- Division of Biological Science, Graduate School of Science and Technology, Nara Institute of Science and Technology, Ikoma, Japan
In eukaryotic species, dysfunction of the endoplasmic reticulum (ER), namely, ER stress, provokes a cytoprotective transcription program called the unfolded protein response (UPR). The UPR is triggered by transmembrane ER-stress sensors, including Ire1, which acts as an endoribonuclease to splice and mature the mRNA encoding the transcription factor Hac1 in many fungal species. Through analyses of the methylotrophic yeast Pichia pastoris (syn. Komagataella phaffii), we revealed a previously unknown function of Ire1. In P. pastoris cells, the IRE1 knockout mutation (ire1Δ) and HAC1 knockout mutation (hac1Δ) caused only partially overlapping gene expression changes. Protein aggregation and the heat shock response (HSR) were induced in ire1Δ cells but not in hac1Δ cells even under non-stress conditions. Moreover, Ire1 was further activated upon high-temperature culturing and conferred heat stress resistance to P. pastoris cells. Our findings cumulatively demonstrate an intriguing case in which the UPR machinery controls cytosolic protein folding status and the HSR, which is known to be activated upon the accumulation of unfolded proteins in the cytosol and/or nuclei.
Introduction
The endoplasmic reticulum (ER) is an interconnected network of flattened or tubular sacs that are commonly found in eukaryotic cells and serves as a site for the folding and modification of secretory and transmembrane proteins. ER-resident molecular chaperones, such as BiP, assist in the folding of ER client proteins translocated from the cytosol (Pobre et al., 2019). The ER also contains various protein-modification enzymes for disulfide bond formation, such as protein disulfide isomerase (PDI) and Ero1, and for N-linked glycosylation (Braakman and Hebert, 2013). After normal folding and modification, ER client proteins are packed into transport vesicles and transported to the cell surface or other membranous organelles via the Golgi apparatus.
Dysfunction or functional deficiency of the ER is collectively called ER stress, which is frequently accompanied by the accumulation of unfolded proteins in the ER. Excessive load of secretory proteins into the ER is a prominent example of ER stress stimuli. Chemicals or antibiotics that cleave disulfide bonds or inhibit N-linked glycosylation also cause ER stress. In response to ER stress, eukaryotic cells induce a cytoprotective gene induction program called the unfolded protein response (UPR) (Mori, 2009).
The intracellular signaling pathway of the UPR was initially revealed through frontier studies using the yeast Saccharomyces cerevisiae as a model organism (Le and Kimata, 2021). Ire1 is an ER-resident type-I transmembrane protein that carries dual enzymatic activities, Ser/Thr protein kinase and endoribonuclease (RNase). Upon ER stress, Ire1 is self-associated and auto-phosphorylated, thus leading to its activation as an RNase that splices the HAC1 gene transcript (Sidrauski and Walter, 1997; Lee et al., 2008; Ishiwata-Kimata et al., 2013). The spliced form of HAC1 mRNA is then translated into a transcription factor that induces a number of genes, such as KAR2 (the BiP-encoding gene), PDI1 (the PDI-encoding gene), and ERO1, many of which support functions of the ER and protein secretory pathway (Cox and Walter, 1996; Travers et al., 2000; Kimata et al., 2006).
In contrast, when not spliced by Ire1, HAC1 mRNA is poorly translated and virtually functionless at least in S. cerevisiae cells (Mori et al., 2000; Rüegsegger et al., 2001; Di Santo et al., 2016). Moreover, according to Niwa et al. (2005), HAC1 mRNA is the sole target of Ire1. These insights indicate that the functions of IRE1 and HAC1 are highly interdependent. As IRE1 and HAC1 precisely belong to the same epistatic group, knockout mutations of these two genes exhibit identical phenotypes and do not show additive or synergistic effects (Travers et al., 2000; Schuldiner et al., 2005).
Also in animal and plant cells, Ire1 is involved in the splicing of mRNAs encoding transcription factors, namely XBP1 in metazoans and bZIP60 in plants (Tran and Kimata, 2018). On the other hand, Ire1 promotes the degradation of mRNAs that mainly encode ER client proteins in these species (Coelho and Domingos, 2014). This reaction is called the regulated Ire1-dependent decay (RIDD) and likely contributes to mitigating the protein load to the ER.
Ire1 exclusively performs the RIDD in Schizosaccharomyces pombe cells, which do not carry HAC1-gene orthologue (Kimmig et al., 2012). In some other fungal species carrying HAC1-gene orthologues, Ire1 may also have a role(s) other than splicing of the HAC1 transcript. According to Feng et al. (2011), the IRE1 gene (IreA) knockout mutant of the filamentous fungus Aspergillus fumigatus exhibits more severe defect in the virulence than the HAC1 gene (HacA) knockout mutant. Moreover, the IRE1 knockout mutant of the pathogenic yeast Candida albicans was reported to be more sensitive to iron depletion than the HAC1 knockout mutant (Ramírez-Zavala et al., 2022). Nevertheless, it is unclear how Ire1 functions in these cases.
Pichia pastoris (alias Komagataella phaffi) has various unique properties that are not seen in S. cerevisiae (Ata et al., 2021), while both yeast species belong to the same taxonomic family of Saccharomycetaceae. For instance, probably because of the high secretion of endogenous proteins, Ire1 is partially but considerably activated even under healthy growing and non-stressed conditions in P. pastoris cells (Fauzee et al., 2020). Also considering that P. pastoris is widely used for the production of heterologous secretory proteins (Puxbaum et al., 2015), we believe that the functions of its UPR-related proteins are intriguing research topic. In the present study, we therefore explored the HAC1-dependent and HAC1-independent physiological roles of Ire1 in P. pastoris cells. Our findings cumulatively indicate that in P. pastoris cells, Ire1 functions to attenuate cytosolic protein aggregation and the heat shock response (HSR) as well as to perform HAC1 mRNA splicing.
Materials and methods
Genetic manipulation of Pichia pastoris cells
We used P. pastoris CBS7435 as the wild-type (WT) strain (Küberl et al., 2011). For transformation of P. pastoris cells, they were electroporated as described in Wu and Letchworth (2004). Genomic DNA samples were extracted using the Dr. GenTLE kit (Takara Bio, Kusatsu, Japan).
For CRISPR/Cas9-based genome editing, we used the plasmid BB3cK_pGAP_23*_pPFK300_Cas9, which carries the Cas9 nuclease gene, guide RNA expression module, and G418-resistant kanMX marker (Gassler et al., 2019). DNA fragments carrying the guide RNA sequences (Supplementary Table S1) were synthesized by Twist Bioscience (South San Francisco, CA, United States) and ligated with BbsI-digested BB3cK_pGAP_23*_pPFK300_Cas9 using the Gibson assembly kit (New England Biolabs, Ipswich, MA, USA). To generate a donor DNA construct for full-length IRE1 gene deletion (ire1Δ0 mutation), 5′- and 3′-flanking regions of the IRE1 gene were PCR-amplified from genomic DNA using primer sets I1/I4 and I3/I2 (Supplementary Table S2), fused using the Gibson assembly kit, and PCR-amplified again using primer set I5/I6 (Supplementary Table S2). To generate a donor DNA construct for the full-length HAC1 gene deletion (hac1Δ0 mutation), 5′- and 3′-flanking regions of the HAC1 gene were PCR-amplified from genomic DNA using primer sets H1/H6 and H5/H2 (Supplementary Table S2), fused using the Gibson assembly kit, and PCR-amplified again using primer set H7/H8 (Supplementary Table S2). Subsequently, 1 μg of the resulting guide RNA/Cas9 expression plasmid and 5 μg of the resulting donor DNA construct were mixed and used to transform P. pastoris cells. The G418-resistant transformant clones were subjected to genomic PCR analysis using primer set I1/I2 or H1/H2 for confirmation of the ire1Δ0 or hac1Δ0 mutation and grown in YPD not containing G418 to eliminate the guide RNA/Cas9 expression plasmid.
We also knocked out the HAC1 gene and IRE1 gene through genomic insertion of the kanMX marker. Using primer sets H9/H10 and H11/H12 (Supplementary Table S3), partial fragments of the HAC1 gene were PCR-amplified from genomic DNA. The kanMX marker was PCR-amplified from BB3cK_pGAP_23*_pPFK300_Cas9 using primer set H13/H14 (Supplementary Table S3). These three PCR products were fused using the Gibson assembly kit and amplified again using the primer set H3/H4 (Supplementary Table S3). Subsequently, the resulting hac1::kanMX gene disruption module (HAC1-fragment (first half)-kanMX-HAC1 fragment (latter half)) was used to transform P. pastoris cells, and the G418-resistant transformant clones were subjected to genomic PCR analysis using the primer set H3/H4 to confirm the hac1::kanMX mutation. The zeocin-resistant marker on the IRE1-knockout module described in our previous publication (Fauzee et al., 2020) was replaced with the kanMX marker to generate the ire1::kanMX allele.
The plasmid pAHYB-GFP was previously created for GFP expression from the AOX1 promoter in P. pastoris cells (Yang et al., 2014). The GAP1 promoter sequence was PCR-amplified from P. pastoris genome using oligonucleotide primer sets ccaagcagatctCTCTGCTACTCTGGTCCCAAGTG and ggctacggtaccTGTGTTTTGATAGTTGTTCAATT [capital letters: sequence for annealing to the GAP1 promoter region, underlined letters: artificially attached restriction sites (BglII and KpnI)]. Then, the PCR product and pAHYB-GFP were digested with BglII and KpnI and ligated, and the resulting plasmid was named pGHYB-GFP, which was used for GFP expression from the GAP1 promoter. To create the plasmid pGHYB-nGFP, a nuclear localization signal (NLS)-encoding sequence [CCAAAGAAGAAAAGAAAAGTT (corresponding to ProLysLysLysArgLysVal)] was in-frame inserted into the C-terminal position of the GFP-coding region on pGHYB-GFP. After linearization by cutting with BamHI, pGHYB-GFP and pGHYB-nGFP were used to transform P. pastoris strains.
Growth and stress exposure of Pichia pastoris cells
For culturing P. pastoris cells, we used glucose-based rich medium (YPD medium) containing 1% yeast extract, 2% Bacto peptone, and 2% glucose. Dithiothreitol (DTT) and tunicamycin were purchased from Tokyo Chemical Industry (Tokyo, Japan) and Sigma-Aldrich (Merck KGaA, Darmstadt, Germany), respectively. For agar plates, YPD was solidified with 2% agar. A spectrophotometer SmartSpec 3,000 (BioRad, Hercules, CA, United States) was used to monitor optical density (OD600) of the cultures.
Unless otherwise noted, YPD cultures of P. pastoris were aerobically shaken at 30°C, and cells in the exponential growth phase were collected. To obtain DTT-treated cells, DTT solution (1 M in water) was added to YPD cultures, which were further shaken at 30°C for 30 min. For the spot growth assay, YPD cultures (OD600 = 1.0) were 10-fold serially diluted with YPD, and 1.0 μL of the cell suspensions were spotted onto YPD agar plates.
RNA analyses
Total RNA samples were extracted from P. pastoris cells using the hot phenol method as previously described (Le et al., 2021). For conventional reverse transcription (RT)-PCR analysis to detect HAC1 mRNA, total RNA samples were subjected to an RT reaction with the HAC1-specific RT primer P1, which was followed by PCR with the HAC1-specific PCR primer set P3 and P4 in accordance with our previous publication (Supplementary Table S4; Fauzee et al., 2020). Because this PCR traversed the HAC1 intron sequence, the spliced and unspliced forms of HAC1 mRNA yielded different-sized PCR products, which were then separated by agarose gel electrophoresis in Tris/borate/EDTA running buffer. Subsequently, ethidium bromide-fluorescent images of the gels were captured using the digital imager E-box (Vilber Lourmat, Marne-la-Vallée, France).
Before RT-quantitative PCR (RT-qPCR) and high-throughput RNA-seq analyses, residual DNA in the total RNA samples was digested with recombinant DNase I (RNase-free; Takara, Kusatsu, Japan) in accordance with the manufacturer’s instruction. Subsequently, DNase I was removed from the total RNA samples by phenol-chloroform extraction and ethanol precipitation.
For the RT-qPCR analysis, total RNA samples were subjected to the RT reaction using poly(dT) oligonucleotide primer (Supplementary Table S4) and PrimeScript II Reverse Transcriptase (Takara, Kusatsu, Japan) as per manufacturer’s instruction. The RT-reaction products were then analyzed by real-time qPCR as described previously (Tran et al., 2019), using the primer sets listed in Supplementary Table S4. The P. pastoris ACT1 gene transcript was used as the reference (Fauzee et al., 2020), and the ΔΔCt method was used to calculate relative gene expression levels.
