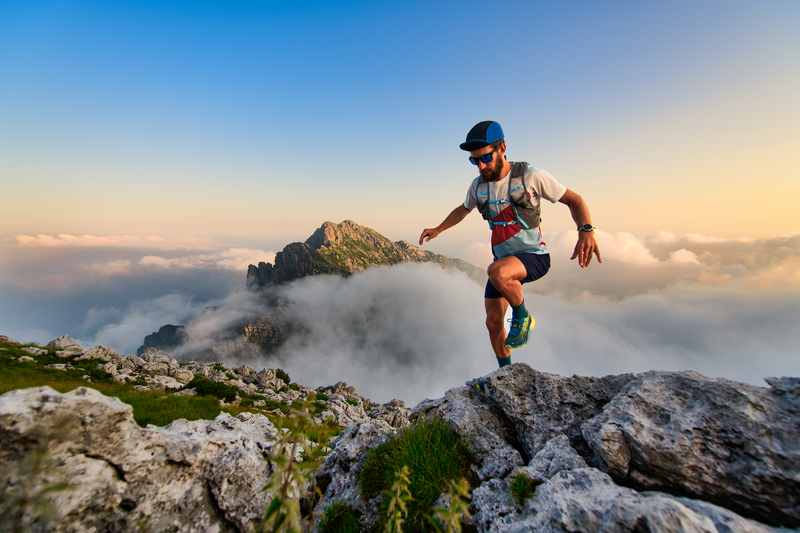
95% of researchers rate our articles as excellent or good
Learn more about the work of our research integrity team to safeguard the quality of each article we publish.
Find out more
REVIEW article
Front. Microbiol. , 23 June 2023
Sec. Microbe and Virus Interactions with Plants
Volume 14 - 2023 | https://doi.org/10.3389/fmicb.2023.1169809
This article is part of the Research Topic Beneficial Microbe-Plant Interactions Under Biotic/Abiotic Stress Conditions View all 23 articles
Soil salinity is one of the main problems that affects global crop yield. Researchers have attempted to alleviate the effects of salt stress on plant growth using a variety of approaches, including genetic modification of salt-tolerant plants, screening the higher salt-tolerant genotypes, and the inoculation of beneficial plant microbiome, such as plant growth-promoting bacteria (PGPB). PGPB mainly exists in the rhizosphere soil, plant tissues and on the surfaces of leaves or stems, and can promote plant growth and increase plant tolerance to abiotic stress. Many halophytes recruit salt-resistant microorganisms, and therefore endophytic bacteria isolated from halophytes can help enhance plant stress responses. Beneficial plant-microbe interactions are widespread in nature, and microbial communities provide an opportunity to understand these beneficial interactions. In this study, we provide a brief overview of the current state of plant microbiomes and give particular emphasis on its influence factors and discuss various mechanisms used by PGPB in alleviating salt stress for plants. Then, we also describe the relationship between bacterial Type VI secretion system and plant growth promotion.
Plants have relationships with various members of an ecosystem and can grow well in their natural environments. Plant microbiome refers to all microorganisms that survive in a specific environmental niche at a given time, and is one of the most important organisms that can have beneficial effects for plants, such as improving nutrient uptake, disease resistance, and stress tolerance (Santoyo et al., 2016; Trivedi et al., 2022; Xiong and Lu, 2022). Microbial communities can improve plant tolerance to biotic and abiotic stresses, and increase plant growth and yield by improving bioavailability for transporting nutrients from the soil. Microbial communities vary across different environments and are generally thought to be tissue-specific; they mainly colonize in root and tissues of plants or around roots in the soil. Bacteria that colonize and survive in the host plant are called endophytes (Bohra et al., 2022). However, the beneficial effects of endophytic bacteria on host plants are generally greater than those of plant growth promoting rhizosphere (PGPR), and these effects may be enhanced especially when plants are under stress (Miliute et al., 2015; Malik and Arora, 2022).
Plants start to establish their microbial community system, known as the seed-borne microbiome that is transmitted from the seed to the plant (Nelson, 2018; Abdelfattah et al., 2022), from the seed stage. The interplay between plants and their endophytes during the plant’s entire life cycle may have profound impacts on plant ecology, health, and productivity. The niche differentiation of microbial communities at the soil-root interface has attracted the most attention, due to different plant compartments provide different ecological niches for microbes living in them (Edwards et al., 2015). Plant roots (in the rhizosphere) act as a bridge connecting plants and soil microbes and secrete many photosynthates to the surrounding soil environment, providing a very attractive and nutrient-rich niche for microbes and making the rhizosphere have the highest microbial diversity (Singh and Mukerji, 2006).
Salt stress is one of the main factors limiting agricultural productivity and causing a significant loss of global crop yields (Ha-Tran et al., 2021). In order to cope with harsh environments, many strategies have been implemented, such as plant breeding, plant genetic engineering and climate-smart agriculture (Upadhyaya et al., 2021). Plant growth promoting bacteria (PGPB) have been shown to effectively improve plant tolerance to abiotic stress and increase crop yield, making them promising biofertilizers (Das et al., 2022; Zilaie et al., 2022). However, its unstable growth-promoting effect and unclear mechanism seriously hinder the use of microbial agents. In this review, we provide a brief overview of the current state of plant microbiomes and give particular emphasis on its influence factors and discuss various mechanisms used by PGPB in alleviating salt stress for plants. Then, we also describe the relationship between bacterial Type VI secretion system and plant growth promotion.
Soil salinity is one of the main abiotic stresses that limit plant growth and yield. Plants under salt stress have significantly reduced productivity, and the extent of these effects depends on salt content, plant type, and the stage of plant growth and development. Many studies have reported that high levels of soil salinity can inhibit see germination, significantly reduce root and shoot growth, as well as decrease plant photosynthesis, stomatal conductance, chlorophyll content, and mineral uptake (Laghmouchi et al., 2017; Sánchez-García et al., 2017). Currently, the mechanisms by which salt stress affects plant growth mainly include disturbances in plant hormone balance, changes in protein metabolism, inhibition of enzyme activity involved in nucleic acid metabolism, and nutrient uptake disorders, which are caused by osmotic effects and salt ion toxicity (Zhu, 2001; Amirjani, 2011). In addition, studies have also found that salt can inhibit cell membrane and cell wall maturation (Ismail and Horie, 2017).
The high salt concentration around the plant roots increases osmotic stress, which in turn leads to ion toxicity. Osmotic stress mainly affects water and nutrient uptake, seed germination, cell elongation, leaf development, lateral bud development, photosynthetic rate, transport from root to stem, and the supply of carbohydrates to meristematic tissues (Van Zelm et al., 2020). The ion toxicity of Na+ and Cl− hinders the absorption of nutrients such as Ca+ and K+, causing an imbalance in plant nutrition (Acosta-Motos et al., 2017). Soil salinity prevents the absorption of Ca+ by plants, affecting the growth of roots, root tips, and root hairs, reducing the areas where rhizobia can invade and the development of nodules (Bouhmouch et al., 2005). In addition, the increase in Na+ and Cl− content reduces the absorption and utilization of some elements (N, P, K, Mg) by plants. The imbalance of minerals usually changes the structure and chemical composition of the lipid bilayer of the membrane, and controls the selective transport of solutes and affects the ability of ions to transport inward, causing solute leakage and forming super-osmotic phenomena (Lodhi et al., 2009).
Ion toxicity also destroys the photosynthetic apparatus by blocking the photosystem II reaction center, oxygen-evolving complex, and electron transport chain, thereby inhibiting photosynthesis (Kan et al., 2017). The accumulation of a large amount of Na+ in plant tissues suppresses photosynthesis, resulting in the accumulation of reactive oxygen species, which have many adverse effects on plants, such as accelerating toxic reactions, DNA mutations, protein degradation, and membrane damage (Islam et al., 2015). Salt also has a negative effect on plant height and root length, causing stomatal closure, increasing leaf temperature, and reducing elongation (Yavaş and Hussain, 2022). As salt concentration increases, plant height and root length tend to decrease, and these negative results are related to changes in osmotic potential and the decreased ability of rice to absorb water and nutrients (Gain et al., 2004). Furthermore, stomatal closure can increase plant CO2 deficiency, leading to a decrease in enzyme activity in the Calvin cycle (Ashraf et al., 2020). In addition to the effects on plant organs and structures mentioned above, root zone salinization also hinders the developmental stages of individual plants. Overall, from seed germination to seed formation stages, salt has a serious impact on the physiological and biochemical activities of plants.
In natural ecosystems, organisms do not live alone, but in close association with a wide variety of microorganisms. In nature, plants do not grow like axenic organisms, but host a diverse microbial community named the plant microbiome (Müller et al., 2016). The plant microbiome plays an essential role in plant growth, improving the bioavailability of nutrients and increasing the host’s tolerance to biotic and abiotic stresses. In turn, host plants provide habitat and nutrients for microbes (Trivedi et al., 2020). In fact, microbial communities exist in almost all plant tissues, such as the soil-root interface (rhizosphere), the interior of plant tissues (endophyte), and the air-plant interface (phyllosphere) (de Medeiros Azevedo et al., 2021). All these microenvironments provide specific biotic and abiotic conditions for microbial communities to survive. Understanding plant-microbe interactions not only provides a better understanding of the role of microbes in plant growth and development, but also allows the use of their relationships for phytoremediation, sustainable crop production, and secondary metabolite production. However, it is important to note that the plant microbiome can be influenced by various environmental factors, host and other biotic and abiotic conditions. The plant microbiome contains mixed populations of taxa from diverse phyla, in which each member adapts to the host microenvironment in some way and co-exists with other members. Nonetheless, determining plant-microbiome interactions can be challenging due to the complexity of microbial community assembly mechanisms.
Healthy plants host taxonomically diverse microbial communities (Trivedi et al., 2020). Terrestrial plants are colonized by a huge variety of microorganisms that affect plant health and growth in beneficial, harmful, or neutral ways. Previous studies have confirmed that the host-microbial communities are not generally random assemblages, but show defined phylogenetic structures, and only a few major groups constitute the plant microbial communities with high abundance, including Proteobacteria, Actinobacteria and Bacteroidetes (Müller et al., 2016).
Many studies aimed to identify the core microbiome by phylogenetically distinct plant species or compartments. Yeoh et al. (2016) determined the core root microbes of sugarcane, including Proteobacteria, Actinobacteria, Bacteroidetes, Chloroflexi, and Firmicutes, which were found to overlap with those of Arabidopsis thaliana, suggesting that some bacterial families may have a long-term association with plants (Yeoh et al., 2016). The study of core phyllosphere microbiomes of Arabidopsis, soybean and clover found that a high consistency of communities in leaves of these different plants (Delmotte et al., 2009). Proteobacteria, Actinobacteria and Bacteroidetes were the core phyllosphere microbiota in these three plants (Delmotte et al., 2009). Kembel et al. (2014) tested the relationships between the phyllosphere bacterial biodiversity and the functional traits, taxonomy and phylogeny of their plant host, and confirmed that the structure of phyllosphere bacterial community was highly correlated with the evolutionary relatedness of hosts and a series of plant functional traits related to host ecological strategies (Kembel et al., 2014). Similarly, Leff et al. (2015) observed the bacterial community composition is highly variable, but this variability is predictable and dependent on plant compartments (Leff et al., 2015). This work highlights the importance of considering plant spatial structure when studying plant-associated microbial communities and their effects on plant hosts.
