- Key Laboratory of Plant Resource Conservation and Germplasm Innovation in Mountainous Region (Ministry of Education), Collaborative Innovation Center for Mountain Ecology Agro-Bioengineering (CICMEAB), College of Life Sciences, Institute of Agro-Bioengineering, Guizhou University, Guiyang, Guizhou, China
The co-existence of hydroxylamine (NH2OH) and nitrite (NO2–-N) can aggravate the difficulty of wastewater treatment. The roles of hydroxylamine (NH2OH) and nitrite (NO2–-N) in accelerating the elimination of multiple nitrogen sources by a novel isolated strain of Acinetobacter johnsonii EN-J1 were investigated in this study. The results demonstrated that strain EN-J1 could eliminate 100.00% of NH2OH (22.73 mg/L) and 90.09% of NO2–-N (55.32 mg/L), with maximum consumption rates of 1.22 and 6.75 mg/L/h, respectively. Prominently, the toxic substances NH2OH and NO2–-N could both facilitate nitrogen removal rates. Compared with the control treatment, the elimination rates of nitrate (NO3–-N) and NO2–-N were enhanced by 3.44 and 2.36 mg/L/h after supplementation with 10.00 mg/L NH2OH, and those of ammonium (NH4+-N) and NO3–-N were improved by 0.65 and 1.00 mg/L/h after the addition of 50.00 mg/L NO2–-N. Furthermore, the nitrogen balance results indicated that over 55.00% of the initial total nitrogen was transformed into gaseous nitrogen by heterotrophic nitrification and aerobic denitrification (HN-AD). Ammonia monooxygenase (AMO), hydroxylamine oxidoreductase (HAO), nitrate reductase (NR), and nitrite reductase (NIR), which are essential for HN-AD, were detected at levels of 0.54, 0.15, 0.14, and 0.01 U/mg protein, respectively. All findings confirmed that strain EN-J1 could efficiently execute HN-AD, detoxify NH2OH and NO2–-N, and ultimately promote nitrogen removal rates.
1. Introduction
During the nitrogen biodegradation process, hydroxylamine (NH2OH) and nitrite (NO2–-N), as intermediate metabolites of heterotrophic nitrification and aerobic denitrification (HN-AD), are toxic to most nitrogen-transforming bacteria. NH2OH can destroy the protein translation process involved in the bacterial ribosome so that bacteria cannot reproduce and grow, thus affecting the nitrogen removal ability of the bacteria (Xing et al., 2020). NO2–-N has been described as an inhibitor of bacterial activity with known toxicity (Baumann et al., 1997). For example, NO2–-N could inhibit oxygen uptake, oxidative phosphorylation, and active transport of glucose and proline in P. aeruginosa (Yarbrough et al., 1980). The mRNA level of amoA (ammonia monooxygenase) in N. multiformis was significantly inhibited by 20 mM NO2–-N, which further affected the nitrogen conversion ability of the strain (Cua and Stein, 2011). The accumulation of NH2OH and NO2–-N can also lower the total nitrogen (TN) conversion efficiency (Ouyang et al., 2020). Therefore, it is necessary to isolate and identify HN-AD strains that can efficiently and simultaneously convert NH2OH and NO2–-N. Although multiple HN-AD, simultaneous nitrification and denitrification (SND) and aerobic denitrification bacteria have been reported and characterized by sustainable growth and low energy consumption, the conversion rates of inorganic nitrogen and TN were universally low, especially for the toxic nitrogen sources of NH2OH and NO2–-N. For example, the NO2–-N removal rates of Bacillus methylotrophicus L7, Pannonibacter phragmitetus B1 and Acinetobacter tandoii MZ-5 were only 0.24, 0.81, and 1.18 mg/L/h, respectively, (Ren et al., 2014; Bai et al., 2019; Ouyang et al., 2020). Alcaligenes faecalis No. 4 was confirmed to have the ability to degrade NH2OH with a conversion rate of 4.70 mg/L/h, but it could not remove NO2–-N (Joo et al., 2005). Although NH2OH and NO2–-N could be oxidized and reduced by Pseudomonas taiwanensis J488, their conversion rates were as low as 0.80 and 1.28 mg/L/h, respectively, (He et al., 2020). Analogously, the actinomycete Streptomyces mediolani EM-B2 was found to be capable of NH2OH and NO2–-N degradation, but the corresponding maximum consumption rates were only 0.43 and 2.01 mg/L/h, respectively, (He et al., 2021). Furthermore, numerous bacteria cannot simultaneously remove several nitrogen sources when NH2OH or NO2–-N coexist in the culture.
To date, most of studies have reported the effects of NH2OH and NO2–-N on the HN-AD process to convert nitrogen. For example, a concentration of NH2OH between 7.50 and 35.0 mg/L could delay NO2–-N reduction (Noophan et al., 2004). When the NH2OH level exceeded 35.00 mg/L, the cytotoxicity of NH2OH could reduce the activities of denitrifying bacteria and further lower the nitrate (NO3–-N) degradation rate (Zhang et al., 2020). Several reports indicated that the presence of NH2OH could promote bacterial abundance and the generation of nitrogen removal products. For instance, the abundance of ammonia-oxidizing bacteria was significantly enhanced when 5.00 mg/L NH2OH was supplemented (Yu et al., 2019). The accumulation of gaseous nitrogen in the form of NO was improved (0 → 0.23 mg/L) by the continuous addition of 5.00 mg/L NH2OH (Zhao et al., 2021). However, most reports on the effects of hydroxylamine on biological nitrogen removal have mainly focused on activated sludge, biofilms, and sequencing batch reactors, which are subject to various environmental factors and could not accurately assess the effects of hydroxylamine on biological nitrogen removal. Regarding the influence of NO2–-N, current reports have mainly focused on its negative effect on the HN-AD process. For instance, He et al. (2017) confirmed that supplementation with a low dosage of NO2–-N (5.33 mg/L) exhibited an inhibitory effect on nitrification and the ammonium (NH4+-N) conversion rate decreased from 0.40 mg/L/h to 0.12 mg/L/h at 15°C. Bettazzi et al. (2010) found that supplementation with 75.00 mg/L NO2–-N lowered anammox activity. However, positive effects of NO2–-N on HN-AD have not yet been reported. In addition, the influence of NH2OH and NO2–-N on the conversion rates of diverse inorganic nitrogen species has not yet been thoroughly studied, which limits the comprehensive evaluation of bacterial nitrogen removal ability in wastewater treatment. Therefore, identifying bacteria that can rapidly transform the toxic nitrogen sources NH2OH and NO2–-N without restricting the degradation rates of multiple inorganic nitrogen forms, may enhance wastewater treatment performance.
A previous report found that A. johnsonii could perform aerobic denitrification and that the lower-molecular-weight organic matter could promote the cell growth of A. johnsonii during the NO3–-N conversion process (Wen et al., 2019). Moreover, A. johnsonii exhibited outstanding partial denitrification performance and the key functional genes napA, nirB, and nirD were expressed under optimal environmental conditions (Zhang et al., 2019). However, the NH2OH and NO2–-N nitrogen conversion capabilities by A. johnsonii and the effects on HN-AD have not been reported. Therefore, the objectives of this study were to (1) focus on the isolation of an efficient HN-AD strain that can rapidly transform the toxic nitrogen sources NO2–-N and NH2OH and then identify it based on its morphology and molecular characteristics; (2) explore the HN-AD capacity in the presence of different nitrogen forms [(NH4)2SO4, KNO3, NaNO2, and HONH3Cl]; (3) investigate the effects of external NH2OH addition on the HN-AD process and cell growth by using batch tests; (4) examine the effects of NO2–-N on the HN-AD process and cell growth; and (5) elucidate the HN-AD mechanism through nitrogen balance analysis and the detection of related enzyme expression. The experimental results showed that strain EN-J1 could efficiently execute HN-AD, detoxify NH2OH and NO2–-N, and promote the nitrogen conversion rates and cell growth; these findings provide a theoretical and experimental basis for enhancing nitrogen conversion rates in wastewater pollution control.