High-throughput RNA-seq analysis was performed by GeomeRead Co. Ltd. (Takamatsu, Japan). First, mRNA was purified from total RNA samples using the KAPA mRNA capture kit (KAPA Biosystems, Potters Bar, United Kingdom). Second, libraries were generated using the MGI Easy RNA directional library prep set (MGI Tech, Shenzhen, China) and then analyzed using the DNBSEQ-G400RS DNA sequencer (MGI Tech, Shenzhen, China; 2 × 150 bp paired-end reads, 1 Gb data/sample). Raw FASTAQ data were processed using the CLC Genomics Workbench (Qiagen, Venlo, Netherlands). Reference data for gene mapping and annotation were obtained from the mRNA-seq data have been deposited in DDBJ database under the accession number of PRJDB15162 Pichiagenome.org (http://pichiagenome-ext.boku.ac.at). We used a WEB site-based analyzer, YeastEnrichr (https://maayanlab.cloud/YeastEnrichr/) for the enrichment analysis.
Protein analyses
After harvesting by centrifugation at 1,600 × g for 1 min, 1.0 = OD600 cells were disrupted by agitation with glass beads (425–600 μm) in 100 μL of the lysis buffer containing 50 mM Tris-Cl (pH 7.9), 5 mM EDTA, 1% Triton X-100, and protease inhibitors (2 mM phenylmethylsulfonyl fluoride, 100 μg/mL leupeptin, 100 μg/mL aprotinin, 20 μg/mL pepstatin A, and Calbiochem Protease Inhibitor cocktail Set III (X100 dilution)) and then clarified by flash centrifugation at 750 × g for 30 s. The protein concentration in the crude lysates was determined using the BioRad Protein assay kit (Hercules, CA, USA) and adjusted to 2.5 mg/mL by adding the lysis buffer. Subsequently, the crude lysates were further centrifuged at 8,400 × g for 20 min, and the pellet fractions were washed twice with the lysis buffer supplemented with 2% NP-40.
Protein samples were fractionated by the standard Laemmli SDS-polyacrylamide gel electrophoresis as previously described (Le et al., 2021), and the resulting gels were silver stained using Silver Stain KANTO III (Kanto Chemical, Tokyo, Japan). Alternatively, the gels were subjected to Western blot analysis as previously described (Le et al., 2021). The primary antibodies used were a rabbit anti-ubiquitin antibody (SPA-200) purchased from Stressgen (Enzo Biochem, Farmingdale, NY, United States), a rabbit anti-GFP IgG purchased from MBL Life Science (Tokyo, Japan), and a mouse monoclonal anti-PGK1 antibody 22C5D8 purchased from Abcam (Cambridge, United Kigdom).
Statistics
Statistical analyses were performed using three independent clones of the same genotype, and values are presented as the means and standard deviations from three biological replicates. To obtain p values, we performed a two-tailed unpaired t-test using Microsoft Excel. Alternatively, RNA-seq data were processed using the CLC Genomics Workbench (Qiagen, Venlo, Netherlands). For multiple comparisons of the RT-qPCR data, we performed Dunnett’s two-tailed test, in which probability values (p values) less than 0.05 were considered statistically significant.
Results
Heat shock response is induced not by the hac1Δ mutation but by the ire1Δ mutation in Pichia pastoris cells
The main research question of this study is whether the IRE1 and HAC1 genes have effects on different pathways in P. pastoris cells. To this end, we constructed P. pastoris cells carrying the IRE1 knockout mutation (ire1Δ) or HAC1 knockout mutation (hac1Δ). Consistent with Gassler et al. (2019), CRISPR/CAS9 technology was used to introduce the ire1Δ mutation. The resulting mutant allele carried a full-length deletion of IRE1 (Supplementary Figure S1A) and is named ire1Δ0. Moreover, we initially introduced the hac1Δ mutation through insertion of the G418-resistant KanMX marker into the genomic HAC1 gene. The resulting mutant allele carried a full-length deletion of the HAC1 gene (Supplementary Figure S1B) and is named hac1::KanMX.
We previously reported that in WT P. pastoris cells, the HAC1 mRNA is partly spliced even under non-stress conditions (Fauzee et al., 2020). This observation was reproduced in Figures 1A,B. Moreover, HAC1 mRNA splicing was almost fully induced by the potent ER stressing agent DTT under our experimental conditions (Figure 1A). As described previously (Fauzee et al., 2020) and shown later in this article, not only splicing but also the cellular abundance of HAC1 mRNA is elevated depending on IRE1 and ER stress. Although not a quantitative measurement of total HAC1 mRNA abundance, our data shown in Figure 1A seem to be consistent with this insight. As expected, HAC1 mRNA was not spliced in ire1Δ cells (Figure 1B).
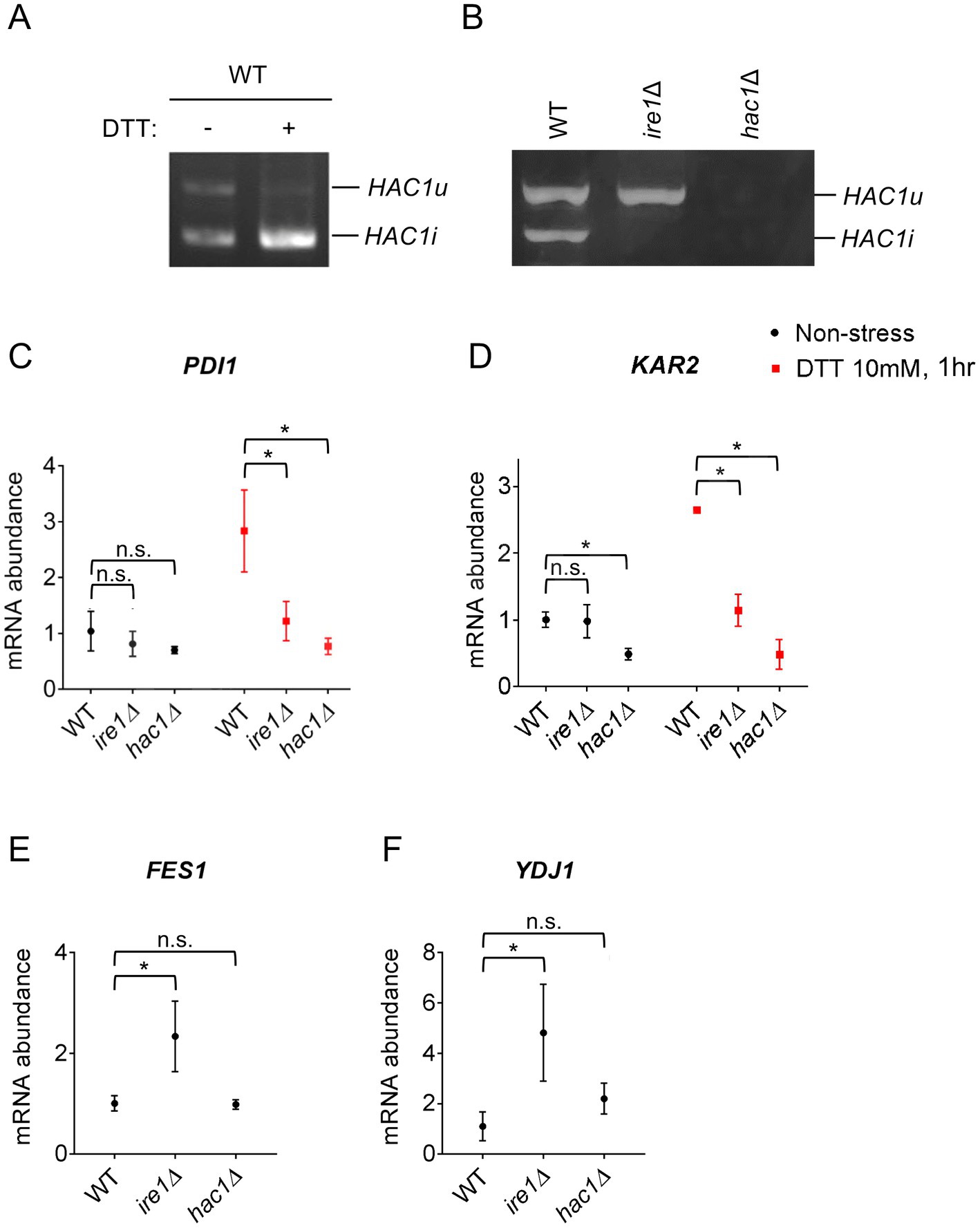
Figure 1. HAC1 mRNA-splicing and UPR or HSR-marker gene-expression profiles in P. pastoris cells carrying the ire1Δ mutation or hac1Δ mutation. WT, ire1Δ (ire1Δ0), and hac1Δ (hac1::kanMX) versions of P. pastoris cells were cultured at 30°C under non-stress conditions or stressed with 10 mM DTT for 30 min. (A,B) Total RNA samples were subjected to RT-PCR to amplify HAC1 cDNA variants, which were then fractionated using agarose gel electrophoresis. (C–F) Total RNA samples were subjected to RT-qPCR analysis using PCR primer sets that were specific to the indicated genes. Values are presented as relative to that of non-stressed WT cells, which is set at 1.0. Dunnett’s test was performed using the data from WT cells as the control group. n.s.: not significant, *: significantly different (p < 0.05).
Next, we examined the expression levels of the traditional UPR target genes, KAR2 and PDI1, in P. pastoris cells stressed or not stressed by DTT. As previously reported by us and others (Whyteside et al., 2011; Fauzee et al., 2020), DTT induced the expression of KAR2 and PDI1 in wild-type (WT) P. pastoris cells (Figures 1C,D). Consistent with the widely accepted view that the IRE1/HAC1-dependent UPR pathway transcriptionally induces the PDI gene, the ire1Δ and hac1Δ mutations almost equally compromised the expression of PDI1 (Figure 1C). Induction of KAR2 by DTT was also attenuated by the ire1Δ and hac1Δ mutations (Figure 1D). However, unexpectedly, only the hac1Δ mutation compromised KAR2 expression under non-stress conditions (Figure 1D).
In S. cerevisiae cells, KAR2 is transcriptionally induced not only by the UPR, but also by the heat shock response (HSR) (Kohno et al., 1993). Therefore, we hypothesized that even under non-stress conditions, the ire1Δ mutation, but not the hac1Δ mutation, induces the HSR, leading to the higher KAR2 expression in ire1Δ cells than in hac1Δ cells. It is widely accepted that the HSR causes the transcriptional induction of genes encoding cytosolic and/or nuclear molecular chaperones and chaperone co-factors (Morano et al., 1998). In this study, we therefore examined the expression of the HSR markers FES1 and YDJ1, both of which encode cytosolic Hsp70 co-factors and are known to be induced by heat shock in other species (Caplan and Douglas, 1991; Kabani et al., 2002; Chen and Qiu, 2020). Intriguingly, the ire1Δ mutation, but not the hac1Δ mutation, considerably elevated the expression of FES1 and YDJ1 under non-stress conditions (Figures 1E,F). In the experiment shown in Supplementary Figure S2, we used another ire1Δ allele, namely ire1::kanMX, and confirmed that the ire1Δ mutation induced FES1 and YDJ1. Similar results as Figures 1E,F were obtained for HSP42, SSA3 (cytosolic/nuclear HSP70 chaperone gene), and SIS1 (cytosolic/nuclear co-chaperone gene), which are also considered HSR marker genes (Supplementary Figure S3).
Global gene expression alteration by the ire1Δ and hac1Δ mutations in Pichia pastoris cells
Therefore, the ire1Δ and hac1Δ mutations likely result in different outcomes in P. pastoris. To elucidate this issue more deeply, we performed a transcriptome analysis of ire1Δ and/or hac1Δ mutant cells. For this analysis, we employed cells carrying CRISPR/CAS9-based gene-deletion mutations (ire1Δ0 and/or hac1Δ0; see Supplementary Figure S1C for the construction of the hac1Δ0 allele). Heterologous drug-resistance markers were not used because their transcripts could be a bias in the mRNA-seq analysis. To generate cells carrying the ire1Δhac1Δ double mutation, cells carrying the hac1Δ0 mutation were further mutagenized to carry the ire1Δ0 mutation.
We cultured WT, ire1Δ, hac1Δ, and ire1Δhac1Δ cells under non-stress conditions for RNA extraction because, as aforementioned, the IRE1/HAC1-dependent UPR system is activated even without external stress stimuli in P. pastoris cells, albeit not strongly. We then subjected the cells to mRNA-seq analysis and presented the total data in Supplementary Table S5. In the volcano plots shown in Figures 2A–C, we compared the transcriptome of ire1Δ cells, hac1Δ cells, and ire1Δhac1Δ cells to that of WT cells and found that the expression of a number of genes is controlled by IRE1 and HAC1. Consistent with our previous observations (Fauzee et al., 2020), the expression level of HAC1 was positively and considerably regulated by IRE1 (Figure 2A). Intriguingly, as shown in Figures 2D,E, the ire1Δ vs. ire1Δhac1Δ and hac1Δ vs. ire1Δhac1Δ comparisons also revealed many differentially expressed genes (DEGs). Thus, we deduced that at least partly, IRE1 and HAC1 act via different pathways in P. pastoris.
