- 1Instituto de Microbiologia Paulo de Góes, Universidade Federal do Rio de Janeiro, Rio de Janeiro, Brazil
- 2Instituto Oswaldo Cruz, Rio de Janeiro, Brazil
- 3Biological and Environmental Sciences and Engineering (BESE), King Abdullah University of Science and Technology, Thuwal, Saudi Arabia
Paenibacillus antarcticus IPAC21, an endospore-forming and bioemulsifier-producing strain, was isolated from King George Island, Antarctica. As psychrotolerant/psychrophilic bacteria can be considered promising sources for novel products such as bioactive compounds and other industrially relevant substances/compounds, the IPAC21 genome was sequenced using Illumina Hi-seq, and a search for genes related to the production of bioemulsifiers and other metabolic pathways was performed. The IPAC21 strain has a genome of 5,505,124 bp and a G + C content of 40.5%. Genes related to the biosynthesis of exopolysaccharides, such as the gene that encodes the extracellular enzyme levansucrase responsible for the synthesis of levan, the 2,3-butanediol pathway, PTS sugar transporters, cold-shock proteins, and chaperones were found in its genome. IPAC21 cell-free supernatants obtained after cell growth in trypticase soy broth at different temperatures were evaluated for bioemulsifier production by the emulsification index (EI) using hexadecane, kerosene and diesel. EI values higher than 50% were obtained using the three oil derivatives when IPAC21 was grown at 28°C. The bioemulsifier produced by P. antarcticus IPAC21 was stable at different NaCl concentrations, low temperatures and pH values, suggesting its potential use in lower and moderate temperature processes in the petroleum industry.
Introduction
The genus Paenibacillus was created by Ash et al. (1993) to accommodate the former ‘group 3’ of the genus Bacillus. Currently, the genus comprises more than 280 validated species1 that harbor endospore-forming, low G + C gram-positive bacterial strains (Lal and Tabacchioni, 2009; Grady et al., 2016; Jeong et al., 2019). Strains belonging to the genus Paenibacillus have already been isolated worldwide from different types of soils and plants, deserts, caves, cold regions, and aquatic environments, many of which are of great importance to humans, animals, plants, the environment and/or as biotechnological agents in industrial processes (Lal and Tabacchioni, 2009; Grady et al., 2016; Patowary and Deka, 2020).
Considering the petroleum industry, Paenibacillus strains are efficient in bioremediating different environments contaminated with pollutants derived from extracting, refining, and transporting petroleum and coal tar (Grady et al., 2016). Some examples are P. ehimensis BS1, which is able to degrade heavy crude oil (Shibulal et al., 2017), Paenibacillus sp. strains D2, D9 and D10 are considered diesel fuel degraders (Ganesh and Lin, 2009), and P. naphthalenovorans PR-N1 (Daane et al., 2002) and Paenibacillus sp. PHE-3 (Zhu et al., 2016), both of which are able to degrade polycyclic aromatic hydrocarbons (naphthalene and phenanthrene, respectively). Moreover, biosurfactants (or other bacterial byproducts such as bioemulsifiers, acids and solvents) produced by Paenibacillus sp. can be used for the bioremediation of oil (and derivatives)-contaminated soils and marine environments. The biosurfactant produced by Paenibacillus sp. D9 was used for the bioremediation of diesel and motor oil (Jimoh and Lin, 2020), and that from P. dendritiformis CN5 was used for polycyclic aromatic hydrocarbon (PAH) and motor oil sludge removal from contaminated soil and sand media (Bezza and Chirwa, 2015). Paenibacillus sp. #510 isolated from crude oil was able to produce a bioemulsifier with high potential to stabilize emulsions with different hydrocarbons (Gudina et al., 2015). In addition, the potential of P. ehimensis BS1 in microbial enhanced oil recovery (MEOR) technology has been demonstrated by the biotransformation of heavy to lighter crude oil under aerobic and reservoir conditions (Shibulal et al., 2017).
Paenibacillus antarcticus has been described as a psychrotolerant organism isolated from Antarctic sediment (Montes et al., 2004). The type strain of the species – 20CMT – produces oxidase, catalase and urease, and it hydrolyzes aesculin, starch, and Tween 80. Psychrotolerant/psychrophilic bacteria can be considered promising sources for novel products such as bioactive compounds and other industrially relevant substances/compounds (Dhakar and Pandey, 2020). Moreover, cold-active enzymes found in psychrotolerant/psychrophilic bacteria are very interesting for different processes in industry (including the petroleum industry), pharmaceuticals, medicine, and food (Yadav et al., 2019).
Vollú et al. (2014) isolated and characterized different endospore-forming and cold-adapted bacteria from soil samples of King George Island, which is part of the South Shetlands archipelago in Maritime Antarctica. The ability to produce extracellular enzymes and antimicrobial substances and to emulsify n-hexadecane was determined in all isolates in an attempt to contribute to a potential source of cold active bioproducts for industrial use. One strain identified as P. antarcticus and denoted IPAC21 was able to efficiently emulsify n-hexadecane in laboratory conditions. However, no further tests have been performed to determine the surfactant effect of reducing surface tension. Although biosurfactants and/or bioemulsifiers have been extensively used in the remediation of oil-contaminated water and soil (Silva et al., 2014; Maia et al., 2019), very little is known concerning their biotechnological potential in this Paenibacillus species.
Therefore, we report the genomic characterization of the psychrotolerant strain IPAC21, which was isolated from soil collected in Ipanema, King George Island, Antarctica, highlighting important metabolic pathways such as those for its adaptation to environmental stress conditions and for bioemulsifier production. Moreover, the physical and chemical conditions for its production and its ability to emulsify oils derived from the petroleum industry were evaluated. Our results provide important information about the potential use of the bioemulsifier produced by P. antarcticus IPAC21 for future biotechnological applications.
Materials and methods
Bacterial strain
The bacterial strain IPAC21 was previously isolated from soil samples from Ipanema (UTM coordinates – latitude/longitude = E:426.570/N:3.116.513), King George Island, Antarctica (Vollú et al., 2014). The strain IPAC21 was stored in tryptic soy broth (TSB) containing 20% glycerol at −80°C. To evaluate the growth of IPAC21 at different temperatures, the strain was grown in TSB at 5°C, 15°C and 28°C for up to 96 h under agitation at 130 rpm.
DNA extraction, genome sequencing and assembly
For DNA extraction, strain IPAC21 was initially cultivated in 5 ml of TSB at 28°C under agitation at 130 rpm for 48 h. Then, the culture was centrifuged at 12,000 g, the supernatant was discarded, and the pellet was recovered. DNA from the IPAC21 strain was extracted and purified according to Seldin et al. (1998) and Seldin and Dubnau (1985), respectively. Subsequently, the DNA was quantified spectrophotometrically using a Qubit™ fluorimeter (Thermo Fisher Scientific, MA, USA).
The genome of strain IPAC21 was sequenced on the Illumina HiSeq 2,500 platform as recommended by the manufacturer. Approximately 5 μg/μg DNA was used for the construction of paired-end sequencing libraries (2 × 150 bp) with a 450 bp insert size. The quality analysis of the final libraries was performed using a 2100 bioanalyzer (Agilent Technologies, CA, USA). Genomic contigs were de novo assembled using SPAdes software, version 3.15.5 (Prjibelski et al., 2020).
Genome annotation
The automatic annotations of the IPAC21 genome were performed using the online server RAST (Aziz et al., 2008) and NCBI Prokaryotic Genome Annotation Pipeline (PGAP, version 2022-04-14.build6021). The KEGG2 and Metacyc3 databases were used for manual annotation and construction of metabolic pathways. The pathways according to the genome annotation of the IPAC21 strain were created with BioRender.com.
Phylogenetic analyses
For the phylogenetic analyses, 16S rRNA-encoding gene sequences of closely related species to Paenibacillus antarcticus were retrieved from the GenBank database. Sequence alignments were performed using the ClustalW method in MEGA-X software. The sequences were aligned through the ClustalW method to analyze the similarity between those sequences. The phylogenetic tree was constructed using the maximum likelihood method and the Jukes-Cantor model (bootstrap = 1,000) (Kumar et al., 2018).
Average nucleotide identity (ANI) and digital DNA–DNA hybridization (dDDH)
The IPAC21 genome was compared with those of ten closely related Paenibacillus strains (listed in Table 1) using the JSpeciesWS database4 with the alignment algorithm blastn (ANIb) (Richter et al., 2016). The draft genomes were downloaded from NCBI5 and JGI6.