2. Materials and methods
2.1. Bacterial isolation
The samples used in this experiment were taken from towel gourd vegetable cultivation soil (5∼10 cm depth) from Jinping County, southeastern Guizhou. For preliminary screening of the strains with the highest HN-AD ability, 1 g of soil was added to the enrichment medium, which consisted of (per liter) HONH3Cl (0.05 g), NaNO2 (0.10 g), C6H5Na3O7.2H2O (1.84 g), K2HPO4 (3.50 g), MgSO4 (0.04 g), CaCI2 (0.01 g), Fe2(SO4)3 (0.01 g), KH2PO4 (1.50 g), pH = 7.20 and transfer was performed 3 times at 4-day intervals (150 rpm, 25°C). The enriched medium (5, 10, 15 μL) was extracted and then purified on bromothymol blue (BTB) solid medium, which was made with (per liter, pH = 7.00) 1 mL BTB reagent (1.50% in ethanol), (NH4)2SO4 (0.24 g), sodium citrate (2.45 g), FeSO4.7H2O (0.59 g), KH2PO4 (1.00 g), CaCl2 (0.09 g), MgSO4 (0.49 g), and agar (18 g) (He et al., 2018). Bacteria with blue colonies and the highest removal rates of NH2OH and NO2–-N were selected as the candidate strain and stored at −20°C (30% glycerol). The basic medium composition for assessment of the nitrogen removal capability by the candidate contained (per liter, pH = 7.20) KH2PO4 (1.50 g), K2HPO4 (3.50 g), C6H5Na3O7.2H2O (3.06 g), Fe2(SO4)3 (0.01 g), MgSO4 (0.04 g), and CaCI2 (0.01 g) (He et al., 2019). (NH4)2SO4 (0.24 g), HONH3Cl (0.10 g), KNO3 (0.36 g), and NaNO2 (0.25 g) were added separately to the basic medium to analyze the nitrogen conversion ability of the target strain. The content of the carbon source was changed to 1.23 g C6H5Na3O7.2H2O with NH2OH only as the nitrogen source. To study the effects of NH2OH on HN-AD, the medium was modified to contain (per liter, pH = 7.20) HONH3Cl (0.05 g), KNO3 (0.36 g), NaNO2 (0.25 g) or (NH4)2SO4 (0.24 g), MgSO4 (0.04 g), K2HPO4 (3.50 g), CaCl2 (0.01 g), Fe2(SO4)3 (0.01 g), KH2PO4 (1.50 g), and C6H5Na3O7.2H2O (3.68 g). Similarly, the medium composition NaNO2 (0.25 g), (NH4)2SO4 (0.24 g) or KNO3 (0.36 g), MgSO4 (0.04 g), K2HPO4 (3.50 g), CaCl2 (0.01 g), KH2PO4 (1.50 g), Fe2(SO4)3 (0.01 g), and C6H5Na3O7.2H2O (6.13 g) was applied to study the influence of NO2–-N on HN-AD. Luria-Bertani (LB) medium containing tryptone (10.00 g), NaCl (10.00 g) and yeast extract (5.00 g) was used for bacterial activation and cultivation before all simulated wastewater experiments. All media were sterilized for 30 min (121°C, 0.11 MPa).
2.2. Molecular identification
Bacterial suspensions of strain EN-J1 were coated on LB plates and cultivated at 25°C until colony formation. Colony morphologies were observed via an SU8100 scanning electron microscope (Beijing, China). Gram staining of the bacterial cells was assessed with an Olympus BX53-DIC optical microscope (Beijing, China). A DNA extraction kit (Magen) was applied to extract genomic DNA of strain EN-J1 that was selected as a template to amplify 16S rRNA gene sequences. Amplification of the 16S rRNA gene by polymerase chain reaction (PCR) (25 μL reaction system) was conducted with the universal primers 27F and 1492R by Bio-Rad (CFXConnect&T100, America) (He and Li, 2015), and the PCR products were detected by Sangon Biotech (Shanghai, China). Afterward, the 16S rRNA gene sequence returned from Sangon Biotech was submitted to the NCBI to obtain accession number. After alignment by BLAST, the phylogenetic tree reflecting the kinship of strain EN-J1 was founded by MEGA 7.0.
2.3. Evaluation of HN-AD capacity and the effects of NH2OH and NO2–-N on HN-AD
To evaluate the HN-AD capability of the selected strain EN-J1, the bacteria (glycerol-preserved) were inoculated in LB medium, cultivated at 25°C for 24 h with a 150 rpm shaking speed, and then harvested under centrifugation conditions (6,500 rpm, 25°C, 5 min). Strain EN-J1 was washed 3 times with sterile pure water to obtain a pure bacterial suspension. Bacteria with an optical density (OD600) value of 0.20 were inoculated into 100 mL media containing (approximately 50 mg/L) NH4+-N, NO3–-N or NO2–-N, and bacteria with an OD600 of 0.50 were added to 20 mg/L NH2OH medium, in which sodium citrate was applied as an electron donor. The procedure for studying the effects of NH2OH or NO2–-N on HN-AD was similar to that described above, except for the use of different carbon and nitrogen sources. Medium without bacterial addition was used as a control treatment. Six milliliters of medium supernatant was collected from the culture every 6 h to measure pH, OD600 and the concentrations of different inorganic nitrogen forms (including NH4+-N, NH2OH, NO3–-N, and NO2–-N) and TN. The conversion rates of inorganic nitrogen were calculated by the formula Ev = (C1-C2)/ΔT, in which Ev represents the nitrogen removal rate, and C1, C2 and ΔT signify the beginning and final nitrogen contents and time interval (Cheng et al., 2020). The results were used to further analyze the nitrogen conversion characteristics of strain EN-J1.
2.4. Nitrogen balance analysis
Bacterial solution cultivated in LB medium for 24 h at 25°C with a rotation speed of 150 rpm was separately inoculated into NH4+-N, NO2–-N, NH2OH, and NO3–-N and media. At the beginning of inoculation (0 h), the mixed bacterial solution (6 mL) was centrifuged at 6,500 rpm for 5 min, and then the supernatant was collected to detect the TN (initial TN1) and inorganic (NH4+-N, NH2OH, NO2–-N, and NO3–-N) concentrations. Simultaneously, another 6 mL of bacterial solution was harvested and lysed by ultrasonication for 15 min under 300 W power and a 3 s working/interval time with a Scientz-IID ultrasonicator. Afterward, a 0.22 mm filter membrane was used to filter the supernatant to detect the contents of NH4+-N, NH2OH, NO3–-N, and NO2–-N and final TN1. After cultivation in the NH4+-N, NO3–-N NH2OH, and NO2–-N media for 12, 18, 30, and 18 h, the new supernatants (6 mL) were collected, and the inorganic nitrogen (NH4+-N, NH2OH, NO2–-N, and NO3–-N) and initial TN2 were determined by repeating the above procedure. Another 6 mL bacterial solution was harvested and lysed, and the final NH4+-N, NH2OH, NO3–-N, NO2–-N and final TN2 were detected. All relevant nitrogen contents were calculated as follows (He et al., 2022):
(1) Organic N = initial TN2–final (NH4+-N + NH2OH + NO2–-N + NO3–-N); (2) Accumulated intracellular N = (final TN2 –initial TN2)–(final TN1–initial TN1); (3) Nitrogen loss (%) = [final TN1–final (NH4+-N + NH2OH + NO2–-N + NO3–-N)–Organic N–Accumulated intracellular N]/final TN1 × 100%.
2.5. Detection of HN-AD enzyme activities
The time with the highest nitrogen removal rate was selected to determine enzyme activity. After cultivation for 5, 21, 10, and 10 h with NH4+-N, NH2OH, NO3–-N, and NO2–-N media alone, strain EN-J1 was collected by centrifugation (6,500 rpm, 5 min, 25°C). Protein extraction in the crude extract of bacterial strain EN-J1 was performed using a BCA bacterial protein extraction kit (Solarbio, Beijing, China). The enzyme activity of ammonia monooxygenase (AMO) was determined by a testing company (Wela, Guiyang, China), and NO2–-N reductase (NIR) was detected by a NO2–-N reduction activity assay kit (COMIN, Suzhou Industrial Park, China). For NO3–-N reductase (NR) activity determination, the 20 mL reaction system contained NO3–-N, enzyme extract, NADH and Tris-HCl. The activity of NR was evaluated by the disappearance of NO3–-N after reaction for 15 min at 25°C (Zhang et al., 2022). In addition, a reaction mixture of 20 mL containing enzyme extract, NH2OH, potassium ferricyanide, EDTA and Tris-HCl was prepared for NH2OH oxidase (HAO) activity evaluation. The reduction in NH2OH content was taken as the measure to detect HAO activity. A control group was designed with no enzyme extract addition. The specific activity (U/mg) was calculated by the content of enzyme needed to degrade 1 μmol of substrate per minute divided by the protein concentration.
2.6. Analytical analysis
An ultraviolet (UV) spectrophotometer (Metash UV-6000) with a 600 nm wavelength was applied to monitor the bacterial solution concentration. After cell culture and centrifugation, the bacterial supernatant was used to evaluate the TN, NH4+-N, NO2–-N, NH2OH, and NO3–-N concentrations by alkaline potassium persulfate digestion-UV spectrophotometry, the indophenol blue method, 8-hydroxyquinoline UV spectrophotometry, UV spectrophotometry, and N-(1-naphthyl)-ethylenediamine photometry, respectively, (Chen et al., 2012). The pH meter of DDS-307A was applied to assess the pH value. All experimental data and figures were processed using SPSS Statistics, Excel, MEGA 7.0, and Origin2021 software and are presented as the mean ± SD.
3. Results
3.1. The isolated bacterium exhibited high NO2–-N and NH2OH removal capacities
A mixed nitrogen source of NH2OH and NO2–-N was applied to obtain the HN-AD strain to increase the possibility of simultaneously removing multiple nitrogen sources. Thus, the newly screened strain EN-J1, which was isolated from a towel gourd vegetable cultivation field and purified on LB and BTB plates, exhibited outstanding NO2–-N and NH2OH removal ability. The colony morphological characteristics of strain EN-J1 were yellow, wet surface, regular edge, and convex (Figure 1A). Strain EN-J1 was found to be gram-negative by Gram staining and appeared short and rod-shaped without flagellum (Figures 1B, C); it was similar to strain Acinetobacter sp. HA2 except for colony color (Yao et al., 2013). The 16S rRNA nucleotide sequences were uploaded to GenBank under accession number ON076880. A phylogenetic tree demonstrating the kinship among related strains and strain EN-J1 was constructed with partial 16S rRNA sequences. Homology searches of strain EN-J1 via BLAST illustrated that it was highly similar (99.23%) to the gene sequences of A. johnsonii ATCC (Figure 2). To our knowledge, the nitrogen removal capability of NH2OH and NO2–-N by A. johnsonii and their effects on the HN-AD process have not been reported.
