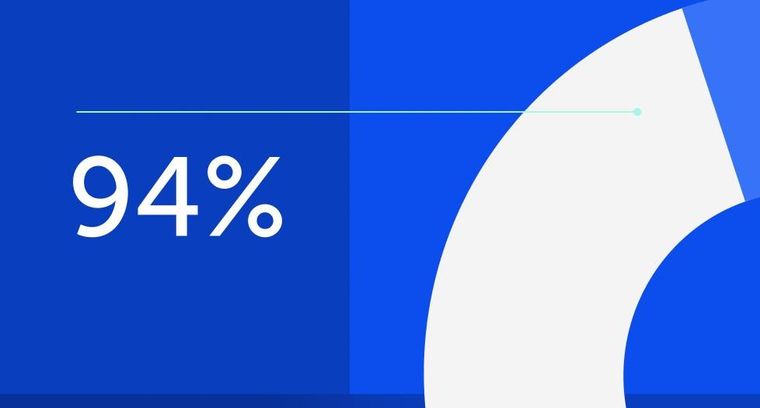
94% of researchers rate our articles as excellent or good
Learn more about the work of our research integrity team to safeguard the quality of each article we publish.
Find out more
METHODS article
Front. Microbiol., 14 April 2023
Sec. Food Microbiology
Volume 14 - 2023 | https://doi.org/10.3389/fmicb.2023.1156058
This article is part of the Research TopicProbiotics for Nutrition Research in Health and DiseaseView all 15 articles
Aim: Antibiotic-associated diarrhea (AAD) is a common side effect during antibiotic treatment, which can cause dysbacteriosis of the gut microbiota. Previous studies have shown beneficial effects in AAD treatment with Bifidobacterium lactis XLTG11, Lactobacillus casei Zhang, Lactobacillus plantarum CCFM8661, and Lactobacillus rhamnosus Probio-M9. However, no studies have been conducted on the immunomodulatory effects and protective intestinal barrier function of four complex probiotics. The aim of our study is to investigate the alleviation effects of complex probiotics on ampicillin-induced AAD.
Methods: Thirty-six BALB/c mice were randomly divided into six groups: normal control group (NC), model control group (MC), low-, medium-, and high-dose probiotics groups (LD, MD, and HD), and positive drug (Bifico, 1 × 107 cfu) control group (PDC; Bifico, also known as Bifidobacterium Triple Live Capsule, is composed of Bifidobacterium longum, Lactobacillus acidophilus, and Enterococcus faecalis). An AAD model was established by intragastric administration of ampicillin, by gavage of different doses of complex probiotics and Bifico. The weight gain, fecal water content, loose stool grade, intestinal permeability, total protein and albumin levels, intestinal barrier, cytokine levels, and gut microbiota were determined.
Results: The results showed that complex probiotics significantly decreased the fecal water content, loose stool grade, intestinal permeability, and ileum tissue damage. Their application increased the weight gain, SIgA, TP, and ALB levels. Additionally, complex probiotics significantly decreased the levels of pro-inflammatory cytokines and increased those of anti-inflammatory cytokines. Meanwhile, the mRNA expression levels of ZO-1, occludin, claudin-1, and MUC2 were significantly upregulated in the probiotic-treated group. Furthermore, the complex probiotics increased the gut microbiota diversity and modulated the changes in the gut microbiota composition caused by ampicillin. At the phylum level, the abundance of Proteobacteria in the HD group was lower than that in the MC group, whereas that of Bacteroidetes was higher. At the genus level, the abundances of Klebsiella and Parabacteroides in the HD group were lower, whereas those of Bacteroides, Muribaculaceae, and Lactobacillus were higher than those in the MC group. Moreover, Spearman’s correlation analysis also found that several specific gut microbiota were significantly correlated with AAD-related indicators.
Conclusion: We found that complex probiotics improved the diarrhea-related indexes, regulated gut microbiota composition and diversity, increased the expression levels of intestinal protective barrier-related genes, preserved the intestinal barrier function, and relieved inflammation and intestinal injury, thereby effectively improving AAD-associated symptoms.
Antibiotics are used to treat illnesses caused by pathogenic infections. Antibiotic-associated diarrhea (AAD) is diarrhea that occurs after the use of antibiotics. It is a common adverse drug reaction (Shao et al., 2020; Yang et al., 2022). Approximately 5–35% of the patients receiving antibiotics have AAD, whose severity ranges from mild to life-threatening (Goodman et al., 2021). The pathogenesis of AAD varies, but the most common mechanism is by damage of the intestinal physiology and microbial community structure caused by antibiotics, resulting in gut dysbiosis (Silverman et al., 2017; Zeng et al., 2019; Mekonnen et al., 2020). Conventional AAD therapy is based on the use of metronidazole and vancomycin and has serious side effects (Silverman et al., 2017). Therefore, it is important to find effective drugs with fewer side effects to alleviate AAD symptoms.
The intestinal tract is the body’s digestive system, and the gut microbiota is the largest and most complex microecological system in the human body. It exerts important immune functions and contributes to host defense against pathogens, plays an important role in the maintenance of intestinal homeostasis, and the development and activation of the host immune system (Nishida et al., 2018; Xu et al., 2023). Probiotics are active microorganisms that are beneficial to the host; they colonize the human body and change the composition of the gut microbiota (Azad et al., 2018; Plaza-Diaz et al., 2019). Probiotics can regulate the gut microbiota environment to improve intestinal health, improve the immune system, and play a beneficial role (Stavropoulou and Bezirtzoglou, 2020; Li Y. et al., 2021). The main immune mechanisms of probiotics include enhancing gastrointestinal mucosal barrier function, activating immune cells, modulating the secretion of immunoglobulins, etc. (La Fata et al., 2018). The supplementation with some probiotics can be done by oral intake; they are safe to use and reportedly had a 7% annual market growth rate worldwide (Zawistowska-Rojek and Tyski, 2018; Peng X. et al., 2022). Probiotics have been confirmed to prevent and improve digestive system diseases, such as acute diarrhea, irritable bowel syndrome (IBS), and AAD (Hempel et al., 2012; Li Z. et al., 2021; Skrzydło-Radomańska et al., 2021; Chen Y. et al., 2022). Bifidobacteria and Lactobacilli are the most important probiotic bacteria of the gut microbiota (Turroni et al., 2018; Fernández-Ciganda et al., 2022). Bifidobacteria was found to exert a protective role in intestinal barrier function in NEC mice by inhibiting proinflammatory cytokine secretion and zonulin protein release, and improving intestinal TJ integrity (Ling et al., 2016). Bifidobacterium pseudocatenulatum regulates the gut microbiota activities and immune functions, participates in the intestinal protective barrier, and inhibits the TLR4/NF-κB pathway (Chen et al., 2021). Oral Lactobacillus administration increased the Paneth cells counts and the intestinal antimicrobial activity in healthy mice (Cazorla et al., 2018).
Previous research has shown that Bifidobacterium lactis XLTG11, Lactobacillus casei Zhang, Lactobacillus plantarum CCFM8661, and Lactobacillus rhamnosus Probio-M9 (Yu et al., 2021; Yao G. et al., 2021; Xu et al., 2022; Zhang et al., 2022) promote immunity and the intestinal protective barrier function. Some studies have shown that probiotic combinations exhibit superior functions in protecting the intestines and regulating the immune system compared to single strains (Chapman et al., 2011). However, the enhancement effects and potential mechanism of complex probiotics consisting of Bifidobacterium lactis XLTG11, Lactobacillus casei Zhang, Lactobacillus plantarum CCFM8661, and Lactobacillus rhamnosus Probio-M9 have not been reported. Broad-spectrum penicillins, cephalosporins, and clindamycin have been observed to cause a high occurrence rate of diarrhea. Ampicillin is a broad-spectrum penicillin antibiotic with antibacterial activity against both Gram-positive and Gram-negative bacteria, and can be used for the development of a model of dysbacteriosis diarrhea (Zhang et al., 2019; Xu et al., 2022). The aim of our study was to investigate the alleviation effects of complex probiotics on the gut microbiota of an AAD mouse model. This study will provide a reference for the development of complex probiotics preparations.
Complex probiotics powder (Bifidobacterium lactis XLTG11, Lactobacillus casei Zhang, Lactobacillus plantarum CCFM8661, and Lactobacillus rhamnosus Probio-M9) was obtained from Jinhua Yinhe Biological Technology Co., Ltd. (Jinhua, China). The complex probiotics powder was stored in a refrigerator at −80°C before the experiment. Normal saline was added to prepare 2.5 × 106, 5 × 106, and 1 × 107 cfu complex probiotics solutions for immediate use.
