- 1Department of Biological Sciences, University of Eldoret, Eldoret, Kenya
- 2Department of Soil and Agri-Food Engineering, Laval University, Quebec, QC, Canada
- 3Department of Biotechnology, Graphic Era (Deemed to be University), Dehradun, Uttarakhand, India
Agricultural areas exhibiting numerous abiotic stressors, such as elevated water stress, temperatures, and salinity, have grown as a result of climate change. As such, abiotic stresses are some of the most pressing issues in contemporary agricultural production. Understanding plant responses to abiotic stressors is important for global food security, climate change adaptation, and improving crop resilience for sustainable agriculture, Over the decades, explorations have been made concerning plant tolerance to these environmental stresses. Plant growth-promoting rhizobacteria (PGPR) and their phytohormones are some of the players involved in developing resistance to abiotic stress in plants. Several studies have investigated the part of phytohormones in the ability of plants to withstand and adapt to non-living environmental factors, but very few have focused on rhizobacterial hormonal signaling and crosstalk that mediate abiotic stress tolerance in plants. The main objective of this review is to evaluate the functions of PGPR phytohormones in plant abiotic stress tolerance and outline the current research on rhizobacterial hormonal communication and crosstalk that govern plant abiotic stress responses. The review also includes the gene networks and regulation under diverse abiotic stressors. The review is important for understanding plant responses to abiotic stresses using PGPR phytohormones and hormonal signaling. It is envisaged that PGPR offer a useful approach to increasing plant tolerance to various abiotic stresses. However, further studies can reveal the unclear patterns of hormonal interactions between plants and rhizobacteria that mediate abiotic stress tolerance.
1. Introduction
Abiotic stressors such as drought, salt, cold, and heat are key limiting variables in modern agriculture and account for more than 50% of plant outputs. These pressures restrict plant development by affecting several physiological processes, including photosynthesis, pigment content, and water relations (Pathak et al., 2014). For instance, cell elongation of plants can be inhibited by the interruption of water flow from the xylem to the surrounding cells (Kaamali et al., 2019). In beans, Mathobo et al. (2017) showed that genotypes had reduced grain yield, as well as chlorophyll levels, and photosynthesis and transpiration under water stress. The effects of salt stress on the physiological traits of wheat (Saddiq et al., 2020), rice (Mekawy et al., 2015; Razzaq et al., 2020), and other plant genotypes have also been established. Drought, heat, and salt stresses are especially important because of the production of reactive oxygen species (ROS) that affect plant metabolism (Hasanuzzaman et al., 2020, 2021). The interactions between plants and soil can also shape the root microbiome under abiotic stress and the general impacts of these abiotic stresses on crops (Koevoets et al., 2016; Hartman and Tringe, 2019; Chai and Schachtman, 2022; Duan et al., 2022). Cognizant of this, the major concern is to enhance crop productivity for food security by increasing crop tolerance to various abiotic stresses.
Plant growth regulators or hormones are chemical compounds that dramatically affect the development and differentiation of plant cells, tissues, and organs and serve as intercellular chemical messengers. Now, it is obvious that these hormones influence plant stress tolerance by modifying plant physiology and/or gene expression patterns. Several studies have investigated and documented the ability of plant growth regulators and phytohormones to improve plant resistance to abiotic stress (Shu et al., 2018; Santhi et al., 2021). To protect themselves, plants employ a variety of biochemical pathways, which stimulate a collection of phytohormones, growth regulators, and signaling molecules. This is the interaction or cross-talk between these events and processes which helps in plant protection (Roychoudhury and Aftab, 2021). Nonetheless, the majority of research has evaluated the phytohormones generated by plants to mediate their tolerance to abiotic stress. It is now evident that the plant rhizomicrobiome, including plant growth-promoting rhizobacteria (PGPR), also generates phytohormones that govern plant development (Kalimuthu et al., 2019). PGPR have been studied for decades (Kloepper et al., 1980), and their numerous mechanisms of action have been divided into direct mechanisms such as nitrogen fixation, phosphate solubilization, siderophore production, and phytohormones production. Indirect mechanisms such as antibiosis, induced systemic resistance, competition for nutrients, parasitism and production of diverse metabolites (Upadhyay et al., 2016) also exist and both direct and indirect mechanisms have been related to promoting plant growth. There have also been efforts over the years to determine the function of rhizobacterial phytohormones in plant tolerance to drought, water, and salt stressors, among others (discussed in Section 4). Such microbiomes are essential for building and sustaining plant resilience to various abiotic stressors.
Hormones exert their effects by activating the production of other hormones or the expression of genes. These transduction cascades regulate gene expression, which directly influences the production or function of several hormones and developmental processes (Khan et al., 2020). Further identification and investigation of these compounds and the crosstalk between them and plant-produced phytohormones that mitigate the negative effect of environmental stress in plants can shed significant light on the hormonal signaling and crosstalk that can be exploited to improve plant tolerance to abiotic stresses. Increased resistance to abiotic stresses such as heat, drought, and acidity is required more than ever as a result of climate change which is anticipated to increase the plant abiotic stressors (Teshome et al., 2020). The objective of this review is to evaluate the functions of PGPR phytohormones in plant abiotic stress tolerance and outline the current research on rhizobacterial hormonal communication and crosstalk that govern plant abiotic stress responses. This review first presents the principal forms of abiotic plant stressors (Section 2), and the functions of rhizobacteria and their phytohormones in plant abiotic stress tolerance in Sections 3 and 4. The review then recalls the rhizobacterial hormonal signaling crosstalk that mediates abiotic stress tolerance (Section 5) and highlights the new elements and ideas on the same (Section 6).
2. Major types of plant abiotic stresses
Plants are very sensitive to drought, heat, temperature, cold, salinity, heavy metals, and other abiotic stresses. It is estimated that abiotic stresses may account for more than 50% of crop yield loss depending on crop type and magnitude of exposure (Kajla et al., 2015). Here we discuss the different types of abiotic stresses and their effects on plant growth. Brief overviews of the main types of plant abiotic stresses are presented in sub-sections 2.1 to 2.5. The effects of several abiotic stresses in plants are summarized in Table 1 and a schematic illustration of the same is shown in Figure 1. Figure 1 shows some important abiotic stress including drought/heat, salinity, pH-related stress, and metal stress. Each of these abiotic stress impacts normal plant functioning and stimulates different types of responses to alleviate the stress. Sections 2.1 to 2.5 detail these different plant abiotic stresses and their effects on plant growth and physiology.
2.1. Drought and heat
Drought is the most severe form of abiotic plant stresses globally and is expected to be more severe in the coming decades due to climate change. The severity and global impact of drought stress are unparalleled relative to other environmental stresses. Drought stress can especially have devastating effects on plant growth since 80–95% of fresh plant biomass is composed of water which is necessary for a multitude of physiological and biochemical processes. Roots are usually the first plant organs to perceive and respond to drought stress. However, the effects of drought stress are apparent throughout the plant to hinder plant function and productivity (Heckathorn et al., 2013). For instance, drought-induced reduced water availability in the rhizosphere limits ionic distribution, turgor pressure, and homeostasis in plant cells, thereby inhibiting nutrient uptake by roots. Short-term consequences for plant growth include decreased carbon assimilation, stomatal closure, osmotic adjustment, inhibition of growth, hydraulic changes, signal transport, and cell-drought signaling (Ahluwalia et al., 2021) Interference with homeostasis can also affect stomatal functions and limit the uptake of CO2 for photosynthesis (Shan et al., 2018; Talbi et al., 2020). Heat stress can be short-term (often called heat-shock) or longer-term (often called heat-wave) and both stress induces strong negative impacts during various stages of plant growth (Jagadish et al., 2021). For example in wheat Akter and Islam (Akter and Islam, 2017) observed that heat stress significantly reduces seed germination and seedling growth, cell turgidity, and plant water-use efficiency, generating excessive reactive oxygen species (ROS), leading to oxidative stress. In addition, wheat encountered leaf senescence, reduction of photosynthesis, deactivation of photosynthetic enzymes, and generation of oxidative damages to the chloroplasts, leading to reduces grain number and size. According to Hussain et al. (2019), the overproduction of ROS such as superoxide (O2) and hydrogen peroxide (H2O2) may be caused by drought and heat stress that denature proteins and peroxidize lipids to impair membrane structure. Among the proteins that can be denatured by ROS under drought stress are chlorophylls (Amiri et al., 2018). Thus, drought and heat stress can hamper photosynthetic activities and crop productivity in the long run (Choukri et al., 2020; Sahithi et al., 2021). The general symptoms of drought and heat-stressed plants include leaf rolling, yellowing, scorching, plant stunting, and permanent wilting (Żurek et al., 2018). Drought stress can also affect important plant functions and interactions like the rhizosphere microbiomes and root exudation patterns as discussed in Chen et al. (2022).
2.2. Salinity
Salinity and drought stresses are closely related in terms of occurrence and consequences on plant growth. As salt levels in soils become more saline, less than optimal water becomes available to plant roots. Nevertheless, plants only become stressed when the concentration of Na+ and Cl− which consequently alters several metabolic activities in them elevates in soil beyond the thresholds for normal functioning. Due to the high salt concentration in soil, plants can suffer from hyperosmotic and hyper-ionic effects (Hasegawa et al., 2000).
Salt stress may increase the outflow of electrolytes from plant cells by dislodging Ca from membranes, hence reducing membrane permeability and causing a greater efflux of electrolytes (Hniličková et al., 2019). This has recently been confirmed by Ilyas et al. (2020) who, while researching the rhizobacteria from salty soils that generate salt tolerance in wheat, noticed greater electrolyte leakage in wheat (Triticum aestivum) at 150 mM salt stress compared to the control. Typically, these pressures cause membrane disorganization, decreased metabolic activity, altered ion, and mineral absorption rates, and reduced water intake. Salinity-induced imbalances in water and nutrient uptake eventually significant losses in plant productivity and yield. Salinity can also affect plant productivity by restricting leaf growth with marked effects on photosynthesis (Chen et al., 2019; Kwon et al., 2019). Besides, plants subjected to salinity show enhanced production of ROS which damages DNA, proteins, and membranes (Saini et al., 2018; Al Kharusi et al., 2019). Salinity also increases heavy metal toxicity as secondary stress (Zhou et al., 2019). More discussions on metal stress in plants are in Section 2.4.
2.3. Acidity
Soil acidity is a substantial worldwide limitation on agricultural output. The United States Department of Agriculture’s Natural Resources Conservation Service defines acidic soil as having a pH of 6.5 or below (Burt, 2014). Since it affects almost every step of the nutrient absorption process, soil pH has a substantial impact on plants. Al3+, Mn2+, and H+ are the three main toxicities that acidic soils expose plants to, and they all prevent plant development. For instance, aluminum toxicity prevents roots from growing, dividing, and absorbing nutrients (Bojórquez-Quintal et al., 2017). The amount of ROS, such as O2 and H2O2, which oxidize essential cellular components, may be affected in acid soils. For instance, in acidic circumstances, rice seedlings have been shown to have enhanced lipid peroxidation and H2O2 content (Zhang et al., 2015). Lipid peroxidation has also been reported in acidic conditions in Plantago (Martins et al., 2013).
2.4. Alkalinity
High levels of bicarbonates (HCO3−) and carbonates (CO32−) are present in alkaline soils as a result of weathering and dryness, which prevent sufficient water from being available to drain soluble salts from the soil (Rashid et al., 2019). Crops are equally impacted by alkalinity, partly because the ROS brought on by both stressors increases plant oxidative damage (Bui et al., 2014; Fatima et al., 2021). Alkalinity and salinity can co-occur in some soils with more detrimental effects on plant growth, nutrient uptake, and root morphology as has been shown in Lotus tenuis (Paz et al., 2012). The combined consequences of alkalinity and salinity, however, are often more severe than either alkalinity or salinity alone (Shi and Sheng, 2005).