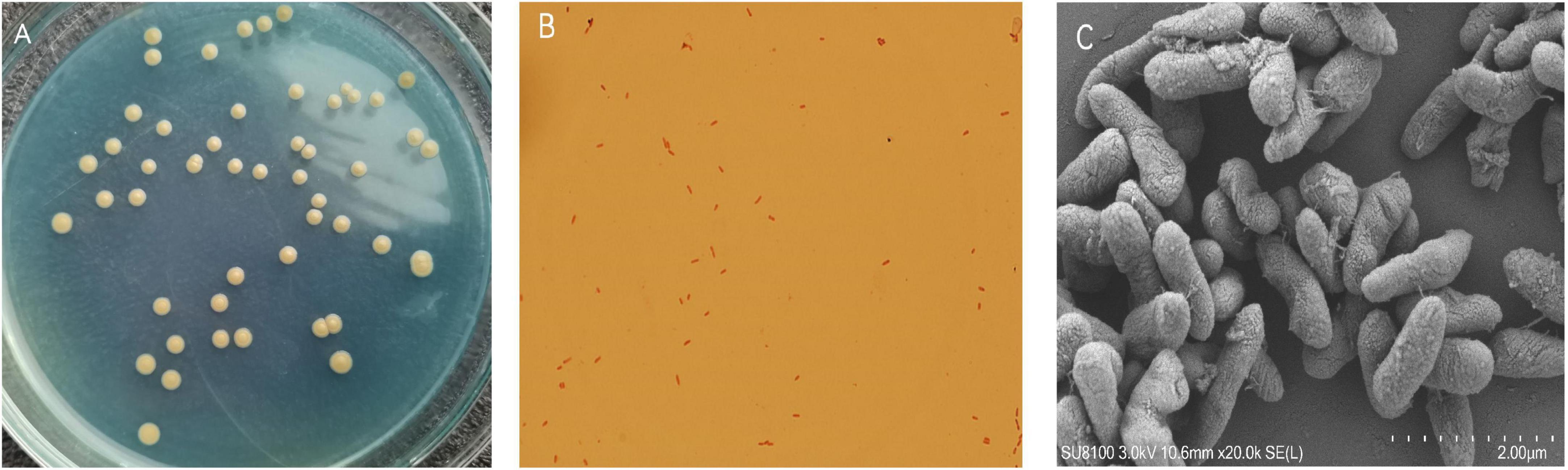
Figure 1. The colony morphologies of the strain EN-J1. (A) Colonies on BTB plate; (B) gram staining result under optical microscope; (C) cells shape by the scanning electron microscope.
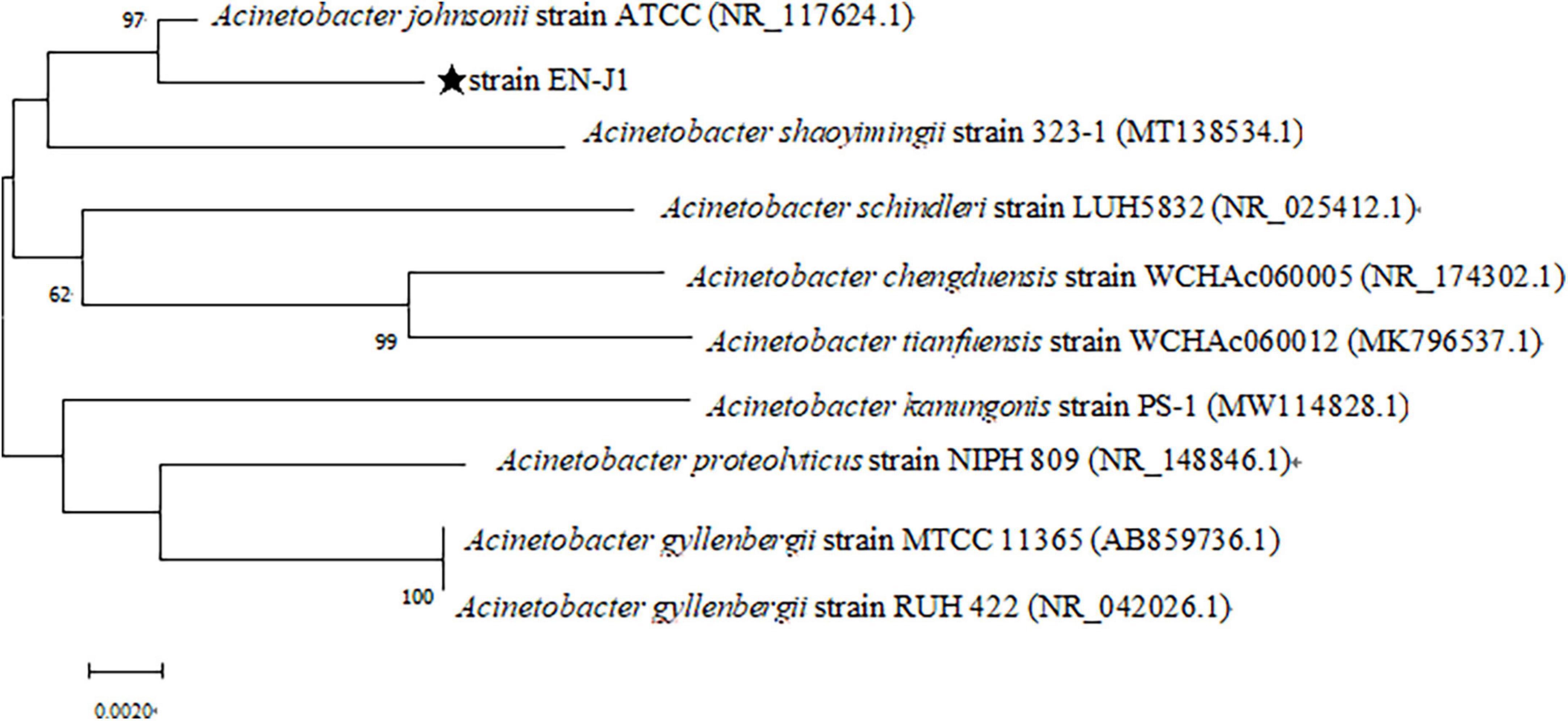
Figure 2. Neighbor-joining phylogenetic tree of the strain EN-J1 was structured based on its 16S rRNA gene sequence and other related strains sequences.
3.2. Characteristics of NH4+-N and NH2OH conversion
The nitrification process was carried out with NH4+-N as the sole nitrogen source, and the nitrification traits of strain EN-J1 were analyzed on the basis of the removal of NH4+-N and the accumulation of intermediate products. Figure 3A shows that bacterial strain EN-J1 grew rapidly for the first 12 h after inoculation, and the OD600 value reached the highest level of 0.97. The NH4+-N content decreased significantly from 53.99 to 11.25 mg/L during the first 6 h, and 99.28% of NH4+-N was converted as the oxidation time was extended to 12 h. In the process of NH4+-N oxidation, the maximum degradation rate of 7.12 mg/L/h appeared during 0−6 h. Compared with previously reported NH4+-N oxidation strains, the rate of NH4+-N degradation was considerably greater than the values of 5.53 mg/L/h for Pseudomonas stutzeri YZN-001 (Xia et al., 2020), 3.70 mg/L/h for Bacillus thuringiensis WXN-23 (Xu et al., 2021), 3.46 mg/L/h for Streptomyces mediolani EM-B2 (He et al., 2022) and 2.29 mg/L/h for Vibrio diabolicus SF16 (Duan et al., 2015). Moreover, total nitrogen consumption reached 92.95%, corresponding to a maximum consumption rate of 6.42 mg/L/h, which indicated that much of the NH4+-N nitrogen was consumed and converted to gaseous nitrogen. After 18 h of continuous cultivation, the concentration of NH4+-N tended to increase based on the breakdown of dead cells (Li et al., 2015), which lowered the removal efficiency of TN (84.72%). During the whole experiment, neither NH2OH nor NO3–-N accumulation was observed, whereas 0.05 mg/L NO2–-N was observed at 6 h, and then NO2–-N was exhausted after cultivation for 12 h. This phenomenon was in contrast to the fact that 2.56 mg/L NO3–-N, instead of NO2–-N, was detected during the nitrification process by strain EM-B2 (He et al., 2022). Moreover, pH exhibited an upward trend (7.18 → 9.00), suggesting that denitrification with NO2–-N as a nitrogen source could also be conducted when EN-J1 performed nitrification with NH4+-N nitrogen alone. This phenomenon was the same as that observed in a study of strain EN-F2, in which 0.11 mg/L NO2–-N was measured after 6 h of reaction (Zhang et al., 2022). Overall, strain EN-J1 displayed excellent NH4+-N and TN conversion rates without the accumulation of intermediates and efficiently transformed a large amount of NH4+-N to gaseous nitrogen. The short NH4+-N oxidation pathway by strain EN-J1 was beneficial for the thorough treatment of NH4+-N-contaminated wastewater.