Six-week-old specific pathogen-free (SPF) BALB/c male mice were purchased from Liaoning Changsheng Biotechnology Co., Ltd. (Shenyang, China). The animals were raised in an animal room with a barrier environment, kept in a room under controlled temperature (20–23°C) and humidity (30–60%) under a 12:12-h light–dark cycle, with free access to feed and water. All animal procedures were carried out in compliance with Heilongjiang University of Traditional Chinese Medicine’s Regulations on the Administration of Laboratory Animals, and the university’s Animal Ethics Committee approved all experiments (ethic approval code: 2021121201).
After 1 week of acclimatization, BALB/c mice were randomly divided into six groups (n = 6), including the normal control group (NC), model control group (MC), positive drug control group (PDC), low-, medium-, and high-dose complex probiotics groups (LD, MD, and HD). The mice in the MC, PDC, LD, MD, and HD groups (except for those in the NC group) received ampicillin by gavage twice daily at a dose of 11.2 g·kg−1 each time. The mice in the NC group were given the same volume of normal saline at the same time every day for 3 consecutive days. After modeling, the mice in the PDC group were given Bifico (1 × 107 cfu) by gavage, and those in the LD, MD, and HD groups were given complex probiotics (2.5 × 106, 5 × 106, and 1 × 107 cfu). For 14 days, the mice in the NC and the MC groups received the same amount of normal saline. After the experiment, blood was sampled from the eyes and stored at −80°C. Fresh feces were collected under sterile conditions and stored at −80°C for gut microbiota analysis. Then, ileum tissue was collected for histopathological analysis and colon tissue for real-time quantitative polymerase chain reaction and ELISA assays.
At 8:00 a.m. on the first day of diarrhea model development, the body weight of the mice in each group was measured and recorded, and then the mice were given ampicillin. At 18:00 every day, the body weight of all mice was also measured and recorded. On the 3rd and 14th day of the experiment, fresh feces of mice in each group were weighed and dried and then weighed again to obtain their dry weight and wet weight, and the degree of loose stool was established using predefined criteria (Table 1).
Further, 1 h after the last administration, blood was sampled from the eyes and let stand for about 1 h, 3,000 r/min−1 centrifuged for 10 min, and then separated the serum. The levels of TP and ALB in the serum samples were determined by ELISA kits (Jiangsu Meimian industrial Co., Ltd., Jiangsu, China).
The levels of LPS and D-LA in the serum samples were determined by ELISA kits (Jiangsu Meimian industrial Co., Ltd., Jiangsu, China).
Ileum tissue was fixed in 4% paraformaldehyde for 48 h and then embedded in paraffin (Wuhan Junjie Electronics Co., Ltd., Wuhan, China), and cut into 4-μm sections (Leica Instrument Shanghai Ltd., Shanghai, China). These slices were dewaxed and then stained with hematoxylin–eosin (HE; Servicebio Co., Ltd., Wuhan, China), then photographed and observed under a light microscope (Nikon Eclipse Ci-L, 100× magnification; Nikon, Tokyo, Japan).
Real-time quantitative PCR (RT-PCR) was used to determine the relative mRNA levels of tight junction protein genes (ZO-1, claudin-1, and occludin) and MUC2. Appropriate samples of colon tissue were taken and homogenized. Using the TaKaRa Kit, total RNA was extracted from the colon tissue. Then, mRNA was subjected to reverse transcription into cDNA with PrimeScriptTmRT reagent kit with gDNA Eraser manufactured (TaKaRa). The TB Green® Premix Ex Taq™II kit (TaKaRa) was used to prepare the reaction solution and amplification was performed using a real-time fluorescent quantitative PCR system (QuantStudio 3). The sequences of the primers are presented in Table 1. The following reaction conditions were applied: pre-denaturation 95°C (30 s), PCR 95°C (5 s), 60°C (34 s), repeated for 40 cycles to determine the Ct value of specific gene and the Ct value of β-actin. The expression of genes was examined using the 2-ΔΔCt method (Table 2).
Colon tissues (100 mg) were homogenized in 900 μL of ice-cold PBS using a homogenizer, and the supernatant was then transferred into sterile tubes after centrifugation at 10,000 rpm for 10 min at 4°C. The levels of SIgA, IL-6, IL-1β, and TNF-α in the colon tissue were determined with ELISA kits (Jiangsu Meimian industrial Co., Ltd., Jiangsu, China).
The 16S amplicon sequencing and analysis were conducted by OE Biotech Co., Ltd. (Shanghai, China). Next, we utilized the MagPure Soil DNA LQ Kit (Shanghai Magen Biotechnology Co., Ltd., Shanghai, China) to extract genomic DNA from the fecal samples of each group (n = 6). The purity and quantity of DNA were confirmed using agarose gel and NanoDrop. Afterward, the bacterial 16S rRNA gene V3-V4 region was amplified using the method/manual of the manufacturer by polymerase chain reaction (PCR) with the forward primer 343F (5′- TACGGRAGGCAGCAG −3′) and the reverse primer 798R (5′- AGGGTATCTAATCCT-3′). Amplicon quality was visualized using gel electrophoresis, purified with Agencourt AMPure Beads (Beckman Coulter, Indianapolis, IN), and amplified for another round of PCR. The final amplicon was measured using the dsDNA HS Assay Kit for Qubit after being once more purified with AMPure XP beads (Yeasen Biotechnology Co., Ltd., Shanghai, China). Sequencing was performed on the Illumina Miseq platform (Illumina Inc., San Diego, CA, United States).
The data of the present study were statistically analyzed using SPSS 26.0 software (IBM, Armonk, NY, United States) and are expressed as mean ± SD. One-way ANOVA was employed for comparing differences between groups, followed by Student–Newman–Keuls (S-N-K) test. p < 0.05 was considered to indicate a statistically significant difference.
Significant differences in the weight gain, fecal water content, and loose stool grade were observed between the NC group and the MC group (p < 0.001). Compared with the MC group, the fecal water content of mice in the PDC, LD, MD, and HD groups decreased in a dose-dependent manner by 25.40% (p < 0.05), 18.15% (p > 0.05), 27.16% (p < 0.05), 37.52% (p < 0.01), respectively; the loose stool grade decreased by 31.49% (p < 0.001), 21.55% (p < 0.001), 27.62% (p < 0.001), and 33.70% (p < 0.001), respectively. Compared with the MC group, the weight gain of the PDC, MD, and HD groups increased by 69.46% (p < 0.05), 62.83% (p > 0.05), and 125.67% (p < 0.001). The weight gain in the LD group was by 46.61% lower (p < 0.05) than that in the PDC group. The results showed that the high-dose of the complex probiotics not only significantly improved the diarrhea symptoms of the AAD model mice, but also promoted their growth. The therapeutic effect of Bifico was better than that of the low-dose complex probiotic (Figures 1A–C).
Figure 1. Effects of complex probiotics on general mice effects in AAD model mice (n = 6). (A) Weight gain; (B) Fecal water content; (C) Loose stool grade; (D) LPS; (E) D-LA. NC, normal control group; MC, model control group; PDC, Positive drug control group; LD, low-dose complex probiotics group; MD, medium-dose complex probiotics group; HD, high-dose complex probiotics group. All data are expressed as mean ± SD. Compared with the NC group **p < 0.01, ***p < 0.001; Compared with the MC group #p < 0.05, ##p < 0.01, ###p < 0.001; Compared with the PDC group ^p < 0.05, ^^p < 0.01.
The contents of LPS and D-LA in the MC group were higher than those in the NC group by 24.87 and 18.59% (p < 0.01 or p < 0.001), respectively, indicating that ampicillin changed the intestinal permeability of the mice. The content of LPS in the PDC, MD, and HD groups was lower than that in the MC group by 19.15% (p < 0.01), 15.46% (p < 0.05), and 16.88% (p < 0.05), correspondingly; the contents of D-LA in these three groups were lower by 12.14% (p < 0.01), 5.99% (p > 0.05), and 11.44% (p < 0.01), respectively, than that in the MC group. Compared with the PDC group, the content of D-LA in the LD group increased by 12.26% (p < 0.01). These findings revealed that the high-dose of complex probiotic had a good effect on reducing intestinal barrier permeability. The therapeutic effect of the low dose of the complex probiotic was weaker than that of Bifico (Figures 1D,E).
