- 1CIBIOP Group, Department of Biological Sciences, Universidad EAFIT, Medellin, Colombia
- 2Department of Microbiology, The Ohio State University, Columbus, OH, United States
- 3Banana Research Center, Augura, Conjunto Residencial Los Almendros, Carepa, Colombia
- 4Division of Medicinal Chemistry and Pharmacognosy, The Ohio State University, Columbus, OH, United States
- 5Center for Applied Plant Sciences, The Ohio State University, Columbus, OH, United States
- 6Infectious Diseases Institute, The Ohio State University, Columbus, OH, United States
Different Bacillus species have successfully been used as biopesticides against a broad range of plant pathogens. Among these, Bacillus tequilensis EA-CB0015 has shown to efficiently control Black sigatoka disease in banana plants, presumably by mechanisms of adaptation that involve modifying the phyllosphere environment. Here, we report the complete genome of strain EA-CB0015, its precise taxonomic identity, and determined key genetic features that may contribute to its effective biocontrol of plant pathogens. We found that B. tequilensis EA-CB0015 harbors a singular 4 Mb circular chromosome, with 3,951 protein-coding sequences. Multi-locus sequence analysis (MLSA) and average nucleotide identity (ANI) analysis classified strain EA-CB0015 as B. tequilensis. Encoded within its genome are biosynthetic gene clusters (BGCs) for surfactin, iturin, plipastatin, bacillibactin, bacilysin, subtilosin A, sporulation killing factor, and other natural products that may facilitate inter-microbial warfare. Genes for indole-acetic acid (IAA) synthesis, the use of diverse carbon sources, and a multicellular lifestyle involving motility, biofilm formation, quorum sensing, competence, and sporulation suggest EA-CB0015 is adept at colonizing plant surfaces. Defensive mechanisms to survive invading viral infections and preserve genome integrity include putative type I and type II restriction modification (RM) and toxin/antitoxin (TA) systems. The presence of bacteriophage sequences, genomic islands, transposable elements, virulence factors, and antibiotic resistance genes indicate prior occurrences of genetic exchange. Altogether, the genome of EA-CB0015 supports its function as a biocontrol agent against phytopathogens and suggest it has adapted to thrive within phyllosphere environments.
Introduction
Endospore forming bacteria of the genus Bacillus are ubiquitous within terrestrial and aquatic environments (Nicholson, 2002). Industrially important species, including B. subtilis, B. amyloliquefaciens, B. velezensis, B. licheniformis, and B. pumilus, form a phylogenetically coherent group known as the B. subtilis species complex (Fritze, 2004). In addition to being an important source of industrial enzymes, vitamins, and cofactors (Harwood et al., 2018), many of these species are used as the active ingredient in several commercial biopesticides and biofertilizers (Velivelli et al., 2014; Ngalimat et al., 2021). Well known strains including B. amyloliquefaciens FZB42 (reclassified as B. velezensis; Chen et al., 2009), B. subtilis QST713 (recently reclassified as B. velezensis; Pandin et al., 2018), B. amyloliquefaciens GB03 (Choi et al., 2014), and B. subtilis MBI600 (Samaras et al., 2021) are used to control soil and foliar bacterial and fungal diseases. These strains provide a natural alternative to synthetic agrochemicals that have detrimental effects to human health and the environment.
The utility of Bacilli as biocontrol and bioaugmentation agents derives from traits inherent to their natural lifestyle as microbial endo- and epiphytes. These bacteria localize to plants via directed movement to phytochemicals (e.g., chemotaxis to organic acids such as malic and fumaric acid; Tsai et al., 2020; Ma et al., 2021) and then use catabolic pathways to consume these compounds as carbon, nitrogen, and energy sources for growth. Colonization of plant surfaces and tissues occurs through the establishment of biofilms (Rudrappa et al., 2008). Equally significant are the natural products Bacilli produce and their effects on modulating plant development and the composition of resident microbiomes. Bacilli produce phytohormones including auxins (indole acetic acid, IAA), cytokinins, and gibberellins that regulate plant growth and differentiation. These compounds are important modulators of many plant processes ranging from abiotic stress response to flowering, fruit development, and seed germination (Poveda and González-Andrés, 2021). Other antimicrobial natural products such as cyclic lipopeptides (surfactin, iturin, and fengycin/plipastatin), siderophores (bacillibactin), bacteriocins (sublancin, subtilosin; Stein, 2005) may provide ecological advantages to the Bacilli by suppressing fungal and bacterial competitors. The importance of these compounds is further underscored by the abundance and conservation of natural product biosynthetic gene clusters (BGCs) within the genomes of plant-associated Bacilli. Indeed, B. velezensis QST713 harbors 15 natural product BGCs (Pandin et al., 2018), while B. amyloliquefaciens GB03 and B. subtilis MBI600 harbor 8 and 7 BGCs, respectively (Choi et al., 2014; Samaras et al., 2021).
While most species in the B. subtilis complex have been well characterized, significantly less is understood about the physiology and ecology of B. tequilensis. First described in 2006, emergent interest in B. tequilensis has been driven by its natural ability to suppress diverse fungal pathogens of commercial grains, vegetables, fruits, and ornamentals (Tam et al., 2020; Xu et al., 2021; Zhou et al., 2021; Kwon et al., 2022). Among B. tequilensis, one of the best characterized strains is EA-CB0015. Originally isolated in Uraba, Colombia, EA-CB0015 was discovered as a natural antagonist of Pseudocercospora fijiensis (Ceballos et al., 2012), the causative agent of black Sigatoka. This agriculturally devastating disease of banana plants causes necrotic streaks on the leaves, reduces photosynthetic capacity, and promotes premature ripening of the fruit. The resulting loses can be greater than 50% if left untreated (Noar et al., 2022). Mitigation of black Sigatoka represents a significant burden to Colombian producers, with a cost $65 million per year due to weekly application of fungicides (S. Zapata, personal communication). EA-CB0015 suppresses black Sigatoka disease through the colonization of banana leaves and the production of antifungal lipopeptides (iturins, plipastatins, and surfactins; Villegas-Escobar et al., 2013; Mosquera et al., 2014; Cuellar-Gaviria et al., 2021). In addition to bananas, the strain also reduced the severity of anthracnose (Colletotrichum spp.) in tamarillo fruits and gray mold (Botrytis cinerea) in chrysanthemum flowers (Gutierrez-Monsalve et al., 2015; Arroyave-Toro et al., 2017). Although B. tequilensis has yet to be commercialized, these studies collectively demonstrate the significant potential of this species for the control and prevention of agricultural diseases.
Here we sequenced the complete genome of strain EA-CB0015 to understand the genetic features integral to its success as a microbial epiphyte and biocontrol agent. Using different molecular taxonomy approaches, we show that EA-CB0015 is classified as B. tequilensis. Our analysis and comparisons of its genome with other plant associated Bacilli identified mechanisms for plant colonization and the inhibition of phytopathogens. These include genes for sporulation, biofilm formation, and the ability to metabolize diverse nutrient sources. Distinct mechanisms of protection against abiotic and biotic factors were present, including genes that direct biosynthesis of an arsenal of natural products. Lastly, features related to horizontal gene transfer including the presence of genomic islands (GEIs), insertion sequences (IS), toxin antitoxin (TA) systems, restriction modification (RM) system, and prophages are described.
Materials and methods
Genome sequencing and assembly
Bacillus tequilensis EA-CB0015 was deposited in ATCC as PTA-123533. The strain was revived from glycerol stocks onto half strength tryptic soy agar [20 g/L of TSA (Oxoid) and 9 g/l of Bacto agar (BD)] and incubated at 37°C for 24 h. A single colony was inoculated into a 100 mL Erlenmeyer containing 20 mL of tryptic soy broth (Oxoid) and grown for 8 h in a shaking incubator (250 rpm, 37°C). Genomic DNA was extracted using the UltraClean Microbial DNA Isolation kit (MoBio) for Illumina sequencing and DNeasy Blood & Tissue kit (Qiagen) for Single Molecule Real-Time (SMRT) sequencing. Samples were sequenced on an Illumina HiSeq 2000 (100 bp paired end reads) and PacBio RS II (Macrogen, Inc). Contigs less than 100 bp were discarded. Unicycler v0.4.8.0 was used for hybrid assembly using the normal bridging mode (Wick et al., 2017).
General annotation
The assembled genome was annotated using the NCBI Prokaryotic Genome Annotation Pipeline (PGAP) v6.0 (Haft et al., 2018) and deposited under accession no. NZ_CP048852. SkewDB was used to predict replication origins and termini (Hubert, 2022). Genome figures were created using the CGView Server (Grant and Stothard, 2008). Predicted proteins were classified into clusters of orthologous groups (COGs) using WebMGA (Wu et al., 2011), and analyzed in the Kyoto Encyclopedia of Genes and Genomes database (KEGG; entry T07089) to assign roles in metabolic pathways (Kanehisa et al., 2016).
Multi-locus sequence analysis
All strains analyzed in this study are listed in Supplementary Table S1. Reference strains included B. subtilis species complex (B. pumilus, B. subtilis, B. tequilensis, B. mojavensis, B. atrophaeus, B. velezensis, and B. amyloliquefaciens, B. licheniformis), the B. cereus species complex (B. cereus, B. thuringiensis, B. anthracis, B. mycoides), and B. coagulans. Clostridium kluyveri DSM 555T served as the outgroup.
Genomes were downloaded from public sequence repositories and annotated using Prokka v1.14.6 (Seemann, 2014). Genes encoding groEL (chaperonin, large subunit), gyrA (DNA gyrase, subunit A), rpoB (RNA polymerase, beta subunit), polC (DNA polymerase III, alpha subunit), and purH (phosphoribosylaminoinidazole carboxamide formyltransferase) were retrieved from each strain, aligned using MAFFT v7.4 (Katoh, 2002), manually trimmed, and concatenated head to tail using AMAS v0.98 (Borowiec, 2016). The matrix (12,483 positions; 1,647 from groEL, 2,517 from gyrA, 3,030 from polC, 1,542 from purH, and 3,747 from rpoB) was analyzed using ModelTest-NG v0.1.6 (Darriba et al., 2020) to determine the best substitution model for each partition. Phylogenetic trees were constructed using maximum-likelihood (ML) and Bayesian inference (BI) methods. The ML phylogeny using the GTR + I + G4 model was best for groEL, gyrA, purH and rpoB, and GTR + G4 for polC, with bootstrapping (1,000) using IQ-TREE v1.6.9 (Nguyen et al., 2015). The BI phylogeny was calculated using Markov Chain Monte Carlo (MCMC) analysis and the GTR + I + G4 model using Mr. Bayes v.3.2 (Ronquist et al., 2012). Two independent runs were performed for 10 million generations (1 sampling every 1,000 generations). Effective sample size, convergency, and stationarity values were evaluated using Tracer v1.7.1 (Rambaut et al., 2018). The final phylogenetic tree was generated using DendroPy v4.4.0 (Sukumaran and Holder, 2010) and visualized using iToL v5.0 (Letunic and Bork, 2019).
Growth assays
The ability of strain EA-CB0015 to utilize different carbon sources was performed using a BioLog GEN III Microplate (Biolog Inc.). EA-CB0015 was grown on Universal Growth Agar (BUG; Biolog Inc.) and incubated at 30°C for 24 h. Isolated colonies were resuspended in the “Inoculation Fluid-A” (IF-A; Biolog Inc.) following manufacturer’s instructions and this suspension was inoculated into the Gen III microplate. After 24 h incubation at 30°C, the microplate was analyzed qualitatively for color development using a MicroStation™ 2 Reader (Biolog Inc.), measuring OD at 595 and 750 nm.
Sporulation, motility, and biofilm formation assays
Colonies grown on TSA plates were stained for endospores using Schaeffer-Fulton method (Gerhardt et al., 1994). Micrographs were recorded using an Axiostar Plus microscope (Carl Zeiss) at 100X equipped with an AxioCam ICc3 (Carl Zeiss) and ZEN 2.3 lite software (Carl Zeiss). Biofilm formation was examined by inoculating a diluted culture of EA-CB0015 into 12-well plates with LBGM media. These were incubated for 48 h at 30°C (statically) and photographed (7.1 MP digital camera, Kodak EasyShare P712). Motility was assayed by inoculating 3 μl EA-CB0015 grown on tryptic soy broth TSB (Oxoid; OD 1.0, 600 nm) onto the center of 10% LB plates solidified with either 0.7% (swarming) or 0.3% agar (swimming), and incubated at 30°C for 24 h (Kearns and Losick, 2003; Ghelardi et al., 2012). Flagella were visualized using Ryu stain (Heimbrook et al., 1989).