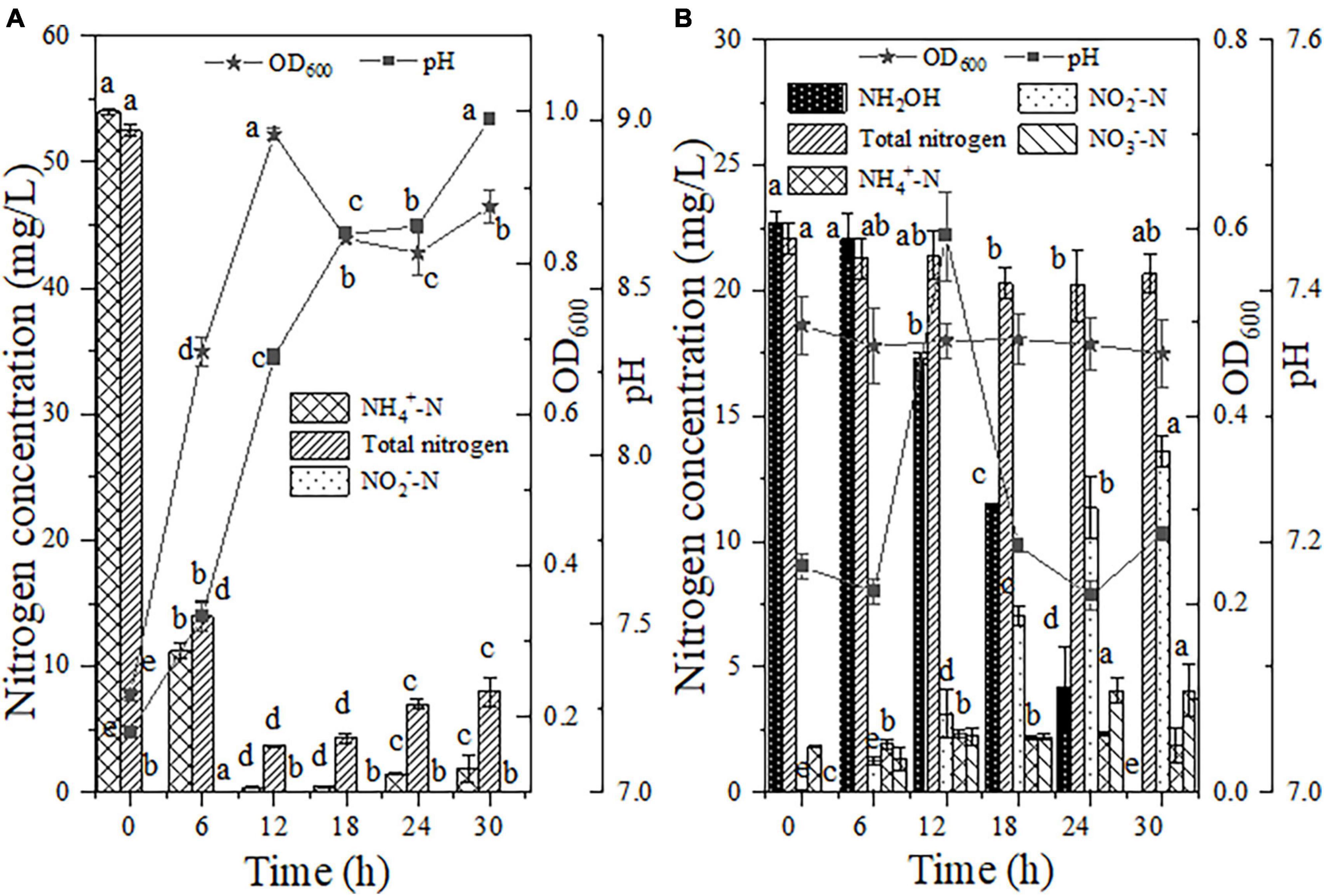
Figure 3. The heterotrophic nitrification characteristics of strain EN-J1. (A) Ammonium (NH4+-N); (B) hydroxylamine (NH2OH). Values are means ± SD. Different letters represent significant differences between treatments (p < 0.05).
Few bacterial strains with the capacity for the removal of NH2OH (one of the intermediate products of nitrification) have been reported, which limits the comprehensive evaluation of the nitrification ability of various strains. Accordingly, NH2OH was selected as the only form of nitrogen for further assessment of the NH2OH oxidation capacity by EN-J1. Within the initial 6 h of inoculation, a decreasing trend of NH2OH was not observed (Figure 3B). After reaction for 18 h, 11.30 mg/L NH2OH was consumed from a 22.73 mg/L initial content. Strain EN-J1 consumed 99.96% of the NH2OH when the reaction time was extended to 30 h. The maximum elimination rate of NH2OH reached 1.22 mg/L/h from 18−24 h, which was lower than the value of 2.12 mg/L/h for Pseudomonas taiwanensis EN-F2 (Zhang et al., 2022). Nonetheless, on the basis of comparison with the values for most reported HN-AD strains that can remove NH2OH, such as 0.21 mg/L/h for Glutamicibacter arilaitensis EM-H8 (Chen et al., 2022) and 0.70 mmol/L/h for Photobacterium sp. NNA4 (Liu et al., 2019), strain EN-J1 was more efficient degrading NH2OH. Throughout the experiment, the pH and OD600 values remained basically stable, and TN slightly decreased from 22.44 to 20.66 mg/L. Furthermore, NO3–-N (4.06 mg/L) and NH4+-N (1.86 mg/L) accumulation was observed. Moreover, NO2–-N production reached the highest value of 13.62 mg/L at 30 h, indicating that strain EN-J1 converted most of the NH2OH to NO2–-N instead of gaseous nitrogen. This phenomenon was consistent with observations of the strain Pseudomonas putida Y-9 (Huang et al., 2019) but distinct from those of Acinetobacter calcoaceticus HNR (Zhao et al., 2010).
3.3. Traits of strain EN-J1 with respect to NO3–-N and NO2–-N removal
The utilization of NO3–-N (50 mg/L) by strain EN-J1 under aerobic conditions was explored to illustrate the denitrification characteristics. A conspicuous increase in OD600 (0.21 → 0.67) and a decrease in NO3–-N concentration were observed after 12 h of incubation (Figure 4A). A total of 84.01% of NO3–-N was reduced with a maximum rate of 4.34 mg/L/h between 6 and 12 h, which was faster than the rates of 1.71 mg/L/h (for 100 mg/L initial NO3–-N) or 1.90 mg/L/h (for 50 mg/L initial NO3–-N) observed for Streptomyces mediolani EM-B2 (He et al., 2021, 2022) and 1.99 mg/L/h observed for P. tolaasii Y-11 (He et al., 2016) but slightly lower than the rates of 4.54 and 5.80 mg/L/h obtained for Pseudomonas mendocina X49 (Xie et al., 2021) and Pseudomonas taiwanensis EN-F2 (Zhang et al., 2022), respectively. It is worth noting that the degradation efficiency and rate of NO3–-N were much lower than those of NH4+-N, indicating that for strain EN-J1, nitrification with NH4+-N as a nitrogen source was stronger than denitrification with NO3–-N-only nitrogen. This conclusion was similar to that reported for Pseudomonas mendocina X49 (Xie et al., 2021). Furthermore, the TN content decreased (57.64 → 19.07 mg/L), corresponding to a maximum conversion efficiency and rate of 66.92% and 5.41 mg/L/h, respectively. The pH value increased to 8.99. A low level of NO2–-N (2.54 mg/L) was detected at 30 h, which was different from the result for the strain Acinetobacter sp. ND7 (Xia et al., 2020), which exhibited no NO2–-N accumulation. Approximately 3.84 mg/L NH4+-N accumulated, which might originate from the disintegration of dead cells. All the results in this section showed that strain EN-J1 could carry out denitrification with a nitrogen source of NO3–-N and transform most of the NO3–-N into gaseous nitrogen.
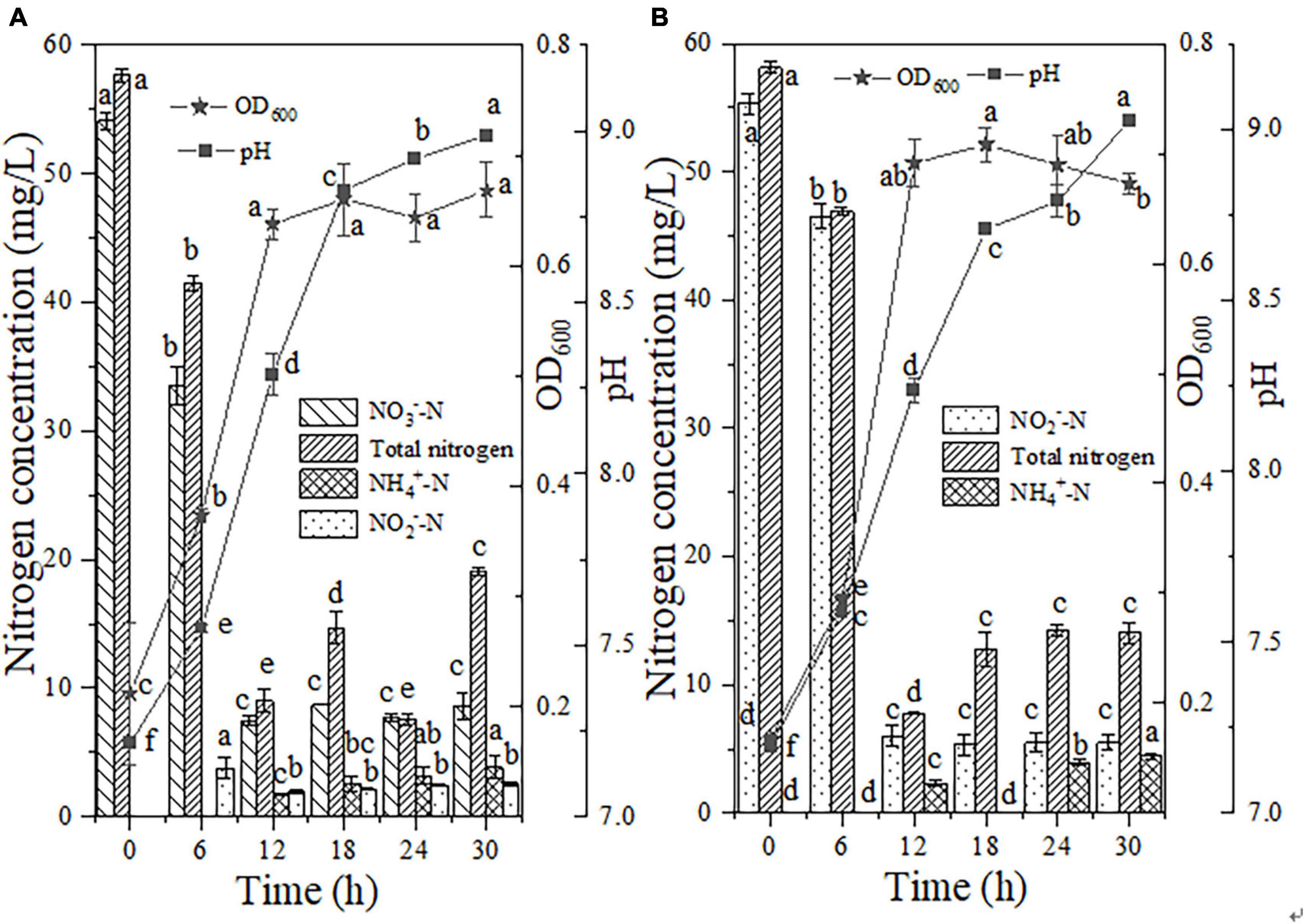
Figure 4. The aerobic denitrification characteristics of strain EN-J1. (A) Nitrate (NO3–-N); (B) nitrite (NO2–-N). Different letters represent significant differences between treatments (p < 0.05).