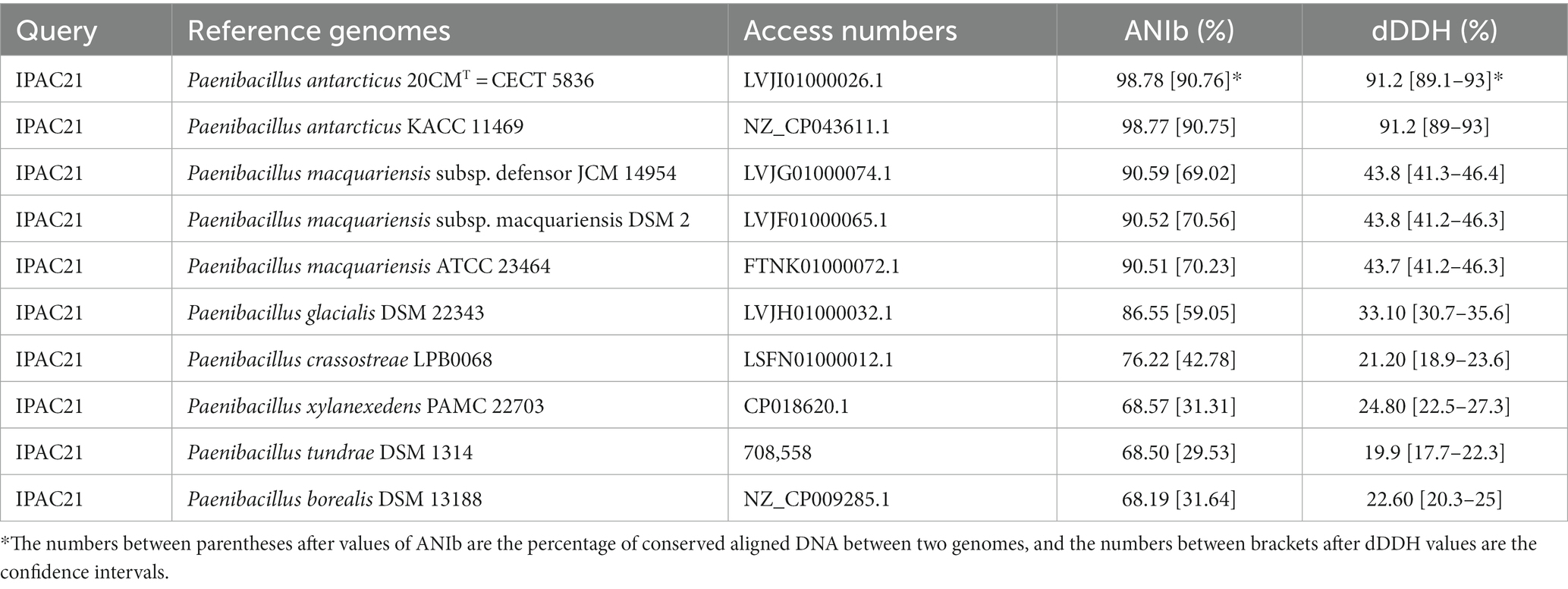
Table 1. Average nucleotide identity (ANI) and digital DNA–DNA hybridization (DDH) values between IPAC21 and closely related species of the genus Paenibacillus.
DNA digital hybridization (dDDH) was performed using the Genome-to-Genome Distance Calculator—GGDC 2.1 (Meier-Kolthoff et al., 2013) provided by Leibniz on the DSMZ Institute website7 with the recommended parameters and/or default settings.
Comparative genomics
A comparative genome map was plotted through a BLASTN-based ring generated by BLAST ring image generator (BRIG) version 0.95 (Alikhan et al., 2011) to compare the draft genomes of six closely related Paenibacillus species with that of P. antarcticus strain IPAC21 used as a reference. A manual annotation of proteins was also performed using BLASTp and RAST, and the KEGG database8 was used to understand the possible metabolic pathways in which some proteins are embedded.
Bioemulsifier production in vitro
Growth and production of the Paenibacillus antarcticus IPAC21 bioemulsifier in different culture media
Different culture media were compared for bioemulsifier production by strain IPAC21: TSB, LB (Luria Bertani), GB (glucose 10 g; peptone 10 g; meat extract 2 g; yeast extract 1 g; NaCl 5 g; H2O q.s. 1000 ml, pH 7.2), MM (NaCl 10 g; Na2HPO4 9.44 g; (NH4)2SO4 2 g; glucose 10 g; MgSO4.7H2O 0.2 g; H2O q.s. 1000 ml), MSS (NaCl 10 g; sucrose 10 g; Na2HPO4 5 g; NH4NO3 2 g; KH2PO4 2 g; MgSO4.7H2O 0.2 g; H2O q.s. 1000 ml). Flasks containing 50 ml of each medium were inoculated with approximately 5 × 108 cells (OD600 = 0.6). The flasks were incubated at 28°C in a rotatory shaker (130 rpm) for 48 h, and 1 ml was centrifuged (13,000× g, 15 min). The cell-free supernatants were used for E24 determination.
Determination of the emulsification index (E24)
The emulsification index (E24) was determined according to Iqbal et al. (1995) by the addition of 1 ml of n-hexadecane (or 1 ml diesel or 1 ml kerosene) to the same volume of cell-free supernatant in test tubes. The tubes were vortexed at high speed for 3 min. The stability of the emulsion was determined after 24 h at room temperature, and the emulsification index (E24) was calculated as the percentage of the height of the emulsified layer (mm) divided by the total height of the liquid column (mm). Tests were performed in triplicate. Statistical analyses were performed using PAST v4.02 software (Hammer et al., 2001) using one-way analysis of variance (ANOVA) followed by Tukey’s test.
Drop collapse test
A volume of 10 μl from cell-free culture broth supernatant (strain IPAC21 grown at 28°C for 48 h) was dropped onto a crude oil-coated glass slide. Whenever the interfacial tension between the liquid and the hydrophobic surface was reduced, the drops spread or collapsed (Jain et al., 1991). The same volumes of sterile TSB and 10% SDS were used as negative and positive controls, respectively.
Oil displacement test
A 90 × 15-mm Petri dish was filled with 40 ml of distilled water, and 20 μl of crude oil was added to form an apolar thin layer of hydrocarbons on the surface. Then, 10 μl of the cell-free culture supernatant (strain IPAC21 grown in TSB at 28°C for 48 h) was placed on the oil layer, and a positive result was considered when a clear halo was observed (Morikawa et al., 2000). The same volumes of sterile TSB and 10% SDS were used as negative and positive controls, respectively.
Surface-activity determination
The cell-free culture supernatant of IPAC21 grown in TSB at 28°C for 48 h (1 ml) was analyzed on a Kruss DSA100 goniometer (Model: DE 3210) to determine the surface tension (ST) by the drop shape method, as described by Song and Springer (1996). To increase the accuracy of the surface tension measurements, an average of triplicates was conducted. All measurements were performed at room temperature.
Determination of optimal physiological parameters for bioemulsifier production
Emulsification was analyzed during P. antarcticus IPAC21 growth in TSB (200 ml) at 28°C under agitation (130 rpm) for 96 h. Aliquots (5 ml) were collected after 24, 48, 72, and 96 h of cultivation to construct growth, absorbance (OD600), and emulsification curves. To determine the number of vegetative cells, 1 ml aliquots were serially diluted (up to 10−5), and each dilution was plated (100 μl) in TSB with the addition of 1.5% agar (TSA). The plates were incubated at 28°C for 48 h, and the colony forming units (CFU/ml) were calculated. The absorbance curve at OD600 was determined using 1 ml aliquots in a UV/VIS spectrophotometer (Mettler Toledo). The E24 assessments were determined following the same conditions previously described. All experiments were performed in triplicate, and the arithmetic means and standard errors were determined. Negative controls were performed using only TSB.
Stability of the bioemulsifier produced by IPAC21 under different salinity, pH and temperature conditions
The effects of different chemical and physical parameters on the bioemulsifier produced by the IPAC21 strain were evaluated. Before E24 determination, the cell-free supernatants containing the bioemulsifier were submitted to different salinity, pH and temperature conditions. The salinity levels ranged from 3 to 12%, whereas the pH values varied from 2 to 12 (pH adjusted with either 6 N HCl or 2 N NaOH). The supernatant was maintained at −20°C, 5°C, 15°C for 15 min, 2 h and 24 h, 100°C for 20 min, and it was also autoclaved at 121°C for 15 min. The IPAC21 growth conditions as well as the E24 experiments followed the same conditions described above. Statistical analyses were performed using PAST v4.02 software as described previously.
Results and discussion
Phylogenetic analysis of the 16S rRNA encoding gene
The results of BLAST sequence analyses of the 16S rRNA-encoding gene (1,510 bp) indicated that the strain, previously isolated from Antarctic soil and named IPAC21 (Vollú et al., 2014), is related to members of the genus Paenibacillus (Figure 1). Its closest relatives were P. antarcticus 20CMT and P. antarcticus KACC 11469, with 99.93 and 99.85% gene sequence similarities, respectively.
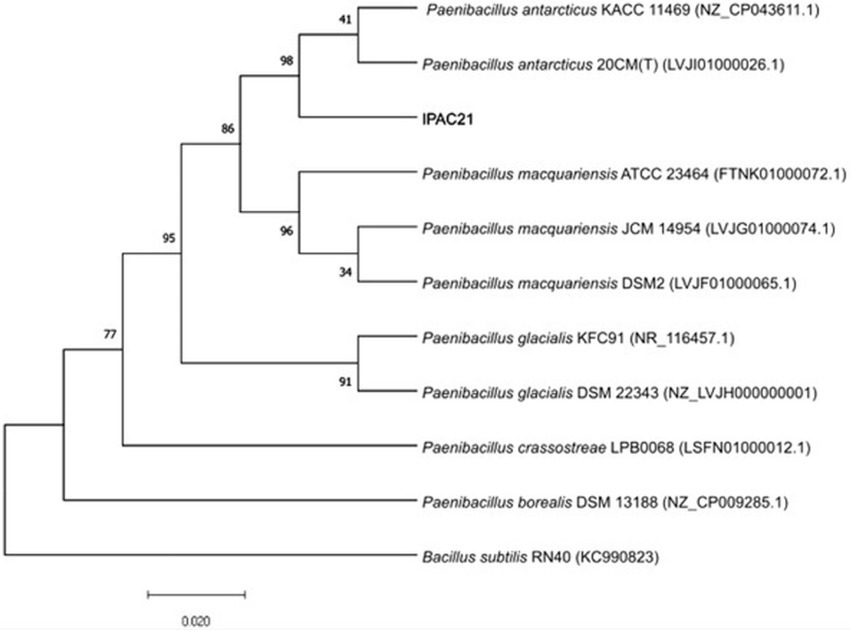
Figure 1. Alignment of the 16S rRNA-encoding gene of Paenibacillus antarcticus IPAC21 and related species. The phylogenetic tree was constructed using the maximum likelihood method and the Jukes–Cantor model. The GenBank accession number of each sequence is shown in parentheses. Bootstrap values are expressed as percentages of 1,000 replications and are shown at branch points. Bacillus subtilis RNA40 was used as an outgroup.
