- 1Microbiomes, Microbes and Informatics Group, School of Biosciences, Cardiff University, Cardiff, Wales, United Kingdom
- 2School of Earth and Environmental Sciences, Cardiff University, Cardiff, Wales, United Kingdom
- 3Aker BP ASA, Lysaker, Norway
- 4The Wales Research and Diagnostic Positron Emission Tomography Imaging Centre (PETIC), School of Medicine, Cardiff University, University Hospital of Wales, Cardiff, Wales, United Kingdom
The Gulf of Cádiz is a tectonically active continental margin with over sixty mud volcanoes (MV) documented, some associated with active methane (CH4) seepage. However, the role of prokaryotes in influencing this CH4 release is largely unknown. In two expeditions (MSM1-3 and JC10) seven Gulf of Cádiz MVs (Porto, Bonjardim, Carlos Ribeiro, Captain Arutyunov, Darwin, Meknes, and Mercator) were analyzed for microbial diversity, geochemistry, and methanogenic activity, plus substrate amended slurries also measured potential methanogenesis and anaerobic oxidation of methane (AOM). Prokaryotic populations and activities were variable in these MV sediments reflecting the geochemical heterogeneity within and between them. There were also marked differences between many MV and their reference sites. Overall direct cell numbers below the SMTZ (0.2–0.5 mbsf) were much lower than the general global depth distribution and equivalent to cell numbers from below 100 mbsf. Methanogenesis from methyl compounds, especially methylamine, were much higher than the usually dominant substrates H2/CO2 or acetate. Also, CH4 production occurred in 50% of methylated substrate slurries and only methylotrophic CH4 production occurred at all seven MV sites. These slurries were dominated by Methanococcoides methanogens (resulting in pure cultures), and prokaryotes found in other MV sediments. AOM occurred in some slurries, particularly, those from Captain Arutyunov, Mercator and Carlos Ribeiro MVs. Archaeal diversity at MV sites showed the presence of both methanogens and ANME (Methanosarcinales, Methanococcoides, and ANME-1) related sequences, and bacterial diversity was higher than archaeal diversity, dominated by members of the Atribacterota, Chloroflexota, Pseudomonadota, Planctomycetota, Bacillota, and Ca. “Aminicenantes.” Further work is essential to determine the full contribution of Gulf of Cádiz mud volcanoes to the global methane and carbon cycles.
Introduction
Submarine mud volcanoes (MV) are seafloor diapir structures that exhibit episodic extrusion of mud, fluids and gases (predominantly methane) from subsurface reservoirs into the overlying strata and water column (Milkov, 2000; Dimitrov, 2003; Joye, 2020). They are formed as a result of different geological processes acting at active, convergent plate boundaries and passive continental margins resulting in high pore fluid pressures, sediment instability and consequently mud and gas extrusion (Kopf, 2002). MVs are structurally diverse, ranging in shape from amorphous mud pies to conical structures, and in size from a few meters to kilometers in diameter, and can be hundreds of meters high (Dimitrov, 2002; Kopf, 2002). They are geochemically variable, differing in the range of hydrocarbons released (Joye, 2020), the source of methane (Nuzzo et al., 2009), salinity (Haffert et al., 2013), and have varying periods of quiescence that allow pelagic material to build up from sedimentation of the water column (Kopf, 2002; Palomino et al., 2016). Despite the mechanisms for MV formation being well known, the actual global distribution and number of submarine MVs is not, with documented occurrences >1,000 MVs (Kopf, 2003; Baloglanov et al., 2018) and suggestions that global numbers are far greater and could be between 103 and 105 MVs (Milkov, 2000). Such large numbers of submarine MVs represent a significant and largely under investigated source of carbon input into overlying sediments, deep ocean, water column and the atmosphere (Milkov, 2000; Kopf, 2002; Dimitrov, 2003; Sauter et al., 2006; Joye, 2020). Estimates of global methane emissions from MVs vary, but it is thought to be in the tens of teragrams of methane per year and may be as much as 10% of the total annual methane emission to the atmosphere (Judd, 2005; Wallmann et al., 2006; Etiope, 2009; Etiope et al., 2011; Mazzini and Etiope, 2017).
Most of the gas venting from submarine MVs is composed of thermogenic methane (Kopf, 2002), but a significant fraction is of mixed or of microbial origin (Mazzini and Etiope, 2017) produced in situ by methanogenic archaea. Methanogenesis is the terminal oxidation process in the anaerobic degradation of organic matter and methanogens can be divided into three metabolic groups based on substrates used: hydrogenotrophic methanogens using H2/CO2, acetoclastic methanogens utilizing acetate and methylotrophic methanogens using methylated compounds, such as methanol, methylamine and methyl sulphide (Liu and Whitman, 2008; Bueno de Mesquita et al., 2023). In marine sediments, it is generally thought that the dominant methanogenic process is the reduction of CO2 by H2 (e.g., Mah et al., 1977; Claypool and Kvenvolden, 1983; Whiticar et al., 1986; Parkes et al., 2007; Beulig et al., 2018), although some studies suggest that acetate is also an important substrate for methanogenesis in subsurface sediments (Crill and Martens, 1986; Fry et al., 2008). However, the dominant methanogenic process occurring within MV sediments remains relatively unknown with only a few studies having specifically investigated methanogenic populations (Lazar et al., 2011b, 2012; Maignien et al., 2013). The main focus of MV microbiological studies has been to investigate prokaryotic diversity and the role microbial communities play in the anaerobic oxidation of methane (AOM; e.g., Pancost et al., 2000; Martinez et al., 2006; Niemann et al., 2006a,b; Heijs et al., 2007; Pachiadaki et al., 2011; Ruff et al., 2019), and how bacteria provide energy to support micro- and macrofaunal ecosystems (e.g., Lösekann et al., 2008; Rodrigues et al., 2010; Coelho et al., 2016; Palomino et al., 2016). In marine sediments, the metabolic process of AOM has been proposed to be due to reversed methanogenesis coupled to the reduction of sulphate involving anaerobic methane-oxidizing archaea (ANME) and sulphate-reducing bacteria (SRB). ANME and SRB are thought to interact syntrophically forming microbial consortia that anaerobically oxidize methane with equimolar amounts of sulphate, yielding bicarbonate and sulphide (Knittel and Boetius, 2009). Sulphide can then be used to support chemosynthetic macrofauna that derive energy from sulphide oxidation by symbiotic bacteria (Stewart et al., 2005).
In this study we investigated a range of mud volcanoes from different sea water depths along a transect of the Gulf of Cádiz with the aim to understand in situ methanogen populations, their activity, substrate use and diversity in variable mud volcano habitats. Anaerobic sediment slurry enrichments were established from sediment samples taken at varying depths from seven MVs and incubated with a wide range of potential methanogenic substrates (acetate, benzoate, hexadecane, methanol, methylamine and H2/CO2) for more than 130 days, after which total methane production was analyzed. Methane producing enrichments were further analyzed for their dominant archaeal and bacterial community structure using 16S rRNA and methanogen-specific mcrA genes. In addition, geochemical, methanogenic activity, total cell numbers and microbial diversity data (16S rRNA genes) from MV sediments was also collected.
Materials and methods
Site description and sample collection
The Gulf of Cádiz (Figure 1) is located in the NE Atlantic Ocean, west of the Gibraltar Arc between the Iberian Peninsula and the Moroccan margin. The area has experienced a complex geological history related to plate tectonic interactions which have resulted in several episodes of rifting, compression and strike-slip motion due to the closure of the Tethys Ocean, the opening of the N Atlantic, and the African-Eurasian convergence since the Cenozoic (Maldonado et al., 1999). Due to this ongoing compression, rapidly deposited sediments have been dewatered progressively and formed many MVs and fluid escape structures (Díaz-del-Rio et al., 2003). Since their discovery in 1999 (Gardner, 2001) more than 60 MVs have now been identified (León et al., 2012), ranging from 800 to 3,500 m in diameter and some as high as 300 m above the seafloor. Most MVs in the area have been built up from episodes of mud-breccia flows (Medialdea et al., 2009) and show evidence of gas saturation, methane hydrate, hydrogen sulphide and the presence of chemosynthetic fauna (e.g., Somoza et al., 2003; Niemann et al., 2006a; Rodrigues et al., 2010, 2011).
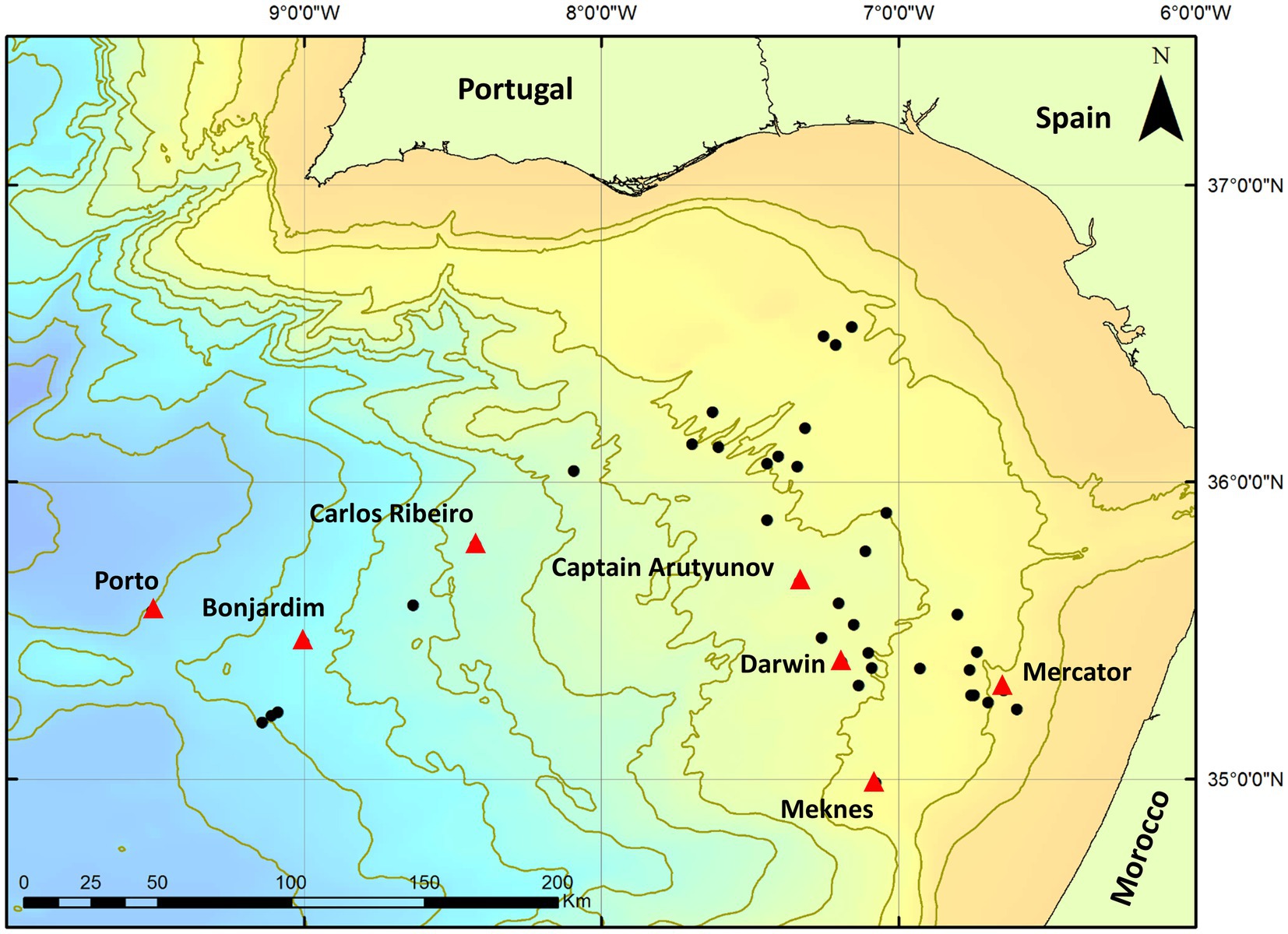
Figure 1. Location of Gulf of Cádiz mud volcanoes sampled during expeditions MSM1-3 and JC10. Red triangles indicate mud volcanoes used in this study. Black circles indicate other mud volcanoes located in the area.
Sediment cores were collected from seven mud volcanoes (Porto, Bonjardim, Carlos Ribeiro, Captain Arutyunov, Darwin, Meknes and Mercator MV) from the Gulf of Cádiz (Figure 1) during the EU HERMES project expedition cruises MSM1-3 on the Research Vessel Maria S. Merian (12th April – 19th May 2006) and JC10 (Leg 1) on the Royal Research Ship James Cook (14th May to 2nd June 2007) using a range of sampling methods and devices (Table 1). Additional sediment cores were also taken during Expedition JC10 from reference seafloor sites >1 km away from each MV sampled.
On board ship, sediment cores from each MV site were sampled aseptically at different depths (ranging from 0.01 to 5.25 mbsf; meters below seafloor; Table 1) using sterile pre-cut (luer-end removed) 50 ml volume syringes. All syringe mini-cores were then capped and sealed inside gas-tight aluminum bags under anoxic conditions with a nitrogen atmosphere and an Anaerocult A (Merck; Cragg et al., 1992), and stored at 4°C or frozen directly at −80°C. On board ship, further sediment samples were taken for cell counts, sediment pore water chemistry, gas analysis and activity measurements (only cruise JC-10). All mini-cores, samples for AODC and chemical analyses were then transported back to the UK for processing. Note 4°C stored samples were used for methanogen enrichments and chemical analysis, and −80°C samples for molecular analysis.
Acridine orange direct counts, chemical analysis, and prokaryotic activity measurements
Samples for AODC were fixed on board ship in filter-sterilized (0.2 μm) 4% (w/v) formaldehyde as described by Fry (1988). Preserved samples were then stained [0.1% (w/v) acridine orange] and counted on black polycarbonate membrane filters using a Zeiss Axioskop epifluorescence microscope.
Sediment for methane gas analysis was transferred on board ship into 20 ml volume serum vials with 10 ml of 10% (w/v) KCl, sealed and stored for equilibration. Headspace gas was analyzed using a Perkin Elmer/Arnel Clarus 500 natural gas analyzer with a flame ionization detector and a thermal conductivity detector. Methane and carbon dioxide concentrations were determined using a calibration with standard gases (Scott Specialty Gases). Pore waters were obtained from sediments on board ship using a pore-water squeezer as described (Wellsbury et al., 2002). Anions (including sulphate and acetate concentrations) were determined using an ICS-2000 Ion Chromatography System with two Ionpac AS15 columns in series and an Anion Self-Regenerating Suppressor (ASRS-ULTRA II 4 mm) in combination with a DS6 heated conductivity cell (Dionex UK Ltd.) as described (Webster et al., 2009). Cations (including methylamines) were analyzed using a DX-120 ion chromatograph (Dionex UK Ltd.) fitted with an IonPac CS16 column, a CSRS 300 4-mm suppressor, and a conductivity detector and methanesulfonic acid eluent (32 mM) at a flow rate of 0.75 ml min−1 (Watkins et al., 2012).
Radiotracer experiments using 14C-labelled substrates were carried out as previously described (Parkes et al., 2007, 2010). Briefly, sediment was subsampled using either Perspex mini-cores (diameter 2.0 cm, length 20 cm) with silicone-filled side injection holes (4 μl substrate per 1 cm depth interval) or 5-ml sterile pre-cut (luer-end removed) syringes closed with rubber stoppers (7.5 μl substrate per syringe). Samples were then preincubated in nitrogen-flushed gas-tight aluminum bags at 10°C to equilibrate for 24 h before radiotracer injection. Samples were injected with one of four 14C-substrates in batches of four (one control and three measurements) using either 14C-sodium bicarbonate (9.90 KBq μl−1), 14C-sodium acetate (6.60 KBq μl−1), 14C-methanol (2.05 KBq μl−1) or 14C-methylamine (7.36 KBq μl−1) and incubated at in situ bottom water temperature for 7 to 24 h in nitrogen-flushed gas-tight aluminum bags. Microbial reactions were stopped by transferring the incubated sediment into 30 ml glass serum vials containing 7 ml of 1 M NaOH and sealed. All samples were stored inverted at room temperature prior to processing. For 14C-CH4 analysis, samples were magnetically stirred whilst the headspace gas was flushed for 20 min with 5% O2: 95% N2 and passed over copper oxide at 900°C to convert 14C-CH4 to 14C-CO2. Flushed gases were bubbled through a series of three scintillation vials of 10 ml of Hi-Safe 3 scintillation cocktail (Canberra-Packard) containing 7% (v/v) ß-phenethylamine to absorb any 14C-CO2. Scintillation vials were counted in a scintillation counter and label turnover rates and potential activity rates calculated as described (Parkes et al., 2010). Methane production rates were calculated based on the proportion of labelled gas produced from the 14C-substrate, the dissolved pore water substrate or total CO2 concentration adjusted for sediment porosity, and the incubation time. For methylamine methanogenesis, rates were obtained by multiplying the substrate turnover value by an assumed methylamine concentration of 5 μM (as methylamine concentration in pore water was consistently below detection limit of approx. 5 μM), as previously used for measuring methylamine methanogenesis in Gulf of Cádiz MVs (Maignien et al., 2013). Therefore, methylamine methanogenesis rates should be considered as maximum potential rates assuming in situ concentration was the detection limit.
