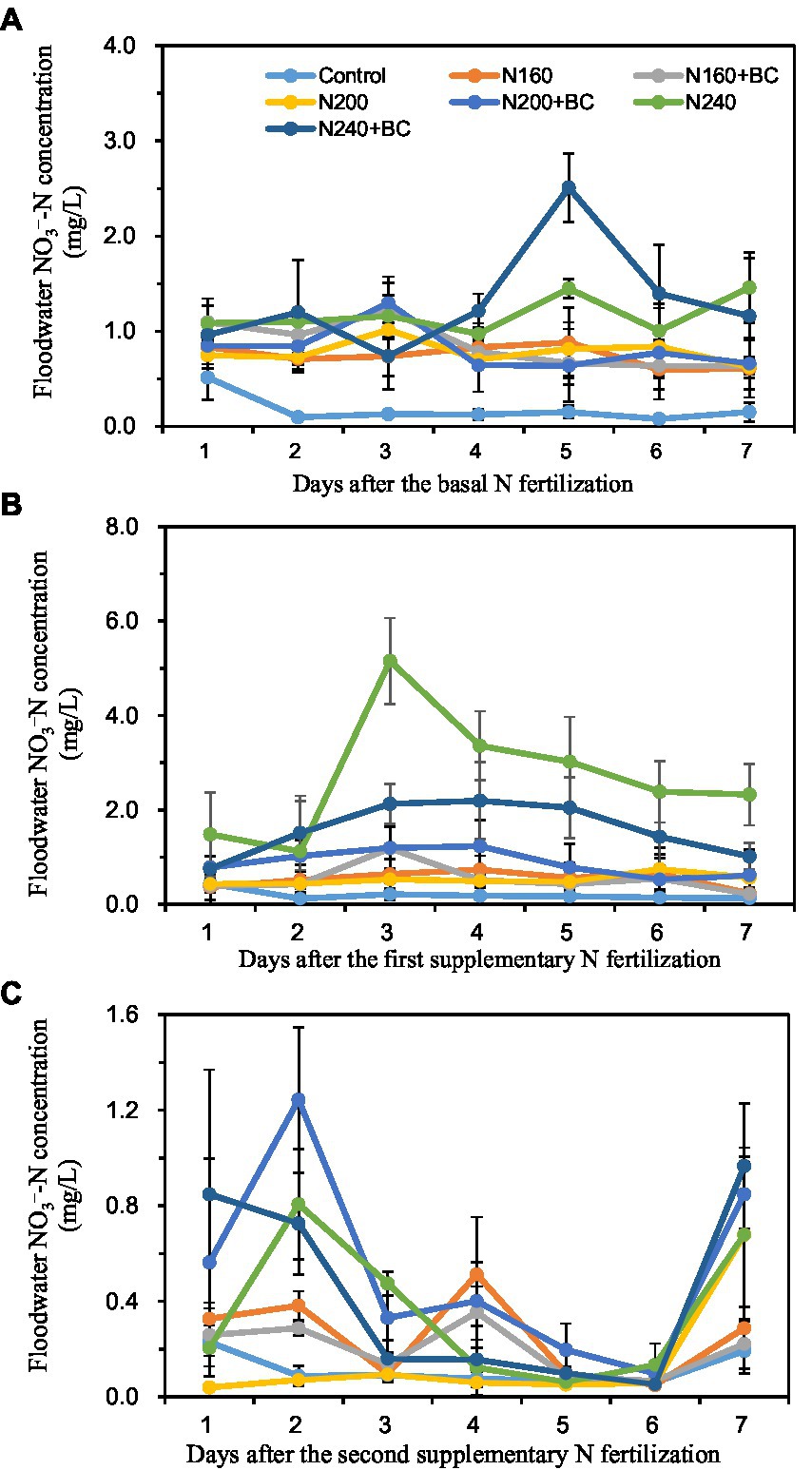
Figure 4. Effect of biochar application and N fertilizer reduction on nitrate N (NO3−-N) concentration of overlying water after applications of basal fertilizer (BF, A), first supplementary fertilizer (SF1, B) and second supplementary fertilizer (SF2, C).
3.5. Soil properties
3.5.1. Topsoil NH4+-N and NO3−-N content
During the BF, biochar had varied effects on soil NH4+-N content under low (160 kg/ha) and high (240 kg/ha) N fertilizer application conditions. The soil NH4+-N content in N160 + BC treatment was significantly (p < 0.05) higher than N160 treatment by 9.8%, while that in N240 + BC was significantly (p < 0.05) lower than N240 treatment by 15.0% (Figure 5A). The soil NH4+-N contents in the N240 + BC treatment were significantly (p < 0.05) 48.8 and 79.7% higher than N240 treatment during the SF1 and SF2, respectively.