Alkaline soils can limit the bioavailability of phosphorus (P), iron (Fe), and zinc, which causes nutritional imbalances in plants (Zn). Alkalinity may also limit photosynthesis because HCO3−decreases iron absorption, which therefore affects how chlorophyll is made (Bayarash et al., 2020). As a result of Fe shortage, Fe-induced chlorosis predominates in calcareous soils. Due to the high concentrations of HCO3-in the soil that prevents root respiration, alkalinity may also impede root development. This is most likely the cause of the stunted development seen in most alkaline-stressed plants (Singh et al., 2018).
2.5. Heavy metals
Heavy metals are often defined as metals with specific weights of more than 5 g/cm3. Lead (Pb), nickel (Ni), cadmium (Cd), aluminium (Al), manganese (Mn), and iron (Fe) among others. These heavy metals may be produced by the soil’s natural weathering processes (Wu et al., 2020; Dinter et al., 2021), the use of metal-based pesticides and fertilizers (Chen et al., 2020), or volcanic eruptions. The majority of soil physio-chemical characteristics, such as pH, soil texture, and the accumulation of nutrients, are impacted by metal toxicity, which has an impact on total plant development (Jia et al., 2020). Metal-contaminated soils impact negatively plant development, root growth, photosynthetic activity, and therefore, mineral nutrient accumulation (Yang et al., 2020). Heavy metal stress damages enzymes and other important proteins through inactivation or denaturation. Heavy metals disrupt the substitution reaction of essential metallic ions with biomolecules, which interferes with membrane integrity and diminishes photosynthetic capacity, respiration, and other cellular processes (Hossain et al., 2012). Heavy stress also triggers the generation of hydrogen peroxide, superoxide radicals, and hydroxyl radicals causing oxidative stress (Hossain et al., 2012; Ghori et al., 2019). Cadmium, for example, may produce phytotoxicity and oxidative stress in other sections of the plant, which can lower plant productivity (Tran and Popova, 2013; Sabir et al., 2020). Inadequate development and morpho-physiological harm may also result from higher Pb concentrations in plant cells (Salam et al., 2019).
3. Rhizobacterial mediation of abiotic stress tolerance in plants
Rhizobacteria are a group of microorganisms that can improve plant growth and health via nutrient solubilization, nitrogen fixation, and the synthesis of phytohormones, siderophores, and pathogen-repelling biomolecules (Ahemad and Kibret, 2014). The importance of PGPR in reducing abiotic stress has grown significantly over time. Several studies on rhizobacteria help plants adapt to abiotic stressors like acidity (Zhang et al., 2020), drought (Kang et al., 2014; Manjunatha et al., 2022), salinity (Islam et al., 2016; Zerrouk et al., 2019; Siddiqui et al., 2022), and heavy metals (Mesa-Marin et al., 2018; Kazerooni et al., 2021).
Plant stress is lessened by PGPR through direct and indirect processes (Figure 2). Aspects of the direct action of PGPR against plant abiotic stresses include increased nutrient availability, mineral acquisition, better water absorption, and development of exopolysaccharides, biofilm, and many organic solutes, including sugars, organic acids, amino acids, and polyamines (Goswami and Suresh, 2020; Kazerooni et al., 2021). As for the indirect mechanisms through which plant stress can be alleviated by PGPR they include: chemotaxis, phytohormone synthesis and level variations, antioxidant defense system activation, 1-aminocyclopropane-1-carboxylate (ACC) deaminase activity, and the control of stress-responsive genes in plants all play a role in indirect action of PGPR against plant abiotic stresses (Rosier et al., 2018). These activities control multiple biological processes in plants and together contribute to local and distant rhizobacterial-induced tolerance to abiotic stresses in plants.
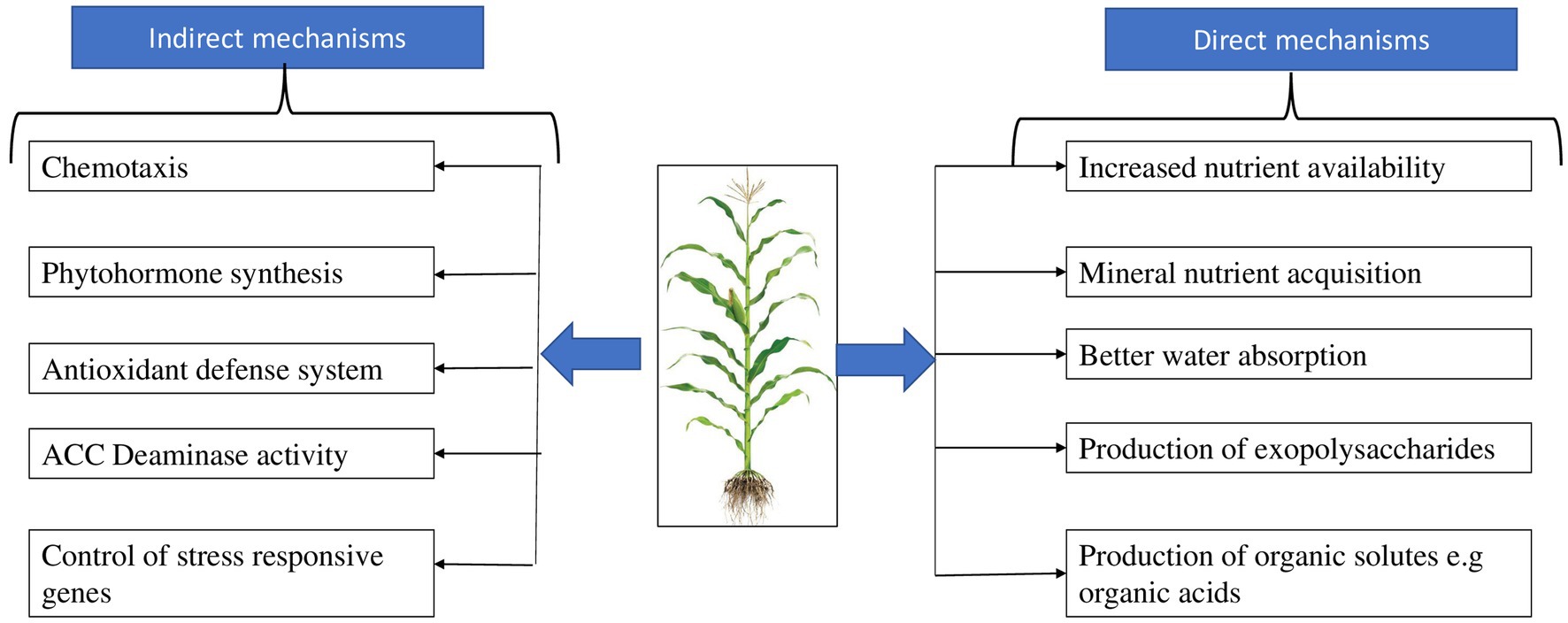
Figure 2. Direct and indirect actions of plant growth promoting rhizobacteria that promote plant abiotic stress tolerance.
Many rhizobacteria that improve or modulate plant tolerance to abiotic stresses have been shown to produce various phytohormones that support plants under these conditions (Hassan and Ahmed, 2018; Ilyas et al., 2020). Phytohormones-mediated systemic plant tolerance to abiotic stresses is achieved through various physical and chemical changes and regulation of genetic and signaling networks affecting the growth and development processes.
Rhizobacteria can also reduce heavy-metal-induced plant stress by detoxifying, biosorption, bioaccumulation in cells, or bioleaching (Huang et al., 2016; Kumar et al., 2020). They release siderophores, which are low-molecular-weight chelators that combine with metals such as Cd, Fe, Pb, and Zn to form complexes (Rajkumar et al., 2009, 2010; Ma et al., 2011). The production of siderophores by rhizosphere microbes has recently been investigated for the mitigation of stress in plants (Singh et al., 2022). This phytohormone-producing and heavy-metal-tolerant PGPR may be utilized to detoxify heavy metal-polluted soils effectively, affordably, and sustainably, even though under heavy metal stress, microorganisms may encounter a significant degree of metal toxicity (Kamnev et al., 2005). Studies that demonstrate the critical roles of rhizobacteria phytohormones in the alleviation of various abiotic stresses in plants are further discussed in Section 4.
4. Rhizobacterial and plant hormones as signaling molecules that modulate abiotic stress tolerance in plants
Phytohormones of plant and rhizobacterial origin are important bioregulators for a variety of cellular functions and signaling pathways in plants. These hormones include auxins (AUX), cytokinins (CK), abscisic acid (ABA), ethylene (ET), salicylic acid (SA), jasmonic acid (JA), gibberellic acids (GA), strigolactones (SL), and brassinosteroids (BRs) (Vocciante et al., 2022). According to reports, each phytohormone has a role in several cellular and biological processes that influence plant growth and development. Although PGPR only produces extremely little levels of phytohormones, they are nonetheless essential for plant development, including the control of abiotic stress tolerance (Table 2). We discuss the major phytohormones that contribute to plant abiotic stress tolerance in sub-Sections 4.1 to 4.7. Figure 3 summarizes the functions of plant and rhizobacterial phytohormones in plant abiotic stress tolerance (see Figure 4).
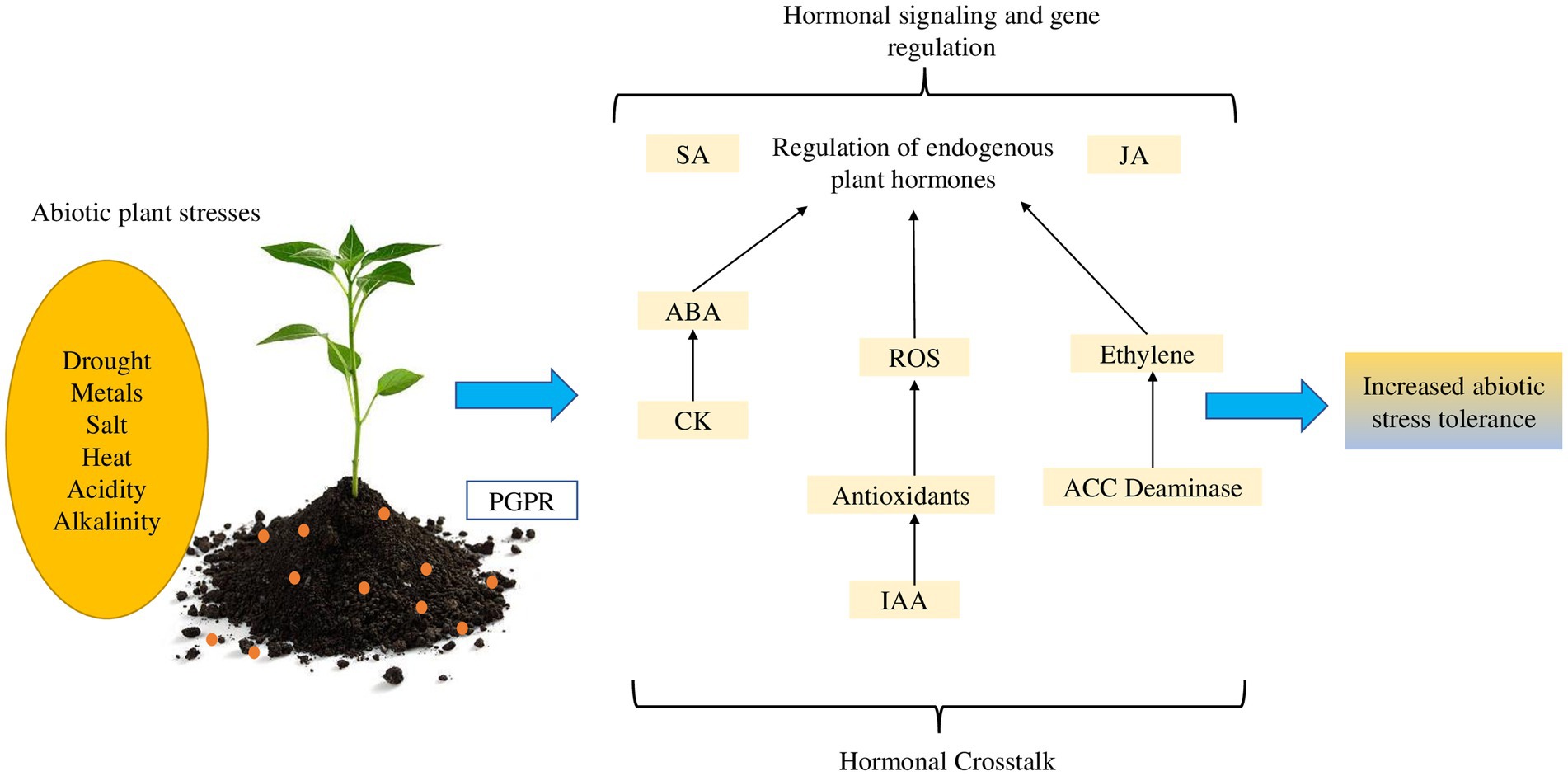
Figure 4. Rhizobacterial hormonal signaling and crosstalk with plant-endogenic phytohormones that mediate abiotic stress tolerance in plants.