Methanogen enrichment slurries
Cold-stored (4°C) sediment mini-cores from each MV were then further sub-sampled under aseptic conditions in an anaerobic chamber and used as inoculum for sediment slurry enrichments. Replicate slurries (50 ml) were made up using 25% (v/v) sediment (see Table 1 for sediment depths used) in artificial seawater (Köpke et al., 2005) contained in 100 ml serum bottles and supplemented with the substrates 12 mM sodium acetate, 1.5 mM benzoic acid, 4 mM hexadecane, 5 mM methanol, or 5 mM methylamine. Additionally, one set of sediment slurries were left without supplements as an unamended control and another set of slurries had their headspace gas replaced with H2/CO2 (80:20) as substrates. All slurries, except those with H2/CO2 had their headspace gas replaced with N2/CO2 and all methanogen enrichments were then incubated at 10°C in the dark for up to 170 days (cruise MSM1-3) or 300 days (cruise JC10).
At several time points during the incubation, samples of headspace gas (2 ml) were removed from all sediment slurries and analyzed by gas chromatography as above. For standardization, potential methanogenesis (methane production) rates were calculated from total methane produced after 130 days incubation (or in some samples after 300 days) with the methane production value from the relevant unamended control subtracted (32 out of 40 control slurries produced methane; Table 2).
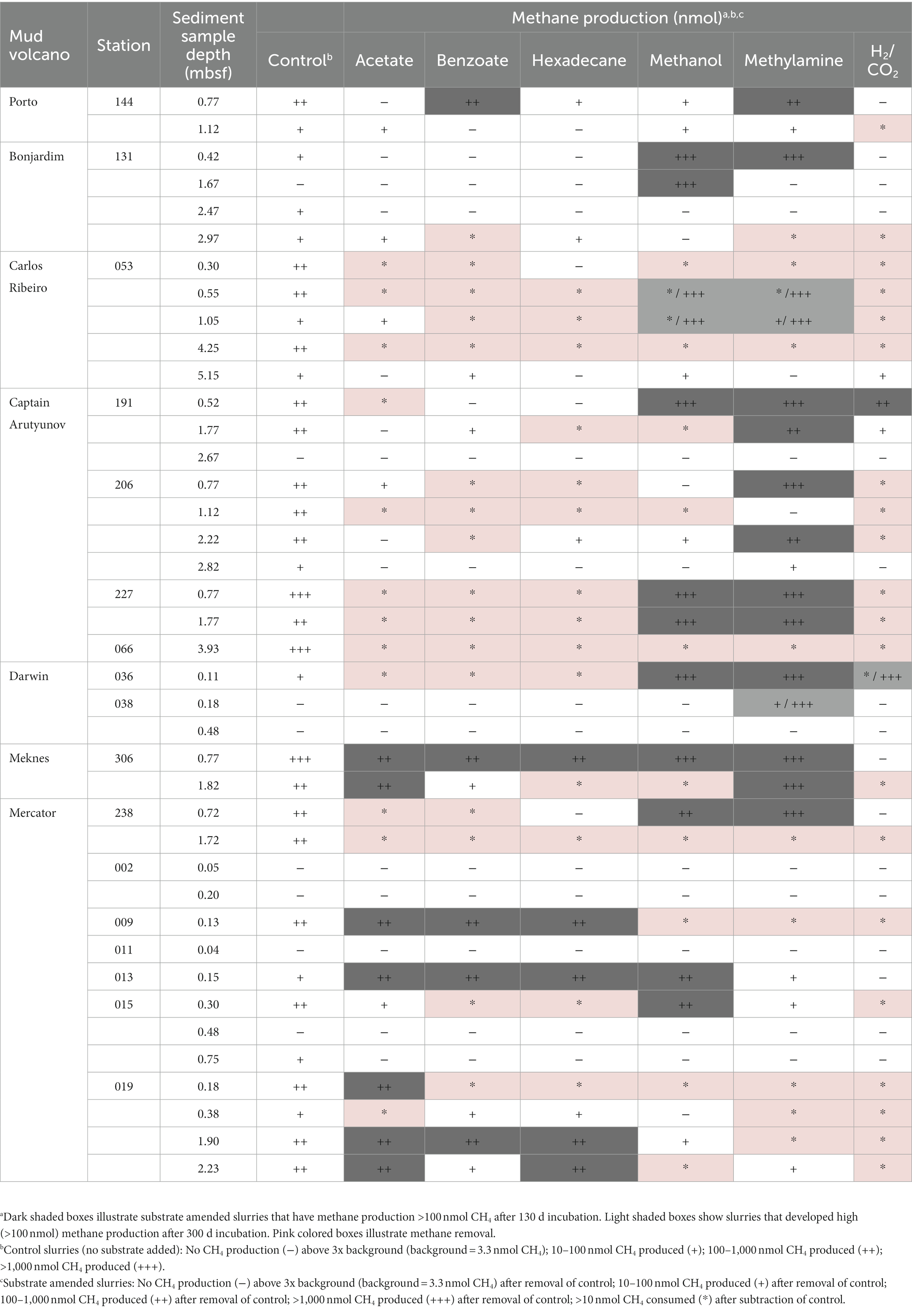
Table 2. Methane production from Gulf of Cádiz mud volcano sediment slurries incubated for 130 days with a range of substrates.
DNA extraction of methanogen enrichments
Genomic DNA was extracted from methanogenic sediment slurry samples using the FastDNA® SPIN Kit for Soil (MP Biomedicals) as described (Webster et al., 2003). Essentially, 1 ml of sediment slurry was placed in a lysing matrix E tube (MP Biomedicals) and centrifuged at 15,000 × g for 1 min to pellet cells and sediment. Sediment pellets were then re-suspended in 800 μl of sodium phosphate buffer and 120 μl MT buffer (MP Biomedicals) before lysis in a FastPrep® 24 instrument (MP Biomedicals) for 2 X 30s at speed 5.5 ms−1. All remaining steps were as the manufacturer’s protocol, except that some spin and incubation times were extended (see Parkes et al., 2010). DNA was eluted in 100 μl molecular grade water (Severn Biotech) and stored at −80°C until required.
16S rRNA and mcrA gene PCR-DGGE analysis of methanogen enrichments
Bacterial and archaeal 16S rRNA genes were amplified by direct PCR from sediment slurry DNA extracts using DreamTaq DNA polymerase (Thermo Fisher Scientific) with primer pairs 357FGC-518R or SAfGC-PARCH519R, respectively and analyzed by DGGE (see Webster et al., 2006). Additionally, methyl-coenzyme M reductase genes (mcrA) were amplified by nested PCR using primers ME1f-ME2r and MLf-MLr without a GC-clamp and analyzed on 6–12% gradient (w/v) polyacrylamide DGGE gels with a 25–50% denaturant gradient (Webster et al., 2011). All DGGE gels were stained with SYBR Gold nucleic acid stain (Invitrogen), viewed under UV and images captured using a Gene Genius Bio Imaging System (Syngene). DGGE bands were excised, re-amplified by PCR, sequenced (O’Sullivan et al., 2008) and analyzed using the NCBI nucleotide BLAST program.1
Methanogen isolation
A selected number of Gulf of Cádiz methanogen enrichments showing methane production were used as inoculum in attempts at isolating pure methanogens. Approximately 1 ml of enrichment culture was subcultured into 10 ml of artificial seawater (ASW) medium as described (Watkins et al., 2012) and supplemented with either 10 mM methylamine, 10 mM methanol or H2/CO2 (80,20, 0.1 MPa) as substrates. Growth was based on methane production monitored by gas chromatography. Methanogens were then isolated using a combination of deep-agar shake tubes and/or a dilution-to-extinction series with antibiotics to inhibit bacterial growth at 25°C (Parkes et al., 2010; Watkins et al., 2012). Methanogens were identified by PCR of the 16S rRNA and mcrA gene as described previously (Watkins et al., 2012).
Archaeal and bacterial 16S rRNA gene sequencing of MV sediments
MV sediment samples stored for molecular analysis were used to conduct a microbial diversity survey using three different sequencing approaches. DNA was extracted from 5 g of each MV sample (Table 1) using the FastDNA® SPIN Kit for Soil (MP Biomedicals) as described (Webster et al., 2003). Bacterial (V1-V5 region) and archaeal (V2-V5 region) 16S rRNA genes were amplified by PCR from sediment samples using DreamTaq DNA polymerase (Thermo Fisher Scientific Inc.) with primers 27F-907R and 109F-958R, respectively, as described (Webster et al., 2006). Three replicate PCR products for each sample were cleaned, pooled and cloned in pGEM-T Easy vector (Promega) and transformed into Escherichia coli JM109 competent cells (Promega) according to manufacturer’s protocol. Recombinant colonies were picked, grown overnight at 37°C in 96-well plates containing LB liquid medium with 7.5% (v/v) glycerol and 100 μg ml−1 ampicillin, and the gene libraries stored at −80°C. Approximately 40–70 recombinant clones from each Archaea and Bacteria 16S rRNA gene library were amplified by PCR with M13 primers, checked and sequenced using an ABI 3130xl Genetic Analyzer (Applied Biosystems). Sequence chromatographs were analyzed using the Chromas Lite software package version 2.01,2 and gene sequences identified using nucleotide BLAST3 against the nucleotide collection and reference RNA sequence databases. New sequences reported here have been submitted to the NCBI database under accession numbers MT825700 - MT826197.
DNA from some MV samples was also amplified using methods of the International Census of Marine Microbes (ICoMM). The V6 region of the 16S rRNA gene from Archaea and Bacteria was amplified and subjected to 454 pyrosequencing on a GS20. All PCR methods, primers and analysis tools are detailed on the ICoMM website (https://vamps2.mbl.edu; Sogin et al., 2006). Clusters were generated using the single-linkage pre-clustering algorithm to smooth sequencing errors and reduce noise, followed by primary pairwise, average linkage clustering. OTUs were created using clustering thresholds of 3%, corresponding to 97% similarity (Huse et al., 2010). Tag sequences are publicly available from ICoMM as the datasets ICM_CFU_Av6 and ICM_CFU_Bv6.
Additionally, MV DNA samples were amplified using the universal 16S rRNA gene primers (V4 region) 515F-806R (Caporaso et al., 2011) and Archaea-specific primers 519F-958R (V4-V5). Amplicons for Illumina Miseq sequencing were made by dual indexed nested PCR (D’Amore et al., 2016) designed to amplify the 16S rRNA gene and incorporate the illumina adapters and sample identification barcodes as described in the Nextera DNA sample preparation guide (Illumina Inc.). All primers were synthesised by Integrated DNA Technologies, Inc. and PCR amplifications, purification, pooling and sequencing on an Illumina MiSeq platform were carried out by the Centre for Genomic Research (CGR), University of Liverpool. Raw Fastq files were trimmed for the presence of Illumina adapter sequences using Cutadapt version 1.2.1 (Martin, 2011) and for a minimum window quality score of 20 using Sickle version 1.200 (Joshi and Fass, 2011) by CGR who provided trimmed data suitable for downstream analysis. Overlapping regions within the paired-end Illumina sequencing reads were aligned to generate contigs and quality filtered using USEARCH (Edgar, 2010). Further analysis of sequencing data was performed in QIIME (Caporaso et al., 2010). Representative OTUs were picked with pick_de_novo_otus.py command in QIIME at 97% similarity and taxonomy assigned with the Greengenes database (DeSantis et al., 2006). Singletons, unidentified and non-specific sequences (e.g., Bacteria sequences in the Archaea-specific library) were removed.
Results
Sediment pore water geochemistry
Geochemical pore water profiles (methane, sulphate, sulphide, and chloride) from seven mud volcanoes in the Gulf of Cádiz sampled during two expeditions (MSM1-3 and JC10) are shown in Figure 2. All MV sites studied differed in their geochemical profiles. The majority of MV sediments had consistent or rapid sulphate removal with depth, demonstrating active prokaryotic sulphate reduction. Sulphate was completely removed in Bonjardim, Porto, Meknes, Carlos Ribeiro, Captain Arutyunov, and Darwin MVs between ~0.2 and 1.2 meters below seafloor (mbsf; Figure 2), whereas Mercator MV had sulphate concentrations of 14.0 mM (station 019) and 26.7 mM (station 238) at the bottom of the core for each station (1.63 mbsf; Figure 2E). For the majority of MV sediments with active sulphate removal, methane concentrations increased rapidly with depth reaching saturation at atmospheric pressure by 0.5 mbsf, indicating that active methanogenesis was occurring in the subsurface (with maximum concentrations of 7.5 and 6.0 mmol CH4 l−1 sediment at Porto MV station 143 and Captain Arutyunov MV station 227, respectively; Figure 2). Despite incomplete sulphate removal at Mercator MV, high methane concentrations (e.g., 4.2 mmol CH4 l−1 sediment at station 009) were detected throughout the sediment profile for all stations analyzed. Sulphide profiles at most MV sites peaked at the interface between the methane and sulphate gradients (Figure 2), indicative of a distinct sulphate methane transition zone (SMTZ) with active anaerobic oxidation of methane (AOM). The exception being Mercator MV which had no detectable sulphide (Figure 2E).
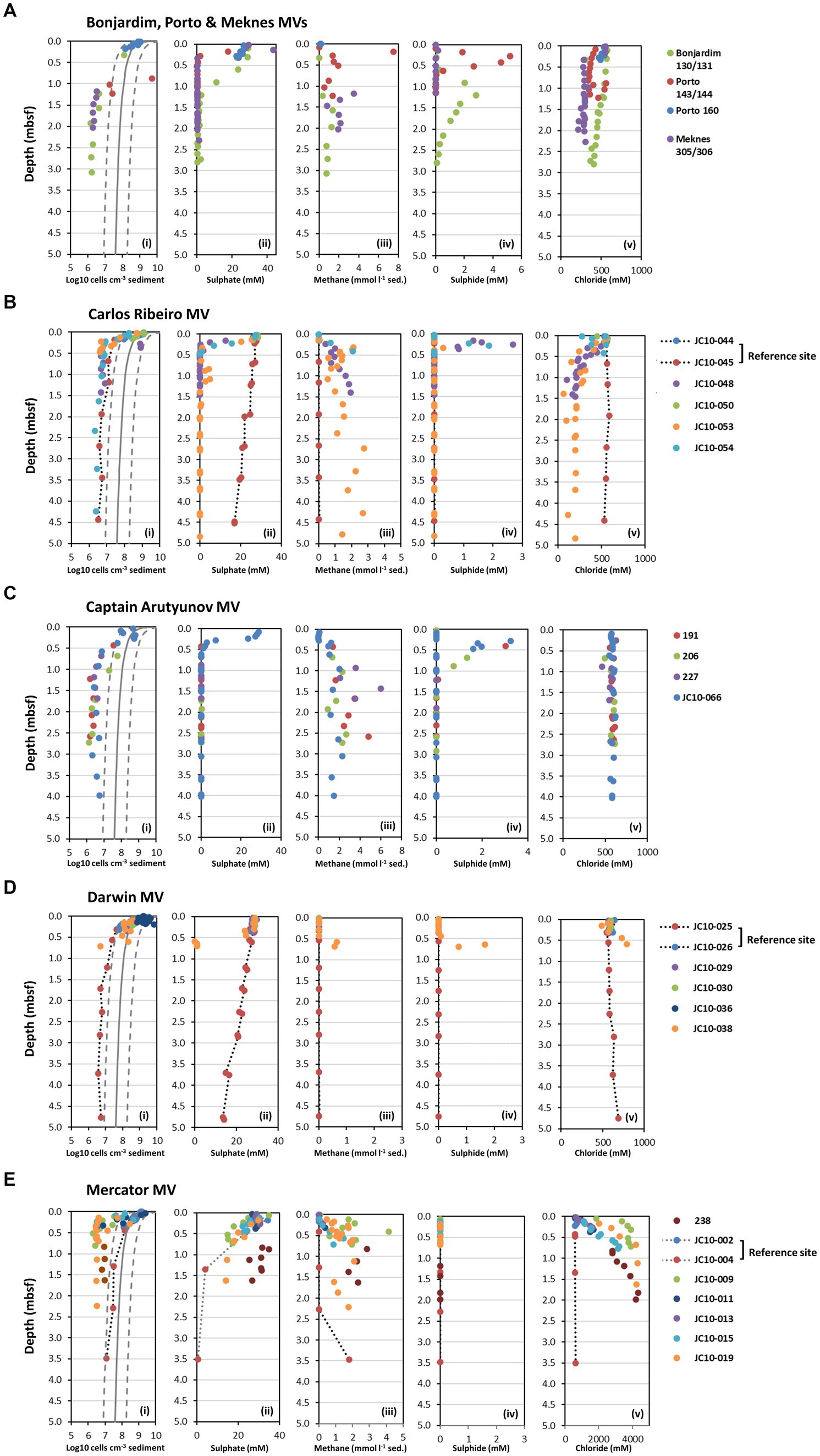
Figure 2. Depth profiles of prokaryotic cell numbers and geochemical data for seven mud volcanoes (MV) from the Gulf of Cádiz. (A) Depth profiles for Bonjardim, Porto and Meknes MVs, (B) Carlos Ribeiro MV, (C) Captain Arutyunov MV, (D) Darwin MV, and (E) Mercator MV. (i) Prokaryotic cell numbers determined by AODC. The solid line shows Parkes et al. (2000) general model for prokaryotic cell distribution in deep marine sediments, and dotted lines represent 95% prediction limits. Pore water concentrations of (ii) sulphate, (iii) in situ methane (iv), sulphide, and (v) chloride. Data shown for Bonjardim MV station 130, Porto MV station 143 and Meknes station 305 obtained from the PANGAEA Data Publisher for Earth and Environmental Science (https://www.pangaea.de/). mbsf, meters below seafloor.