Analysis of natural product biosynthetic gene clusters
Genomes were analyzed using antiSMASH v5.1.2 (Blin et al., 2019). BGCs with similarity scores > 70% were reported for all 58 Bacillus strains. Regions encoding with similarity scores less than <70% were further analyzed for strain EA-CB0015 using BLAST (Johnson et al., 2008) and HMMER v3.3.1 (Eddy, 2011). BGCs for natural products known to be produced by Bacilli but not detected by antiSMASH were manually identified by BLAST.
Analysis of virulence factors, antibiotic resistance, and genetic exchange
Putative phage and phage-like regions were identified using PHASTER (Zhou et al., 2011; Arndt et al., 2016). Data ordering, sub-setting and reshaping was performed using Tidyverse v1.3.0 (Wickham et al., 2019) and an in-house script uploaded on GitHub1 (García-Botero et al., 2021). GEIs were identified using IslandViewer 4 (Bertelli et al., 2017) and ISs identified using ISfinder (e-value cutoff of 1e-07; Siguier et al., 2006). Integrative or conjugative elements (ICEBs1) were predicted with ICEBerg 2.0 (Liu M. et al., 2019). Antimicrobial resistance genes were identified using ResFinder-4.0 (70% identity threshold; minimum length of 70%; Bortolaia et al., 2020), and putative virulence factors from the Virulence Factors of Pathogenic Database (VFDB; Liu B. et al., 2019). RM systems were retrieved from REBASE (Roberts et al., 2015). Cluster BLAST analysis of identified RM systems were performed using Cblaster v1.3.0 (Gilchrist et al., 2021). Potential TA systems identified using TAfinder (Xie et al., 2018) and manually BLAST searches. Finally, CRISPRCasFinder (Couvin et al., 2018) was used to identify CRISPRs and Cas genes.
Comparative genomics
Synteny was analyzed using Easyfig v2.2.2 (Sullivan et al., 2011). ANI values (Richter and Rosselló-Móra, 2009) were calculated using GTDB-Tk v0.3.2 (Chaumeil et al., 2019). Comparisons between B. subtilis 168, B. tequilensis ATCC BAA 819T and B. tequilensis EA-CB0015 were performed using proteome and protein family functions in PATRIC (Davis et al., 2020). Presence or absence of predicted genes and proteins in EA-CB0015 were compared to B. subtilis 168 (NBCI Reference Sequence: NC_000964.3) using SubtiWiki (Zhu and Stülke, 2018). E-values < 1e-5 and sequence identities > 70% were used as cutoffs.
Results
The genome of EA-CB0015
Hybrid assembly of Illumina and SMRT sequencing reads produced a single circularized chromosome of 4,012,371 bp with an average GC content of 43.7%. In total, 4,112 CDS (3,951 genes, 161 pseudogenes), 10 copies of rRNAs (5S, 16S, and 23S), 86 tRNAs, and 5 ncRNAs were present. The forward strand encoded 2,134 genes and the reverse strand 1,978 genes (Figure 1). GC-skew suggests single symmetrical, bi-directional replication of the genome (Supplementary Figure S1). COG analysis assigned these into 5,163 protein families, most of which belonged to general or unknown function (categories R and S). Besides these, the largest groups of proteins are dedicated for amino acid transport and metabolism (E), energy production and conversion (C), translation, ribosomal structure, and biogenesis (J), DNA replication, recombination, and repair (L), and transcription (K; Supplementary Figure S2).
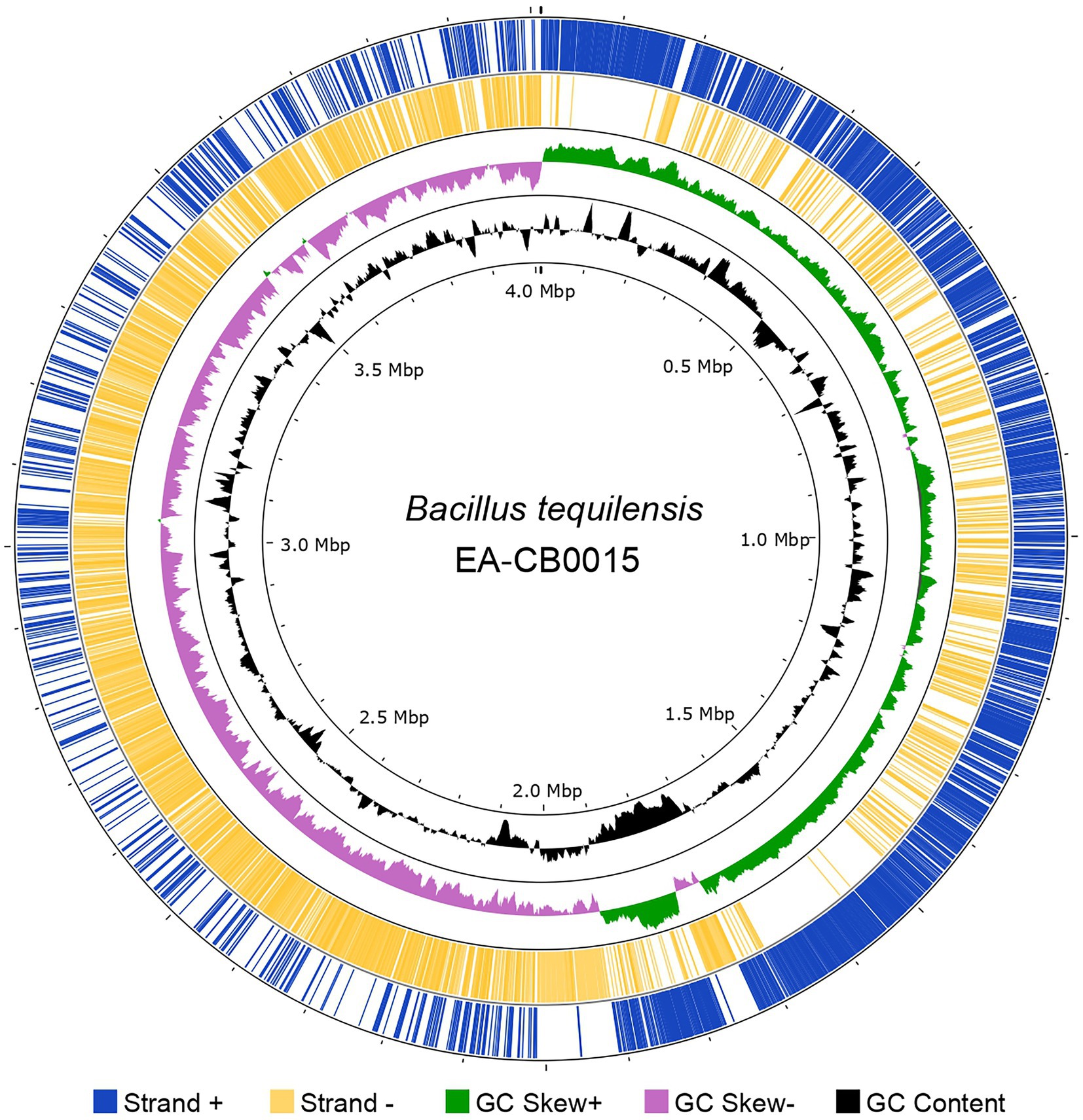
Figure 1. The genome of Bacillus tequilensis EA-CB0015. GenBank features (CDS) in the forward strand (blue) and reverse strand (yellow); GC skew (green and purple) and GC content (black). Image created with Proksee.
Molecular taxonomy classifies EA-CB0015 as Bacillus tequilensis
EA-CB0015 was originally identified as B. subtilis (Ceballos et al., 2012) and later referred to as B. tequilensis (Cuellar-Gaviria et al., 2021). This ambiguity derived from the use of 16S rRNA gene phylogenies, which do not provide sufficient resolution at the species level for strains within the B. subtilis complex (Rooney et al., 2009). To definitively determine the species identity of the strain, we performed multilocus sequence analysis (MLSA) using established marker genes shown to effectively determine speciation in Bacillus. EA-CB0015 was more closely related to B. tequilensis ATCC BAA 819T than to other B. subtilis subspecies, including subtilis, natto, stercoris, spizizenii and inaquosorum (Figure 2A). This is supported by high ANI values between the genomes of strain EA-CB0015 and B. tequilensis ATCC BAA 819T (98.6%), vs. B. subtilis subsp. inaquosorum KCTC 13429T (92.3%) B. subtilis subsp. subtilis 168 (91.4%; Supplementary Table S2). Thus, EA-CB0015 belongs to the phylogenetically homogeneous B. subtilis species complex and is classified as B. tequilensis.
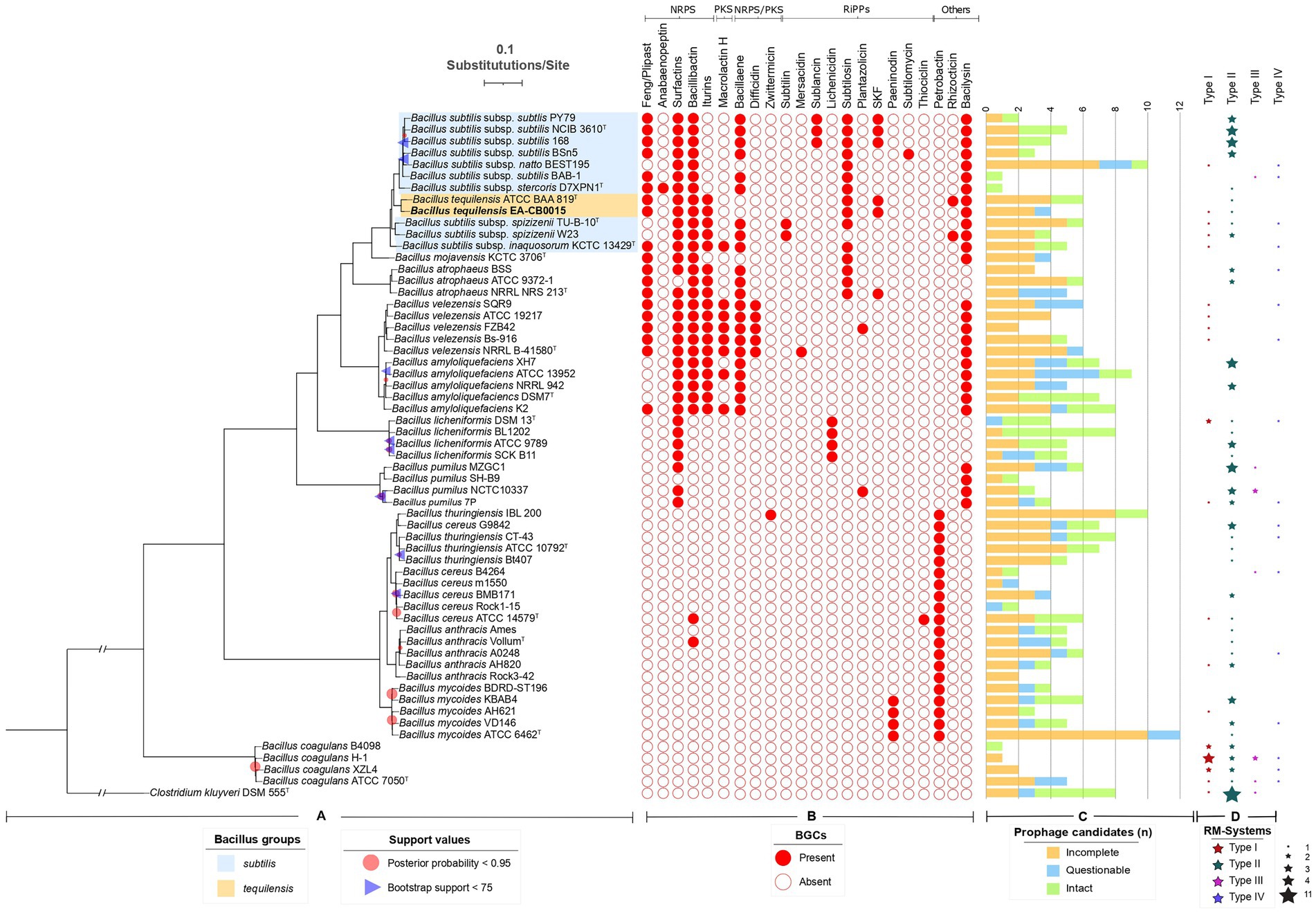
Figure 2. Phylogeny of EA-CB0015 and related Bacilli with their natural product BGCs, prophages and RM systems. (A) Consensus MLSA phylogeny from maximum likelihood (ML) and Bayesian inference (BI). Node supports with posterior probability values <0.95 and bootstrap support values < 75 are shown. (B) Natural product BGCs predicted by antiSMASH. NRPs, non-ribosomally synthetized peptides; PKs, polyketides; RiPPs, ribosomally produced post-translationally modified peptides. (C) Number of regions annotated as prophages according to PHASTER. Green bars indicate intact prophages (scores > 90); blue bars, questionable (70–90); orange bars, incomplete (<70). (D) Distribution and abundance of RM-Systems according to REBASE or manually searched.