4.1. Indole-3-acetic acid
One of the most prevalent phytohormones, indole-3-acetic acid (IAA), promotes root nodulation, cell proliferation, and differentiation to increase the root surface area available for the absorption of nutrients and water (Zhang et al., 2005). While IAA and other AUX are among the most researched phytohormones, we still know very little about how they could modulate plant development in response to stress. Rhizobacteria may also synthesize the hormone, albeit in small quantities, and increase plant tolerance to abiotic stressors (Table 2).
The inoculation of plants with IAA-producing rhizobacteria has also been associated with improved root volume and length and better absorption of nutrients and water under drought stress (Manjunatha et al., 2022). The production of IAA by rhizobacteria has recently been related to reduced ET levels in plants (Barnawal et al., 2017; Saleem et al., 2018). While studying the drought response of Velvet beans (Mucuna pruriens), Saleem (Saleem et al., 2018) found considerably lower ET levels in the roots and shoots of plants inoculated with IAA-producing rhizobacteria compared to un-inoculated controls. Ethylene is a stress hormone that controls plant senescence and changes or restricts plant development in response to abiotic stress (see Section 4.6). The inoculation of water-stressed purple basil with IAA-producing Azospirillum baldaniorum also improved the leaf water status (Mariotti et al., 2021). These and other results summarized in Table 2 demonstrate that IAA-producing rhizobacteria are ubiquitous and enhance plant growth under abiotic stress.
Several routes for IAA production in plants and microorganisms have been discovered through genetic analysis. The indole-3-pyruvate route, which produces IAA most often, relies on the indole-3-pyruvate decarboxylase enzyme, which is encoded by the ipdC gene. The process of turning indole-3-pyruvate into indole-3-acetaldehyde is mediated by the enzyme indole-3-pyruvate decarboxylase (Patten and Glick, 2002; Baudoin et al., 2010). An alternate mechanism comprises the decarboxylation of tryptophan into indole-3-acetamide by tryptophan monooxygenase (iaaM) and the hydrolysis of indole-3-acetamide into indole acetamide by an indole acetamide hydrolase (iaaH) (Spaepen et al., 2007). Indirect-based mechanisms such as the production of siderophore are also associated with IAA production by Bacillus subtilis which in turn improved sesame plant growth and oil content (Nithyapriya et al., 2021).
4.2. Cytokinins
Cytokinins control plant growth and development via cell division, seed germination, apical dominance, delayed leaf senescence, and chlorophyll buildup in leaves (Zwack and Rashotte, 2013, 2015). The most prevalent cytokinin in plants is zeatin, which may exist in both trans and cis forms. Trans zeatin is an active cytokinin in all plant species, but cis-zeatin is active in just a subset of plant species and is ubiquitously present in select plant species (Gajdošová et al., 2011). In plants, adenosine phosphate-isopentenyl transferases (IPTs) and CK oxidases/dehydrogenases control CK metabolism (CKXs). Whereas the former is encoded by IPT genes and is responsible for the production of CK, the latter is often involved in the degradation of CK by cleaving its side chains and causing its reversible inactivation (Mok and Mok, 2001).
Although plants generally respond to drought by reducing their CK levels, a PGPR-induced increase in CK levels may help plants to tolerate drought. Recent research has shown that Azospirillum brasilense enhances wheat development under water deficit (Zaheer et al., 2022). Similarly, CK-producing Pseudomonas fluorescens has recently been shown to improve the growth of tomatoes under water deficit (Mekureyaw et al., 2022). A strain of Sinorhizobium meliloti was engineered by Xu et al. (2012) to overproduce CK and then tested for the ability to protect alfalfa crops against drought stress (Xu et al., 2012). After a period of severe drought stress, alfalfa plants inoculated with the bacteria producing higher CK were significantly bigger than plants that were inoculated with the non-transformed strain. This experiment demonstrated how CK can boost the tolerance of alfalfa (and possibly other crops) to severe drought stress (Xu et al., 2012). However, there is limited research on the role of CK-producing rhizobacteria in mediating plant tolerance to abiotic stresses. Cognizant of this, there is a need for more research since the mechanisms that confer tolerance to one abiotic stress involving CK may or may not apply to other types of abiotic stressors.
4.3. Gibberellic acids
Gibberellic acids constitute a class of active or inactive tetracyclic diterpenoid carboxylic acids involved in a variety of plant developmental processes (Bao et al., 2020). Two soluble oxoglutarate-dependent dioxygenases catalyze the divergence of GA12 into two branches in the cytosol, one of which leads to the creation of GA4 and the other to GA1 and GA3 (Hedden and Thomas, 2012). In response to abiotic stress, GA-mediated signaling demonstrates crosstalk with other phytohormones such as ABA and IAA, therefore integrating numerous hormonal signaling pathways.
As with other hormones, GA-producing PGPR may control the endogenous GA content of host plants to compensate for the decreased synthesis of the hormone by stressed plants. For example, GA-producing bacteria promote the growth and development of many crops in salty environments (Kang et al., 2014). Under abiotic stress, gibberellic acids produced by PGPR are also responsible for leaf expansion and stem elongation. Bacillus aryabhattai generated several forms of GA in vitro and boosted the development of heat-stressed soybean plants (Park et al., 2017). Arthrobacter woluwensis, Microbacterium oxydans, Arthrobacter aurescens, Bacillus megaterium, and B. aryabhattai generate GA that promotes soybean growth in salt conditions (Kang et al., 2019). Nevertheless, the role of GA-producing bacteria in reducing the impacts of drought on host plants remains largely understood.
4.4. Abscisic acid
Abscisic acid is the most common stress hormone in plants with classical roles in many osmotic responses under salt and drought stress. It controls most plant-water relations under drought stress, for example, in maize (Zhang et al., 2012). its synthesis is stimulated by water-limiting conditions in both plants and PGPR. Under water-limiting conditions, PGPR and plant-produced ABA control stomatal closure to decrease transpiration rates, and regulate dehydration by stress signal transduction (Asghari et al., 2020). Stomatal closure is the most typical ABA response to drought stress through the limitation of water loss. ABA production increases in the roots of drought-stressed plants, from where it is transported to the shoots to trigger stomatal closure (Verma et al., 2016). This way, PGPR inoculation can increase drought tolerance by increasing ABA accumulation in plants, which leads to stomatal closure and decreased rates of leaf transpiration. In rice (Oryza sativa), inoculation with ABA-producing B. amyloliquefaciens has recently been shown to increase salinity tolerance, confirming that phytohormone production by PGPR is responsible for plant resistance to antibiotics (Shahzad et al., 2017). Hence, ABA and/or its analog produced by rhizobacteria may control phytohormonal status, boost plant growth, and regulate plant responses to drought stress. The production of ABA is controlled by 9-cis epoxycarotenoid dioxygenase; whose expression is proportional to endogenous ABA levels (Thompson et al., 2000). The effect of Azospirillum lipoferum in maize plants treated with ABA and GA inhibitor showed that Azospirrillum-inoculated plants better-alleviated water stress. Among the mechanisms involved in water stress alleviation by plants by Azospirillum is the production of stress-type hormones such as ABA together with auxins and GA (Cohen et al., 2009).
4.5. Salicylic and jasmonic acids
Salicylic acid is a phenolic molecule that enhances photosynthetic and growth characteristics in plants and protects against oxidative damage caused by abiotic stressors (Wani et al., 2017). During drought stress, it works as a signaling molecule, activating genes that encode heat-shock proteins, chaperones, and antioxidants. Moreover, jasmonic acid participates in the activation of the antioxidant system and the control of stomatal opening (Yang et al., 2019).
Similarly, to other hormones, bacterial SA synthesis may have a protective function in abiotic stress tolerance by adding to the pool of endogenously generated plant SA and its signaling pathway. Yet, there is minimal evidence that bacterial SA directly contributes to abiotic tolerance. Recently, A. baldaniorum-treated purple basil plants recorded increased SA and JA contents in the xylem sap which improved water stress tolerance in purple basil (Mariotti et al., 2021). Bacillus aryabhattai-treated soybean plants have also shown higher levels of JA than uninoculated plants and produced longer roots and shoots compared to those of control plants (Park et al., 2017). Similarly, B. amyloliquefaciens increased JA contents in Glycyrrhiza uralensis roots under drought stress (Yue et al., 2022). These reports provide evidence that PGPR may function in the modulation of plant JA and SA contents by activating the biosynthesis pathways involved.
4.6. Ethylene
Ethylene plays a crucial role in plant response to abiotic stressors in addition to governing essential plant activities such as blooming, seed germination, and senescence. For example, under drought circumstances, ET decreased stomatal conductance, which reduced intercellular CO2 concentration and lowered photosynthetic (Pierik et al., 2006). During plant ET biosynthesis, methionine is first transformed to S-adenosyl methionine and then ACC is formed. Drought-induced stimulation of root ET biosynthesis results in increased ET biosynthesis by the ACC-oxidase enzyme in plants. This is a typical response to the stimulation of root ET biosynthesis. Moreover, ethylene interacts with other hormones, such as auxins and ABA, to regulate the plant’s reaction to a lack of water. In Section 5 of this article, these interactions are examined in further detail. Yet, under stress, excessive ET synthesis might inhibit root growth and plant development. By breaking down the ET precursor ACC into alpha-ketobutyrate and ammonia, the production of the enzyme ACC-deaminase by PGPR serves to control ET biosynthesis during drought stress (Van de Poel and Van Der Straeten, 2014). This has previously been demonstrated in red pepper (Capsicum annuum) by Siddikee et al. (2011). Overall, this facilitates the survival of plants by lowering the ET levels.
5. Rhizobacterial hormonal signaling and crosstalk with plant hormones that mediate abiotic stress tolerance in plants
It is now obvious that both plants and PGPR create phytohormones that, among other crucial activities, influence plant tolerance to abiotic stressors. Yet, via influencing plant hormones and physiological responses, the PGPR may also regulate abiotic stress tolerance in plants. There are reports on rhizobacterial hormonal signaling and interplay with phytohormones that mediate abiotic stress tolerance in plants (Defez et al., 2017; Kang et al., 2019). It is widely known that crosstalk between ABA, ET, SA, and JA with AUX and GA affects gene networks controlling stress tolerance in plants (Khan et al., 2020). According to Poupin et al. (2016), Many hormonal signaling pathways in plants are carefully controlled by PGPR strains, hence influencing several physiological functions. For instance, crosstalk with other phytohormones improves plant salt stress resistance as reviewed by Egamberdieva et al. (2017).