The plant microbiome interacts with its host in a variety of manners and affect host’s growth. However, relatively little information is available on the composition and diversity of bacterial communities in different aboveground plant organs, especially those associated with flowers. Zarraonaindia et al. (2015) revealed some unique plant-associated microbiota by comparing the bacterial communities in grapevine leaves and flowers; the flower-associated microbiota almost entirely composed of Proteobacteria (Zarraonaindia et al., 2015). Junker and Keller (2015) determined that the leaves microbiome of Metrosideros polymorpha hosts a unique indicator community composed of relatively abundant bacterial groups; these indicator communities are accompanied by a large number of ubiquitous or rare bacteria with lower abundances.
Microorganisms colonizing the host plant benefit from nutrients provided by the plant and form taxonomically consistent community patterns (Müller et al., 2016). This mechanism consists of two aspects: on the one hand, plants provide unoccupied niches for invading microorganisms, which are able to take advantage of the provided niche or nutrients, resulting in stochastic colonization events; on the other hand, plant-microbe coevolution might provide the basis for plant-driven selection processes, leading to key species that actively recruit microbial members or at least provide functions to plant hosts, which contribute to the shaping of the ultimate microbial community during plant development (Müller et al., 2016).
Plants act as environmental filters to shape the microbial communities, and there are many studies about the effects of microbial communities on their hosts (Adair and Douglas, 2017). However, the study of the mechanisms underlying the assembly of the plant microbiome is still in its infancy. Based on microbial communities and genetic evidence, Reinhold-Hurek et al. (2015) proposed a enrichment model of root-related microbiomes, explaining the reasons for the decreased diversity and increased specificity of microbial communities from the bulk soil to the root interior (Reinhold-Hurek et al., 2015). Soils provide a highly diverse microbiome that is influenced by soil physicochemical factors, environmental factors, and vegetation types. This process is mainly divided into three steps. In the first step, in the rhizosphere, the community may be refined due to the influence of roots, such as carbon source, oxygen, pH or nutrient consumption. In the second step, the community shows more obvious refinement in close contact with the host on the root surface, and plant genotype has a significant influence. Biofilm formation or specific adhesion mechanisms may be involved in this enrichment step. Third, in the least complex community, Proteobacteria is a fast-growing bacterium with high metabolic activity, which dominates the endosphere bacterial community. For endophytic bacteria, specific bacterial traits are required to colonize in plant tissues, such as community sensing, plant polysaccharide degradation or resistance to reactive oxygen species. Compared with other plant compartments, host genotype may have the greatest influence on community structure in this habitat.
The establishment of plant microbiome is a dynamic process, reflecting the changes of community composition over time in response to environmental changes. Among them, environmental conditions, root exudates, microbe-microbe and plant-microbe interactions, and various stages of plant development all affect the assembly of microbial communities (Huang et al., 2014). It is not clear whether bacteria establish a founding community early in plant development, and then constantly colonize in the new habitat by clonal propagation (Müller et al., 2016). Therefore, it is very difficult to understand a system as complex as the plant microbiome and to distinguish between co-evolving interactions and stochastic processes.
So far, little is known about the source of microbial communities and how plants shape their specific microbial communities. Although the initial bacterial communities are similar to their respective seed banks, they become more specific and more diverse as plants grow and develop (Chaparro et al., 2014; Müller et al., 2016). Hardoim et al. (2012) found that about 45% of the bacterial communities in rice progeny seeds were similar to the parental plant, indicating that the rice microbiome could be directly obtained from seeds and transmitted in the form of endophyte (Hardoim et al., 2012). The diversity and dynamics of seed microbiota represent the culmination of a complex process of microbial interactions mediated by the plant throughout its life cycle (Nelson, 2018). Microbes associated with endosperm are more likely to vertically spread, while those associated with seed coat are more likely to horizontally transmit (Barret et al., 2016).
Soil represents an extremely rich microbial pool on Earth, serving as a major seed bank for the microbiota of the rhizosphere and root, and a driving force for community formation (Müller et al., 2016). In many studies, it has been confirmed that soil type has a significant impact on rhizosphere microbial communities (Gottel et al., 2011; Lundberg et al., 2012; Edwards et al., 2015). In addition, under the same environmental and soil conditions, plant species and genotype are one of the driving factors for the structural and functional diversity of plant microbiomes (Matthews et al., 2019). The functional diversity of the microbiome is also affected by plant varieties or cultivars. Even the sister lines of the same hybrid had different root-associated diazotrophs in rice (Knauth et al., 2005). Moreover, the influence of plants on microbial communities seems to be heritable, reflected in the finding that the gene expression of the microbiota in interspecific hybrid rice showed the intermediate level between the parental species (Knauth et al., 2005). These studies suggest that plants act as filters for their microbiome. However, quantitative analysis of microbial community composition between ecotypes or plant varieties showed that differences in microbial diversity related to genotypes were relatively small compared with environmental factors (Edwards et al., 2015).
Finally, microorganisms themselves can also influence the establishment of microbial communities, which can be mediated via either directly at the microbial-microbial interaction or indirectly through host-microbial interactions. This feature has been confirmed in many studies. For example, Chapelle et al. (2016) analyzed the rhizosphere microbiome of sugar beet seedlings, and found that invasive pathogenic fungi could induce stress responses in rhizobacterial communities, leading to shifts in microbial community composition (Chapelle et al., 2016). Han et al. (2020) determined that some rhizosphere microbes in nodules were related to the composition of rhizobia, and confirmed that bacterial communities played a crucial role in the interaction between soybean rhizobia and host (Han et al., 2020). Niu et al. (2017) assembled a simple and representative synthetic bacterial model and studied the community assembly process of maize seedlings. The abundance of representative strains was monitored by selective culture-dependent method, and the role of each strain in community assembly was studied. When Enterobacter cloacae was removed, the community structure was completely lossed, indicating that E. cloacae plays the role of key species in this model ecosystem (Niu et al., 2017). In summary, current studies indicate that microbe-microbe-host interactions are one of the factors affecting the assembly of the plant microbiome.
Plant growth-promoting bacteria (PGPB) is a kind of beneficial free-living bacteria in soil or colonized in plant tissue, which can promote plant growth and nutrient uptake, improve plant disease resistance, and suppress harmful microbes (Hoque et al., 2022; Khatoon et al., 2022). The most important thing is that PGPB is safe for the environment, humans and animals (Khabbaz et al., 2019). PGPB includes microorganisms isolated from different ecosystems such as soil, plants, and oceans, which can colonize the internal plant tissues and have several beneficial effects on the host directly or indirectly under salinity stress (Figure 1). Although thousands of PGPB species have been isolated over the past few decades, the mechanism of plant-growth promotion is still uncertain. Once successfully colonized in the rhizosphere as well as plant tissues, PGPB can help to facilitate plant growth through various mechanisms, such as regulating plant hormones and nutrient acquisition, and reducing ethylene production (Afzal et al., 2019). PGPB can also indirectly control plant health by suppressing infectious diseases (e.g., production of antibiotics and lyases, inhibition of nutrient uptake by pathogens, and activation of plant defense mechanisms), thereby protecting plants from pathogen attack (Miliute et al., 2015). Because of their extensive properties in maintaining crop health, as well as their environmentally friendly nature, PGPB have become an important component of sustainable agricultural development (Das et al., 2022).
PGPB includes two groups of bacteria, namely, rhizospheric bacteria near plant roots and endophytic bacteria in plant tissues. In most cases, rhizospheric and endophytic PGPB use similar mechanisms to promote plant growth, and the main difference is that endophytic PGPB once colonizes the tissues of the host plant and is no longer affected by the vagaries of soil environmental conditions (Akhtar et al., 2022). These changing soil conditions may inhibit the function and proliferation of PGPB in the rhizosphere, and other soil bacteria may compete for the same invasive sites in the root surface of host plants. PGPB have been found as a promising way of boosting the yield and growth of salinity-stressed plants (Table 1).
Many agricultural soils are of poor quality due to deficiency of one or more plant nutrients, so plant growth in this condition is suboptimal. In order to solve this problem and achieve higher crop yields, cultivators increasingly rely heavily on inorganic chemical-based fertilizers of nitrogen and phosphorus. The production of chemical fertilizers is not only costly, but also depletes non-renewable resources, such as oil and gas, and causes harmful effects to humans and the environment (Shirokov and Tikhnenko, 2021). It is clearly beneficial and sustainable if effective biological means of providing nitrogen and phosphorus to plants could be used to replace part of the chemical nitrogen and phosphorus usage (Glick, 2012). PGPB can help host plants to uptake nutrients, including nitrogen, iron, and phosphorus, which is considered a direct mechanism of plant growth promotion (Figure 2).
Figure 2. The potential mechanism of bacteria-mediated plant growth promotion under salt stress. Plant Growth Promoting Bacteria (PGPB) serve as a biofertilizer promote plant growth by enhancing nutrient availability, regulating plant hormones, and increasing plant stress tolerance. They increase the concentrations of growth hormones, such as gibberellins, cytokinins, while decreasing ethylene levels through ACC deaminase. Moreover, PGPB also produce volatile organic compounds (VOCs) that induce disease resistance and abiotic stress tolerance. In addition, PGPB alleviates stress by augmenting extracellular polysaccharides, osmoregulants, and antioxidants, thereby reducing reactive oxygen species and oxidative stress.
PGPB may prevent nutrient loss by fixing soil nutrients to increase the concentration and availability of nutrients in the rhizosphere. Common bacteria with biological nitrogen fixation are rhizobia and nitrogen-fixing bacteria, such as Rhizobia spp., Azospirillum brasilense, Burkholderia spp., Gluconacetobacter diazotrophicus, and Herbaspirillum seropedicae have been reported to increase host plant biomass by fixing atmospheric nitrogen (Bhattacharjee et al., 2008). In non-leguminous plants, PGPB has nitrogen-fixing effects by inducing the formation of nodules and secretes a unique nitrogenase that converts atmospheric nitrogen into ammonia (Aasfar et al., 2021). Endophytic bacteria are inferior to rhizobia in nitrogen fixation, while endophytic diazotrophic are very good in this respect. It has been found that G. diazotrophicus co-exists with plants such as spruce and pine, helping the conifers to grow in soils with severe nitrogen deficiency (Carrell and Frank, 2014).
Although the content of phosphorus in soil is generally quite high, it occurs mostly in insoluble form and cannot be used by plants (Etesami, 2020). In addition, much of the soluble inorganic phosphorus used as fertilizer can form insoluble P-complexes with soil particles (Elhaissoufi et al., 2021). The limited bioavailability of phosphorus in soil, coupled with the importance of this element for plant growth, limits plant growth. Therefore, phosphate solubilizing PGPB can promote plant growth by the solubilization and mineralization of phosphorus, which is achieved by the release of phosphate from inorganic phosphorus and organic phosphorus by organic acids such as acetate, succinate, citrate and gluconate, or by phosphatases (Rebi et al., 2022). Endophytic bacteria can use many mechanisms such as acidification, chelation, ion exchange, and production of organic acids and phosphatases to increase the availability of soil phosphorus (Walia et al., 2017). Recent studies have proposed a new mechanism of phosphorus solubilization, which 2, 3-dimethyl fumaric acid, gluconic acid and butylamine secreted by Pseudomonas prosekii can dissolve phosphate by providing H+ ions and organic anions (Yu et al., 2019). The phosphate-solubilizing bacterium Burkholderia cepacia can secrete gluconic, formic acids and an unknown acid, which might be an important mechanism for phosphate solubilization (Pande et al., 2019).