Comparative genomics of Bacillus tequilensis EA-CB0015, Bacillus tequilensis ATCC BAA 819T, and Bacillus subtilis 168
The phylogenetic analysis suggests B. tequilensis and B. subtilis share a close evolutionary history. To understand the nature of this relationship, we identified genetic similarities and differences between EA-CB0015, ATCC BAA 819T, and 168. The GC content of all three strains were similar (43.5%–44.0%) and they contained the identical number of rRNA and tRNA encoding genes. The B. tequilensis genomes were comparable in size (4 Mbp) and the number of protein coding sequences, but both were smaller than B. subtilis 168 (4.2 Mbp; Supplementary Table S3). Except for an extra 1.4 kbp plasmid in ATCC BAA 819T, both B. tequilensis genomes were largely syntenic (Figure 3A). Although less conservation was observed between 168 and EA-CB0015, large segments of their genomes remained homologous with each other. A 132 kbp inversion corresponding to bacteriophage SPβ was near the replication terminus (terC) of both strains (Figure 3A). In strain 168, the prophage was encoded on the complementary strand 134 kb upstream terC, while in strain EA-CB0015 it was on the positive strand 186 kb downstream of terC. Interestingly, this region was highly variable between 168 and EA-CB0015, and completely absent from the genome of B. tequilensis ATCC BAA 819T. Compared to 168, the SPβ prophage region of EA-CB0015 lacked the sublancin BGC and its immunity gene (Denham et al., 2019), a gene encoding for DNA cytosine-5-methyltransferase (mtbP), and numerous hypothetical proteins with unknown function. Most of the encoded proteins and their families were shared between all three strains (Figure 3B; Supplementary Table S4).
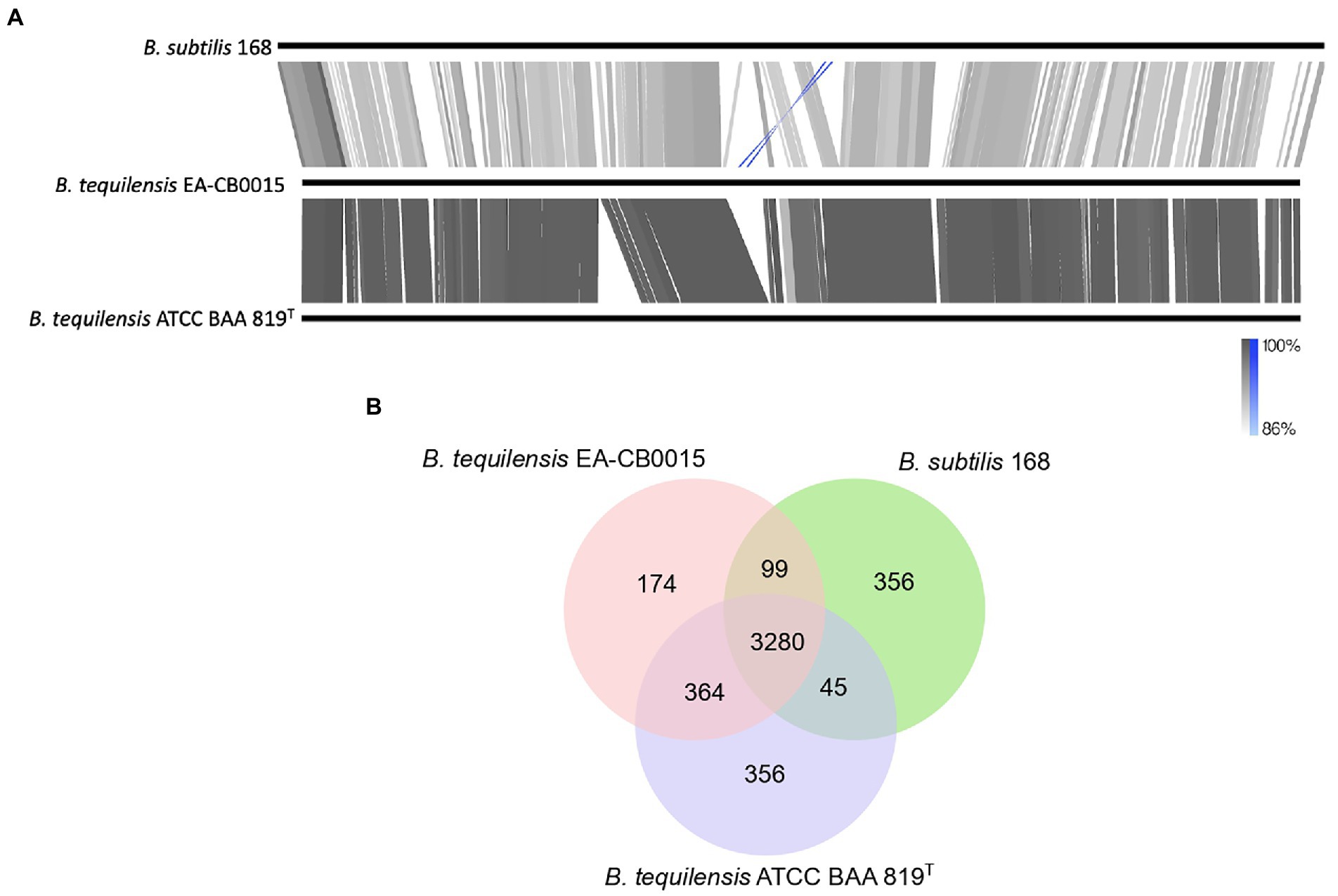
Figure 3. Comparison of Bacillus subtilis 168, Bacillus tequilensis EA-CB0015 and B. tequilensis ATCC BAA 819T (A) Global genome alignments. Gray lines and blocks indicate regions and degree of shared similarity. Blue represents inversions (B) Shared and unique proteins between the three strains.
Motility and biofilm formation
Multicellular behavior is used by B. subtilis to afford survival in its natural environment. These mechanisms include motility, biofilm formation, cannibalism, quorum sensing, competence, and sporulation (González-Pastor, 2017). As B. subtilis 168 is well studied for its ability to assemble multicellular communities and closely related to EA-CB0015 (O’Toole et al., 2000; González-Pastor, 2017), we examined if genes for these phenotypes were also present in our strain.
Genes for flagellum-mediated motility including chemotaxis and swarming were present in the EA-CB0015 genome. All thirty-two genes required to form the flagellar basal body and hook were encoded within a 27-kb fla/che operon, as well as all the genes needed for filament formation (flagellin monomer protein Hag, FliD, FlgK, FlgL), assembly, and rotation (Supplementary Table S5A; Mukherjee and Kearns, 2014). Other genes required for the basal body formation (FlhO and FlhP), torque in flagellar rotation (MotA, MotB), cell separation (LytC), bistable regulation (SigD, SwrA, SwrB, DegS/DegU, YmdB, and SlrA/SinR/SlrR), chemotaxis and associated chemoreceptors (2 soluble and 7 membrane-bound) were also present (Supplementary Tables S5A,B). Microscopy showed motile, polarly flagellated cells (Supplementary Figure S3). These results suggest EA-CB0015 can sense and swim toward a wide range of attractants (pH, amino acids, oxygen, etc.) that are present in its local environment.
To form a biofilm, cells must transition from motile to sessile states, aggregate, and embed themselves within a self-produced extracellular matrix (EM; Arnaouteli et al., 2021). EA-CB0015 formed biofilms in static culture (Supplementary Figure S3), and as expected, the genome of EA-CB0015 encoded genes essential for this process including exopolysaccharide biosynthesis (epsA-O), protein fiber TasA (tapAsipWtasA operon), the hydrophobin-like protein BslA, and genes for poly-γ-glutamic acid (γ-PGA) biosynthesis (pgsBCAE; Supplementary Table S5C). Production of EM is also linked to multiple regulatory proteins that were all present within the genome (Supplementary Table S5C). The Spo0A pathway (KinC, KinD, Spo0A, SinI/SinR/SlrR, AbrB) controls the expression of more than 100 genes including biofilm matrix gene expression and sporulation, while the YwcC-SlrA stress response pathway facilitates adaptation to changing environmental conditions. The DegS–DegU two-component system regulates competence, motility and secretion of degradative enzymes. Lastly, genes that mediate expression of slrR, an essential regulator of biofilm formation were also present (abh, ymdB, remA, remB; Vlamakis et al., 2014).
Interestingly, EA-CB0015 does not harbor the yitPOM operon. The paralogous sdpABC operon, is also absent from the genome of the strain. The yitPOM operon expresses the biofilm associated toxin (YitM) and the extracellular protease (NprB), both of which suppress competitors in B. subtilis biofilms (Kobayashi and Ikemoto, 2019). In addition, surfactin, encoded in the srfA operon (Supplementary Table S5B), may induce potassium leakage that stimulates the sensor kinase KinC. This may also activate expression of biofilm formation genes (López et al., 2009).
Cannibalism
Cannibalistic behavior delays the entry into sporulation within a subpopulation of cells. This is controlled by the production of sporulation delaying protein-Sdp and sporulation killing factor-Skf, which lyse and kill sensitive siblings (González-Pastor et al., 2003). Interestingly, EA-CB0015 contained genes for Skf (skfA-H), but not Sdp (sdpABC and sdpRI operons were absent; Supplementary Table S5D). These results suggest EA-CB0015 cells may exhibit an accelerated sporulating phenotype. As Spo0A-inactive cells are not lysed, the pool of nutrients released into the environment from cell death is reduced. Thus, neighboring cells experience starvation to initiate spore formation (González-Pastor et al., 2003).
Quorum sensing and competence
Genes encoding mechanisms of quorum sensing were present in the EA-CB0015 genome (Supplementary Table S5E). These may allow strain EA-CB0015 to coordinate physiological processes such as the synthesis of exoproteases and other extracellular enzymes in response to cell density. The lipopeptide surfactin is also positively regulated by the phosphorylated form of ComA, which is part of the quorum sensing (QS) system (ComQXPA) in B. subtilis (Nakano et al., 1991; Kalamara et al., 2018). Interestingly, the putative ComQ, ComX, and ComP proteins shared low sequence identity with their corresponding homologs in 168 (Supplementary Table S5E). This suggests the Com system may handle separate social communication groups or pherotypes (Stefanic and Mandic-Mulec, 2009; Oslizlo et al., 2014). The genome also encoded six putative receptor-signal pairs of the Rap-Phr system (Rap-Phr A, C, E, F, H; Supplementary Table S5E). Similar to 168, accumulation of Phr peptides in EA-CB0015 may suppress effects of Rap proteins to allow expression of genes for swarming motility, biofilm formation, exoprotease secretion and genetic competence (Kalamara et al., 2018). Related to quorum sensing, EA-CB0015 also contained genes for the acquisition and incorporation of extracellular DNA into the host cell (genetic competence). These include the master competence regulator comK, genes encoding proteins essential for DNA binding and import (comC, operons comE, comF, comG, bdbD, bdbC), and cytosolic proteins that modulate recombination and transformation efficiency (RecA, SsbB, DprA, CoiA, NucA). Genes encoding proteins for transcriptional (Rok, CodY, Kre) and post-translational regulation (MecA, ComS) of ComK were also present (Supplementary Table S5F).
Sporulation
Endospores were visible when cells were subjected to Ryu stain (Supplementary Figure S3). Indeed, genes needed for sporulation were present in the genome (Supplementary Table S5G). These included genes encoding for morphogenetic proteins (SpoIVA, SpoVM, SpoVID, SafA, CotE, CotX/CotY/CotZ), their interacting partners during spore coat assembly, and spore crust proteins. Interestingly, some shared < 60% sequence identity with the homologs in 168, while others were completely absent (Supplementary Table S5G). The biosynthetic genes for legionaminic acid, which is used in crust formation, were notably missing (Supplementary Table S5G). Their absence suggests that the surface of EA-CB0015 spores may be reduced in hydrophilicity and charge (Dubois et al., 2020).