When equivalent amounts of NO2–-N instead of NO3–-N were added to the medium, the reduction of NO2–-N and accumulation of intermediates could be used to further reflect the denitrification characteristics of strain EN-J1. Figure 4B shows that 89.10% of NO2–-N with an initial concentration of 55.32 mg/L was removed at a maximum degradation rate of 6.75 mg/L/h after 12 h of reaction. The OD600 value reached 0.69. The high nitrogen removal rate and cell growth contradicted the conclusion that a high concentration of NO2–-N inhibits cell growth and denitrification (Wan et al., 2011). After 18 h of cultivation, the removal efficiency of NO2–-N remained at 90.09%, and the cells reached the stationary phase. The incomplete removal of NO2–-N further indicated that the heterotrophic nitrification capacity of EN-J1 was superior to its aerobic denitrification capacity, which was also found for the strain Pseudomonas taiwanensis EN-F2 (Zhang et al., 2022). Fortunately, the conversion rate (6.75 mg/L/h) was noticeably higher than that of previous HN-AD strains for NO2–-N removal, such as 3.25 mg/L/h of Pseudomonas putida Y-12 (Ye et al., 2017), 1.74 mg/L/h of Pseudomonas tolaasii Y-11 (He et al., 2016) and 4.12 mg/L/h of Ochrobactrum anthropic LJ81 (Lei et al., 2019). Moreover, 86.84% of TN was transformed with a maximum rate of 6.52 mg/L/h, which was a much higher rate than that of the NO2–-N-removing strains mentioned above. In addition, during the reduction of NO2–-N, the level of NH4+-N increased to the highest value of 4.42 mg/L at 30 h, which decreased the removal efficiency of TN (86.84% → 75.88%). Similar to a report on the strain Streptomyces mediolani EM-B2 (He et al., 2021), NO3–-N could not be detected in this study. The pH increased from 7.19 to 9.03 based on alkali production from NO2–-N reduction, which further confirmed that strain EN-J1 could efficiently execute denitrification.
3.4. Effects of NH2OH on the HN-AD process
To investigate the effects of NH2OH on the HN-AD process and bacterial cells of strain EN-J1, approximately 10 mg/L NH2OH hydrochloride was added to individual samples of nitrogenous media containing NO3–-N, NO2–-N, and NH4+-N.
The intermediate product accumulation and cell growth values obtained with the addition of NH2OH to NO3–-N medium are shown in Figure 5A. During the initial 12 h of inoculation, 11.93 mg/L NH2OH was consumed with an oxidation efficiency of 100.00%. The maximum NH2OH consumption rate was 1.11 mg/L/h, which was similar to the result obtained from the NH2OH-only removal system (1.22 mg/L/h). The above results signified that the presence of NO3–-N had almost no influence on NH2OH removal. The NO3–-N concentration slightly increased by 2.07 mg/L within 12 h due to NH2OH oxidation, and then decreased promptly to 6.20 mg/L after NH2OH was completely depleted, which implied that nitrification with NH2OH as a nitrogen source was preferentially performed by strain EN-J1. Within 30 h of inoculation, NO3–-N consumption reached 89.56%, and a degradation rate of 7.78 mg/L/h was observed, which was markedly superior to the value of 4.34 mg/L/h obtained with the NO3–-N-only reduction system. Concurrently, the cell growth of strain EN-J1 ranged from 0.49 to 1.15, which was also higher than that in the NO3–-N-only reaction system. It could be concluded that when NH2OH was replenished, the maximum rate of NO3–-N was increased by 3.44 mg/L/h, and the OD600 value was boosted by 0.48. The positive effect of NH2OH on NO3–-N removal was consistent with the finding that a low dosage of NH2OH promoted NO3–-N conversion (Zhang et al., 2020). With the consumption of NH2OH and NO3–-N, the pH increased to 9.11. The TN content exhibited a conspicuous reduction from 72.09 to 18.20 mg/L, and the corresponding conversion maximum efficiency and rate reached 74.75% and 8.50 mg/L/h, respectively. The high TN removal rate was also prominently higher than that in the sole NO3–-N reduction system (5.41 mg/L/h) and the system with NH2OH alone (0.60 mg/L/h). Throughout the experiment, only 3.26 mg/L NO2–-N and 3.53 mg/L NH4+-N accumulation was observed. All results indicated that NH2OH exhibited a position effect on NO3–-N and TN removal.
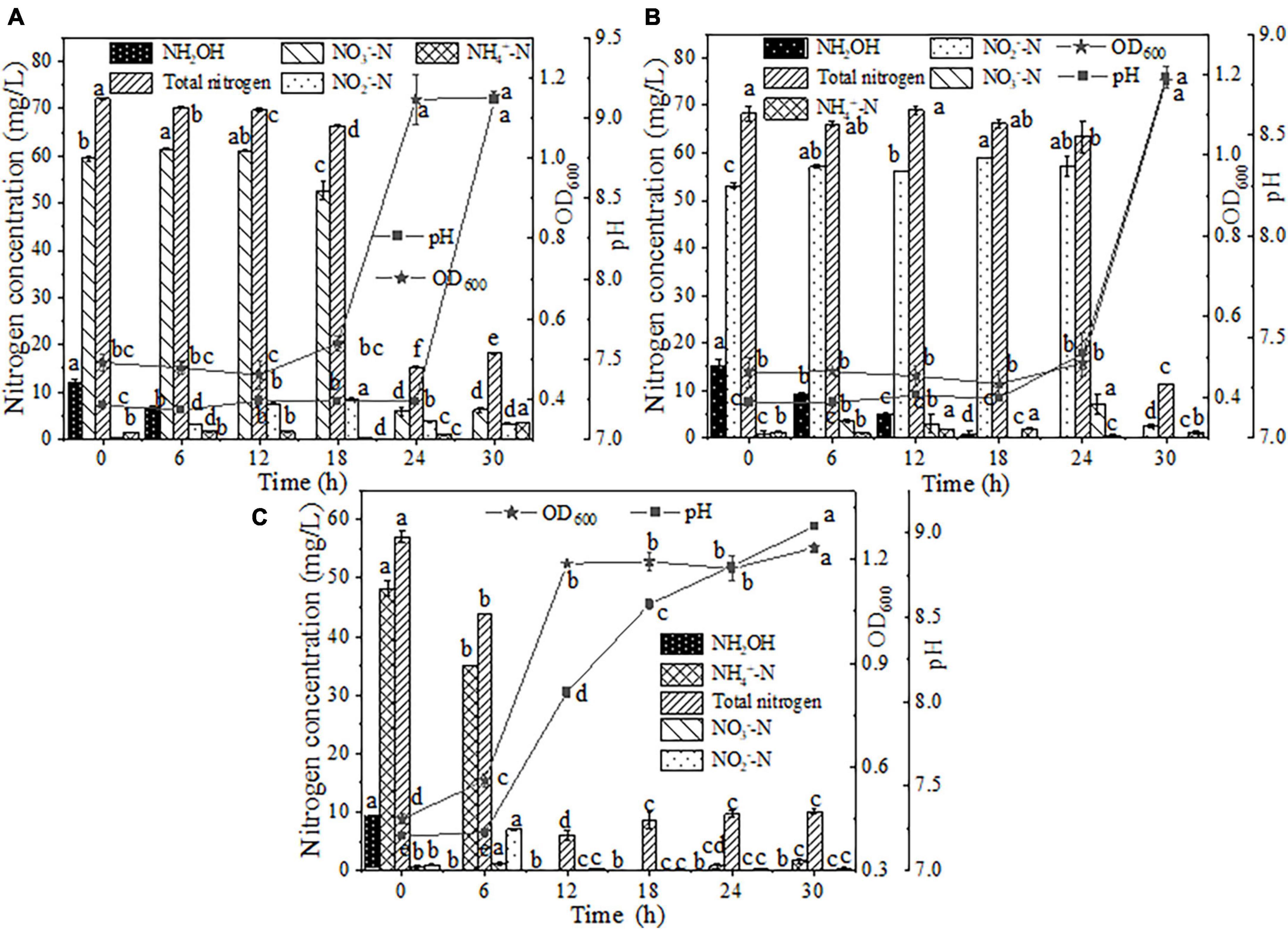
Figure 5. The effects of hydroxylamine (NH2OH) on HN-AD. (A) Nitrate (NO3–-N); (B) nitrite (NO2–-N); (C) ammonium (NH4+-N). Different letters represent significant differences between treatments (p < 0.05).