Compared with the NC group, the levels of TP and ALB in the serum in the MC group were reduced by 25.09% (p < 0.01) and 16.45% (p < 0.05). Compared with the MC group, the level of TP in the PDC, MD, and HD groups increased by 28.12% (p < 0.05), 36.40% (p < 0.01), 55.06% (p < 0.001), and the level of ALB increased by 19.32% (p < 0.05), 35.23% (p < 0.001), and 37.28% (p < 0.001). The levels of TP in the HD group were higher than those in the PDC group by 21.02% (p < 0.05), indicating that the high-dose complex probiotic have a better therapeutic effect than Bifico (Figures 2A,B).
Figure 2. Effects of complex probiotics on the index of intestinal immune barrier in AAD model mice (n = 6). (A) TP; (B) ALB; (C) SIgA; (D) IL-1β; (E) IL-10; (F) TNF-α; (G) IL-6. NC, normal control group; MC, model control group; PDC, Positive drug control group; LD, low-dose complex probiotics group; MD, medium-dose complex probiotics group; HD, high-dose complex probiotics group. All data are expressed as mean ± SD. Compared with the NC group *p < 0.05, **p < 0.01, ***p < 0.001; Compared with the MC group #p < 0.05, ##p < 0.01, ###p < 0.001; Compared with the PDC group ^p < 0.05.
We found that the levels of IL-6, IL-1β, and TNF-α in the colon tissue in the MC group were higher than those in the NC group by 37.66% (p < 0.001), 27.95% (p < 0.01), 23.89% (p < 0.01), respectively, whereas the levels of IL-10 and SIgA were lower by 15.01% (p < 0.001) and 20.58% (p < 0.001), correspondingly. These results indicated that ampicillin triggered inflammation by increasing the secretion of pro-inflammatory factors and decreasing the secretion of anti-inflammatory factors. Compared with the MC group, the levels of SIgA in the PDC, LD, MD, and HD groups increased by 12.33% (p < 0.05), 10.66% (p < 0.05), 13.67% (p < 0.05), and 20.15% (p < 0.001), respectively. The levels of IL-6 decreased by 16.54% (p < 0.05), 10.31% (p > 0.05), 14.97% (p < 0.05), and 18.59% (p < 0.01), correspondingly. The levels of IL-1β decreased by 21.42% (p < 0.01), 10.28% (p > 0.05), 13.81% (p < 0.05), and 19.34% (p < 0.01), respectively, whereas the levels of IL-10 increased by 14.39% (p < 0.01), 4.19% (p > 0.05), 10.90% (p < 0.05), and 16.86% (p < 0.01), correspondingly. These results suggested that the complex probiotics decreased the levels of IL-6, IL-1β, and increased the levels of IL-10 in the colon tissue in a dose-dependent manner. Compared with the MC group, the levels of TNF-α in the HD group decreased by 14.29% (p < 0.05). However, there was no significant difference in the levels of TNF-α between the MD group and the MC group (p > 0.05). Compared with the PDC group, the levels of TNF-α in the LD group increased by 14.80% (p < 0.05), the levels of IL-10 decreased by 8.92% (p < 0.05). These results showed that the high-dose complex probiotics regulated the level of inflammatory factors and promoted the immune function of the AAD model mice. The therapeutic effect of the low-dose complex probiotic was weaker than that of Bifico (Figures 2C–G).
The photomicrographs of (HE)-stained intestinal sections are displayed in Figure 3A. In the NC group, the small intestinal mucosa structure was intact, and the villi were arranged closely and neatly. In the MC group, the small intestinal mucosa was damaged severely, and the villi were arranged sparsely. The probiotic administration attenuated the damage degree of the small intestinal mucosa in different degrees in a dose-dependent manner. These findings showed that ampicillin can damage the intestinal structural integrity, whereas the complex probiotic effectively prevented and ameliorated the intestinal damage in a dose-dependent fashion.
Figure 3. Effects of complex probiotics on the intestinal in AAD model mice (n = 6). (A) HE staining of jejunum section (×100), (B) ZO-1, (C) occludin, (D) claudin-1, (E) MUC2. NC, normal control group; MC, model control group; PDC, Positive drug control group; LD, low-dose complex probiotics group; MD, medium-dose complex probiotics group; HD, high-dose complex probiotics group. All data are expressed as mean ± SD. Compared with the NC group ***p < 0.001; Compared with the MC group #p < 0.05, ##p < 0.01, ###p < 0.001; Compared with the PDC group ^p < 0.05, ^^p < 0.01, ^^^p < 0.001.
The mRNA expression levels of ZO-1, occludin, claudin-1, and MUC2 in the MC group were lower than those in the NC group by 60.74% (p < 0.001), 51.20% (p < 0.001), 51.83% (p < 0.001), and 61.24% (p < 0.001), respectively, indicating that the AAD model was successfully established. Compared with the MC group, the mRNA expression levels of ZO-1 in the PDC, LD, MD, and HD groups increased by 72.17% (p < 0.01), 44.26% (p > 0.05), 64.58% (p < 0.01), and 119.76% (p < 0.001), the mRNA expression levels of occludin increased by 86.40% (p < 0.001), 42.83% (p < 0.05), 55.74% (p < 0.01), and 98.03% (p < 0.001), the mRNA expression levels of claudin-1 increased by 86.55% (p < 0.001), 33.02% (p < 0.05), 36.27% (p < 0.05), and 82.05% (p < 0.001), and the mRNA expression levels of MUC2 in the PDC and HD groups increased by 47.44% (p < 0.01) and 81.03% (p < 0.001). However, there was no significant difference in the mRNA expression levels of MUC2 between the MD group and the MC group. Compared with the PDC group, the mRNA expression levels of ZO-1 in the HD group were increased by 27.24% (p < 0.05), the mRNA expression levels of occludin and MUC2 in the LD group were reduced by 23.37% (p < 0.05) and 35.35% (p < 0.01). The mRNA expression levels of MUC2 in the HD group were 22.78% higher (p < 0.05), whereas the mRNA expression levels of claudin-1 in the LD and MD groups were lower by 28.69% (p < 0.001) and 26.95% (p < 0.001), respectively. The aforementioned results indicated that the high dose of the complex probiotics effectively alleviated the damage of the intestinal barrier functional protein in the AAD model mice (Figures 3B–E).
The species richness and evenness of distribution within a sample were assessed by alpha-diversity analysis. The Chao1, Shannon, and Simpson indices were employed for the evaluations in this experiment. The Chao1, Shannon, and Simpson indices in the MC group were significantly lower than those in the NC group (p < 0.01). Notably, the administration of the high-dose complex probiotics significantly increased the values of the Chao1, Shannon, and Simpson indices (p < 0.05 or p < 0.01). The combined use of the complex probiotics effectively promoted the diversity and uniformity of the gut microbiota (Figures 4A–C).
Figure 4. Effects of complex probiotics on the diversity and structure of gut microbiota in AAD model mice (n = 6). (A) Chao1; (B) Shannon; (C) Simpson; (D) Principle coordinate analysis (PCoA); (E) Gut microbiota composition at the phylum level; (F) Gut microbiota composition at the genus level; (G) The abundance of Bacteroidetes; (H) The abundance of Proteobacteria; (I) The abundance of Bacteroides; (J) The abundance of Muribaculaceae. NC, normal control group; MC, model control group; PDC, Positive drug control group; LD, low-dose complex probiotics group; MD, medium-dose complex probiotics group; HD, high-dose complex probiotics group. All data are expressed as mean ± SD. Compared with the NC group ***p < 0.001; Compared with the MC group #p < 0.05, ##p < 0.01, ###p < 0.001; Compared with the PDC group ^^p < 0.01, ^^^p < 0.001.
Principal coordinates analysis was applied to assess community similarity and difference (PCoA). The results showed that the gut microbiota of the MC and NC groups were significantly different, indicating that the AAD model was successfully developed. Significant differences were found between the microflora structure of the MC group and the complex probiotics groups (Figure 4D).