Most of the genes encoding signal transduction proteins (histidine sensor kinases KinA-E, master regulator Spo0A, phosphotransferases Spo0F and Spo0B, etc) related to sporulation were identified (Supplementary Table S5G). Homologs of lrpAB were missing, but other studies have indicated negligible effects on glyA transcription or sporulation through KinB (Dartois et al., 1997). The absence of sivC may result in a greater sporulation efficiency, as it functions as an inhibitor of the KinB and KinC pathway (Garti-levi et al., 2013). As expected, genes encoding proteins involved with major events in spore gemination were also present (Setlow et al., 2017). These include genes needed for germinant sensing (germinant receptors), release of dipicolinic acid (DPA) related to heat resistance (spoVA proteins, GerD), and hydrolysis of cortex peptidoglycan (CwlJ, SleB, SleL; Supplementary Table S5G). Altogether, these data suggest EA-CB0015 endospores can fully germinate once in favorable environmental conditions.
Pathways for carbon assimilation
Nutrient availability on leaves, especially for organic compounds, is spatially heterogeneous and limited (Leveau and Lindow, 2001; Lindow and Brandl, 2003). A major carbon source that leach from the interior of the plant are common sugars (Wildman and Parkinson, 1981; Fiala et al., 1990; Lindow and Brandl, 2003). EA-CB0015 encodes all the genes necessary for glucose assimilation into the TCA cycle (KEGG pathway map ID bteq00020) by glycolysis (bteq00010). Likewise, genes for the metabolism sucrose and starch (bteq00500), and fructose (via fructose-1P and frutokinase (bteq00051)) were present. Pathways for galactose, maltose, and raffinose catabolism were also predicted (bteq00052, bteq00500). However, not all the genes needed for inositol utilization were present (bteq00562), such as those that encode transporters (iolF, iolT). Genes needed for scyllo-inosose transformation (iolE, iolD, iolB, iolC, iolJ) were also absent (Supplementary Table S6).
In addition to sugars, organic acids (e.g., L-lactic acid, citric acid, and L-malic acid) are common on the foliage of plants (Morgan and Tukey, 1964). EA-CB0015 utilized these and other compounds as carbon sources in API 50 CHB/E (Villegas-Escobar et al., 2013) and Biolog GEN III Microplate growth assays (Supplementary Table S7). Although methanol is readily available on plant surfaces (Kutschera, 2007), the absence of genes for methanol dehydrogenase suggest EA-CB0015 is unable to consume it as carbon source. However, genes for formaldehyde fixation (hxlA, hxlB) shared high sequence identity (>90%) with their corresponding homologs in 168, suggesting formaldehyde may be assimilated through the ribulose monophosphate pathway (bteq_M00345, Supplementary Table S6). This may allow EA-CB0015 to scavenge formaldehyde produced by neighboring methylotrophs during growth on plant surfaces.
Hydrolytic enzymes
EA-CB0015 contained genes for β-glucanases (bglC, bglS), chitinase (Csn), extracellular proteases (aprE, nprE), bacillopeptidase F protein degradation (Bpr), endolevanase (levC), xylanase (xynA, B, and C), α-amylase (amyE, amyX), and pectate lyases (pel, pelB; Supplementary Table S8). These enzymes are be used to liberate sugars from complex polysaccharides that are then be assimilated by the strain. Chitinase may also function to inhibit nearby fungi by hydrolyzing their cell walls and inhibiting formation of hyphae (Kobayashi and Ikemoto, 2019; Legein et al., 2020). Lastly, a gene encoding for the quorum quenching enzyme YtnP (lactonase-homolog protein) was also found in the genome. This may degrade quorum sensing autoinducers of competitive strains in the same plant environment (Schneider et al., 2012).
Nitrogen and phosphorous
EA-CB0015 contained genes required to reduce nitrate to ammonia via the dissimilatory nitrate reduction pathway (bteq_M00530, Supplementary Table S7). The strain lacks genes for a nitrogenase enzyme complex, and thus is unable to fix atmospheric dinitrogen. Genes required for metabolism of aspartate/glutamate (bteq00250), arginine/proline (bteq00330), glycine/threonine/serine (bteq00260) were present (Supplementary Table S7), all of which have been detected in the foliage of plants (Morgan and Tukey, 1964; Parangan-Smith and Lindow, 2013).
Under phosphate limiting conditions, many microorganisms can solubilize inorganic sources of phosphate or mineralize organophosphorous compounds (Hanif et al., 2015). Genes encoding for the pho regulon, including alkaline phosphatase, extracellular enzymes to catabolize organophosphorous compounds (PhoA, PhoB, PhoD, GlpQ), phosphate transporters, and the tat secretion system for protein export were indeed present within the genome (Supplementary Table S9; Allenby et al., 2005). Interestingly, phytase (phy) was only present as a pseudongene (Supplementary Table S9), suggesting strain is unable to hydrolyze phytate, a major source of organic phosphate.
Iron
EA-CB0015 encoded genes for the biosynthesis of the siderophore bacillibactin (dhbACEBF), transporters for importing it into the cell (FeuABC-YusV), and the esterase BesA to release the iron in the cytosol (Supplementary Table S10; Miethke et al., 2006). Genes encoding hydoxamate (fhuBCDG), petrobactin/catecholate (fpbOPQ), schizokinen/anthrobactin (yfhAYZ) transporters suggest the strain may scavenge siderophores produced by other species (Supplementary Table S10). Genes for citrate-iron transporter (fecCDEF), high-affinity iron transporter (efeUOB), and heme degradation (hmoAB) suggest these as additional mechanisms for iron acquisition.
Protective mechanisms against oxidative stress and UV
Residing on plant leaves and surfaces, epiphytes are continually challenged by photooxidative stress. In addition to sporulation, biofilm formation, and motility, EA-CB0015 contained genes to protect itself from desiccation, UV light, and oxidative damage (Mols and Abee, 2011). The flavin-dependent photoreceptor (ytvA) activates general stress response mechanisms in the presence of blue light. This includes spore-product photolyase (splB; Supplementary Table S11) to repair thymine dimer adducts produced from UV radiation (Herrou and Crosson, 2011; Vanhaelewyn et al., 2020). Genes for catalase, superoxide dismutase, thioredoxin reductase, hydroperoxide reductase, peroxiredoxin, and antioxidants including bacillithiol, phytoene, and sporulene (Supplementary Table S11), may help EA-CB0015 reduce damage from oxidative stress (Engelmann and Hecker, 1996; Newton et al., 2009; Zuber, 2009; Jeong et al., 2018). Production of extracellular polysaccharides and the siderophore bacillibactin (a UV-B absorbing compound) may further allow EA-CB0015 to avoid damage from UV exposure (Supplementary Table S11).
Biosynthetic pathways for natural products
Epiphytic bacteria scavenge nutrients, influence development of plants, and the composition of local microbial communities through their natural products (Legein et al., 2020). To identify the repertoire of molecules EA-CB0015 may produce, we first analyzed its genome using antiSMASH. We found seven BGCs for known natural products and two of unknown function (Supplementary Table S12). Encoded within the genome were non-ribosomal peptide synthetase (NRPS) BGCs for lipopeptides (plipastatin/fengycin C, surfactin, iturin) and the siderophore bacillibactin. The BGCs for subtilosin A (thiopeptide), sporulation killing factor (SKF; sactipeptide), and bacilysin were also detected (Figure 4). Annotation of the NRPS domains for fengycin C (Villegas-Escobar et al., 2013), suggests the strain actually produces plipastatin. Specifically, the lack of an epimerization domain in module 3 of fenB suggests incorporation of L-Tyr instead of D-Tyr. Moreover, the domain annotation of module 9 predicts epimerization, suggesting D-Thr instead of a L-Thr in the final peptide natural product (Hussein, 2019). The neighborhoods from EA-CB0015 were all conserved to reference BGCs for these natural products (Supplementary Figure S4).
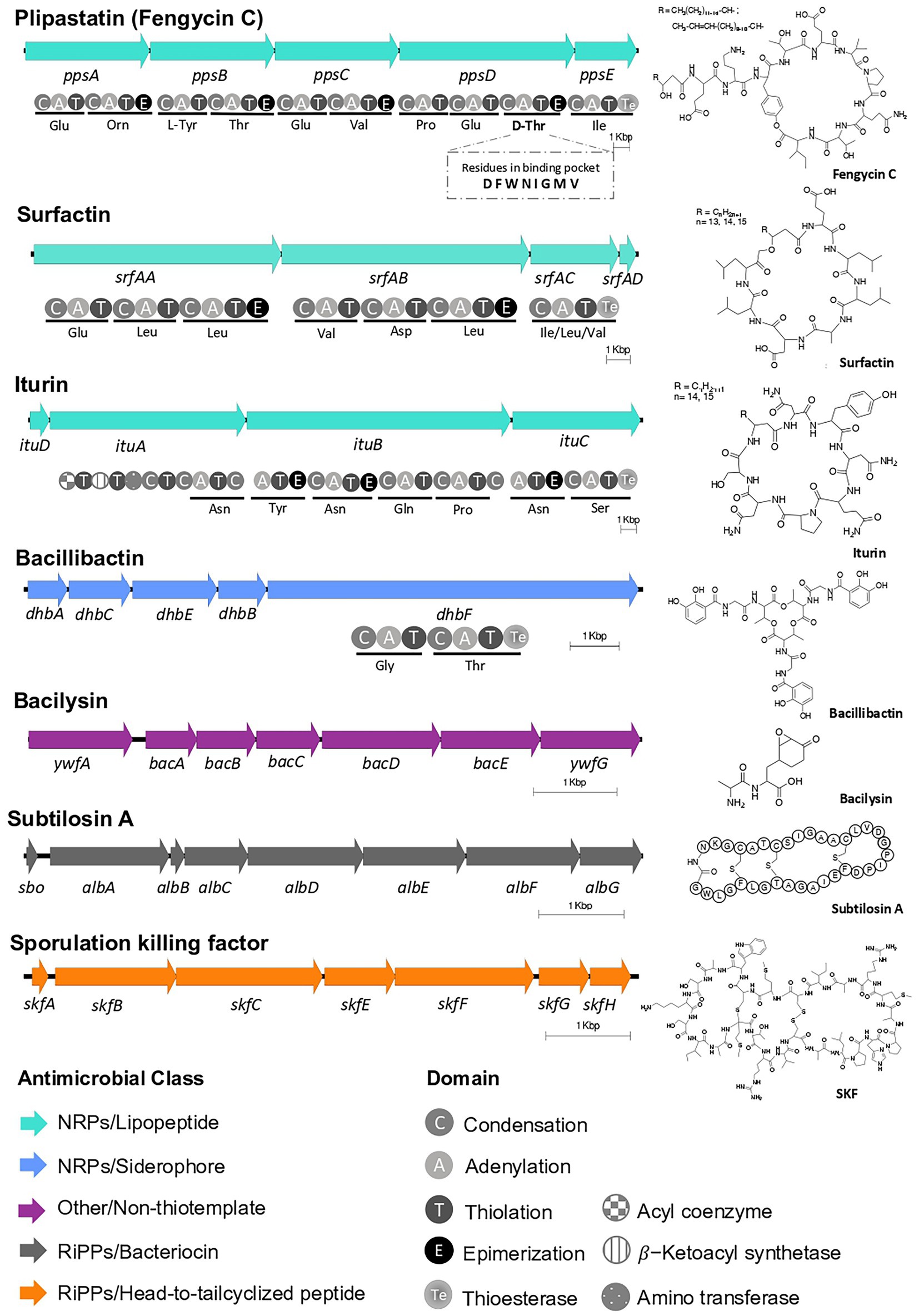
Figure 4. BGCs of known natural products within Bacillus tequilensis EA-CB0015. Predicted domains for NRPSs and amino acid specificity are shown. The third adenylation domain in the putative PpsD protein is predicted to load threonine (fengycin C) instead of tyrosine (plipastatin B). The BGCs are located on the following positions on the chromosome: SKF (209,791–215,884); surfactin (359,914–386,063); iturin* (1,919,24–1,955,499); plipastatin (fengycin C)* (1,969,256–2,007,030); bacillibactin* (3,075,812–3,087,611); subtilosin A* (3,667,130–3,674,071); bacilysin* (3,701,710–3,708,994). (*Encoded on negative strand).
Two genomic regions were annotated to encode for potential terpene and polyketide compounds. The first region (2,107,965 to 2,129,124 bp) contained a gene annotated as squalene-hopene cyclase, but its neighborhood lacked commonly associated genes for condensation of farnesyl diphosphate to squalene (SQase, or hpnD, hpnC, hpnE; Supplementary Table S13; van der Donk, 2015). The second region (2,174,022 and 2,213,530 bp) contained a putative type III polyketide synthase (chalcone synthase; BpsA) and an isoprenylcysteine carboxyl methyltraferase (BpsB), suggesting a role for biosynthesis of aliphatic polyketides, such as triketide pyrones, tetraketide pyrones and alkylresorcinols (Supplementary Table S14; Nakano et al., 2009).