When NH2OH was added to NO2–-N medium, a stagnation period of strain EN-J1 was observed and the OD600 value remained at approximately 0.45 within 24 h of cultivation (Figure 5B) but then rapidly increased to 1.18 at 30 h. Cell growth was boosted by 0.51 compared to that in the only NO2–-N removal system. The pH increased from 7.18 to 8.79. During this period, the degradation efficiency and maximum removal rate of NH2OH reached 100.00% and 1.01 mg/L/h, respectively, at an initial concentration of 15.14 mg/L. This rate was slightly lower than that of the NH2OH only system (1.22 mg/L/h), which indicated that NO2–-N could delay the consumption of NH2OH. Nonetheless, NO2–-N exhibited no influence on NH2OH conversion efficiency, which was the same as the result for Pseudomonas taiwanensis EN-F2 (Zhang et al., 2022). Similar to the phenomenon in the case of mixed NH2OH and NO3–-N, NO2–-N increased by approximately 4.00 mg/L because of NH2OH oxidation within 24 h and then declined to 2.54 mg/L after NH2OH depletion. The degradation efficiency and maximum degradation rate of NO2–-N reached 95.22% and 9.11 mg/L/h, respectively. Markedly, the NO2–-N reduction rate (9.11 mg/L/h) was higher than that (6.75 mg/L/h) of the NO2–-N-only nitrogen degradation system after NH2OH addition. The NO2–-N conversion rate was improved by 2.36 mg/L/h because of the addition of NH2OH, which was opposite to a report that NO2–-N consumption was inhibited by NH2OH (Noophan et al., 2004). During the nitrogen removal process, only 1.06 mg/L NH4+-N, rather than NO3–-N, accumulated. The maximum TN consumption rate reached 8.69 mg/L/h, and this value was enhanced by 2.17 mg/L/h after NH2OH was added. All of the above phenomena indicated that the presence of NH2OH could promote cell growth and NO2–-N and TN removal rates.
Similarly, NH2OH (approximately 10 mg/L) as a supplementary substance was added to the NH4+-N-containing medium. Strain EN-J1 proliferated rapidly after 6 h of inoculation and entered the logarithmic phase (Figure 5C). The peak value of OD600 reached 1.23 in the mixed media, which was substantially higher than that (0.87) in the NH4+-N-only treatment system. The OD600 value was boosted by 0.36 after NH2OH addition, which was also found by Yu et al. (2019). NH2OH was exhausted, and a maximum rate of 1.54 mg/L/h was achieved. This result indicated that NH4+-N played a positive role in the NH2OH removal process. The NH4+-N content started to drop from 48.19 to 35.11 mg/L in the first 6 h, and then it took another 6 h to completely remove the remaining 35.11 mg/L NH4+-N, corresponding to a maximum degradation rate of 5.85 mg/L/h. The addition of NH2OH lowered the removal rate of NH4+-N compared with that (7.12 mg/L/h) for NH4+-N nitrogen biodegradation alone, which implied that NH2OH retarded NH4+-N conversion. Even so, NH4+-N was still completely removed at 12 h. The content of NO3–-N correspondingly dropped from 0.53 to 0 mg/L. NO2–-N accumulated to the highest value of 7.06 mg/L at 6 h and then dropped to 0.28 mg/L. The pH increased to 9.04, which suggested that strain EN-J1 performed denitrification during the nitrification process. In addition, 82.34% of TN was removed at a maximum rate of 6.34 mg/L/h, which was close to the rate of 6.42 mg/L/h obtained with NH4+-N as the only nitrogen source. These results indicated that TN removal was not affected by NH2OH, which was inconsistent with a study showing that low levels of NH2OH could promote TN removal efficiency (Zekker et al., 2012). Overall, the conversion efficiencies of NH4+-N and TN were not affected by NH2OH addition.
In summary, when a low level of NH2OH (10.00 mg/L) was supplemented to NO3–-N or NO2–-N media as a mixed nitrogen source, the cell growth of strain EN-J1 was boosted by 0.48 and 0.51, respectively. Synchronously, the degradation rates of NO3–-N and NO2–-N were enhanced by 3.44 and 2.36 mg/L/h, and the corresponding TN degradation rates were enhanced by 3.09 and 2.17 mg/L/h, respectively. However, the presence of NH2OH hydrochloride had no effect on NH4+-N removal efficiency.
3.5. The effects of NO2–-N on NO3–-N/NH4+-N removal
The bacterial cell adaptation and nitrogen consumption performance after the addition of a high dosage of NO2–-N (55.97 mg/L) to the NO3–-N reaction medium are shown in Figure 6A. Within 6 h of the reaction, the NO2–-N content decreased by 3.51 mg/L in the presence of strain EN-J1. The degradation rates of NO2–-N and NO3–-N reached individual peak values of 4.31 and 5.34 mg/L/h between 6 and 12 h. Compared with that in the NO3–-N-only treatment system, the NO3–-N reduction rate was enhanced by 1.0 mg/L/h after NO2–-N addition, which demonstrated that the presence of NO2–-N significantly promoted the consumption of NO3–-N. This result was opposite to a report on the strain Pseudomonas taiwanensis EN-F2 (Zhang et al., 2022), in which the NO3–-N removal rate was limited by the addition of NO2–-N. However, NO3–-N exhibited an inhibitory effect on NO2–-N, which was consistent with the result for Thauera sp. SND5 (Wang and He, 2020). With nitrogen elimination, strain EN-J1 grew rapidly, and the OD600 value reached a peak of 1.32 after inoculation for 30 h. The pH showed an upward trend from 7.19 to 9.21. A total of 64.52% of the high concentration of TN was consumed with the highest rate of 9.25 mg/L/h, which was notably faster than that in the NO3–-N-only (5.41 mg/L/h) and NO2–-N-only (6.52 mg/L/h) treatment systems. During the whole experiment, only a low dosage of NH4+-N (3.12 mg/L) accumulated. These results showed that cell growth and the maximum conversion rates of NO3–-N and TN were enhanced by supplementation with NO2–-N. This conclusion was contradictory to most previous reports that NO2–-N inhibited the nitrogen removal process.
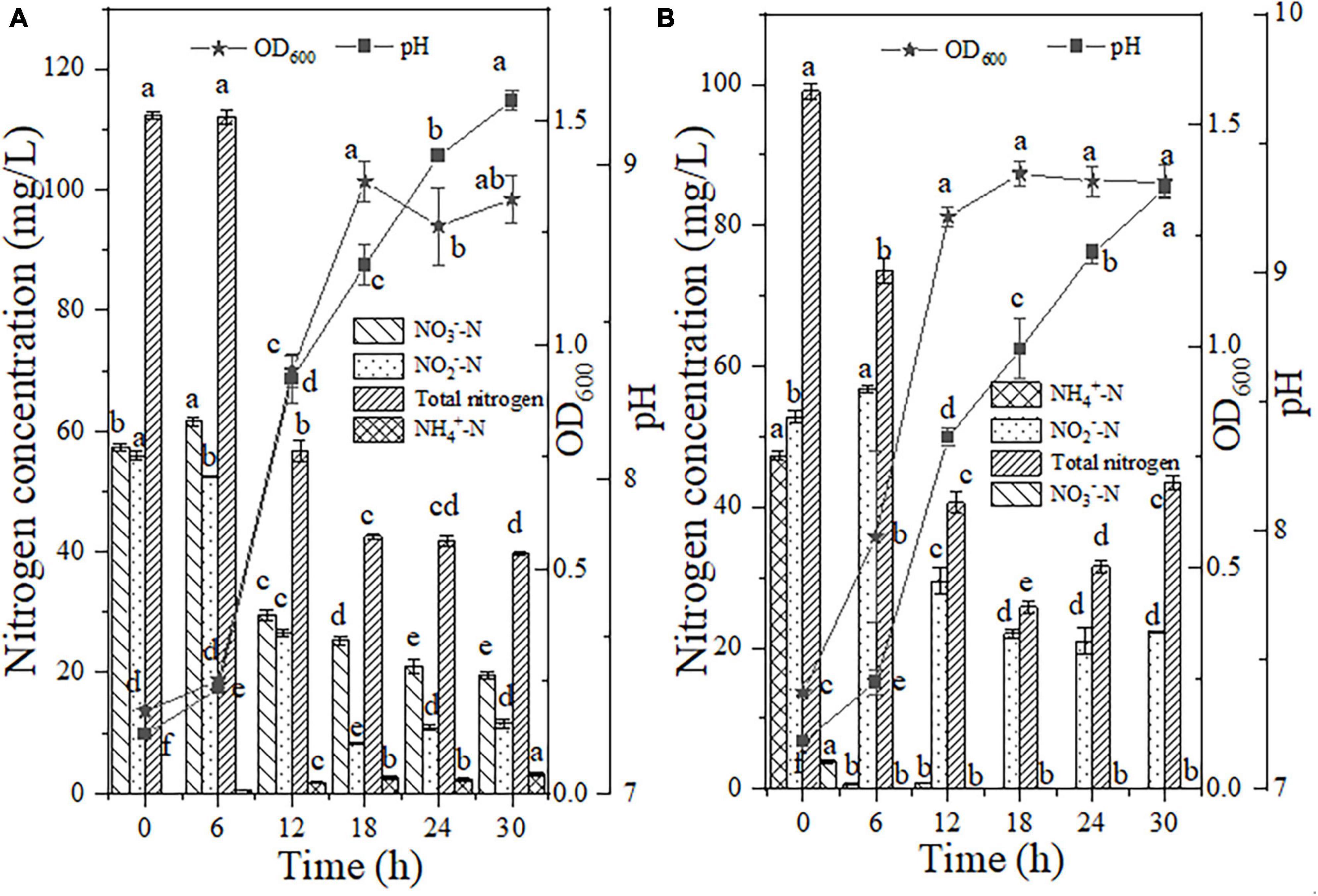
Figure 6. The effects of nitrite (NO2–-N) on HN-AD. (A) Nitrate (NO3–-N); (B) ammonium (NH4+-N). Different letters represent significant differences between treatments (p < 0.05).