Hormonal cross-talk facilitates the organization of several genes and their regulators in response to stress. Similar to phytohormones that are administered exogenously, microbe-produced phytohormones alter the hormone levels in plants. This may occur when microorganisms change the hormonal balance of the plant by secreting growth regulators or promoting their production inside the plant (Cabot et al., 2014). According to Barnawal et al. (2017) and Sorty et al. (2016), many PGPR enhance plant tolerance to water stress by secreting phytohormones and modifying plant endogenous hormone levels. This has recently been confirmed by Manjunatha et al. (2022) who investigated the effects of two PGPRs on pearl millet under drought stress and established higher contents of plant-produced ABA, GA, and IAA in inoculated plants than in the controls. The inoculants increased the drought resistance of the plants by modifying the hormones, physiology, and gene expression.
Modifications in endogenous IAA levels in plants owing to inoculation with IAA-secreting PGPR result in enhanced drought resistance have been previously reviewed by Dodd et al. (2010). Yet, there is no proof that the higher IAA level in these plants is a result of the PGPRs’ absorption of IAA. It is hypothesized that a greater accumulation of IAA in the shoot and root of the inoculated plant, whether stressed or not, indicates either the uptake of IAA secreted by Pseudomonas putida by the plants or the stimulation of a signaling cascade by bacterial IAA to upregulate endogenous IAA biosynthesis in Arabidopsis (Ghosh et al., 2019). Strong cross-talk between ABA and CK is one of the most important components of drought stress detection in plants since increased ABA accumulation inhibits CK production. Recent results of decreased concentrations of endogenous CK and elevated levels of ABA in water-stressed non-inoculated plants support this (Ghosh et al., 2019).
Several PGPR produce hormones that augment the plant-derived hormones to improve the abiotic stress tolerance of plants. The PGPR may increase ABA or GA production and control plant hormone levels (Dodd et al., 2010). In investigating CK-producing, PGPR-mediated drought resistance in Platycladus orientalis seedlings, we observed CK-producing, PGPR-mediated drought resistance. Previous studies by Liu et al. (2013) have confirmed that CK and ABA produced by Bacillus subtilis increased drought tolerance in P. orientalis. In a second investigation, A. brasilense inoculation of potatoes in planta affected the hormonal system by raising the levels of IAA and CK in the stems and leaves. The researchers determined that the bacteria produce this hormone for absorption by the plants (Arkhipova et al., 2020).
The modulation of plant hormonal and stress physiology under abiotic stresses has been demonstrated by several studies. For instance, P. putida inoculation under drought stress has also been shown to reduce this gap by 10.22% and the concentration of ABA was greater in inoculated plants than in untreated ones (Kang et al., 2014). The addition of P. putida to drought-and salt-stressed plants mitigated the negative effects of the stressors by lowering ABA levels. Another stress hormone, JA, was reduced in P. putida-inoculated plants relative to their control counterparts. Similar amounts of JA were detected in salt-stressed and drought-stressed plants, respectively. The interaction between P. putida and drought-and salt-stressed plants resulted in a considerable increase in JA concentration. Furthermore, abiotic stressors and bacterial contact also affected SA concentration in inoculated plants relative to other treatments, and the control, plants subjected to salt stress followed by drought stress had a considerably higher SA concentration. P. putida improved soybean development under drought or salt stress by raising or reducing the levels of endogenous SA (Kang et al., 2014). It has been postulated that the exogenous application of beneficial bacterial cultures may offset the inhibitory effects of various abiotic stressors by modulating SA biosynthesis. The stress resistance given by PGPRs may be due to the modulation of phytohormones such as ABA, JA, and SA, which correlate to plant defense and growth (Kang et al., 2014; Carvalhais et al., 2015, 2017). In Kang’s (Kang et al., 2019) study, in heat-stressed soybean plants inoculated with B. tequilensis, the endogenous levels of JA and SA were dramatically elevated, but the endogenous level of ABA was significantly downregulated. In contrast, the stress-responsive hormone ABA content of soybean plants was considerably downregulated by PGPR-inoculation in this research, suggesting that PGPR lowered the amount of stress in the plants. These results suggest a potential cross-talk between auxin and SA, which modulates plant tolerance responses.
6. Emerging areas and perspectives
Overall, it is well-established that PGPR-produced phytohormones alongside those of plant origin help plants to withstand abiotic stresses by improving the upregulation of antioxidant systems, enhancing photosynthetic potential, regulating the stomatal conductance, and stimulating root development for increased uptake of water and mineral nutrients (Khan et al., 2020). However, it is still not fully understood how the PGPR-produced phytohormones coordinate cellular activities and regulate the cellular process in abiotic stress responses. Many current studies are underway that will further define the role of rhizobacterial hormones in PGPR-primed abiotic stress tolerance.
Fluctuations in temperature and precipitation are likely to increase in a changing global climate (Intergovernmental Panel on Climate Change, 2013). Since most abiotic stresses occur together, specific plant responses mediated by rhizobacterial hormonal signaling for plants under combined stresses cannot be predicted from the results of studies based on single abiotic stresses. Hence, it is increasingly becoming important to assess rhizobacterial hormonal signaling for plant tolerance to multiple abiotic stresses which is normally the scenario with climate change. Most studies using rhizobacterial isolates for plant abiotic stress tolerance have dwelt on isolates from abiotically-stressed environments for plant inoculation and testing. This is especially very promising since such PGPR isolated can have a stronger potential to mitigate similar stresses in plants due to their natural adaptive mechanisms to specific stress conditions. Therefore, they have likely developed mechanisms to tolerate and mitigate the adverse effects of abiotic stresses. By inoculating plants with such PGPR, the plants can benefit from the inherent stress tolerance mechanisms possessed by these strains. Plant inoculation with such PGPR inoculation may improve crop growth and yield under a variety of abiotic situations. These indigenous PGPR strains are well suited to local conditions, but they must be studied and documented before they can be used as crop inoculants.
Moreover, soil-plant-microbe interactions should be studied to determine the effectiveness of these microorganisms with varying physicochemical properties under various abiotic stresses. Specialist research is also required to explore the mechanisms that lead to the synthesis of different metabolites, as well as their synergistic and antagonistic interactions with host plants. Genetic studies can also discover the best time to locate receptors for the synthesis of certain genes in host plants after microbial inoculation, as well as the genetic modifications of related bacteria. Plant-microbe interactions should be optimized using molecular genetics, bioinformatics, and modeling tools to maximize agricultural productivity and soil and environmental health. Such new frontiers have been evidenced by some studies (Prabhavathi et al., 2002; Abd El-Daim et al., 2018; Jha and Subramanian, 2018). There are, however, some challenges regarding designing experiments that accurately mimic the complex and dynamic conditions of natural ecosystems. Abiotic stresses, such as drought, salinity, or temperature extremes, vary in intensity, duration, and frequency in different environments. Therefore, replicating these conditions in controlled settings while maintaining experimental rigor can be difficult. Abiotic stresses can also vary spatially and temporally within a given ecosystem. Thus, accounting for this variability in experimental designs and interpreting results across different stress conditions can be complex. Similarly, abiotic stress responses in plants and microbes often involve dynamic physiological and molecular changes over time. Understanding the temporal dynamics of soil-plant-microbe interactions under abiotic stresses requires long-term monitoring, which can be resource-intensive. Maintaining experimental conditions over extended periods and capturing the full range of temporal responses is challenging.
Advances in technology and genetics, such as the use of transcriptomics to identify the transcript components involved in the early stages of rhizobacteria-induced systemic tolerance to drought stress, may provide light on the molecular mechanism behind the signal transmission. Many studies have shown that phytohormone-secreting PGPR has a good influence on the general health, physiological condition, and endogenous levels of a few hormones in plants under drought stress. Little is known, however, about how a particular strain of PGPR might assist plants to cope with drought stress by altering the accumulation and localization patterns of the four primary endogenous hormones. It is unclear if these changes are the consequence of bacterial phytohormone absorption by plants, a change in the plant’s endogenous hormone metabolism driven by bacteria, or a combination of the two. Moreover, there is a lack of thorough knowledge of how PGPR controls endogenous plant hormones.
7. Conclusion
Rhizobacterial phytohormones are key macromolecules that influence several physiological processes in plants. Despite advancements in understanding the physiological and molecular processes of plant abiotic stress tolerance, the particular mechanisms of rhizobacteria-mediated plant tolerance to abiotic stresses remain unexplained. Nevertheless, an increased understanding of rhizobacterial hormones and hormonal signaling in improving crop growth and yield under multiple abiotic stresses might provide an alternative strategy for developing environmentally friendly and sustainable agricultural practices, especially in the wake of climate change which brings about multiple stresses to plants. Hormonal crosstalk is pervasive and occurs in many forms. To deconstruct the multilayered responses under abiotic stress conditions, a considerable understanding of plant responses to combined stress exposure is also required. The present review has deconstructed signaling and crosstalk of rhizobacterial and plant hormones that mediate abiotic stress tolerance in plants. This discovery opens up new opportunities for the investigation of beneficial interactions in plants. A deeper understanding of root-microorganism consortiums might also lead to new research avenues for vital agricultural areas whose sustainability depends on these interactions.
Author contributions
All authors listed have made a substantial, direct, and intellectual contribution to the work and approved it for publication.
Funding
The publication of this manuscript was supported with funds from Laval University, Quebec-Canada.
Publisher’s note
All claims expressed in this article are solely those of the authors and do not necessarily represent those of their affiliated organizations, or those of the publisher, the editors and the reviewers. Any product that may be evaluated in this article, or claim that may be made by its manufacturer, is not guaranteed or endorsed by the publisher.
Conflict of interest
The authors declare that the research was conducted in the absence of any commercial or financial relationships that could be construed as a potential conflict of interest.