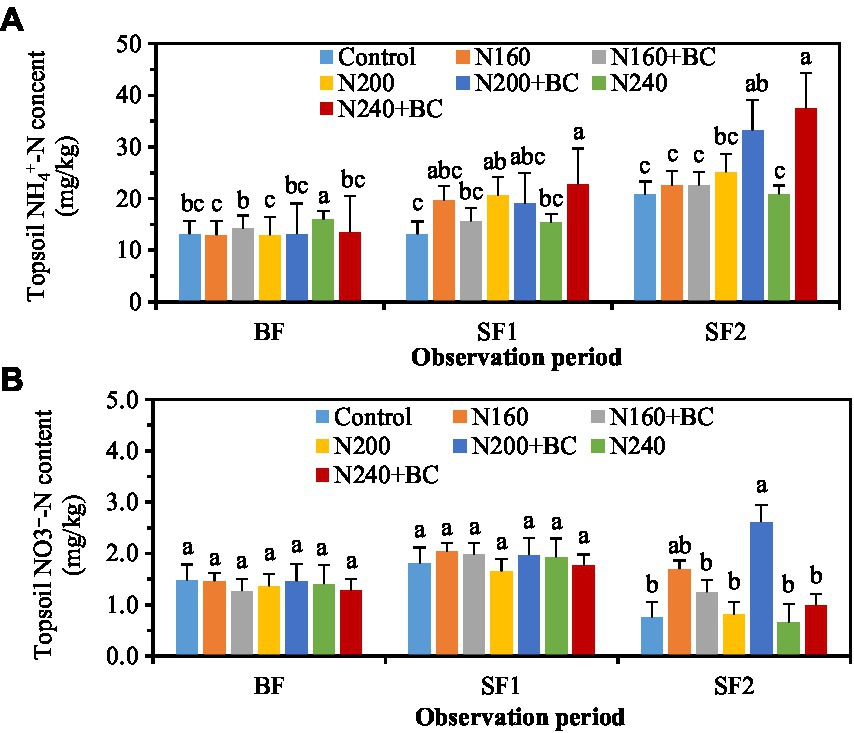
Figure 5. Effects of biochar addition and N reduction on the contents of NH4+-N (A) and NO3−-N (B) in soil at different fertilizer stages. The BF, SF1, and SF2 refer to the basal, first and second supplementary fertilizations, respectively. Different lowercase letters above the columns indicate significant differences between treatments (p < 0.05).
The soil NO3−-N content was not remarkably affected by N fertilizer application and biochar addition during the BF and SF1 (Figure 5B). During the SF2, biochar amendment at medium (200 kg/ha) N levels significantly (p < 0.05) increased the soil NO3−-N content, and the N200 + BC treatment had 3.3 times more soil NO3−-N content than the N200 treatment (Figure 5B).
3.5.2. Community abundance of ammonia oxidizing and denitrifying bacteria
Table 4 shows that the number of functional genes of ammonia-oxidizing archaea (AOA) and ammonia-oxidizing bacteria (AOB) amoA in paddy soil ranged from 2.23–6.22 × 105 copies/g and 1.73–5.09 × 105 copies/g, respectively. And the number of functional genes of denitrifying bacteria nirS, nirK and nosZ ranged from 1.51–16.34 × 107, 2.76–14.03 × 106 copies/g and 0.33–3.83 × 106 copies/g. For all treatments, the nirS gene showed higher dominance role. The community abundance of AOB and denitrifying bacteria showed a pattern of decreasing with increasing N application levels (Table 4). However, in N160 + BC and N240 + BC treatments, the soil AOA abundance significantly (p < 0.05) increased by 37 and 146%, relative to their counterparts N160 and N240 treatments, respectively. However, soil AOB abundance under medium (200 kg/ha) N supply conditions was significantly (p < 0.05) increased by 1.5 times with the addition of biochar.
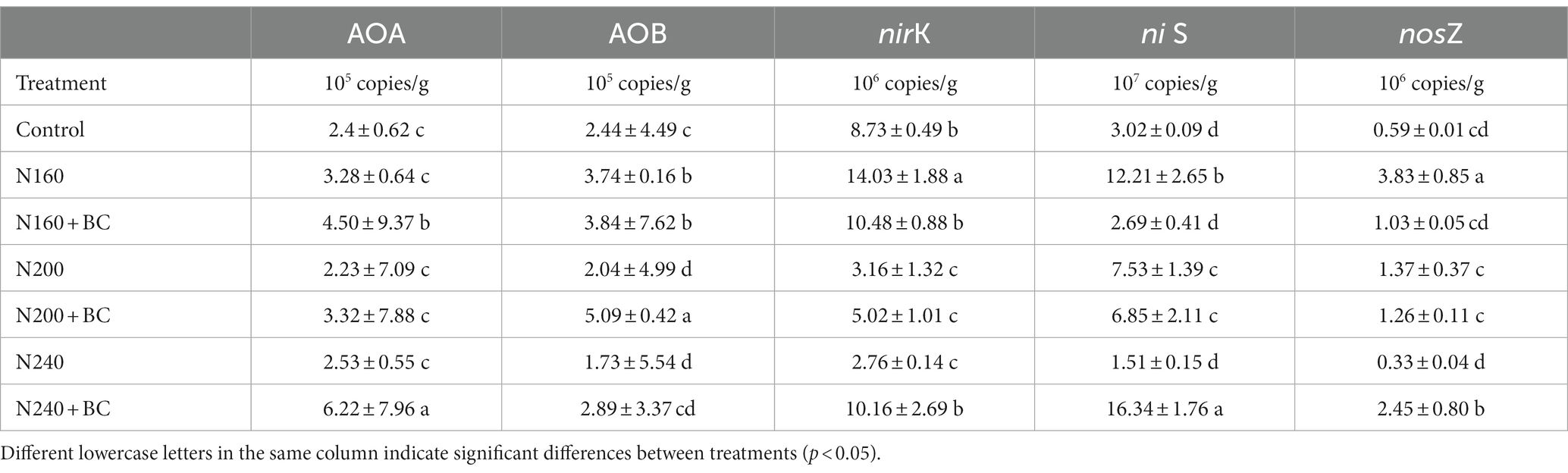
Table 4. Effect of biochar application and nitrogen fertilizer reduction on the abundance of soil ammonia oxidizing and denitrifying bacteria communities.