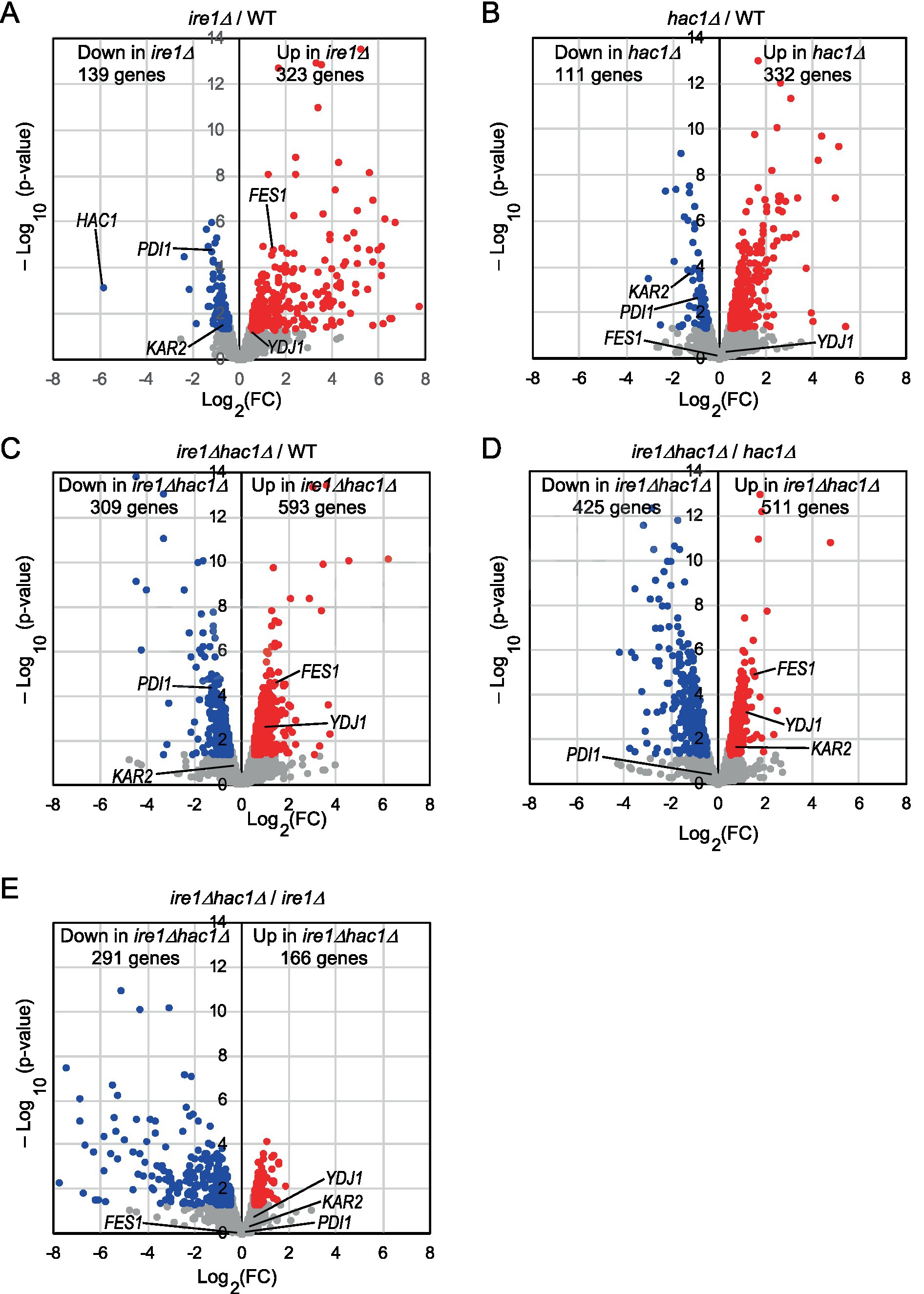
Figure 2. (A–E) Volcano plots displaying DEGs between two types of cells. WT, ire1Δ (ire1Δ0), hac1Δ (hac1Δ0), and ire1Δhac1Δ (ire1Δ0 hac1Δ0) versions of P. pastoris cells were cultured at 30°C under non-stress conditions, and their mRNA samples were subjected to RNA-seq analysis. See Supplementary Table S5 for the total data. In the volcano plots, the x-axis represents the Log2 of the fold change (FC), and the y-axis represents the negative decade logarithm of the value of p. DEGs (p < 0.05; Log2(FC) < −0.5 or > 0.5) are colored. We did not set the cut-off value for Log2(FC) greater than 0.5 because Ire1 was only moderately activated under our experimental conditions.
This idea is supported by the Venn diagrams shown in Figure 3, which indicate that the DEGs of the ire1Δ mutation (ire1Δ vs. WT comparison) and those of the hac1Δ mutation (hac1Δ vs. WT comparison) overlapped only partially. The DEGs of the ire1Δhac1Δ mutation (ire1Δhac1Δ vs. WT comparison) also overlapped, but not perfectly, implying that IRE1 and HAC1 control the expression of various genes in both independent and interdependent manners (Figures 3A,B). To address the HAC1-independent function of IRE1, we also compared ire1Δhac1Δ vs. hac1Δ in Venn diagrams (Figures 3C,D). The DEGs in the hac1Δ vs. WT and ire1Δhac1Δ vs. hac1Δ comparisons overlapped only slightly (two induced and eleven repressed DEGs), supporting our proposal that the HAC1-dependent and the HAC1-independent functions of IRE1 are distinct.
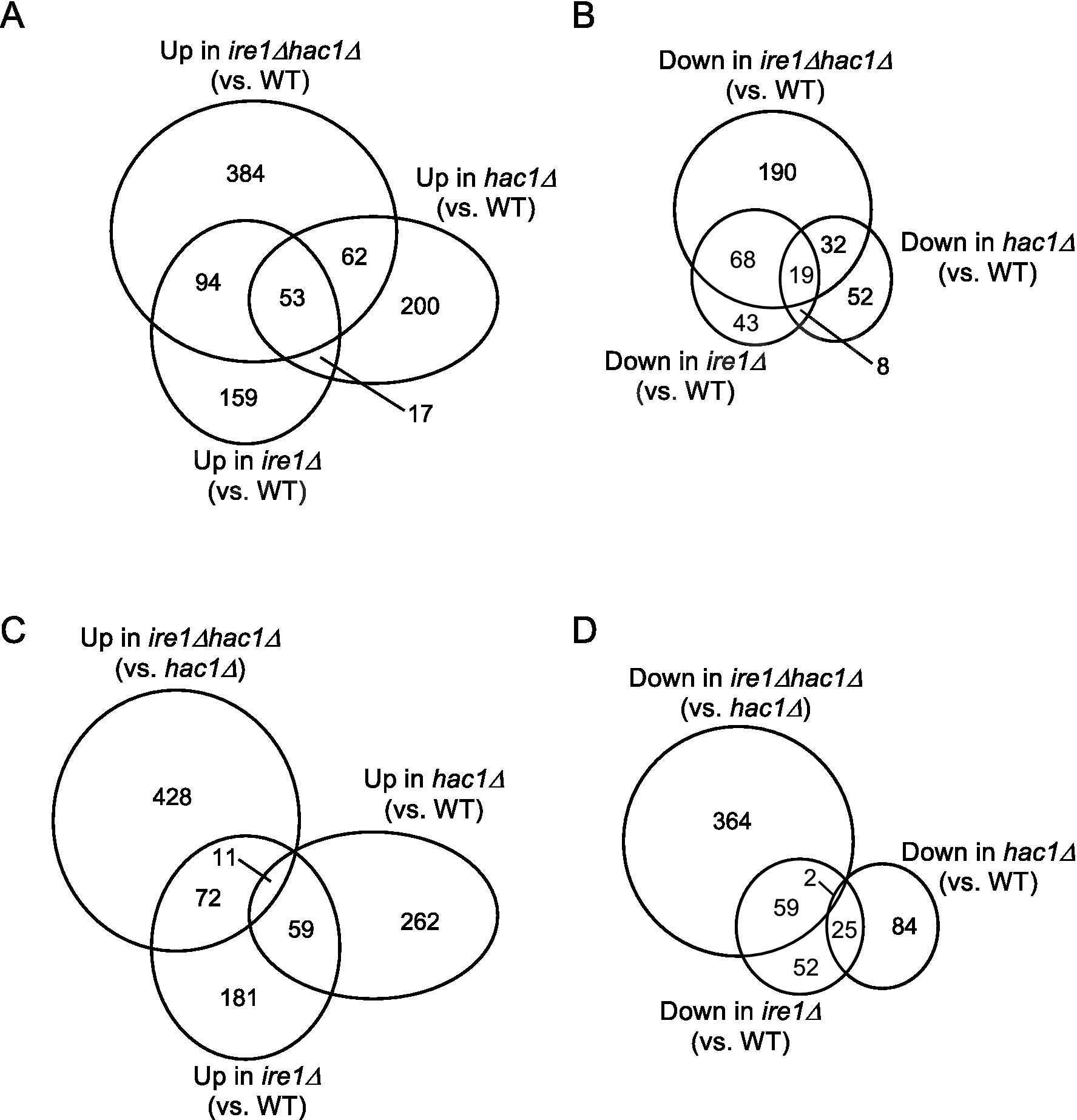
Figure 3. (A–D) Venn diagram presentation for DEGs between two types of cells. DEGs (p < 0.05; Log2(FC) < −0.5 or > 0.5) were extracted from the mRNA-seq data shown in Supplementary Table S5 and are presented as Venn diagrams.
Next, we screened the total RNA-seq data for DEGs cooperatively induced by IRE1 and HAC1 (Category A) and controlled only by IRE1 (Category B or C). Using the screening criteria presented in Figure 4, we selected 15 named genes as the Category-A DEGs (Figure 4 and Supplementary Table S6). Consistent with our expectation that Category-A DEGs are targets of the traditional UPR, many of them are known to be involved in ER protein translocation, folding, and modification (see the Discussion section for details).
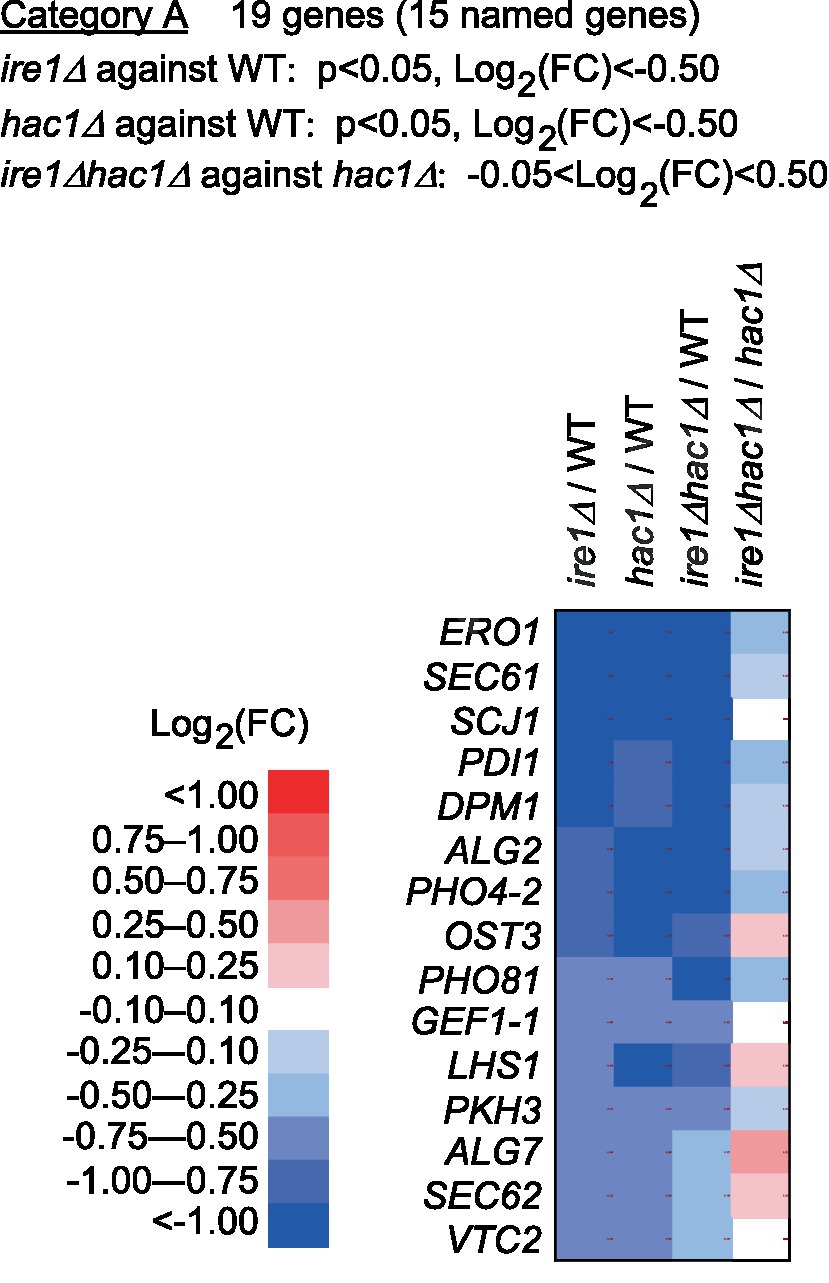
Figure 4. Genes cooperatively induced by IRE1 and HAC1. The mRNA-seq data shown in Supplementary Table S5 were screened using the indicated criteria to extract the DEGs belonging to Category A. The heat map presents the expression profiles of the named genes in Category A, which are listed in Supplementary Table S6.