High proportions of NO2–-N and NH4+-N were mixed as nitrogen sources to reveal the effect of NO2–-N on the HN process. In this mixed nitrogen reaction system, NH4+-N conversion was performed with an efficiency of 98.50% in the first 6 h (Figure 6B). During NH4+-N degradation, the NO2–-N content was enhanced by 4.00 mg/L with an increase in cell growth from 0.22 to 0.57, implying that NH4+-N was preferentially converted to NO2–-N, which was different from the report that NH4+-N and NO2–-N could be degraded together by Streptomyces mediolani EM-B2 (He et al., 2022). The increase in and disappearance of NO2–-N (4.00 mg/L) implied that NH4+-N was oxidized through the pathway NH4+-N → NO2–-N → nitrogenous gas. After 18 h of reaction, NH4+-N was exhausted, corresponding to the highest rate of 7.77 mg/L/h. The rate of NH4+-N degradation in this period was slightly enhanced by 0.65 mg/L/h after NO2–-N addition, which was similar to the result for Ochrobactrum anthropic LJ81 (Lei et al., 2019). The NO2–-N content was greatly reduced to 29.54 mg/L and the corresponding maximum degradation rate of 4.54 mg/L/h was detected between 6 and 12 h. When compared with the NO2–-N-only removal system (6.75 mg/L/h), the rate in mixed media was lower. An inhibitory effect on NO2–-N consumption was shown in the presence of NH4+-N, which was in contrast to the results for strain Arthrobacter arilaitensis Y-10 reported by He et al. (2017). Strain EN-J1 did not produce NO3–-N when cultured with mixed NO2–-N and NH4+-N. The pH increased (7.18 → 9.32) with nitrogen consumption. Moreover, at an initial TN content of 99.05 mg/L, 56.15% was removed with a maximum rate of 5.49 mg/L/h, which was slower than that of NH4+-N (6.42 mg/L/h) or NO2–-N (6.51 mg/L/h) alone. All the above findings demonstrated that although the removal rate of TN was not enhanced, NH4+-N removal and cell growth were promoted by NO2–-N addition.
In summary, after the addition of 50 mg/L NO2–-N, the conversion rates of NO3–-N and NH4+-N increased by 1.00 and 0.65 mg/L/h, respectively, and the corresponding cell growth increased by 0.65 and 0.50, respectively. Therefore, the additional addition of NH2OH and NO2–-N promoted the conversion rates of inorganic nitrogen species, which has great advantages for thorough wastewater treatment. However, deeper investigation of strain EN-J1 needs to be performed, especially regarding its potential in real wastewater treatment.
3.6. Nitrogen balance analysis of HN-AD
A nitrogen balance analysis of NH4+-N, NO2–-N, NH2OH, and NO3–-N in the current (bacterial strain EN-J1) system was calculated and is described in Table 1. The initial level of TN in the NH2OH-only system was 27.44 mg/L, and the NH4+-N, NO3–-N, and NO2–-N levels were approximately 54 mg/L. After 12 h of continuous reaction, 75.59% of the initial NH4+-N was lost, implying that the formation of gaseous nitrogen was the main route of NH4+-N removal by strain EN-J1. More importantly, the TN loss efficiency was considerably higher than the values of 40.20% for Acinetobacter calcoaceticus HNR (Zhao et al., 2010) and 27.11% for Exiguobacterium mexicanum SND-01 (Cui et al., 2021). During NH4+-N oxidation, intracellular-N (8.37 ± 0.70) and organic-N (4.34 ± 0.49) were detected, whereas the accumulation of NH2OH, NO2–-N, and NO3–-N was not observed, which was the same as the results in the NH4+-N-only nitrogen oxidation system. NO2–-N was detected at 6 h and was then exhausted at 12 h when NH4+-N was selected as a single nitrogen source, which further demonstrated that the oxidation route of NH4+-N was NH4+-N → NO2–-N → gaseous nitrogen. For NH2OH, 7.18% nitrogen loss was detected, and most NH2OH was converted to other nitrogen forms after cultivation for 30 h, in which 1.43 mg/L NH4+-N, 11.56 mg/L NO2–-N, and 1.62 mg/L NO3–-N were detected. These results were inconsistent with reports that NO2–-N was not observed with Glutamicibacter arilaitensis EM-H8 (Chen et al., 2022) and Alcaligenes faecalis NR (Zhao et al., 2012). This accumulation of intermediate products further proved that the pathway for NH2OH conversion was NH2OH → NO2–-N → NO3–-N → gaseous nitrogen.
In addition, approximately 57.79% of the initial TN was lost, and 8.72% of it was converted to organic-N when strain EN-J1 was cultivated with NO3–-N medium for 18 h, which illustrated that NO3–-N was almost completely reduced into gaseous nitrogen. The nitrogen loss from NO3–-N in the initial TN was higher than that of Pseudomonas mendocina LYX (51.90%) (Li et al., 2020) and Acinetobacter sp. YT03 (28.33%) (Li et al., 2019). On the basis of the detection of intermediate products, low concentrations of NH4+-N (1.28 mg/L) and NO2–-N (1.99 mg/L) accumulated, which further confirmed that the NO3–-N reduction pathway was NO3–-N → NO2–-N → gaseous nitrogen. Similarly, strain EN-J1 could transform most NO2–-N into gaseous nitrogen, and 64.25% nitrogen loss was achieved. This was markedly higher than the result of 38.88% for Streptomyces mediolani EM-B2 (He et al., 2022). To date, few studies have reported that HN-AD strains have the capacity to convert large amounts of NO2–-N into gas. Moreover, 3.16 mg/L organic-N and 2.42 mg/L NH4+-N were detected. According to the above results, the removal route of NO2–-N could be inferred to be NO2–-N → gaseous nitrogen. All of the above observations illustrated that NH4+-N, NO3–-N, and NO2–-N were mainly converted into gaseous nitrogen by strain EN-J1 to efficiently implement the HN-AD process.
3.7. Enzyme activity analysis
According to previous reports, the enzyme activities of AMO, HAO, NR, and NIR are related to the oxidation of NH4+-N and NH2OH and the reduction of NO3–-N and NO2–-N, respectively, (Ren et al., 2014). Therefore, the successful detection of related enzymes could verify the HN-AD pathway for bacteria. To date, no reports have studied HN-AD-related enzyme activities in A. johnsonii. In this study, the specific activities of AMO, HAO, NR, and NIR were successfully detected as 0.54, 0.15, 0.14, and 0.01 U/mg protein, respectively, (Table 2). By comparison, AMO exhibited the highest specific activity, which further confirmed the maximum conversion rate (7.12 mg/L/h) of NH4+-N. More importantly, the specific activities of these enzymes in strain EN-J1 were notably stronger than those in previously studied bacteria. For example, although the NR activity of strain EM-B2 (0.12 U/mg protein) was similar to that (0.15 U/mg protein) of EN-J1, the activities of both AMO (0.43 U/mg protein) and NIR (0.01 U/mg protein) were significantly lower than those of EN-J1 (He et al., 2022). Lower activities of HAO and NR in Pseudomonas taiwanensis J488 were detected, with values of 0.05 and 0.09 U/mg protein (He et al., 2020). HAO and NR activities of only 0.04 and 0.02 U/mg protein were obtained for Pseudomonas putida NP5 (Yang et al., 2019), and AMO, HAO, and NR activities of 0.05, 0.05, and 0.02 U/mg protein were obtained for Halomonas salifodinae (Hu et al., 2021). The high enzyme activities of AMO, HAO, NR, and NIR further verified the excellent HN-AD performance of strain EN-J1.
4. Conclusion
Acinetobacter johnsonii EN-J1 presented an outstanding nitrogen conversion capacity. The maximum conversion rates of NH4+-N, NH2OH, NO3–-N, and NO2–-N reached 7.12, 1.22, 4.34, and 6.75 mg/L/h, respectively. The addition of NH2OH and NO2–-N enhanced the HN-AD performance of strain EN-J1. The removal rates of NO3–-N and NO2–-N were increased by 3.44 and 2.36 mg/L/h, respectively, after the addition of 10 mg/L NH2OH, while the removal rates of NO3–-N and NH4+-N were increased by 1.00 and 0.65 mg/L/h under 50 mg/L NO2–-N addition. The enzymes involved in HN-AD were measured at 0.54, 0.15, 0.14, and 0.01 U/mg protein. The nitrogen balance results revealed that more than 55.00% of the initial TN was converted to gaseous nitrogen when the wastewater contained NH4+-N, NO3–-N, or NO2–-N. Altogether, strain EN-J1 exhibits potential for application in the treatment of mixed nitrogen-polluted wastewater.