The methane and sulphate profiles at the Darwin and Carlos Ribeiro MV reference sites (stations 025 and 045, respectively) were very different to those of the MV sediments, with slower, linear sulphate removal (14.1 and 17.1 mM at ~4.5 mbsf), and very low CH4 concentrations in the subsurface (1–7 μmol CH4 l−1 sediment; Figure 2). Geochemical profiles at the Mercator MV reference sites 002 and 004 were similar to MV sediments with sulphate depletion and CH4 production in the subsurface, albeit at deeper depths (>2 mbsf). This may suggest that the Mercator MV reference sites are influenced by nearby MV activity, despite pore water salinity not being elevated. Discovery of a nearby buried mud volcano may also be influencing the observed biogeochemistry at this station (Perez-Garcia et al., 2011).
Mercator MV was clearly distinct from the other MV sites due to extremely high salinity at this site (Haffert et al., 2013) with chloride concentration in the surface sediments (e.g., stations 009 and 019) being 3.5-fold higher (2,011 mM) than typical sea water values (Figure 2E). Salinity further increased with depth to 10x seawater chloride values (5,585 mM) at 2.3 mbsf to almost halite saturation levels (~ 5,800 mM) as reported previously (Maignien et al., 2013). Carlos Ribeiro MV and Meknes MV had salinity values lower than that of seawater with chloride around 200 and 300 mM, respectively, at depth. All other MV (Bonjardim, Porto, Captain Arutyunov and Darwin) and reference sites had salinities close to seawater concentrations throughout their depth profiles (Figure 2).
Prokaryotic cell numbers
At all sites, AODC cell numbers at the sediment surface (0.01 to 0.20 mbsf) were within the range observed previously (0.03–6.96 ×109 cells cm−3 sediment) for subseafloor sediments (Parkes et al., 2000) after which they decreased rapidly to 1.16 to 8.10 ×106 cells cm−3 sediment at 0.2–0.5 mbsf (Figure 2) corresponding with the approximate depth of the SMTZ (Figure 2). Cell numbers below the SMTZ (varying depth 0.2–0.5 mbsf) then fell below the expected lower limit of the global subseafloor sediment trend line (0.95–3.89 × 106 cells cm−3 sediment for 0.5–4.0 mbsf), where they remained relatively constant to the bottom of the sediment core. Mercator MV reference station 004 and Porto MV station 144 were however, exceptions to this observation. Cell counts at Mercator MV station 004 followed the global trend in subseafloor sediments (Figure 2E), showing a steady decline with depth. In contrast, Porto MV station 144 had exceptionally high numbers which were outside of the upper expected limit for subseafloor sediments at 0.9 mbsf (5.11 × 109 cm−3 sediment) and then cell numbers declined rapidly and were close to the lower expected limit (Figure 2A). Interestingly, the low cell counts for most MV sediments below the SMTZ (0.2–0.5 mbsf) were equivalent to cell numbers found below 100 mbsf in other subsurface sediments (Supplementary Figure S1) based on the global subseafloor sediment trend (Parkes et al., 2000). This may suggest that prokaryotic populations from these depths are derived from populations at much greater depths and have been transported upwards during episodic MV eruptions. Such low numbers of cells presumably impacted on the downstream analysis of MV samples, as the majority of sediment samples analyzed for DNA analysis were not readily amplifiable by direct PCR with either archaeal or bacterial 16S rRNA gene primers (Supplementary Table S1).
Microbial diversity of gulf of Cádiz MV sediments
Microbial populations from the methanogenic zones of different Gulf of Cádiz MVs were investigated by a combination of molecular methods including 16S rRNA and mcrA gene clone libraries, 16S rRNA gene tag sequencing and illumina 16S rRNA gene amplicon sequencing. However, DNA was only successfully amplified by direct PCR from seven different sampling stations covering four different MV sites (Darwin, Bonjardim, Captain Arutyunov and Porto MVs; Supplementary Figures S2–S4). Bacterial and archaeal 16S rRNA gene libraries made by different methods were generally in agreement with the exception of the Archaea V4-V5 region 16S rRNA gene amplicon library (Supplementary Figure S2) which differed considerably in identification of major archaeal taxonomic groups when compared with the other methods used. This may be due to PCR bias introduced by this set of archaeal primers (Teske and Sørensen, 2008).
Archaeal diversity at all four MV sites analyzed (Supplementary Figure S2) showed that Archaea involved in methane cycling (methanogens and ANME) were present at each site by at least one PCR-sequencing method. However, their proportions varied between MV sites and between stations of the same MV. For example, ANME-1 phylotypes at Captain Arutyunov MV were prevalent but varied with station; 88.4% at station 066, 52% at station 206, 39.2% at station 227 and 14% at station 191 when comparing the V2-V5 region 16S rRNA gene clone libraries. Whereas methanogens from the Euryarchaeota orders Methanosarcinales and Methanobacteriales were dominant at station 191 (65% of V2-V5 region 16S rRNA gene library) but were a minor component or absent at the other three Captain Arutyunov MV stations (0–6% of V2-V5 region 16S rRNA gene libraries). A high proportion (67.3%) of ANME-1 sequences were also present at station 191 in the V4-V5 region 16S rRNA gene library. Other major taxa at Captain Arutyunov included members of the Euryarchaeota belonging to Thermoprofundales (also known as Marine Benthic Group-D or Izemarchaea; Baker et al., 2020), Poseidonales (formerly Marine Group II; Rinke et al., 2019), Pontarchaea (formerly Marine Group III; Adam et al., 2017), Methanomicrobiales, Hadesarchaea (formerly SAGMEG; Baker et al., 2016) and Terrestrial Miscellaneous Euryarchaeotal group (Takai et al., 2001), along with members of the Bathyarchaeota (formerly Miscellaneous Crenarchaeotal Group; Meng et al., 2014), Lokiarchaeota (formerly Marine Benthic Group-B or Deep-Sea Archaeal Group; Baker et al., 2020), Parvarchaeota and Thaumarchaeota (including Marine Group 1 and Marine Benthic Group-A; Baker et al., 2020; Supplementary Figure S2).
Similar groups of Archaea were also present at Darwin MV station 038 and Bonjardim MV station 131; with Bonjardim MV having a high proportion of 16S rRNA gene sequences belonging to Methanosarcinales and Lokiarchaeota, and Darwin MV having a high number of ANME-1 and Bathyarchaeota 16S rRNA gene sequences, as well as Lokiarchaeota identified by V4-V5 16S rRNA gene amplicon sequencing. In contrast, Porto MV at 0.9 mbsf was dominated almost entirely by members of the ANME-1 with 96.8–100% of archaeal 16S rRNA gene sequences identified by all PCR-sequencing methods. Methanogens and ANME were also confirmed in all four MV sediments successfully analyzed for mcrA genes. ANME-1 and ANME-2 mcrA gene sequences were dominant in all MVs with the exception of Bonjardim MV which had 100% of mcrA gene sequences belonging to the methanogen genus Methanococcoides (Supplementary Figure S3).
Overall, bacterial diversity (Supplementary Figure S4) was higher than archaeal diversity with a greater average number of higher taxonomic groupings being identified per MV sediment analyzed (14 bacterial groups compared with 9 archaeal groups). However, all MVs were dominated or had a high proportion of Atribacterota (class JS1; Webster et al., 2004; Nobu et al., 2016), Chloroflexota, Pseudomonadota (formerly Proteobacteria), Deltaproteobacteria, Planctomycetota, Bacillota (formerly Firmicutes) and Ca. “Aminicenantes” (formerly OP8; Farag et al., 2014) with other bacterial lineages being less abundant (Supplementary Figure S4). Interestingly, Porto MV station 144 (0.9 mbsf) and Captain Arutyunov MV station 206 (0.7 mbsf) had up to 94 and 73% Atribacterota (class JS1) 16S rRNA gene sequences, respectively.
Methanogenic activity ex situ
Methanogenesis from four different 14C-substrates (acetate, bicarbonate, methylamine and methanol) was detected at all MV sites (Carlos Ribeiro, Captain Arutyunov, Darwin and Mercator) analyzed, albeit at low rates (0.001–50.5 pmol cm−3 d−1) and at discrete sediment depths (Supplementary Figure S5; Supplementary Table S5). However, despite these low rates, the average rate of methanogenesis for each MV was elevated when compared to the respective non-mud volcano reference site, with average MV methanogenic rates being up to two orders of magnitude higher (Supplementary Table S2). Maximum rates of methanogenesis tended to occur in the upper 0.5 m of MV sediments (Supplementary Figure S5) and at some sites this corresponded with decreases in sulphate concentrations (e.g., Carlos Ribeiro and Captain Arutyunov; Figure 2). Interestingly, the average proportion of combined methanogenic rates for the four MV sites due to hydrogenotrophic and acetoclastic methanogenesis (Supplementary Table S2) were similar at 22.8 and 23.7%, respectively, with methylotrophic rates using methylamine and methanol accounting for the remainder (48.9 and 4.6%, respectively). However, methylotrophic methanogenesis was less dominant in the reference station sediments, accounting for 35.9% with methylamine (and 0% from methanol) of the combined average methanogenic rates, with acetate and H2/CO2 being responsible for the remainder (33.3 and 30.8%, respectively; Supplementary Table S2).
Methanogenesis from different substrates in MV sediment slurries
Methanogenesis from added substrates (Table 2) was detected in 68 (28.3%) of the MV sediment slurries (total 240 sediment slurries) incubated for 130 days, with 50% of the methane producing slurry enrichments having been supplemented with the methylotrophic substrates, methanol and methylamine (15 and 19 enrichments, respectively). Acetoclastic methanogenesis represented 17.7% of the methanogenic slurries, while only 4.4% of the slurries produced methane with H2/CO2. The remaining methane producing slurries were supplemented with either benzoate (14.7%) or hexadecane (13.2%). An additional subset of sediment slurries (40 control sediment slurries) without substrates also showed methane production ranging from no detection to >1,000 nmol CH4 (see Table 2), presumably due to methane degassing and/or methane production from in situ substrates, confirming the above methanogenesis activity measurements. This control methane value was then removed from the parallel amended slurries.
Closer inspection of the sediment slurries taking into account MV and sediment depth (Table 2) demonstrated that 55% of the different sediments tested (40 different sediments) had the potential to produce methane from methylamine or methanol. Whereas methane from other substrates was only found in 30 and 7.5% of sediments with acetate and H2/CO2, and 25 and 22.5% of sediments for benzoate and hexadecane, respectively. Interestingly, of all the different metabolic groups of methanogens tested for, only methylotrophic methanogenesis occurred at all seven MV sites across the Gulf of Cádiz. However, despite methylotrophic methanogenesis being prevalent in the Gulf of Cádiz MV sediments, methane production varied considerably depending on MV site and/or sediment depth. For example, methane produced ranged from 0.11–105.91 nmol CH4 cm−3 d−1 for methylamine and 0.08–118.24 nmol CH4 cm−3 d−1 for methanol, with highest methane production for both substrates being in sediments from Darwin MV at 0.11 mbsf (Figure 3; Table 2). Generally, rates of methylotrophic methanogenesis were highest in near surface sediments and decreased steadily with sediment depth at all MV sites (Figure 3B).
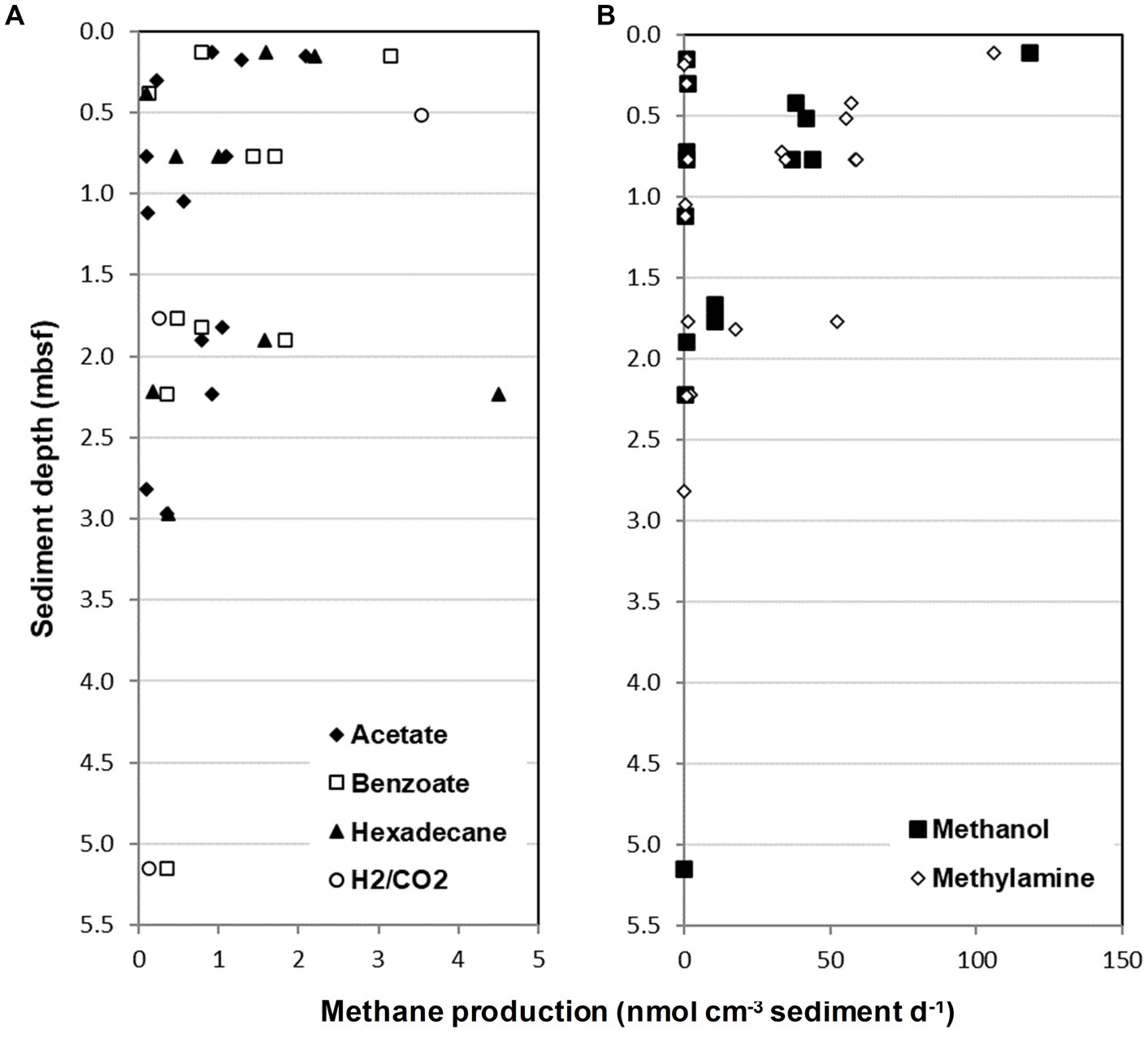
Figure 3. Methane production from Gulf of Cádiz mud volcano sediment slurries incubated for 130 days with a range of methanogenic substrates. (A) Methane produced from sediment slurries incubated with either acetate, benzoate, hexadecane or H2/CO2 plotted against sediment sample depth. (B) Methane produced from sediment slurries incubated with either methanol or methylamine. Only methane producing sediment slurries are shown (see Table 2 for further information). mbsf, meters below seafloor.