Category B is a group of genes that were repressed by IRE1 independent of HAC1 (Figure 5 and Supplementary Table S6). The screening criteria for Category B are DEGs with elevated expression in comparison of ire1Δhac1Δ cells against hac1Δ cells. As shown in Figure 5, many of the Category-B genes were induced in ire1Δ cells and ire1Δhac1Δ cells compared to WT cells. As expected from our observations shown in Figure 1, KAR2, YDJ1 and FES1 fell into Category B. Expression of FES1 and YDJ1 was high in ire1Δ and ire1Δhac1Δ cells (Figure 5). Moreover, the expression of KAR2 was considerably lowest in hac1Δ cells (Figure 5), presumably because it was induced by the HSR in ire1Δ and ire1Δhac1Δ cells.
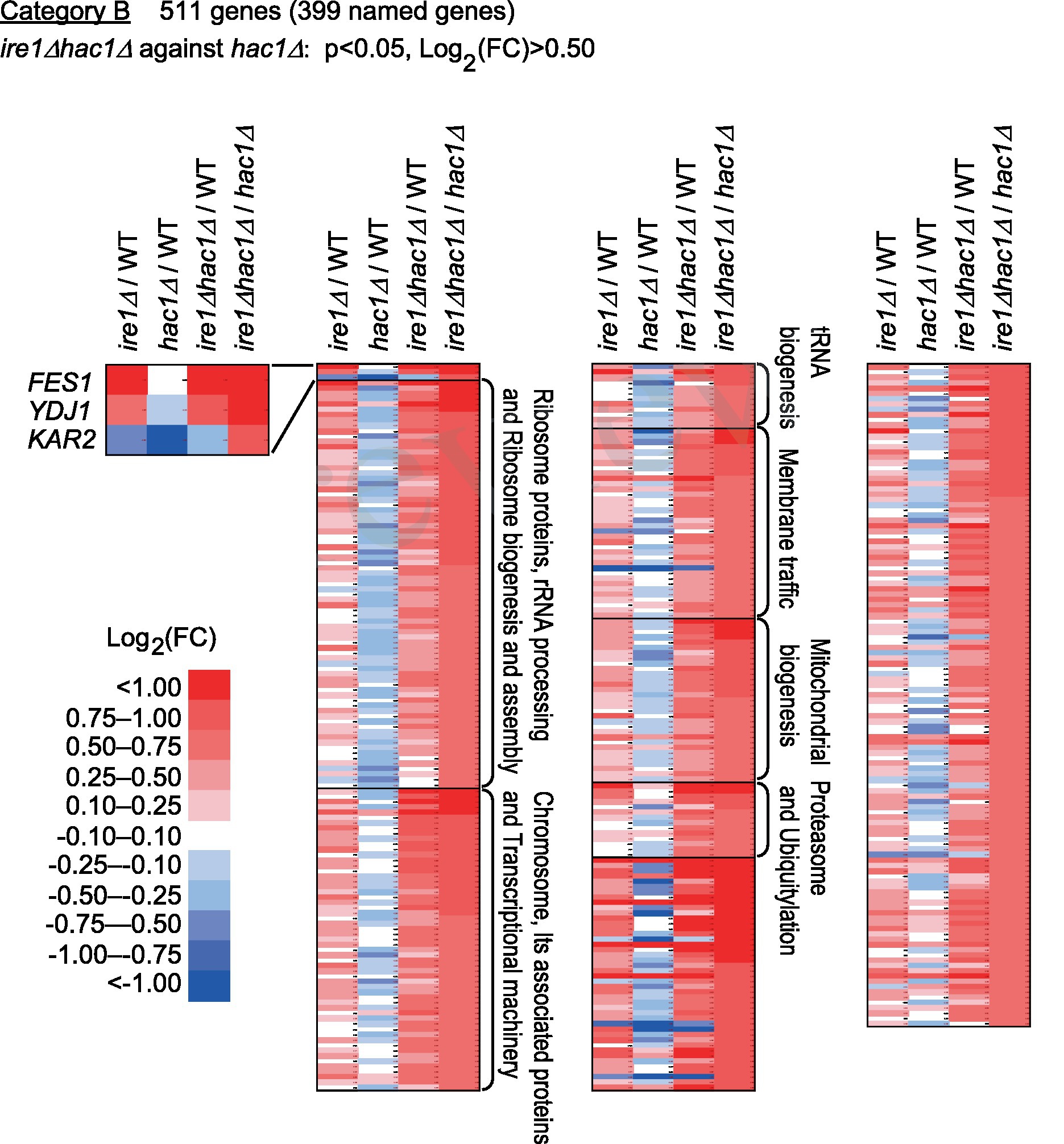
Figure 5. Genes suppressed by IRE1 independently of HAC1. The mRNA-seq data shown in Supplementary Table S5 were screened using the indicated criteria to extract the DEGs belonging to Category B. The heat map presents the expression profiles of the named genes in Category B, which are listed in Supplementary Table S6.
According to the enrichment analysis shown in Supplementary Table S7, genes encoding ribosomal proteins and those related to ribosome biogenesis were highly enriched in Category B. The MA plot shown in Supplementary Figure S4 indicates that many of the ribosomal protein genes were abundantly expressed and induced in ire1Δhac1Δ cells compared to hac1Δ cells. It should also be noted that many genes related to the proteasome and ubiquitylation fell into Category B (Figure 5 and Supplementary Table S6).
Category C is a group of genes induced by IRE1 independent of HAC1 (Figure 6 and Supplementary Table S6). As shown in Supplementary Table S7, genes for glycolysis/gluconeogenes and various metabolic pathways were highly enriched in Category C.
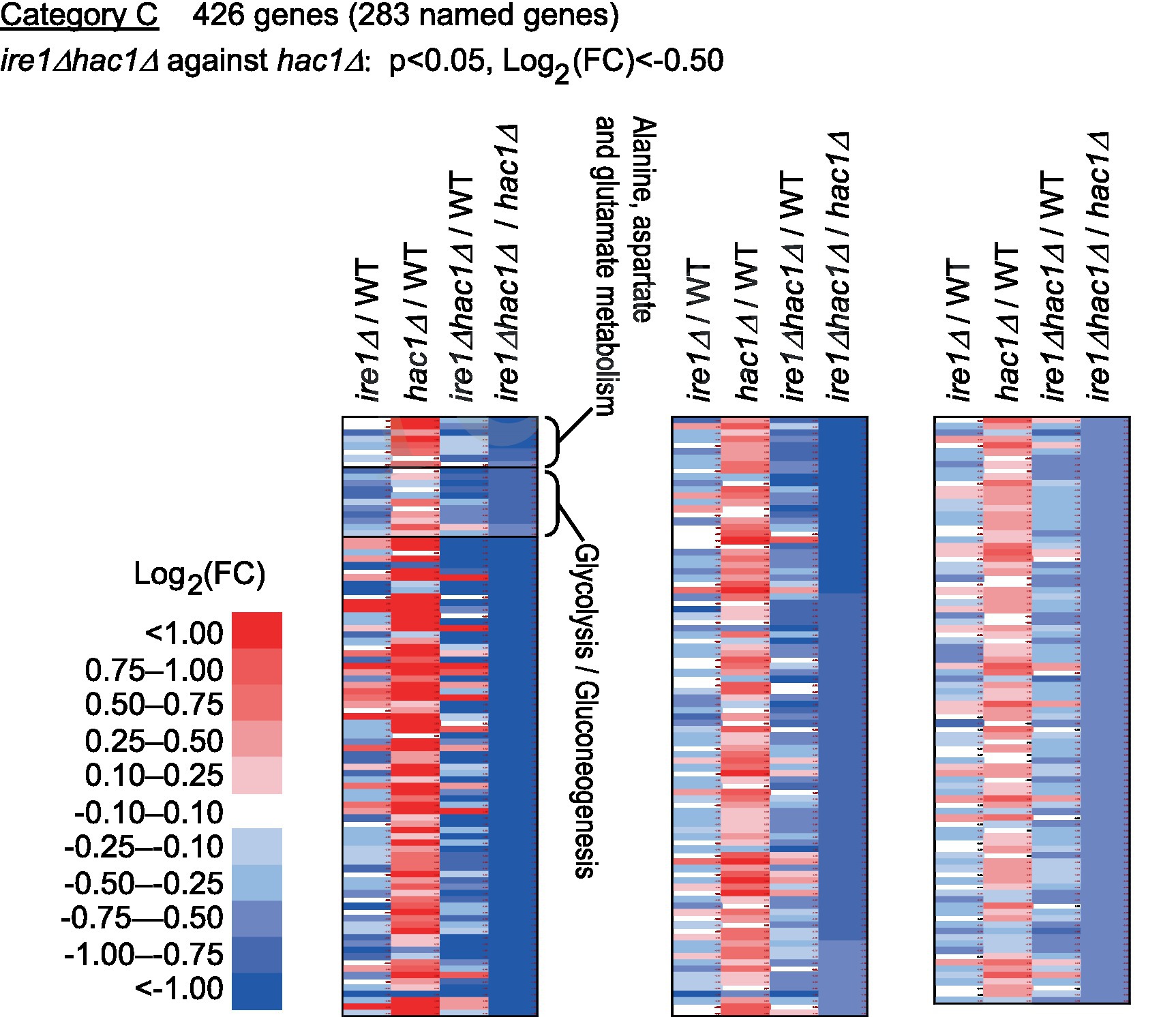
Figure 6. Genes induced by IRE1 independently of HAC1. The mRNA-seq data shown in Supplementary Table S5 were screened using the indicated criteria to extract DEGs belonging to Category C. The heat map presents the expression profiles of the named genes in Category C, which are listed in Supplementary Table S6.
Effect of the ire1Δ and hac1Δ mutations on cellular growth, protein aggregation, and stress tolerance in Pichia pastoris cells
In addition to the gene expression profile, we compared other phenotypes of the ire1Δ and hac1Δ mutations. In the experiment shown in Figure 7, we examined the growth of P. pastoris cells at 30°C in liquid media under non-stress conditions. Consistent with our previous study (Fauzee et al., 2020), ire1Δ cells grew slower than WT cells. We also noticed that the hac1Δ mutation retarded the growth of WT cells. Intriguingly, ire1Δhac1Δ cells grew slower than ire1Δ or hac1Δ cells. This observation supports our proposition that IRE1 and HAC1 act partly on different pathways.
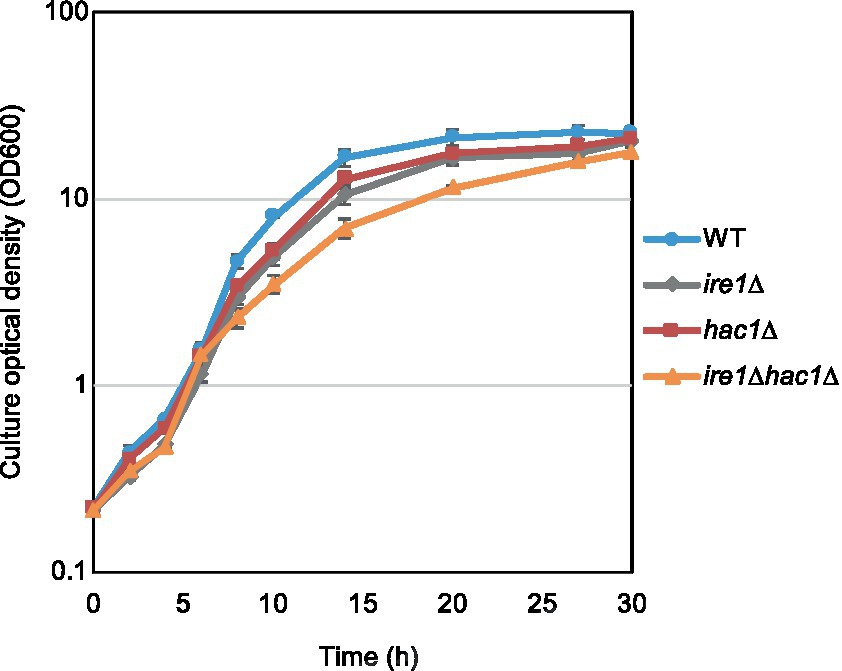
Figure 7. Growth profile of P. pastoris cells carrying the ire1Δ mutation and/or the hac1Δ mutation. After setting the initial OD600 values to approximately 0.3, the WT, ire1Δ (ire1Δ0), hac1Δ (hac1Δ0), and ire1Δhac1Δ (ire1Δ0 hac1Δ0) versions of P. pastoris cells were incubated at 30°C under non-stress conditions, and the optical density of the cultures was monitored.