Putative pathways for other natural products were identified by KEGG and manual annotation. EA-CB0015 encoded genes for the antibiotic kanosamine, which is biosynthesized in three steps from glucose-6-phosphate (enzymes NtdC, NtdA, NtdB; KEGG accession bteq00998, Supplementary Table S8). Biosynthesis of the plant hormone IAA included genes for tryptophan aminotransferase (patB), idole-3-pyruvate decarboxylase (yclC), and indole-3-acetaldehyde dehydrogenase (dhaS). These would produce indole 3-pyruvic acid pathway (IPyA) the predominant precursor to IAA (Shao et al., 2015). Genes related to the tryptamine-TAM pathway (bsdC, flavin monamine oxidase, and dhaS), known as an alternative pathway for the synthesis of IAA, were found (Shao et al., 2015). YhcX, predicted to act as a nitrilase in the last step of the indole 3-acetonitrile (IAN) pathway, was also present (Supplementary Table S15).
Genetic exchange
Leaf surfaces are proposed as hot spots for lateral gene transfer and important breeding grounds for microbial diversity (Lindow and Leveau, 2002). Thus, we sought to understand both the degree to which EA-CB0015 may have been affected by gene transfer events and mechanisms it may have to maintain genome integrity.
EA-CB0015 encoded genes needed to take up DNA by genetic competence (Supplementary Table S5), but did not contain plasmids nor any complete integrative or conjugative transposons (Auchtung et al., 2016). However, some pseudogenes (immA, immR, phrI) or integrases (int) for excision of ICEBs were present in the genome (Supplementary Table S16). Four prophage regions were predicted, suggesting EA-CB0015 was susceptible to bacteriophages (Figure 5; Supplementary Figure S5). PHASTER analysis classified three as incomplete (Bacillus phi4J1, Brevibacillus Jimmer2, and Staphylococcus SPβ-like) and one as questionable (Bacillus SPβ). While insertions and recombination from prophages may disrupt important genes, they may also introduce phage resistance or prototrophy to improve the competitive fitness (Kohm and Hertel, 2021).
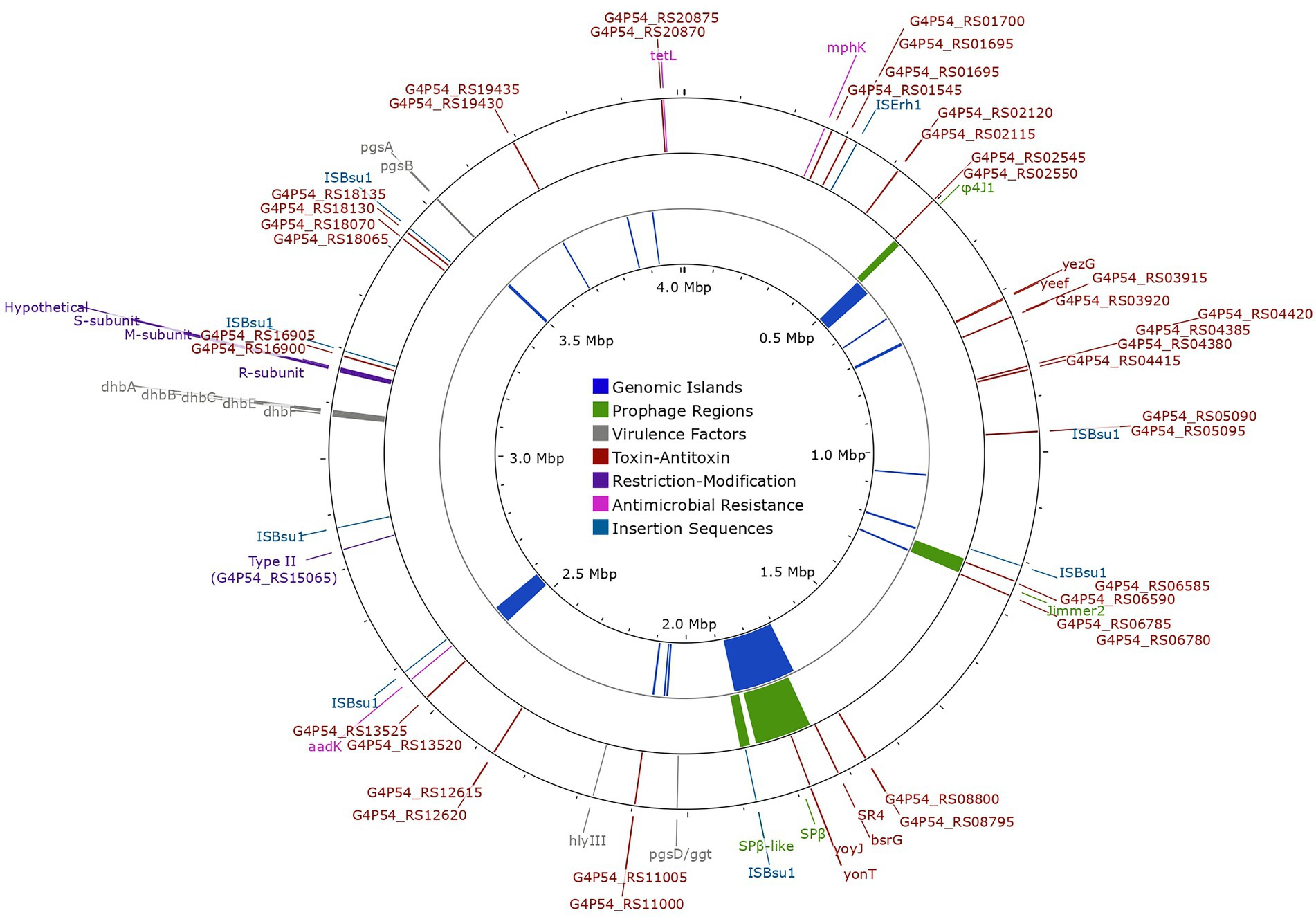
Figure 5. Chromosomal map of Bacillus tequilensis EA-CB0015 showing genetic exchange features, antimicrobial resistance genes, restriction modification systems, and virulence factors. The outer circle shows the location of insertion sequences (light-blue), antimicrobial resistance genes (pink), restriction modification systems (purple), toxin-antitoxin systems (brown) and virulence factors (gray). Intact (high confidence) prophage regions are shown in the second circle (green). The inner circle shows the location of genomic islands (dark-blue).
Other hallmarks of horizonal gene transfer include GEIs and transposable elements. GEIs encode cluster of genes for specialized functions (pathogenicity, symbiosis, metabolism, resistance, fitness) presumed to be of foreign origin (Juhas et al., 2009). Interestingly, GEIs accounted for 10% of the total genome, with many phage-related genes overlapping the same regions (Figure 5; Supplementary Figures S5, S6). Fourteen ISs (transposable elements) were present in the genome (Supplementary Table S17). However, ISs belonging to IS1182 and IS1595 families were pseudogenes and only those belonging to the IS3 family were complete. The latter was the most abundant, with 8 copies of ISBsuI and 1 copy of ISErh (Siguier et al., 2006; Figure 5). These may offer a selective advantage by accelerating genome rearrangement, introducing virulence factors, or resistance genes to antibiotics (Lindow and Leveau, 2002). Genes for putative virulence factors including hemolysin III (hlyIII), capsular polyglutamate and bacillibactin were encoded outside of mobile elements (Figure 5; Supplementary Table S18). Resistance genes for streptomycin (aadK), spiramycin and telithromycin (mphK), and tetracycline (tetL; Figure 5; Supplementary Tables S18, S19) were also detected. The streptomycin resistance gene was encoded within a genomic island.
Both type I and type II RM systems were encoded in the genome of EA-CB0015. The type I system contained genes for the HsdR endonuclease (R), specificity subunit (S), and DNA methyltransferase (M; Figure 5; Supplementary Figure S7). While genes for the endonuclease and methyltransferase of the type I system were highly conserved (>85% sequence identity), the specificity subunit (G4P54_RS16760) exhibited significantly lower sequence identity (33%–40%; Supplementary Figure S8). This suggests variability in the recognition site where the restriction endonuclease cleaves DNA. Additionally, a type II RM system with a site-specific DNA methyltransferase (locus tag G4P54_RS15065) was found.
EA-CB0015 contained 22 TA systems (Figure 5; Supplementary Figure S9; Supplementary Table S20). These included two type I TA systems (bsrG/SR4 and yonT-yoyJ/SR6 located in SPβ region) and three type II TA systems (ndoA/ndoB, spoIISA/spoIISB/spoIISC and yeeF/yezG) which are also in strain 168. TA systems may provide fitness advantages when the strain experiences stress from pH changes, oxygen deficiency, or iron limitation (Brantl and Müller, 2019). Lastly, no RNA-based defense systems were found using CRISPRCasFinder (Couvin et al., 2018).
Natural product BGCs, prophages and restriction modification systems among Bacillus spp.
To understand how EA-CB0015 compares with these closely related bacteria and the presence of any distinguishing trends, we cataloged natural product biosynthesis, prophage, and RM across type strains within the B. subtilis species complex, B. cereus species complex and B. coagulans (Figure 2). In general, strains that are non-pathogenic to humans (B. subtilis, B. tequilensis, B. mojavensis, B. atrophaeus, B. velezensis, B. amyloliquefaciens, B. licheniformis and B. pumilus) encoded a more natural product BGCs. Among the 22 different natural product BGCs we identified, the largest number (7–9) were associated with B. subtilis, B. velezensis, B. amyloliquefacies, and B. tequilensis (Figure 2B). These was consistent with known biological control agents produced by these species (Chen et al., 2009; Gutierrez-Monsalve et al., 2015; Pandin et al., 2018; Samaras et al., 2021). Surfactin, bacilysin, bacillibactin, and bacillaene were the most prevalent BGCs. Except for the antifungal phosphonopeptide rhizocticin (Borisova et al., 2010), EA-CB0015 and BAA-819 share the same composition of natural product BGCs. The most common BGC in pathogenic bacilli was petrobactin. None of these 22 BGCs were present in B. coagulans.
Prophages account for substantial genetic variation and confer phage resistance (Casjens, 2003; Fortier and Sekulovic, 2013; Kohm and Hertel, 2021). Across all taxa, the number of candidate prophage regions and prophage CDSs was similar within the B. subtilis species complex (4.68 regions and 217.74 CDSs) and B. cereus group (5.15 regions and 196.95 CDSs; Figure 2C; Supplementary Figure S10). Intact prophages included members of the Siphoviridae, Myoviridae and Tectiviridae families (Supplementary Figure S11). Twelve phages were unique to B. subtilis species complex, 8 unique to the B. cereus species complex, and 3 were shared between both groups (Supplementary Figures S10, S12). Altogether, these results indicate phages are evolutionary conserved within species complexes.
As natural selection is one of the most important evolutionary processes, RM systems serve as an important defense against the introduction of foreign DNA (Bourniquel and Bickle, 2002). Type II RM systems were the most common among both groups, present in 68 and 81% of the strains from B. subtilis and B. cereus complexes, respectively. Other types of RM systems were also widely distributed but less abundant than type II systems (Figure 2D), but no differential patterns were observed between these two groups of strains.
Discussion
Successful biocontrol agents have been suggested to require distinct mechanisms that confer survival to its habitat and contribute to the health of its host (Legein et al., 2020). In sequencing the genome of B. tequilensis EA-CB0015, we uncovered the genetic basis for several physiological adaptations underlying its survival in the phyllosphere including biofilm formation, motility, competence, protection from oxidative stress, and sporulation. Spores of EA-CB0015 are predicted to lack legionaminic acid, and their reduced charge may favor adherence to the naturally hydrophobic surface of leaves. Genes for chemotaxis, and swarming suggest colonization of plant surfaces may be mediated through motility toward optimal growth conditions including microenvironments rich in nutrients. EA-CB0015 may also leverage acquisition of nutrients through its biosynthesis of IAA and surfactin. Production of IAA induces physiological changes in plants including loosening of plant cell walls and the release of nutrients (Vanderhoef and Dute, 1981). Surfactin could improve the wettability of leaves and thus facilitate adherence (Lindow and Brandl, 2003). Both may increase access of nutrients that could then be assimilated by EA-CB0015 through its diverse pathways for carbon (sugars, organic acids, formaldehyde) nitrogen (dissimilatory nitrate reduction, amino acid catabolism), and phosphorous assimilation.