N160 + BC had significantly (p < 0.05) lower number of nirS, nirK, and nosZ functional genes by 78.0, 25.3, and 73.1%, respectively compared to N160. In contrast, the copies of nirS, nirK, and nosZ in N240 + BC soil were 10.8, 3.7, and 7.4 times significantly (p < 0.05) higher than N240 soil. No significant effect of biochar on the copies of all three denitrifying bacterial functional genes was found at medium (200 kg/ha) N supply level.
4. Discussion
4.1. Effect and mechanism of biochar on rice yield
Both exogenous material addition and N fertilizer application played a crucial role in the formation of rice yield (Timsina et al., 2001; Zhang et al., 2020). The results of this study showed that the combinations of N200 + BC and N240 + BC were effective in improving rice yield (Table 1). Similar results have been confirmed by Feng et al. (2021). They demonstrated that application of N fertilizer at 240 kg/ha with biochar increased the rice yield by 9.3% in a pot experiment. Results presented in the current study demonstrated that the addition of biochar at medium (200 kg/ha) and high (240 kg/ha) N levels promoted rice grain yield through increasing effective panicle number and 1,000 seed weight. However, there was no significant difference in panicle number, grain number per panicle and 1,000 seed weight between N160 + BC and N160 treatments, explaining the no yield-increasing effect of biochar on rice at 160 kg/ha N addition. Meanwhile, it has been shown that biochar can increase the growth rate of seedling rice and its mineral element uptake and dry matter accumulation (Ray et al., 2012). Further, combination of biochar and N at 240 kg/ha increased the rice plant height and leaf SAPD values (Figure 1). Therefore, this effect can also be inferred that promoting dry matter accumulation in rice and increasing leaf SPAD values are also one of the mechanisms of biochar to increase crop yield.
In terms of nutrient uptake, biochar addition has been well reported to improve crop N fertilizer utilization (Van Zwieten et al., 2010; Huang et al., 2018). Similarly, in this experiment we found that the addition of biochar at N200 and N240 treatments increased rice seed N and total plant N uptake capacities (Table 2). Therefore, promoting N uptake and utilization of rice is the second mechanism of biochar to improve rice yield under 200–240 kg/ha N supply conditions. The increase in N uptake under these treatments can be associated with the effect of biochar increasing the activity of soil microorganisms by providing them with an unstable carbon source. The presence of a C source can promote soil N mineralization thus improving soil N availability, and promotes plant N uptake (Saifullah et al., 2018). In addition, the addition of biochar can improve the stability of soil aggregates and crop root architecture (especially increase the number of fine roots) (Backer et al., 2017), as well as promote the uptake of other fast-acting nutrients by rice, thus ensuring the nutrient supply for the whole growth cycle of rice.
4.2. Effect of biochar on soil NH3 volatilization under different N fertilizer levels
This study found that the effect of biochar on NH3 volatilization in rice season was related to N input level, with a significant reduction of NH3 volatilization only at low (160 kg/ha) N level (Table 3). Under different experimental conditions, different views on the impact of biochar addition on NH3 volatilization in rice field ecosystem including promotion, reduction, and no impact, were reported (Meng et al., 2019; Shaukat et al., 2019). Liu et al. (2020) reported that appropriate N reduction form 180 kg/ha to 150 kg/ha with biochar helped to reduce NH3 volatilization and these results are consistent with the findings of our present study. In addition to environmental factors such as wind speed and temperature, the main factors affecting NH3 volatilization in rice fields include soil and overlying water pH and NH4+-N concentration, especially the changes in the corresponding indicators within 1 week after N fertilizer application (Soares et al., 2012; Mandal et al., 2018). The reduction effects of biochar on NH3 volatilization under low (160 kg/ha) N condition in this experiment were mainly found the SF1 (39%) and SF2 (36%). As shown in the Supplementary Figure 2, the addition of biochar at low (160 kg/ha) N input at both the SF1and SF2 reduced the mean pH of overlying water by 0.05 units. In addition, the mean soil NH4+-N and pH decreased by 4.07 mg/kg and 0.07 units, respectively, during the SF1 (Figure 5A; Supplementary Figure 3). In this study, the reduction of pH value and NH4+-N content by the N160 + BC at the SF1 and SF2 is linked to the reduction of NH4+-N potential for conversion to NH3, thus inhibiting the volatilization of NH3 (Feng et al., 2022).