As aforementioned, the ire1Δ mutation, but not the hac1Δ mutation, induced the HSR in P. pastoris cells. It is widely accepted that the HSR is a cellular protective response that is activated alongside the aggregation of proteins in the cytosol and/or nuclei (Chen and Qiu, 2020). Therefore, we monitored protein aggregation in cells carrying the ire1Δ and/or hac1Δ mutations. In the experiment shown in Figure 8, the cells were cultured at 30°C, and their lysates were fractionated by centrifugation. Figure 8A indicates that the pellet fractions of ire1Δ cells and ire1Δhac1Δ cells contained more abundant proteins than those of WT or hac1Δ cells. Anti-ubiquitin Western blot analysis showed that proteins in the pellet fractions were ubiquitylated, at least partly (Figure 8B). These observations strongly suggest a role of IRE1 to prevent protein aggregation in the nuclei/cytosol.
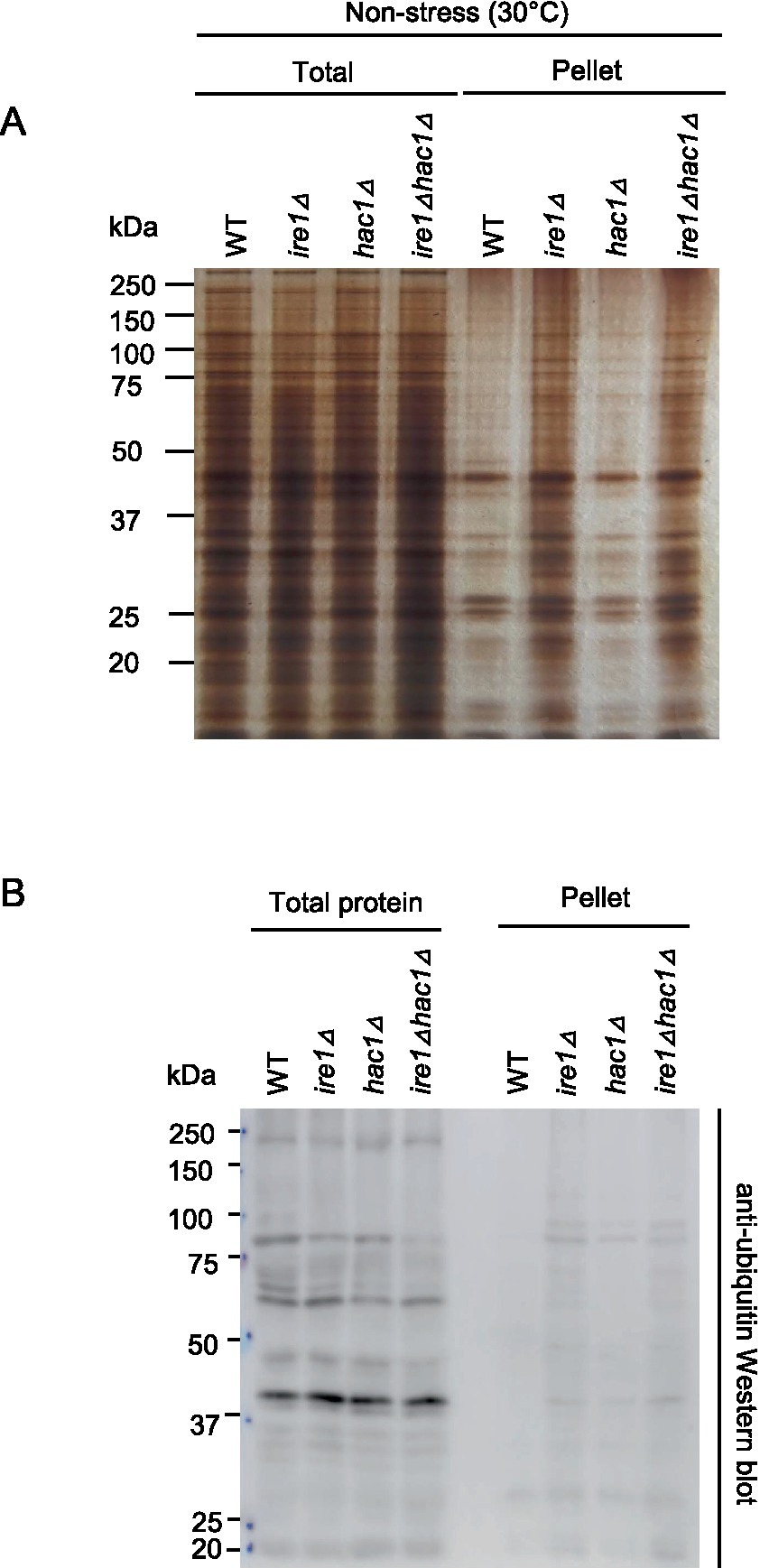
Figure 8. Induction of protein aggregation by the ire1Δ mutation. After culturing at 30°C under non-stress conditions, the WT, ire1Δ (ire1Δ0), hac1Δ (hac1Δ0), and ire1Δhac1Δ (ire1Δ0 hac1Δ0) versions of P. pastoris cells were harvested and lysed. The crude lysates (Total) were subjected to high-speed centrifugation, and pellet fractions (Pellet) were obtained. (A) Protein samples (Total: crude lysates corresponding to 6 μg protein; Pellet: preparation from crude lysates corresponding to 16 μg protein) were separated by SDS-PAGE and visualized by silver staining. (B) Protein samples (Total: crude lysates corresponding to 6 μg protein; Pellet: preparation from crude lysates corresponding to 16 μg protein) were subjected to SDS-PAGE, which was followed by anti-ubiquitin Western blotting.
Tunicamycin is an N-glycosylation-inhibiting antibiotic that is frequently used as a potent ER stressor. S. cerevisiae cells carrying the ire1Δ or hac1Δ mutation are known to be hypersensitive to tunicamycin. Figure 9 shows the growth of the P. pastoris cells on agar plates. In addition to the liquid medium (Figure 7), ire1Δhac1Δ cells appeared to grow slower than the other strains on agar plates under non-stress conditions (Figure 9A). As shown in Figure 9B, tunicamycin retarded the growth of cells carrying the ire1Δ and/or hac1Δ mutations more severely compared to that of WT cells. Moreover, hac1Δ cells were more susceptible to tunicamycin than ire1Δ and ire1Δhac1Δ cells (Figure 9B). In the experiment shown in Figures 9C,D, cells were incubated at 39°C for 1 h before being spotted onto agar plates. Intriguingly, this heat shock treatment partly mitigated the severe sensitivity of hac1Δ cells to tunicamycin (compare Figures 9D to B). As we mentioned above, the ire1Δ mutation induces the HSR in P. pastoris cells. Therefore, we presume that high tunicamycin sensitivity associated with UPR impairment, which is caused by the ire1Δ mutation or the hac1Δ mutation, is partially rescued by the HSR, which is induced by the ire1Δ mutation or heat shock treatment.
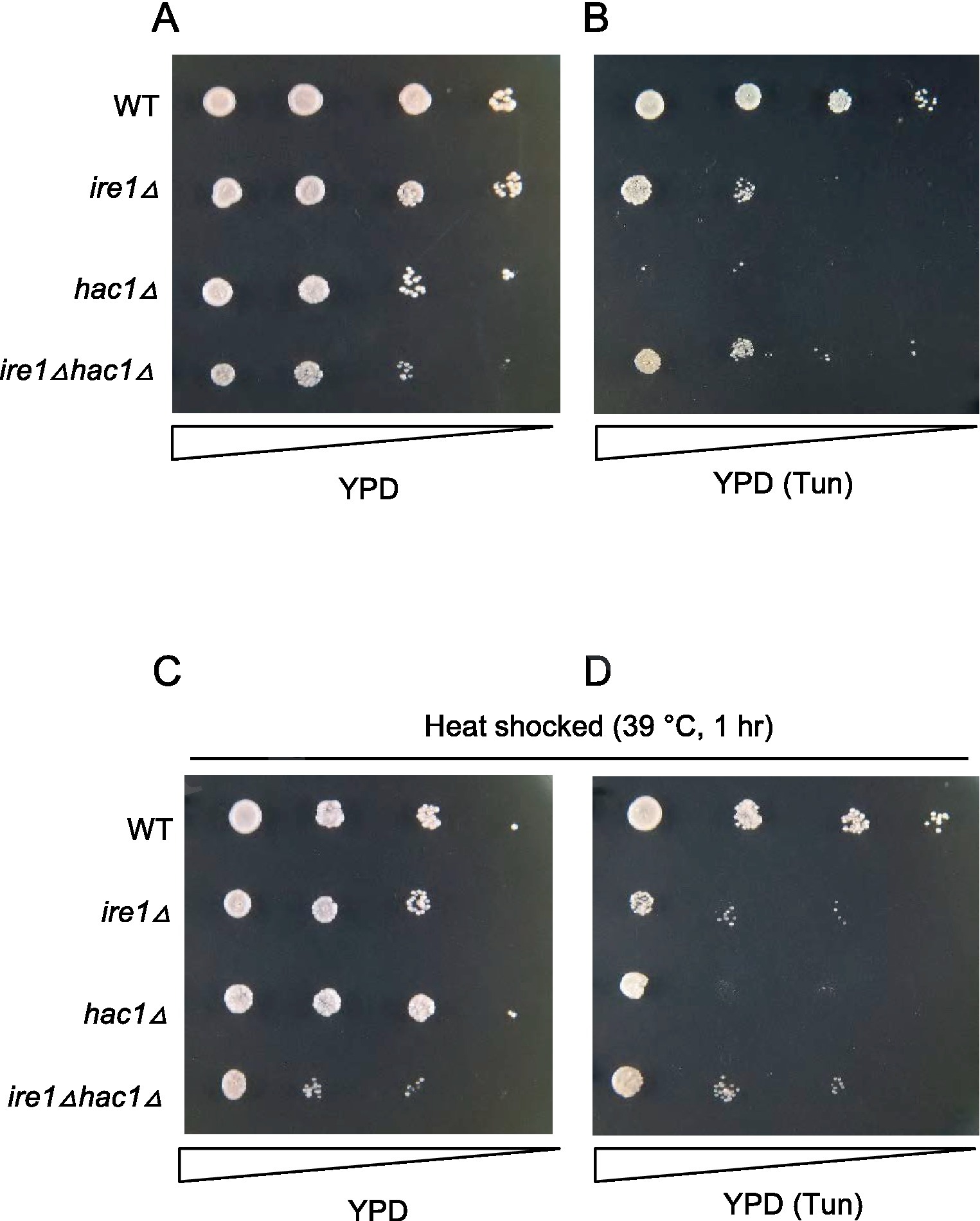
Figure 9. Tunicamycin sensitivity of P. pastoris cells carrying the ire1Δ mutation and/or the hac1Δ mutation. Cultures (OD600 = 1.0) of the WT, ire1Δ (ire1Δ0), hac1Δ (hac1Δ0), and ire1Δhac1Δ (ire1Δ0 hac1Δ0) versions of P. pastoris cells were 10-fold serially diluted and spotted onto YPD agar plates, which were incubated at 30°C for 2 days before being photographed. In panel (A), cell were unstressed. In panels (B,D), agar plates contained 4.0 μg/mL tunicamycin (Tun). In panels (C,D), cultures were incubated at 39°C for 1 h before spotting.
Involvement of IRE1 and HAC1 in properties of heat-shocked Pichia pastoris cells
To elucidate the involvement of the UPR factors in the HSR more deeply, we examined the response of P. pastoris cells to high-temperature culturing. Figure 10A shows that splicing of HAC1 mRNA was induced by a temperature shift from 30°C to 39°C, indicating UPR induction upon this temperature shift. Consistent with our proposal that PDI expression is positively regulated by the UPR but not by the HSR, it was induced by this temperature shift in WT cells but not in ire1Δ cells, hac1Δ cells, or ire1Δhac1Δ cells (Figure 10B).