Microbial natural products contribute the health of host plants by modulating plant hormone concentrations, inducing systemic resistance, and inhibiting both growth and intercellular communication of pathogens (Legein et al., 2020). At least seven natural product BGCs were present within the genome of EA-CB0015. In addition to previously characterized lipopeptides surfactin, iturin A and fengycin C (Villegas-Escobar et al., 2013; Mosquera et al., 2014), four additional natural product BGCs for bacillibactin, bacilysin, subtilosin A, and the sporulation killing factor were identified. These later compounds could contribute to the biological control activities of this strain. In addition to iron scavenging and biofilm formation, bacillibactin has been associated with alternative functions including transport of other metals, sequestration of toxic metals, and protection from oxidative stress (Rizzi et al., 2019; Legein et al., 2020). Production of bacilysin by B. velezensis FZB42 was found to regulate the expression of several virulence genes in X. oryzae (Özcengiz and Öğülür, 2015; Wu et al., 2015), while subtilosin A was inhibitory against a variety of gram-positive and-negative bacteria (Shelburne et al., 2007). The cannibalistic peptide, SKF is known to permeabilize of cytoplasmic membranes of E. coli cells (Nonejuie et al., 2016) and inhibit the growth of plant pathogens such as X. oryzae (Lin et al., 2001). Determining the production of these compounds by EA-CB0015 on plants will provide valuable insight into their functional role in biocontrol and if beneficial synergistic effects may exist.
We identified significant variations between related Bacilli that arose from bacteriophage activity. Long-term associations between temperate phages may provide benefit to bacteria through resistance against infection and introduction of accessory genes for metabolism, stress tolerance, and antibiotic resistance. These may facilitate survival of EA-CB0015 when exposed to natural products produced from other epiphytic microbes and antibiotics commonly applied to commercial crops (Schnabel and Jones, 1999; Feiner et al., 2015; Howard-Varona et al., 2018; Ramisetty and Sudhakari, 2019). Moreover, mobile genetic elements in EA-CB0015 and related Bacilli may accelerate transfer of genes that are advantageous for their survival in the phyllosphere (Lindow and Leveau, 2002). The contribution of phages, mobile elements, and associated TA systems on the competitive fitness of epiphytic Bacillus spp., influencing the phyllosphere microbiome, and effecting the physiology of host plants remain important areas of future investigation.
Data availability statement
The original contributions presented in the study are publicly available. This data can be found at: https://www.ncbi.nlm.nih.gov/nuccore/NZ_CP048852.1.
Author contributions
TC-G, K-SJ, and VV-E: conceptualization and writing the manuscript. TC-G, CG-B, K-SJ, and VV-E: methodology, investigation, and formal analysis. K-SJ and VV-E: supervision and funding acquisition. All authors contributed to the article and approved the submitted version.
Funding
This project was funded with support from Universidad EAFIT (VV-E), Association of Banana Producers of Colombia (AUGURA; VV-E), Colciencias (Convocatoria 617 Doctorados Nacionales for the PhD studies of TC-G), and the National Institutes for Health (GM137135 to K-SJ).
Acknowledgments
This research was made possible by the subscribed Contract Number 166 and 139 with Ministerio de Medio Ambiente y Desarrollo Territorial of Colombia in the categories “Contrato de Acceso a Recursos Genéticos y Productos Derivados para Investigación Científica” and “Contrato de Acceso a Recursos Genéticos y Productos Derivados con Fines Comerciales,” respectively.
Conflict of interest
The authors declare that the research was conducted in the absence of any commercial or financial relationships that could be construed as a potential conflict of interest.
Publisher’s note
All claims expressed in this article are solely those of the authors and do not necessarily represent those of their affiliated organizations, or those of the publisher, the editors and the reviewers. Any product that may be evaluated in this article, or claim that may be made by its manufacturer, is not guaranteed or endorsed by the publisher.
Supplementary material
The Supplementary material for this article can be found online at: https://www.frontiersin.org/articles/10.3389/fmicb.2023.1135487/full#supplementary-material
Footnotes
References
Allenby, N. E. E., O’Connor, N., Prágai, Z., Ward, A. C., Wipat, A., and Harwood, C. R. (2005). Genome-wide transcriptional analysis of the phosphate starvation stimulon of Bacillus subtilis. J. Bacteriol. 187, 8063–8080. doi: 10.1128/JB.187.23.8063-8080.2005
Arnaouteli, S., Bamford, N. C., Stanley-Wall, N. R., and Kovács, Á. T. (2021). Bacillus subtilis biofilm formation and social interactions. Nat. Rev. Microbiol. 19, 600–614. doi: 10.1038/s41579-021-00540-9
Arndt, D., Grant, J. R., Marcu, A., Sajed, T., Pon, A., Liang, Y., et al. (2016). PHASTER: a better, faster version of the PHAST phage search tool. Nucleic Acids Res. 44, W16–W21. doi: 10.1093/nar/gkw387
Arroyave-Toro, J. J., Mosquera, S., and Villegas-Escobar, V. (2017). Biocontrol activity of Bacillus subtilis EA-CB0015 cells and lipopeptides against postharvest fungal pathogens. Biol. Control 114, 195–200. doi: 10.1016/j.biocontrol.2017.08.014
Auchtung, J. M., Aleksanyan, N., Bulku, A., and Berkmen, M. B. (2016). Biology of ICEBs1, an integrative and conjugative element in Bacillus subtilis. Plasmid 86, 14–25. doi: 10.1016/j.plasmid.2016.07.001
Bertelli, C., Laird, M. R., Williams, K. P., Lau, B. Y., Hoad, G., Winsor, G. L., et al. (2017). IslandViewer 4: expanded prediction of genomic islands for larger-scale datasets. Nucleic Acids Res. 45, W30–W35. doi: 10.1093/nar/gkx343
Blin, K., Shaw, S., Steinke, K., Villebro, R., Ziemert, N., Lee, S. Y., et al. (2019). antiSMASH 5.0: updates to the secondary metabolite genome mining pipeline. Nucleic Acids Res. 47, W81–W87. doi: 10.1093/nar/gkz310
Borisova, S. A., Circello, B. T., Zhang, J. K., van der Donk, W. A., and Metcalf, W. W. (2010). Biosynthesis of rhizocticins, antifungal phosphonate oligopeptides produced by Bacillus subtilis ATCC6633. Chem. Biol. 17, 28–37. doi: 10.1016/j.chembiol.2009.11.017
Borowiec, M. L. (2016). AMAS: a fast tool for alignment manipulation and computing of summary statistics. PeerJ 4:e1660. doi: 10.7717/peerj.1660
Bortolaia, V., Kaas, R. S., Ruppe, E., Roberts, M. C., Schwarz, S., Cattoir, V., et al. (2020). ResFinder 4.0 for predictions of phenotypes from genotypes. J. Antimicrob. Chemother. 75, 3491–3500. doi: 10.1093/jac/dkaa345
Bourniquel, A. A., and Bickle, T. A. (2002). Complex restriction enzymes: NTP-driven molecular motors. Biochimie 84, 1047–1059. doi: 10.1016/S0300-9084(02)00020-2
Brantl, S., and Müller, P. (2019). Toxin-antitoxin systems in Bacillus subtilis. Toxins 11:262. doi: 10.3390/toxins11050262
Casjens, S. (2003). Prophages and bacterial genomics: what have we learned so far? Mol. Microbiol. 49, 277–300. doi: 10.1046/j.1365-2958.2003.03580.x
Ceballos, I., Mosquera, S., Angulo, M., Mira, J. J., Argel, L. E., Uribe-Velez, D., et al. (2012). Cultivable bacteria populations associated with leaves of banana and plantain plants and their antagonistic activity against Mycosphaerella fijiensis. Microb. Ecol. 64, 641–653. doi: 10.1007/s00248-012-0052-8
Chaumeil, P.-A., Mussig, A. J., Hugenholtz, P., and Parks, D. H. (2019). GTDB-Tk: a toolkit to classify genomes with the genome taxonomy database. Bioinformatics 36, 1925–1927. doi: 10.1093/bioinformatics/btz848
Chen, X. H., Koumoutsi, A., Scholz, R., Schneider, K., Vater, J., Süssmuth, R., et al. (2009). Genome analysis of bacillus amyloliquefaciens FZB42 reveals its potential for biocontrol of plant pathogens. J. Biotechnol. 140, 27–37. doi: 10.1016/j.jbiotec.2008.10.011
Choi, S. K., Jeong, H., Kloepper, J. W., and Ryu, C. M. (2014). Genome sequence of bacillus amyloliquefaciens GB03, an active ingredient of the first commercial biological control product. Genome Announc. 2, 2–3. doi: 10.1128/genomeA.01092-14
Couvin, D., Bernheim, A., Toffano-Nioche, C., Touchon, M., Michalik, J., Néron, B., et al. (2018). CRISPRCasFinder, an update of CRISRFinder, includes a portable version, enhanced performance and integrates search for Cas proteins. Nucleic Acids Res. 46, W246–W251. doi: 10.1093/nar/gky425
Cuellar-Gaviria, T. Z., González-Jaramillo, L. M., and Villegas-Escobar, V. (2021). Role of bacillus tequilensis EA-CB0015 cells and lipopeptides in the biological control of black Sigatoka disease. Biol. Control 155:104523. doi: 10.1016/j.biocontrol.2020.104523
Darriba, D., Posada, D., Kozlov, A. M., Stamatakis, A., Morel, B., and Flouri, T. (2020). ModelTest-NG: a new and scalable tool for the selection of DNA and protein evolutionary models. Mol. Biol. Evol. 37, 291–294. doi: 10.1093/molbev/msz189
Dartois, V., Liu, J., and Hoch, J. A. (1997). Alterations in the flow of one-carbon units affect KinB-dependent sporulation in Bacillus subtilis. Mol. Microbiol. 25, 39–51. doi: 10.1046/j.1365-2958.1997.4491805.x
Davis, J. J., Wattam, A. R., Aziz, R. K., Brettin, T., Butler, R., Butler, R. M., et al. (2020). The PATRIC bioinformatics resource center: expanding data and analysis capabilities. Nucleic Acids Res. 48, D606–D612. doi: 10.1093/nar/gkz943
Denham, E. L., Piersma, S., Rinket, M., Reilman, E., de Goffau, M. C., and van Dijl, J. M. (2019). Differential expression of a prophage-encoded glycocin and its immunity protein suggests a mutualistic strategy of a phage and its host. Sci. Rep. 9, 2845–2812. doi: 10.1038/s41598-019-39169-3
Dubois, T., Krzewinski, F., Yamakawa, N., Lemy, C., Hamiot, A., Brunet, L., et al. (2020). The sps genes encode an original legionaminic acid pathway required for crust assembly in bacillus subtilis. MBio 11, 1–17. doi: 10.1128/mBio.01153-20
Eddy, S. R. (2011). Accelerated profile HMM searches. PLoS Comput. Biol. 7:e1002195. doi: 10.1371/journal.pcbi.1002195
Engelmann, S., and Hecker, M. (1996). Impaired oxidative stress resistance of Bacillus subtilis sigB mutants and the role of katA and katE. FEMS Microbiol. Lett. 145, 63–69. doi: 10.1016/0378-1097(96)00388-6
Feiner, R., Argov, T., Rabinovich, L., Sigal, N., Borovok, I., and Herskovits, A. A. (2015). A new perspective on lysogeny: Prophages as active regulatory switches of bacteria. Nat. Rev. Microbiol. 13, 641–650. doi: 10.1038/nrmicro3527
Fiala, V., Glad, C., Martin, M., Jolivet, E., and Derridj, S. (1990). Occurrence of soluble carbohydrates on the phylloplane of maize (Zea mays L.): variations in relation to leaf heterogeneity and position on the plant. New Phytol. 115, 609–615. doi: 10.1111/j.1469-8137.1990.tb00492.x
Fortier, L., and Sekulovic, O. (2013). Importance of prophages to evolution and virulence of bacterial pathogens. Virulence 4, 354–365. doi: 10.4161/viru.24498
Fritze, D. (2004). Taxonomy of the genus bacillus and related genera: the aerobic endospore-forming bacteria. Phytopathology 94, 1245–1248. doi: 10.1094/PHYTO.2004.94.11.1245
García-Botero, C., Cuellar-Gaviria, T. Z., and Villegas-Escobar, V. (2021). Supplementary code for some figures of the paper about B. tequilensis genome analysis (version 0.0.3). Available at: https://doi.org/10.5281/zenodo.5225119.
Garti-levi, S., Eswara, A., Smith, Y., Fujita, M., and Ben-Yehuda, S. (2013). Novel modulators controlling entry into sporulation in Bacillus subtilis. J. Bacteriol. 195, 1475–1483. doi: 10.1128/JB.02160-12
Gerhardt, P., Murray, R. G., Wood, W., and Krieg, N. (1994). Methods for general and molecular bacteriology. Washington DC: ASM Press.