Like phosphorus, a great amount of insoluble iron in soil is not available for plants. In iron-deficient conditions, bacteria can effectively bind iron through two strategies, including releasing protons and organic acids to reduce soil pH, or secreting iron-chelating complexes (siderophore) that bind ferric ions. Plants can obtain iron from siderophore through root chelation degradation or ligand exchange (Albelda-Berenguer et al., 2019). PGPB prevents the proliferation of plant pathogens by secreting siderophores with an extremely high affinity for ferric iron, and the mechanism is that these siderophores bind tightly to most of the Fe3+ present in the rhizosphere and absorb the bound iron into the PGPB or host plant, preventing any fungal and bacterial pathogens from obtaining enough iron for their growth (Olanrewaju et al., 2017). As a result, pathogens are unable to reproduce due to iron deficiency, causing them to lose their ability to act as pathogens. The effectiveness of this biological control approach is based on the fact that the siderophores secreted by PGPB have a much higher affinity for iron than fungal siderophores (usually by many orders of magnitude) (Olanrewaju et al., 2017). Siderophores as iron chelators have been shown to promote plant growth significantly in many studies. When iron is not available, PGPB G. diazotrophicus and A. brasilense could produce hydroxamate and catechol type siderophores, respectively, to chelate iron and promote host absorption (Delaporte-Quintana et al., 2020). Another study has shown that some bacteria secrete growth-inhibitory siderophores that alter the interaction between the microbiome and pathogens. Rhizosphere microbiome members secreting growth-inhibiting siderophores could inhibit pathogens in vitro as well as in natural and greenhouse soils. Conversely, rhizosphere microbiome members with growth-promotive siderophores are often at a disadvantage in the competition and easily facilitate plant infection by the pathogen (Gu et al., 2020). This is one of the reasons why plants inoculated with PGPB show less of a growth promotion effect than expected.
The physiological activities of most plants are regulated by one or more plant hormones. In addition to plants, many beneficial microbes can also synthesize plant hormones (Figure 2) (Khan et al., 2020). PGPB can promote nutrient uptake and metabolism of host plants by producing plant hormones (Stegelmeier et al., 2022). Studies have found that PGPB can secrete plant hormones, resulting in facilitated seed germination, accelerated root growth, changed root morphology, and increased root biomass (Olanrewaju et al., 2017). At present, many studies found that PGPB Azospirillum, Aeromonas, Azotobacter, Bacillus, Paenibacillus, Burkholderia, Enterobacter, Pantoea, Pseudomonas and Rhizobium are able to secrete plant hormones (Khabbaz et al., 2019). Plant hormones generally include abscisic acid, cytokinin, ethylene, gibberellin and indole-3-acetic acid (IAA); among them, IAA and ethylene are the two classical phytohormones in the plant-bacteria interaction (Stegelmeier et al., 2022).
As a major plant hormone, IAA is involved in plant development and physiological processes, including intercellular signal transduction, regulation of plant growth, and induction of plant defense system (Tian et al., 2018). It can also facilitate the formation of lateral and adventive roots, affect photosynthesis and biosynthesis of metabolites, and mediate resistance to stress conditions (Li et al., 2021). IAA produced by PGPB and the plant’s endogenous IAA stimulate auxin signal transduction pathways, including various auxin response factors, thus resulting in stimulation of cell growth and proliferation (Ilangumaran and Smith, 2017).
IAA synthesized by bacteria may be involved in plant-bacteria interaction at different levels, especially in plant growth and nodule formation (Lobo et al., 2023). IAA produced by PGPB can either stimulate root development when the plant inherent IAA is suboptimal, or inhibit root growth when the concentration of IAA is already optimal (Munir et al., 2022). In addition, a plant’s endogenous IAA levels can also determine whether bacterial IAA promotes or inhibits plant growth, since bacterial IAA production generally favors plants with low endogenous IAA levels. Overall, bacterial IAA increases plant’s root surface area and length, thus providing the plant more access to soil nutrients (Yadav et al., 2022). In addition, bacterial IAA can loosen the plant cell walls, thus promoting an increase in the amount of root exudation that provide additional nutrients to support the growth of rhizosphere bacteria (Ahmad et al., 2022).
Ethylene is an important plant hormone involved in different developmental and physiological processes such as aging, shedding, and pathogen defense signals (Bayanati et al., 2021). Some PGPB possess 1-aminocyclopropane-1-carboxylate (ACC) deaminase activity, which hydrolyzes the ethylene precursor, ACC, into α-ketobutyric acid and ammonia (Yadav et al., 2022). Therefore, hydrolysis of ACC can alleviate ethylene stress on plants and improve plant growth under stress conditions. Many PGPB can utilize ACC as a sole nitrogen source, indicating that these strains contain ACC deaminase (del Carmen Orozco-Mosqueda et al., 2020). So far, ACC deaminase in PGPB has proven to be a very effective mechanism to counter stress conditions in plants. However, these studies are limited to greenhouses and growing chambers.
Studies have shown that IAA promotes the transcription of genes encoding ACC synthase, thereby increasing ACC concentration and ultimately leading to ethylene accumulation (Glick, 2014). After environmental stress, PGPB containing ACC deaminase hydrolyzes ACC and reduces the ethylene level of plants, thus reducing the stress response of plants. In the absence of ACC deaminase-producing bacteria, ethylene restricts cell growth and proliferation by restricting auxin response factor transcription and stimulating IAA to produce additional ethylene (Khabbaz et al., 2019). Thus, in the presence of ACC deaminase, auxin response factor transcription is not inhibited, and IAA can stimulate cell growth and proliferation without causing ethylene accumulation. Therefore, ACC deaminase can not only reduce the inhibitory effect of ethylene on plant growth, but also enable IAA to promote plant growth to the maximum extent in the presence or absence of plant stress.
In addition to IAA, many PGPB also produce cytokinin and gibberellin (Kumar et al., 2020). Cytokinin influences not only many aspects of plant growth, development and physiology, including cell division, chloroplast differentiation and delay of senescence, but also numerous plant-biotic interactions (Akhtar et al., 2019). It is worth noting that cytokinins are produced by both pathogenic and beneficial microbes and improve the resistance of plants against pathogen infections. Transgenic plants that overproduce cytokinins, especially during abiotic stress, can significantly mitigate the harmful effects of stress (Rivero et al., 2007). PGPB R. leguminosarum, Bacillus, and Pseudomonas can produce cytokinin, promote cell division and growth, enhance the absorption efficiency of nutrients, and thus as a result improve plant yield (Olanrewaju et al., 2017). In terms of biotic stress, studies have confirmed that cytokinin can initiate plant responses to trauma and insect pests by activating the expression of trauma-inducing genes and by inducing the increase of compounds against insect (Giron et al., 2013), which indicates that the physiological and metabolic signals of cytokinin may interfere on anti-herbivore defense in foliage (Akhtar et al., 2019).
Gibberellin is a plant growth regulator mainly involved in seed germination, leaf and stem growth, flowering, fruit formation and plant senescence (Desta and Amare, 2021). Bacteria that have been shown to produce a variety of gibberellins include: Acetobacter diazotrophicus, H. seropedicae, A. lipoferum, Rhizobium phaseoli, Enterococcus faecium, Sphingomonas, etc. (Ali et al., 2017). Compared with the research on cytokinins, relatively limited research has been done on the interactions among gibberellins and plants and microbes. In most cases, gibberellin produced by PGPB also exhibits other mechanisms that promote plant growth, and the phenotypic changes of different plant varieties are related to changes in plant growth hormone biosynthesis (Kurepin et al., 2015).
Endophytic bacteria indirectly promote the growth of host plants by inhibiting plant pathogens and pests with the production of antagonistic compounds such as antibiotics, toxins, siderophores, lytic enzymes, and antimicrobial volatile organic compounds (Figure 2) (Elnahal et al., 2022). Among them, Actinobacteria, Bacillus, Enterobacter, Paenibacillus, Pseudomonas and other bacteria are common genera with antibacterial activity against plant pathogens (Afzal et al., 2019). Some of the enzymes produced by PGPB, including chitinase, cellulase, glucanase, protease and lipase, can lyse a portion of the cell walls of pathogens (Ghosh et al., 2021). PGPB that secrete one or more of these enzymes have been shown to have biocontrol activities against a wide range of competitor pathogens (Selari et al., 2023). Naama-Amar et al. (2022) reported that a candidate biocontrol agent Frateuria defendens could inhibit Spiroplasma melliferum growth by secreting antimicrobial metabolites (Naama-Amar et al., 2022). A plant growth-promoting endophytic bacterium Pseudomonas protegens MP12 can produce important antifungal compounds (2, 4-diacetylphloroglucinol, pyoluteorin and pyrrolnitrin), and exhibits inhibitory effects on the mycelial growth of phytopathogens such as Botrytis cinerea, Alternaria alternata, Aspergillus niger, Penicillium expansum and Neofusicoccum parvum (Andreolli et al., 2019). Bacillus produced antibiotic lipopeptides, such as iturin, bacillomycin, bacilysin, fengycin, surfactin, and zwittermycin, which have broad spectrum of action against pathogens (Saravanakumar et al., 2019).
PGPB can also activate plant-induced systemic resistance (ISR) against a broad spectrum of pathogens (Datta et al., 2022). ISR is phenotypically similar to systemic acquired resistance (SAR) that occurs when plants activate their defense mechanisms in response to pathogen infection (Meena et al., 2020). The prime function of ISR is to activate plant defense mechanisms and protect unexposed parts of plants against pathogenic microbe and herbivorous insect invasions (Sardar et al., 2021). PGPB-mediated ISR depones on jasmonic acid and ethylene signaling pathways in plants (Abid and Karim, 2021). These hormones stimulate the host plant’s defense responses to a range of pathogens without the need for PGPB to interact directly with pathogens. In addition to involvement in jasmonate and ethylene signaling pathways, ISR also plays a crucial role in host resistance by enhancing the activity of pattern recognition receptors through cellular or hormonal defenses (Hossain and Chung, 2019).
PGPB can also emit volatile organic compounds (VOCs) as biocontrol factors or deterrents against pathogens (Choub et al., 2022). Recently, many studies have reported the production of VOCs secreted by PGPB that disrupted cell membrane integrity, spore germination and mycelial growth of plant pathogenic fungi and other competing bacteria species (Chatterjee and Niinemets, 2022). Recent advances in analytical science such as the headspace solid-phase microextraction/gas chromatography–mass spectrometry (HS-SPME/GC–MS), have been used for extraction, identification, and characterization of VOCs emitted by PGPB and other bacterial species (Drabińska et al., 2022; Tabbal et al., 2022; Wu et al., 2022). Additionally, some VOCs can repress the expression of virulence traits involved in host colonization, such as motility, root colonization, and biofilm formation, thus, for example, effectively controlling tomato wilt (Raza et al., 2016). In summary, VOCs produced by PGPB help plants cope with abiotic and biotic stresses by inducing systemic resistance or inhibiting the growth of a wide range of pathogen.