At the phylum level, the composition of the intestinal microorganisms in each group at the phylum level included Bacteroidetes, Firmicutes, Proteobacteria, Epsilonbacteraeota, and Actinobacteriota. Of them, Bacteroidetes, Firmicutes, and Proteobacteria were the dominant flora representatives in the intestinal tract. The relative abundance of Bacteroidetes in the MC group (p < 0.001) was lower, whereas the relative abundance of Proteobacteria was higher (p < 0.001). The obtained results indicated that ampicillin transformed the composition of the gut microbiota in the various groups, leading to the overgrowth of Proteobacteria but restricted growth of Bacteroidetes, which was generally considered to be a feature of dysbiosis. Compared with the MC group, the relative abundance of Bacteroidetes in the MD and HD groups increased by 20.93% (p < 0.05), 57.76% (p < 0.001), and the relative abundance of Proteobacteria decreased by 37.06% (p < 0.001) and 98.25% (p < 0.001). Compared with the PDC group, the relative abundance of Bacteroidetes in the LD group decreased by 19.46% (p < 0.01), the relative abundance of Bacteroidetes in the HD group increased by 21.96% (p < 0.001), and the relative abundance of Proteobacteria in the HD group decreased by 97.03% (p < 0.001). Therefore, the complex probiotics were found to significantly alter the relative abundance of various phyla after antibiotic administration (Figures 4E,G,H).
At the genus level, the composition of the intestinal microorganisms in each group at the genus level included Bacteroides, Muribaculaceae, Klebsiella, Lactobacillus, and Parabacteroides. Compared with the NC group, the relative abundance of Bacteroides and Muribaculaceae in the MC group decreased by 62.06% (p < 0.001) and 75.78% (p < 0.001). The relative abundance of Klebsiella and Parabacteroides in the MC group were increased. Compared with the MC group, the abundance of Klebsiella and Parabacteroides in the HD group were reduced, the relative abundance of Bacteroides in the MD and HD groups increased by 71.51% (p < 0.01), 135.12% (p < 0.001), and the relative abundance of Muribaculaceae increased by 38.69% (p > 0.05), 202.28% (p < 0.001). Compared with the PDC group, the relative abundance of Bacteroides in the LD and MD groups decreased by 34.77% (p < 0.001) and 29.83% (p < 0.01), the relative abundance of Muribaculaceae in the LD, MD, and HD groups decreased by 66.72% (p < 0.001), 65.11% (p < 0.001), and 23.97% (p < 0.001). The complex probiotics supplementation after antibiotic administration significantly altered the relative intestinal microbial abundance at the genus level (Figures 4F,I,J).
To understand the involved and interacted roles of the intestinal microbiota in probiotics-mediated benefits, the correlation analysis between diarrhea-related indexes, cytokine levels, intestinal protective barrier-related genes expression, and dominant gut microbiota at the genus levels was analyzed. The relative abundance of Bacteroides and Muribaculaceae were significantly positively correlated with weight gain, TP, ALB, tight junction proteins (ZO-1, occludin, and claudin-1), MUC2, SIgA, and IL-10, while was significantly negatively correlated with fecal water content, loose stool grade, LPS, D-LA, and pro-inflammation cytokines (IL-1β, TNF-α, and IL-6). Klebsiella and Parabacteroides were positively associated with fecal water content, loose stool grade, LPS, D-LA, and pro-inflammation cytokines (IL-1β, TNF-α, and IL-6), while were negatively associated with weight gain, TP, ALB, tight junction proteins (ZO-1, occludin, and claudin-1), MUC2, SIgA, and IL-10. However, the Lactobacillus was positively associated with weight gain, TP, ALB, tight junction proteins (ZO-1, occludin, and claudin-1), MUC2, SIgA, and IL-10, but was significantly negatively correlated with fecal water content, LPS, IL-1β, and TNF-α. The relative abundance of Muribaculum was positively associated with occludin and claudin-1, while was significantly negatively correlated with D-LA, IL-1β, and TNF-α. The relative abundance of Lachnospiraceae NK4A136 group was negatively associated with ALB. The relative abundance of Acinetobacter was significantly negatively correlated with claudin-1. The relative abundance of Lachnoclostridium was positively correlated with IL-6, but was negatively correlated with SIgA (Figure 5).
Figure 5. Correlation analysis between diarrhea-related indexes, cytokine levels, intestinal protective barrier-related genes expression, and dominant gut microbiota by Spearman. *, **, and *** indicate the associations significant (p < 0.05, p < 0.01, and p < 0.001, respectively).
Antibiotic use over an extended period alters the balance of the intestinal microbiota, causes an imbalance in the gut microbiota, and can result in AAD (Overeem et al., 2008; Cui et al., 2020). Probiotics, on the other hand, regulate the gut microbiota, increase the integrity of the intestinal barrier, and enhance immunity (Wang X. et al., 2021). Therefore, finding complex probiotics that can better regulate gut microbiota is the first task of anti-AAD researchers.
Different types of probiotics exist, but the most common of them include Lactobacilli and Bifidobacteria, which are widely used in many functional foods and dietary supplements (Turroni et al., 2011; Xue et al., 2017). Studies have demonstrated that Bifidobacterium lactis XLTG11 can regulate the secretion of inflammatory cytokines and gut microbiota activities. Additionally, it produces substantial amounts of acetic, propionic, and butyric acid, which lower the intestinal pH, inhibit the proliferation of harmful bacteria, and strengthen gut protection, thereby improving AAD (Wang N. et al., 2021; Xu et al., 2022; Ma W. et al., 2023; Ma Y. et al., 2023). Additionally, Lactobacillus casei Zhang was established to regulate tight-junction proteins and inflammatory factor expression, alleviating gut microbiota disorders (Wang et al., 2019; Li X. et al., 2021). In addition, it also enhanced immunity by increasing the production of IgA and IFN-γ (Wang et al., 2013), and induced gut mucosal responses by promoting the production of SIgA (Ya et al., 2008). Studies have shown that Lactobacillus plantarum CCFM8661 can regulate the diversity and composition of the gut microbiota, alleviate the pathological damage to the colon and ileum, increase jejunum villus height-crypt depth ratio (Tian et al., 2012; Wang et al., 2019; Yu et al., 2021). Lacticaseibacillus rhamnosus Probio-M9 is a novel probiotic strain isolated from human breast milk of healthy women (Zhang et al., 2022). Oral Probio-M9 administration regulated the stability of the gut microbiota (Zheng et al., 2021). Probio-M9 has demonstrated excellent tolerance to gastrointestinal digestive fluids. Its survival rate after being subjected to artificial gastric fluid for 3 h is 83.72%, and after being subjected to artificial intestinal fluid for 11 h, it is 78.33%. Even when exposed to a pH of 2.5, Probio-M9 can pass through the stomach and reach the intestines to exert its beneficial effects (Liu et al., 2020). Furthermore, Probio-M9 can improve the intestinal environment by increasing the diversity of gut microbiota and regulating metabolic pathways (Xu et al., 2021). Furthermore, research has demonstrated that the symbiotic relationship between Lactobacillus and Bifidobacterium can synergistically enhance the production of metabolites, bolster their antibacterial capabilities, suppress the growth of pathogenic microorganisms, reinforce the integrity of the mucosal barrier, and promote the upregulation of immune responses (Chang et al., 2017). These studies showed that single species can regulate immunity and gut through different mechanisms, and we speculated that the combined use of multiple strains can enhance the immunomodulatory effects and protect the intestinal barrier function. In this experiment, Bifidobacterium lactis XLTG11, Lactobacillus casei Zhang, Lactobacillus plantarum CCFM8661, and Lactobacillus rhamnosus Probio-M9 were mixed at a ratio of 1:1:1:1 to prepare the complex probiotics utilized to study their alleviative effect on ampicillin-induced AAD.
Commonly used indicators to assess the severity of diarrhea include body weight gain, fecal water content, and fecal consistency (Hu et al., 2020; Ma Y. et al., 2023). Bifidobacterium animalis subsp. lactis XLTG11 not only significantly improved the diarrhea symptoms of AAD model mice, but also promoted the growth of mice (Ma Y. et al., 2023), which was consistent with our results. LPS and D-LA are important indicators to detect the degree of intestinal damage and permeability (Li et al., 2020; Ma Y. et al., 2023). Our results showed that the high-dose of the complex probiotics reduced the contents of LPS and D-LA to decrease intestinal permeability, which was similar to the results of Xu et al. (2022). TP can be divided into albumin and globulin. Serum protein can maintain the normal colloid osmotic pressure and PH of the blood, transport various metabolites, and regulate immune function. The results we obtained showed that the complex probiotics increased the levels of TP and ALB in the serum. These findings are similar to those of Jiang et al., who found that Smilax glabra extract elevated the levels of TP and ALB in antibiotic-associated diarrhea mice, and repaired the damage of the intestinal immune barrier (Jiang et al., 2022).