Ghelardi, E., Salvetti, S., Ceragioli, M., Gueye, S. A., Celandroni, F., and Senesi, S. (2012). Contribution of Surfactin and SwrA to Flagellin expression, swimming, and surface motility in Bacillus subtilis. Appl. Environ. Microbiol. 78, 6540–6544. doi: 10.1128/AEM.01341-12
Gilchrist, C. L. M., Booth, T. J., van Wersch, B., van Grieken, L., Medema, M. H., and Chooi, Y.-H. (2021). Cblaster: a remote search tool for rapid identification and visualization of homologous gene clusters. Bioinforma. Adv. 1, 1–10. doi: 10.1093/bioadv/vbab016
González-Pastor, J. E. (2017). “Multicellularity and social behaviour in Bacillus subtilis” in Bacillus cellular and molecular biology. ed. P. L. Graumann (Marburg: Caister Academic Press), 367–394.
González-Pastor, J. E., Hobbs, E. C., and Losick, R. (2003). Cannibalism by sporulating bacteria. Science 301, 510–513. doi: 10.1126/science.1086462
Grant, J. R., and Stothard, P. (2008). The CGView server: a comparative genomics tool for circular genomes. Nucleic Acids Res. 36, W181–W184. doi: 10.1093/nar/gkn179
Gutierrez-Monsalve, J. A., Mosquera, S., González-Jaramillo, L. M., Mira, J. J., and Villegas-Escobar, V. (2015). Effective control of black Sigatoka disease using a microbial fungicide based on Bacillus subtilis EA-CB0015 culture. Biol. Control 87, 39–46. doi: 10.1016/j.biocontrol.2015.04.012
Haft, D. H., DiCuccio, M., Badretdin, A., Brover, V., Chetvernin, V., O’Neill, K., et al. (2018). RefSeq: An update on prokaryotic genome annotation and curation. Nucleic Acids Res. 46, D851–D860. doi: 10.1093/nar/gkx1068
Hanif, M. K., Hameed, S., Imran, A., Naqqash, T., Shahid, M., and Van Elsas, J. D. (2015). Isolation and characterization of a β-propeller gene containing phosphobacterium Bacillus subtilis strain KPS-11 for growth promotion of potato (Solanum tuberosum L.). Front. Microbiol. 6, 1–12. doi: 10.3389/fmicb.2015.00583
Harwood, C. R., Mouillon, J. M., Pohl, S., and Arnau, J. (2018). Secondary metabolite production and the safety of industrially important members of the Bacillus subtilis group. FEMS Microbiol. Rev. 42, 721–738. doi: 10.1093/femsre/fuy028
Heimbrook, M. E., Wang, W. L. L., and Campbell, G. (1989). Staining bacterial flagella easily. J. Clin. Microbiol. 27, 2612–2615. doi: 10.1128/jcm.27.11.2612-2615.1989
Herrou, J., and Crosson, S. (2011). Function, structure and mechanism of bacterial photosensory LOV proteins. Nat. Rev. Microbiol. 9, 713–723. doi: 10.1038/nrmicro2622
Howard-Varona, C., Hargreaves, K. R., Solonenko, N. E., Markillie, L. M., White, R. A., Brewer, H. M., et al. (2018). Multiple mechanisms drive phage infection efficiency in nearly identical hosts. ISME J. 12, 1605–1618. doi: 10.1038/s41396-018-0099-8
Hubert, B. (2022). SkewDB, a comprehensive database of GC and 10 other skews for over 30,000 chromosomes and plasmids. Sci. Data 9, 92–99. doi: 10.1038/s41597-022-01179-8
Hussein, W. (2019). Fengycin or plipastatin? A confusing question in bacilli. Biotechnologia 100, 47–55. doi: 10.5114/bta.2019.83211
Jeong, S. W., Kang, C. K., and Choi, Y. J. (2018). Metabolic engineering of deinococcus radiodurans for the production of phytoene. J. Microbiol. Biotechnol. 28, 1691–1699. doi: 10.4014/jmb.1808.08019
Johnson, M., Zaretskaya, I., Raytselis, Y., Merezhuk, Y., McGinnis, S., and Madden, T. L. (2008). NCBI BLAST: a better web interface. Nucleic Acids Res. 36, W5–W9. doi: 10.1093/nar/gkn201
Juhas, M., Van Der Meer, J. R., Gaillard, M., Harding, R. M., Hood, D. W., and Crook, D. W. (2009). Genomic islands: tools of bacterial horizontal gene transfer and evolution. FEMS Microbiol. Rev. 33, 376–393. doi: 10.1111/j.1574-6976.2008.00136.x
Kalamara, M., Spacapan, M., Mandic-Mulec, I., and Stanley-Wall, N. R. (2018). Social behaviours by Bacillus subtilis: quorum sensing, kin discrimination and beyond. Mol. Microbiol. 110, 863–878. doi: 10.1111/mmi.14127
Kanehisa, M., Sato, Y., and Morishima, K. (2016). BlastKOALA and GhostKOALA: KEGG tools for functional characterization of genome and metagenome sequences. J. Mol. Biol. 428, 726–731. doi: 10.1016/j.jmb.2015.11.006
Katoh, K. (2002). MAFFT: a novel method for rapid multiple sequence alignment based on fast Fourier transform. Nucleic Acids Res. 30, 3059–3066. doi: 10.1093/nar/gkf436
Kearns, D. B., and Losick, R. (2003). Swarming motility in undomesticated Bacillus subtilis. Mol. Microbiol. 49, 581–590. doi: 10.1046/j.1365-2958.2003.03584.x
Kobayashi, K., and Ikemoto, Y. (2019). Biofilm-associated toxin and extracellular protease cooperatively suppress competitors in Bacillus subtilis biofilms. PLoS Genet. 15, e1008232–e1008229. doi: 10.1371/journal.pgen.1008232
Kohm, K., and Hertel, R. (2021). The life cycle of SPβ and related phages. Arch. Virol. 166, 2119–2130. doi: 10.1007/s00705-021-05116-9
Kutschera, U. (2007). Plant-associated methylobacteria as co-evolved phytosymbionts: a hypothesis. Plant Signal. Behav. 2, 74–78. doi: 10.4161/psb.2.2.4073
Kwon, H. T., Lee, Y., Kim, J., Balaraju, K., Kim, H. T., and Jeon, Y. (2022). Identification and characterization of bacillus tequilensis GYUN-300: an antagonistic bacterium against red pepper anthracnose caused by Colletotrichum acutatum in Korea. Front. Microbiol. 13, 1–18. doi: 10.3389/fmicb.2022.826827
Legein, M., Smets, W., Vandenheuvel, D., Eilers, T., Muyshondt, B., Prinsen, E., et al. (2020). Modes of action of microbial biocontrol in the phyllosphere. Front. Microbiol. 11:1619. doi: 10.3389/fmicb.2020.01619
Letunic, I., and Bork, P. (2019). Interactive tree of life (iTOL) v4: recent updates and new developments. Nucleic Acids Res. 47, W256–W259. doi: 10.1093/nar/gkz239
Leveau, J. H. J., and Lindow, S. E. (2001). Appetite of an epiphyte: quantitative monitoring of bacterial sugar consumption in the phyllosphere. Proc. Natl. Acad. Sci. U. S. A. 98, 3446–3453. doi: 10.1073/pnas.061629598
Lin, D., Qu, L. J., Gu, H., and Chen, Z. (2001). A 3.1-kb genomic fragment of Bacillus subtilis encodes the protein inhibiting growth of Xanthomonas oryzae pv. Oryzae. J. Appl. Microbiol. 91, 1044–1050. doi: 10.1046/j.1365-2672.2001.01475.x
Lindow, S. E., and Brandl, M. T. (2003). Microbiology of the phyllosphere. Appl. Environ. Microbiol. 69, 1875–1883. doi: 10.1128/AEM.69.4.1875
Lindow, S. E., and Leveau, J. H. J. (2002). Phyllosphere microbiology. Curr. Opin. Biotechnol. 13, 238–243. doi: 10.1016/S0958-1669(02)00313-0
Liu, M., Li, X., Xie, Y., Bi, D., Sun, J., Li, J., et al. (2019). ICEberg 2.0: An updated database of bacterial integrative and conjugative elements. Nucleic Acids Res. 47, D660–D665. doi: 10.1093/nar/gky1123
Liu, B., Zheng, D., Jin, Q., Chen, L., and Yang, J. (2019). VFDB 2019: a comparative pathogenomic platform with an interactive web interface. Nucleic Acids Res. 47, D687–D692. doi: 10.1093/nar/gky1080
López, D., Fischbach, M. A., Chu, F., Losick, R., and Kolter, R. (2009). Structurally diverse natural products that cause potassium leakage trigger multicellularity in Bacillus subtilis. Proc. Natl. Acad. Sci. U. S. A. 106, 280–285. doi: 10.1073/pnas.0810940106
Ma, L., Wang, W. Q., Shi, R., Zhang, X. M., Li, X., Yang, Y. S., et al. (2021). Effects of organic acids on the chemotaxis profiles and biocontrol traits of antagonistic bacterial endophytes against root-rot disease in Panax notoginseng. Antonie van Leeuwenhoek. Int. J. Gen. Mol. Microbiol. 114, 1771–1789. doi: 10.1007/s10482-021-01636-1
Miethke, M., Klotz, O., Linne, U., May, J. J., Beckering, C. L., and Marahiel, M. A. (2006). Ferri-bacillibactin uptake and hydrolysis in Bacillus subtilis. Mol. Microbiol. 61, 1413–1427. doi: 10.1111/j.1365-2958.2006.05321.x
Mols, M., and Abee, T. (2011). Primary and secondary oxidative stress in bacillus. Environ. Microbiol. 13, 1387–1394. doi: 10.1111/j.1462-2920.2011.02433.x
Morgan, J. V., and Tukey, H. B. (1964). Characterization of leachate from plant foliage. Plant Physiol. 39, 590–593. doi: 10.1104/pp.39.4.590
Mosquera, S., González-Jaramillo, L. M., Orduzb, S., and Villegas-Escobar, V. (2014). Multiple response optimization of Bacillus subtilis EA-CB0015 culture and identification of antifungal metabolites. Biocatal. Agric. Biotechnol. 3, 378–385. doi: 10.1016/j.bcab.2014.09.004i
Mukherjee, S., and Kearns, D. B. (2014). The structure and regulation of flagella in Bacillus subtilis. Annu. Rev. Genet. 48, 319–340. doi: 10.1146/annurev-genet-120213-092406
Nakano, C., Ozawa, H., Akanuma, G., Funa, N., and Horinouchi, S. (2009). Biosynthesis of aliphatic polyketides by type III polyketide synthase and methyltransferase in Bacillus subtilis. J. Bacteriol. 191, 4916–4923. doi: 10.1128/JB.00407-09
Nakano, M. M., Xia, L., and Zuber, P. (1991). Transcription initiation region of the srfA operon, which is controlled by the comP-comA signal transduction system in Bacillus subtilis. J. Bacteriol. 173, 5487–5493. doi: 10.1128/jb.173.17.5487-5493.1991
Newton, G. L., Rawat, M., La Clair, J. J., Jothivasan, V. K., Budiarto, T., Hamilton, C. J., et al. (2009). Bacillithiol is an antioxidant thiol produced in bacilli. Nat. Chem. Biol. 5, 625–627. doi: 10.1038/nchembio.189
Ngalimat, M. S., Yahaya, R. S. R., Baharudin, M. M. A. A., Yaminudin, S. M., Karim, M., Ahmad, S. A., et al. (2021). A review on the biotechnological applications of the operational group bacillus amyloliquefaciens. Microorganisms 9, 1–18. doi: 10.3390/microorganisms9030614
Nguyen, L. T., Schmidt, H. A., Von Haeseler, A., and Minh, B. Q. (2015). IQ-TREE: a fast and effective stochastic algorithm for estimating maximum-likelihood phylogenies. Mol. Biol. Evol. 32, 268–274. doi: 10.1093/molbev/msu300
Nicholson, W. L. (2002). Roles of bacillus endospores in the environment. Cell. Mol. Life Sci. 59, 410–416. doi: 10.1007/s00018-002-8433-7
Noar, R. D., Thomas, E., and Daub, M. E. (2022). Genetic characteristics and metabolic interactions between Pseudocercospora fijiensis and banana: progress toward controlling black Sigatoka. Plan. Theory 11:948. doi: 10.3390/plants11070948
Nonejuie, P., Trial, R. M., Newton, G. L., Lamsa, A., Ranmali Perera, V., Aguilar, J., et al. (2016). Application of bacterial cytological profiling to crude natural product extracts reveals the antibacterial arsenal of Bacillus subtilis. J. Antibiot. 69, 353–361. doi: 10.1038/ja.2015.116
O’Toole, G., Kaplan, H. B., and Kolter, R. (2000). Biofilm formation as microbial development. Annu. Rev. Microbiol. 54, 49–79. doi: 10.1146/annurev.micro.54.1.49