Further, the addition of biochar at low (160 kg/hm2) N levels can increase the community abundance of AOB (Table 4). This is because previous study has found that the surface structural properties of biochar could provide habitat for AOB and increase the abundance and activity of AOB, which is consistent with the results of this work (Cheng et al., 2012). Increased abundance of AOB communities enhances soil nitrification, which can improve soil utilization of NH4+-N and reduce NH4+-N concentration in the soil liquid phase, thus reducing NH3 volatilization from rice fields (He et al., 2019). The soil urease activity directly affects the urea hydrolysis process, and largely determines the soil NH3 emission rate and amount (Yi et al., 2021). Previous studies have shown that biochar can adsorb urease molecules, and then protect the binding sites of enzymatic reaction, thus to prevent the enzymatic reaction and reduce NH3 volatilization (Noyce et al., 2015). In this experiment, the addition of biochar at low (160 kg/ha) N level may inhibit soil urease activity and thus decreased NH3 volatilization from rice fields, but the exact effect needs to be further investigated. In addition, according to the suggestion of Nannipieri et al. (2019), more frequent soil sampling should be conducted, after the N fertilization, to reveal the enzymatic mechanism of biochar effects on the NH3 volatilization from paddy systems.
4.3. Effect of biochar on soil N2O emission under different N fertilizer levels
The results of this experiment showed that biochar addition under different N application conditions was effective in reducing N2O emissions in the rice season (Figure 2), and this finding is consistent with previous report (Fan et al., 2020). The effect of biochar on N2O emissions is associated with the ability of biochar to alter the conversion of soil N nutrients (NO3−-N and NH4+-N) (Harter et al., 2014; Liu et al., 2019). Nguyen et al. (2017) found that biochar addition reduced the effective source of N for soil nitrifying and denitrifying bacteria, which in turn contributed to the reduction of N2O emissions. In this study, soil NH4+-N and NO3−-N contents were reduced by 0.91 and 0.23 mg/kg, respectively, after biochar addition with N at 160 kg/ha (Figures 5A,B), which corresponded with nitrification and denitrification inhibited, thus reducing N2O emissions. In addition, pH changes are also the main controlling factor for differences in soil N2O emission between the treatments with or without biochar (Wang et al., 2018), especially when the nitrification processes dominate are reduced in soils with lower pH (Yoo et al., 2016). In this study, the average pH values of the soil following biochar addition at 200–240 kg/ha were reduced (Supplementary Figure 3), which also contributed to the reduction of soil N2O emissions by biochar addition at medium and high N inputs. Our results further demonstrated that under different N application levels, the mechanism of biochar to reduce N2O emission is varied.
Functional soil microbial communities represented by nirS, nirK, and nosZ genes play key roles in regulating N2O emissions (Wang et al., 2013; Harter et al., 2016). In this study, biochar application at low (160 kg/ha) N levels decreased soil nirS and nirK gene copy numbers, while biochar application at high (240 kg/ha) N levels increased soil nosZ gene copy numbers (Table 4). According to previous study, the reduction of N2O emissions as biochar was linked to the decreased nirS and nirK genes copies but the increased nosZ gene copies or a decrease in the ratio of (nirK + nirS)/nosZ in other word (Shi et al., 2019). Duan et al. (2018) found that biochar could increase the abundance of nosZ genes in soil, thus effectively reducing N2O emissions, like the results of this study. The addition of biochar at low (160 kg/ha) N level in this study inhibited soil autotrophic nitrification and denitrification processes by reducing soil nirS and nirK gene abundance, and at medium (200 kg/ha) N level by reducing soil nirS gene abundance. In contrast, the addition of biochar at high (240 kg/ha) N levels is effective in reducing N2O emissions by increasing the abundance of soil nosZ genes, increasing N2O reductase activity, and promoting the catalytic process of N2O reduction to N2.
5. Conclusion
The combined effects of biochar addition and N reduction on rice yield and N uptake, NH3 and N2O losses in paddy soil and the underlying mechanisms were evaluated by a soil column experiment. The main conclusions were:
(1) The yield increasing effect of biochar can be realized at medium (200 kg/ha) to high (240 kg/ha) N inputs, attributing to the improving N uptake and increasing panicle number, grain number per panicle and 1,000 seed weight. In particular, the highest yield was achieved by combination of biochar and N fertilizer at medium (200 kg/ha) level.
(2) Biochar addition only effectively reduced the NH3 volatilization in rice season with low (160 kg/ha) N supply condition. Interestingly, however, at equal N input level, yield-scale NH3 volatilizations were reduced by biochar addition.
(3) Biochar addition was effective in reducing the N2O emissions from rice paddy receiving inorganic N fertilizer from low (160 kg/ha) to high (240 kg/ha) inputs. Varied changes in the functional genes, including nirS, nirK, and nosZ, explained the inhibiting effects of biochar on the N2O emission at different N inputs.
(4) We recommend the addition of biochar at medium (200 kg/hm2) N levels, which can archive the synergistic benefits of reducing inorganic N fertilizer, promoting crop yield, and decreasing N environmental losses.
Data availability statement
The raw data supporting the conclusions of this article will be made available by the authors, without undue reservation.