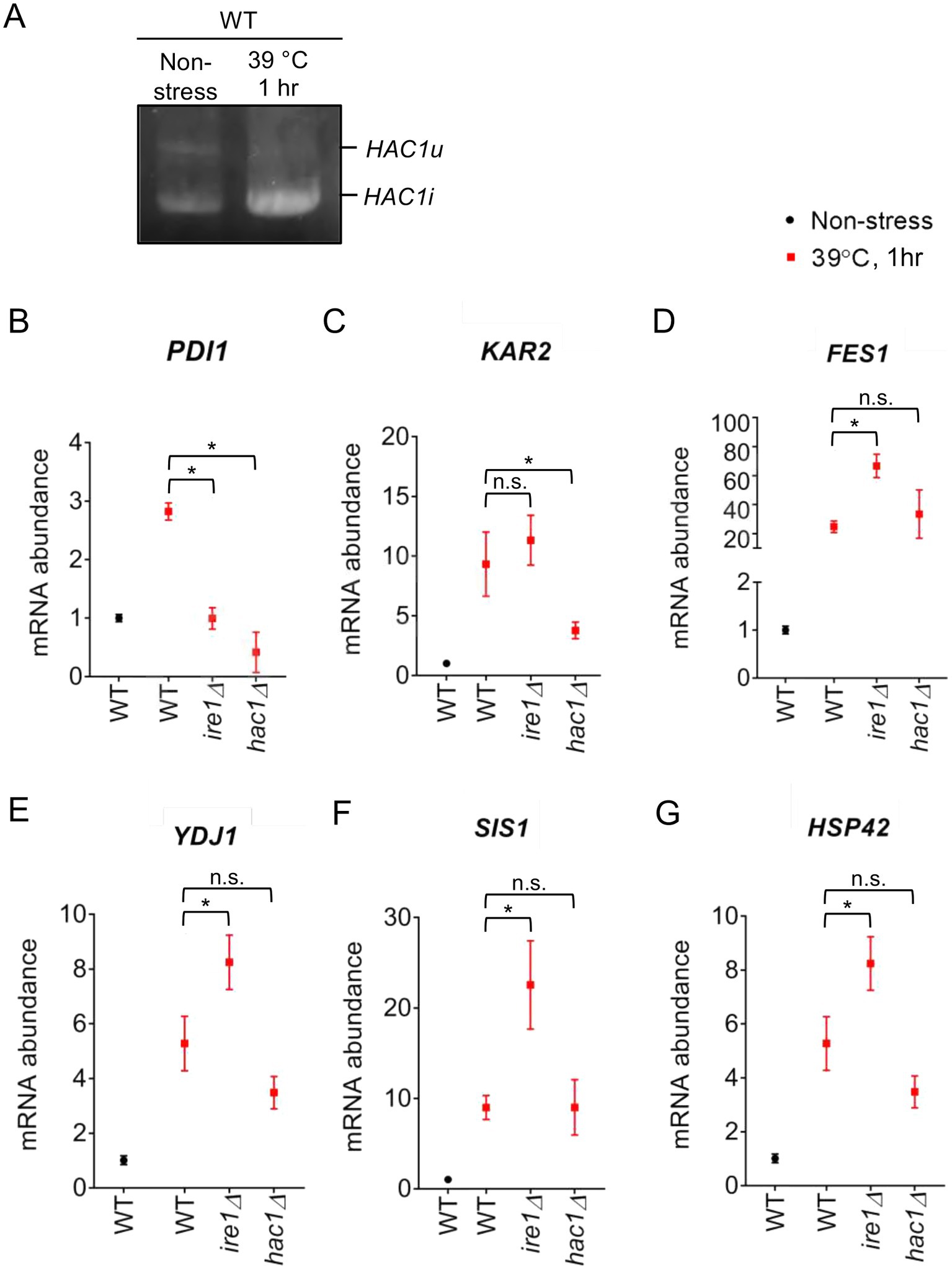
Figure 10. Heat shock-induced alteration of HAC1 mRNA-splicing and gene-expression profiles of P. pastoris cells. (A) WT P. pastoris cells were cultured at 30°C under non-stress condition or shifted to 39°C for 1 h. RNA samples were subjected to RT-PCR to amplify the HAC1 cDNA variants, which were then fractionated by agarose gel electrophoresis. (B–G) After culture at 30°C under non-stress conditions, WT, ire1Δ (ire1Δ0), hac1Δ (hac1Δ0), and ire1Δhac1Δ (ire1Δ0 hac1Δ0) versions of P. pastoris cells were shifted to 39°C for 1 h. Total RNA samples were subjected to RT-qPCR analysis using PCR primer sets that were specific to the indicated genes. Values are presented as relative to that of WT cells cultured at 30°C, which is set at 1.0. Dunnett’s test was performed using the data from WT cells as the control group. n.s.: not significant, *: significantly different (p < 0.05).
Moreover, as shown in Figures 10D–G, this temperature shift also elevated the expression of the HSR marker genes FES1, YDJ1, HSP42, and SIS1. Because the temperature-dependent induction of these genes was stronger than that caused by the ire1Δ mutation at 30°C (compare Figures 10D,E to Figures 1E,F), we deduced that the ire1Δ mutation alone only moderately induces the HSR. Figures 10D–G also show that this temperature shift led to greater upregulation of the HSR marker genes in ire1Δ cells than in WT cells or hac1Δ cells. Thus, we presume that the HSR was additively or cooperatively induced by the temperature shift and the ire1Δ mutation. The expression pattern of KAR2 (Figure 10C) can be explained by our proposition that in P. pastoris cells, KAR2 is dually regulated by the UPR and HSR.
In the experiment shown in Figure 11, we examined the growth of cells on agar plates at different temperatures. All the strains were unable to grow at 39°C (Figure 11B). This agar plate was then shifted from 39°C to 30°C, resulting in the growth of all strains other than the ire1Δhac1Δ strain (Figure 11C). Therefore, we assume that IRE1 and HAC1 confer heat resistance to P. pastoris cells in different ways.
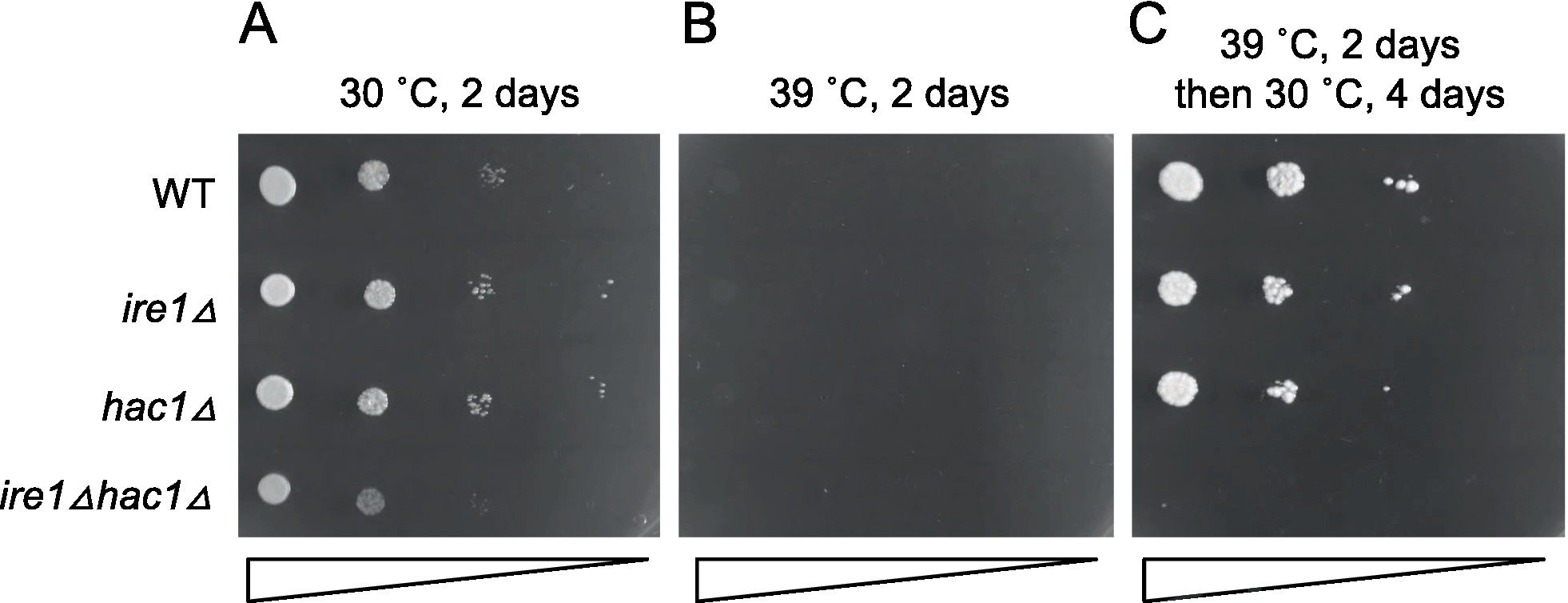
Figure 11. High-temperature sensitivity of P. pastoris cells carrying the ire1Δ and/or hac1Δ mutations. Cultures (OD600 = 1.0) of the WT, ire1Δ (ire1Δ0), hac1Δ (hac1Δ0), and ire1Δhac1Δ (ire1Δ0 hac1Δ0) versions of P. pastoris cells were 10-fold serially diluted and spotted onto YPD agar plates. (A) Agar plate was incubated at 30°C for 2 days and photographed. (B) Agar plate was incubated at 39°C for 2 days and photographed. (C) After incubation at 39°C for 2 days, the agar plate was incubated at 30°C for 4 days and photographed.
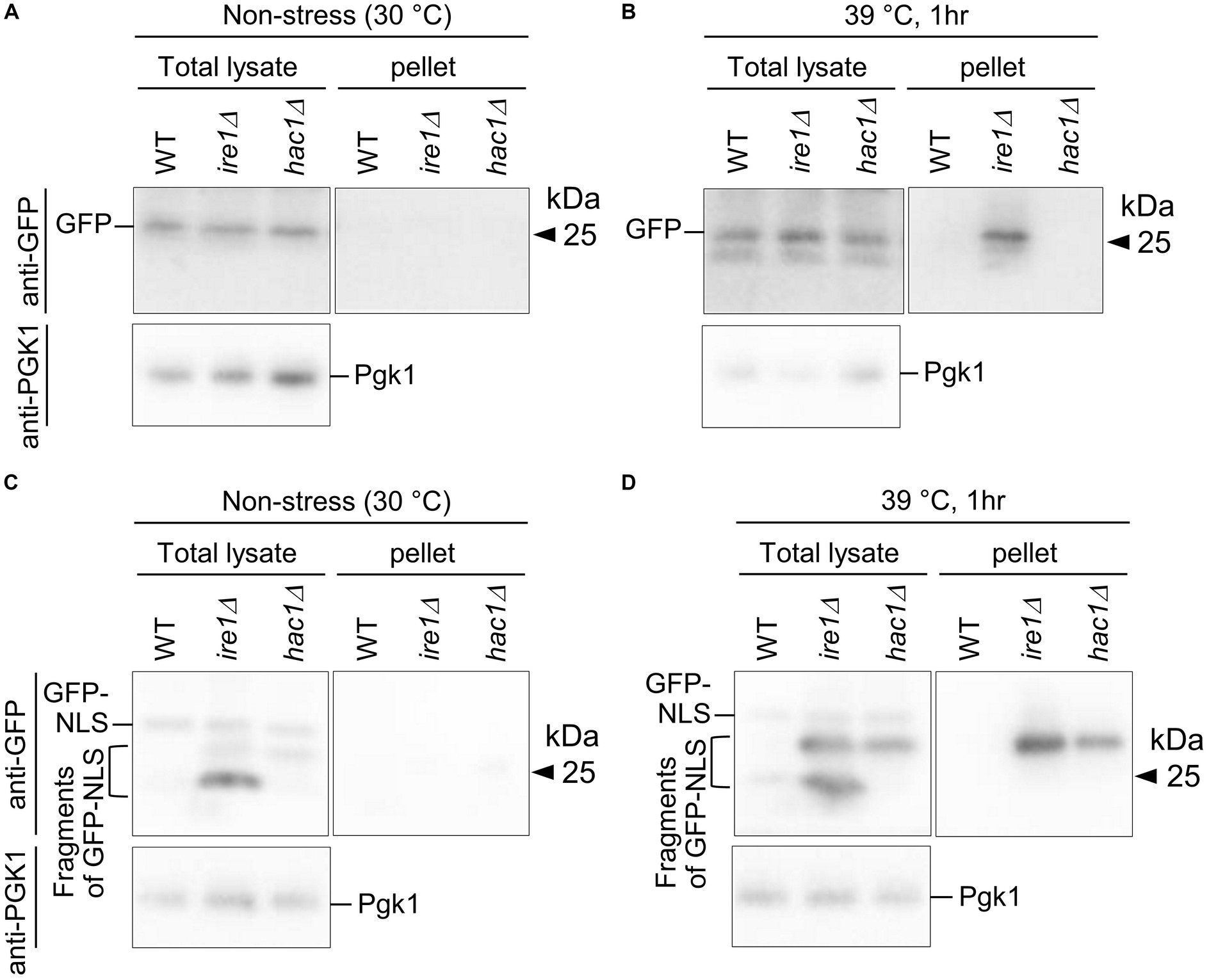
Figure 12. Aggregation and truncation of GFP and GFP-NLS in P. pastoris cells carrying the ire1Δ mutation or hac1Δ mutation. After culturing at 30°C under non-stress conditions (A,C) or shifting to 39°C for 1 h (B,D), the WT, ire1 (ire1Δ0), and hac1Δ (hac1Δ0) versions of P. pastoris cells expressing GFP (A,B) or GFP-NLS (C,D) were harvested and lysed. The crude lysates (Total) were subjected to high-speed centrifugation, and pellet fractions (Pellet) were obtained. Protein samples (Total: crude lysates corresponding to 6 μg protein; Pellet: preparation from crude lysates corresponding to 16 μg protein) were subjected to SDS-PAGE, which was followed by anti-GFP Western blotting. Two anti-GFP blot panels in each of (A–D) are from the same membrane image. Anti-Pgk1 Western blots are used as loading control.