Similarly, potential methanogenesis also varied between MV sites and sediment depths for all other substrates tested, but methane production was often several orders of magnitude lower than with methylamine or methanol (Figure 3; Table 2). For example, methane produced by acetoclastic methanogenesis varied from 0.09–2.09 nmol CH4 cm−3 d−1, hydrogenotrophic methanogenesis ranged from 0.12–3.54 nmol CH4 cm−3 d−1 and hexadecane and benzoate were 0.1–4.51 and 0.12–3.16 nmol CH4 cm−3 d−1, respectively (Figure 3A; Table 2). Interestingly, the highest methane produced for these substrates occurred at different MVs to that with methylamine and methanol, with acetate, benzoate and hexadecane showing the highest rates of methanogenesis at Mercator MV, and H2/CO2 at Captain Arutyunov MV. Also, in contrast to methylotrophic methanogenesis, methane production for acetate, benzoate, hexadecane, and H2/CO2 did not show a clear trend with sediment depth (Figure 3A).
Methanogenesis in substrate amended slurries from different MVs
At the deep water (3,860 m) Porto MV, 58.3% of sediment slurry enrichments produced methane with 16.6% of these showing methane production higher than 100 nmol CH4 after 130 days, whereas at the other two deep water (>2,000 m) sites (Bonjardim and Carlos Ribeiro MV), the numbers of positive methanogenic slurries were lower, with 20.8% at Bonjardim MV (12.5% with >100 nmol CH4 after 130 days) and 16.6% at Carlos Ribeiro MV (0% with >100 nmol CH4 after 130 days). The proportion of sediment slurries from the intermediate water depth (~1,000 m) MV sites, Captain Arutyunov MV and Darwin MV, producing methane were 26.7 and 16.6%, respectively, with a similar low percentage of these methanogenic slurries (16.7 and 11.1%, respectively) having methane production values >100 nmol CH4 after 130 days. In contrast, the shallow Meknes MV site (694 m) showed both a high percentage of positive methane producing slurries (66.6%) and a high number (58.3%) of methanogenic slurries with methane production >100 nmol CH4 after 130 days. However, Mercator MV, the shallowest MV site (346–470 m) which also had the largest number of sediments sampled (14 different sediments) for methanogenesis only had 28.6% positive methanogenic slurries, and 19% of these were >100 nmol CH4 after 130 days. Such contrasting results suggest that the potential for MVs to produce methane is not linked with their seawater depth.
However, the sediment slurries that produced the highest amounts of methane (>100 nmol CH4 after 130 days) from MVs with a water depth > 1,000 m (Porto, Bonjardim, Captain Arutyunov and Darwin MVs) were dominated by methylotrophic methanogenesis (15 out of 17 high-rate slurries; Table 2). Whereas MVs located below 1,000 m water depth (Meknes and Mercator MVs) were capable of producing high amounts of methane from a much broader range of metabolic substrates. For example, sediments from Meknes and Mercator MVs had only 7 out of 23 high-rate methanogenic slurries with added methylamine or methanol (Table 2).
Furthermore, increased incubation times (up to 300 days) led to several MV sediment slurries, which at 130 days showed no or low rates of methanogenesis (<10 nmol CH4 after 130 days), developing the ability to generate high amounts of methane (>100 nmol CH4 after 300 days). These were all from sediments of Carlos Ribeiro MV (0.55 and 1.05 mbsf) and Darwin MV (0.11 and 0.18 mbsf; Table 2), and the majority (83.3%) of these extended incubation time high-rate methanogenic slurries were incubated with the methanogenic substrates, methanol or methylamine.
Methane consumption in MV sediment slurries
Methane removal was also observed in a number of sediment slurries incubated with methanogenic substrates (Table 2) presumably due to anaerobic oxidation of methane (AOM). Interestingly, the number of slurries with detectable AOM (defined as >10 nmol CH4 consumed after subtraction of unamended control slurry) were similar for each substrate tested (10–16 slurries per substrate) with the exception of H2/CO2 which accounted for 24.7% (21 slurries) of AOM slurries. The highest number of sediment slurries detected with AOM were obtained from sediments from Captain Arutyunov, Mercator and Carlos Ribeiro MVs with 27, 26 and 21 AOM slurries, respectively. Furthermore, Carlos Ribeiro MV sediments were particularly effective at removing methane as this MV had 70% (21 out of 30) of its sediment slurries showing AOM.
Methanogen diversity in MV sediment methanogenic slurries
All MV sediment slurries showing relatively high rates of methanogenesis (>100 nmol CH4) after 130 days incubation were screened for archaeal 16S rRNA and methanogen-specific mcrA genes using PCR-DGGE and sequencing (Figure 4). Despite a range of substrates shown to produce methane (Table 2), the majority of successful 16S rRNA gene PCR amplifications from extracted DNA were mainly obtained from sediments incubated with either methanol or methylamine. Presumably due to the potential rates of methanogenesis in these methylotrophic slurries often being 10-100-fold higher (Figure 3; Table 2) than those from other substrates, resulting in a stimulation of methanogen cell numbers and increased biomass and/or extractable DNA.
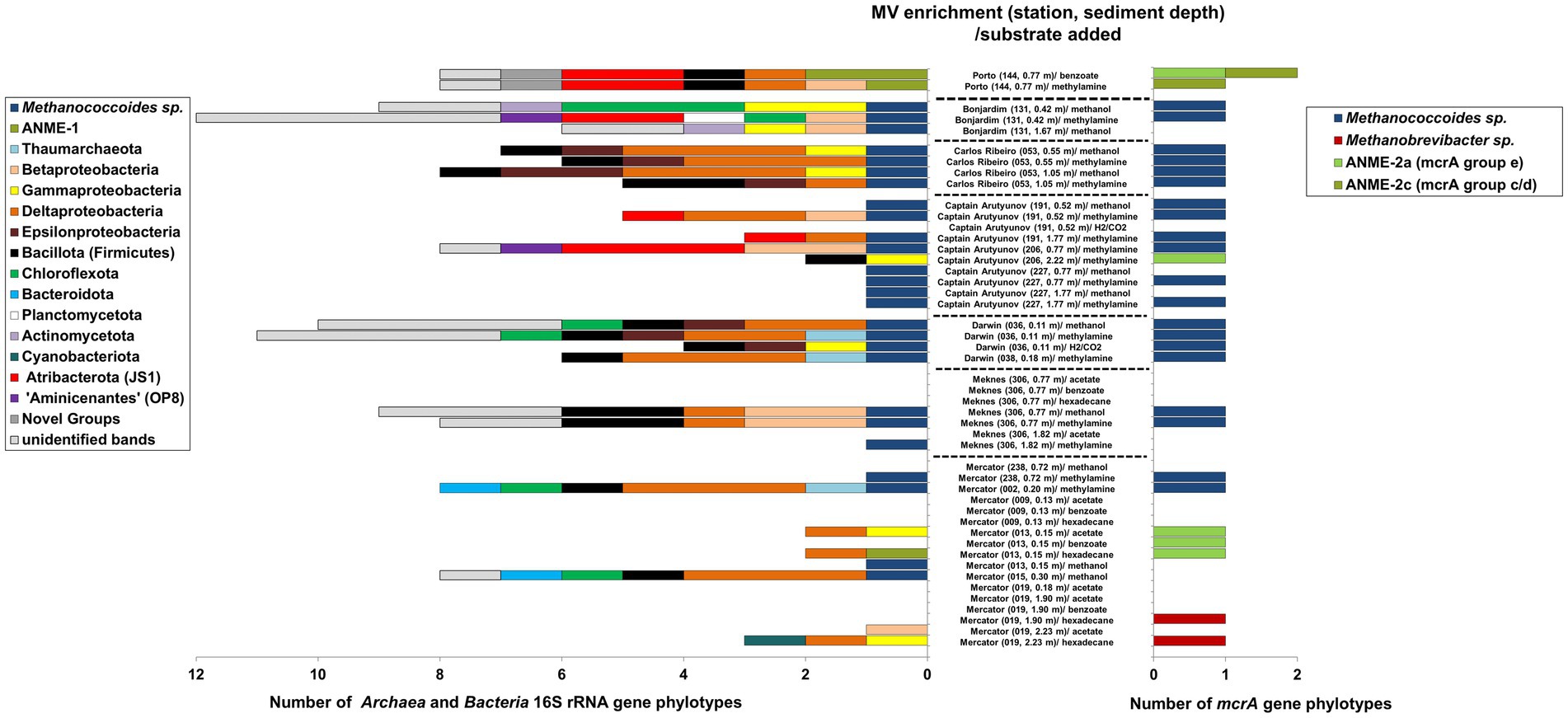
Figure 4. Archaeal and bacterial diversity in methane producing Gulf of Cádiz mud volcano sediment slurries incubated with a range of methanogenic substrates. Diversity was assessed by PCR-DGGE analysis of 16S rRNA and mcrA genes after 130 d incubation. Unidentified bands refer to faint bands that were not excised and sequenced.
Sequencing excised archaeal 16S rRNA gene DGGE bands revealed that most of the methanogenic enrichments incubated with either methanol or methylamine were dominated by Archaea belonging to the methanogen order Methanosarcinales and were related (92–100% sequence similarity) to sequences previously found in a number of mud volcanoes and marine sediments (Supplementary Table S3), as well as pure cultures of Methanococcoides alaskense (97–99%) and Methanococcoides methylutens (99–100%). However, one methanogenic sediment slurry (Figure 4) from Porto MV (0.77 m) and incubated with methylamine did not contain detectable methanogens, but surprisingly was dominated by anaerobic methane-oxidizing Archaea ANME-1. These sequences were related (98% sequence similarity) to 16S rRNA gene sequences previously found in sulphate–methane transition zone (SMTZ) sediments from Aarhus Bay (Webster et al., 2011). Similar ANME-1 sequences were also found in Porto MV sediment enrichments incubated with benzoate (Figure 4), along with a second ANME-1 16S rRNA gene phylotype related to sequences from a mud volcano (Amsterdam MV) in the Eastern Mediterranean Sea (Pachiadaki et al., 2011). ANME-1 sequences were also the dominant archaeal group in the methanogenic slurry from Mercator MV near surface sediment (0.15 mbsf) incubated with hexadecane (Supplementary Table S3). However, it should be noted that the presence of ANME-1 within the enrichments could be due to their persistence in the sediment slurry rather than their use of the methanogen substrates directly.
Methanogen diversity in all methanogenic slurries were also investigated using the mcrA gene. The majority of methylotrophic sediment slurries analyzed by PCR-DGGE resulted in congruent mcrA gene sequences to those found by 16S rRNA gene analysis (Figure 4). Many slurries contained mcrA gene sequences that were 96–100% similar to those found in several Methanococcoides species and shared 97–99% sequence similarity to sequences previously found in marine sediments from Guaymas Basin, Marennes-Oléron Bay and Cascadia Margin (Dhillon et al., 2005; Roussel et al., 2009; Yoshioka et al., 2010). However, no mcrA gene sequences were found that corresponded with ANME-1 archaea, instead all ANME-related mcrA sequences identified in Gulf of Cádiz MV sediment slurries were related to sequences that grouped with ANME-2 (Supplementary Table S3). For example, Porto MV (0.77 mbsf) methanogenic sediment slurries incubated with benzoate or methylamine contained ANME-2a (mcrA group e) and/or ANME-2c (mcrA groupc/d) mcrA sequences similar (96–100% sequence similarity) to those found in sediments from Napoli MV (Lazar et al., 2011a) and Eel River Basin (Beal et al., 2009). Interestingly, methanogenic slurries from Mercator MV site 019 at depths 1.90 and 2.23 msf incubated with hexadecane contained mcrA gene sequences that were closely (100% sequence similarity) related to the hydrogenotrophic methanogen Methanobrevibacter arboriphilus.
Bacterial 16S rRNA gene diversity in MV sediment methanogenic slurries
In contrast to Archaea, the bacterial diversity within many of the methanogenic slurries was relatively high (Figure 4) with slurries containing sequences representative of several phyla (sometimes up to 5 phyla per slurry) and up to 11 detectable bacterial phylotypes (e.g., Bonjardim MV). Overall bacterial phylotypes belonged to 13 major taxa, including members of the Betaproteobacteria, Gammaproteobacteria, Deltaproteobacteria, Campylobacterota class Epsilonproteobacteria, Bacillota, Chloroflexota, Bacteroidota, Planctomycetota, Actinomycetota, Cyanobacteria, Atribacterota (JS1) and Ca. “Aminicenantes” (OP8), and one novel phylum level group previously found in deep marine sediments of the Japan Sea (Yanagawa et al., 2011). However, despite high bacterial diversity within the sediment slurries no clear pattern was observed to suggest that bacterial diversity was related to the methanogenic substrates added. Interestingly, sequences from Atribacterota, Ca. “Aminicenantes,” Actinomycetota, Planctomycetota and the novel bacterial group were only found in sediment slurries from MVs located in water depths >1,000 m, whereas Pseudomonadota and Bacillota were found in most MV sediments. It should also be noted that several methanogenic slurries dominated by Methanococcoides species contained no detectable Bacteria (e.g., Captain Arutyunov MV), and that many of the bacterial phylotypes that were detected in slurries were related to sequences previously found in other marine sediments (Supplementary Table S4), including those from cold seeps and submarine mud volcanoes (e.g., Heijs et al., 2008; Orcutt et al., 2010; Pachiadaki et al., 2011; Yanagawa et al., 2011).
Isolation of methanogens
MV sediment slurries (from Bonjardim, Carlos Ribeiro, Captain Arutyunov, Darwin, Meknes and Mercator) showing high methanogenic activity and detectable methanogens, by both 16S rRNA and mcrA genes, were used to isolate novel methanogens. After further enrichment, subculture and isolation (see Watkins et al., 2012, 2014) five out of six MV sediments yielded pure methanogen cultures. The majority of pure methanogens were able to utilise methylotrophic substrates and one was isolated on H2/CO2. All isolated methanogens belonged to the methylotrophic genus Methanococcoides (Table 3), with the exception of the one hydrogenotrophic Methanogenium species from the surface sediments of Darwin MV.