Author contributions
ZY and CY conducted the experiment, obtained the data, and drafted the manuscript. PJ drafted and revised the manuscript. HS designed the experiment, provided the fund support, and drafted and revised the manuscript. All authors contributed to the article and approved the submitted version.
Funding
This work was finically supported by the Science and Technology Innovation Program of Jiangsu Province, China for “Carbon Dioxide Emission Peaking and Carbon Neutrality” (BE2022307), the Jiangsu Agricultural Science and Technology Independent Innovation Fund [CX(21)3066], the National Natural Science Foundation of China (31972518), and the Qing Lan Project of Jiangsu Province of China.
Conflict of interest
The authors declare that the research was conducted in the absence of any commercial or financial relationships that could be construed as a potential conflict of interest.
Publisher’s note
All claims expressed in this article are solely those of the authors and do not necessarily represent those of their affiliated organizations, or those of the publisher, the editors and the reviewers. Any product that may be evaluated in this article, or claim that may be made by its manufacturer, is not guaranteed or endorsed by the publisher.
Supplementary material
The Supplementary material for this article can be found online at: https://www.frontiersin.org/articles/10.3389/fmicb.2023.1174805/full#supplementary-material
References
Ali, I., He, L., Ullah, S., Quan, Z., Wei, S. Q., Iqbal, A., et al. (2020). Biochar addition coupled with nitrogen fertilization impacts on soil quality, crop productivity, and nitrogen uptake under double-cropping system. Food Energy Secur. 9:e208. doi: 10.1002/fes3.208
Ali, I., Ullah, S., He, L., Zhao, Q., Iqbal, A., Wei, S. Q., et al. (2020). Combined application of biochar and nitrogen fertilizer improves rice yield, microbial activity and N-metabolism in a pot experiment. PeerJ 8:e10311. doi: 10.7717/peerj.10311
Backer, R. G. M., Saeed, W., Seguin, P., and Smith, D. L. (2017). Root traits and nitrogen fertilizer recovery efficiency of corn grown in biochar-amended soil under greenhouse conditions. Plant Soil 415, 465–477. doi: 10.1007/s11104-017-3180-6
Cai, F., Pang, G., Li, R. X., Li, R., Gu, X., Shen, Q. R., et al. (2017). Bioorganic fertilizer maintains a more stable soil microbiome than chemical fertilizer for mono-cropping. Biol. Fertil. Soils 53, 861–872. doi: 10.1007/s00374-017-1216-y
Cao, Y. S., Tian, Y. H., Yin, B., and Zhu Zhaoliang, Z. L. (2013). Assessment of ammonia volatilization from paddy fields under crop management practices aimed to increase grain yield and N efficiency. Field Crop Res. 147, 23–31. doi: 10.1016/j.fcr.2013.03.015
Chen, C. R., Phillips, I. R., Condron, L. M., Goloran, J., Xu, Z. H., and Chan, K. Y. (2013). Impacts of greenwaste biochar on ammonia volatilisation from bauxite processing residue sand. Plant Soil 367, 301–312. doi: 10.1007/s11104-012-1468-0
Chen, T. T., Xu, Y. J., Wang, J. C., Wang, Z. Q., Yang, J. C., and Zhang, J. H. (2013). Polyamines and ethylene interact in rice grains in response to soil drying during grain filling. J. Exp. Bot. 64, 2523–2538. doi: 10.1093/jxb/ert115
Chen, X. P., Cui, Z. L., Fan, M. S., Vitousek, P., Zhao, M., Ma, W. Q., et al. (2014). Producing more grain with lower environmental costs. Nature 514, 486–489. doi: 10.1038/nature13609
Cheng, Y., Cai, Z. C., Chang, S. X., Wang, J., and Zhang, J. B. (2012). Wheat straw and its biochar have contrasting effects on inorganic N retention and N2O production in a cultivated Black Chernozem. Biol. Fertil. Soils 48, 941–946. doi: 10.1007/s00374-012-0687-0
Chu, Q., Xu, S., Xue, L., Liu, Y., Feng, Y., Yu, S., et al. (2020). Bentonite hydrochar composites mitigate ammonia volatilization from paddy soil and improve nitrogen use efficiency. Sci. Total Environ. 718:137301. doi: 10.1016/j.scitotenv.2020.137301
Clough, T. J., Bertram, J. E., Ray, J. L., Condron, L. M., O'Callaghan, M., Sherlock, R. R., et al. (2010). Unweathered wood biochar impact on nitrous oxide emissions from a bovine-urine-amended pasture soil. Soil Sci. Soc. Am. J. 74, 852–860. doi: 10.2136/sssaj2009.0185
Clough, T., Condron, L., Kammann, C., and Müller, C. (2013). A review of biochar and soil nitrogen dynamics. Agronomy 3, 275–293. doi: 10.3390/agronomy3020275