In the final part of our study, we checked cytosolic/nuclear protein aggregation using GFP as a marker protein. First, WT, ire1Δ, and hac1Δ cells expressing GFP were cultured at 30°C or shifted to 39°C before cell lysis, the products of which were then high-speed centrifuged (Figures 12A,B). Figure 12B shows that the high-temperature treatment at 39°C caused GFP aggregation in ire1Δ cells. This observation supports our proposition that the ire1Δ mutation aggravates protein aggregation in the cytosol and/or nuclei of cells. Next, a similar experiment was performed using cells expressing GFP that carries an NLS at the C-terminus (GFP-NLS). Figure 12C shows that, in addition to full-length GFP-NLS, shorter versions (probably degraded fragments) of GFP-NLS were detected abundantly from ire1Δ cells, and to a lesser extent, from hac1Δ cells. A GFP-NLS fragment was delivered to the pellet fractions at least partly when cells were sifted to 39°C before lysis. Therefore, we deduce that there exits an Ire1-dependent system to cope with aberrant proteins accumulated in the cytosol and/or nuclei.
Discussion
As described thus far, it is widely believed that in S. cerevisiae, HAC1 mRNA is the sole target of Ire1. Tam et al. (2014) proposed that the RIDD occurs in S. cerevisiae cells, but this observation was not reproduced in our study (data not shown). Moreover, HAC1 mRNA is virtually functionless unless it is spliced by Ire1. Thus, the functions of IRE1 and HAC1 are severely interdependent. However, here we note that this insight is not applicable to P. pastoris. Our observations cumulatively indicate that in P. pastoris cells, IRE1 and HAC1 play both interdependent and independent roles.
Category A is a group of genes induced by the traditional UPR, for which IRE1 and HAC1 function cooperatively (Figure 4). Genes in Category A included those encoding the ER-located molecular chaperone (LHS1), factors for disulfide bond formation in the ER (ERO1 and PDI1), factors for glycosylation (DPM1, OST3, ALG2, and ALG7), and factors for protein translocation into the ER (SEC61 and SEC62). We deduce that Category-A genes are transcriptionally induced by the translation product of spliced HAC1 mRNA, which acts as a nuclear transcription factor. Using a DNA microarray technique, Graf et al. (2008) listed genes that were induced by DTT treatment and artificial expression of spliced HAC1 mRNA in P. pastoris cells. As expected, Category-A genes were included in Grafs’ list. The number of genes in Category A in our study was smaller than that in Grafs’ list, probably because, in our case, P. pastoris cells were cultured under non-stress conditions and provoked the UPR only moderately.
Moreover, the list of Category-A genes overlaps with that of the UPR target genes in S. cerevisiae cells (Travers et al., 2000; Kimata et al., 2006). In this context, the UPR in P. pastoris cells and S. cerevisiae cells has the same biological meaning of enhancing the activity of the ER and protein secretory pathway. Nevertheless, it should be also noted that the UPR target genes in these two species were not identical. For instance, membrane lipid biosynthesis genes, such as INO1 and SCS3, were not induced by the UPR in P. pastoris cells (Supplementary Table S5). Unlike the case of S. cerevisiae cells (Schuck et al., 2009; Nguyen et al., 2022), expansion of the ER membrane may not be an outcome of the UPR in P. pastoris cells.
Meanwhile, the main argument of the present study is that in P. pastoris cells, Ire1 also functions independently of HAC1. Here we note that the ire1Δ mutation, but not the hac1Δ mutation, provoked the HSR and protein aggregation. Because aggregated proteins were at least partially ubiquitylated, we assume that they were formed in the cytosol and/or nuclei. Therefore, it is likely that in P. pastoris cells carrying the ire1Δ mutation, cytosolic and/or nuclear protein aggregation provokes the HSR. To the best of our knowledge, the role of Ire1 to suppress cytosolic and/or nuclear protein aggregation and the HSR has not been previously reported in any eukaryotic species. As shown in Figure 7, the ire1Δ mutation retarded cellular growth, even in the hac1Δ background. This observation supports the physiological importance of the HAC1-indepencent function of Ire1 in P. pastoris.
Category B is a group of genes induced by the ire1Δ mutation in the hac1Δ background (Figure 5). Probably because the ire1Δ mutation only modestly provoked the HSR, certain genes that are deduced to be heat shock genes, namely, genes for cytosolic molecular chaperones and their co-factors, did not fall into Category B. Nevertheless, Category B is composed of a number of other genes, including those encoding ribosomal proteins and ribosome biogenesis factors. According to Tye et al. (2019), aberrant ribosome biogenesis yields unassembled ribosomal proteins, which are proteotoxic and induce the HSR. Therefore, Ire1 may suppress the expression of ribosomal proteins and ribosome biogenesis factors, thus leading to the attenuation of the HSR. As shown in Supplementary Figure S4, ribosomal proteins were abundantly expressed in cells. Thus, we assume that the suppression of ribosomal protein expression by Ire1 is important for reducing the protein load into the cytosol and/or the nuclei, which may result in attenuation of the HSR.
Some genes related to the proteasome and ubiquitylation were also grouped into Category B. Upregulation of the ubiquitin/proteasome-dependent protein-degradation pathway may be a cellular response to cope with cytosolic and/or nuclear protein aggregation.
Nevertheless, the proximal role of Ire1 besides splicing HAC1 mRNA in P. pastoris cells has not been clarified. One possibility is the RIDD, through which Ire1 decreases the cellular abundance of specific mRNAs, many of which encode ER client proteins, independent of HAC1 (Coelho and Domingos, 2014). Indeed, as aforementioned, the ire1Δ mutation increased the expression of a number of genes, namely, Category B genes, in the hac1Δ background. However, it should also be noted that genes for ER client proteins were not enriched in Category B. Moreover, according to the structure prediction by Li et al. (Li et al., 2021), the RNase domain of Ire1 in P. pastoris, as well as that in S. cerevisiae, has a narrow substrate specificity and is unlikely to perform the RIDD. As proposed in a study on mammalian cells (Urano et al., 2000), it may also be possible that the kinase domain of Ire1 performs not only auto-phosphorylation but also phosphorylation of other proteins.
What is the physiological meaning of Ire1, which is widely believed to be a factor to cope with ER stress, to mitigate cytosolic and/or nuclear protein aggregation and the HSR? According to Hamdan et al. (2017), ER stress totally damages the cellular protein-folding status, thus leading to protein aggregation not only in the ER but also in the cytosol in S. cerevisiae. On the other hand, in some human neurodegenerative diseases including Parkinson’s disease, proteins aggregated in the cytosol are thought to trigger ER stress (Melo et al., 2018). In other words, cytosolic protein aggregation can be a cause and an outcome of ER stress.
In this study, we also demonstrated another intriguing relationship between the UPR and HSR. Based on our observation shown in Figure 9, we propose that the high sensitivity of UPR-deficient cells to ER stress can be partly rescued by inducing the HSR in both P. pastoris and S. cerevisiae cells (Liu and Chang, 2008). However, whether this observation can be explained solely by the expression level of KAR2, which is positively regulated by both the UPR and HSR, has not been clarified.
Meanwhile, according to our observations presented here, not only Ire1 but also HAC1 plays a role(s) other than performing the traditional UPR, in which Ire1 splices HAC1 mRNA, in P. pastoris cells. Since HAC1 worked both dependently and independently of Ire1, we assume not only the spliced form but also the unspliced form of HAC1 mRNA has a biological function(s), which should be addressed in future studies.
Because, in general, high temperature impairs protein folding, heat stress likely induces both the HSR and UPR. Nevertheless, the UPR is only slightly induced at high temperatures in S. cerevisiae cells (Hata et al., 2022). On the other hand, here we observed strong activation of Ire1 in heat-stressed P. pastoris cells (Figure 10). The expression of the UPR marker gene PDI1 was elevated by a temperature shift from 30°C to 39°C dependently on both IRE1 and HAC1. Moreover, this temperature shift strongly induced the HSR, which was mitigated by Ire1. We also demonstrated the involvement of Ire1 in the heat resistance of P. pastoris cells (Figure 11).
Also in plant cells, heat stress considerably activates Ire1, which then splices and matures bZIP60 mRNA (Deng et al., 2011; Li et al., 2012). Moreover, bZIP60 induces the transcription factor HSFTF13, which upregulates the HSR (Li et al., 2020). Therefore, unlike the case of P. pastoris, Ire1 contributes to inducing the HSR under heat stress conditions in plant cells.
In conclusion, we demonstrated a new function of Ire1 through genetic analyses of P. pastoris cells. In addition to splicing of HAC1 mRNA, Ire1 suppressed cytosolic and/or nuclear protein aggregation and the HSR, possibly by avoiding excessive production of ribosomal proteins. The role of Ire1 to mitigate the HSR was also observed under heat stress conditions, and Ire1 conferred heat resistance to P. pastoris cells. Further studies are required to elucidate whether this insight is applicable to other yeast and fungal species.
Importance of this research
In eukaryotic cells, secretory and cell-surface proteins are mainly folded in the endoplasmic reticulum (ER) while cytosolic and nuclear proteins are folded in the cytosol. Dysfunction of the ER, namely, ER stress, is accompanied by the accumulation of unfolded proteins in the ER and provokes the unfolded protein response (UPR), by which ER-located molecular chaperones and their co-factors are transcriptionally induced. The ER-located transmembrane protein Ire1 is the most prominent ER stress sensor that triggers the UPR. On the other hand, the heat shock response (HSR) is provoked by the accumulation of unfolded proteins in the cytosol or the nuclei, resulting in transcriptional induction of cytosolic molecular chaperones and their co-factors. Here we show that in addition to UPR induction, Ire1 functions to attenuate cytosolic/nuclear protein aggregation and the HSR in cells of the methylotrophic yeast Pichia pastoris (syn. Komagataella phaffi). Moreover, Ire1 was activated by heat stress to confer heat resistance to P. pastoris cells. Our findings presented here reveal a previously unknown case in which the UPR and HSR are tightly coupled.
Data availability statement
The datasets presented in this study can be found in online repositories. The names of the repository/repositories and accession number(s) can be found in the article/Supplementary material.
Author contributions
YK led the project and wrote the final manuscript. YF designed and performed the experiments. YF and YY constructed yeast strains. All authors have read and approved the final manuscript.
Funding
This research was funded by JSPS KAKENHI (grant number 22 K19135) to YK and a research grant to YK from Noda Institute for Scientific Research.
Acknowledgments
The authors deeply thank Kenji Kohno and Hiroshi Takagi (Nara Inst. Sci. Tech.) for a wide variety of supports, which were indispensable for this study. The authors are also grateful to Diethard Mattanovich and Brigitte Gasser (BOKU) for instruction of Pichia techniques.
Conflict of interest
The authors declare that the research was conducted in the absence of any commercial or financial relationships that could be construed as a potential conflict of interest.
Publisher’s note
All claims expressed in this article are solely those of the authors and do not necessarily represent those of their affiliated organizations, or those of the publisher, the editors and the reviewers. Any product that may be evaluated in this article, or claim that may be made by its manufacturer, is not guaranteed or endorsed by the publisher.