Genome annotation
The draft genome sequence of strain IPAC21 was determined in this study, and the whole genome shotgun project has been deposited at DDBJ/ENA/GenBank under the accession number BioProject PRJNA913778, BioSample ID SAMN32307255.
The genome of strain IPAC21 revealed 5,505,124 bp with a G + C content of 40.5%, and a total of 5,240 coding DNA sequences (CDSs) were predicted. The identified CDSs were classified into subsystems, such as amino acids and derivates (260 CDSs), carbohydrates (230 CDSs), protein metabolism (167 CDSs), cofactors/vitamins/pigments (137 CDSs), stress response (40 CDSs), secondary metabolism (4 CDSs) and metabolism of aromatic compounds (3 CDSs) (Figure 2).
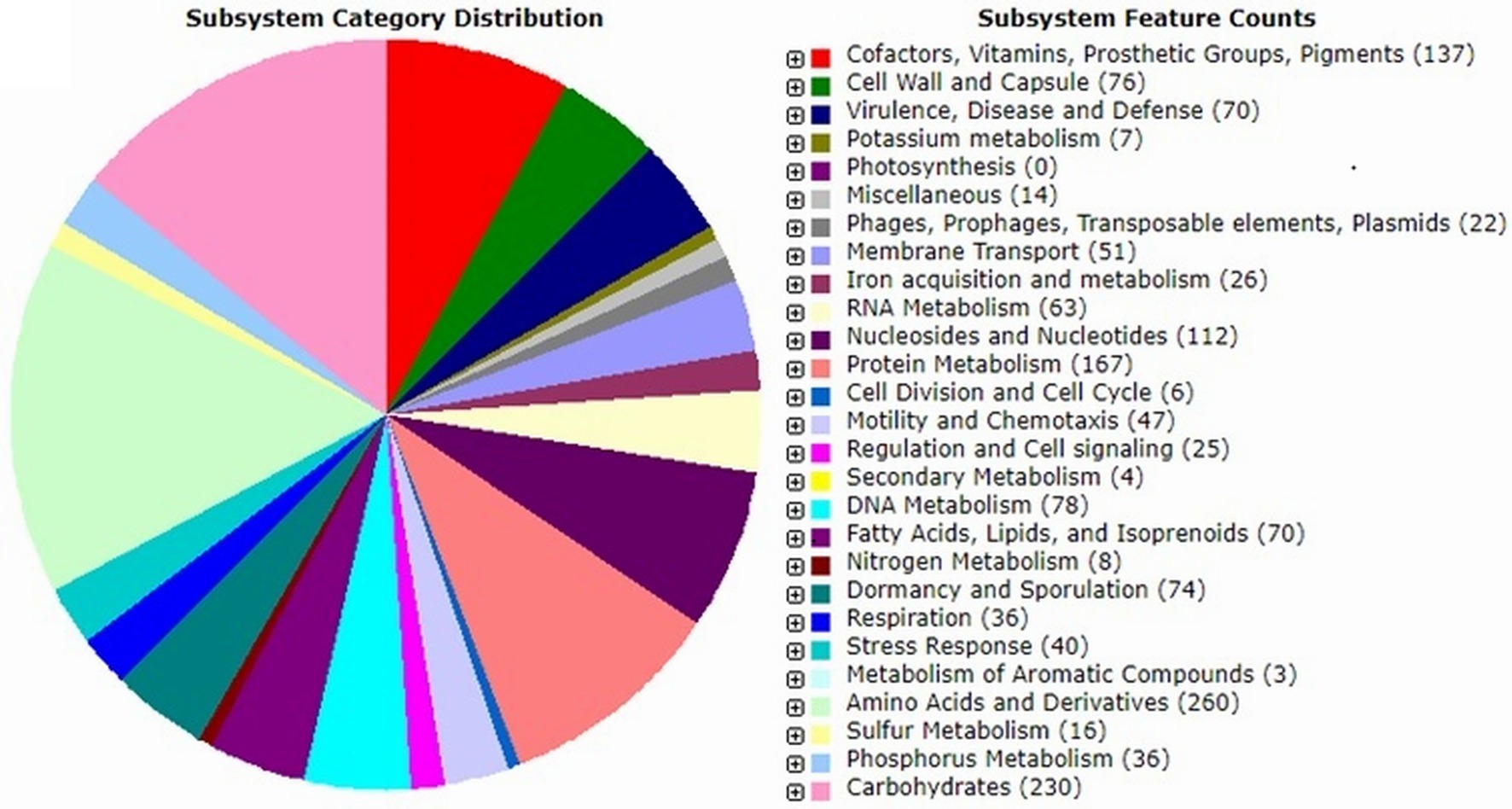
Figure 2. Paenibacillus antarcticus strain IPAC21 subsystem features based on the RAST annotation server.
To explain the taxonomic relationship between the closely related Paenibacillus species and IPAC21 strain, the average nucleotide identity (ANI) and digital DNA–DNA hybridization (dDDH) values were determined between strain IPAC21 and the other ten type genomes of the members of the genus Paenibacillus (Table 1). The ANI values varied between 68.19 and 98.78%, where the accepted limit for species delimitation can be considered 95–96% (Richter and Rosselló-Móra, 2009). In addition, the results of DDH in silico were mostly below 70%, which is the cutoff value for species delimitation (Goris et al., 2007), with the exception of the value observed between the IPAC21 strain and either P. antarcticus 20CMT or P. antarcticus KACC 11469, which was 91.2% (Table 1). Therefore, both the ANI and the DDH results suggest that the IPAC21 strain can be considered to belong to the species P. antarcticus, as previously suggested by Vollú et al. (2014).
Genome features
2,3-Butanediol fermentation pathway
The analysis of the P. antarcticus IPAC21 genome revealed the presence of the butanediol fermentation pathway. 2,3-Butanediol (2,3-BDO) is important as a liquid fuel additive, a softening and moistening agent, a solvent, a synthetic rubber precursor, an antifreeze
agent in different industries, including the petroleum industry (Celinska and Grajek, 2009; Dias et al., 2018). The asl gene encoding R-acetolactate synthase (EC 2.2.1.6), the budA gene encoding R-acetolactate decarboxylase (EC 4.1.1.5), and the budB gene encoding 2,3-butanediol dehydrogenase, R-alcohol forming, (R)- and (S)-acetoin-specific (EC 1.1.1.4) were identified in the IPAC21 genome. The presence of genes involved in butanediol fermentation suggests that IPAC21 is a facultative anaerobic strain similar to the strain P. antarcticus 20CMT described by Montes et al. (2004). The asl and butAB genes have previously been demonstrated in the genome of P. brasilensis PB24 (Dias et al., 2018). However, while genes related to three butanediol isomers were detected in P. brasilensis PB24, only one isomer was observed in the IPAC21 and P. polymyxa PM 3605 genomes (Tinôco et al., 2021; this study).
Adaptations to environmental stress conditions
Microorganisms in cold habitats need to face several adaptive challenges, such as ice formation that damages cell structures, low nutrient availability, high salinity, high UV irradiation, and oxidative stress (Margesin and Collins, 2019). To better understand the survival strategies of P. antarcticus IPAC21, genes associated with adaptation to environmental stress conditions, such as cold response, membrane fluidity mechanisms, DNA repair, oxidative and osmotic stress, chaperones and transport of compatible solutes, were highlighted in its genome.
Three cold shock-domain family proteins (Csps) were detected in the IPAC21 genome. Csps are a family of highly conserved small proteins that bind to single-stranded nucleic acids (Horn et al., 2007). These proteins are involved in metabolic processes of the stress response within the cell, such DNA replication, transcription, and translation (Casanueva et al., 2010; Chaudhary et al., 2022). The CspA family constitutes the main response to cold and is exclusive to psychrophilic bacteria (Leeson et al., 2000). CspA together with CspB acts as an RNA chaperone, destabilizing mRNA and improving translation efficiency at low temperatures (Aliyu et al., 2016; Garcia-Lopez et al., 2021). Other heat stress responses found in the IPAC21 genome are the DNAj and DNAk chaperones.
The repair system found in the IPAC21 genome involves genes that encode the SOS regulon proteins, consisting of three genes that encode the LexA repressor. These genes are a diverse family of bacterial transcription factors that repress genes in the cellular SOS response to DNA damage (Sánchez-Osuna et al., 2021). Furthermore, three genes involved in excision repair for UvrD were found. UvrD is a DNA helicase that participates in nucleotide excision repair and processes associated with replication (Newton et al., 2012).
DEAD-box RNA helicase and recAFON recombinases were also found in the IPAC21 genome. The recA gene product is a multifunctional enzyme that plays a role in homologous recombination, DNA repair and induction of the SOS response (Selbitschka et al., 1991). Microorganisms that are exposed to cold form unfavorable secondary mRNA structures, resulting in inhibition of translation. DEAD box RNA helicase is capable of reversing the secondary structure of the mRNA so that Csps can bind and prevent refolding before translation can take place (Phadtare and Severinov, 2010).
Low temperatures reduce membrane fluidity and permeability, and microorganisms respond by producing less saturated fatty acids to improve membrane fluidity (Chattopadhyay, 2006). In the genome of IPAC21, the enzyme fatty acid desaturase (EC 1.14.19.-) can be related to membrane fluidity that reduces fatty acid saturation and is upregulated at low temperatures (Rodrigues and Tiedje, 2008).