A primary function of the intestinal epithelium is to form a biological barrier that prevents the invasion of pathogenic antigens and maintains intestinal homeostasis (Feng et al., 2019; Jiang et al., 2021). Tight junctions are the main connection type between intestinal epithelial cells (Peterson and Artis, 2014). ZO-1, occludin, and claudin-1 are the major intestinal barrier proteins. MUC2 participates in the synthesis and secretion of goblet cells, which affect the permeability and integrity of the intestinal mucosa (Zhang et al., 2018; Zhuang et al., 2019). Lack of tight junction protein and MUC2 can induce intestinal epithelial dysfunction, increase intestinal barrier permeability, and increase the entry of harmful bacteria, which can lead to inflammation (Furuse et al., 1993; Van der Sluis et al., 2006; Al-Sadi et al., 2011; Pope et al., 2014; Kuo et al., 2021; Yao D. et al., 2021). Our results showed that the expression levels of ZO-1, claudin-1, occludin, and MUC2 in the colon of the MC group were decreased, indicating that ampicillin caused impairment of intestinal barrier function. However, the supplementation with complex probiotics improved intestinal epithelial permeability. In addition, studies have shown that Lactobacillus plantarum can increase the expression levels of ZO-1, occludin, and claudin-1, strengthen epithelial defense functions, and reduce intestinal mucosal permeability (Wang et al., 2018), Bacteroides fragilis can increase the expression levels of MUC2 and maintain the integrity of the intestinal barrier, alleviating symptoms of AAD in mice (Zhang et al., 2018), which are consistent with our results.
Antibiotic-associated diarrhea occurrence is accompanied by systemic inflammation (Ling et al., 2015). IL-6 can induce the differentiation of B cells. IL-6 promotes host defense through the stimulation of acute phase responses, immune reactions, and hematopoiesis (Popko et al., 2010; Tanaka et al., 2014). IL-1β is a major pro-inflammatory cytokine, it will aggravate the damage during chronic disease and acute tissue damage (Lopez-Castejon and Brough, 2011). TNF-α is an inflammatory cytokine, which can cause inflammation or cell apoptosis. TNF-α induces the release of a large number of cytokines, including IL-6, IL-8, and IL-1β by stimulating macrophages (Idriss and Naismith, 2000; Ma et al., 2016). IL-10 is a cytokine with a significant anti-inflammatory effect. It inhibits the release of pro-inflammatory factors, and plays an essential role in maintaining gastrointestinal homeostasis (Iyer and Cheng, 2012; Li et al., 2014). The results of this experiment showed that complex probiotics significantly reduced the levels of IL-6, IL-1β, and TNF-α while increasing the level of IL-10 in the colon. Therefore, complex probiotics have a good anti-inflammatory effect in AAD. Studies have shown that echinacin attenuated LPS-induced secretion and mRNA expression of TNF-α and IL-6, enhanced the secretion and mRNA expression of IL-10, and weakened the inflammatory response, which is consistent with our previous results (Li et al., 2018). SIgA is the main component of the mucosal defense system of the body and plays a decisive role in the first line of defense against diseases (Kumar et al., 2020; Chen S. et al., 2022). Our present results showed that complex probiotics increased the SIgA level in the colon tissue, which was similar to the results of Peng C. et al. (2022).
In this study, we further analyzed the diversity and richness of the intestinal microorganisms at the phylum and genus levels. At the phylum level, Bacteroidetes, Proteobacteria, and Firmicutes were the dominant phyla (Shin et al., 2015; Guan et al., 2018). Bacteroides and Firmicutes were also established to be abundant in the intestinal tract of healthy people (Schultz et al., 2017). An increase in the relative abundance of Proteobacteria led to a decrease in short-chain fatty acid (SCFA) production (Meng et al., 2020), which indicates a risk of infection and metabolic disorders (Shao et al., 2020). Our results showed that ampicillin administration transformed the composition of the gut microbiota in the examined groups, leading to Proteobacteria overgrowth and restricted growth of Bacteroidetes. Notably, the complex probiotic treatment reversed these changes, which was similar to the results obtained by Wang N. et al. (2021). At the genus level, Bacteroides is a Gram-negative, obligate anaerobic bacteria that controls lymphocyte and cytokine expression and suppresses inflammation (Tan et al., 2019). Muribaculateae can reduce cholesterol content and prevent excessive cholesterol levels by regulating intestinal microbiota and its metabolites (Li et al., 2022). Klebsiella is the pathogen of antibiotic-associated hemorrhagic colitis (Högenauer et al., 2006), it was positively correlated with inflammatory factors such as TNF-α and IL-6 (Liang et al., 2022). Lactic acid and other metabolites produced by the Lactobacillus living in the intestine can kill pathogenic microorganisms. In addition, such microbial metabolites also regulate gut microbiota diversity, enhance immunity, improve intestinal function, and maintain normal intestinal homeostasis (Zafar and Saier, 2020). The results of this experiment showed that the treatment with complex probiotics regulated the abundance of Bacteroides, Muribaculateae, Klebsiella, Lactobacillus, and Parabolides, which was similar to earlier results (Xu et al., 2022). All of the aforementioned experimental findings imply that complex probiotics may exert therapeutic effects in patients with diarrhea and gut microbiota imbalance caused by antibiotics.
This study used mice as the animal model for diarrhea because mice share a high genetic similarity with humans; mice have pure strains, which can reduce interference from different strains; mice are small and easy to breed indoors on a large scale. However, there are significant differences between mice and humans in anatomy and physiology. Moreover, the mice model cannot reflect how human lifestyle, diet, age, and other factors affect the gut microbiota and immune system. These limitations may reduce the generalizability and validity of the experimental results, making it hard to apply the research on complex probiotics for treating AAD to clinical practice. Therefore, future research should consider using animal models closer to humans or testing the findings of this study in clinical trials.
In summary, complex probiotics composed of Bifidobacterium lactis XLTG11, Lactobacillus casei Zhang, Lactobacillus plantarum CCFM8661, and Lactobacillus rhamnosus Probio-M9 could alleviate ampicillin-induced AAD in mice including protecting intestinal barrier function, regulating the secretion of proinflammatory factors, the gut microbiota diversity and composition. Our research will provide further evidence and theoretical support for the treatment of antibiotic-associated diarrhea with complex probiotics. In the future, it will be feasible to study the differential therapeutic efficacy among various combinations of microbial strains and explore which strains or combinations are more effective in treating AAD, thereby providing more robust evidence for their clinical application.
The datasets presented in this study can be found in online repositories. The names of the repository/repositories and accession number(s) can be found in the article/Supplementary material.
The animal study was reviewed and approved by all animal procedures were carried out by Heilongjiang University of Traditional Chinese Medicine’s Regulations on the Administration of Laboratory Animals, and the University’s Animal Ethics Committee approved all experiments (Ethic approval code: 2021121201).
WL and SZ participated in the analysis of experimental data, analyzed and discussed the results, drew graphs, and wrote the paper. YW, HB, and SY conducted experiments, analyzed and discussed the results, and helped to revise the manuscript. LH and WM were mainly responsible for conceiving and designing the study, directing and supervising the experiment, and helping to revise the manuscript. All authors contributed to the article and approved the submitted version.
This work was funded by Natural Science Foundation of Heilongjiang Province of China (LH2019H106), Heilongjiang Province Touyan Team.
We would like to thank all authors for their mutual help and hard work, as well as their guidance and help for this experiment.
The authors declare that the research was conducted in the absence of any commercial or financial relationships that could be construed as a potential conflict of interest.
All claims expressed in this article are solely those of the authors and do not necessarily represent those of their affiliated organizations, or those of the publisher, the editors and the reviewers. Any product that may be evaluated in this article, or claim that may be made by its manufacturer, is not guaranteed or endorsed by the publisher.