Dong, D., Feng, Q., Mcgrouther, K., Yang, M., Wang, H., and Wu, W. (2015). Effects of biochar amendment on rice growth and nitrogen retention in a waterlogged paddy field. J. Soils Sediments 15, 153–162. doi: 10.1007/s11368-014-0984-3
Duan, P. P., Zhang, X., Zhang, Q. Q., Wu, Z., and Xiong, Z. Q. (2018). Field-aged biochar stimulated N2O production from greenhouse vegetable production soils by nitrification and denitrification. Sci. Total Environ. 642, 1303–1310. doi: 10.1016/j.scitotenv.2018.06.166
Fan, C. H., Duan, P. P., Zhang, X., Shen, H. J., and Chen, M. (2020). Mechanisms underlying the mitigation of both N2O and NO emissions with field-aged biochar in an Anthrosol. Geoderma 364:114178. doi: 10.1016/j.geoderma.2020.114178
Feng, Y. Y., Han, L. F., Li, D. T., Sun, M. M., Wang, X. W., Xue, L. H., et al. (2022). Presence of microplastics alone and co-existence with hydrochar unexpectedly mitigate ammonia volatilization from rice paddy soil and affect structure of soil microbiome. J. Hazard. Mater. 422:126831. doi: 10.1016/j.jhazmat.2021.126831
Feng, Y. F., He, H. Y., Xue, L. H., Liu, Y., Sun, H. J., Guo, Z., et al. (2021). The inhibiting effects of biochar-derived organic materials on rice production. J. Environ. Manag. 293:112909. doi: 10.1016/j.jenvman.2021.112909
Gaskin, J. W., Steiner, C., Harris, K., Das, K. C., and Bibens, B. (2008). Effect of low-temperature pyrolysis conditions on biochar for agricultural use. Trans. ASABE 51, 2061–2069. doi: 10.13031/2013.25409
Harter, J., Krause, H. M., Schuettler, S., Ruser, R., Fromme, M. S. T., Scholten, T., et al. (2014). Linking N2O emissions from biochar-amended soil to the structure and function of the N-cycling microbial community. ISME J. 8, 660–674. doi: 10.1038/ismej.2013.160
Harter, J., Weigold, P., El-hadidi, M., Huson, D. H., Kappler, A., and Behrens, S. (2016). Soil biochar amendment shapes the composition of N2O-reducing microbial communities. Sci. Total Environ. 562, 379–390. doi: 10.1016/j.scitotenv.2016.03.220
He, L. L., Shan, J., Zhao, X., Wang, S. Q., and Yan, X. Y. (2019). Variable responses of nitrification and denitrification in a paddy soil to long-term biochar amendment and short-term biochar addition. Chemosphere 234, 558–567. doi: 10.1016/j.chemosphere.2019.06.038
Huang, M., Fan, L., Chen, J. N., Jiang, L. G., and Zou, Y. B. (2018). Continuous applications of biochar to rice: effects on nitrogen uptake and utilization. Sci. Rep. 8, 11461–11469. doi: 10.1038/s41598-018-29877-7
Li, B., Bi, Z. C., and Xiong, Z. Q. (2017). Dynamic responses of nitrous oxide emission and nitrogen use efficiency to nitrogen and biochar amendment in an intensified vegetable field in southeastern China. Glob. Change Biol. Bioenergy. 9, 400–413. doi: 10.1111/gcbb.12356
Li, M. J., Li, R. H., Zhang, J. E., Liu, S. W., Hei, Z. W., and Qiu, S. Q. (2019). A combination of rice cultivar mixed-cropping and duck co-culture suppressed weeds and pests in paddy fields. Basic Appl. Ecol. 40, 67–77. doi: 10.1016/j.baae.2019.09.003
Liu, X. D., Chen, L. Y., Hua, Z. L., Mei, S. C., Wang, P., and Wang, S. W. (2020). Comparing ammonia volatilization between conventional and slow-release nitrogen fertilizers in paddy fields in the Taihu Lake region. Environ. Sci. Pollut. Res. 27, 8386–8394. doi: 10.1007/s11356-019-07536-2
Liu, Q., Liu, B. J., Zhang, Y. H., Hu, T. L., Lin, Z. B., Liu, G., et al. (2019). Biochar application as a tool to decrease soil nitrogen losses (NH3 volatilization, N2O emission, and N leaching) from croplands: options and mitigation strength in a global perspective. Glob. Chang. Biol. 25, 2077–2093. doi: 10.1111/gcb.14613
Ma, Y. C., Kong, X. W., Yang, B., Zhang, X. L., Yan, X. Y., Yang, J. C., et al. (2013). Net global warming potential and greenhouse gas intensity of annual rice-wheat rotations with integrated soil-crop system management. Agric. Ecosyst. Environ. 164, 209–219. doi: 10.1016/j.agee.2012.11.003
Mandal, S., Donner, E., Vasileiadis, S., Skinner, W., Smith, E., and Lombi, E. (2018). The effect of biochar feedstock, pyrolysis temperature, and application rate on the reduction of ammonia volatilisation from biochar-amended soil. Sci. Total Environ. 627, 942–950. doi: 10.1016/j.scitotenv.2018.01.312