Genes related to oxidative stress were also identified in the IPAC21 strain, including four genes encoding catalases, seven coding for peroxidases, three for hydroperoxides, two for superoxide dismutase, three for thioredoxins, and ten for thioredoxin reductase. In cold environments, the solubility of oxygen increases, generating reactive oxygen species (ROS), resulting in DNA damage (Russo et al., 2010). Some mechanisms of adaptation to oxidative stress have already been described, such as enzymatic mechanisms that involve the use of enzymes superoxide dismutase (SOD), catalase (CAT) and/or glutathione peroxidase (GPx) (Barria et al., 2013). For example, the enzyme superoxide dismutase converts oxygen into hydrogen peroxide, which is less reactive and is an important antioxidant defense in cells exposed to oxygen. Similar to IPAC21, other bacterial strains found in Antarctica - Serratia sp. I1P (Monsalves et al., 2020), Nesterenkonia sp. AN (Aliyu et al., 2016) and Bacillus weihenstephanensis (den Besten et al., 2013) show this mechanism against ROS.
Two genes encoding proteins involved in adaptation to osmotic stress are also present in the IPAC21 genome, opuAA and opuAB, which are related to the ABC transport system of glycine/betaine and proline. Compatible solutes are compounds that protect and stabilize cellular components exposed to stress conditions without significantly interfering with their functions (Angelidis and Smith, 2003). Osmolyte transport proteins act as osmoprotectants and are involved in protection in environments with high osmolarity.
All the genetic features observed in the P. antarcticus IPAC21 genome suggest that this strain has developed survival strategies for its adaptation to environmental stress conditions found in Antarctic soils.
Bioemulsifier production in silico
The analysis of the IPAC21 genome revealed the presence of the gene that encodes the extracellular enzyme levansucrase (EC 2.4.1.10) responsible for the synthesis of levan (Figure 3). Levan is a naturally occurring fructan, the homopolymer of fructose, found in many plants and microbial products, and it has many applications in different industrial fields, such as foods and pharmaceuticals (Mummaleti et al., 2022). Its main chain is composed of repeating five-member fructofuranosyl rings connected by β-(2 → 6) links. The main chain is branched through the β-(2 → 1) linkage of the fructofuranosyl rings (Mendonça et al., 2021). The production of levan starts in the extracellular medium through the conversion of sucrose to fructose/glucose by the enzyme SacB, which synthesizes fructose polymers through a transfructosylation reaction using sucrose as a donor fructose/glucose (Xu et al., 2021). The production of levan has already been described in other Paenibacillus species, such as P. polymyxa DSM 365 (Okonkwo et al., 2020), Paenibacillus sp. #210 (Mendonça et al., 2021) and P. bovis BD3526 (Xu et al., 2016).
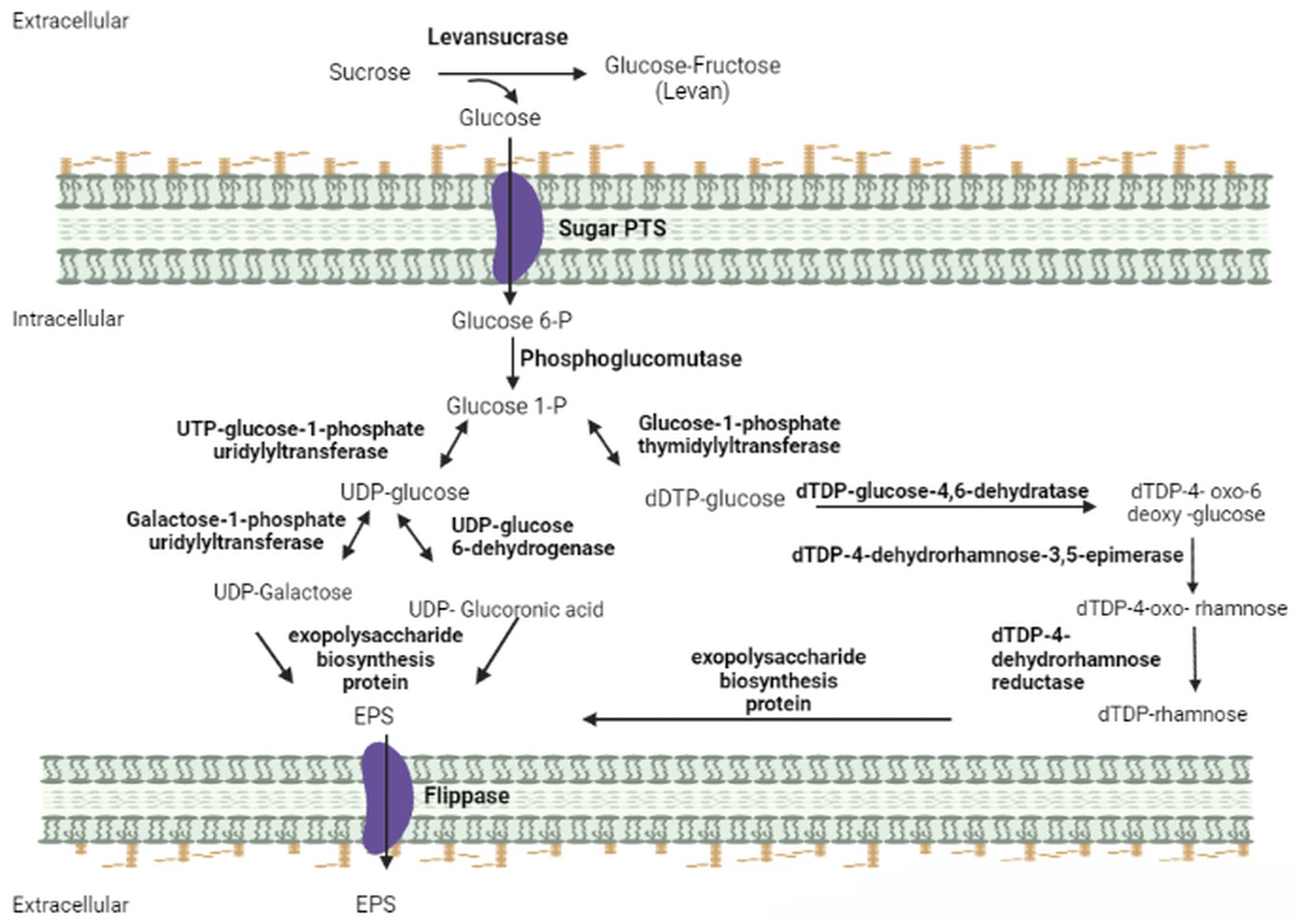
Figure 3. Representation of the metabolic pathway of EPS production found in the IPAC21 genome. The diagram demonstrates the enzymes found using KEGG and Metacyclic annotation programs. Levansucrase (EC 2.4.1.10); sugar PTS (EC 2.7.1.-); phosphoglucomutase (EC 5.4.2.2); UTP-glucose-1-phosphate uridylyltransferase (EC 2.7.7.9); glucose-1-phosphate thymidylyltransferase (EC 2.7.7.24); galactose-1-phosphate uridylyltransferase (EC 2.7.7.12); UDP-glucose-6-dehydrogenase (EC 1.1.1.22); dTDP-glucose-4,6-dehydratase (EC 4.2.1.46); dTDP-4-dehydrorhamnose-3,5-epimerase (EC 5.1.3.13); dTDP-4-dehydrorhamnose reductase (EC 5.1.3.- 1.1.1.-); exopolysaccharide biosynthesis protein; flippase (EC 7.6.2.1).
The sacB gene encoding levansucrase was found in the IPAC21 genome with 97% similarity with that of P. antarcticus 20CMT. The genes coding for phosphoglucomutase (EC 5.4.2.2), responsible for the first reaction of the pathway, uridyltransferase (EC 2.7.7.23), UDP-glucose 6-dehydrogenase (EC 1.1.1.22), glucose-1-phosphate thymidyltransferase (EC 2.7.7.24), dTDP-glucose 4,6-dehydratase (EC 4.2.1.46), and three genes for exopolysaccharide biosynthesis proteins (EC 2.4.1.-) were also observed (Figure 3).
In addition, transmembrane transporters responsible for the acquisition of sugar compounds were detected in the genome of the IPAC21 strain. The presence of 483 transporters, of which 366 showed similarities with transporters ABC (ATP-binding system cassette) and 11 PTS (phosphoenolpyruvate-dependent sugar phosphotransferase system), was observed. Both transport systems act in the transport of several types of sugars, such as D-glucose (EC 2.7.1.-), D-fructose (EC 2.7.1.-), D-galactose (EC 7.5.2.11), maltose (EC 7.5.2.1), lactose (EC 7.5.2.2) and arabinose (EC 7.5.2.7). Furthermore, twenty-three MFS-type transporters, seven drug/metabolite transporters, and four HrtAB transporters were also identified in the IPAC21 genome.
Comparative genomics
The similarity between the DNA regions involved in bioemulsifier production between IPAC21 and related species is highlighted in the comparative genome map (Figure 4). The similarity between the sacB gene that produces a levansucrase enzyme in the genome of IPAC21, of P. antarcticus 20CMT, and P. antarcticus KACC 11469 presents a value of 87.7 and 87.5% identity, respectively. Paenibacillus species such as P. glacialis DSM 22343, P. macquariensis ATCC 23464, P. macquariensis DSM 2, and P. macquariensis JCM 14954 showed an identity of less than 55% when compared to IPAC21.