The Supplementary material for this article can be found online at: https://www.frontiersin.org/articles/10.3389/fmicb.2023.1156058/full#supplementary-material
Al-Sadi, R., Khatib, K., Guo, S., Ye, D., Youssef, M., and Ma, T. (2011). Occludin regulates macromolecule flux across the intestinal epithelial tight junction barrier. Am. J. Physiol. Gastrointest. Liver Physiol. 300, G1054–G1064. doi: 10.1152/ajpgi.00055.2011
Azad, M. A. K., Sarker, M., Li, T., and Yin, J. (2018). Probiotic species in the modulation of gut microbiota: an overview. Biomed. Res. Int. 2018, 9478630–9478638. doi: 10.1155/2018/9478630
Cazorla, S. I., Maldonado-Galdeano, C., Weill, R., De Paula, J., and Perdigón, G. D. V. (2018). Oral Administration of Probiotics Increases Paneth Cells and Intestinal Antimicrobial Activity. Front. Microbiol. 9:736. doi: 10.3389/fmicb.2018.00736
Chang, H. Y., Chen, J. H., Chang, J. H., Lin, H. C., Lin, C. Y., and Peng, C. C. (2017). Multiple strains probiotics appear to be the most effective probiotics in the prevention of necrotizing enterocolitis and mortality: an updated meta-analysis. PLoS One 12:e0171579. doi: 10.1371/journal.pone.0171579
Chapman, C. M., Gibson, G. R., and Rowland, I. (2011). Health benefits of probiotics: are mixtures more effective than single strains? Eur. J. Nutr. 50, 1–17. doi: 10.1007/s00394-010-0166-z
Chen, S., Hao, M., and Zhang, L. (2022). Antidiarrheal effect of fermented millet bran on diarrhea induced by Senna leaf in mice. Foods 11:2082. doi: 10.3390/foods11142082
Chen, Y., Lu, S., Ye, Z., Cai, X., Wu, S., Li, P., et al. (2022). New compound probiotic beverage protects against antibiotic-associated diarrhea in mice by modulating the microbiota. Future Microbiol. 17, 943–956. doi: 10.2217/fmb-2021-0240
Chen, Y., Yang, B., Stanton, C., Ross, R. P., Zhao, J., Zhang, H., et al. (2021). Bifidobacterium pseudocatenulatum ameliorates DSS-induced colitis by maintaining intestinal mechanical barrier, blocking Proinflammatory cytokines, inhibiting TLR4/NF-κB signaling, and altering gut microbiota. J. Agric. Food Chem. 69, 1496–1512. doi: 10.1021/acs.jafc.0c06329
Cui, M., Zhou, R., Wang, Y., Zhang, M., Liu, K., and Ma, C. (2020). Beneficial effects of sulfated polysaccharides from the red seaweed Gelidium pacificum Okamura on mice with antibiotic-associated diarrhea. Food Funct. 11, 4625–4637. doi: 10.1039/d0fo00598c
Feng, Y., Huang, Y., Wang, Y., Wang, P., Song, H., and Wang, F. (2019). Antibiotics induced intestinal tight junction barrier dysfunction is associated with microbiota dysbiosis, activated NLRP3 inflammasome and autophagy. PLoS One 14:e0218384. doi: 10.1371/journal.pone.0218384
Fernández-Ciganda, S., Fraga, M., and Zunino, P. (2022). Probiotic lactobacilli administration induces changes in the fecal microbiota of Preweaned dairy calves. Probiotics Antimicrob Proteins 14, 804–815. doi: 10.1007/s12602-021-09834-z
Furuse, M., Hirase, T., Itoh, M., Nagafuchi, A., Yonemura, S., Tsukita, S., et al. (1993). Occludin: a novel integral membrane protein localizing at tight junctions. J. Cell Biol. 123, 1777–1788. doi: 10.1083/jcb.123.6.1777
Goodman, C., Keating, G., Georgousopoulou, E., Hespe, C., and Levett, K. (2021). Probiotics for the prevention of antibiotic-associated diarrhoea: a systematic review and meta-analysis. BMJ Open 11:e043054. doi: 10.1136/bmjopen-2020-043054
Guan, W. J., Yuan, J. J., Li, H. M., Gao, Y. H., Chen, C. L., Huang, Y., et al. (2018). Altered community compositions of Proteobacteria in adults with bronchiectasis. Int. J. Chron. Obstruct. Pulmon. Dis. 13, 2173–2182. doi: 10.2147/copd.S159335
Hempel, S., Newberry, S. J., Maher, A. R., Wang, Z., Miles, J. N., Shanman, R., et al. (2012). Probiotics for the prevention and treatment of antibiotic-associated diarrhea: a systematic review and meta-analysis. JAMA 307, 1959–1969. doi: 10.1001/jama.2012.3507
Högenauer, C., Langner, C., Beubler, E., Lippe, I. T., Schicho, R., Gorkiewicz, G., et al. (2006). Klebsiella oxytoca as a causative organism of antibiotic-associated hemorrhagic colitis. N. Engl. J. Med. 355, 2418–2426. doi: 10.1056/NEJMoa054765
Hu, J. S., Huang, Y. Y., Kuang, J. H., Yu, J. J., Zhou, Q. Y., and Liu, D. M. (2020). Streptococcus thermophiles DMST-H2 promotes recovery in mice with antibiotic-associated diarrhea. Microorganisms 8:1650. doi: 10.3390/microorganisms8111650
Idriss, H. T., and Naismith, J. H. (2000). TNF alpha and the TNF receptor superfamily: structure-function relationship(s). Microsc. Res. Tech. 50, 184–195. doi: 10.1002/1097-0029(20000801)50:3<184::Aid-jemt2>3.0.Co;2-h
Iyer, S. S., and Cheng, G. (2012). Role of interleukin 10 transcriptional regulation in inflammation and autoimmune disease. Crit. Rev. Immunol. 32, 23–63. doi: 10.1615/critrevimmunol.v32.i1.30
Jiang, X., Lin, Z., Zhang, B., Wang, Y., Xu, H., and Mao, Q. (2022). Effect of smilax glabra extract on intestinal barriers in mice with antibiotic-associated diarrhea: an experimental study. Chin. J. Pharmacovig. 19, 862–867. doi: 10.19803/j.1672-8629.2022.08.10
Jiang, Y., Song, J., Xu, Y., Liu, C., Qian, W., Bai, T., et al. (2021). Piezo1 regulates intestinal epithelial function by affecting the tight junction protein claudin-1 via the ROCK pathway. Life Sci. 275:119254. doi: 10.1016/j.lfs.2021.119254
Kumar, N., Arthur, C. P., Ciferri, C., and Matsumoto, M. L. (2020). Structure of the secretory immunoglobulin a core. Science 367, 1008–1014. doi: 10.1126/science.aaz5807
Kuo, W. T., Zuo, L., Odenwald, M. A., Madha, S., Singh, G., Gurniak, C. B., et al. (2021). The tight junction protein ZO-1 is dispensable for barrier function but critical for effective mucosal repair. Gastroenterology 161, 1924–1939. doi: 10.1053/j.gastro.2021.08.047
La Fata, G., Weber, P., and Mohajeri, M. H. (2018). Probiotics and the gut immune system: indirect regulation. Probiotics Antimicrob Proteins 10, 11–21. doi: 10.1007/s12602-017-9322-6
Li, B., Alli, R., Vogel, P., and Geiger, T. L. (2014). IL-10 modulates DSS-induced colitis through a macrophage-ROS-NO axis. Mucosal Immunol. 7, 869–878. doi: 10.1038/mi.2013.103
Li, Y., Chen, M., Ma, Y., Yang, Y., Cheng, Y., Ma, H., et al. (2022). Regulation of viable/inactivated/lysed probiotic lactobacillus plantarum H6 on intestinal microbiota and metabolites in hypercholesterolemic mice. NPJ Sci Food 6:50. doi: 10.1038/s41538-022-00167-x
Li, X., Liu, Y., Guo, X., Ma, Y., Zhang, H., and Liang, H. (2021). Effect of lactobacillus casei on lipid metabolism and intestinal microflora in patients with alcoholic liver injury. Eur. J. Clin. Nutr. 75, 1227–1236. doi: 10.1038/s41430-020-00852-8
Li, L., Wan, G., Han, B., and Zhang, Z. (2018). Echinacoside alleviated LPS-induced cell apoptosis and inflammation in rat intestine epithelial cells by inhibiting the mTOR/STAT3 pathway. Biomed. Pharmacother. 104, 622–628. doi: 10.1016/j.biopha.2018.05.072
Li, X., Wu, Y., Xu, Z., Chen, J., Li, Y., Xing, H., et al. (2020). Effects of Hetiao Jianpi decoction on intestinal injury and repair in rats with antibiotic-associated diarrhea. Med. Sci. Monit. 26:e921745. doi: 10.12659/msm.921745
Li, Y., Xia, S., Jiang, X., Feng, C., Gong, S., Ma, J., et al. (2021). Gut microbiota and diarrhea: an updated review. Front. Cell. Infect. Microbiol. 11:625210. doi: 10.3389/fcimb.2021.625210
Li, Z., Zhu, G., Li, C., Lai, H., Liu, X., and Zhang, L. (2021). Which probiotic is the Most effective for treating acute diarrhea in children? A Bayesian network meta-analysis of randomized controlled trials. Nutrients 13:4319. doi: 10.3390/nu13124319
Liang, H., Song, H., Zhang, X., Song, G., Wang, Y., Ding, X., et al. (2022). Metformin attenuated sepsis-related liver injury by modulating gut microbiota. Emerg. Microb. Infect. 11, 815–828. doi: 10.1080/22221751.2022.2045876
Ling, X., Linglong, P., Weixia, D., and Hong, W. (2016). Protective effects of Bifidobacterium on intestinal barrier function in LPS-induced enterocyte barrier injury of Caco-2 monolayers and in a rat NEC model. PLoS One 11:e0161635. doi: 10.1371/journal.pone.0161635
Ling, Z., Liu, X., Cheng, Y., Luo, Y., Yuan, L., Li, L., et al. (2015). Clostridium butyricum combined with Bifidobacterium infantis probiotic mixture restores fecal microbiota and attenuates systemic inflammation in mice with antibiotic-associated diarrhea. Biomed. Res. Int. 2015:582048. doi: 10.1155/2015/582048
Liu, W., Chen, M., Duo, L., Wang, J., Guo, S., Sun, H., et al. (2020). Characterization of potentially probiotic lactic acid bacteria and bifidobacteria isolated from human colostrum. J. Dairy Sci. 103, 4013–4025. doi: 10.3168/jds.2019-17602
Lopez-Castejon, G., and Brough, D. (2011). Understanding the mechanism of IL-1β secretion. Cytokine Growth Factor Rev. 22, 189–195. doi: 10.1016/j.cytogfr.2011.10.001
Ma, W., Li, W., Yu, S., Bian, H., Wang, Y., Jin, Y., et al. (2023). Immunomodulatory effects of complex probiotics on the immuno-suppressed mice induced by cyclophosphamide. Front. Microbiol. 14:1055197. doi: 10.3389/fmicb.2023.1055197
Ma, Y., Wang, Z., Yang, J., Li, Z., Peng, X., Shan, X., et al. (2023). Alleviating effect of Bifidobacterium animalis subsp. lactis XLTG11 on antibiotic-associated diarrhea induced by clindamycin. Food Sci. 44, 170–178.