In addition to producing substances that harm or inhibit plant pathogens, some PGPB may compete for nutrients or niches with pathogens (Selari et al., 2023). In fact, it is widely believed that this competitiveness of PGPB works together with other biocontrol mechanisms to repress the growth of plant pathogens (Olanrewaju et al., 2017). It is important to note that the effectiveness of PGPB depends on their versatility and adaptation to new niches as well as their ability to colonize and compete with other members of plant microbiome (Rilling et al., 2019). Root exudates contain chemo-attractants like amino acids, organic acids and specific sugars that are good carbon sources for PGPB activity and competitive colonization (Feng et al., 2021). Additionally, the mutual signal exchange between plants and microsymbionts, along with flagellar motility, further enhances the affinity of PGPB in root surface (Raina et al., 2019).
In addition to beneficial traits such as IAA production, nitrogen fixation and phosphate dissolution, PGPB can accumulate osmotic adjustment substances under stressful conditions (Figure 2) (Feng et al., 2023). Under drought and salt stress conditions, plant cells can improve the water activity of cells and maintain normal metabolic activities by accumulating compatible solutes (soluble sugars, polyols, glycine betaine, proline, free amino acids) (Ali et al., 2022). Under abiotic stress, one of the most significant changes is the accumulation of plant proline that can improve the activity of various enzymes, stabilize intracellular pH and remove reactive oxygen species, thereby maintaining antioxidant activity (Abdelaal et al., 2021). Many PGPB can induce plant proline synthesis under stress conditions, which helps to maintain cell osmotic pressure and improve plant salt tolerance (Khabbaz et al., 2019).
Trehalose, a non-reducing disaccharide, can act as a protective agent to alleviate a variety of environmental stresses, including drought, salinity and extreme temperatures (Greco et al., 2021). When cells are dehydrated, trehalose can form a gel phase to bind with surrounding macromolecules and membranes thereby replacing water loss and decreasing the damage to cells (del Carmen Orozco-Mosqueda et al., 2022). In addition, trehalose prevents the degradation, denaturation and aggregation of proteins under high and low temperature stress (Vinciguerra et al., 2022). Under stress conditions, trehalose and ACC deaminase synergistically protect crops from stress by stabilizing biological structures and lowering ethylene levels, respectively (del Carmen Orozco-Mosqueda et al., 2022). Compared with wild type Rhizobium etli, the number of nodules, nitrogenase activity and biomass of Phaseolus vulgaris increased when inoculated with R. etli overexpressing trehalose synthase, and the host plant inoculated with mutants recovered more strongly under drought stress (Suárez et al., 2008). Although it is also possible to overexpress trehalose genes in transgenic plants, it is much simpler to achieve the same goal through co-inoculation with PGPB. Moreover, since most strains have no specific host, therefore, the same strain can effectively help different plants mitigate stress.
Many bacteria have evolved specialized protein secretion systems to transport proteins out of the bacterial cells and even directly into the host target cells. Bacterial secretory systems are divided into at least nine classes (Tat system, type I-VII, and type IX) based on their structure, function, and specific effects, with each system transporting a specific subset of proteins, known as effectors (Green and Mecsas, 2016). Among them, Type VI Secretion System (T6SS) is a sophisticated nano-weapon to inject toxic effectors into eukaryotic or prokaryotic cells, and presents in nearly 25% of all Gram-negative bacteria, including many plant symbiotic bacteria (Salinero-Lanzarote et al., 2019). Bacteria can use T6SS to manipulate and destroy eukaryotic cells and/or fight other bacteria to gain a dominant status in the ecological niche (Kim et al., 2020). Indeed, bacteria with T6SS appear to have a significant adaptive advantage in the microbial community. Bacteria containing T6SS synthesize both immune proteins and T6SS effectors to ensure against self-poisoning or targeting by sister-cells (Steele et al., 2017). Functional T6SS contributes to the virulence of the host, improves bacterial robustness, and enhances environmental adaptation through bacterial competition (Russell et al., 2014; Kim et al., 2020). Although T6SS is ubiquitous, its general mechanism and physiological role are still not fully understood. To date, the studies related to T6SS and plant growth promotion characteristics are very few and mainly focus on biological control agents, antagonism, biofilm formation and environmental adaptability (Bernal et al., 2018).
Biofilms are structurally complex microbial communities attached to living or abiotic surfaces and surrounded by complex extracellular polymers. Microbial colonization of plant roots can be promoted by the formation of biofilms (Niu et al., 2020). Gallique et al. (2017) reported that mutations in three T6SS-related genes (hcp1, hcp2, or hcp3) of Pseudomonas fluorescens MFE01 did not reduce biofilm formation, but these three Hcp proteins are essential for the formation of mature biofilm structures. However, in another similar study, these three T6SS-related gene mutants of P. aeruginosa PAO1 had no impact on biofilm formation or environmental adaptation ability, but swarming motility was reduced, which is another important aspect of biofilm formation ability, suggesting that T6SS is associated with biofilm formation but not environmental adaptation (Chen et al., 2020). The T6SS gene cluster present in the Acidovorax citrulli was confirmed to be involved in multiple biological processes, including colonization, competition and biofilm formation (Fei et al., 2022).
Evidence is also emerging that T6SSs could contribute to inter-bacterial competition. Interestingly, many PGPB harbor one or more T6SS, however, the function of T6SS in PGPB is poorly understand. In a previous study, it was found that the T6SS of PGPB Azospirillum brasilense provides antibacterial activities against a number of plant pathogens in vitro, and might confer T6SS-dependent bio-control protection to microalgae and plants against bacterial pathogens (Cassan et al., 2021). However, Lin et al. (2018) found instead of being an antihost or antibacterial weapon of the bacterium, the T6SS in Azorhizobium caulinodans ORS571 seems to participate specifically in symbiosis by increasing its symbiotic competitiveness.
Most organisms with T6SS are not pathogenic and are found in diverse environments as symbionts. Although the secretory system was thought to be involved in the interaction between PGPB and plants, only recently research has been functionally dissected in nitrogen-fixing Azoarcus olearius, in which T6SS positively affected plant colonization efficiency (Jiang et al., 2019). The T6SS effector Hcp1 up-regulated in rice inoculated with a well-known growth-promoting rhizobacteria Herbaspirillum rubrisubalbicans, suggesting that T6SS plays a role in rice colonization (Valdameri et al., 2017). Similarly, the T6SS mutant of PGPB Enterobacter sp. J49 showed a significant decrease in the epiphytic and endophytic colonization, indicating that although T6SS is not essential, it may participate in bacterial colonization (Lucero et al., 2022). Salinero-Lanzarote et al. (2019) described the growth-promoting trait of T6SS in Rhizobium etli Mim1, which can effectively increase nodule size in legumes, suggesting that T6SS plays an active role in the Rhizobium-legumes symbiosis. The knockout mutant of T6SS gene cluster in rice endophyte Kosakonia showed a decreased ability to colonize the rhizoplane and endosphere, suggesting that T6SS is involved in the colonization process of plant-bacteria interaction (Mosquito et al., 2020). Another plant-associated microbe, P. taiwanensis, has been shown to use its T6SS to mediate colonization resistance against bacterial plant pathogens through T6SS-mediated secretion of pyoverdine, an iron chelator (Chen et al., 2016). These results suggest that there may be a relationship between T6SS and plant growth promoting properties.
Soil salinity is expected to increase dramatically in the coming years and will hinder agricultural production. Various conventional reclamation methods of saline-alkali land are found to be unsustainable and economically difficult to achieve. The use of PGPB to inoculate plants has become an important method to alleviate soil salt stress and improve crop yield, but better results have only been obtained in laboratory or greenhouse conditions, not in the field. To effectively utilize PGPB in salinized agroecosystems, a bottom-up approach is necessary, starting with the screening of halotolerant PGPB strains and careful formulation design for field application. Additionally, more molecular studies of plant-microbe interactions are needed to better understand the mechanisms involved in inducing systemic tolerance and performing rhizosphere engineering under salt stress. Studying the metabolic and genetic behavior of halotolerant PGPB is also important to understand how they work and adapt to high salt environments. This will provide a reference for the development of reliable biological inoculants in saline soils.
Although PGPB has various mechanisms to improve plant growth and biological control against plant pathogens under saline conditions, there are still many constraints to its wide application in different agro-ecosystems. Our understanding of the molecular mechanism by which halotolerant PGPB alleviates salt stress is also in its infancy, but it offers a sustainable way to increase the productivity of saline soils and contribute to the goals of food security and controlling desertification of agro-ecosystems. Improving our understanding of the mechanism of PGPB regulating plant stress resistance and restricting bacterial activity under stress conditions can enhance the effectiveness of using bacterial inoculants.
MP wrote the initial draft and made figure. MP and ZJ edited the manuscript. FZ and ZW helped in collection and review of literature. All authors contributed to the article and approved the submitted version.
This study was financially supported by the National Natural Science Foundation of China (No. 32200094), the Natural Science Foundation of Hubei Province (2022CFB674), and the Open Fund of Hubei Key Laboratory of Biological Resources Protection and Utilization (Hubei Minzu University) (No. PT012201).
The authors declare that the research was conducted in the absence of any commercial or financial relationships that could be construed as a potential conflict of interest.
All claims expressed in this article are solely those of the authors and do not necessarily represent those of their affiliated organizations, or those of the publisher, the editors and the reviewers. Any product that may be evaluated in this article, or claim that may be made by its manufacturer, is not guaranteed or endorsed by the publisher.
Aasfar, A., Bargaz, A., Yaakoubi, K., Hilali, A., Bennis, I., Zeroual, Y., et al. (2021). Nitrogen fixing Azotobacter species as potential soil biological enhancers for crop nutrition and yield stability. Front. Microbiol. 12:628379. doi: 10.3389/fmicb.2021.628379
Abdelaal, K., AlKahtani, M., Attia, K., Hafez, Y., Király, L., and Künstler, A. (2021). The role of plant growth-promoting bacteria in alleviating the adverse effects of drought on plants. Biology 10:520. doi: 10.3390/biology10060520
Abdelfattah, A., Tack, A. J., Lobato, C., Wassermann, B., and Berg, G. (2022). From seed to seed: the role of microbial inheritance in the assembly of the plant microbiome. Trends Microbiol. 31, 346–355. doi: 10.1016/j.tim.2022.10.009
Abid, S. A., and Karim, S. H. (2021). Salicylic acid, Jasmonic acid and ethylene involved in the resistance induced by the Rhizobacterium Pta-Ct2 in Arabidopsis thaliana against Botrytis cinerea. J. Plant Product. 12, 361–364. doi: 10.21608/jpp.2021.166404
Acosta-Motos, J. R., Ortuño, M. F., Bernal-Vicente, A., Diaz-Vivancos, P., Sanchez-Blanco, M. J., and Hernandez, J. A. (2017). Plant responses to salt stress: adaptive mechanisms. Agronomy 7:18. doi: 10.3390/agronomy7010018
Adair, K. L., and Douglas, A. E. (2017). Making a microbiome: the many determinants of host-associated microbial community composition. Curr. Opin. Microbiol. 35, 23–29. doi: 10.1016/j.mib.2016.11.002
Afzal, I., Shinwari, Z. K., Sikandar, S., and Shahzad, S. (2019). Plant beneficial endophytic bacteria: mechanisms, diversity, host range and genetic determinants. Microbiol. Res. 221, 36–49. doi: 10.1016/j.micres.2019.02.001
Agha, M. S., Haroun, S. A., Abbas, M. A., Sofy, M. R., and Mowafy, A. M. (2023). Growth and metabolic response of Glycine max to the plant growth-promoting Enterobacter Delta PSK and Bradyrhizobium japonicum under salinity stress. J. Plant Growth Regul., 1–15. doi: 10.1007/s00344-023-10967-4
Ahmad, E., Sharma, P. K., and Khan, M. S. (2022). “IAA biosynthesis in bacteria and its role in plant-microbe interaction for drought stress management” in Plant stress Mitigators (Singapore: Springer), 235–258.