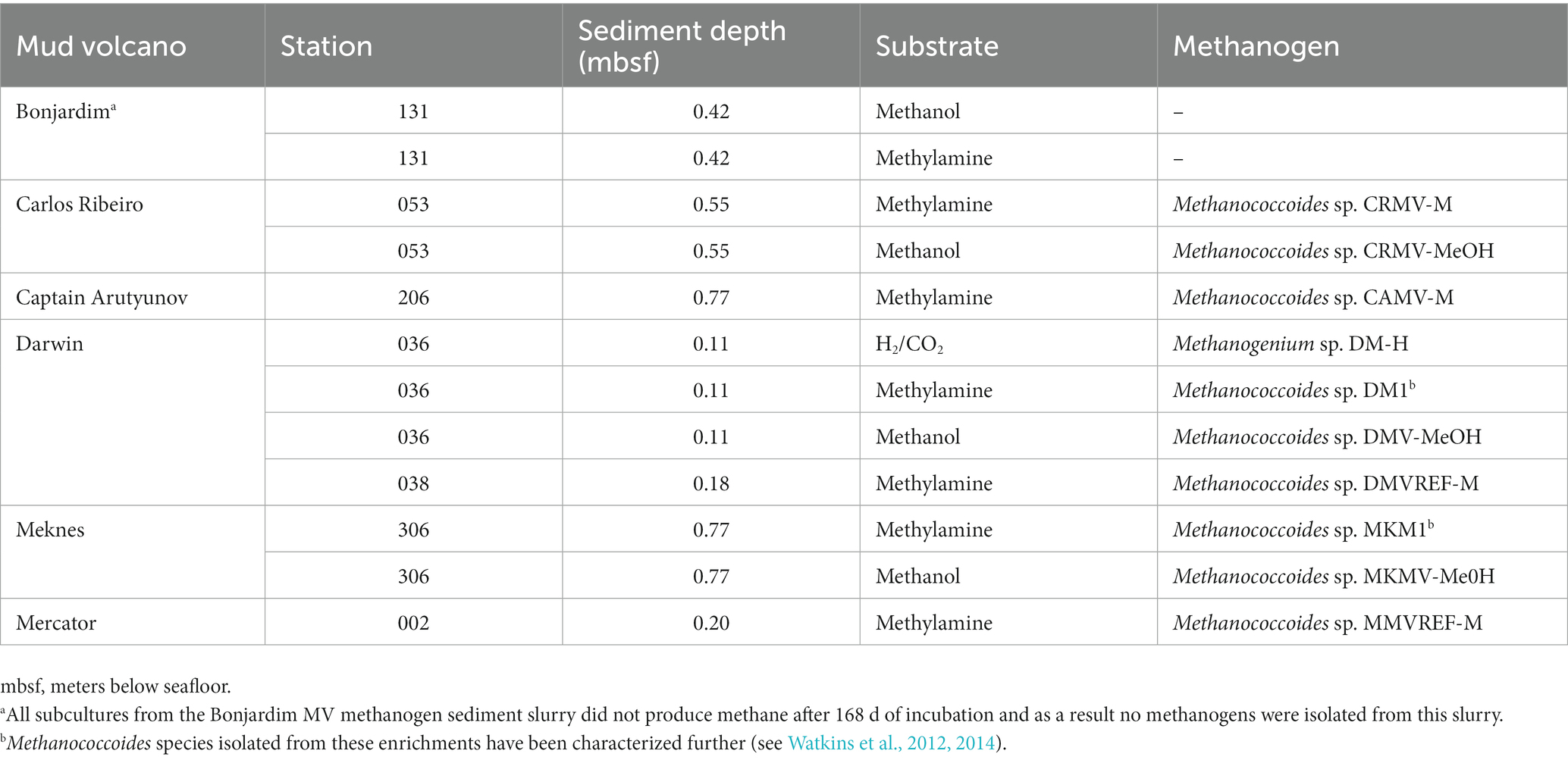
Table 3. Pure methanogen cultures isolated from Gulf of Cádiz mud volcano methanogen sediment slurries.
Discussion
The Gulf of Cádiz is a tectonically active region of the European continental margin characterized by a high number of submarine mud volcanoes (Figure 1) that have been frequently studied since their discovery in 1999 (Kenyon et al., 2000; Pinheiro et al., 2003; Niemann et al., 2006a; Medialdea et al., 2009; Maignien et al., 2013; Nuzzo et al., 2019). Research findings at many of these MVs indicate past and present methane seepage, presence of methane hydrate-bearing sediments, authigenic carbonates and seep-related macrofauna (Gardner, 2001; Díaz-del-Rio et al., 2003; Pinheiro et al., 2003; Cunha et al., 2013). However, despite these studies and the extensive interest in mud volcanoes from the Gulf of Cádiz, there is relatively little information on methanogenesis, and the prokaryotic populations involved.
Mud volcano microbial diversity
Our microbial diversity survey of mud volcanoes from the Gulf of Cádiz showed that sediments from below the SMTZ and within the methanogenic zone (below approx. 0.3 mbsf) have generally low numbers of prokaryotic cells, except Porto MV at 0.9 mbsf which had exceptionally high numbers (Supplementary Figures S6). Based on 16S rRNA gene sequences, microbial populations were dominated by methanogenic archaea (Methanosarcinales), anaerobic methane-oxidizing archaea (ANME), Bathyarchaeota, Lokiarchaeaota and bacteria such as, Atribacterota, Chloroflexota, Bacillota and Deltaproteobacteria (Supplementary Figures S2, S4, and S6) frequently found in other methane-rich sediments (Inagaki et al., 2006; Parkes et al., 2014; Vigneron et al., 2015; Katayama et al., 2016; Teske et al., 2021). Similar groups of archaea and bacteria have also been identified at submarine MVs from other locations including Håkon Mosby, Amsterdam, Napoli, Kazan, Chefren, Ryukyu Trench and Beaufort Sea MVs (Niemann et al., 2006b; Heijs et al., 2007; Omoregie et al., 2008; Lazar et al., 2011b, 2012; Pachiadaki and Kormas, 2013; Hoshino et al., 2017; Lee et al., 2019) and from the Gulf of Cádiz (Captain Arutyunov MV, Niemann et al., 2006a; Mercator MV, Maignien et al., 2013). For example, ANME-1 and ANME-2 were found to be the dominant archaea groups in sediments below the SMTZ (0.15 mbsf) at Amsterdam MV, ranging between 74 and 91% of the sequences found with only a few recognized methanogen groups (4% of sequences), whereas Deltaproteobacteria and Atribacterota accounted for 34 and 24%, respectively of the bacterial population (Pachiadaki et al., 2011). Similarly, ANME-1 and ANME-2 phylotypes dominated the sediments (0.4 mbsf) at Captain Arutyunov MV with members of the Deltaproteobacteria SEEP SRB-1 group (the AOM syntrophic partners of ANME-1 and ANME-2) accounting for 85% of bacterial sequences with a further 6% of sequences belonging to Atribacterota (Niemann et al., 2006a). Interestingly, in the study by Niemann et al. (2006a) a novel Bacillota clone CAMV300B902 was also identified, and similar 16S rRNA gene sequences were present in our study of Captain Arutyunov MV site 191 (0.45 mbsf) at high frequency (up to 55% in the V1-V5 16S rRNA gene library). Thus far, closely related sequences (>99% sequence similarity) to this phylotype have only been found at Captain Arutyunov and Kazan MVs (Niemann et al., 2006a; Pachiadaki et al., 2010), and their function is unknown. However, of note is that the phylotype’s nearest pure culture relative (90% 16S rRNA gene sequence similarity) is the SRB, Desulforudis audaxviator (Karnachuk et al., 2019), found in a deep South African gold mine, where it formed a near single-species ecosystem fueled by H2 from water radiolysis (Chivian et al., 2008). This may suggest that novel Bacillota involved in hydrogen metabolism could be important bacteria at specific mud volcanoes. However, further investigations are necessary to determine the role of these bacteria.
Methanogens and methanotrophic archaea were also evident from analysis of the gene encoding for methyl coenzyme M reductase (mcrA), an enzyme which catalyzes the final step of the methanogenic pathway present in all methanogens and anaerobic methanotrophs (Hallam et al., 2003). The mcrA gene libraries were dominated by sequences belonging to ANME, with three (Darwin, Captain Arutyunov and Porto MV) out of the four mud volcanoes analyzed having 89–100% of mcrA gene sequences belonging to ANME-1 or ANME-2 lineages. Interestingly, only the gene library from Bonjardim MV was clearly dominated by methanogen mcrA genes, with 100% of sequences being related (93–98% sequence similarity) to mcrA genes found previously in gas hydrate sediments of the Cascadia Margin (Expedition IODP 1327; Yoshioka et al., 2010) and known cultured Methanococcoides species (Webster et al., 2019; Supplementary Figure S3). Similar Methanococcoides-related mcrA sequences were also present at Captain Arutyunov MV stations 206 and 191 but at much lower abundance, comprising 3–7% of the respective gene libraries. In support of the mcrA gene findings, low numbers of 16S rRNA gene sequences of Methanococcoides species were also identified in all Gulf of Cádiz MV sites analyzed here and ranged from 25% at Bonjardim MV to 0.01% at Porto MV (Supplementary Figure S2), and 1.2% in a separate study at Mercator MV (Maignien et al., 2013). In addition, other novel members of the Methanosarcinales were also identified that were related to 16S rRNA gene sequences from sediments of the Kazan MV (Heijs et al., 2007; Pachiadaki et al., 2010) and the terrestrial Lei-Gong-Huo MV (Chang et al., 2012).
Methanogenesis in mud volcano sediments
Whilst measurements of methanogenesis in global marine sediments are relatively widespread there is comparatively little information from on or around mud volcanoes. Krüger et al. (2005) measured methanogenesis and methane oxidation at a wide variety of ocean sites, including the Håkon Mosby MV, using both in vitro and 14C-radiotracer techniques. This study found that methanogenic rates at Håkon Mosby MV were in the range of those found at other marine sediment sites, with 0.02–0.08 μmol CH4 g−1 sediment d−1 (Krüger et al., 2005). However, these rates of methanogenesis were considerably higher than those found in our study of MV sediments of the Gulf of Cádiz (Supplementary Table S2; Supplementary Figure S5) and that by Maignien et al. (2013). Interestingly, an earlier study of the Håkon Mosby MV demonstrated that there was substantial variation (up to 4 orders of magnitude) in methanogenesis rates depending on where samples were located within the MV crater (Pimenov et al., 1999), with some rates being comparable to those measured at Gulf of Cádiz MVs. Clear differences in methanogenesis due to sample location were also observed in our study, demonstrating a high degree of variation between sample location at each MV site (Supplementary Figure S5). For example, Mercator MV had a peak rate of hydrogenotrophic methanogenesis of 50.54 pmol cm−3 d−1 at station 013 located within the crater rim but no activity was detected at any other station, including sediment cores from the crater center (stations 009 and 019). Furthermore, low rates of methanogenesis were also reported for the submarine mud volcano KMV#5 in the Nankai accretionary complex where hydrogenotrophic and acetoclastic methanogenesis were 0.6–128 and 0.004–0.10 pmol cm−3 d−1, respectively (Ijiri et al., 2018). But unlike KMV#5 where hydrogen was the dominant substrate for methanogenesis, it appears that methylotrophic methanogenesis is the dominant pathway with nearly 50% of methanogenesis across all MV sites at the Gulf of Cádiz using methylamine (Supplementary Table S2).
Methylotrophic methanogenesis in MV sediment enrichments
Despite the low rates of methanogenesis measured in this study we were able to enrich methanogens and measure potential rates of methanogenesis in vitro from a number of mud volcanoes and sediment depths using a range of different substrates. These sediment enrichments further confirm that MV sediments from the Gulf of Cádiz are able to carry out methanogenesis and that the majority of MVs are dominated by methylotrophic methanogenesis rather than hydrogenotrophic or acetoclastic methanogenesis.
All methane producing sediment slurries incubated with methylamine or methanol were dominated by Methanococcoides-related methanogens. These methanogens are obligate methylotrophs that utilise C1 compounds as methanogenic substrates (Liu and Whitman, 2008). Unlike acetoclastic and CO2-reducing methanogens, methylotrophic methanogens are not outcompeted by SRB in sulphate-rich marine sediments for substrates (Purdy et al., 2003), enabling them to undertake methanogenesis in shallower anoxic sediments. In turn, this allows them to take advantage of methylated compounds (e.g., methanol and methylamines) that are typically released during the decay of organic osmolytes from marine organisms, including both prokaryotic and eukaryotic organisms present in marine sediments (Jones et al., 2019). In this study all positive methylotrophic sediment slurries were from the first 2 mbsf sediments, suggesting that in situ populations of Methanococcoides are abundant in shallow subsurface sediments of mud volcanoes in the Gulf of Cádiz, presumably where methylotrophic (non-competitive) substrates are available, and that methylotrophic methanogens are not outcompeted by SRB.
In addition, as sediment depths increased, potential in vitro rates of methylotrophic methanogenesis generally decreased, which may reflect decreasing numbers of viable Methanococcoides cells. For example, in coastal marine sediments it has been shown by quantitative real-time PCR that Methanococcoides 16S rRNA genes can represent up to 20% of the near surface (~10 cmbsf) archaeal population, and then decline to >1% in deeper sediments (Webster et al., 2015), similar to the Methanococcoides abundance values detected in 16S rRNA gene libraries in this study. Furthermore, Methanococcoides species were abundant in South China Sea sediments down to ~8.3 mbsf (Xu et al., 2021). Methanol and methylamines have been shown to be important substrates for methanogens in salt marsh sediments (Oremland et al., 1982), mangroves (Lyimo et al., 2009) and marine sediments (King et al., 1983; Zhuang et al., 2018; Xu et al., 2021), plus methylamines are the main methanogenic substrates in MV sediments from the Eastern Mediterranean Sea (Lazar et al., 2011b, 2012). Some Methanococcoides species isolated from the Gulf of Cádiz (Table 3) and the Napoli MV have also been shown to be capable of utilizing tri-, di and mono-methylamine and methanol, as well as other methylotrophic substrates like choline, dimethylethanolamine and glycine betaine directly (Watkins et al., 2012, 2014; L’Haridon et al., 2014). Studies of coastal salt marsh sediments have shown that closely related Methanococcoides species also undertake syntrophic partnerships with bacteria in a two-step process involving the formation of trimethylamine from glycine betaine (Jones et al., 2019) or choline (Jameson et al., 2019) to produce methane. It is conceivable that similar syntrophic relationships could also be occurring in MV sediments when more complex methylotrophic substrates are available, especially as glycine betaine-degrading bacteria are thought to be widespread in subsurface sediments (Jones et al., 2019). In the Gulf of Cádiz, sources of such compounds (methylamines, choline, and glycine betaine) could be made available to methanogens from the large populations of chemosynthetic macrofaunal communities that are often found around the crater of these MVs (Rodrigues et al., 2010; Cunha et al., 2013), as well as from the burial of microbial biofilms at the MV surface (Magalhães et al., 2012). Furthermore, detectable levels of methanol have also been found in sediments of several MVs including Captain Arutyunov and Carlos Ribeiro at depth (Supplementary Figure S7) as well as other subsurface sediments (Yanagawa et al., 2016; Zhuang et al., 2018; Xu et al., 2021), and methylamine has been shown to reversibly adsorb to clay minerals in sediments making it difficult to detect but remain biologically available to methylotrophic methanogens (Xiao et al., 2022). It maybe that such methanogens and methylotrophic methanogenesis is more widespread than previously thought (Valentine, 2011; Xu et al., 2021; Bueno de Mesquita et al., 2023) and that they are well suited to adapt to fluctuating geochemical environments, because of their ability to use a range of methylotrophic substrates. Furthermore, novel, and putative methylotrophic methanogens may also exist in the phyla Bathyarchaeota (Evans et al., 2015) and Verstraetearchaeota (Vanwonterghem et al., 2016), as well as the Euryarchaeota order Methanomassiliicoccales (Borrel et al., 2014), and may occur by ANME archaea (Bertram et al., 2013); all members of these archaeal groups can be identified in mud volcano sediments (Supplementary Figure S2). However, confirmation of methylotrophic methanogenesis within these groups can only be addressed by further investigations into the metagenomics or focused cultivation studies of MV sediments.
Methanogenesis from acetate, hydrogen and other substrates in MV sediment enrichments
Hydrogenotrophic and acetoclastic methanogenesis activities occurred throughout sediments of the Gulf of Cádiz MVs, but potential rates were low (Supplementary Table S2). No methanogens were identified in any sediment slurries incubated with acetate or H2/CO2, and only six slurries (five with acetate) incubated with these two important methanogenic substrates produced methane that were > 100 nmol CH4 after 130 days incubation (Table 2). This is in contrast to the generally accepted idea that methanogenesis in marine sediments is dominated by CO2 reduction (Whiticar et al., 1986) and that acetate is an important substrate in deep subsurface sediments (Wellsbury et al., 2002). Hydrogenotrophic methanogenesis was reported to provide the majority of biogenic methane in the mud volcano KMV#5 from the Nankai accretionary complex, with active methanogenesis down to ~120 mbsf (Ijiri et al., 2018). Whereas, at the Amsterdam MV in the Eastern Mediterranean Sea, acetoclastic methanogenesis occurred, and was supported by high concentrations of acetate (up to 2 mM) in deeper sediment layers (Lazar et al., 2012). Similarly, methanogenic rates and mcrA gene sequences from an active brine seep mud volcano in the Gulf of Mexico revealed a predominance of acetoclastic over hydrogenotrophic methanogenesis (Joye et al., 2009). However, it maybe that longer incubations times (up to 2 years) are needed for batch enrichments of methanogens using these substrates (Katayama et al., 2022) or that more sophisticated methods similar to continuous-flow cultivation system used by Imachi et al. (2011) are necessary to culture methanogens from subseafloor sediments.