References
Abd El-Daim, I., Bejai, S., Fridborg, I., and Meijer, J. (2018). Identifying potential molecular factors involved in Bacillus amyloliquefaciens 5113 mediated abiotic stress tolerance in wheat. Plant Biol. 20, 271–279. doi: 10.1111/plb.12680
Ahemad, M., and Kibret, M. (2014). Mechanisms and applications of plant growth promoting rhizobacteria: current perspective. J. King Saud Univ. Sci. 26, 1–20. doi: 10.1016/j.jksus.2013.05.001
Ahluwalia, O., Singh, P. C., and Bhatia, R. (2021). A review on drought stress in plants: implications, mitigation and the role of plant growth promoting rhizobacteria. Resour. Environ. Sustain. 5:100032. doi: 10.1016/j.resenv.2021.100032
Ahmed, M., Kheir, A. M., Mehmood, M. Z., Ahmad, S., and Hasanuzzaman, M. (2022). Changes in germination and seedling traits of sesame under simulated drought. Phyton 91, 713–726. doi: 10.32604/phyton.2022.018552
Akter, N., and Islam, M. R. (2017). Heat stress effects and management in wheat. A review. Agron. Sustain. Dev. 37, 1–17. doi: 10.1007/s13593-017-0443-9
Al Kharusi, L., Al Yahyai, R., and Yaish, M. W. (2019). Antioxidant response to salinity in salt-tolerant and salt-susceptible cultivars of date palm. Agriculture 9:8. doi: 10.3390/agriculture9010008
Amiri, H., Dousty, B., and Hosseinzedeh, S. R. (2018). Water stress-induced changes of morphological, physiological and essential oil compounds in Thymus eriocalyx from Iran. J. Essent. Oil Bear. Plants 21, 1210–1223. doi: 10.1080/0972060X.2018.1538817
Arkhipova, T., Evseeva, N. V., Tkachenko, O. V., Burygin, G. L., Vysotskaya, L. B., Akhtyamova, Z. A., et al. (2020). Rhizobacteria inoculation effects on phytohormone status of potato microclones cultivated in vitro under osmotic stress. Biomol. Ther. 10:1231. doi: 10.3390/biom10091231
Asghari, B., Khademian, R., and Sedaghati, B. (2020). Plant growth promoting rhizobacteria (PGPR) confer drought resistance and stimulate biosynthesis of secondary metabolites in pennyroyal (Mentha pulegium L.) under water shortage condition. Sci. Hortic. 263:109132. doi: 10.1016/j.scienta.2019.109132
Atieno, J., Li, Y., Langridge, P., Dowling, K., Brien, C., Berger, B., et al. (2017). Exploring genetic variation for salinity tolerance in chickpea using image-based phenotyping. Sci. Rep. 7, 1–11. doi: 10.1038/s41598-017-01211-7
Bao, S., Hua, C., Shen, L., and Yu, H. (2020). New insights into gibberellin signaling in regulating flowering in Arabidopsis. J. Integr. Plant Biol. 62, 118–131. doi: 10.1111/jipb.12892
Barnawal, D., Bharti, N., Pandey, S. S., Pandey, A., Chanotiya, C. S., and Kalra, A. (2017). Plant growth-promoting rhizobacteria enhance wheat salt and drought stress tolerance by altering endogenous phytohormone levels and TaCTR1/TaDREB2 expression. Physiol. Plant. 161, 502–514. doi: 10.1111/ppl.12614
Baudoin, E., Lerner, A., Mirza, M. S., El Zemrany, H., Prigent-Combaret, C., Jurkevich, E., et al. (2010). Effects of Azospirillum brasilense with genetically modified auxin biosynthesis gene ipdC upon the diversity of the indigenous microbiota of the wheat rhizosphere. Res. Microbiol. 161, 219–226. doi: 10.1016/j.resmic.2010.01.005
Bayarash, M., Raghami, M., Roosta, H., and Karimi, H. (2020). Evaluation of growth, physiological and photosynthetic characteristics of two spinach cultivars (hybrid and Iranian) under alkaline water stress. J. Veg. Sci. 4, 25–39. doi: 10.22034/iuvs.2020.127057.1096
Belimov, A. A., Dodd, I. C., Safronova, V. I., Shaposhnikov, A. I., Azarova, T. S., Makarova, N. M., et al. (2015). Rhizobacteria that produce auxins and contain 1-amino-cyclopropane-1-carboxylic acid deaminase decrease amino acid concentrations in the rhizosphere and improve growth and yield of well-watered and water-limited potato (Solanum tuberosum). Ann. Appl. Biol. 167, 11–25. doi: 10.1111/aab.12203
Bojórquez-Quintal, E., Escalante-Magaña, C., Echevarría-Machado, I., and Martínez-Estévez, M. (2017). Aluminum, a friend or foe of higher plants in acid soils. Front. Plant Sci. 8:1767. doi: 10.3389/fpls.2017.01767
Bui, E. N., Thornhill, A., and Miller, J. T. (2014). Salt-and alkaline-tolerance are linked in Acacia. Biol. Lett. 10:20140278. doi: 10.1098/rsbl.2014.0278
Burt, R. Soil survey staff: soil survey field and laboratory methods manual Report No. 51. Washington, DC: US Department of Agriculture; (2014) 227–234. Available at: https://www.nrcs.usda.gov/sites/default/files/2023-01/SSIR51.pdf
Cabot, C., Sibole, J. V., Barceló, J., and Poschenrieder, C. (2014). Lessons from crop plants struggling with salinity. Plant Sci. 226, 2–13. doi: 10.1016/j.plantsci.2014.04.013
Carvalhais, L. C., Dennis, P. G., Badri, D. V., Kidd, B. N., Vivanco, J. M., and Schenk, P. M. (2015). Linking jasmonic acid signaling, root exudates, and rhizosphere microbiomes. Mol. Plant-Microbe Interact. 28, 1049–1058. doi: 10.1094/MPMI-01-15-0016-R
Carvalhais, L. C., Schenk, P. M., and Dennis, P. G. (2017). Jasmonic acid signalling and the plant holobiont. Curr. Opin. Microbiol. 37, 42–47. doi: 10.1016/j.mib.2017.03.009
Chai, Y. N., and Schachtman, D. P. (2022). Root exudates impact plant performance under abiotic stress. Trends Plant Sci. 27, 80–91. doi: 10.1016/j.tplants.2021.08.003
Chakma, P., Hossain, M. M., and Rabbani, M. G. (2019). Effects of salinity stress on seed germination and seedling growth of tomato: salinity stress on seed germination and seedling growth. J. Bangladesh Agric. Univ. 17, 490–499. doi: 10.3329/jbau.v17i4.44617
Chandra, D., Srivastava, R., and Sharma, A. K. (2018). Influence of IAA and ACC Deaminase producing fluorescent pseudomonads in alleviating drought stress in wheat (Triticum aestivum). Agric. Res. 7, 290–299. doi: 10.1007/s40003-018-0305-y
Chen, X. X., Liu, Y. M., Zhao, Q. Y., Cao, W. Q., Chen, X. P., and Zou, C. Q. (2020). Health risk assessment associated with heavy metal accumulation in wheat after long-term phosphorus fertilizer application. Environ. Pollut. 262:114348. doi: 10.1016/j.envpol.2020.114348
Chen, S., Wang, Z., Guo, X., Rasool, G., Zhang, J., Xie, Y., et al. (2019). Effects of vertically heterogeneous soil salinity on tomato photosynthesis and related physiological parameters. Sci. Hortic. 249, 120–130. doi: 10.1016/j.scienta.2019.01.049
Chen, Y., Yao, Z., Sun, Y., Wang, E., Tian, C., Sun, Y., et al. (2022). Current studies of the effects of drought stress on root exudates and rhizosphere microbiomes of crop plant species. Int. J. Mol. Sci. 23:2374. doi: 10.3390/ijms23042374
Choukri, H., Hejjaoui, K., El-Baouchi, A., El Haddad, N., Smouni, A., Maalouf, F., et al. (2020). Heat and drought stress impact on phenology, grain yield, and nutritional quality of lentil (Lens culinaris Medikus). Front. Nutr. 7:596307. doi: 10.3389/fnut.2020.596307
Cohen, A. C., Travaglia, C. N., Bottini, R., and Piccoli, P. N. (2009). Participation of abscisic acid and gibberellins produced by endophytic Azospirillum in the alleviation of drought effects in maize. Botany 87, 455–462. doi: 10.1139/B09-023
Curá, J. A., Franz, D. R., Filosofía, J. E., Balestrasse, K. B., and Burgueño, L. E. (2017). Inoculation with Azospirillum sp. and Herbaspirillum sp. bacteria increases the tolerance of maize to drought stress. Microorganisms 5:41. doi: 10.3390/microorganisms5030041
Defez, R., Andreozzi, A., Dickinson, M., Charlton, A., Tadini, L., Pesaresi, P., et al. (2017). Improved drought stress response in alfalfa plants nodulated by an IAA over-producing Rhizobium strain. Front. Microbiol. 8:02466. doi: 10.3389/fmicb.2017.02466
Dehnavi, R. A., Zahedi, M., Ludwiczak, A., Cardenas, P. S., and Piernik, A. (2020). Effect of salinity on seed germination and seedling development of sorghum (Sorghum bicolor (L.) Moench) genotypes. Agronomy 10:859. doi: 10.3390/agronomy10060859
Dinter, T. C., Gerzabek, M. H., Puschenreiter, M., Strobel, B. W., Couenberg, P. M., and Zehetner, F. (2021). Heavy metal contents, mobility and origin in agricultural topsoils of the Galápagos Islands. Chemosphere 272:129821. doi: 10.1016/j.chemosphere.2021.129821
Dodd, I., Zinovkina, N., Safronova, V., and Belimov, A. (2010). Rhizobacterial mediation of plant hormone status. Ann. Appl. Biol. 157, 361–379. doi: 10.1111/j.1744-7348.2010.00439.x
Duan, D., Jiang, F., Lin, W., Tian, Z., Wu, N., Feng, X., et al. (2022). Effects of drought on the growth of Lespedeza davurica through the alteration of soil microbial communities and nutrient availability. J. Fungi 8:384. doi: 10.3390/jof8040384
Egamberdieva, D., Wirth, S. J., Alqarawi, A. A., Abd Allah, E. F., and Hashem, A. (2017). Phytohormones and beneficial microbes: essential components for plants to balance stress and fitness. Front. Microbiol. 8:2104. doi: 10.3389/fmicb.2017.02104
Fatima, A., Hussain, S., Hussain, S., Ali, B., Ashraf, U., Zulfiqar, U., et al. (2021). Differential morphophysiological, biochemical, and molecular responses of maize hybrids to salinity and alkalinity stresses. Agronomy 11:1150. doi: 10.3390/agronomy11061150
Gajdošová, S., Spíchal, L., Kamínek, M., Hoyerová, K., Novák, O., Dobrev, P. I., et al. (2011). Distribution, biological activities, metabolism, and the conceivable function of cis-zeatin-type cytokinins in plants. J. Exp. Bot. 62, 2827–2840. doi: 10.1093/jxb/erq457
Ghori, N. H., Ghori, T., Hayat, M., Imadi, S., Gul, A., Altay, V., et al. (2019). Heavy metal stress and responses in plants. Int. J. Environ. Sci. Technol. 16, 1807–1828. doi: 10.1007/s13762-019-02215-8
Ghosh, D., Gupta, A., and Mohapatra, S. (2019). Dynamics of endogenous hormone regulation in plants by phytohormone secreting rhizobacteria under water-stress. Symbiosis 77, 265–278. doi: 10.1007/s13199-018-00589-w
Goswami, M., and Suresh, D. (2020). Plant growth-promoting rhizobacteria—alleviators of abiotic stresses in soil: a review. Pedosphere 30, 40–61. doi: 10.1016/S1002-0160(19)60839-8
Gupta, S., and Pandey, S. (2019). ACC Deaminase producing Bacteria with multifarious plant growth promoting traits alleviates salinity stress in French bean (Phaseolus vulgaris) plants. Front. Microbiol. 10:01506. doi: 10.3389/fmicb.2019.01506
Haque, M., Biswas, M., Mosharaf, M. K., Islam, M., Nahar, K., and Shozib, H. B. (2022). Halotolerant biofilm-producing rhizobacteria mitigate seawater-induced salt stress and promote growth of tomato. Sci. Rep. 12, 1–22. doi: 10.1038/s41598-022-09519-9
Hartman, K., and Tringe, S. G. (2019). Interactions between plants and soil shaping the root microbiome under abiotic stress. Biochem. J. 476, 2705–2724. doi: 10.1042/BCJ20180615
Hasanuzzaman, M., Bhuyan, M. B., Zulfiqar, F., Raza, A., Mohsin, S. M., Mahmud, J. A., et al. (2020). Reactive oxygen species and antioxidant defense in plants under abiotic stress: revisiting the crucial role of a universal defense regulator. Antioxidants 9:681. doi: 10.3390/antiox9080681
Hasanuzzaman, M., Raihan, M. R., Masud, A. A., Rahman, K., Nowroz, F., Rahman, M., et al. (2021). Regulation of reactive oxygen species and antioxidant defense in plants under salinity. Int. J. Mol. Sci. 22:9326. doi: 10.3390/ijms22179326
Hasegawa, P. M., Bressan, R. A., Zhu, J. K., and Bohnert, H. J. (2000). Plant cellular and molecular responses to high salinity. Annu. Rev. Plant Biol. 51, 463–499. doi: 10.1146/annurev.arplant.51.1.463
Hassan, A., and Ahmed, S. A. A. H. (2018). Role of plant growth-promoting rhizobacteria in reducing the harmful effect of water stress on some physiological traits of popcorn1. Res. Crops 19, 398–304. doi: 10.31830/2348-7542.2018.0001.6
Heckathorn, S. A., Giri, A., Mishra, S., and Bista, D. (2013). “Heat stress and roots” in Climate change and plant abiotic stress tolerance. eds. N. Tuteja and S. S. Gill (Wiley), 109–136.