Ahmed, S., Heo, T.-Y., Roy Choudhury, A., Walitang, D. I., Choi, J., and Sa, T. (2021). Accumulation of compatible solutes in rice (Oryza sativa L.) cultivars by inoculation of endophytic plant growth promoting bacteria to alleviate salt stress. Appl. Biol. Chem. 64, 1–14. doi: 10.1186/s13765-021-00638-x
Akhtar, S. S., Mekureyaw, M. F., Pandey, C., and Roitsch, T. (2019). Role of cytokinins for interactions of plants with microbial pathogens and pest insects. Front. Plant Sci. 10, 1777–1789. doi: 10.3389/fpls.2019.01777
Akhtar, N., Rashid, M. M., Perween, S., Kumar, G., and Nanda, S. (2022). “Mode of action of different microbial products in plant growth promotion” in New and future developments in microbial biotechnology and bioengineering (Netherlands: Elsevier), 85–120.
Albelda-Berenguer, M., Monachon, M., and Joseph, E. (2019). Siderophores: from natural roles to potential applications. Adv. Appl. Microbiol. 106, 193–225. doi: 10.1016/bs.aambs.2018.12.001
Al-Garni, S. M., Khan, M. M. A., and Bahieldin, A. (2019). Plant growth-promoting bacteria and silicon fertilizer enhance plant growth and salinity tolerance in Coriandrum sativum. J. Plant Interact. 14, 386–396. doi: 10.1080/17429145.2019.1641635
Ali, S., Charles, T. C., and Glick, B. R. (2017). “Endophytic phytohormones and their role in plant growth promotion” in Functional importance of the plant microbiome (Cham, Switzerland: Springer), 89–105.
Ali, B., Wang, X., Saleem, M. H., Hafeez, A., Afridi, M. S., Khan, S., et al. (2022). PGPR-mediated salt tolerance in maize by modulating plant physiology, antioxidant defense, compatible solutes accumulation and bio-surfactant producing genes. Plan. Theory 11:345. doi: 10.3390/plants11030345
Amirjani, M. R. (2011). Effect of salinity stress on growth, sugar content, pigments and enzyme activity of rice. Int. J. Bot. 7, 73–81. doi: 10.3923/ijb.2011.73.81
Andreolli, M., Zapparoli, G., Angelini, E., Lucchetta, G., Lampis, S., and Vallini, G. (2019). Pseudomonas protegens MP12: a plant growth-promoting endophytic bacterium with broad-spectrum antifungal activity against grapevine phytopathogens. Microbiol. Res. 219, 123–131. doi: 10.1016/j.micres.2018.11.003
Ashraf, M. Y., Tariq, S., Saleem, M., Khan, M. A., Hassan, S. W. U., and Sadef, Y. (2020). Calcium and zinc mediated growth and physio-biochemical changes in mungbean grown under saline conditions. J. Plant Nutr. 43, 512–525. doi: 10.1080/01904167.2019.1685098
Bakhshandeh, E., Gholamhosseini, M., Yaghoubian, Y., and Pirdashti, H. (2020). Plant growth promoting microorganisms can improve germination, seedling growth and potassium uptake of soybean under drought and salt stress. Plant Growth Regul. 90, 123–136. doi: 10.1007/s10725-019-00556-5
Barret, M., Guimbaud, J. F., Darrasse, A., and Jacques, M. A. (2016). Plant microbiota affects seed transmission of phytopathogenic microorganisms. Mol. Plant Pathol. 17, 791–795. doi: 10.1111/mpp.12382
Bayanati, M., Lajayer, B. A., and Pessarakli, M. (2021). “Senescence: the final phase of plant life” in Handbook of plant and crop physiology (Boca Raton, Florida, USA: CRC Press), 98–109.
Bernal, P., Llamas, M. A., and Filloux, A. (2018). Type VI secretion systems in plant-associated bacteria. Environ. Microbiol. 20, 1–15. doi: 10.1111/1462-2920.13956
Bhattacharjee, R. B., Singh, A., and Mukhopadhyay, S. (2008). Use of nitrogen-fixing bacteria as biofertiliser for non-legumes: prospects and challenges. Appl. Microbiol. Biotechnol. 80, 199–209. doi: 10.1007/s00253-008-1567-2
Bohra, N., Singh, R. K., Jain, R., Sharma, L., Sathyanarayana, E., Quiroz-Figueroa, F. R., et al. (2022). “Endophytic bacteria for plant growth promotion” in Bacterial endophytes for sustainable agriculture and environmental management (Singapore: Springer), 187–199.
Bouhmouch, I., Souad-Mouhsine, B., Brhada, F., and Aurag, J. (2005). Influence of host cultivars and rhizobium species on the growth and symbiotic performance of Phaseolus vulgaris under salt stress. J. Plant Physiol. 162, 1103–1113. doi: 10.1016/j.jplph.2004.12.003
Carrell, A. A., and Frank, A. C. (2014). Pinus flexilis and Picea engelmannii share a simple and consistent needle endophyte microbiota with a potential role in nitrogen fixation. Front. Microbiol. 5:333. doi: 10.3389/fmicb.2014.00333
Cassan, F. D., Coniglio, A., Amavizca, E., Maroniche, G., Cascales, E., Bashan, Y., et al. (2021). The Azospirillum brasilense type VI secretion system promotes cell aggregation, biocontrol protection against phytopathogens and attachment to the microalgae Chlorella sorokiniana. Environ. Microbiol. 23, 6257–6274. doi: 10.1111/1462-2920.15749
Castaldi, S., Valkov, V. T., Ricca, E., Chiurazzi, M., and Isticato, R. (2023). Use of halotolerant Bacillus amyloliquefaciens RHF6 as a bio-based strategy for alleviating salinity stress in Lotus japonicus cv Gifu. Microbiol. Res. 268:127274. doi: 10.1016/j.micres.2022.127274
Chaparro, J. M., Badri, D. V., and Vivanco, J. M. (2014). Rhizosphere microbiome assemblage is affected by plant development. ISME J. 8, 790–803. doi: 10.1038/ismej.2013.196
Chapelle, E., Mendes, R., Bakker, P. A. H., and Raaijmakers, J. M. (2016). Fungal invasion of the rhizosphere microbiome. ISME J. 10, 265–268. doi: 10.1038/ismej.2015.82
Chatterjee, P., and Niinemets, Ü. (2022). Improving plant stress resistance by growth-promoting bacteria and evaluating the improvements by volatile emissions. Plant Soil 476, 403–419. doi: 10.1007/s11104-022-05576-1
Chen, W.-J., Kuo, T.-Y., Hsieh, F.-C., Chen, P.-Y., Wang, C.-S., Shih, Y.-L., et al. (2016). Involvement of type VI secretion system in secretion of iron chelator pyoverdine in Pseudomonas taiwanensis. Sci. Rep. 6:32950. doi: 10.1038/srep32950
Chen, L., Zou, Y., Kronfl, A. A., and Wu, Y. (2020). Type VI secretion system of Pseudomonas aeruginosa is associated with biofilm formation but not environmental adaptation. MicrobiologyOpen 9:e991. doi: 10.1002/mbo3.991
Choub, V., Won, S.-J., Ajuna, H. B., Moon, J.-H., Choi, S.-I., Lim, H.-I., et al. (2022). Antifungal activity of volatile organic compounds from Bacillus velezensis CE 100 against Colletotrichum gloeosporioides. Horticulturae 8:557. doi: 10.3390/horticulturae8060557
Das, P. P., Singh, K. R., Nagpure, G., Mansoori, A., Singh, R. P., Ghazi, I. A., et al. (2022). Plant-soil-microbes: a tripartite interaction for nutrient acquisition and better plant growth for sustainable agricultural practices. Environ. Res. 214:113821. doi: 10.1016/j.envres.2022.113821
Datta, D., Behera, L., Chaudhary, V. T., Kumar, S., and Bisen, K. (2022). “Endophytes: rendering systemic resistance to plants” in Rhizosphere microbes (Singapore: Springer), 175–195.
de Medeiros Azevedo, T., Aburjaile, F. F., Ferreira-Neto, J. R. C., Pandolfi, V., and Benko-Iseppon, A. M. (2021). The endophytome (plant-associated microbiome): methodological approaches, biological aspects, and biotech applications. World J. Microbiol. Biotechnol. 37, 1–25. doi: 10.1007/s11274-021-03168-2
de Oliveira Lopes, Á. L., Setubal, I. S., da Costa Neto, V. P., Zilli, J. E., Rodrigues, A. C., and Bonifacio, A. (2022). Synergism of Bradyrhizobium and Azospirillum baldaniorum improves growth and symbiotic performance in lima bean under salinity by positive modulations in leaf nitrogen compounds. Appl. Soil Ecol. 180:104603. doi: 10.1016/j.apsoil.2022.104603
del Carmen Orozco-Mosqueda, M., Glick, B. R., and Santoyo, G. (2020). ACC deaminase in plant growth-promoting bacteria (PGPB): an efficient mechanism to counter salt stress in crops. Microbiol. Res. 235:126439. doi: 10.1016/j.micres.2020.126439
del Carmen Orozco-Mosqueda, M., Kumar, A., Glick, B. R., and Santoyo, G. (2022). “The role of bacterial ACC deaminase and trehalose in increasing salt and drought tolerance in plants,” in Mitigation Plant Abiotic Stress Microorgan (USA: Academic Press), 41–52.
Delaporte-Quintana, P., Lovaisa, N. C., Rapisarda, V. A., and Pedraza, R. O. (2020). The plant growth promoting bacteria Gluconacetobacter diazotrophicus and Azospirillum brasilense contribute to the iron nutrition of strawberry plants through siderophores production. Plant Growth Regul. 91, 185–199. doi: 10.1007/s10725-020-00598-0
Delmotte, N., Knief, C., Chaffron, S., Innerebner, G., Roschitzki, B., Schlapbach, R., et al. (2009). Community proteogenomics reveals insights into the physiology of phyllosphere bacteria. Proc. Natl. Acad. Sci. 106, 16428–16433. doi: 10.1073/pnas.0905240106
Desta, B., and Amare, G. (2021). Paclobutrazol as a plant growth regulator. Chem. Biol. Technol. Agricult. 8, 1–15. doi: 10.1186/s40538-020-00199-z
Drabińska, N., Hewett, K., White, P., Avison, M. B., Persad, R., Ratcliffe, N. M., et al. (2022). Application of a solid-phase microextraction-gas chromatography-mass spectrometry/metal oxide sensor system for detection of antibiotic susceptibility in urinary tract infection-causing Escherichia coli–a proof of principle study. Adv. Med. Sci. 67, 1–9. doi: 10.1016/j.advms.2021.09.001
Edwards, J., Johnson, C., Santos-Medellín, C., Lurie, E., Podishetty, N. K., Bhatnagar, S., et al. (2015). Structure, variation, and assembly of the root-associated microbiomes of rice. Proc. Natl. Acad. Sci. 112, 911–920. doi: 10.1073/pnas.1414592112
Elhaissoufi, W., Ghoulam, C., Barakat, A., Zeroual, Y., and Bargaz, A. (2021). Phosphate bacterial solubilization: a key rhizosphere driving force enabling higher P use efficiency and crop productivity. J. Adv. Res. 38, 13–28. doi: 10.1016/j.jare.2021.08.014
Elnahal, A. S., El-Saadony, M. T., Saad, A. M., Desoky, E.-S. M., El-Tahan, A. M., Rady, M. M., et al. (2022). The use of microbial inoculants for biological control, plant growth promotion, and sustainable agriculture: a review. Eur. J. Plant Pathol. 162, 1–34. doi: 10.1007/s10658-021-02393-7
Etesami, H. (2020). “Enhanced phosphorus fertilizer use efficiency with microorganisms” in Nutrient dynamics for sustainable crop production (Singapore: Springer), 215–245.