Methane production from hexadecane (Table 2), a long-chain alkane, in some methanogenic slurries (e.g., Mercator MV) demonstrated that methanogenesis from saturated hydrocarbons might be significant in MV sediments as previously shown in other anoxic environments (Zengler et al., 1999; Fowler et al., 2016). Methane from hexadecane often occurs by acetogenic bacteria decomposing alkanes to acetate and H2, which in turn are available substrates for acetoclastic and hydrogenotrophic methanogens (Dolfing et al., 2008). In our study no direct evidence for such bacteria was observed in any hexadecane enrichment, although sequences related to acetogens (Acidaminobacter species; Stams and Hansen, 1984) were identified in other substrate-amended enrichments from the same MV sediment. However, syntrophic associations involving hydrogenotrophic methanogens and other bacteria, including SRB that can degrade hexadecane to CO2 are also known (Brennan and Sanford, 2002). Intriguingly, Deltaproteobacteria 16S rRNA gene sequences related to SRB (Supplementary Table S4) and hydrogenotrophic Methanobrevibacter mcrA genes were found in hexadecane-amended Mercator MV enrichments (Supplementary Table S3), suggesting the presence of hexadecane-utilizing syntrophic associations. Turnover of hexadecane to methane was also shown to occur in hypersaline sediments of the Napoli MV (Lazar et al., 2011a,b), and terrestrial mud volcanoes from the Carpathian Mountains and Indonesia (Jiménez et al., 2016). It is possible that in high salinity MV sediments with elevated concentrations of alkanes, such as the Mercator MV (Perez-Garcia et al., 2011), methane production from alternative substrates is additionally important. Further experiments with increased incubation times (Katayama et al., 2022) may be necessary to determine the importance of alkanes as a substrate for methanogenesis in the Gulf of Cádiz.
Similarly, methane production from benzoate (Nottingham and Hungate, 1969) was also evident from sediments from several MVs, including Porto, Meknes and Mercator, but detection of the prokaryotes involved remained elusive. Only bacteria and archaea from the Porto MV sediment with benzoate were identified, with sequences mainly belonging to ANME and Atribacterota class JS1. Previous studies have shown that methanogenic benzoate degradation to carbon dioxide and methane is mediated by a consortium of bacteria (e.g., Syntrophus species) and hydrogen-utilizing methanogens (Stams and Plugge, 2009). It is therefore fascinating to think that a consortium of Atribacterota, thought to be heterotrophic (Nobu et al., 2016) and ANME which can produce methane by CO2 reduction under certain conditions (Bertram et al., 2013) could be carrying out complete benzoate degradation through to methane. However, 16S rRNA gene sequences belonging to the genus Syntrophus (>0.01% of sequences) and hydrogenotrophic methanogens were found in sediments from all MVs analyzed from the Gulf of Cádiz, and previously 16S rRNA genes of Syntrophus species have been retrieved from the Kazan MV (Pachiadaki et al., 2010), suggesting that microbial communities in MVs have the potential to produce methane from aromatic compounds. The lack of a clear identification of methanogens from benzoate amended enrichments may also be due to the need for longer incubations or alternative methods of enrichment (Imachi et al., 2011; Katayama et al., 2022).
Summary
In summary, mud volcano microbial populations and activities were quite variable in the Gulf of Cádiz, reflecting the heterogeneity within and between individual MVs. There were also marked differences between the microbial biogeochemistry of many MV sediments and their reference sites. Surprisingly, overall direct cell numbers enumerated below the SMTZ (0.2–0.5 mbsf) were much lower than the general global depth distribution for subsurface sediments and were equivalent to cell numbers from depths below 100 mbsf in other subsurface sediments. This may suggest that the environment below the SMTZ is relatively inhospitable for prokaryotes, or that populations may have been transported upwards in fluids or mud breccia from greater depths during episodic eruptions. However, one site at Porto MV (Station 144) was an exception having highly elevated cell numbers at 0.9 mbsf. The general low cell numbers at most MV samples reflected unsuccessful DNA extraction and PCR amplification, and very low detectable rates of methanogenesis from 14C-substrates. Interestingly, methanogenic activities from methyl compounds, especially methylamine, were much higher than with H2/CO2 or acetate which are usually the dominant methanogenic substrates. Consistent with this use of substrates, significant CH4 production occurred in 50% of slurry enrichments with added methylated compounds and only methylotrophic CH4 production occurred at all seven MV sites. These slurries were dominated by Methanosarcinales methanogens related to Methanococcoides pure cultures and gene sequences detected in a number of other MVs. AOM also occurred in a number of slurries, particularly, those from Captain Arutyunov, Mercator and Carlos Ribeiro, and was supported by the dominant archaea found. Archaeal diversity at four MV sites showed the presence of both methanogen and ANME (e.g., Methanosarcinales, Methanococcoides, and ANME-1, respectively) sequences, although their proportions varied within and between MVs. Overall bacterial diversity was higher than archaeal diversity with a greater number of higher taxonomic groups found and was often dominated by Atribacterota class JS1. These results demonstrate the biogeochemical complexity of Gulf of Cádiz MV environments and the potential of their prokaryotes to be involved in methane cycling. Further work is essential to determine the full contribution of Gulf of Cádiz MV sediments to CH4 emissions and the global methane and carbon cycles.
Data availability statement
The datasets presented in this study can be found in online repositories. The names of the repository/repositories and accession number(s) can be found in the article/Supplementary material.
Author contributions
We describe author contributions to the paper using the contributor roles taxonomy (CRediT). GW, AWe, and RP: conceptualization. GW and BC: data curation and formal analysis. AWe and RP: funding acquisition, project administration, resources, and supervision. GW, BC, JR, AWa, and HS: investigation. GW, BC, AWe, and RP: methodology and validation. GW and RP: visualization. GW: writing—original draft. GW, BC, JR, AWa, HS, AWe, and RP: writing—review and editing. All authors contributed to the article and approved the submitted version.
Funding
This work was supported by the “HERMES” (Hot-Spot Ecosystems along European Margins) project grant funded under FP6 of the European Commission and the Natural Environment Research Council (NERC) grant numbers NE/F018983/1 and NE/J011177/1.
In Memoriam
This paper is dedicated to the late Dr. Barry A. Cragg, a pioneer in the field of deep biosphere microbiology, his influence on this research was as great as many of his other studies on the deep subseafloor.
Acknowledgments
We wish to acknowledge the contribution of the crew and scientific party of the MSM1-3 cruise aboard the RV Maria S. Merian. We also acknowledge the valuable contribution by the crew and shipboard party of the JC10 cruise on the Research Vessel “James Cook” and the ROV ISIS pilots/engineers.
Conflict of interest
The authors declare that the research was conducted in the absence of any commercial or financial relationships that could be construed as a potential conflict of interest.
Publisher’s note
All claims expressed in this article are solely those of the authors and do not necessarily represent those of their affiliated organizations, or those of the publisher, the editors and the reviewers. Any product that may be evaluated in this article, or claim that may be made by its manufacturer, is not guaranteed or endorsed by the publisher.
Supplementary material
The Supplementary material for this article can be found online at: https://www.frontiersin.org/articles/10.3389/fmicb.2023.1157337/full#supplementary-material
Footnotes
References
Adam, P. S., Borrel, G., Brochier-Armanet, C., and Gribaldo, S. (2017). The growing tree of Archaea: new perspectives on their diversity, evolution and ecology. ISME J. 11, 2407–2425. doi: 10.1038/ismej.2017.122
Baker, B. J., De Anda, V., Seitz, K. W., Dombrowski, N., Santoro, A. E., and Lloyd, K. G. (2020). Diversity, ecology and evolution of Archaea. Nat. Microbiol. 5, 887–900. doi: 10.1038/s41564-020-0715-z
Baker, B. J., Saw, J. H., Lind, A. E., Lazar, C. S., Hinrichs, K. U., Teske, A. P., et al. (2016). Genomic inference of the metabolism of cosmopolitan subsurface Archaea, Hadesarchaea. Nat. Microbiol. 1:16002. doi: 10.1038/nmicrobiol.2016.2
Baloglanov, E. E., Abbasov, O. R., and Akhundov, R. V. (2018). Mud volcanoes of the world: classifications, activities and environmental hazard (informational-analytical review). Eur. J. Nat. Hist. 5, 12–26.
Beal, E. J., House, C. H., and Orphan, V. J. (2009). Manganese- and Iron-dependent marine methane oxidation. Science 325, 184–187. doi: 10.1126/science.1169984
Bertram, S., Blumenberg, M., Michaelis, W., Siegert, M., Krüger, M., and Seifert, R. (2013). Methanogenic capabilities of ANME-archaea deduced from 13C-labelling approaches. Environ. Microbiol. 15, 2384–2393. doi: 10.1111/1462-2920.12112
Beulig, F., Røy, H., Glombitza, C., and Jørgensen, B. B. (2018). Control on rate and pathway of anaerobic organic carbon degradation in the seabed. Proc. Natl. Acad. Sci. U.S.A. 115, 367–372. doi: 10.1073/pnas.1715789115
Borrel, G., Parisot, N., Harris, H. M. B., Peyretaillade, E., Gaci, N., Tottey, W., et al. (2014). Comparative genomics highlights the unique biology of Methanomassiliicoccales, a Thermoplasmatales-related seventh order of methanogenic archaea that encodes pyrrolysine. BMC Genomics 15:679. doi: 10.1186/1471-2164-15-679
Brennan, R. A., and Sanford, R. A. (2002). Continuous steady-state method using Tenax for delivering tetrachloroethene to chloro-respiring bacteria. Appl. Environ. Microbiol. 68, 1464–1467. doi: 10.1128/AEM.68.3.1464-1467.2002
Bueno de Mesquita, C. P., Wu, D., and Tringe, S. G. (2023). Methyl-based Methanogenesis: an ecological and genomic review. Microbiol. Mol. Biol. Rev. 87:e0002422. doi: 10.1128/mmbr.00024-22
Caporaso, J. G., Kuczynski, J., Stombaugh, J., Bittinger, K., Bushman, F. D., Costello, E. K., et al. (2010). QIIME allows analysis of high-throughput community sequencing data. Nat. Methods 7, 335–336. doi: 10.1038/nmeth.f.303
Caporaso, J. G., Lauber, C. L., Walters, W. A., Berg-Lyons, D., Lozupone, C. A., Turnbaugh, P. J., et al. (2011). Global patterns of 16S rRNA diversity at a depth of millions of sequences per sample. Proc. Natl. Acad. Sci. U.S.A. 108, 4516–4522. doi: 10.1073/pnas.1000080107
Chang, Y. H., Cheng, T. W., Lai, W. J., Tsai, W. Y., Sun, C. H., Lin, L. H., et al. (2012). Microbial methane cycling in a terrestrial mud volcano in eastern Taiwan. Environ. Microbiol. 14, 895–908. doi: 10.1111/j.1462-2920.2011.02658.x
Chivian, D., Brodie, E. L., Alm, E. J., Culley, D. E., Dehal, P. S., DeSantis, T. Z., et al. (2008). Environmental genomics reveals a single-species ecosystem deep within earth. Science 322, 275–278. doi: 10.1126/science.1155495
Claypool, G. E., and Kvenvolden, K. A. (1983). Methane and other hydrocarbon gases in marine sediment. Annu. Rev. Earth Planet. Sci. 11, 299–327. doi: 10.1146/annurev.ea.11.050183.001503
Coelho, F. J. R. C., Louvado, A., Domingues, P. M., Cleary, D. F. R., Ferreira, M., Almeida, A., et al. (2016). Integrated analysis of bacterial and microeukaryotic communities from differentially active mud volcanoes in the Gulf of Cádiz. Sci. Rep. 6:35272. doi: 10.1038/srep35272
Cragg, B. A., Bale, S. J., and Parkes, R. J. (1992). A novel method for the transport and long-term storage of cultures and samples in an anaerobic atmosphere. Lett. Appl. Microbiol. 15, 125–128. doi: 10.1111/j.1472-765X.1992.tb00743.x
Crill, P. M., and Martens, C. S. (1986). Methane production from bicarbonate and acetate in an anoxic marine sediment. Geochim. Cosmochim. Acta 50, 2089–2097. doi: 10.1016/0016-7037(86)90262-0
Cunha, M. R., Rodrigues, C. F., Génio, L., Hilário, A., Ravara, A., and Pfannkuche, O. (2013). Macrofaunal assemblages from mud volcanoes in the Gulf of Cádiz: abundance, biodiversity and diversity partitioning across spatial scales. Biogeosciences 10, 2553–2568. doi: 10.5194/bg-10-2553-2013
D’Amore, R., Ijaz, U. Z., Schirmer, M., Kenny, J., Gregory, R., Darby, A. C., et al. (2016). A comprehensive benchmarking study of protocols and sequencing platforms for 16S rRNA community profiling. BMC Genomics 17:55. doi: 10.1186/s12864-015-2194-9
DeSantis, T. Z., Hugenholtz, P., Larsen, N., Rojas, M., Brodie, E. L., Keller, K., et al. (2006). Greengenes, a chimera-checked 16S rRNA gene database and workbench compatible with ARB. Appl. Environ. Microbiol. 72, 5069–5072. doi: 10.1128/AEM.03006-05
Dhillon, A., Lever, M., Lloyd, K. G., Albert, D. B., Sogin, M. L., and Teske, A. (2005). Methanogen diversity evidenced by molecular characterization of methyl coenzyme M reductase A (mcrA) genes in hydrothermal sediments of the Guaymas Basin. Appl. Environ. Microbiol. 71, 4592–4601. doi: 10.1128/AEM.71.8.4592-4601.2005
Díaz-del-Rio, V., Somoza, L., Martinez-Frias, J., Mata, M. P., Delgado, A., Hernandez-Molina, F. J., et al. (2003). Vast fields of hydrocarbon-derived carbonate chimneys related to the accretionary wedge/olistostrome of the Gulf of Cádiz. Mar. Geol. 195, 177–200. doi: 10.1016/S0025-3227(02)00687-4
Dimitrov, L. I. (2002). Mud volcanoes - the most important pathway for degassing deeply buried sediments. Earth Sci. Rev. 59, 49–76. doi: 10.1016/S0012-8252(02)00069-7
Dimitrov, L. I. (2003). Mud volcanoes - a significant source of atmospheric methane. Geo-Mar. Lett. 23, 155–161. doi: 10.1007/s00367-003-0140-3
Dolfing, J., Larter, S. R., and Head, I. M. (2008). Thermodynamic constraints on methanogenic crude oil biodegradation. ISME J. 2, 442–452. doi: 10.1038/ismej.2007.111
Edgar, R. C. (2010). Search and clustering orders of magnitude faster than BLAST. Bioinformatics 26, 2460–2461. doi: 10.1093/bioinformatics/btq461
Etiope, G. (2009). Natural emissions of methane from geological seepage in Europe. Atmos. Environ. 43, 1430–1443. doi: 10.1016/j.atmosenv.2008.03.014
Etiope, G., Nakada, R., Tanaka, K., and Yoshida, N. (2011). Gas seepage from Tokamachi mud volcanoes, onshore Niigata Basin (Japan): origin, post-genetic alterations and CH4-CO2 fluxes. Appl. Geochem. 26, 348–359. doi: 10.1016/j.apgeochem.2010.12.008
Evans, P. N., Parks, D. H., Chadwick, G. L., Robbins, S. J., Orphan, V. J., Golding, S. D., et al. (2015). Methane metabolism in the archaeal phylum Bathyarchaeota revealed by genome-centric metagenomics. Science 350, 434–438. doi: 10.1126/science.aac7745
Farag, I. F., Davis, J. P., Youssef, N. H., and Elshahed, M. S. (2014). Global patterns of abundance, diversity and community structure of the Aminicenantes (candidate phylum OP8). PLoS One 9:e92139. doi: 10.1371/journal.pone.0092139
Fowler, S. J., Toth, C. R. A., and Gieg, L. M. (2016). Community structure in methanogenic enrichments provides insight into syntrophic interactions in hydrocarbon-impacted environments. Front. Microbiol. 7:562. doi: 10.3389/fmicb.2016.00562
Fry, J. C. (1988). “Determination of biomass” in Methods in aquatic bacteriology. ed. B. Austin (Chichester: John Wiley), 27–77.