Hedden, P., and Thomas, S. G. (2012). Gibberellin biosynthesis and its regulation. Biochem. J. 444, 11–25. doi: 10.1042/BJ20120245
Hniličková, H., Hnilička, F., Orsák, M., and Hejnák, V. (2019). Effect of salt stress on growth, electrolyte leakage, Na+ and K+ content in selected plant species. Plant Soil Environ. 65, 90–96. doi: 10.17221/620/2018-PSE
Hossain, M. A., Piyatida, P., da Silva, J. A. T., and Fujita, M. (2012). Molecular mechanism of heavy metal toxicity and tolerance in plants: central role of glutathione in detoxification of reactive oxygen species and methylglyoxal and in heavy metal chelation. J. Bot. 2012:872875. doi: 10.1155/2012/872875
Huang, J., Liu, Z., Li, S., Xu, B., Gong, Y., Yang, Y., et al. (2016). Isolation and engineering of plant growth promoting rhizobacteria Pseudomonas aeruginosa for enhanced cadmium bioremediation. J. Gen. Appl. Microbiol. 62, 258–265. doi: 10.2323/jgam.2016.04.007
Hussain, S., Rao, M. J., Anjum, M. A., Ejaz, S., Zakir, I., Ali, M. A., et al. (2019). “Oxidative stress and antioxidant defense in plants under drought conditions” in Plant abiotic stress tolerance. eds. M. Hasanuzzaman, K. Hakeem, K. Nahar, and H. Alharby (Cham: Springer), 207–219.
Ilyas, N., Mazhar, R., Yasmin, H., Khan, W., Iqbal, S., Enshasy, H. E., et al. (2020). Rhizobacteria isolated from saline soil induce systemic tolerance in wheat (Triticum aestivum L.) against salinity stress. Agronomy 10:989. doi: 10.3390/agronomy10070989
Intergovernmental Panel on Climate Change. The physical science basis. Contribution of working group I to the fifth assessment report of the intergovernmental panel on climate change. Report No. 1535. (2013). Available at: https://www.klimamanifest-von-heiligenroth.de/wp/wp-content/uploads/2016/06/IPCC_2013_WG1AR5_S916_S917_Extremwetter_Zitate_mitTitelCover.pdf
Islam, F., Yasmeen, T., Arif, M. S., Ali, S., Ali, B., Hameed, S., et al. (2016). Plant growth promoting bacteria confer salt tolerance in Vigna radiata by up-regulating antioxidant defense and biological soil fertility. Plant Growth Regul. 80, 23–36. doi: 10.1007/s10725-015-0142-y
Jagadish, S. K., Way, D. A., and Sharkey, T. D. (2021). Plant heat stress: concepts directing future research. Plant Cell Environ. 44, 1992–2005. doi: 10.1111/pce.14050
Jayawardhane, J., Goyali, J. C., Zafari, S., and Igamberdiev, A. U. (2022). The response of cowpea (Vigna unguiculata) plants to three abiotic stresses applied with increasing intensity: hypoxia, salinity, and water deficit. Metabolites 12:38. doi: 10.3390/metabo12010038
Jha, Y., and Subramanian, R. (2018). “From interaction to gene induction: an eco-friendly mechanism of PGPR-mediated stress management in the plant” in Plant microbiome: stress response (Microorganisms for Sustainability). eds. D. Egamberdieva and P. Ahmad (Singapore: Springer), 217–232.
Ji, J., Yuan, D., Jin, C., Wang, G., Li, X., and Guan, C. (2020). Enhancement of growth and salt tolerance of rice seedlings (Oryza sativa L.) by regulating ethylene production with a novel halotolerant PGPR strain Glutamicibacter sp. YD01 containing ACC deaminase activity. Acta Physiol. Plant. 42:42. doi: 10.1007/s11738-020-3034-3
Jia, X., Fu, T., Hu, B., Shi, Z., Zhou, L., and Zhu, Y. (2020). Identification of the potential risk areas for soil heavy metal pollution based on the source-sink theory. J. Hazard. Mater. 393:122424. doi: 10.1016/j.jhazmat.2020.122424
Jorge, G. L., Kisiala, A., Morrison, E., Aoki, M., Nogueira, A. P. O., and Emery, R. J. N. (2019). Endosymbiotic Methylobacterium oryzae mitigates the impact of limited water availability in lentil (Lens culinaris Medik.) by increasing plant cytokinin levels. Environ. Exp. Bot. 162, 525–540. doi: 10.1016/j.envexpbot.2019.03.028
Kaamali, M., Shour, M., Neamati, S., Lakzian, A., and Khazaei, H. (2019). Effect of drought stress on physio-morphological characteristics and proline content of three varieties of petunia. J. Hortic. Sci. 32, Fa519–Fa530. doi: 10.22067/jhorts4.v32i2.58195
Kajla, M., Yadav, V., Chhokar, R., and Sharma, R. (2015). Management practices to mitigate the impact of high temperature on wheat. J. Wheat Res. 7, 1–12.
Kalimuthu, R., Suresh, P., Varatharaju, G., Balasubramanian, N., Rajasekaran, K. M., and Shanmugaiah, V. (2019). Isolation and characterization of indole acetic acid [IAA] producing tomato Rhizobacterium Pseudomonas sp VSMKU4050 and its potential for plant growth promotion. Int. J. Curr. Microbiol. 8, 443–455. doi: 10.20546/ijcmas.2019.806.050
Kamnev, A. A., Tugarova, A. V., Antonyuk, L. P., Tarantilis, P. A., Polissiou, M. G., and Gardiner, P. H. (2005). Effects of heavy metals on plant-associated rhizobacteria: comparison of endophytic and non-endophytic strains of Azospirillum brasilense. J. Trace Elem. Med. Biol. 19, 91–95. doi: 10.1016/j.jtemb.2005.03.002
Kang, S. M., Khan, A. L., Waqas, M., Asaf, S., Lee, K. E., Park, Y. G., et al. (2019). Integrated phytohormone production by the plant growth-promoting rhizobacterium Bacillus tequilensis SSB07 induced thermotolerance in soybean. J. Plant Interact. 14, 416–423. doi: 10.1080/17429145.2019.1640294
Kang, S. M., Radhakrishnan, R., Khan, A. L., Kim, M. J., Park, J. M., Kim, B. R., et al. (2014). Gibberellin secreting rhizobacterium, Pseudomonas putida H-2-3 modulates the hormonal and stress physiology of soybean to improve the plant growth under saline and drought conditions. Plant Physiol. Biochem. 84, 115–124. doi: 10.1016/j.plaphy.2014.09.001
Kazerooni, E. A., Maharachchikumbura, S. S., Adhikari, A., Al-Sadi, A. M., Kang, S. M., Kim, L. R., et al. (2021). Rhizospheric Bacillus amyloliquefaciens protects Capsicum annuum cv. Geumsugangsan from multiple abiotic stresses via multifarious plant growth-promoting attributes. Front. Plant Sci. 12:669693. doi: 10.3389/fpls.2021.669693
Khan, M. A., Asaf, S., Khan, A. L., Ullah, I., Ali, S., Kang, S. M., et al. (2019). Alleviation of salt stress response in soybean plants with the endophytic bacterial isolate Curtobacterium sp. SAK1. Ann. Microbiol. 69, 797–808. doi: 10.1007/s13213-019-01470-x
Khan, N., Bano, A., Ali, S., and Babar, M. (2020). Crosstalk amongst phytohormones from planta and PGPR under biotic and abiotic stresses. Plant Growth Regul. 90, 189–203. doi: 10.1007/s10725-020-00571-x
Khumairah, F., Nurbaity, A., Setiawati, M., Fitriatin, B., and Simarmata, T. (2019). The ability of Phosphorhizobacteria isolates to produce organic acid and promote phosphatase activity to increase the growth of maize (Zea mays L.) in selected medium. IOP Conf. Ser.: Earth Environ. Sci. :012023. doi: 10.1088/1755-1315/334/1/012023
Kloepper, J. W., Leong, J., Teintze, M., and Schroth, M. N. (1980). Enhanced plant growth by siderophores produced by plant growth-promoting rhizobacteria. Nature 286, 885–886. doi: 10.1038/286885a0
Koevoets, I. T., Venema, J. H., Elzenga, J. T. M., and Testerink, C. (2016). Roots withstanding their environment: exploiting root system architecture responses to abiotic stress to improve crop tolerance. Front. Plant Sci. 7:1335. doi: 10.3389/fpls.2016.01335
Kumar, V., Singh, G., Chauhan, R. S., and Sinam, G. (2020). “Role of plant growth-promoting rhizobacteria in mitigation of heavy metals toxicity to Oryza sativa L” in Emerging technologies in environmental bioremediation. eds. M. P. Shah, S. Rodriguez-Couto, and S. Sevinç Şengör (Amsterdam: Elsevier), 373–390.