Oslizlo, A., Stefanic, P., Dogsa, I., and Mandic-Mulec, I. (2014). Private link between signal and response in Bacillus subtilis quorum sensing. Proc. Natl. Acad. Sci. U. S. A. 111, 1586–1591. doi: 10.1073/pnas.1316283111
Özcengiz, G., and Öğülür, İ. (2015). Biochemistry, genetics and regulation of bacilysin biosynthesis and its significance more than an antibiotic. N. Biotechnol. 32, 612–619. doi: 10.1016/j.nbt.2015.01.006
Pandin, C., Le Coq, D., Deschamps, J., Védie, R., Rousseau, T., Aymerich, S., et al. (2018). Complete genome sequence of bacillus velezensis QST713: a biocontrol agent that protects Agaricus bisporus crops against the green mould disease. J. Biotechnol. 278, 10–19. doi: 10.1016/j.jbiotec.2018.04.014
Parangan-Smith, A., and Lindow, S. (2013). Contribution of nitrate assimilation to the fitness of pseudomonas syringae pv. Syringae B728a on plants. Appl. Environ. Microbiol. 79, 678–687. doi: 10.1128/AEM.02511-12
Poveda, J., and González-Andrés, F. (2021). Bacillus as a source of phytohormones for use in agriculture. Appl. Microbiol. Biotechnol. 105, 8629–8645. doi: 10.1007/s00253-021-11492-8
Rambaut, A., Drummond, A. J., Xie, D., Baele, G., and Suchard, M. A. (2018). Posterior summarization in Bayesian phylogenetics using tracer 1.7. Syst. Biol. 67, 901–904. doi: 10.1093/sysbio/syy032
Ramisetty, B. C. M., and Sudhakari, P. A. (2019). Bacterial grounded prophages: hotspots for genetic renovation and innovation. Front. Genet. 10, 1–17. doi: 10.3389/fgene.2019.00065
Richter, M., and Rosselló-Móra, R. (2009). Shifting the genomic gold standard for the prokaryotic species definition. Proc. Natl. Acad. Sci. U. S. A. 106, 19126–19131. doi: 10.1073/pnas.0906412106
Rizzi, A., Roy, S., Bellenger, J. P., and Beauregard, P. B. (2019). Iron homeostasis in Bacillus subtilis requires siderophore production and biofilm formation. Appl. Environ. Microbiol. 85, 1–10. doi: 10.1128/AEM.02439-18
Roberts, R. J., Vincze, T., Posfai, J., and Macelis, D. (2015). REBASE-a database for DNA restriction and modification: enzymes, genes and genomes. Nucleic Acids Res. 43, D298–D299. doi: 10.1093/nar/gku1046
Ronquist, F., Teslenko, M., Van Der Mark, P., Ayres, D. L., Darling, A., Höhna, S., et al. (2012). MrBayes 3.2: efficient Bayesian phylogenetic inference and model choice across a large model space. Syst. Biol. 61, 539–542. doi: 10.1093/sysbio/sys029
Rooney, A. P., Price, N. P. J., Ehrhardt, C., Sewzey, J. L., and Bannan, J. D. (2009). Phylogeny and molecular taxonomy of the Bacillus subtilis species complex and description of Bacillus subtilis subsp. inaquosorum subsp. nov. Int. J. Syst. Evol. Microbiol. 59, 2429–2436. doi: 10.1099/ijs.0.009126-0
Rudrappa, T., Czymmek, K. J., Paré, P. W., and Bais, H. P. (2008). Root-secreted malic acid recruits beneficial soil bacteria. Plant Physiol. 148, 1547–1556. doi: 10.1104/pp.108.127613
Samaras, A., Roumeliotis, E., Ntasiou, P., and Karaoglanidis, G. (2021). Bacillus subtilis MBI600 promotes growth of tomato plants and induces systemic resistance contributing to the control of soilborne pathogens. Plan. Theory 10:1113. doi: 10.3390/plants10061113
Schnabel, E. L., and Jones, A. L. (1999). Distribution of tetracycline resistance genes and transposons among phylloplane bacteria in Michigan apple orchards. Appl. Environ. Microbiol. 65, 4898–4907. doi: 10.1128/aem.65.11.4898-4907.1999
Schneider, J., Yepes, A., Garcia-Betancur, J. C., Westedt, I., Mielich, B., and López, D. (2012). Streptomycin-induced expression in Bacillus subtilis of YtnP, a lactonase-homologous protein that inhibits development and streptomycin production in Streptomyces griseus. Appl. Environ. Microbiol. 78, 599–603. doi: 10.1128/AEM.06992-11
Seemann, T. (2014). Prokka: rapid prokaryotic genome annotation. Bioinformatics 30, 2068–2069. doi: 10.1093/bioinformatics/btu153
Setlow, P., Wang, S., and Li, Y. Q. (2017). Germination of spores of the orders Bacillales and Clostridiales. Annu. Rev. Microbiol. 71, 459–477. doi: 10.1146/annurev-micro-090816-093558
Shao, J., Li, S., Zhang, N., Cui, X., Zhou, X., Zhang, G., et al. (2015). Analysis and cloning of the synthetic pathway of the phytohormone indole-3-acetic acid in the plant-beneficial bacillus amyloliquefaciens SQR9. Microb. Cell Fact. 14, 130–113. doi: 10.1186/s12934-015-0323-4
Shelburne, C. E., An, F. Y., Dholpe, V., Ramamoorthy, A., Lopatin, D. E., and Lantz, M. S. (2007). The spectrum of antimicrobial activity of the bacteriocin subtilosin a. J. Antimicrob. Chemother. 59, 297–300. doi: 10.1093/jac/dkl495
Siguier, P., Perochon, J., Lestrade, L., Mahillon, J., and Chandler, M. (2006). ISfinder: the reference Centre for bacterial insertion sequences. Nucleic Acids Res. 34, D32–D36. doi: 10.1093/nar/gkj014
Stefanic, P., and Mandic-Mulec, I. (2009). Social interactions and distribution of Bacillus subtilis pherotypes at microscale. J. Bacteriol. 191, 1756–1764. doi: 10.1128/JB.01290-08
Stein, T. (2005). Bacillus subtilis antibiotics: structures, syntheses and specific functions. Mol. Microbiol. 56, 845–857. doi: 10.1111/j.1365-2958.2005.04587.x
Sukumaran, J., and Holder, M. T. (2010). DendroPy: a python library for phylogenetic computing. Bioinformatics 26, 1569–1571. doi: 10.1093/bioinformatics/btq228
Sullivan, M. J., Petty, N. K., and Beatson, S. A. (2011). Easyfig: A genome comparison visualizer. Bioinformatics 27, 1009–1010. doi: 10.1093/bioinformatics/btr039
Tam, L. T. T., Jähne, J., Luong, P. T., Thao, L. T. P., Chung, L. T. K., Schneider, A., et al. (2020). Draft genome sequences of 59 endospore-forming gram-positive bacteria associated with crop plants grown in Vietnam. Microbiol. Resour. Announc. 9, 1–4. doi: 10.1128/mra.01154-20
Tsai, A. Y. L., Oota, M., and Sawa, S. (2020). Chemotactic host-finding strategies of plant endoparasites and endophytes. Front. Plant Sci. 11, 1–12. doi: 10.3389/fpls.2020.01167
van der Donk, W. A. (2015). Bacteria do it differently: An alternative path to squalene. ACS Cent. Sci. 1, 64–65. doi: 10.1021/acscentsci.5b00142
Vanderhoef, L. N., and Dute, R. R. (1981). Auxin-regulated wall loosening and sustained growth in elongation. Plant Physiol. 67, 146–149. doi: 10.1104/pp.67.1.146
Vanhaelewyn, L., Van Der Straeten, D., De Coninck, B., and Vandenbussche, F. (2020). Ultraviolet radiation from a plant perspective: the plant-microorganism context. Front. Plant Sci. 11, 1–18. doi: 10.3389/fpls.2020.597642
Velivelli, S. L. S., De Vos, P., Kromann, P., Declerck, S., and Prestwich, B. D. (2014). Biological control agents: from field to market, problems, and challenges. Trends Biotechnol. 32, 493–496. doi: 10.1016/j.tibtech.2014.07.002
Villegas-Escobar, V., Ceballos, I., Mira, J. J., Argel, L. E., Orduz Peralta, S., and Romero-Tabarez, M. (2013). Fengycin C produced by Bacillus subtilis EA-CB0015. J. Nat. Prod. 76, 503–509. doi: 10.1021/np300574v
Vlamakis, H., Chai, Y., Beauregard, P., Losick, R., and Kolter, R. (2014). Sticking together: building a biofilm the Bacillus subtilis way Hera. Nat. Rev. Microbiol. 11, 157–168. doi: 10.1038/nrmicro2960.Sticking
Wick, R. R., Judd, L. M., Gorrie, C. L., and Holt, K. E. (2017). Unicycler: resolving bacterial genome assemblies from short and long sequencing reads. PLoS Comput. Biol. 13:e1005595. doi: 10.1371/journal.pcbi.1005595
Wickham, H., Averick, M., Bryan, J., Chang, W., McGowan, L., François, R., et al. (2019). Welcome to the Tidyverse. J. Open Source Softw. 4:1686. doi: 10.21105/joss.01686
Wildman, H. G., and Parkinson, D. (1981). Seasonal changes in water-soluble carbohydrates of Populus tremuloides leaves. Can. J. Bot. 59, 862–869. doi: 10.1139/b81-120
Wu, L., Wu, H., Chen, L., Yu, X., Borriss, R., and Gao, X. (2015). Difficidin and bacilysin from bacillus amyloliquefaciens FZB42 have antibacterial activity against Xanthomonas oryzae rice pathogens. Sci. Rep. 5, 1–9. doi: 10.1038/srep12975
Wu, S., Zhu, Z., Fu, L., Niu, B., and Li, W. (2011). WebMGA: a customizable web server for fast metagenomic sequence analysis. BMC Genomics 12:444. doi: 10.1186/1471-2164-12-444
Xie, Y., Wei, Y., Shen, Y., Li, X., Zhou, H., Tai, C., et al. (2018). TADB 2.0: An updated database of bacterial type II toxin-antitoxin loci. Nucleic Acids Res. 46, D749–D753. doi: 10.1093/nar/gkx1033
Xu, M., Guo, J., Li, T., Zhang, C., Peng, X., Xing, K., et al. (2021). Antibiotic effects of volatiles produced by bacillus tequilensis XK29 against the black spot disease aaused by Ceratocystis fimbriata in postharvest sweet potato. J. Agric. Food Chem. 69, 13045–13054. doi: 10.1021/acs.jafc.1c04585
Zhou, Y., Liang, Y., Lynch, K. H., Dennis, J. J., and Wishart, D. S. (2011). PHAST: a fast phage search tool. Nucleic Acids Res. 39, W347–W352. doi: 10.1093/nar/gkr485
Zhou, H., Zhu, H., Ren, Z., Li, X., Zhong, J., and Liu, E. (2021). Efficacy of bacillus tequilensis strain JN-369 to biocontrol of rice blast and enhance rice growth. Biol. Control 160:104652. doi: 10.1016/j.biocontrol.2021.104652
Zhu, B., and Stülke, J. (2018). SubtiWiki in 2018: from genes and proteins to functional network annotation of the model organism Bacillus subtilis. Nucleic Acids Res. 46, D743–D748. doi: 10.1093/nar/gkx908
Keywords: Bacillus tequilensis, comparative genomics, biocontrol, natural products, banana epiphyte
Citation: Cuellar-Gaviria TZ, García-Botero C, Ju K-S and Villegas-Escobar V (2023) The genome of Bacillus tequilensis EA-CB0015 sheds light into its epiphytic lifestyle and potential as a biocontrol agent. Front. Microbiol. 14:1135487. doi: 10.3389/fmicb.2023.1135487
Edited by:
Sijun Zheng, Yunnan Academy of Agricultural Sciences, ChinaReviewed by:
Gang Fu, Guangxi Academy of Agricultural Sciences, ChinaChunyu Li, Guangdong Academy of Agricultural Sciences, China
Copyright © 2023 Cuellar-Gaviria, García-Botero, Ju and Villegas-Escobar. This is an open-access article distributed under the terms of the Creative Commons Attribution License (CC BY). The use, distribution or reproduction in other forums is permitted, provided the original author(s) and the copyright owner(s) are credited and that the original publication in this journal is cited, in accordance with accepted academic practice. No use, distribution or reproduction is permitted which does not comply with these terms.
*Correspondence: Kou-San Ju, anUuMTA5QG9zdS5lZHU=; Valeska Villegas-Escobar, dnZpbGxlZzJAZWFmaXQuZWR1LmNv