Fry, J. C., Parkes, R. J., Cragg, B. A., Weightman, A. J., and Webster, G. (2008). Prokaryotic biodiversity and activity in the deep subseafloor biosphere. FEMS Microbiol. Ecol. 66, 181–196. doi: 10.1111/j.1574-6941.2008.00566.x
Gardner, J. (2001). Mud volcanoes revealed and sampled on the Western Moroccan continental Margin. Geophys. Res. Lett. 28, 339–342. doi: 10.1029/2000GL012141
Haffert, L., Haeckel, M., Liebetrau, V., Berndt, C., Hensen, C., Nuzzo, M., et al. (2013). Fluid evolution and authigenic mineral paragenesis related to salt diapirism - the Mercator mud volcano in the Gulf of Cádiz. Geochim. Cosmochim. Acta 106, 261–286. doi: 10.1016/j.gca.2012.12.016
Hallam, S. J., Girguis, P. R., Preston, C. M., Richardson, P. M., and DeLong, E. F. (2003). Identification of methyl coenzyme M reductase A (mcrA) genes associated with methane-oxidizing Archaea. Appl. Environ. Microbiol. 69, 5483–5491. doi: 10.1128/AEM.69.9.5483-5491.2003
Heijs, S. K., Haese, R. R., van der Wielen, P. W. J. J., Forney, L. J., and van Elsas, J. D. (2007). Use of 16S rRNA gene based clone libraries to assess microbial communities potentially involved in anaerobic methane oxidation in a Mediterranean cold seep. Microb. Ecol. 53, 384–398. doi: 10.1007/s00248-006-9172-3
Heijs, S. K., Laverman, A. M., Forney, L. J., Hardoim, P. R., and van Elsas, J. D. (2008). Comparison of deep-sea sediment microbial communities in the eastern Mediterranean. FEMS Microbiol. Ecol. 64, 362–377. doi: 10.1111/j.1574-6941.2008.00463.x
Hoshino, T., Toki, T., Ijiri, A., Morono, Y., Machiyama, H., Ashi, J., et al. (2017). Atribacteria from the subseafloor sedimentary biosphere disperse to the hydrosphere through submarine mud volcanoes. Front. Microbiol. 8:1135. doi: 10.3389/fmicb.2017.01135
Huse, S. M., Welch, D. M., Morrison, H. G., and Sogin, M. L. (2010). Ironing out the wrinkles in the rare biosphere through improved OTU clustering. Environ. Microbiol. 12, 1889–1898. doi: 10.1111/j.1462-2920.2010.02193.x
Ijiri, A., Inagaki, F., Kubo, Y., Adhikari, R. R., Hattori, S., Hoshino, T., et al. (2018). Deep-biosphere methane production stimulated by geofluids in the Nankai accretionary complex. Sci. Adv. 4:eaao4631. doi: 10.1126/sciadv.aao4631
Imachi, H., Aoi, K., Tasumi, E., Saito, Y., Yamanaka, Y., Saito, Y., et al. (2011). Cultivation of methanogenic community from subseafloor sediments using a continuous-flow bioreactor. ISME J. 5, 1913–1925. doi: 10.1038/ismej.2011.64
Inagaki, F., Nunoura, T., Nakagawa, S., Teske, A., Lever, M., Lauer, A., et al. (2006). Biogeographical distribution and diversity of microbes in methane hydrate-bearing deep marine sediments on the Pacific Ocean Margin. Proc. Natl. Acad. Sci. U.S.A. 103, 2815–2820. doi: 10.1073/pnas.0511033103
Jameson, E., Stephenson, J., Jones, H., Millard, A., Kaster, A. K., Purdy, K. J., et al. (2019). Deltaproteobacteria (Pelobacter) and Methanococcoides are responsible for choline-dependent methanogenesis in a coastal saltmarsh sediment. ISME J. 13, 277–289. doi: 10.1038/s41396-018-0269-8
Jiménez, N., Richnow, H. H., Vogt, C., Treude, T., and Krüger, M. (2016). Methanogenic hydrocarbon degradation: evidence from field and laboratory studies. J. Mol. Microbiol. Biotechnol. 26, 227–242. doi: 10.1159/000441679
Jones, H. J., Kröber, E., Stephenson, J., Mausz, M. A., Jameson, E., Millard, A., et al. (2019). A new family of uncultivated bacteria involved in methanogenesis from the ubiquitous osmolyte glycine betaine in coastal saltmarsh sediments. Microbiome 7:120. doi: 10.1186/s40168-019-0732-4
Joshi, NA, and Fass, JN (2011). Sickle: A sliding-window, adaptive, quality-based trimming tool for FastQ files [software].
Joye, S. B. (2020). “The geology and biogeochemistry of hydrocarbon seeps” in Annual review of earth and planetary sciences, vol. 48 (Annual Reviews Inc), 205–231. doi: 10.1146/annurev-arth-063016-020052
Joye, S. B., Samarkin, V. A., Orcutt, B. N., MacDonald, I. R., Hinrichs, K. U., Elvert, M., et al. (2009). Metabolic variability in seafloor brines revealed by carbon and Sulphur dynamics. Nat. Geosci. 2, 349–354. c
Judd, A. (2005). “Gas emissions from mud volcanoes” in Mud volcanoes, geodynamics and seismicity. eds. G. Martinelli and B. Panahi, NATO Science Series (Series IV: Earth and Environmental Series), vol. 51 (Dordrecht: Springer), 147–157.
Karnachuk, O. V., Frank, Y. A., Lukina, A. P., Kadnikov, V. V., Beletsky, A. V., Mardanov, A. V., et al. (2019). Domestication of previously uncultivated Candidatus Desulforudis audaxviator from a deep aquifer in Siberia sheds light on its physiology and evolution. ISME J. 13, 1947–1959. doi: 10.1038/s41396-019-0402-3
Katayama, T., Yoshioka, H., Kaneko, M., Amo, M., Fujii, T., Takahashi, H. A., et al. (2022). Cultivation and biogeochemical analyses reveal insights into methanogenesis in deep subseafloor sediment at a biogenic gas hydrate site. ISME J. 16, 1464–1472. doi: 10.1038/s41396-021-01175-7
Katayama, T., Yoshioka, H., Takahashi, H. A., Amo, M., Fujii, T., and Sakata, S. (2016). Changes in microbial communities associated with gas hydrates in subseafloor sediments from the Nankai Trough. FEMS Microbiol. Ecol. 92:fiw093. doi: 10.1093/femsec/fiw093
Kenyon, NH, Ivanov, MK, Akhmetzhanov, AM, and Akhmanov, GG (2000) Multidisciplinary study of geological processes on the North East Atlantic and Western Mediterranean Margins. IOC Technical Series No. 56.
King, G. M., Klug, M. J., and Lovely, D. R. (1983). Metabolism of acetate, methanol, and methylated amines in intertidal sediments of Lowes Cove, Maine. Appl. Environ. Microbiol. 45, 1848–1853. doi: 10.1128/aem.45.6.1848-1853.1983
Knittel, K., and Boetius, A. (2009). Anaerobic oxidation of methane: progress with an unknown process. Annu Rev Microbiol. 63, 311–34. doi: 10.1146/annurev.micro.61.080706.093130
Kopf, A. J. (2002). Significance of mud volcanism. Rev. Geophys. 40, 2-1–2-52. doi: 10.1029/2000RG000093
Kopf, A. J. (2003). Global methane emission through mud volcanoes and its past and present impact on the Earth's climate. Int. J. Earth Sci. 92, 806–816. doi: 10.1007/s00531-003-0341-z
Köpke, B., Wilms, R., Engelen, B., Cypionka, H., and Sass, H. (2005). Microbial diversity in coastal subsurface sediments – a cultivation approach using various electron acceptors and substrate gradients. Appl. Environ. Microbiol. 71, 7819–7830. doi: 10.1128/AEM.71.12.7819-7830.2005
Krüger, M., Treude, T., Wolters, H., Nauhaus, K., and Boetius, A. (2005). Microbial methane turnover in different marine habitats. Palaeogeogr. Palaeoclimatol. Palaeoecol. 227, 6–17. doi: 10.1016/j.palaeo.2005.04.031
L’Haridon, S., Chalopin, M., Colombo, D., and Toffin, L. (2014). Methanococcoides vulcani sp. nov., a marine methylotrophic methanogen that uses betaine, choline and N,N-dimethylethanolamine for methanogenesis, isolated from a mud volcano, and emended description of the genus Methanococcoides. Int. J. Syst. Evol. Microbiol. 64, 1978–1983. doi: 10.1099/ijs.0.058289-0
Lazar, C. S., L’Haridon, S., Pignet, P., and Toffin, L. (2011a). Archaeal populations in hypersaline sediments underlying orange microbial mats in the Napoli mud volcano. Appl. Environ. Microbiol. 77, 3120–3131. doi: 10.1128/AEM.01296-10
Lazar, C. S., Parkes, R. J., Cragg, B. A., L’Haridon, S., and Toffin, L. (2011b). Methanogenic diversity and activity in hypersaline sediments of the Centre of the Napoli mud volcano, eastern Mediterranean Sea. Environ. Microbiol. 13, 2078–2091. doi: 10.1111/j.1462-2920.2011.02425.x
Lazar, C. S., Parkes, R. J., Cragg, B. A., L’Haridon, S., and Toffin, L. (2012). Methanogenic activity and diversity in the Centre of the Amsterdam mud volcano, Eastern Mediterranean Sea. FEMS Microbiol. Ecol. 81, 243–254. doi: 10.1111/j.1574-6941.2012.01375.x
Lee, D. H., Lee, Y. M., Kim, J. H., Jin, Y. K., Paull, C., Niemann, H., et al. (2019). Discriminative biogeochemical signatures of methanotrophs in different chemosynthetic habitats at an active mud volcano in the Canadian Beaufort Sea. Sci. Rep. 9:17592. doi: 10.1038/s41598-019-53950-4
León, R., Somoza, L., Medialdea, T., Vázquez, J. T., González, F. J., López-González, N., et al. (2012). New discoveries of mud volcanoes on the Moroccan Atlantic continental margin (Gulf of Cádiz): morpho-structural characterization. Geo-Mar. Lett. 32, 473–488. doi: 10.1007/s00367-012-0275-1
Liu, Y., and Whitman, W. B. (2008). Metabolic, phylogenetic, and ecological diversity of the methanogenic Archaea. Ann. N. Y. Acad. Sci. 1125, 171–189. doi: 10.1196/annals.1419.019
Lösekann, T., Robador, A., Niemann, H., Knittel, K., Boetius, A., and Dubilier, N. (2008). Endosymbioses between bacteria and deep-sea siboglinid tubeworms from an Arctic cold seep (Haakon Mosby mud volcano, Barents Sea). Environ. Microbiol. 10, 3237–3254. doi: 10.1111/j.1462-2920.2008.01712.x
Lyimo, T. J., Pol, A., Jetten, M. S. M., and Op den Camp, H. J. M. (2009). Diversity of methanogenic archaea in a mangrove sediment and isolation of a new Methanococcoides strain. FEMS Microbiol. Lett. 291, 247–253. doi: 10.1111/j.1574-6968.2008.01464.x
Magalhães, V. H., Pinheiroa, L. M., Ivanov, M. K., Kozlova, E., Blinova, V., Kolganova, J., et al. (2012). Formation processes of methane-derived authigenic carbonates from the Gulf of Cádiz. Sediment. Geol. 243-244, 155–168. doi: 10.1016/j.sedgeo.2011.10.013
Mah, R. A., Ward, D. M., Baresi, L., and Glass, T. L. (1977). Biogenesis of methane. Annu. Rev. Microbiol. 31, 309–341. doi: 10.1146/annurev.mi.31.100177.001521
Maignien, L., Parkes, R. J., Cragg, B., Niemann, H., Knittel, K., Coulon, S., et al. (2013). Anaerobic oxidation of methane in hypersaline cold seep sediments. FEMS Microbiol. Ecol. 83, 214–231. doi: 10.1111/j.1574-6941.2012.01466.x
Maldonado, A., Somoza, L., and Pallares, L. (1999). The Betic orogen and the Iberian–African boundary in the Gulf of Cadiz: geological evolution (Central North Atlantic). Mar. Geol. 155, 9–43. doi: 10.1016/S0025-3227(98)00139-X
Martin, M. (2011). Cutadapt removes adapter sequences from high-throughput sequencing reads. EMBnet j. 17, 10–12. doi: 10.14806/ej.17.1.200
Martinez, R. J., Mills, H. J., Story, S., and Sobecky, P. A. (2006). Prokaryotic diversity and metabolically active microbial populations in sediments from an active mud volcano in the Gulf of Mexico. Environ. Microbiol. 8, 1783–1796. doi: 10.1111/j.1462-2920.2006.01063.x
Mazzini, A., and Etiope, G. (2017). Mud volcanism: an updated review. Earth Sci. Rev. 168, 81–112. doi: 10.1016/j.earscirev.2017.03.001
Medialdea, T., Somoza, L., Pinheiro, L. M., Fernández-Puga, M. C., Vázquez, J. T., León, R., et al. (2009). Tectonics and mud volcano development in the Gulf of Cádiz. Mar. Geol. 261, 48–63. doi: 10.1016/j.margeo.2008.10.007
Meng, J., Xu, J., Qin, D., He, Y., Xiao, X., and Wang, F. (2014). Genetic and functional properties of uncultivated MCG archaea assessed by metagenome and gene expression analyses. ISME J. 8, 650–659. doi: 10.1038/ismej.2013.174
Milkov, A. V. (2000). Worldwide distribution of submarine mud volcanoes and associated gas hydrates. Mar. Geol. 167, 29–42. doi: 10.1016/S0025-3227(00)00022-0
Niemann, H., Duarte, J., Hensen, C., Omoregie, E., Magalhães, V. H., Elvert, M., et al. (2006a). Microbial methane turnover at mud volcanoes of the Gulf of Cádiz. Geochim. Cosmochim. Acta 70, 5336–5355. doi: 10.1016/j.gca.2006.08.010
Niemann, H., Lösekann, T., de Beer, D., Elvert, M., Nadalig, T., Knittel, K., et al. (2006b). Novel microbial communities of the Haakon Mosby mud volcano and their role as a methane sink. Nature 443, 854–858. doi: 10.1038/nature05227
Nobu, M. K., Dodsworth, J. A., Murugapiran, S. K., Rinke, C., Gies, E. A., Webster, G., et al. (2016). Phylogeny and physiology of candidate phylum ‘Atribacteria’ (OP9/JS1) inferred from cultivation-independent genomics. ISME J. 10, 273–286. doi: 10.1038/ismej.2015.97
Nottingham, P. M., and Hungate, R. E. (1969). Methanogenic fermentation of benzoate. J. Bacteriol. 98, 1170–1172. doi: 10.1128/jb.98.3.1170-1172.1969
Nuzzo, M., Hornibrook, E. R. C., Gill, F., Hensen, C., Pancost, R., Haeckel, M., et al. (2009). Origin of light volatile hydrocarbon gases in mud volcano fluids, Gulf of Cádiz - evidence for multiple sources and transport mechanisms in active sedimentary wedges. Chem. Geol. 266, 350–363. doi: 10.1016/j.chemgeo.2009.06.023
Nuzzo, M., Tomonaga, Y., Schmidt, M., Valadares, V., Faber, E., Piñero, E., et al. (2019). Formation and migration of hydrocarbons in deeply buried sediments of the Gulf of Cádiz convergent plate boundary - insights from the hydrocarbon and helium isotope geochemistry of mud volcano fluids. Mar. Geol. 410, 56–69. doi: 10.1016/j.margeo.2019.01.005
Omoregie, E. O., Mastalerz, V., de Lange, G., Straub, K. L., Kappler, A., Røy, H., et al. (2008). Biogeochemistry and community composition of iron- and sulfur-precipitating microbial mats at the Chefren mud volcano (Nile Deep Sea Fan, Eastern Mediterranean). Appl. Environ. Microbiol. 74, 3198–3215. doi: 10.1128/AEM.01751-07
Orcutt, B. N., Joye, S. B., Kleindienst, S., Knittel, K., Ramette, A., Rietz, A., et al. (2010). Impact of natural oil and higher hydrocarbons on microbial diversity, distribution, and activity in Gulf of Mexico cold-seep sediments. Deep-Sea Res. II Top. Stud. Oceanogr. 57, 2008–2021. doi: 10.1016/j.dsr2.2010.05.014
Oremland, R. S., Marsh, L. M., and Polcin, S. (1982). Methane production and simultaneous sulphate reduction in anoxic, salt marsh sediments. Nature 296, 143–145. doi: 10.1038/296143a0
O’Sullivan, L. A., Webster, G., Fry, J. C., Parkes, J. C., and Weightman, A. J. (2008). Modified linker-PCR primers facilitate complete sequencing of DGGE DNA fragments. J. Microbiol. Methods 75, 579–581. doi: 10.1016/j.mimet.2008.08.006
Pachiadaki, M. G., Kallionaki, A., Dählmann, A., de Lange, G. J., and Kormas, K. A. (2011). Diversity and spatial distribution of prokaryotic communities along a sediment vertical profile of a deep-sea mud volcano. Microb. Ecol. 62, 655–668. doi: 10.1007/s00248-011-9855-2
Pachiadaki, M. G., and Kormas, K. A. (2013). Interconnectivity vs. isolation of prokaryotic communities in European deep-sea mud volcanoes. Biogeosciences 10, 2821–2831. doi: 10.5194/bg-10-2821-2013
Pachiadaki, M. G., Lykousis, V., Stefanou, E. G., and Kormas, K. A. (2010). Prokaryotic community structure and diversity in the sediments of an active submarine mudvolcano (Kazan mud volcano, East Mediterranean Sea). FEMS Microbiol. Ecol. 72, 429–444. doi: 10.1111/j.1574-6941.2010.00857.x
Palomino, D., López-González, N., Vázquez, J. T., Fernández-Salas, L. M., Rueda, J. L., Sánchez-Leal, R., et al. (2016). Multidisciplinary study of mud volcanoes and diapirs and their relationship to seepages and bottom currents in the Gulf of Cádiz continental slope (northeastern sector). Mar. Geol. 378, 196–212. doi: 10.1016/j.margeo.2015.10.001
Pancost, R. D., Sinninghe Damste, J. S., de Lint, S., van der Maarel, M. J. E. C., and Gottschal, J. C., the Medinaut Shipboard Scientific Party (2000). Biomarker evidence for widespread anaerobic methane oxidation in Mediterranean sediments by a consortium of methanogenic Archaea and Bacteria. Appl. Environ. Microbiol. 66, 1126–1132. doi: 10.1128/AEM.66.3.1126-1132.2000
Parkes, R. J., Cragg, B. A., Banning, N., Brock, F., Webster, G., Fry, J. C., et al. (2007). Biogeochemistry and biodiversity of methane cyclingin subsurface marine sediments (Skagerrak, Denmark). Environ. Microbiol. 9, 1146–1161. doi: 10.1111/j.1462-2920.2006.01237.x
Parkes, R. J., Cragg, B., Roussel, E., Webster, G., Weightman, A., and Sass, H. (2014). A review of prokaryotic populations and processes in sub-seafloor sediments, including biosphere:geosphere interactions. Mar. Geol. 352, 409–425. doi: 10.1016/j.margeo.2014.02.009
Parkes, R. J., Cragg, B. A., and Wellsbury, P. (2000). Recent studies on bacterial populations and processes in subseafloor sediments: a review. Hydrogeol. J. 8, 11–28. doi: 10.1007/PL00010971
Parkes, R. J., Sass, H., Webster, G., Watkins, A. J., Weightman, A. J., O’Sullivan, L. A., et al. (2010). “Methods for studying methanogens and methanogenesis in marine sediments” in Handbook of hydrocarbon and lipid microbiology. ed. K. N. Timmis (Berlin: Springer-Verlag), 3799–3826.