Kwon, O. K., Mekapogu, M., and Kim, K. S. (2019). Effect of salinity stress on photosynthesis and related physiological responses in carnation (Dianthus caryophyllus). Hortic. Environ. Biotechnol. 60, 831–839. doi: 10.1007/s13580-019-00189-7
Li, X., Lv, X., Wang, X., Wang, L., Zhang, M., and Ren, M. (2018). Effects of abiotic stress on anthocyanin accumulation and grain weight in purple wheat. Crop Pasture Sci. 69, 1208–1214. doi: 10.1071/CP18341
Liu, F., Xing, S., Ma, H., Du, Z., and Ma, B. (2013). Cytokinin-producing, plant growth-promoting rhizobacteria that confer resistance to drought stress in Platycladus orientalis container seedlings. Appl. Microbiol. Biotechnol. 97, 9155–9164. doi: 10.1007/s00253-013-5193-2
Ma, Y., Prasad, M., Rajkumar, M., and Freitas, H. (2011). Plant growth promoting rhizobacteria and endophytes accelerate phytoremediation of metalliferous soils. Biotechnol. Adv. 29, 248–258. doi: 10.1016/j.biotechadv.2010.12.001
Manjunatha, B. S., Nivetha, N., Krishna, G. K., Elangovan, A., Pushkar, S., Chandrashekar, N., et al. (2022). Plant growth-promoting rhizobacteria Shewanella putrefaciens and Cronobacter dublinensis enhance drought tolerance of pearl millet by modulating hormones and stress-responsive genes. Physiol. Plant. 174:e13676. doi: 10.1111/ppl.13676
Mariotti, L., Scartazza, A., Curadi, M., Picciarelli, P., and Toffanin, A. (2021). Azospirillum baldaniorum sp245 induces physiological responses to alleviate the adverse effects of drought stress in purple basil. Plan. Theory 10:1141. doi: 10.3390/plants10061141
Martins, N., Gonçalves, S., and Romano, A. (2013). Metabolism and aluminum accumulation in Plantago almogravensis and P. algarbiensis in response to low pH and aluminum stress. Biol. Plant. 57, 325–331. doi: 10.1007/s10535-012-0271-3
Mathobo, R., Marais, D., and Steyn, J. M. (2017). The effect of drought stress on yield, leaf gaseous exchange and chlorophyll fluorescence of dry beans (Phaseolus vulgaris L.). Agric. Water Manag. 180, 118–125. doi: 10.1016/j.agwat.2016.11.005
Mekawy, A. M. M., Assaha, D. V., Yahagi, H., Tada, Y., Ueda, A., and Saneoka, H. (2015). Growth, physiological adaptation, and gene expression analysis of two Egyptian rice cultivars under salt stress. Plant Physiol. Biochem. 87, 17–25. doi: 10.1016/j.plaphy.2014.12.007
Mekureyaw, M. F., Pandey, C., Hennessy, R. C., Nicolaisen, M. H., Liu, F., Nybroe, O., et al. (2022). The cytokinin-producing plant beneficial bacterium Pseudomonas fluorescens G20-18 primes tomato (Solanum lycopersicum) for enhanced drought stress responses. J. Plant Physiol. 270:153629. doi: 10.1016/j.jplph.2022.153629
Mesa-Marin, J., Del-Saz, N. F., Rodríguez-Llorente, I. D., Redondo-Gómez, S., Pajuelo, E., Ribas-Carbó, M., et al. (2018). PGPR reduce root respiration and oxidative stress enhancing Spartina maritima root growth and heavy metal rhizoaccumulation. Front. Plant Sci. 9:1500. doi: 10.3389/fpls.2018.01500
Mohammed, S., and Mohammed, M. I. (2019). Effect of abiotic stress on irrigated maize forage yield as compared to sorghum. J. Hortic. Plant Res. 6, 27–36. doi: 10.18052/www.scipress.com/JHPR.6.27
Mok, D. W., and Mok, M. C. (2001). Cytokinin metabolism and action. Annu. Rev. Plant Biol. 52:89. doi: 10.1146/annurev.arplant.52.1.89
Muneer, S., Ko, C. H., Wei, H., Chen, Y., and Jeong, B. R. (2016). Physiological and proteomic investigations to study the response of tomato graft unions under temperature stress. PLoS One 11:e0157439. doi: 10.1371/journal.pone.0157439
Nithyapriya, S., Lalitha, S., Sayyed, R., Reddy, M., Dailin, D. J., El Enshasy, H. A., et al. (2021). Production, purification, and characterization of bacillibactin siderophore of Bacillus subtilis and its application for improvement in plant growth and oil content in sesame. Sustainability 13:5394. doi: 10.3390/su13105394
Park, Y. G., Mun, B. G., Kang, S. M., Hussain, A., Shahzad, R., Seo, C. W., et al. (2017). Bacillus aryabhattai SRB02 tolerates oxidative and nitrosative stress and promotes the growth of soybean by modulating the production of phytohormones. PLoS One 12:e0173203. doi: 10.1371/journal.pone.0173203
Pathak, M. R., Teixeira da Silva, J. A., and Wani, S. H. (2014). Polyamines in response to abiotic stress tolerance through transgenic approaches. GM Crops Food 5, 87–96. doi: 10.4161/gmcr.28774
Patten, C. L., and Glick, B. R. (2002). Role of Pseudomonas putida indoleacetic acid in development of the host plant root system. Appl. Environ. Microbiol. 68, 3795–3801. doi: 10.1128/AEM.68.8.3795-3801.2002
Paz, R. C., Rocco, R. A., Reinoso, H., Menéndez, A. B., Pieckenstain, F. L., and Ruiz, O. A. (2012). Comparative study of alkaline, saline, and mixed saline–alkaline stresses with regard to their effects on growth, nutrient accumulation, and root morphology of Lotus tenuis. J. Plant Growth Regul. 31, 448–459. doi: 10.1007/s00344-011-9254-4
Pierik, R., Tholen, D., Poorter, H., Visser, E. J. W., and Voesenek, L. A. C. J. (2006). The Janus face of ethylene: growth inhibition and stimulation. Trends Plant Sci. 11, 176–183. doi: 10.1016/j.tplants.2006.02.006
Poupin, M. J., Greve, M., Carmona, V., and Pinedo, I. (2016). A complex molecular interplay of auxin and ethylene signaling pathways is involved in Arabidopsis growth promotion by Burkholderia phytofirmans PsJN. Front. Plant Sci. 7:492. doi: 10.3389/fpls.2016.00492
Prabhavathi, V., Yadav, J., Kumar, P., and Rajam, M. (2002). Abiotic stress tolerance in transgenic eggplant (Solanum melongena L.) by introduction of bacterial mannitol phosphodehydrogenase gene. Mol. Breed. 9, 137–147. doi: 10.1023/A:1026765026493
Rabiei, Z., Hosseini, S. J., Pirdashti, H., and Hazrati, S. (2020). Physiological and biochemical traits in coriander affected by plant growth-promoting rhizobacteria under salt stress. Heliyon 6:e05321. doi: 10.1016/j.heliyon.2020.e05321
Rajkumar, M., Ae, N., and Freitas, H. (2009). Endophytic bacteria and their potential to enhance heavy metal phytoextraction. Chemosphere 77, 153–160. doi: 10.1016/j.chemosphere.2009.06.047
Rajkumar, M., Ae, N., Prasad, M. N. V., and Freitas, H. (2010). Potential of siderophore-producing bacteria for improving heavy metal phytoextraction. Trends Biotechnol. 28, 142–149. doi: 10.1016/j.tibtech.2009.12.002
Rashid, M., Hussain, Q., Khan, K. S., Alwabel, M. I., Ahmad, M., Alvi, S., et al. (2019). “Carbon sequestration in alkaline soils” in Sustainable agriculture reviews 38: carbon sequestration: Volume 2 materials and chemical methods. eds. A. A. M. Inamuddin and E. Lichtfouse (Cham: Springer), 149–167.
Razzaq, A., Ali, A., Safdar, L. B., Zafar, M. M., Rui, Y., Shakeel, A., et al. (2020). Salt stress induces physiochemical alterations in rice grain composition and quality. J. Food Sci. 85, 14–20. doi: 10.1111/1750-3841.14983
Rosier, A., Medeiros, F. H., and Bais, H. P. (2018). Defining plant growth promoting rhizobacteria molecular and biochemical networks in beneficial plant-microbe interactions. Plant Soil 428, 35–55. doi: 10.1007/s11104-018-3679-5
Roychoudhury, A., and Aftab, T. (2021). Phytohormones, plant growth regulators and signaling molecules: cross-talk and stress responses. Plant Cell Rep. 40, 1301–1303. doi: 10.1007/s00299-021-02755-9
Sabir, A., Naveed, M., Bashir, M. A., Hussain, A., Mustafa, A., Zahir, Z. A., et al. (2020). Cadmium mediated phytotoxic impacts in Brassica napus: managing growth, physiological and oxidative disturbances through combined use of biochar and Enterobacter sp. MN17. J. Environ. Manag. 265:110522. doi: 10.1016/j.jenvman.2020.110522
Saddiq, M. S., Afzal, I., Basra, S. M., Iqbal, S., and Ashraf, M. (2020). Sodium exclusion affects seed yield and physiological traits of wheat genotypes grown under salt stress. J. Soil Sci. Plant Nutr. 20, 1442–1456. doi: 10.1007/s42729-020-00224-y
Saghafi, D., Ghorbanpour, M., and Lajayer, B. A. (2018). Efficiency of Rhizobium strains as plant growth promoting rhizobacteria on morpho-physiological properties of Brassica napus L. under salinity stress. J. Soil Sci. Plant Nutr. 18, 253–268. doi: 10.4067/S0718-95162018005000903
Sahithi, B. M., Razi, K., Al Murad, M., Vinothkumar, A., Jagadeesan, S., Benjamin, L. K., et al. (2021). Comparative physiological and proteomic analysis deciphering tolerance and homeostatic signaling pathways in chrysanthemum under drought stress. Physiol. Plant. 172, 289–303. doi: 10.1111/ppl.13142
Saikia, J., Sarma, R. K., Dhandia, R., Yadav, A., Bharali, R., Gupta, V. K., et al. (2018). Alleviation of drought stress in pulse crops with ACC deaminase producing rhizobacteria isolated from acidic soil of northeast India. Sci. Rep. 8, 1–16. doi: 10.1038/s41598-018-21921-w
Saini, S., Kaur, N., and Pati, P. K. (2018). Reactive oxygen species dynamics in roots of salt sensitive and salt tolerant cultivars of rice. Anal. Biochem. 550, 99–108. doi: 10.1016/j.ab.2018.04.019
Salam, A., Bashir, S., Khan, I., Hussain, Q., Gao, R., and Hu, H. (2019). Biochar induced Pb and Cu immobilization, phytoavailability attenuation in Chinese cabbage, and improved biochemical properties in naturally co-contaminated soil. J. Soils Sediments 19, 2381–2392. doi: 10.1007/s11368-019-02250-5
Saleem, A. R., Brunetti, C., Khalid, A., Della Rocca, G., Raio, A., Emiliani, G., et al. (2018). Drought response of Mucuna pruriens (L.) DC. Inoculated with ACC deaminase and IAA producing rhizobacteria. PLoS One 13:e0191218. doi: 10.1371/journal.pone.0191218
Santhi, C., Rajesh, M., Ramesh, S., Muralikrishna, K., Gangaraj, K., and Payal, G. (2021). Genome-wide exploration of auxin response factors (ARFs) and their expression dynamics in response to abiotic stresses and growth regulators in coconut (Cocos nucifera L.). plant. Gene 28:100344. doi: 10.1016/j.plgene.2021.100344
Shahzad, R., Khan, A. L., Bilal, S., Waqas, M., Kang, S. M., and Lee, I. J. (2017). Inoculation of abscisic acid-producing endophytic bacteria enhances salinity stress tolerance in Oryza sativa. Environ. Exp. Bot. 136, 68–77. doi: 10.1016/j.envexpbot.2017.01.010
Shan, Z., Luo, X., Wei, M., Huang, T., Khan, A., and Zhu, Y. (2018). Physiological and proteomic analysis on long-term drought resistance of cassava (Manihot esculenta Crantz). Sci. Rep. 8, 1–12. doi: 10.1038/s41598-018-35711-x
Sharma, A., Singh, R. K., Singh, P., Vaishnav, A., Guo, D. J., Verma, K. K., et al. (2021). Insights into the bacterial and nitric oxide-induced salt tolerance in sugarcane and their growth-promoting abilities. Microorganisms 9:2203. doi: 10.3390/microorganisms9112203
Shi, D., and Sheng, Y. (2005). Effect of various salt-alkaline mixed stress conditions on sunflower seedlings and analysis of their stress factors. Environ. Exp. Bot. 54, 8–21. doi: 10.1016/j.envexpbot.2004.05.003
Shu, K., Zhou, W., Chen, F., Luo, X., and Yang, W. (2018). Abscisic acid and gibberellins antagonistically mediate plant development and abiotic stress responses. Front. Plant Sci. 9:416. doi: 10.3389/fpls.2018.00416
Siddikee, M. A., Glick, B. R., Chauhan, P. S., Jong Yim, W., and Sa, T. (2011). Enhancement of growth and salt tolerance of red pepper seedlings (Capsicum annuum L.) by regulating stress ethylene synthesis with halotolerant bacteria containing 1-aminocyclopropane-1-carboxylic acid deaminase activity. Plant Physiol. Biochem. 49, 427–434. doi: 10.1016/j.plaphy.2011.01.015
Siddiqui, Z. S., Wei, X., Umar, M., Abideen, Z., Zulfiqar, F., Chen, J., et al. (2022). Scrutinizing the application of saline endophyte to enhance salt tolerance in rice and maize plants. Front. Plant Sci. 12:3334. doi: 10.3389/fpls.2021.770084
Singh, P., Chauhan, P. K., Upadhyay, S. K., Singh, R. K., Dwivedi, P., Wang, J., et al. (2022). Mechanistic insights and potential use of siderophores producing microbes in rhizosphere for mitigation of stress in plants grown in degraded land. Front. Microbiol. :13. doi: 10.3389/fmicb.2022.898979
Singh, D., Singh, C. K., Singh, Y. P., Singh, V., Singh, R., Tomar, R. S. S., et al. (2018). Evaluation of cultivated and wild genotypes of Lens species under alkalinity stress and their molecular collocation using microsatellite markers. PLoS One 13:e0199933. doi: 10.1371/journal.pone.0199933
Sorty, A. M., Meena, K. K., Choudhary, K., Bitla, U. M., Minhas, P., and Krishnani, K. (2016). Effect of plant growth promoting bacteria associated with halophytic weed (Psoralea corylifolia L) on germination and seedling growth of wheat under saline conditions. Appl. Biochem. Biotechnol. 180, 872–882. doi: 10.1007/s12010-016-2139-z
Spaepen, S., Versées, W., Gocke, D., Pohl, M., Steyaert, J., and Vanderleyden, J. (2007). Characterization of phenylpyruvate decarboxylase, involved in auxin production of Azospirillum brasilense. J. Bacteriol. 189, 7626–7633. doi: 10.1128/JB.00830-07
Talbi, S., Rojas, J. A., Sahrawy, M., Rodríguez-Serrano, M., Cárdenas, K. E., Debouba, M., et al. (2020). Effect of drought on growth, photosynthesis and total antioxidant capacity of the Saharan plant Oudeneya africana. Environ. Exp. Bot. 176:104099. doi: 10.1016/j.envexpbot.2020.104099
Teshome, D. T., Zharare, G. E., and Naidoo, S. (2020). The threat of the combined effect of biotic and abiotic stress factors in forestry under a changing climate. Front. Plant Sci. 11:601009. doi: 10.3389/fpls.2020.601009
Thompson, A. J., Jackson, A. C., Symonds, R. C., Mulholland, B. J., Dadswell, A. R., Blake, P. S., et al. (2000). Ectopic expression of a tomato 9-cis-epoxycarotenoid dioxygenase gene causes over-production of abscisic acid. Plant J. 23, 363–374. doi: 10.1046/j.1365-313x.2000.00789.x
Tóth, B., Juhász, C., Labuschagne, M., and Moloi, M. J. (2020). The influence of soil acidity on the physiological responses of two bread wheat cultivars. Int. J. Agron. 9:1472. doi: 10.3390/plants9111472
Tran, T. A., and Popova, L. P. (2013). Functions and toxicity of cadmium in plants: recent advances and future prospects. Turk. J. Bot. 37, 1–13. doi: 10.3906/bot-1112-16
Upadhyay, S., Singh, G., and Singh, D. (2016). “Mechanism and understanding of PGPR: an approach for sustainable agriculture under abiotic stresses” in Microbes and environmental management. eds. J. S. Singh and D. P. Singh (India: Studium Press Pvt. Ltd.), 225–254.