Fei, N., Ji, W., Yang, L., Yu, C., Qiao, P., Yan, J., et al. (2022). Hcp of the type VI secretion system (T6SS) in Acidovorax citrulli group II strain Aac5 has a dual role as a core structural protein and an effector protein in colonization, growth ability, competition, biofilm formation, and ferric iron absorption. Int. J. Mol. Sci. 23:9632. doi: 10.3390/ijms23179632
Feng, H., Fu, R., Hou, X., Lv, Y., Zhang, N., Liu, Y., et al. (2021). Chemotaxis of beneficial rhizobacteria to root exudates: the first step towards root–microbe rhizosphere interactions. Int. J. Mol. Sci. 22:6655. doi: 10.3390/ijms22136655
Feng, D., Gao, Q., Liu, J., Tang, J., Hua, Z., and Sun, X. (2023). Categories of exogenous substances and their effect on alleviation of plant salt stress. Eur. J. Agron. 142:126656. doi: 10.1016/j.eja.2022.126656
Gain, P., Mannan, M., Pal, P., Hossain, M. M., and Parvin, S. (2004). Effect of salinity on some yield attributes of rice. Pak. J. Biol. Sci. 7, 760–762. doi: 10.3923/pjbs.2004.760.762
Gallique, M., Decoin, V., Barbey, C., Rosay, T., Feuilloley, M. G., Orange, N., et al. (2017). Contribution of the Pseudomonas fluorescens MFE01 type VI secretion system to biofilm formation. PLoS One 12:e0170770. doi: 10.1371/journal.pone.0170770
Ghosh, S., Bhagwat, T., and Webster, T. J. (2021). “Endophytic microbiomes and their plant growth-promoting attributes for plant health” in Current trends in microbial biotechnology for sustainable agriculture (Singapore: Springer), 245–278.
Giron, D., Frago, E., Glevarec, G., Pieterse, C. M., and Dicke, M. (2013). Cytokinins as key regulators in plant-microbe-insect interactions: connecting plant growth and defence. Funct. Ecol. 27, 599–609. doi: 10.1111/1365-2435.12042
Glick, B. R. (2012). Plant growth-promoting bacteria: mechanisms and applications. Scientifica 2012, 1–15. doi: 10.6064/2012/963401
Glick, B. R. (2014). Bacteria with ACC deaminase can promote plant growth and help to feed the world. Microbiol. Res. 169, 30–39. doi: 10.1016/j.micres.2013.09.009
Gottel, N. R., Castro, H. F., Kerley, M., Yang, Z., Pelletier, D. A., Podar, M., et al. (2011). Distinct microbial communities within the endosphere and rhizosphere of Populus deltoides roots across contrasting soil types. Appl. Environ. Microbiol. 77, 5934–5944. doi: 10.1128/AEM.05255-11
Greco, V., Sciuto, S., and Rizzarelli, E. (2021). Mono-and dialdehyde of trehalose: new synthons to prepare trehalose bio-conjugates. Org. Biomol. Chem. 19, 9427–9432. doi: 10.1039/D1OB01816G
Green, E. R., and Mecsas, J. (2016). Bacterial secretion systems: an overview. Virulence Mech. Bacterial Pathog., 4, 213–239. doi: 10.1128/9781555819286.ch8
Gritli, T., Boubakri, H., Essahibi, A., Hsouna, J., Ilahi, H., Didier, R., et al. (2022). Salt stress mitigation in Lathyrus cicera by combining different microbial inocula. Physiol. Mol. Biol. Plants 28, 1191–1206. doi: 10.1007/s12298-022-01205-4
Gu, S., Wei, Z., Shao, Z., Friman, V.-P., Cao, K., Yang, T., et al. (2020). Competition for iron drives phytopathogen control by natural rhizosphere microbiomes. Nat. Microbiol. 5, 1002–1010. doi: 10.1038/s41564-020-0719-8
Han, Q., Ma, Q., Chen, Y., Tian, B., Xu, L., Bai, Y., et al. (2020). Variation in rhizosphere microbial communities and its association with the symbiotic efficiency of rhizobia in soybean. ISME J. 14, 1915–1928. doi: 10.1038/s41396-020-0648-9
Hardoim, P. R., Hardoim, C. C., Van Overbeek, L. S., and Van Elsas, J. D. (2012). Dynamics of seed-borne rice endophytes on early plant growth stages. PLoS One 7:e30438. doi: 10.1371/journal.pone.0030438
Haroon, U., Khizar, M., Liaquat, F., Ali, M., Akbar, M., Tahir, K., et al. (2021). Halotolerant plant growth-promoting rhizobacteria induce salinity tolerance in wheat by enhancing the expression of SOS genes. J. Plant Growth Regul. 41, 1–14. doi: 10.1007/s00344-021-10457-5
Ha-Tran, D. M., Nguyen, T. T. M., Hung, S.-H., Huang, E., and Huang, C.-C. (2021). Roles of plant growth-promoting rhizobacteria (PGPR) in stimulating salinity stress defense in plants: a review. Int. J. Mol. Sci. 22:3154. doi: 10.3390/ijms22063154
Hoque, M., Hannan, A., Imran, S., Paul, N. C., Mondal, M., Sadhin, M., et al. (2022). Plant growth-promoting Rhizobacteria-mediated adaptive responses of plants under salinity stress. J. Plant Growth Regul., 42, 1–20. doi: 10.1007/s00344-022-10633-1
Hossain, M. T., and Chung, Y. R. (2019). “Endophytic bacillus species induce systemic resistance to plant diseases” in Bacilli and Agrobiotechnology: Phytostimulation and biocontrol (Singapore: Springer), 151–160.
Huang, X.-F., Chaparro, J. M., Reardon, K. F., Zhang, R., Shen, Q., and Vivanco, J. M. (2014). Rhizosphere interactions: root exudates, microbes, and microbial communities. Botany 92, 267–275. doi: 10.1139/cjb-2013-0225
Ilangumaran, G., and Smith, D. L. (2017). Plant growth promoting rhizobacteria in amelioration of salinity stress: a systems biology perspective. Front. Plant Sci. 8:1768. doi: 10.3389/fpls.2017.01768
Islam, F., Yasmeen, T., Ali, S., Ali, B., Farooq, M. A., and Gill, R. A. (2015). Priming-induced antioxidative responses in two wheat cultivars under saline stress. Acta Physiol. Plant. 37:153. doi: 10.1007/s11738-015-1897-5
Ismail, A. M., and Horie, T. (2017). Genomics, physiology, and molecular breeding approaches for improving salt tolerance. Annu. Rev. Plant Biol. 68, 405–434. doi: 10.1146/annurev-arplant-042916-040936
Jiang, X., Beust, A., Sappa, P. K., Völker, U., Dinse, T., Herglotz, J., et al. (2019). Two functionally deviating type 6 secretion systems occur in the nitrogen-fixing endophyte Azoarcus olearius BH72. Front. Microbiol. 10:459. doi: 10.3389/fmicb.2019.00459
Junker, R. R., and Keller, A. (2015). Microhabitat heterogeneity across leaves and flower organs promotes bacterial diversity. FEMS Microbiol. Ecol. 91, 97–104. doi: 10.1093/femsec/fiv097
Kaleh, A. M., Singh, P., Mazumdar, P., Chua, K. O., and Harikrishna, J. A. (2022). Halotolerant rhizobacteria isolated from a mangrove forest alleviate saline stress in Musa acuminata cv. Berangan. Microbiol. Res. 265:127176. doi: 10.1016/j.micres.2022.127176
Kan, X., Ren, J., Chen, T., Cui, M., Li, C., Zhou, R., et al. (2017). Effects of salinity on photosynthesis in maize probed by prompt fluorescence, delayed fluorescence and P700 signals. Environ. Exp. Bot. 140, 56–64. doi: 10.1016/j.envexpbot.2017.05.019
Kembel, S. W., O’Connor, T. K., Arnold, H. K., Hubbell, S. P., Wright, S. J., and Green, J. L. (2014). Relationships between phyllosphere bacterial communities and plant functional traits in a neotropical forest. Proc. Natl. Acad. Sci. 111, 13715–13720. doi: 10.1073/pnas.1216057111
Khabbaz, S. E., Ladhalakshmi, D., Babu, M., Kandan, A., Ramamoorthy, V., Saravanakumar, D., et al. (2019). Plant growth promoting bacteria (PGPB)—a versatile tool for plant health management. Can. J. Pesticides Pest Manag. 1, 1–25. doi: 10.34195/can.j.ppm.2019.05.001
Khan, N., Bano, A., Ali, S., and Babar, M. (2020). Crosstalk amongst phytohormones from planta and PGPR under biotic and abiotic stresses. Plant Growth Regul. 90, 189–203. doi: 10.1007/s10725-020-00571-x
Khatoon, Z., Huang, S., Farooq, M. A., Santoyo, G., Rafique, M., Javed, S., et al. (2022). Role of plant growth-promoting bacteria (PGPB) in abiotic stress management. in Mitigation Plant Abiotic Stress Microorgan (USA: Academic press), 257–272.