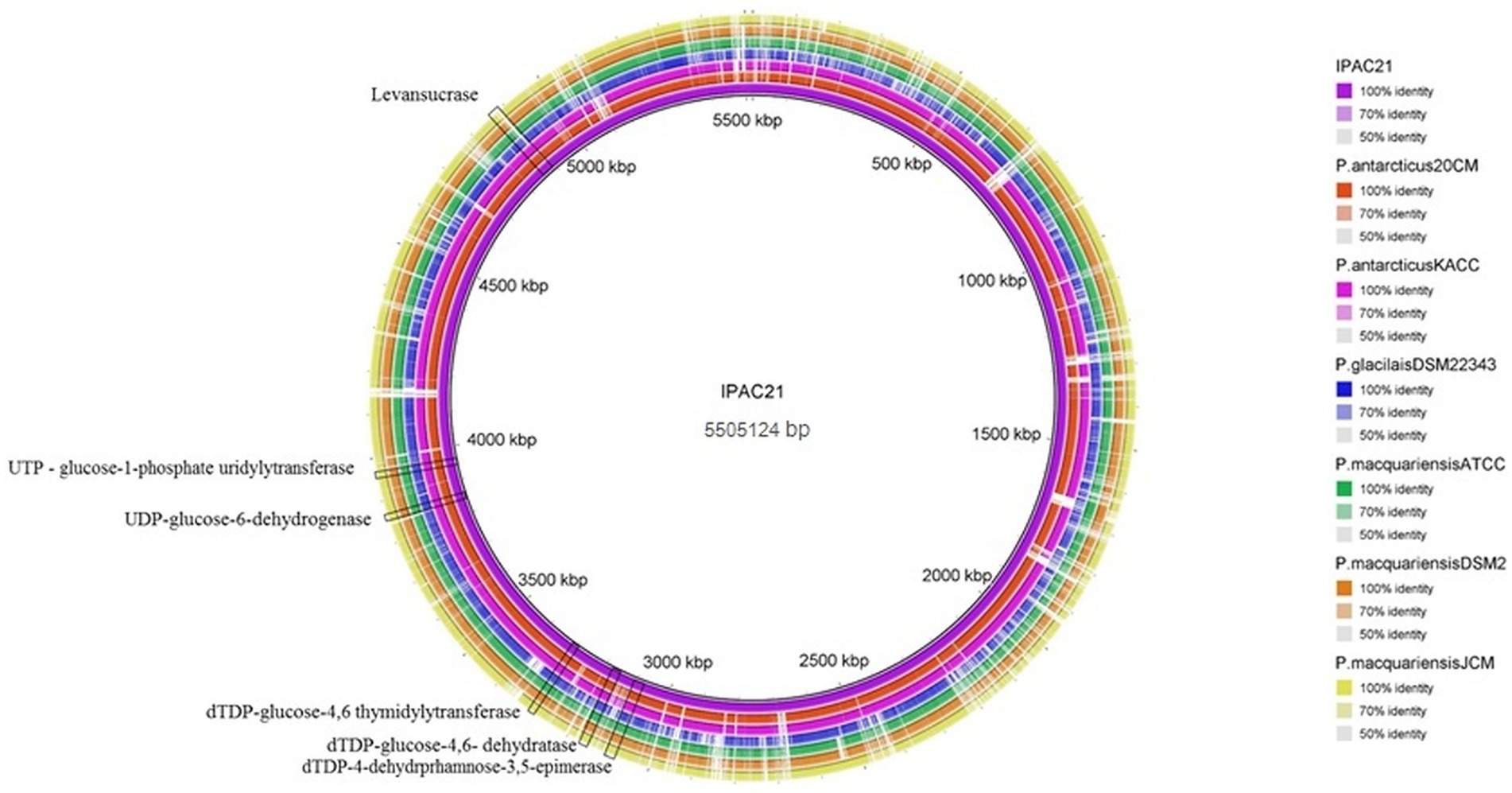
Figure 4. Circular diagram illustrating the nucleotide similarity between P. antarcticus IPAC21 (in purple, inside the circle) and other Paenibacillus genomes represented by concentric rings.
Bioemulsifier production in vitro
Growth and production of the Paenibacillus antarcticus IPAC21 bioemulsifier in different culture media
Strain IPAC21 was able to grow in four media tested (TSB, LB, GB and MM). The highest number of cells and E24 were obtained in TSB. The emulsification activity with n-hexadecane was directly proportional to the growth of P. antarcticus IPAC21 and reached the highest value (63.7% ±1.7) at the end of the log phase (48 h), corresponding to approximately 1.5 × 109 CFU/ml and an absorbance of 1.7.
The cell-free supernatant obtained after IPAC21 growth in TSB was submitted to drop collapse, oil displacement tests and superficial tension tests. Negative results were obtained in all tests. No capacity to reduce surface tension (68.69 mN/m) was observed. These results are expected for bioemulsifiers, which are molecules that emulsify two immiscible liquids, such as hydrocarbons or other types of hydrophobic substrates, even at low concentrations and are less effective in reducing surface tension (Uzoigwe et al., 2015). Bioemulsifiers have already been described in other mesophilic Paenibacillus species, such as P. polymyxa (Gudina et al., 2015), P. dendritiformis CN5 (Bezza and Chirwa, 2017), and Paenibacillus sp. 210 (Mendonça et al., 2021). In cold environments, such as Antarctica, other bacterial species are also producers of bioemulsifiers and/or biosurfactants and are considered reservoirs of new biotechnological molecules (Perfumo et al., 2018; Schultz and Rosado, 2020).
Emulsification of hydrocarbons by Paenibacillus antarcticus IPAC21
The bioemulsifier produced by P. antarcticus IPAC21 was evaluated for its potential application in the oil industry. E24 experiments were conducted with kerosene, hexadecane and diesel at three different temperatures (5°C, 15°C, and 28°C). IPAC21 showed emulsification in the three types of oils and at the three temperatures tested, with the highest values at 28°C and the lowest at 5°C (Figure 5). The highest emulsification value was for n-hexadecane at 28°C (E24 = 56.2% ±1.5), followed by the temperature of 15°C (E24 = 55.2% ±1.6), and at 5°C (E24 = 47.5% ±5.6). The emulsions maintained their stability for at least 30 days. No significant differences (p > 0.05) were observed in the E24 values using kerosene at the three temperatures tested. However, using hexadecane and diesel, significant differences (p < 0.05) were observed at a temperature of 5°C when compared to 15°C and 28°C (Figure 5).
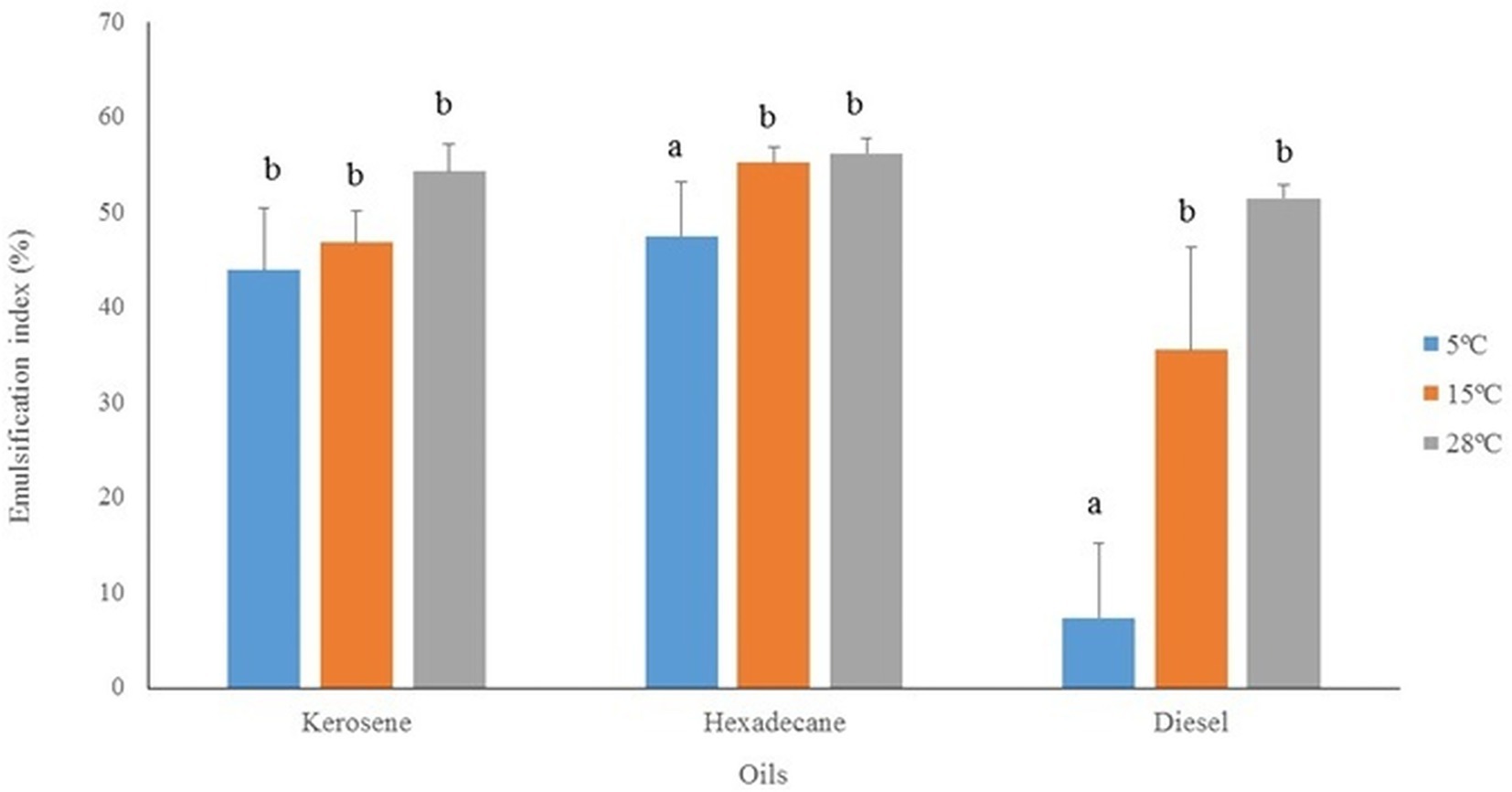
Figure 5. Emulsifying indexes (E24) of P. antarcticus IPAC21 with different oils (kerosene, n-hexadecane, diesel) after IPAC growth in TSB at 5°C, 15°C, and 28°C. Different letters indicate statistically significant differences (Tukey, p < 0.05).