Ma, K., Zhang, H., and Baloch, Z. (2016). Pathogenetic and therapeutic applications of tumor necrosis factor-α (TNF-α) in major depressive disorder: a systematic review. Int. J. Mol. Sci. 17:733. doi: 10.3390/ijms17050733
Mekonnen, S. A., Merenstein, D., Fraser, C. M., and Marco, M. L. (2020). Molecular mechanisms of probiotic prevention of antibiotic-associated diarrhea. Curr. Opin. Biotechnol. 61, 226–234. doi: 10.1016/j.copbio.2020.01.005
Meng, X., Zhang, G., Cao, H., Yu, D., Fang, X., de Vos, W. M., et al. (2020). Gut dysbacteriosis and intestinal disease: mechanism and treatment. J. Appl. Microbiol. 129, 787–805. doi: 10.1111/jam.14661
Nishida, A., Inoue, R., Inatomi, O., Bamba, S., Naito, Y., and Andoh, A. (2018). Gut microbiota in the pathogenesis of inflammatory bowel disease. Clin. J. Gastroenterol. 11, 1–10. doi: 10.1007/s12328-017-0813-5
Overeem, K., van Soest, G., and Blankenstein, N. (2008). Antibiotic-associated diarrhoea. Br. J. Gen. Pract. 58, 283.3–283.284. doi: 10.3399/bjgp08X279959
Peng, X., Ed-Dra, A., and Yue, M. (2022). Whole genome sequencing for the risk assessment of probiotic lactic acid bacteria. Crit. Rev. Food Sci. Nutr. 1–19. doi: 10.1080/10408398.2022.2087174
Peng, C., Li, J., Miao, Z., Wang, Y., Wu, S., Wang, Y., et al. (2022). Early life administration of Bifidobacterium bifidum BD-1 alleviates long-term colitis by remodeling the gut microbiota and promoting intestinal barrier development. Front. Microbiol. 13:916824. doi: 10.3389/fmicb.2022.916824
Peterson, L. W., and Artis, D. (2014). Intestinal epithelial cells: regulators of barrier function and immune homeostasis. Nat. Rev. Immunol. 14, 141–153. doi: 10.1038/nri3608
Plaza-Diaz, J., Ruiz-Ojeda, F. J., Gil-Campos, M., and Gil, A. (2019). Mechanisms of action of probiotics. Adv. Nutr. 10, S49–s66. doi: 10.1093/advances/nmy063
Pope, J. L., Bhat, A. A., Sharma, A., Ahmad, R., Krishnan, M., Washington, M. K., et al. (2014). Claudin-1 regulates intestinal epithelial homeostasis through the modulation of notch-signalling. Gut 63, 622–634. doi: 10.1136/gutjnl-2012-304241
Popko, K., Gorska, E., and Demkow, U. (2010). Influence of interleukin-6 and G174C polymorphism in IL-6 gene on obesity and energy balance. Eur. J. Med. Res. 15, 123–127. doi: 10.1186/2047-783x-15-s2-123
Schultz, B. M., Paduro, C. A., Salazar, G. A., Salazar-Echegarai, F. J., Sebastián, V. P., Riedel, C. A., et al. (2017). A potential role of salmonella infection in the onset of inflammatory bowel diseases. Front. Immunol. 8:191. doi: 10.3389/fimmu.2017.00191
Shao, H., Zhang, C., Xiao, N., and Tan, Z. (2020). Gut microbiota characteristics in mice with antibiotic-associated diarrhea. BMC Microbiol. 20:313. doi: 10.1186/s12866-020-01999-x
Shin, N. R., Whon, T. W., and Bae, J. W. (2015). Proteobacteria: microbial signature of dysbiosis in gut microbiota. Trends Biotechnol. 33, 496–503. doi: 10.1016/j.tibtech.2015.06.011
Silverman, M. A., Konnikova, L., and Gerber, J. S. (2017). Impact of antibiotics on necrotizing enterocolitis and antibiotic-associated diarrhea. Gastroenterol. Clin. N. Am. 46, 61–76. doi: 10.1016/j.gtc.2016.09.010
Skrzydło-Radomańska, B., Prozorow-Król, B., Cichoż-Lach, H., Majsiak, E., Bierła, J. B., Kanarek, E., et al. (2021). The effectiveness and safety of multi-strain probiotic preparation in patients with diarrhea-predominant irritable bowel syndrome: a randomized controlled study. Nutrients 13:756. doi: 10.3390/nu13030756
Stavropoulou, E., and Bezirtzoglou, E. (2020). Probiotics in medicine: a long debate. Front. Immunol. 11:2192. doi: 10.3389/fimmu.2020.02192
Tan, H., Zhai, Q., and Chen, W. (2019). Investigations of Bacteroides spp. towards next-generation probiotics. Food Res. Int. 116, 637–644. doi: 10.1016/j.foodres.2018.08.088
Tanaka, T., Narazaki, M., and Kishimoto, T. (2014). IL-6 in inflammation, immunity, and disease. Cold Spring Harb. Perspect. Biol. 6:a016295. doi: 10.1101/cshperspect.a016295
Tian, F., Zhai, Q., Zhao, J., Liu, X., Wang, G., Zhang, H., et al. (2012). Lactobacillus plantarum CCFM8661 alleviates lead toxicity in mice. Biol. Trace Elem. Res. 150, 264–271. doi: 10.1007/s12011-012-9462-1
Turroni, F., Milani, C., Duranti, S., Ferrario, C., Lugli, G. A., Mancabelli, L., et al. (2018). Bifidobacteria and the infant gut: an example of co-evolution and natural selection. Cell. Mol. Life Sci. 75, 103–118. doi: 10.1007/s00018-017-2672-0
Turroni, F., van Sinderen, D., and Ventura, M. (2011). Genomics and ecological overview of the genus Bifidobacterium. Int. J. Food Microbiol. 149, 37–44. doi: 10.1016/j.ijfoodmicro.2010.12.010
van der Sluis, M., de Koning, B. A. E., de Bruijn, A. C. J. M., Velcich, A., Meijerink, J. P., van Goudoever, J. B., et al. (2006). Muc2-deficient mice spontaneously develop colitis, indicating that MUC2 is critical for colonic protection. Gastroenterology 131, 117–129. doi: 10.1053/j.gastro.2006.04.020
Wang, J., Ji, H., Wang, S., Liu, H., Zhang, W., Zhang, D., et al. (2018). Probiotic lactobacillus plantarum promotes intestinal barrier function by strengthening the epithelium and modulating gut microbiota. Front. Microbiol. 9:1953. doi: 10.3389/fmicb.2018.01953
Wang, N., Wang, S., Xu, B., Liu, F., Huo, G., and Li, B. (2021). Alleviation effects of Bifidobacterium animalis subsp. lactis XLTG11 on dextran sulfate sodium-induced colitis in mice. Microorganisms 9:2093. doi: 10.3390/microorganisms9102093
Wang, Y., Xie, J., Wang, N., Li, Y., Sun, X., Zhang, Y., et al. (2013). Lactobacillus casei Zhang modulate cytokine and toll-like receptor expression and beneficially regulate poly I:C-induced immune responses in RAW264.7 macrophages. Microbiol. Immunol. 57, 54–62. doi: 10.1111/j.1348-0421.516.x
Wang, Y., Yan, X., Zhang, W., Liu, Y., Han, D., Teng, K., et al. (2019). Lactobacillus casei Zhang prevents Jejunal epithelial damage to early-weaned piglets induced by Escherichia coli K88 via regulation of intestinal mucosal integrity, tight junction proteins and immune factor expression. J. Microbiol. Biotechnol. 29, 863–876. doi: 10.4014/jmb.1903.03054