Kim, N., Kim, J. J., Kim, I., Mannaa, M., Park, J., Kim, J., et al. (2020). Type VI secretion systems of plant-pathogenic Burkholderia glumae BGR1 play a functionally distinct role in interspecies interactions and virulence. Mol. Plant Pathol. 21, 1055–1069. doi: 10.1111/mpp.12966
Knauth, S., Hurek, T., Brar, D., and Reinhold-Hurek, B. (2005). Influence of different Oryza cultivars on expression of nifH gene pools in roots of rice. Environ. Microbiol. 7, 1725–1733. doi: 10.1111/j.1462-2920.2005.00841.x
Kong, W., Xu, X., Li, Z., Wang, Y., and Wu, X. (2022). Combining ectomycorrhizal fungi and plant growth-promoting rhizobacteria to enhance salt tolerance of Metasequoia glyptostroboides. J. For. Res. doi: 10.1007/s11676-022-01578-y
Kumar, A., Singh, S., Gaurav, A. K., Srivastava, S., and Verma, J. P. (2020). Plant growth-promoting bacteria: biological tools for the mitigation of salinity stress in plants. Front. Microbiol. 11:1216. doi: 10.3389/fmicb.2020.01216
Kumar, A., Singh, S., Mukherjee, A., Rastogi, R. P., and Verma, J. P. (2021). Salt-tolerant plant growth-promoting Bacillus pumilus strain JPVS11 to enhance plant growth attributes of rice and improve soil health under salinity stress. Microbiol. Res. 242:126616. doi: 10.1016/j.micres.2020.126616
Kurepin, L. V., Park, J. M., Lazarovits, G., and Bernards, M. A. (2015). Burkholderia phytofirmans-induced shoot and root growth promotion is associated with endogenous changes in plant growth hormone levels. Plant Growth Regul. 75, 199–207. doi: 10.1007/s10725-014-9944-6
Laghmouchi, Y., Belmehdi, O., Bouyahya, A., Senhaji, N. S., and Abrini, J. (2017). Effect of temperature, salt stress and pH on seed germination of medicinal plant Origanum compactum. Biocatal. Agricult. Biotechnol. 10, 156–160. doi: 10.1016/j.bcab.2017.03.002
Leff, J. W., Del Tredici, P., Friedman, W. E., and Fierer, N. (2015). Spatial structuring of bacterial communities within individual Ginkgo biloba trees. Environ. Microbiol. 17, 2352–2361. doi: 10.1111/1462-2920.12695
Li, Q., Huang, Z., Deng, C., Lin, K.-H., Hua, S., and Chen, S.-P. (2022). Endophytic Klebsiella sp. San01 promotes growth performance and induces salinity and drought tolerance in sweet potato (Ipomoea batatas L.). J. Plant Interact. 17, 608–619. doi: 10.1080/17429145.2022.2077464
Li, Y., Sun, D., Xu, K., Jin, L., and Peng, R. (2021). Hydrogen sulfide enhances plant tolerance to waterlogging stress. Plan. Theory 10:1928. doi: 10.3390/plants10091928
Lin, H.-H., Huang, H.-M., Yu, M., Lai, E.-M., Chien, H.-L., and Liu, C.-T. (2018). Functional exploration of the bacterial type VI secretion system in mutualism: Azorhizobium caulinodans ORS571–Sesbania rostrata as a research model. Mol. Plant-Microbe Interact. 31, 856–867. doi: 10.1094/MPMI-01-18-0026-R
Lobo, L. L. B., da Silva, M. S. R. D. A., Carvalho, R. F., and Rigobelo, E. C. (2023). The negative effect of coinoculation of plant growth-promoting bacteria is not related to indole-3-acetic acid synthesis. J. Plant Growth Regul., 42, 2317–2326. doi: 10.1007/s00344-022-10706-1
Lodhi, A., Sajjad, M. H., Mahmood, A., Tahir, S., and Azam, F. (2009). Photosynthate partitioning in wheat (Triticum aestivum L.) as affected by root zone salinity and form of N. Pak. J. Bot. 41, 1363–1372.
Lucero, C. T., Lorda, G. S., Ludueña, L. M., Nievas, F., Bogino, P. C., Angelini, J., et al. (2022). Participation of type VI secretion system in plant colonization of phosphate solubilizing bacteria. Rhizosphere 24:100582. doi: 10.1016/j.rhisph.2022.100582
Lundberg, D. S., Lebeis, S. L., Paredes, S. H., Yourstone, S., Gehring, J., Malfatti, S., et al. (2012). Defining the core Arabidopsis thaliana root microbiome. Nature 488, 86–90. doi: 10.1038/nature11237
Malik, G., and Arora, R. (2022). “Endophytic bacteria: mitigating abiotic stress from inside” in Bacterial endophytes for sustainable agriculture and environmental management (Singapore: Springer), 23–43.
Matthews, A., Pierce, S., and Raymond, B. (2019). Rhizobacterial community assembly patterns vary between crop species. Front. Microbiol. 10:581. doi: 10.3389/fmicb.2019.00581
Meena, M., Swapnil, P., Divyanshu, K., Kumar, S., Tripathi, Y. N., Zehra, A., et al. (2020). PGPR-mediated induction of systemic resistance and physiochemical alterations in plants against the pathogens: current perspectives. J. Basic Microbiol. 60, 828–861. doi: 10.1002/jobm.202000370
Meza, C., Valenzuela, F., Echeverría-Vega, A., Gomez, A., Sarkar, S., Cabeza, R. A., et al. (2022). Plant-growth-promoting bacteria from rhizosphere of Chilean common bean ecotype (Phaseolus vulgaris L.) supporting seed germination and growth against salinity stress. Front. Plant Sci. 13:1052263. doi: 10.3389/fpls.2022.1052263
Miliute, I., Buzaite, O., Baniulis, D., and Stanys, V. (2015). Bacterial endophytes in agricultural crops and their role in stress tolerance: a review. Zemdirbyste Agricult. 102, 465–478. doi: 10.13080/z-a.2015.102.060
Moon, Y.-S., Khan, M., Khan, M. A., and Ali, S. (2023). Ameliorative symbiosis of Serratia fonticola (S1T1) under salt stress condition enhance growth-promoting attributes of Cucumis sativus L. Symbiosis 89, 283–297. doi: 10.1007/s13199-023-00897-w
Mosquito, S., Bertani, I., Licastro, D., Compant, S., Myers, M. P., Hinarejos, E., et al. (2020). In planta colonization and role of T6SS in two rice Kosakonia endophytes. Mol. Plant-Microbe Interact. 33, 349–363. doi: 10.1094/MPMI-09-19-0256-R
Mousavi, S. S., Karami, A., Saharkhiz, M. J., Etemadi, M., and Ravanbakhsh, M. (2022). Microbial amelioration of salinity stress in endangered accessions of Iranian licorice (Glycyrrhiza glabra L.). BMC Plant Biol. 22, 1–17. doi: 10.1186/s12870-022-03703-9
Müller, D. B., Vogel, C., Bai, Y., and Vorholt, J. A. (2016). The plant microbiota: systems-level insights and perspectives. Annu. Rev. Genet. 50, 211–234. doi: 10.1146/annurev-genet-120215-034952
Munir, N., Hanif, M., Abideen, Z., Sohail, M., El-Keblawy, A., Radicetti, E., et al. (2022). Mechanisms and strategies of plant microbiome interactions to mitigate abiotic stresses. Agronomy 12:2069. doi: 10.3390/agronomy12092069
Naama-Amar, A., Gerchman, Y., Iasur Kruh, L., and Naor, V. (2022). Evaluation of the biocontrol activity of Frateuria defendens-derived metabolites against mollicutes. Plant Signal. Behav. 17:2070355. doi: 10.1080/15592324.2022.2070355
Nelson, E. B. (2018). The seed microbiome: origins, interactions, and impacts. Plant Soil 422, 7–34. doi: 10.1007/s11104-017-3289-7
Neshat, M., Abbasi, A., Hosseinzadeh, A., Sarikhani, M. R., Dadashi Chavan, D., and Rasoulnia, A. (2022). Plant growth promoting bacteria (PGPR) induce antioxidant tolerance against salinity stress through biochemical and physiological mechanisms. Physiol. Mol. Biol. Plants 28, 347–361. doi: 10.1007/s12298-022-01128-0
Nigam, B., Dubey, R. S., and Rathore, D. (2022). Protective role of exogenously supplied salicylic acid and PGPB (Stenotrophomonas sp.) on spinach and soybean cultivars grown under salt stress. Sci. Hortic. 293:110654. doi: 10.1016/j.scienta.2021.110654
Niu, B., Paulson, J. N., Zheng, X., and Kolter, R. (2017). Simplified and representative bacterial community of maize roots. Proc. Natl. Acad. Sci. 114, 2450–2459. doi: 10.1073/pnas.1616148114
Niu, B., Wang, W., Yuan, Z., Sederoff, R. R., Sederoff, H., Chiang, V. L., et al. (2020). Microbial interactions within multiple-strain biological control agents impact soil-borne plant disease. Front. Microbiol. 11:585404. doi: 10.3389/fmicb.2020.585404
Olanrewaju, O. S., Glick, B. R., and Babalola, O. O. (2017). Mechanisms of action of plant growth promoting bacteria. World J. Microbiol. Biotechnol. 33, 197–116. doi: 10.1007/s11274-017-2364-9
Orozco-Mosqueda, M. D. C., Duan, J., DiBernardo, M., Zetter, E., Campos-García, J., Glick, B. R., et al. (2019). The production of ACC deaminase and trehalose by the plant growth promoting bacterium pseudomonas sp. UW4 synergistically protect tomato plants against salt stress. Front. Microbiol. 10:1392. doi: 10.3389/fmicb.2019.01392
Pande, A., Kaushik, S., Pandey, P., and Negi, A. (2019). Isolation, characterization, and identification of phosphate-solubilizing Burkholderia cepacia from the sweet corn cv. Golden bantam rhizosphere soil and effect on growth-promoting activities. Int. J. Veg. Sci. 26, 591–607. doi: 10.1080/19315260.2019.1692121
Patel, P., Gajjar, H., Joshi, B., Krishnamurthy, R., and Amaresan, N. (2022). Inoculation of salt-tolerant Acinetobacter sp (RSC9) improves the sugarcane (Saccharum sp. hybrids) growth under salinity stress condition. Sugar Tech. 24, 494–501. doi: 10.1007/s12355-021-01043-w
Patel, M., Vurukonda, S., and Patel, A. (2023). Multi-trait halotolerant plant growth-promoting bacteria mitigate induced salt stress and enhance growth of Amaranthus viridis. J. Soil Sci. Plant Nutr. 23, 1860–1883. doi: 10.1007/s42729-023-01143-4
Prittesh, P., Avnika, P., Kinjal, P., Jinal, H. N., Sakthivel, K., and Amaresan, N. (2020). Amelioration effect of salt-tolerant plant growth-promoting bacteria on growth and physiological properties of rice (Oryza sativa) under salt-stressed conditions. Arch. Microbiol. 202, 2419–2428. doi: 10.1007/s00203-020-01962-4
Raina, J. B., Fernandez, V., Lambert, B., Stocker, R., and Seymour, J. R. (2019). The role of microbial motility and chemotaxis in symbiosis. Nat. Rev. Microbiol. 17, 284–294. doi: 10.1038/s41579-019-0182-9
Raza, W., Ling, N., Yang, L., Huang, Q., and Shen, Q. (2016). Response of tomato wilt pathogen Ralstonia solanacearum to the volatile organic compounds produced by a biocontrol strain Bacillus amyloliquefaciens SQR-9. Sci. Rep. 6, 1–13. doi: 10.1038/srep24856
Rebi, A., Kashif, H. M., Chaudhry, U. F., Zaib, M., Shahid, M. Z., Safdar, M., et al. (2022). Phosphorus availability in soil and uptake by maize from rock phosphate inoculated with PGPR: a review. Nat. Essent. Oils J. NVEO, 9, 341–355.