Stability of the bioemulsifier produced by IPAC21 under different salinity, pH and temperature conditions
The effect of salinity, pH, and temperature was evaluated from cell-free supernatant containing the bioemulsifier produced by IPAC21 in TSB at 28°C for 48 h, as shown in Figure 6. The E24 values for the addition of NaCl ranged from 45.6 ± 2.2 to 53.8 ± 1.2%, with the highest value for the salt concentration in the supernatant being 6%, whereas the lowest value for the salt concentration was 12%. Regarding the pH variation, the E24 values were very close, with the highest value of E24 = 59.1 ± 0.9% observed at pH 4 and the lowest value of E24 = 57.9 ± 0.5% at pH 10. At low temperatures, the lowest E24 value (55.6 ± 2.2%) was observed at 5°C for 15 min, and the highest value (60.9 ± 1.3%) was observed at 15°C for 2 h. No statistically significant differences in the abovementioned stability tests were observed (Tukey’s test, p > 0.05) (Figure 6). Regarding the heat treatment, the values of the emulsification index were negligible: E24 = 6.8 ± 3.4% for the heat treatment at 100°C for 20 min and E24 = 8 ± 3.5% for the heat treatment by autoclaving.
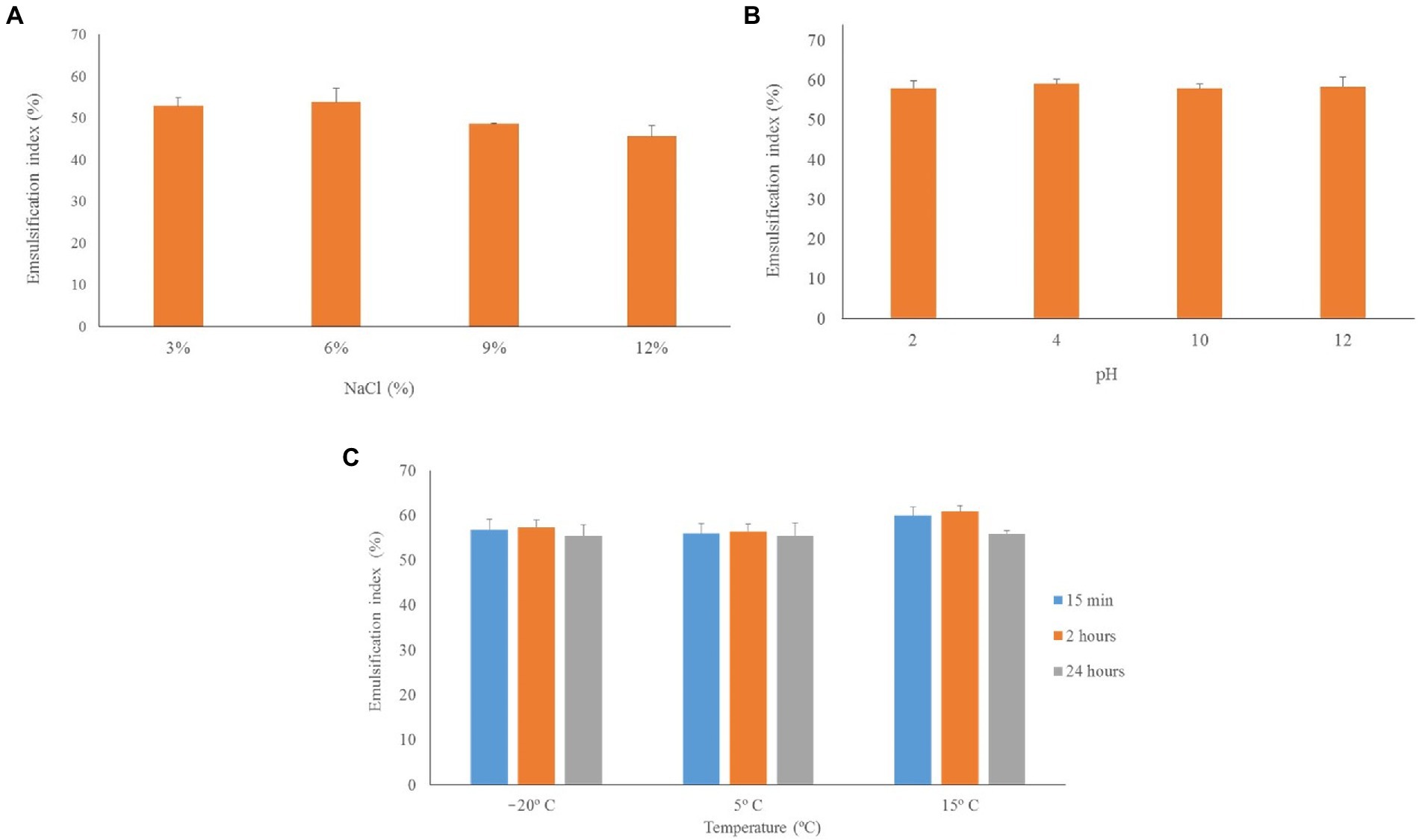
Figure 6. Stability of the bioemulsifier produced by IPAC21 under different salinity, pH and temperature conditions. (A) NaCl concentrations of 3, 6, 9 and 12%. (B) pH of 2, 4, 10, 12. (C) Temperatures of −20°C, 5°C and 15°C.
Other Paenibacillus strains showed similar responses as those observed in IPAC21 to salt and pH variations. The E24 values of the bioemulsifier produced by Paenibacillus sp.#510 were almost constant (56.2–59.7%) over the entire pH range tested (Gudina et al., 2015). Likewise, only small variations were observed in the E24% with the addition of NaCl and over a wide range of pH values in P. alvei ARN63 (Amirabadi et al., 2013). However, the sensitivity to high temperature observed in the IPAC21 bioemulsifier was not shared by Paenibacillus sp. # 510 and P. alvei ARN63. Heat treatment did not reduce the emulsion formation capacity of the bioemulsifiers produced by these strains (Amirabadi et al., 2013; Gudina et al., 2015). This variation in thermal stability can be explained mainly by the differences in the structure, density and molecular weight of the bioemulsifiers and in fermentation parameters, including the medium and the microorganism used for their production (Mummaleti et al., 2022).
Conclusion
In this study, the genome of P. antarcticus IPAC21 was presented, showing the presence of genes associated with adaptation to environmental stress conditions such as cold response, membrane fluidity mechanisms, DNA repair, oxidative and osmotic stress, chaperones and transport of compatible solutes. Genes for the biosynthesis of the bioemulsifier levan are described and compared to those of closely related Paenibacillus species. To better characterize the bioemulsifier produced by IPAC21, emulsification experiments were performed, and emulsification values using kerosene and hexadecane at three different temperatures (5°C, 15°C, and 28°C) were close to 50% or higher. The bioemulsifier was stable at different NaCl concentrations, low temperatures and pH values but not at high temperatures. All the data obtained contribute to a better knowledge of this psychrotolerant strain isolated from Antarctic soil, showing potential biotechnological applications in the petroleum industry. Given the great stability of the bioemulsifier produced by P. antarcticus IPAC21, even in a wide range of temperature, pH, and NaCl, we see as extremely relevant the future study concerning its effectiveness in bioremediating different environments (including cold marine environments) contaminated with pollutants derived from extracting and/or transporting petroleum.
Data availability statement
The datasets presented in this study can be found in online repositories. The names of the repository/repositories and accession number(s) can be found in the article/supplementary material.
Author contributions
EL, DJ, and LS conceived and designed the study. EL conducted the experiments. LP, AR, and FM contributed to the genomic data analyses. EL, LP, and LS wrote the first draft of the manuscript. All authors revised the manuscript, provided comments, and approved the final version of the manuscript.
Funding
This study was supported by grants from Conselho Nacional de Desenvolvimento Científico e Tecnológico (CNPq), Coordenação de Aperfeiçoamento de Pessoal de Nível Superior (CAPES – financial code 001) and Fundação de Amparo à Pesquisa do Estado do Rio de Janeiro (FAPERJ). AR was supported by KAUST Baseline Grant (BAS/1/1096-01-01).
Acknowledgments
The authors thank the financial support received from Conselho Nacional de Desenvolvimento Científico e Tecnológico (CNPq), Coordenação de Aperfeiçoamento de Pessoal de Nível Superior (CAPES – financial code 001), and Fundação de Amparo à Pesquisa do Estado do Rio de Janeiro (FAPERJ).
Conflict of interest
The authors declare that the research was conducted in the absence of any commercial or financial relationships that could be construed as a potential conflict of interest.
Publisher’s note
All claims expressed in this article are solely those of the authors and do not necessarily represent those of their affiliated organizations, or those of the publisher, the editors and the reviewers. Any product that may be evaluated in this article, or claim that may be made by its manufacturer, is not guaranteed or endorsed by the publisher.