Uzma, M., Iqbal, A., and Hasnain, S. (2022). Drought tolerance induction and growth promotion by indole acetic acid producing Pseudomonas aeruginosa in Vigna radiata. PLoS One 17:e0262932. doi: 10.1371/journal.pone.0262932
Van de Poel, B., and Van Der Straeten, D. (2014). 1-aminocyclopropane-1-carboxylic acid (ACC) in plants: more than just the precursor of ethylene! Front. Plant Sci. 5:640. doi: 10.3389/fpls.2014.00640
Vardharajula, S., Zulfikar Ali, S., Grover, M., Reddy, G., and Bandi, V. (2011). Drought-tolerant plant growth promoting Bacillus spp.: effect on growth, osmolytes, and antioxidant status of maize under drought stress. J. Plant Interact. 6, 1–14. doi: 10.1080/17429145.2010.535178
Verma, V., Ravindran, P., and Kumar, P. P. (2016). Plant hormone-mediated regulation of stress responses. BMC Plant Biol. 16, 1–10. doi: 10.1186/s12870-016-0771-y
Vibhuti, C. S., Bargali, K., and Bargali, S. (2015). Seed germination and seedling growth parameters of rice (Oryza sativa L.) varieties as affected by salt and water stress. Indian J. Agric. Sci. 85, 102–108.
Vives-Peris, V., Gómez-Cadenas, A., and Pérez-Clemente, R. M. (2018). Salt stress alleviation in citrus plants by plant growth-promoting rhizobacteria Pseudomonas putida and Novosphingobium sp. Plant Cell Rep. 37, 1557–1569. doi: 10.1007/s00299-018-2328-z
Vocciante, M., Grifoni, M., Fusini, D., Petruzzelli, G., and Franchi, E. (2022). The Role of Plant Growth-Promoting Rhizobacteria (PGPR) in Mitigating Plant’s Environmental Stresses. Appl. Sci. 12, 1231. doi: 10.3390/app12031231
Wani, A. B., Chadar, H., Wani, A. H., Singh, S., and Upadhyay, N. (2017). Salicylic acid to decrease plant stress. Environ. Chem. Lett. 15, 101–123. doi: 10.1007/s10311-016-0584-0
Wu, W., Qu, S., Nel, W., and Ji, J. (2020). The impact of natural weathering and mining on heavy metal accumulation in the karst areas of the Pearl River Basin. China. Sci. Total Environ. 734:139480. doi: 10.1016/j.scitotenv.2020.139480
Xu, J., Li, X. L., and Luo, L. (2012). Effects of engineered Sinorhizobium meliloti on cytokinin synthesis and tolerance of alfalfa to extreme drought stress. Appl. Environ. Microbiol. 78, 8056–8061. doi: 10.1128/AEM.01276
Yang, J., Duan, G., Li, C., Liu, L., Han, G., Zhang, Y., et al. (2019). The crosstalks between Jasmonic acid and other plant hormone signaling highlight the involvement of jasmonic acid as a core component in plant response to biotic and abiotic stresses. Front. Plant Sci. 10:01349. doi: 10.3389/fpls.2019.01349
Yang, Y., Zhang, L., Huang, X., Zhou, Y., Quan, Q., Li, Y., et al. (2020). Response of photosynthesis to different concentrations of heavy metals in Davidia involucrata. PLoS One 15:e0228563. doi: 10.1371/journal.pone.0228563
Yasmin, H., Naeem, S., Bakhtawar, M., Jabeen, Z., Nosheen, A., Naz, R., et al. (2020). Halotolerant rhizobacteria Pseudomonas pseudoalcaligenes and Bacillus subtilis mediate systemic tolerance in hydroponically grown soybean (Glycine max L.) against salinity stress. PLoS One 15:e0231348. doi: 10.1371/journal.pone.0231348
Yue, L., Uwaremwe, C., Tian, Y., Liu, Y., Zhao, X., Zhou, Q., et al. (2022). Bacillus amyloliquefaciens rescues glycyrrhizic acid loss under drought stress in Glycyrrhiza uralensis by activating the Jasmonic acid pathway. Front. Microbiol. 12:798525. doi: 10.3389/fmicb.2021.798525
Zaheer, M. S., Ali, H. H., Iqbal, M. A., Erinle, K. O., Javed, T., Iqbal, J., et al. (2022). Cytokinin production by Azospirillum brasilense contributes to increase in growth, yield, antioxidant, and physiological systems of wheat (Triticum aestivum L.). Front. Microbiol. 13:886041. doi: 10.3389/fmicb.2022.886041
Zerrouk, I. Z., Rahmoune, B., Khelifi, L., Mounir, K., Baluska, F., and Ludwig-Müller, J. (2019). Algerian Sahara PGPR confers maize root tolerance to salt and aluminum toxicity via ACC deaminase and IAA. Acta Physiol. Plant. 41:91. doi: 10.1007/s11738-019-2881-2
Zhang, L., Gao, M., Hu, J., Zhang, X., Wang, K., and Ashraf, M. (2012). Modulation role of abscisic acid (ABA) on growth, water relations and glycinebetaine metabolism in two maize (Zea mays L.) cultivars under drought stress. Int. J. Mol. Sci. 13, 3189–3202. doi: 10.3390/ijms13033189
Zhang, S., Jiang, Q., Liu, X., Liu, L., and Ding, W. (2020). Plant growth promoting rhizobacteria alleviate aluminum toxicity and ginger bacterial wilt in acidic continuous cropping soil. Front. Microbiol. 11:569512. doi: 10.3389/fmicb.2020.569512
Zhang, C., Yi, X., Gao, X., Wang, M., Shao, C., Lv, Z., et al. (2020). Physiological and biochemical responses of tea seedlings (Camellia sinensis) to simulated acid rain conditions. Ecotoxicol. Environ. Saf. 192:110315. doi: 10.1016/j.ecoenv.2020.110315
Zhang, Z., Zhou, W., and Li, H. (2005). The role of GA, IAA and BAP in the regulation of in vitro shoot growth and microtuberization in potato. Acta Physiol. Plant. 27, 363–369. doi: 10.1007/s11738-005-0013-7
Zhang, Y. K., Zhu, D. F., Zhang, Y. P., Chen, H. Z., Xiang, J., and Lin, X. Q. (2015). Low pH-induced changes of antioxidant enzyme and ATPase activities in the roots of rice (Oryza sativa L.) seedlings. PLoS One 10:e0116971. doi: 10.1371/journal.pone.0116971
Zhou, M., Engelmann, T., and Lutts, S. (2019). Salinity modifies heavy metals and arsenic absorption by the halophyte plant species Kosteletzkya pentacarpos and pollutant leaching from a polycontaminated substrate. Ecotoxicol. Environ. Saf. 182:109460. doi: 10.1016/j.ecoenv.2019.109460
Zhou, R., Kong, L., Yu, X., Ottosen, C. O., Zhao, T., Jiang, F., et al. (2019). Oxidative damage and antioxidant mechanism in tomatoes responding to drought and heat stress. Acta Physiol. Plant. 41:20. doi: 10.1007/s11738-019-2805-1
Zhou, C., Zhu, L., Xie, Y., Li, F., Xiao, X., Ma, Z., et al. (2017). Bacillus licheniformis SA03 confers increased saline–alkaline tolerance in Chrysanthemum plants by induction of abscisic acid accumulation. Front. Plant Sci. 8:01143. doi: 10.3389/fpls.2017.01143
Zhu, J., Cai, D., Wang, J., Cao, J., Wen, Y., He, J., et al. (2021). Physiological and anatomical changes in two rapeseed (Brassica napus L.) genotypes under drought stress conditions. Oil Crop Sci. 6, 97–104. doi: 10.1016/j.ocsci.2021.04.003
Żurek, G., Prokopiuk, K., and Rachwalska, A. (2018). Effect of drought on the performance of three turf grass species. J. Plant Breed. Crop Sci. 78, 3–22. doi: 10.1515/plass-2018-0010
Zwack, P. J., and Rashotte, A. M. (2013). Cytokinin inhibition of leaf senescence. Plant Signal. Behav. 8:e24737. doi: 10.4161/psb.24737
Keywords: plant growth promoting rhizobacteria, phytohormones, abiotic stress tolerance, water stress, drought stress, temperature stress
Citation: Aloo BN, Dessureault-Rompré J, Tripathi V, Nyongesa BO and Were BA (2023) Signaling and crosstalk of rhizobacterial and plant hormones that mediate abiotic stress tolerance in plants. Front. Microbiol. 14:1171104. doi: 10.3389/fmicb.2023.1171104
Edited by:
Kunal, Shree Guru Gobind Singh Tricentenary University, IndiaReviewed by:
Devendra Singh, Dr. Rajendra Prasad Central Agricultural University, IndiaNitesh Singh, Shree Guru Gobind Singh Tricentenary University, India
Copyright © 2023 Aloo, Dessureault-Rompré, Tripathi, Nyongesa and Were. This is an open-access article distributed under the terms of the Creative Commons Attribution License (CC BY). The use, distribution or reproduction in other forums is permitted, provided the original author(s) and the copyright owner(s) are credited and that the original publication in this journal is cited, in accordance with accepted academic practice. No use, distribution or reproduction is permitted which does not comply with these terms.
*Correspondence: B. N. Aloo, YmFsb29AdW9lbGQuYWMua2U=; J. Dessureault-Rompré, SmFjeW50aGUuRGVzc3VyZWF1bHQtUm9tcHJlQGZzYWEudWxhdmFsLmNh
†These authors have contributed equally to this work