Perez-Garcia, C., Berndt, C., Klaeschen, D., Mienert, J., Haffert, L., Depreiter, D., et al. (2011). Linked halokinesis and mud volcanism at the Mercator mud volcano, gulf of Cádiz. J. Geophys. Res. 116:B05101. doi: 10.1029/2010JB008061
Pimenov, N., Savvichev, A., Rusanov, I., Lein, A., Egorov, A., Gebruk, A., et al. (1999). Microbial processes of carbon cycle as the base of food chain of Håkon Mosby mud volcano benthic community. Geo-Mar. Lett. 19, 89–96. doi: 10.1007/s003670050097
Pinheiro, L. M., Ivanov, M. K., Sautkin, A., Akhmanov, G., Magalhães, V. H., Volkonskaya, A., et al. (2003). Mud volcanism in the Gulf of Cádiz: results from the TTR-10 cruise. Mar. Geol. 195, 131–151. doi: 10.1016/S0025-3227(02)00685-0
Purdy, K. J., Munson, M. A., Cresswell-Maynard, T., Nedwell, D. B., and Embley, T. M. (2003). Use of 16S rRNA-targeted oligonucleotide probes to investigate function and phylogeny of sulphate-reducing bacteria and methanogenic archaea in a UK estuary. FEMS Microbiol. Ecol. 44, 361–371. doi: 10.1016/S0168-6496(03)00078-3
Rinke, C., Rubino, F., Messer, L. F., Youssef, N., Parks, D. H., Chuvochina, M., et al. (2019). A phylogenomic and ecological analysis of the globally abundant marine group II archaea (Ca. Poseidoniales ord. nov.). ISME J. 13, 663–675. doi: 10.1038/s41396-018-0282-y
Rodrigues, C. F., Hilário, A., Cunha, M. R., Weightman, A. J., and Webster, G. (2011). Microbial diversity in Frenulata (Siboglinidae, Polychaeta) species from mud volcanoes in the Gulf of Cádiz (NE Atlantic). Antonie Van Leeuwenhoek 100, 83–98. doi: 10.1007/s10482-011-9567-0
Rodrigues, C. F., Webster, G., Cunha, M. R., Duperron, S., and Weightman, A. J. (2010). Chemosynthetic bacteria found in bivalve species from mud volcanoes of the Gulf of Cádiz. FEMS Microbiol. Ecol. 73, 486–499. doi: 10.1111/j.1574-6941.2010.00913.x
Roussel, E. G., Sauvadet, A. L., Allard, J., Chaduteau, C., Richard, P., Bonavita, M. A. C., et al. (2009). Archaeal methane cycling communities associated with gassy subsurface sediments of Marennes-Oléron Bay (France). Geomicrobiol J. 26, 31–43. doi: 10.1080/01490450802599284
Ruff, S. E., Felden, J., Gruber-Vodicka, H. R., Marcon, Y., Knittel, K., Ramette, A., et al. (2019). In situ development of a methanotrophic microbiome in deep-sea sediments. ISME J. 13, 197–213. doi: 10.1038/s41396-018-0263-1
Sauter, E. J., Muyakshin, S. I., Charlou, J. L., Schlüter, M., Boetius, A., Jerosch, K., et al. (2006). Methane discharge from a deep-sea submarine mud volcano into the upper water column by gas hydrate-coated methane bubbles. Earth Planet. Sci. Lett. 243, 354–365. doi: 10.1016/j.epsl.2006.01.041
Sogin, M. L., Morrison, H. G., Huber, J. A., Welch, D. M., Huse, S. M., Neal, P. R., et al. (2006). Microbial diversity in the deep sea and the underexplored “rare biosphere”. Proc. Natl. Acad. Sci. U.S.A. 103, 12115–12120. doi: 10.1073/pnas.0605127103
Somoza, L., Díaz-del-Rio, V., León, R., Ivanov, M., Fernández-Puga, M. C., Gardner, J. M., et al. (2003). Seabed morphology and hydrocarbon seepage in the Gulf of Cádiz mud volcano area: acoustic imagery, multibeam and ultra-high resolution seismic data. Mar. Geol. 195, 153–176. doi: 10.1016/S0025-3227(02)00686-2
Stams, A. J. M., and Hansen, T. A. (1984). Fermentation of glutamate and other compounds by Acidaminobacter hydrogenoformans gen.Nov., sp.nov., an obligate anaerobe isolated from black mud. Studies with pure cultures and mixed cultures with sulfate-reducing and methanogenic bacteria. Arch. Microbiol. 137, 329–337. doi: 10.1007/BF00410730
Stams, A. J. M., and Plugge, C. M. (2009). Electron transfer in syntrophic communities of anaerobic bacteria and archaea. Nat. Rev. Microbiol. 7, 568–577. doi: 10.1038/nrmicro2166
Stewart, F. J., Newton, I. L. G., and Cavanaugh, C. M. (2005). Chemosynthetic endosymbioses adaptations to oxic-anoxic interfaces. Trends Microbiol. 13, 439–448. doi: 10.1016/j.tim.2005.07.007
Takai, K., Moser, D. P., DeFlaun, M., Onstott, T. C., and Fredrickson, J. K. (2001). Archaeal diversity in waters from deep South African gold mines. Appl. Environ. Microbiol. 67, 5750–5760. doi: 10.1128/AEM.67.21.5750-5760.2001
Teske, A., and Sørensen, K. B. (2008). Uncultured archaea in deep marine subsurface sediments: have we caught them all? ISME J. 2, 3–18. doi: 10.1038/ismej.2007.90
Teske, A., Wegener, G., Chanton, J. P., White, D., Macgregor, B., Hoer, D., et al. (2021). Microbial communities under distinct thermal and geochemical regimes in axial and off-axis sediments of Guaymas Basin. Front. Microbiol. 12:633649. doi: 10.3389/fmicb.2021.633649
Valentine, D. L. (2011). Emerging topics in marine methane biogeochemistry. Annu. Rev. Mar. Sci. 3, 147–171. doi: 10.1146/annurev-marine-120709-142734
Vanwonterghem, I., Evans, P. N., Parks, D. H., Jensen, P. D., Woodcroft, B. J., Hugenholtz, P., et al. (2016). Methylotrophic methanogenesis discovered in the archaeal phylum Verstraetearchaeota. Nat. Microbiol. 1:16170. doi: 10.1038/nmicrobiol.2016.170
Vigneron, A., L'Haridon, S., Godfroy, A., Roussel, E. G., Cragg, B. A., Parkes, R. J., et al. (2015). Evidence of active methanogen communities in shallow sediments of the Sonora Margin cold seeps. Appl. Environ. Microbiol. 81, 3451–3459. doi: 10.1128/AEM.00147-15
Wallmann, K., Drews, M., Aloisi, G., and Bohrmann, G. (2006). Methane discharge into the Black Sea and the global ocean via fluid flow through submarine mud volcanoes. Earth Planet. Sci. Lett. 248, 545–560. doi: 10.1016/j.epsl.2006.06.026
Watkins, A. J., Roussel, E. G., Parkes, R. J., and Sass, H. (2014). Glycine betaine as a direct substrate for methanogens (Methanococcoides spp.). Appl. Environ. Microbiol. 80, 289–293. doi: 10.1128/AEM.03076-13
Watkins, A. J., Roussel, E. G., Webster, G., Parkes, R. J., and Sass, H. (2012). Choline and NN-dimethylethanolamine as direct substrates for methanogens. Appl. Environ. Microbiol. 78, 8298–8303. doi: 10.1128/AEM.01941-12
Webster, G., Blazejak, A., Cragg, B. A., Schippers, A., Sass, H., Rinna, J., et al. (2009). Subsurface microbiology and biogeochemistry of a deep, cold-water carbonate mound from the porcupine Seabight (IODP expedition 307). Environ. Microbiol. 11, 239–257. doi: 10.1111/j.1462-2920.2008.01759.x
Webster, G., Mullins, A. J., Watkins, A. J., Cunningham-Oakes, E., Weightman, A. J., Mahenthiralingam, E., et al. (2019). Genome sequences of two choline-utilizing methanogenic archaea, Methanococcoides spp., isolated from marine sediments. Microbiol. Resour. Announc. 8, e00342–e00319. doi: 10.1128/MRA.00342-19
Webster, G., Newberry, C. J., Fry, J. C., and Weightman, A. J. (2003). Assessment of bacterial community structure in the deep sub-seafloor biosphere by 16S rDNA-based techniques: a cautionary tale. J. Microbiol. Methods 55, 155–164. doi: 10.1016/S0167-7012(03)00140-4
Webster, G., O'Sullivan, L. A., Meng, Y., Williams, A. S., Sass, A. M., Watkins, A. J., et al. (2015). Archaeal community diversity and abundance changes along a natural salinity gradient in estuarine sediments. FEMS Microbiol. Ecol. 91, 1–18. doi: 10.1093/femsec/fiu025
Webster, G., Parkes, R. J., Cragg, B. A., Newberry, C. J., Weightman, A. J., and Fry, J. C. (2006). Prokaryotic community composition and biogeochemical processes in deep subseafloor sediments from the Peru Margin. FEMS Microbiol. Ecol. 58, 65–85. doi: 10.1111/j.1574-6941.2006.00147.x
Webster, G., Parkes, R. J., Fry, J. C., and Weightman, A. J. (2004). Widespread occurrence of a novel division of bacteria identified by 16 S rRNA gene sequences originally found in deep marine sediments. Appl. Environ. Microbiol. 70, 5708–5713. doi: 10.1128/AEM.70.9.5708-5713.2004
Webster, G., Sass, H., Cragg, B. A., Gorra, R., Knab, N. J., Green, C. J., et al. (2011). Enrichment and cultivation of prokaryotes associated with the sulphate-methane transition zone of diffusion-controlled sediments of Aarhus Bay, Denmark, under heterotrophic conditions. FEMS Microbiol. Ecol. 77, 248–263. doi: 10.1111/j.1574-6941.2011.01109.x
Wellsbury, P., Mather, I., and Parkes, R. J. (2002). Geomicrobiology of deep, low organic carbon sediments in the Woodlark Basin, Pacific Ocean. FEMS Microbiol. Ecol. 42, 59–70. doi: 10.1111/j.1574-6941.2002.tb00995.x
Whiticar, M. J., Faber, E., and Schoell, M. (1986). Biogenic methane formation in marine and freshwater environments: CO2 reduction vs. acetate fermentation-isotope evidence. Geochim. Cosmochim. Acta 50, 693–709. doi: 10.1016/0016-7037(86)90346-7
Xiao, K. Q., Moore, O. W., Babakhani, P., Curti, L., and Peacock, C. L. (2022). Mineralogical control on methylotrophic methanogenesis and implications for cryptic methane cycling in marine surface sediment. Nat. Commun. 13:272. doi: 10.1038/s41467-022-30422-4
Xu, L., Zhuang, G. C., Montgomery, A., Liang, Q., Joye, S. B., and Wang, F. (2021). Methyl-compounds driven benthic carbon cycling in the sulfate-reducing sediments of South China Sea. Environ. Microbiol. 23, 641–651. doi: 10.1111/1462-2920.15110
Yanagawa, K., Sunamura, M., Lever, M. A., Morono, Y., Hiruta, A., Ishizaki, O., et al. (2011). Niche separation of methanotrophic Archaea (ANME-1 and -2) in methane-seep sediments of the eastern Japan Sea offshore Joetsu. Geomicrobiol J. 28, 118–129. doi: 10.1080/01490451003709334
Yanagawa, K., Tani, A., Yamamoto, N., Hachikubo, A., Kano, A., Matsumoto, R., et al. (2016). Biogeochemical cycle of methanol in anoxic deep-sea sediments. Microbes Environ. 31, 190–193. doi: 10.1264/jsme2.ME15204
Yoshioka, H., Maruyama, A., Nakamura, T., Higashi, Y., Fuse, H., Sakata, S., et al. (2010). Activities and distribution of methanogenic and methane-oxidizing microbes in marine sediments from the Cascadia margin. Geobiology 8, 223–233. doi: 10.1111/j.1472-4669.2009.00231.x
Zengler, K., Richnow, H. H., Rosselló-Mora, R., Michaelis, W., and Widdel, F. (1999). Methane formation from long-chain alkanes by anaerobic microorganisms. Nature 401, 266–269. doi: 10.1038/45777
Keywords: methanogens, marine sediments, biogeochemical cycles, mud volcanoes, Archaea , anaerobic methane oxidation, Bacteria
Citation: Webster G, Cragg BA, Rinna J, Watkins AJ, Sass H, Weightman AJ and Parkes RJ (2023) Methanogen activity and microbial diversity in Gulf of Cádiz mud volcano sediments. Front. Microbiol. 14:1157337. doi: 10.3389/fmicb.2023.1157337
Edited by:
Yuki Morono, Japan Agency for Marine-Earth Science and Technology (JAMSTEC), JapanReviewed by:
Taiki Katayama, National Institute of Advanced Industrial Science and Technology (AIST), JapanGunter Wegener, University of Bremen, Germany
Copyright © 2023 Webster, Cragg, Rinna, Watkins, Sass, Weightman and Parkes. This is an open-access article distributed under the terms of the Creative Commons Attribution License (CC BY). The use, distribution or reproduction in other forums is permitted, provided the original author(s) and the copyright owner(s) are credited and that the original publication in this journal is cited, in accordance with accepted academic practice. No use, distribution or reproduction is permitted which does not comply with these terms.
*Correspondence: Gordon Webster, d2Vic3RlcmdAY2FyZGlmZi5hYy51aw==
†Deceased