Footnotes
4. ^http://jspecies.ribohost.com/jspeciesws/
5. ^www.ncbi.nlm.nih.gov/refseq
6. ^https://genome.jgi.doe.gov/portal/
References
Alikhan, N. F., Petty, N. K., Ben Zakour, N. L., and Beatson, S. A. (2011). BLAST ring image generator (BRIG): simple prokaryote genome comparisons. BMC Genomics 12:402. doi: 10.1186/1471-2164-12-402
Aliyu, H., De Maayer, P., and Cowan, D. (2016). The genome of the Antarctic polyextremophile Nesterenkonia sp. AN1 reveals adaptive strategies for survival under multiple stress conditions. FEMS Microbiol. 92, 4–32. doi: 10.1093/femsec/fiw032
Amirabadi, S. S., Jahanmiri, A., Rahimpour, M. R., Rafie, B., Darvishi, P., and Niazi, A. (2013). Investigation of Paenibacillus alvei ARN63 ability for biodemulsifier production: medium optimization to break heavy crude oil emulsion. Colloids Surf. B. Biointerfaces 109, 244–252. doi: 10.1016/j.colsurfb.2013.03.029
Angelidis, A. S., and Smith, G. M. (2003). Three transporters mediate uptake of glycine betaine and carnitine by Listeria monocytogenes in response to hyperosmotic stress. Appl. Environ. Microbiol. 69, 1013–1022. doi: 10.1128/AEM.69.2.1013-1022.2003
Ash, C., Priest, F. G., and Collins, M. D. (1993). Molecular identification of rRNA group 3 bacilli (Ash, Farrow, Wallbanks and Collins) using a PCR probe test. Proposal for the creation of a new genus Paenibacillus. Antonie Van Leeuwenhoek 64, 253–260. doi: 10.1007/BF00873085
Aziz, R. K., Bartels, D., Best, A. A., DeJongh, M., Disz, T., Edwards, R. A., et al. (2008). The RAST Server: rapid annotations using subsystems technology. BMC Genomics 9:75. doi: 10.1186/1471-2164-9-75
Barria, C., Malecki, M., and Arraiano, C. M. (2013). Bacterial adaptation to cold. Microbiology 159, 2437–2443. doi: 10.1099/mic.0.052209-0
Bezza, F. A., and Chirwa, E. M. N. (2015). Biosurfactant from Paenibacillus dendritiformis and its application in assisting polycyclic aromatic hydrocarbon (PAH) and motor oil sludge removal from contaminated soil and sand media. Process. Saf. Environ. Prot. 98, 354–364. doi: 10.1016/j.psep.2015.09.004
Bezza, F. A., and Chirwa, E. M. N. (2017). Pyrene biodegradation enhancement potential of lipopeptide biosurfactant produced by Paenibacillus dendritiformis CN5 strain. J. Hazard. Mater. 321, 218–227. doi: 10.1016/j.jhazmat.2016.08.035
Casanueva, A., Tuffin, M., Cary, C., and Cowan, D. A. (2010). Molecular adaptations to psychrophily: the impact of ‘omic’ technologies. Trends Microbiol. 18, 374–381. doi: 10.1016/j.tim.2010.05.002
Celinska, E., and Grajek, W. (2009). Biotechnological production of 2,3-butanediol–current state and prospects. Biotechnol. Adv. 27, 715–725. doi: 10.1016/j.biotechadv.2009.05.002
Chattopadhyay, M. K. (2006). Mechanism of bacterial adaptation to low temperature. J. Biosci. 31, 157–165. doi: 10.1007/BF02705244
Chaudhary, A., Chaurasia, P. K., Kushwaha, S., Chauhan, P., Chawade, A., and Mani, A. (2022). Correlating multifunctional role of cold shock domain proteins with intrinsically disordered regions. Int. J. Biol. Macromol. 220, 743–753. doi: 10.1016/j.ijbiomac.2022.08.100
Daane, L. L., Harjono, I., Barns, S. M., Launen, L. A., Palleron, N. J., and Häggblom, M. M. (2002). PAH-degradation by Paenibacillus spp. and description of Paenibacillus naphthalenovorans sp. nov., a naphthalene-degrading bacterium from the rhizosphere of salt marsh plants. Int. J. Syst. Evol. Microbiol. 52, 131–139. doi: 10.1099/00207713-52-1-131
den Besten, H. M. W., Effraimidou, S., and Abee, T. (2013). Catalase activity as a biomarker for mild-stress-induced robustness in Bacillus weihenstephanensis. Appl. Environ. Microbiol. 79, 57–62. doi: 10.1128/AEM.02282-12
Dhakar, K., and Pandey, A. (2020). Microbial ecology from the Himalayan cryosphere perspective. Microorganisms 8:257. doi: 10.3390/microorganisms8020257
Dias, B. D. C., Lima, M. E. D. N. V., Vollú, R. E., da Mota, F. F., da Silva, A. J. R., de Castro, A. M., et al. (2018). 2,3-Butanediol production by the nonpathogenic bacterium Paenibacillus brasilensis. Appl. Microbiol. Biotechnol. 102, 8773–8782. doi: 10.1007/s00253-018-9312-y
Ganesh, A., and Lin, J. (2009). Diesel degradation and biosurfactant production by gram-positive isolates. Afr. J. Biotechnol. 8, 5847–5854. doi: 10.5897/AJB09.811
Garcia-Lopez, E., Alcazar, P., and Cid, C. (2021). Identification of biomolecules involved in the adaptation to the environment of cold-loving microorganisms and metabolic pathways for their production. Biomol. Ther. 11:1155. doi: 10.3390/biom11081155
Goris, J., Konstantinidis, K. T., Klappenbach, J. A., Coenye, T., Vandamme, P., and Tiedje, J. M. (2007). DNA–DNA hybridization values and their relationship to whole-genome sequence similarities. Int. J. Syst. Evol. Microbiol. 57, 81–91. doi: 10.1099/ijs.0.64483-0
Grady, E. N., MacDonald, J., Liu, L., Richman, A., and Yuan, Z. C. (2016). Current knowledge and perspectives of Paenibacillus: a review. Microb. Cell Factories 15:203. doi: 10.1186/s12934-016-0603-7
Gudina, E. J., Pereira, J. F. B., Costa, R., Evtuguin, D. V., Coutinho, J. A. P., Teixeira, J. A., et al. (2015). Novel bioemulsifier produced by a Paenibacillus strain isolated from crude oil. Microb. Cell Factories 14:14. doi: 10.1186/s12934-015-0197-5
Hammer, Ø., Harper, D. A. T., and Ryan, P. D. (2001). PAST: paleontological statistics software package for education and data analysis. Paleontol. Electronica 4:9. http://palaeo-electronica.org/2001_1/past/issue1_01.htm
Horn, G., Hofweber, R., Kremer, W., and Kalbitzer, H. R. (2007). Structure and function of bacterial cold shock proteins. Cell. Mol. Life Sci. 64, 1457–1470. doi: 10.1007/s00018-007-6388-4
Iqbal, S., Khalid, Z. M., and Malek, K. A. M. (1995). Enhanced biodegradation and emulsification of crude oil and hyperproduction of biosurfactants by gamma ray-induced mutant of Pseudomonas aeruginosa. Lett. Appl. Microbiol. 21, 176–179. doi: 10.1111/j.1472-765X.1995.tb01035.x
Jain, D. K., Collins-Thompson, D. L., Lee, H., and Trevors, J. T. (1991). A drop-collapsing test for screening surfactant-producing microorganisms. Microbiol. Meth. 13, 271–279. doi: 10.1016/0167-7012(91)90064-W
Jeong, H., Choi, S. -K., Ryu, C. -M., and Park, S. -H. (2019). Chronicle of a soil bacterium: Paenibacillus polymyxa E681 as a tiny guardian of plant and human health. Front. Microbiol. 10:467. doi: 10.3389/fmicb.2019.00467
Jimoh, A. A., and Lin, J. (2020). Bioremediation of contaminated diesel and motor oil through the optimization of biosurfactant produced by Paenibacillus sp. D9 on waste canola oil. Biorem. J. 24, 21–40. doi: 10.1080/10889868.2020.1721425
Kumar, S., Stecher, G., Li, M., Knyaz, C., and Tamura, K. (2018). MEGA X: molecular evolutionary genetics analysis across computing platforms. Mol. Biol. Evol. 35, 1547–1549. doi: 10.1093/molbev/msy096
Lal, S., and Tabacchioni, S. (2009). Ecology and biotechnological potential of Paenibacillus polymyxa: a minireview. Indian J. Microbiol. 49, 2–10. doi: 10.1007/s12088-009-0008-y
Leeson, D. T., Gai, F., Rodriguez, H. M., Gregoret, L. M., and Dyer, R. B. (2000). Protein folding and unfolding on a complex energy land-scape. Proc. Natl. Acad. Sci. 97, 2527–2532. doi: 10.1073/pnas.040580397
Maia, M., Capão, A. S. V., and Procópio, L. (2019). Biosurfactant produced by oil-degrading Pseudomonas putida AM-b1 strain with potential for microbial enhanced oil recovery. Biorem. J. 23, 302–310. doi: 10.1080/10889868.2019.1669527
Margesin, R., and Collins, T. (2019). Microbial ecology of the cryosphere (glacial and permafrost habitats): current knowledge. Appl. Microbiol. Biotechnol. 103, 2537–2549. doi: 10.1007/s00253-019-09631-3
Meier-Kolthoff, J. P., Auch, A. F., Klenk, H. P., and Goker, M. (2013). Genome sequence-based species delimitation with confidence intervals and improved distance functions. BMC Bioinform. 14:60. doi: 10.1186/1471-2105-14-60
Mendonça, C. M. N., Oliveira, R. C., Freire, R. K. B., Piazentin, A. C. M., Pereira, W. A., Gudina, E. J., et al. (2021). Characterization of Levan produced by a Paenibacillus sp. isolated from Brazilian crude oil. Int. J. Biol. Macromol. 186, 788–799. doi: 10.1016/j.ijbiomac.2021.07.036
Monsalves, M. T., Ollivet-Besson, G. P., Amenabar, M. J., and Blamey, J. M. (2020). Isolation of a psychrotolerant and UV-C-resistant bacterium from Elephant Island, Antarctica with a highly thermoactive and thermostable catalase. Microorganisms 8:95. doi: 10.3390/microorganisms8010095
Montes, M. J., Mercadé, E., Bozal, N., and Guinea, J. (2004). Paenibacillus antarcticus sp. nov., a novel psychrotolerant organism from the Antarctic environment. Int. J. Syst. Evol. Microbiol. 54, 1521–1526. doi: 10.1099/ijs.0.63078-0
Morikawa, M., Hirata, Y., and Imanaka, T. (2000). A study on the structure function relationship of lipopeptide biosurfactants. Acta Biochem. Biophys. 1488, 211–218. doi: 10.1016/S1388-1981(00)00124-4
Mummaleti, G., Sarma, C., Kalakandan, S. K., Gazula, H., Sivanandham, V., and Anandharaj, A. (2022). Characterization of Levan produced from coconut inflorescence sap using Bacillus subtilis and its application as a sweetener. LWT Food Sci. Technol. 154:112697. doi: 10.1016/j.lwt.2021.112697
Newton, K. N., Courcelle, C. T., and Courcelle, J. (2012). UvrD participation in nucleotide excision repair is required for the recovery of DNA synthesis following UV-induced damage in Escherichia coli. J. Nucleic Acids 2012:271453. doi: 10.1155/2012/271453
Okonkwo, C. C., Ujor, V., Cornish, K., and Ezeji, T. C. (2020). Inactivation of the levansucrase gene in Paenibacillus polymyxa DSM 365 diminishes exopolysaccharide biosynthesis during 2,3- butanediol fermentation. Appl. Environ. Microbiol. 86, e00196–e00120. doi: 10.1128/AEM.00196-20
Patowary, R., and Deka, H. (2020). “Paenibacillus” in Beneficial microbes in agro-ecology. Bacteria and fungi. eds. N. Amaresan, M. S. Kumar, K. Annapurna, K. Kumar, and A. Sankaranarayanan (London: Academic Press, Elsevier), 339–354.