McConnell, J. R., Edwards, R., Kok, G. L., Flanner, M. G., Zender, C. S., Saltzman, E. S., et al. (2007). 20th-century industrial black carbon emissions altered arctic climate forcing. Science 317, 1381–1384. doi: 10.1126/science.1144856
Meng, J., He, T. Y., Sanganyado, E., Lan, Y., Zhang, W. M., Han, X. R., et al. (2019). Development of the straw biochar returning concept in China. Biochar 1, 139–149. doi: 10.1007/s42773-019-00019-0
Min, J., Sun, H., Wang, Y., Pan, Y., Kronzucker, H. J., Zhao, D., et al. (2021). Mechanical side-deep fertilization mitigates ammonia volatilization and nitrogen runoff and increases profitability in rice production independent of fertilizer type and split ratio. J. Clean. Prod. 316:128370. doi: 10.1016/j.jclepro.2021.128370
Nannipieri, P., Penton, C. R., Purahong, W., Schloter, M., and Elsas, J. D. (2019). Recommendations for soil microbiome analyses. Biol Fert Soils 55, 765–766. doi: 10.1007/s00374-019-01409-z
Nguyen, T. T. N., Xu, C. Y., Tahmasbian, I., Che, R. X., Xu, Z. H., Zhou, X. H., et al. (2017). Effects of biochar on soil available inorganic nitrogen: a review and meta-analysis. Geoderma 288, 79–96. doi: 10.1016/j.geoderma.2016.11.004
Novak, J. M., Busscher, W. J., Laird, D. L., Ahmedna, M., Watts, D. W., and Niandou, M. A. S. (2009). Impact of biochar amendment on fertility of a southeastern coastal plain soil. Soil Sci. 174, 105–112. doi: 10.1097/SS.0b013e3181981d9a
Noyce, G. L., Basiliko, N., Fulthorpe, R., Sackett, T. E., and Thomas, S. C. (2015). Soil microbial responses over 2 years following biochar addition to a north temperate forest. Biol. Fertil. Soils 51, 649–659. doi: 10.1007/s00374-015-1010-7
Pyoungchung, K., Amy, J., Charles, W. E., Mark, R., Frank, V., Timothy, G. R., et al. (2011). Surface functionality and carbon structures in lignocellulosic-derived biochars produced by fast pyrolysis. J. Energy Fuels 25, 4693–4703. doi: 10.1021/ef200915s
Ray, D. K., Ramankutty, N., Mueller, N. D., West, P. C., and Foley, J. A. (2012). Recent patterns of crop yield growth and stagnation. Nat. Commun. 3:1293. doi: 10.1038/ncomms2296
Rochette, P., Angers, D. A., Chantigny, M. H., Gasser, M., MacDonald, J. D., Pelster, D. E., et al. (2013). Ammonia volatilization and nitrogen retention: how deep to incorporate urea? J. Environ. Qual. 42, 1635–1642. doi: 10.2134/jeq2013.05.0192
Saifullah, U., Dahlawi, S., Naeem, A., Rengel, Z., and Naidu, R. (2018). Biochar application for the remediation of salt-affected soils; challenges and opportunities. Sci. Total Environ. 625, 320–335. doi: 10.1016/j.scitotenv.2017.12.257
Sha, Z. P., Li, Q. Q., Lv, T. T., Misselbrook, T., and Liu, X. J. (2019). Response of ammonia volatilization to biochar addition: a meta-analysis. Sci. Total Environ. 655, 1387–1396. doi: 10.1016/j.scitotenv.2018.11.316
Shaukat, M., Samoy-Pascual, K., Maas, E. D. V. L., and Ahmad, A. (2019). Simultaneous effects of biochar and nitrogen fertilization on nitrous oxide and methane emissions from paddy rice. J. Environ. Manag. 248:109242. doi: 10.1016/j.jenvman.2019.07.013
Shi, Y. L., Liu, X. R., and Zhang, Q. W. (2019). Effect of combined biochar and organic fertilizer on nitrous oxide fluxes and the related nitrifier and denitrifier communities in a saline-alkali soil. Sci. Total Environ. 686, 199–211. doi: 10.1016/j.scitotenv.2019.05.394
Soares, J. R., Cantarella, H., and Menegale, M. L. D. C. (2012). Ammonia volatilization losses from surface-applied urea with urease and nitrification inhibitors. Soil Biol. Biochem. 52, 82–89. doi: 10.1016/j.soilbio.2012.04.019
Steiner, C., Teixeira, W. G., Lehmann, J., Nehls, T., Macêdo, J. L. V. D., and Blum, W. E. H. (2007). Long term effects of manure, charcoal and mineral fertilization on crop production and fertility on a highly weathered central Amazonian upland soil. Plant Soil 291, 275–290. doi: 10.1007/s11104-007-9193-9
Sun, H., Jeyakumar, P., Xiao, H., Li, X., Liu, J., Yu, M., et al. (2022). Biochar can increase Chinese cabbage (Brassica oleracea L.) yield, decrease nitrogen and phosphorus leaching losses in intensive vegetable soil. Phyton Int. J. Exp. Bot. 91, 197–206. doi: 10.32604/phyton.2022.016492
Sun, H., Zhang, H., Powlson, D., Min, J., and Shi, W. (2015). Rice production, nitrous oxide emission and ammonia volatilization as impacted by the nitrification inhibitor 2-chloro-6-(trichloromethyl)-pyridine. Field Crop Res. 173, 1–7. doi: 10.1016/j.fcr.2014.12.012