Wang, X., Zhang, P., and Zhang, X. (2021). Probiotics regulate gut microbiota: an effective method to improve immunity. Molecules 26:6076. doi: 10.3390/molecules26196076
Xu, H., Hiraishi, K., Kurahara, L. H., Nakano-Narusawa, Y., Li, X., Hu, Y., et al. (2021). Inhibitory effects of breast Milk-derived lactobacillus rhamnosus Probio-M9 on colitis-associated carcinogenesis by restoration of the gut microbiota in a mouse model. Nutrients 13:1143. doi: 10.3390/nu13041143
Xu, B., Liang, S., Zhao, J., Li, X., Guo, J., Xin, B., et al. (2022). Bifidobacterium animalis subsp. lactis XLTG11 improves antibiotic-related diarrhea by alleviating inflammation, enhancing intestinal barrier function and regulating intestinal flora. Food Funct. 13, 6404–6418. doi: 10.1039/d1fo04305f
Xu, H., Wang, S., Jiang, Y., Wu, J., Chen, L., Ding, Y., et al. (2023). Poria cocos polysaccharide ameliorated antibiotic-associated diarrhea in mice via regulating the homeostasis of the gut microbiota and intestinal mucosal barrier. Int. J. Mol. Sci. 24:1423. doi: 10.3390/ijms24021423
Xue, L., He, J., Gao, N., Lu, X., Li, M., Wu, X., et al. (2017). Probiotics may delay the progression of nonalcoholic fatty liver disease by restoring the gut microbiota structure and improving intestinal endotoxemia. Sci. Rep. 7:45176. doi: 10.1038/srep45176
Ya, T., Zhang, Q., Chu, F., Merritt, J., Bilige, M., Sun, T., et al. (2008). Immunological evaluation of lactobacillus casei Zhang: a newly isolated strain from koumiss in Inner Mongolia, China. BMC Immunol. 9:68. doi: 10.1186/1471-2172-9-68
Yang, N., Zhan, Y., Wan, J., Li, Y., Hu, X., and Liu, W. (2022). Effects of Lacidophilin tablets, yogurt, and bifid triple viable capsules on the gut microbiota of mice with antibiotic-associated diarrhea. Can. J. Infect. Dis. Med. Microbiol. 2022:6521793. doi: 10.1155/2022/6521793
Yao, G., Cao, C., Zhang, M., Kwok, L. Y., Zhang, H., and Zhang, W. (2021). Lactobacillus casei Zhang exerts probiotic effects to antibiotic-treated rats. Comput. Struct. Biotechnol. J. 19, 5888–5897. doi: 10.1016/j.csbj.2021.10.026
Yao, D., Dai, W., Dong, M., Dai, C., and Wu, S. (2021). MUC2 and related bacterial factors: therapeutic targets for ulcerative colitis. EBioMedicine 74:103751. doi: 10.1016/j.ebiom.2021.103751
Yu, L., Zhang, L., Duan, H., Zhao, R., Xiao, Y., Guo, M., et al. (2021). The protection of Lactiplantibacillus plantarum CCFM8661 against Benzopyrene-induced toxicity via regulation of the gut microbiota. Front. Immunol. 12:736129. doi: 10.3389/fimmu.2021.736129
Zafar, H., and Saier, M. H. Jr. (2020). Comparative genomics of the transport proteins of ten lactobacillus strains. Genes (Basel) 11:1234. doi: 10.3390/genes11101234
Zawistowska-Rojek, A., and Tyski, S. (2018). Are probiotic really safe for humans? Pol. J. Microbiol. 67, 251–258. doi: 10.21307/pjm-2018-044
Zeng, A., Peng, M., Liu, H., Guo, Z., Xu, J., Wang, S., et al. (2019). Effects of Debaryomyces hansenii treatment on intestinal mucosa microecology in mice with antibiotic-associated diarrhea. PLoS One 14:e0224730. doi: 10.1371/journal.pone.0224730
Zhang, N., Liang, T., Jin, Q., Shen, C., Zhang, Y., and Jing, P. (2019). Chinese yam (Dioscorea opposita Thunb.) alleviates antibiotic-associated diarrhea, modifies intestinal microbiota, and increases the level of short-chain fatty acids in mice. Food. Res. Int. 122, 191–198. doi: 10.1016/j.foodres.2019.04.016
Zhang, W., Zhang, Y., Li, Y., Ma, D., Zhang, H., and Kwok, L. Y. (2022). Lacticaseibacillus rhamnosus Probio-M9-driven mouse mammary tumor-inhibitory effect is accompanied by modulation of host gut microbiota, immunity, and serum metabolome. Nutrients 15:5. doi: 10.3390/nu15010005
Zhang, W., Zhu, B., Xu, J., Liu, Y., Qiu, E., Li, Z., et al. (2018). Bacteroides fragilis protects against antibiotic-associated diarrhea in rats by modulating intestinal defenses. Front. Immunol. 9:1040. doi: 10.3389/fimmu.2018.01040
Zheng, Y., Yu, Z., Zhang, W., and Sun, T. (2021). Lactobacillus rhamnosus Probio-M9 improves the quality of life in stressed adults by gut microbiota. Foods 10:2384. doi: 10.3390/foods10102384
Keywords: complex probiotics, antibiotic-associated diarrhea, immunomodulatory, 16S rRNA gene sequencing, gut microbiota
Citation: Li W, Zhang S, Wang Y, Bian H, Yu S, Huang L and Ma W (2023) Complex probiotics alleviate ampicillin-induced antibiotic-associated diarrhea in mice. Front. Microbiol. 14:1156058. doi: 10.3389/fmicb.2023.1156058
Received: 01 February 2023; Accepted: 29 March 2023;
Published: 14 April 2023.
Edited by:
Min Yue, Zhejiang University, ChinaReviewed by:
Guangzhi Zhang, Institute of Animal Sciences, Chinese Academy of Agricultural Sciences, ChinaCopyright © 2023 Li, Zhang, Wang, Bian, Yu, Huang and Ma. This is an open-access article distributed under the terms of the Creative Commons Attribution License (CC BY). The use, distribution or reproduction in other forums is permitted, provided the original author(s) and the copyright owner(s) are credited and that the original publication in this journal is cited, in accordance with accepted academic practice. No use, distribution or reproduction is permitted which does not comply with these terms.
*Correspondence: Lili Huang, aHVhbmdsaWxpQGhsanVjbS5uZXQ=; Weiwei Ma, MTg1MDQ1MTY5MTFAMTYzLmNvbQ==
†These authors have contributed equally to this work and share first authorship
Disclaimer: All claims expressed in this article are solely those of the authors and do not necessarily represent those of their affiliated organizations, or those of the publisher, the editors and the reviewers. Any product that may be evaluated in this article or claim that may be made by its manufacturer is not guaranteed or endorsed by the publisher.
Research integrity at Frontiers
Learn more about the work of our research integrity team to safeguard the quality of each article we publish.