Perfumo, A., Banat, I. M., and Marchant, R. (2018). Going green and cold: biosurfactants from low-temperature environments to biotechnology applications. Trends Biotechnol. 36, 277–289. doi: 10.1016/j.tibtech.2017.10.016
Phadtare, S., and Severinov, K. (2010). RNA remodeling ang gene regulation by cold shock proteins. RNA Biol. 7, 788–795. doi: 10.4161/rna.7.6.13482
Prjibelski, A., Antipov, D., Meleshko, D., Lapidus, A., and Korobeynikov, A. (2020). Using SPAdes de novo assembler. Curr. Protoc. Bioinformatics 70:e102. doi: 10.1002/cpbi.102
Richter, M., and Rosselló-Móra, R. (2009). Shifting the genomic gold standard for the prokaryotic species definition. Proc. Natl. Acad. Sci. U. S. A. 106, 19126–19131. doi: 10.1073/pnas.0906412106
Richter, M., Rosselló-Móra, R., Glöckner, F. O., and Peplies, J. (2016). JSpeciesWS: a web server for prokaryotic species circumscription based on pairwise genome comparison. Bioinformatics 32, 929–931. doi: 10.1093/bioinformatics/btv681
Rodrigues, D. F., and Tiedje, J. M. (2008). Coping with our cold planet. Appl. Environ. Microbiol. 74, 1677–1686. doi: 10.1128/AEM.02000-07
Russo, R., Giordano, D., Riccio, A., di Prisco, G., and Verde, C. (2010). Cold-adapted bacteria and the globin case study in the Antarctic bacterium Pseudoalteromonas haloplanktis TAC125. Mar. Genomics 3, 125–131. doi: 10.1016/j.margen.2010.09.001
Sánchez-Osuna, M., Cortes, P., Lee, M., Smith, A. T., Barbe, J., and Erill, I. (2021). Noncanonical LexA proteins regulate the SOS response in the bacteroidetes. Nucleic Acids Res. 49, 11050–11066. doi: 10.1093/nar/gkab773
Schultz, J., and Rosado, A. (2020). Extreme environments: a source of biosurfactants for biotechnological applications. Extremophiles 24, 189–206.
Selbitschka, W., Arnold, W., Priefer, U. B., Rottschafer, T., Schmidt, M., Simon, R., et al. (1991). Characterization of recA genes and recA mutants of rhizobium meliloti and rhizobium leguminosarum biovar viciae. Mol. Gen. Genet. 229, 86–95. doi: 10.1007/BF00264217
Seldin, L., and Dubnau, D. (1985). DNA homology among Bacillus polymyxa, Bacillus macerans, Bacillus azotofixans and others nitrogen fixing Bacillus strains. Int. Syst. Bacteriol. 35, 151–154. doi: 10.1099/00207713-35-2-151
Seldin, L., Rosado, A. S., Cruz, D. W., Nobrega, A., van Elsas, J. D., and Paiva, E. (1998). Comparison of Paenibacillus azotofixans strains isolated from rhizoplane, rhizosphere and non-rhizosphere soil from maize planted in two different Brazilian soils. Appl. Environ. Microbiol. 64, 3860–3868. doi: 10.1128/AEM.64.10.3860-3868.1998
Shibulal, B., Al-Bahry, S. N., Al-Wahaibi, Y. M., Elshafie, A. E., Al-Bemani, A. S., and Joshi, S. J. (2017). The potential of indigenous Paenibacillus ehimensis BS1 for recovering heavy crude oil by biotransformation to light fractions. PLoS One 12:e0171432. doi: 10.1371/journal.pone.0171432
Silva, R. C. F. S., Almeida, D. G., Rufino, R. D., Luna, J. M., Santos, V. A., and Sarubbo, L. A. (2014). Applications of biosurfactants in the petroleum industry and the remediation of oil spills. Int. J. Mol. Sci. 15, 12523–12542. doi: 10.3390/ijms150712523
Song, B., and Springer, J. (1996). Determination of interfacial tension from the profile of a pendant drop using computer-aided image processing. J. Colloid Interface Sci. 184, 77–91.
Tinôco, D., de Castro, A. M., Seldin, L., and Freire, D. M. G. (2021). Production of (2R,3R)-butanediol by Paenibacillus polymyxa PM 3605 from crude glycerol supplemented with sugarcane molasses. Process Biochem. 106, 88–95. doi: 10.1016/j.procbio.2021.03.030
Uzoigwe, C., Burgess, J. G., Ennis, C. J., and Rahman, P. K. (2015). Bioemulsifiers are not biosurfactants and require different screening approaches. Front. Microbiol. 6:245. doi: 10.3389/fmicb.2015.00245
Vollú, R. E., Jurelevicius, D., Ramos, L. R., Peixoto, R. S., Rosado, A. S., and Seldin, L. (2014). Aerobic endospore-forming bacteria isolated from Antarctic soils as producers of bioactive compounds of industrial interest. Polar Biol. 37, 1121–1131. doi: 10.1007/s00300-014-1505-y
Xu, X., Gao, C., Liu, Z., Wu, J., Han, J., Yan, M., et al. (2016). Characterization of the Levan produced by Paenibacillus bovis sp. nov BD3526 and its immunological activity. Carbohydr. Polym. 144, 178–186. doi: 10.1016/j.carbpol.2016.02.049
Xu, M., Zhang, L., Zhao, F., Wang, J., Zhao, B., Zhou, Z., et al. (2021). Cloning and expression of levansucrase gene of Bacillus velezensis BM-2 and enzymatic synthesis of levan. PRO 9:317. doi: 10.3390/pr9020317
Yadav, A. N., Yadav, N., Sachan, S. G., and Saxena, A. K. (2019). Biodiversity of psychrotrophic microbes and their biotechnological applications. J. Appl. Biol. Biotechnol. 7, 99–108. doi: 10.7324/JABB.2019.70415
Keywords: Paenibacillus antarcticus, genome, bioemulsifier, levan, Antarctica
Citation: de Lemos EA, Procópio L, da Mota FF, Jurelevicius D, Rosado AS and Seldin L (2023) Molecular characterization of Paenibacillus antarcticus IPAC21, a bioemulsifier producer isolated from Antarctic soil. Front. Microbiol. 14:1142582. doi: 10.3389/fmicb.2023.1142582
Edited by:
Syed Gulam Dastager, National Chemical Laboratory (CSIR), IndiaReviewed by:
Satya P. Singh, Saurashtra University, IndiaAlysson Wagner Fernandes Duarte, Federal University of Alagoas, Brazil
Copyright © 2023 de Lemos, Procópio, da Mota, Jurelevicius, Rosado and Seldin. This is an open-access article distributed under the terms of the Creative Commons Attribution License (CC BY). The use, distribution or reproduction in other forums is permitted, provided the original author(s) and the copyright owner(s) are credited and that the original publication in this journal is cited, in accordance with accepted academic practice. No use, distribution or reproduction is permitted which does not comply with these terms.
*Correspondence: Lucy Seldin, bHNlbGRpbkBtaWNyby51ZnJqLmJy