Supplementary material
The Supplementary material for this article can be found online at: https://www.frontiersin.org/articles/10.3389/fmicb.2023.1157146/full#supplementary-material
References
Ata, Ö., Ergün, B. G., Fickers, P., Heistinger, L., Mattanovich, D., Rebnegger, C., et al. (2021). What makes Komagataella phaffii non-conventional? FEMS Yeast Res. 21:foab059. doi: 10.1093/femsyr/foab059
Braakman, I., and Hebert, D. N. (2013). Protein folding in the endoplasmic reticulum. Cold Spring Harb. Perspect. Biol. 5:a013201. doi: 10.1101/cshperspect.a013201
Caplan, A. J., and Douglas, M. G. (1991). Characterization of YDJ1: a yeast homologue of the bacterial dnaJ protein. J. Cell Biol. 114, 609–621. doi: 10.1083/jcb.114.4.609
Chen, S., and Qiu, G. (2020). Heat-stress induced expression of stress-inducible nucleotide exchange factor Fes1 in seagrass Zostera japonica. Ecotoxicology 29, 932–940. doi: 10.1007/s10646-020-02185-5
Coelho, D. S., and Domingos, P. M. (2014). Physiological roles of regulated Ire1 dependent decay. Front. Genet. 5:76. doi: 10.3389/fgene.2014.00076
Cox, J. S., and Walter, P. (1996). A novel mechanism for regulating activity of a transcription factor that controls the unfolded protein response. Cells 87, 391–404. doi: 10.1016/S0092-8674(00)81360-4
Deng, Y., Humbert, S., Liu, J. X., Srivastava, R., Rothstein, S. J., and Howell, S. H. (2011). Heat induces the splicing by IRE1 of a mRNA encoding a transcription factor involved in the unfolded protein response in Arabidopsis. Proc. Natl. Acad. Sci. U. S. A. 108, 7247–7252. doi: 10.1073/pnas.1102117108
Di Santo, R., Aboulhouda, S., and Weinberg, D. E. (2016). The fail-safe mechanism of post-transcriptional silencing of unspliced HAC1 mRNA. elife 5:e20069. doi: 10.7554/eLife.20069
Fauzee, Y. N. B. M., Taniguchi, N., Ishiwata-Kimata, Y., Takagi, H., and Kimata, Y. (2020). The unfolded protein response in Pichia pastoris without external stressing stimuli. FEMS Yeast Res. 20:foaa053. doi: 10.1093/femsyr/foaa053
Feng, X., Krishnan, K., Richie, D. L., Aimanianda, V., Hartl, L., Grahl, N., et al. (2011). HacA-independent functions of the ER stress sensor IreA synergize with the canonical UPR to influence virulence traits in Aspergillus fumigatus. PLoS Pathog. 7:e1002330. doi: 10.1371/journal.ppat.1002330
Gassler, T., Heistinger, L., Mattanovich, D., Gasser, B., and Prielhofer, R. (2019). CRISPR/Cas9-mediated homology-directed genome editing in Pichia pastoris. Methods Mol. Biol. 1923, 211–225. doi: 10.1007/978-1-4939-9024-5_9
Graf, A., Gasser, B., Dragosits, M., Sauer, M., Leparc, G. G., Tüchler, T., et al. (2008). Novel insights into the unfolded protein response using Pichia pastoris specific DNA microarrays. BMC Genomics 9:390. doi: 10.1186/1471-2164-9-390
Hamdan, N., Kritsiligkou, P., and Grant, C. M. (2017). ER stress causes widespread protein aggregation and prion formation. J. Cell Biol. 216, 2295–2304. doi: 10.1083/jcb.201612165
Hata, T., Ishiwata-Kimata, Y., and Kimata, Y. (2022). Induction of the unfolded protein response at high temperature in Saccharomyces cerevisiae. Int. J. Mol. Sci. 23:1669. doi: 10.3390/ijms23031669
Ishiwata-Kimata, Y., Promlek, T., Kohno, K., and Kimata, Y. (2013). BiP-bound and nonclustered mode of Ire1 evokes a weak but sustained unfolded protein response. Genes Cells 18, 288–301. doi: 10.1111/gtc.12035
Kabani, M., Beckerich, J. M., and Brodsky, J. L. (2002). Nucleotide exchange factor for the yeast Hsp70 molecular chaperone Ssa1p. Mol. Cell. Biol. 22, 4677–4689. doi: 10.1128/MCB.22.13.4677-4689.2002
Kimata, Y., Ishiwata-Kimata, Y., Yamada, S., and Kohno, K. (2006). Yeast unfolded protein response pathway regulates expression of genes for anti-oxidative stress and for cell surface proteins. Genes Cells 11, 59–69. doi: 10.1111/j.1365-2443.2005.00921.x
Kimmig, P., Diaz, M., Zheng, J., Williams, C. C., Lang, A., Aragón, T., et al. (2012). The unfolded protein response in fission yeast modulates stability of select mRNAs to maintain protein homeostasis. elife 1:e00048. doi: 10.7554/eLife.00048
Kohno, K., Normington, K., Sambrook, J., Gething, M. J., and Mori, K. (1993). The promoter region of the yeast KAR2 (BiP) gene contains a regulatory domain that responds to the presence of unfolded proteins in the endoplasmic reticulum. Mol. Cell. Biol. 13, 877–890.
Küberl, A., Schneider, J., Thallinger, G. G., Anderl, I., Wibberg, D., Hajek, T., et al. (2011). High-quality genome sequence of Pichia pastoris CBS7435. J. Biotechnol. 154, 312–320. doi: 10.1016/j.jbiotec.2011.04.014
Le, Q. G., Ishiwata-Kimata, Y., Phuong, T. H., Fukunaka, S., Kohno, K., and Kimata, Y. (2021). The ADP-binding kinase region of Ire1 directly contributes to its responsiveness to endoplasmic reticulum stress. Sci. Rep. 11:4506. doi: 10.1038/s41598-021-83890-x
Le, Q. G., and Kimata, Y. (2021). Multiple ways for stress sensing and regulation of the endoplasmic reticulum-stress sensors. Cell Struct. Funct. 46, 37–49. doi: 10.1247/csf.21015
Lee, K. P., Dey, M., Neculai, D., Cao, C., Dever, T. E., and Sicheri, F. (2008). Structure of the dual enzyme Ire1 reveals the basis for catalysis and regulation in nonconventional RNA splicing. Cells 132, 89–100. doi: 10.1016/j.cell.2007.10.057
Li, W., Crotty, K., Garrido, R. D., Voorhies, M., Rivera, C., Sil, A., et al. (2021). Protomer alignment modulates specificity of RNA substrate recognition by Ire1. elife 10:e67425. doi: 10.7554/eLife.67425
Li, Y., Humbert, S., and Howell, S. H. (2012). ZmbZIP60 mRNA is spliced in maize in response to ER stress. BMC. Res. Notes 5:144. doi: 10.1186/1756-0500-5-144
Li, Z., Tang, J., Srivastava, R., Bassham, D. C., and Howell, S. H. (2020). The transcription factor bZIP60 links the unfolded protein response to the heat stress response in maize. Plant Cell 32, 3559–3575. doi: 10.1105/tpc.20.00260
Liu, Y., and Chang, A. (2008). Heat shock response relieves ER stress. EMBO J. 27, 1049–1059. doi: 10.1038/emboj.2008.42
Melo, T. Q., Copray, S. J. C. V., and Ferrari, M. F. R. (2018). Alpha-Synuclein toxicity on protein quality control, mitochondria and endoplasmic reticulum. Neurochem. Res. 43, 2212–2223. doi: 10.1007/s11064-018-2673-x
Morano, K. A., Liu, P. C., and Thiele, D. J. (1998). Protein chaperones and the heat shock response in Saccharomyces cerevisiae. Curr. Opin. Microbiol. 1, 197–203. doi: 10.1016/S1369-5274(98)80011-8
Mori, K. (2009). Signalling pathways in the unfolded protein response: development from yeast to mammals. J. Biochem. 146, 743–750. doi: 10.1093/jb/mvp166
Mori, K., Ogawa, N., Kawahara, T., Yanagi, H., and Yura, T. (2000). mRNA splicing-mediated C-terminal replacement of transcription factor Hac1p is required for efficient activation of the unfolded protein response. Proc. Natl. Acad. Sci. U. S. A. 97, 4660–4665. doi: 10.1073/pnas.050010197
Nguyen, P. T. M., Ishiwata-Kimata, Y., and Kimata, Y. (2022). Fast-growing Saccharomyces cerevisiae cells with a constitutive unfolded protein response and their potential for lipidic molecule production. Appl. Environ. Microbiol. 88:e0108322. doi: 10.1128/aem.01083-22
Niwa, M., Patil, C. K., Derisi, J., and Walter, P. (2005). Genome-scale approaches for discovering novel nonconventional splicing substrates of the Ire1 nuclease. Genome Biol. 6:R3. doi: 10.1186/gb-2004-6-1-r3
Pobre, K. F. R., Poet, G. J., and Hendershot, L. M. (2019). The endoplasmic reticulum (ER) chaperone BiP is a master regulator of ER functions: getting by with a little help from ERdj friends. J. Biol. Chem. 294, 2098–2108. doi: 10.1074/jbc.REV118.002804
Puxbaum, V., Mattanovich, D., and Gasser, B. (2015). Quo vadis? The challenges of recombinant protein folding and secretion in Pichia pastoris. Appl. Microbiol. Biotechnol. 99, 2925–2938. doi: 10.1007/s00253-015-6470-z
Ramírez-Zavala, B., Krüger, I., Dunker, C., Jacobsen, I. D., and Morschhäuser, J. (2022). The protein kinase Ire1 has a Hac1-independent essential role in iron uptake and virulence of Candida albicans. PLoS Pathog. 18:e1010283. doi: 10.1371/journal.ppat.1010283
Rüegsegger, U., Leber, J. H., and Walter, P. (2001). Block of HAC1 mRNA translation by long-range base pairing is released by cytoplasmic splicing upon induction of the unfolded protein response. Cells 107, 103–114. doi: 10.1016/S0092-8674(01)00505-0
Schuck, S., Prinz, W. A., Thorn, K. S., Voss, C., and Walter, P. (2009). Membrane expansion alleviates endoplasmic reticulum stress independently of the unfolded protein response. J. Cell Biol. 187, 525–536. doi: 10.1083/jcb.200907074
Schuldiner, M., Collins, S. R., Thompson, N. J., Denic, V., Bhamidipati, A., Punna, T., et al. (2005). Exploration of the function and organization of the yeast early secretory pathway through an epistatic miniarray profile. Cells 123, 507–519. doi: 10.1016/j.cell.2005.08.031
Sidrauski, C., and Walter, P. (1997). The transmembrane kinase Ire1p is a site-specific endonuclease that initiates mRNA splicing in the unfolded protein response. Cells 90, 1031–1039. doi: 10.1016/S0092-8674(00)80369-4
Tam, A. B., Koong, A. C., and Niwa, M. (2014). Ire1 has distinct catalytic mechanisms for XBP1/HAC1 splicing and RIDD. Cell Rep. 9, 850–858. doi: 10.1016/j.celrep.2014.09.016
Tran, D. M., Ishiwata-Kimata, Y., Mai, T. C., Kubo, M., and Kimata, Y. (2019). The unfolded protein response alongside the diauxic shift of yeast cells and its involvement in mitochondria enlargement. Sci. Rep. 9:12780. doi: 10.1038/s41598-019-49146-5
Tran, D. M., and Kimata, Y. (2018). The unfolded protein response of yeast Saccharomyces cerevisiae and other organisms. Plant Morphol. 30, 15–24. doi: 10.5685/plmorphol.30.15
Travers, K. J., Patil, C. K., Wodicka, L., Lockhart, D. J., Weissman, J. S., and Walter, P. (2000). Functional and genomic analyses reveal an essential coordination between the unfolded protein response and ER-associated degradation. Cells 101, 249–258. doi: 10.1016/S0092-8674(00)80835-1
Tye, B. W., Commins, N., Ryazanova, L. V., Wühr, M., Springer, M., Pincus, D., et al. (2019). Proteotoxicity from aberrant ribosome biogenesis compromises cell fitness. elife 8:e43002. doi: 10.7554/eLife.43002
Urano, F., Wang, X., Bertolotti, A., Zhang, Y., Chung, P., Harding, H. P., et al. (2000). Coupling of stress in the ER to activation of JNK protein kinases by transmembrane protein kinase IRE1. Science 287, 664–666. doi: 10.1126/science.287.5453.664
Whyteside, G., Nor, R. M., Alcocer, M. J., and Archer, D. B. (2011). Activation of the unfolded protein response in Pichia pastoris requires splicing of a HAC1 mRNA intron and retention of the C-terminal tail of Hac1p. FEBS Lett. 585, 1037–1041. doi: 10.1016/j.febslet.2011.02.036
Wu, S., and Letchworth, G. J. (2004). High efficiency transformation by electroporation of Pichia pastoris pretreated with lithium acetate and dithiothreitol. BioTechniques 36, 152–154. doi: 10.2144/04361DD02
Keywords: unfolded protein response, yeast, Pichia pastoris , endoplasmic reticulum, stress response
Citation: Fauzee YNBM, Yoshida Y and Kimata Y (2023) Endoplasmic stress sensor Ire1 is involved in cytosolic/nuclear protein quality control in Pichia pastoris cells independent of HAC1. Front. Microbiol. 14:1157146. doi: 10.3389/fmicb.2023.1157146
Edited by:
Dimitris G. Hatzinikolaou, National and Kapodistrian University of Athens, GreeceCopyright © 2023 Fauzee, Yoshida and Kimata. This is an open-access article distributed under the terms of the Creative Commons Attribution License (CC BY). The use, distribution or reproduction in other forums is permitted, provided the original author(s) and the copyright owner(s) are credited and that the original publication in this journal is cited, in accordance with accepted academic practice. No use, distribution or reproduction is permitted which does not comply with these terms.
*Correspondence: Yukio Kimata, a2ltYXRhQGJzLm5haXN0Lmpw