Sun, X., Zhong, T., Zhang, L., Zhang, K. S., and Wu, W. X. (2019). Reducing ammonia volatilization from paddy field with rice straw derived biochar. Sci. Total Environ. 660, 512–518. doi: 10.1016/j.scitotenv.2018.12.450
Tian, H. Q., Xu, R. T., Canadell, G. J., Thompson, L. R., Winiwarter, W., Suntharalingam, P., et al. (2020). A comprehensive quantification of global nitrous oxide sources and sinks. Nature 586, 248–256. doi: 10.1038/s41586-020-2780-0
Timsina, J., Singh, U., Badaruddin, M., Meisner, C., and Amin, M. R. (2001). Cultivar, nitrogen, and water effects on productivity, and nitrogen-use efficiency and balance for rice-wheat sequences of Bangladesh. Field Crop Res. 72, 143–161. doi: 10.1016/S0378-4290(01)00171-X
Ullah, S., Ali, I., Yang, M., Zhao, Q., Iqbal, A., Wu, X. Y., et al. (2023). Partial substitution of urea with biochar induced improvements in soil enzymes activity, ammonia-nitrite oxidizers, and nitrogen uptake in the double-cropping rice system. Microorganisms 11:527. doi: 10.3390/microorganisms11020527
Van Zwieten, L., Kimber, S., Morris, S., Chan, K. Y., Downie, A., Rust, J., et al. (2010). Effects of biochar from slow pyrolysis of papermill waste on agronomic performance and soil fertility. Plant Soil 327, 235–246. doi: 10.1007/s11104-009-0050-x
Wang, Y. J., Guo, J. H., Vogt, R. D., Mulder, J., Wang, J. G., and Zhang, X. S. (2018). Soil pH as the chief modifier for regional nitrous oxide emissions: new evidence and implications for global estimates. Glob. Chang. Biol. 24, e617–e626. doi: 10.1111/gcb.13966
Wang, C., Lu, H. H., Dong, D., Deng, H., Strong, P. J., Wang, H. L., et al. (2013). Insight into the effects of biochar on manure composting: evidence supporting the relationship between N2O emission and denitrifying community. Environ. Sci. Technol. 47, 7341–7349. doi: 10.1021/es305293h
Wang, J. Y., Zhang, M., Xiong, Z. Q., Liu, P. L., and Pan, G. X. (2011). Effects of biochar addition on N2O and CO2 emissions from two paddy soils. Biol. Fertil. Soils 47, 887–896. doi: 10.1007/s00374-011-0595-8
Yang, Z. B., Yu, Y., Hu, R. J., Xu, X. X., Xian, J. R., and Yang, Y. X. (2020). Effect of rice straw and swine manure biochar on N2O emission from paddy soil. Sci. Rep. 10, 10843–10811. doi: 10.1038/s41598-020-67705-z
Ye, M., Yin, C., Fan, X., Gao, Z., Chen, H., Tan, L., et al. (2021). Procyanidin inhibited N2O emissions from paddy soils by affecting nitrate reductase activity and nirS- and nirK-denitrifier populations. Biol. Fert. Soils 57, 935–947. doi: 10.1007/s00374-021-01576-y
Yi, M. L., Zhou, S. H., Zhang, L. L., and Ding, S. Y. (2021). The effects of three different microplastics on enzyme activities and microbial communities in soil. Water Environ. Res. 93, 24–32. doi: 10.1002/wer.1327
Yoo, G., Kim, Y. J., Lee, Y. O., and Ding, W. X. (2016). Investigation of greenhouse gas emissions from the soil amended with rice greenhouse gas emissions from the soil amended with rice straw biochar. KSCE J. Civ. Eng. 20, 2197–2207. doi: 10.1007/s12205-015-0449-2
Zhang, H., Ullah, F., Ahmad, R., Shah, S. U. A., Khan, A., and Adnan, M. (2020). Response of soil proteobacteria to biochar amendment in sustainable agriculture- a mini review. J. Soil Plant Environ. 1, 16–30. doi: 10.56946/jspae.v1i2.56
Keywords: biochar, N fertilizer, rice yield, NH3 volatilization, N2O emission
Citation: Yi Z, Jeyakumar P, Yin C and Sun H (2023) Effects of biochar in combination with varied N inputs on grain yield, N uptake, NH3 volatilization, and N2O emission in paddy soil. Front. Microbiol. 14:1174805. doi: 10.3389/fmicb.2023.1174805
Edited by:
Xi-En Long, Nantong University, ChinaReviewed by:
Izhar Ali, Guangxi University, ChinaPengfu Hou, Jiangsu Academy of Agricultural Sciences (JAAS), China
Copyright © 2023 Yi, Jeyakumar, Yin and Sun. This is an open-access article distributed under the terms of the Creative Commons Attribution License (CC BY). The use, distribution or reproduction in other forums is permitted, provided the original author(s) and the copyright owner(s) are credited and that the original publication in this journal is cited, in accordance with accepted academic practice. No use, distribution or reproduction is permitted which does not comply with these terms.
*Correspondence: Haijun Sun, aGpzdW5AbmpmdS5lZHUuY24=