Reinhold-Hurek, B., Bünger, W., Burbano, C. S., Sabale, M., and Hurek, T. (2015). Roots shaping their microbiome: global hotspots for microbial activity. Annu. Rev. Phytopathol. 53, 403–424. doi: 10.1146/annurev-phyto-082712-102342
Rilling, J., Acuña, J., Nannipieri, P., Cassan, F., Maruyama, F., and Jorquera, M. (2019). Current opinion and perspectives on the methods for tracking and monitoring plant growth–promoting bacteria. Soil Biol. Biochem. 130, 205–219. doi: 10.1016/j.soilbio.2018.12.012
Rivero, R. M., Kojima, M., Gepstein, A., Sakakibara, H., Mittler, R., Gepstein, S., et al. (2007). Delayed leaf senescence induces extreme drought tolerance in a flowering plant. Proc. Natl. Acad. Sci. 104, 19631–19636. doi: 10.1073/pnas.0709453104
Romero-Munar, A., and Aroca, R. (2023). A non-K+-solubilizing PGPB (Bacillus megaterium) increased K+ deprivation tolerance in Oryza sativa seedlings by up-regulating root K+ transporters. Plant Physiol. Biochem. 196, 774–782. doi: 10.1016/j.plaphy.2023.02.027
Russell, A. B., Peterson, S. B., and Mougous, J. D. (2014). Type VI secretion system effectors: poisons with a purpose. Nat. Rev. Microbiol. 12, 137–148. doi: 10.1038/nrmicro3185
Salinero-Lanzarote, A., Pacheco-Moreno, A., Domingo-Serrano, L., Durán, D., Ormeño-Orrillo, E., Martínez-Romero, E., et al. (2019). The type VI secretion system of Rhizobium etli Mim1 has a positive effect in symbiosis. FEMS Microbiol. Ecol. 95:fiz054. doi: 10.1093/femsec/fiz054
Sánchez-García, E. A., Rodríguez-Medina, K., and Moreno-Casasola, P. (2017). Effects of soil saturation and salinity on seed germination in seven freshwater marsh species from the tropical coast of the Gulf of Mexico. Aquat. Bot. 140, 4–12. doi: 10.1016/j.aquabot.2017.04.002
Santoyo, G., Moreno-Hagelsieb, G., del Carmen Orozco-Mosqueda, M., and Glick, B. R. (2016). Plant growth-promoting bacterial endophytes. Microbiol. Res. 183, 92–99. doi: 10.1016/j.micres.2015.11.008
Sapre, S., Gontia-Mishra, I., and Tiwari, S. (2022). Plant growth-promoting Rhizobacteria ameliorates salinity stress in pea (Pisum sativum). J. Plant Growth Regul. 41, 647–656. doi: 10.1007/s00344-021-10329-y
Saravanakumar, D., Thomas, A., and Banwarie, N. (2019). Antagonistic potential of lipopeptide producing Bacillus amyloliquefaciens against major vegetable pathogens. Eur. J. Plant Pathol. 154, 319–335. doi: 10.1007/s10658-018-01658-y
Sardar, M. F., Abbas, T., Naveed, M., Siddique, S., Mustafa, A., Abbasi, B., et al. (2021). “Biopesticides: importance and challenges” in Pesticide contamination in freshwater and soil environs (USA: Apple Academic Press), 129–151.
Selari, P. J. R. G., Tsui, S., de Almeida, T. T., Olchanheski, L. R., and Dourado, M. N. (2023). Biological control of Phytopathogenic fungi: mechanisms and potentials. Agricult. Biocatal. (Singapore: Jenny Stanford Publishing) 3–39.
Shirokov, Y., and Tikhnenko, V. (2021). "Analysis of environmental problems of crop production and ways to solve them", in: E3S Web of Conferences: EDP Sciences), 01025, 273, doi: 10.1051/e3sconf/202127301025
Singh, G., and Mukerji, K. G. (2006). “Root exudates as determinant of rhizospheric microbial biodiversity” in Microbial activity in the rhizoshere (Singapore: Springer), 39–53.
Steele, M. I., Kwong, W. K., Whiteley, M., and Moran, N. A. (2017). Diversification of type VI secretion system toxins reveals ancient antagonism among bee gut microbes. mBio 8, e01630–e01617. doi: 10.1128/mBio.01630-17
Stegelmeier, A. A., Rose, D. M., Joris, B. R., and Glick, B. R. (2022). The use of PGPB to promote plant hydroponic growth. Plan. Theory 11:2783. doi: 10.3390/plants11202783
Suárez, R., Wong, A., Ramírez, M., Barraza, A., Orozco, M. C., Cevallos, M. A., et al. (2008). Improvement of drought tolerance and grain yield in common bean by overexpressing trehalose-6-phosphate synthase in rhizobia. Mol. Plant-Microbe Interact. 21, 958–966. doi: 10.1094/MPMI-21-7-0958
Tabbal, S., El Aroussi, B., Bouchard, M., Marchand, G., and Haddad, S. (2022). A new headspace solid-phase microextraction coupled with gas chromatography-tandem mass spectrometry method for the simultaneous quantification of 21 microbial volatile organic compounds in urine and blood. Chemosphere 296:133901. doi: 10.1016/j.chemosphere.2022.133901
Tian, H., Lv, B., Ding, T., Bai, M., and Ding, Z. (2018). Auxin-BR interaction regulates plant growth and development. Front. Plant Sci. 8:2256. doi: 10.3389/fpls.2017.02256
Trivedi, P., Batista, B. D., Bazany, K. E., and Singh, B. K. (2022). Plant–microbiome interactions under a changing world: responses, consequences and perspectives. New Phytol. 234, 1951–1959. doi: 10.1111/nph.18016
Trivedi, P., Leach, J. E., Tringe, S. G., Sa, T., and Singh, B. K. (2020). Plant–microbiome interactions: from community assembly to plant health. Nat. Rev. Microbiol. 18, 607–621. doi: 10.1038/s41579-020-0412-1
Upadhyaya, D. C., Bagri, D. S., Upadhyaya, C. P., Kumar, A., Thiruvengadam, M., and Jain, S. K. (2021). Genetic engineering of potato (Solanum tuberosum L.) for enhanced α-tocopherols and abiotic stress tolerance. Physiol. Plant. 173, 116–128. doi: 10.1111/ppl.13252
Valdameri, G., Alberton, D., Moure, V. R., Kokot, T. B., Kukolj, C., Brusamarello-Santos, L. C. C., et al. (2017). Herbaspirillum rubrisubalbicans, a mild pathogen impairs growth of rice by augmenting ethylene levels. Plant Mol. Biol. 94, 625–640. doi: 10.1007/s11103-017-0629-1
Van Zelm, E., Zhang, Y., and Testerink, C. (2020). Salt tolerance mechanisms of plants. Annu. Rev. Plant Biol. 71, 403–433. doi: 10.1146/annurev-arplant-050718-100005
Vinciguerra, D., Gelb, M. B., and Maynard, H. D. (2022). Synthesis and application of Trehalose materials. JACS Au 2, 1561–1587. doi: 10.1021/jacsau.2c00309
Walia, A., Guleria, S., Chauhan, A., and Mehta, P. (2017). Endophytic bacteria: role in phosphate solubilization. Endophytes: Crop Product. Protect., 16, 61–93. doi: 10.1007/978-3-319-66544-3_4
Wu, X., Li, W., Li, C., Yin, J., Wu, T., Zhang, M., et al. (2022). Discrimination and characterization of the volatile organic compounds of Acori tatarinowii rhizoma based on headspace-gas chromatography-ion mobility spectrometry and headspace solid phase microextraction-gas chromatography-mass spectrometry. Arab. J. Chem. 15:104231. doi: 10.1016/j.arabjc.2022.104231
Xiong, C., and Lu, Y. (2022). Microbiomes in agroecosystem: diversity, function and assembly mechanisms. Environ. Microbiol. Rep. 14, 833–849. doi: 10.1111/1758-2229.13126
Yadav, J., Mahawer, A. K., Prashantha, S., and Sagar, H. P. (2022). Plant growth-promoting Rhizobacteria (PGPRS) and their mechanisms of action. Biotica Res. Today 4, 103–105.
Yavaş, İ., and Hussain, S. (2022). Recent Progress on melatonin-induced salinity tolerance in plants: an overview. Turkish J. Agricult. Food Sci. Technol. 10, 1447–1454. doi: 10.24925/turjaf.v10i8.1447-1454.5125
Yeoh, Y. K., Paungfoo-Lonhienne, C., Dennis, P. G., Robinson, N., Ragan, M. A., Schmidt, S., et al. (2016). The core root microbiome of sugarcanes cultivated under varying nitrogen fertilizer application. Environ. Microbiol. 18, 1338–1351. doi: 10.1111/1462-2920.12925
Yin, Z., Wang, X., Hu, Y., Zhang, J., Li, H., Cui, Y., et al. (2022). Metabacillus dongyingensis sp. nov. is represented by the plant growth-promoting bacterium BY2G20 isolated from saline-alkaline soil and enhances the growth of Zea mays L. under salt stress. Msystems 7, e01426–e01421. doi: 10.1128/msystems.01426-21
Yu, L. Y., Huang, H. B., Wang, X. H., Li, S., Feng, N. X., Zhao, H. M., et al. (2019). Novel phosphate-solubilising bacteria isolated from sewage sludge and the mechanism of phosphate solubilisation. Sci. Total Environ. 658, 474–484. doi: 10.1016/j.scitotenv.2018.12.166
Zarraonaindia, I., Owens, S. M., Weisenhorn, P., West, K., Hampton-Marcell, J., Lax, S., et al. (2015). The soil microbiome influences grapevine-associated microbiota. mBio 6, e02527–e02514. doi: 10.1128/mBio.02527-14
Zhang, Y., Li, C., Yao, T., Li, M., Lan, X., and Wang, Z. (2022). Plant growth–promoting Rhizobacteria enhance salt tolerance in oat by upregulating the antioxidant system and promoting root growth. J. Plant Growth Regul. 42, 3568–3581. doi: 10.1007/s00344-022-10821-z
Zhu, J. K. (2001). Plant salt stress. Trends Plant Sci. 6, 66–71. doi: 10.1016/S1360-1385(00)01838-0
Zilaie, M. N., Arani, A. M., Etesami, H., Dinarvand, M., and Dolati, A. (2022). Halotolerant plant growth-promoting rhizobacteria-mediated alleviation of salinity and dust stress and improvement of forage yield in the desert halophyte Seidlitzia rosmarinus. Environ. Exp. Bot. 201:104952. doi: 10.1016/j.envexpbot.2022.104952
Keywords: plant growth-promoting bacteria, salinity stress, plant microbiome, Type VI secretion system, plant nutrients, phytohormones, osmolytes
Citation: Peng M, Jiang Z, Zhou F and Wang Z (2023) From salty to thriving: plant growth promoting bacteria as nature’s allies in overcoming salinity stress in plants. Front. Microbiol. 14:1169809. doi: 10.3389/fmicb.2023.1169809
Received: 20 February 2023; Accepted: 09 June 2023;
Published: 23 June 2023.
Edited by:
Yang Song, Utrecht University, NetherlandsReviewed by:
Sahil Mehta, University of Delhi, IndiaCopyright © 2023 Peng, Jiang, Zhou and Wang. This is an open-access article distributed under the terms of the Creative Commons Attribution License (CC BY). The use, distribution or reproduction in other forums is permitted, provided the original author(s) and the copyright owner(s) are credited and that the original publication in this journal is cited, in accordance with accepted academic practice. No use, distribution or reproduction is permitted which does not comply with these terms.
*Correspondence: Mu Peng, cGVuZ211MTAyNUBob3RtYWlsLmNvbQ==; Zhiyong Wang, d2FuZ3poaXlvbmdAaGJtenUuZWR1LmNu
†These authors have contributed equally to this work
Disclaimer: All claims expressed in this article are solely those of the authors and do not necessarily represent those of their affiliated organizations, or those of the publisher, the editors and the reviewers. Any product that may be evaluated in this article or claim that may be made by its manufacturer is not guaranteed or endorsed by the publisher.
Research integrity at Frontiers
Learn more about the work of our research integrity team to safeguard the quality of each article we publish.