Data availability statement
The raw data supporting the conclusions of this article will be made available by the authors, without undue reservation.
Author contributions
MZ: experimental design, data calculation, figures construction, manuscript writing, and experimental operation. TH: investigation, experimental idea, experimental design, experimental operation, data calculation, figures construction, manuscript revising, and fund investment. QW: experimental operation and investigation. MC: experimental operation. All authors contributed to the article and approved the submitted version.
Funding
This work was supported by the National Natural Science Foundation of China (42007223); Guizhou Provincial Science and Technology Plan Project [Qianke He Foundation-ZK (2021) General 233]; Guizhou Provincial General Colleges and Universities Youth Science and Technology Talent Growth Project [Qianjiaohe KY word (2021) 086]; and the Guizhou University Cultivation Project [Guizhou University Cultivation (2019) No. 50].
Conflict of interest
The authors declare that the research was conducted in the absence of any commercial or financial relationships that could be construed as a potential conflict of interest.
Publisher’s note
All claims expressed in this article are solely those of the authors and do not necessarily represent those of their affiliated organizations, or those of the publisher, the editors and the reviewers. Any product that may be evaluated in this article, or claim that may be made by its manufacturer, is not guaranteed or endorsed by the publisher.
References
Bai, H., Liao, S. A., Wang, A. L., Huang, J. H., Shu, W., and Ye, J. M. (2019). High-efficiency inorganic nitrogen removal by newly isolated Pannonibacter phragmitetus B1. Biosour. Technol. 271, 91–99. doi: 10.1016/j.biortech.2018.09.090
Baumann, B., van der Meer, J. R., Snozzi, M., and Zehnder, A. J. (1997). Inhibition of denitrification activity but not of mRNA induction in Paracoccus denitrificans by nitrite at a suboptimal pH. J. Microbiol. 72, 183–189. doi: 10.1023/A:1000342125891
Bettazzi, E., Caffaz, S., Vannini, C., and Lubello, C. (2010). nitrite inhibition and intermediates effects on Anammox bacteria: a batch-scale experimental study. Process Biochem. 45, 573–580. doi: 10.1016/j.procbio.2009.12.003
Chen, M. P., Ding, C. Y., He, T. X., Zhang, M. M., and Wu, Q. F. (2022). Efficient hydroxylamine removal through heterotrophic nitrification by novel bacterium Glutamicibacter arilaitensis EM-H8. Chemosphere. 288(Pt. 1), 132475. doi: 10.1016/j.chemosphere.2021.132475
Chen, X., Yang, L., Xiao, L., Miao, A., and Xi, B. (2012). Nitrogen removal by denitrification during cyanobacterial bloom in Lake Taihu. J. Freshw. Ecol. 27, 243–258.
Cheng, H. Y., Xu, A. A., Kumar Awasthi, M. K., Kong, D. D., Chen, J. S., Wang, Y. F., et al. (2020). Aerobic denitrification performance and nitrate removal pathway analysis of a novel fungus Fusarium solani RADF-77. Biosour. Technol. 295:122250. doi: 10.1016/j.biortech.2019.122250
Cua, L. S., and Stein, L. Y. (2011). Effects of nitrite on ammonia-oxidizing activity and gene regulation in three ammonia-oxidizing bacteria. FEMS Microbiol. Lett. 319, 169–175. doi: 10.1111/j.1574-6968.2011.02277.x
Cui, Y., Cui, Y. W., and Huang, J. L. (2021). A novel halophilic Exiguobacterium mexicanum strain removes nitrogen from saline wastewater via heterotrophic nitrification and aerobic denitrification. Biosour. Technol. 333:125189. doi: 10.1016/j.biortech.2021.125189
Duan, J. M., Fang, H. D., Su, B., Chen, J. F., and Lin, J. M. (2015). Characterization of a halophilic heterotrophic nitrification-aerobic denitrification bacterium and its application on treatment of saline wastewater. Biosour. Technol. 179, 421–428. doi: 10.1016/j.biortech.2014.12.057
He, T. X., and Li, Z. L. (2015). Identification and denitrification characterization of a novel hypothermia and aerobic nitrite-denitrifying bacterium, Arthrobacter arilaitensis strain Y-10. Desalination Water Treatment. 57, 19181–19189.
He, T. X., Li, Z. L., Sun, Q., Xu, Y., and Ye, Q. (2016). Heterotrophic nitrification and aerobic denitrification by Pseudomonas tolaasii Y-11 without nitrite accumulation during nitrogen conversion. Biosour. Technol. 200, 493–499. doi: 10.1016/j.biortech.2015.10.064
He, T. X., Wu, Q. F., Ding, C. Y., Chen, M. P., and Zhang, M. M. (2021). hydroxylamine and nitrite are removed effectively by Streptomyces mediolani strain EM-B2. Ecotoxicol. Environ. Saf. 224:112693. doi: 10.1016/j.ecoenv.2021.112693
He, T. X., Xie, D. T., Li, Z. L., Ni, J. P., and Sun, Q. (2017). Ammonium stimulates nitrate reduction during simultaneous nitrification and denitrification process by Arthrobacter arilaitensis Y-10. Biosour. Technol. 239, 66–73. doi: 10.1016/j.biortech.2017.04.125
He, T. X., Xie, D. T., Ni, J. P., Cai, X., and Li, Z. (2019). Investigating the effect of copper and magnesium ions on nitrogen removal capacity of pure cultures by modified non-competitive inhibition model. Ecotoxicol. Environ. Saf. 170, 479–487. doi: 10.1016/j.ecoenv.2018.12.019
He, T. X., Xie, D., Ni, J. P., Li, Z. L., and Li, Z. (2020). Nitrous oxide produced directly from ammonium, nitrate and nitrite during nitrification and denitrification. J. Hazard. Mater. 388:122114. doi: 10.1016/j.jhazmat.2020.122114
He, T. X., Ye, Q., Sun, Q., Cai, X., Ni, J. P., Li, Z. L., et al. (2018). Removal of nitrate in simulated water at low temperature by a novel psychrotrophic and aerobic bacterium, Pseudomonas taiwanensis strain. J. Biomed. Res. Int. 2018:4984087. doi: 10.1155/2018/4984087
He, T. X., Zhang, M. M., Ding, C. Y., Wu, Q. F., Chen, M. P., Mou, S. L., et al. (2022). New insight into the nitrogen removal capacity and mechanism of Streptomyces mediolani EM-B2. Biosour. Technol. 348:126819. doi: 10.1016/j.biortech.2022.126819
Hu, J., Yan, J. B., Wu, L., Bao, Y. Z., Yu, D. Q., and Li, J. (2021). Simultaneous nitrification and denitrification of hypersaline wastewater by a robust bacterium Halomonas salifodinae from a repeated-batch acclimation. Biosour. Technol. 341:125818. doi: 10.1016/j.biortech.2021.125818
Huang, X. J., Xu, Y., He, T. X., Jia, H. J., Feng, M., Xiang, S. D., et al. (2019). Ammonium transformed into nitrous oxide via nitric oxide by Pseudomonas putida Y-9 under aerobic conditions without hydroxylamine as intermediate. Biosour. Technol. 277, 87–93. doi: 10.1016/j.biortech.2019.01.040
Joo, H. S., Hirai, M., and Shoda, M. (2005). Characteristics of ammonium removal by heterotrophic nitrification-aerobic denitrification by Alcaligenes faecalis No. 4. J. Biosci. Bioengineer. 100, 184–191. doi: 10.1263/jbb.100.184
Lei, X., Jia, Y. T., Chen, Y. C., and Hu, Y. Y. (2019). Simultaneous nitrification and denitrification without nitrite accumulation by a novel isolated Ochrobactrum anthropic LJ81. Biosour. Technol. 272, 442–450. doi: 10.1016/j.biortech.2018.10.060
Li, B., Lv, R., Xiao, Y., Hu, W., Mai, Y. L., Zhang, J. W., et al. (2019). A novel nitrite-base aerobic denitrifying bacterium Acinetobacter sp. YT03 and its transcriptome analysis. Front. Microbiol. 10:2580. doi: 10.3389/fmicb.2019.02580
Li, C. N., Yang, J. H., Wang, X., Wang, E. T., Li, B. Z., He, R. X., et al. (2015). Removal of nitrogen by heterotrophic nitrification-aerobic denitrification of a phosphate accumulating bacterium Pseudomonas stutzeri YG-24. Biosour. Technol. 182, 18–25. doi: 10.1016/j.biortech.2015.01.100
Li, Y. X., Ling, J. Y., Chen, P. C., Chen, J. L., Dai, R. Z., Liao, J., et al. (2020). Pseudomonas mendocina LYX: a novel aerobic bacterium with advantage of removing nitrate high effectively by assimilation and dissimilation simultaneously. Front. Environ. Sci. Engineer. 15:57. doi: 10.1007/s11783-020-1349-3
Liu, Y., Ai, G. M., Wu, M. R., Li, S. S., Miao, L. L., and Liu, Z. P. (2019). Photobacterium sp. NNA4, an efficient hydroxylamine-transforming heterotrophic nitrifier/aerobic denitrifier. J. Biosci. Bioengineer. 128, 64–71. doi: 10.1016/j.jbiosc.2018.12.014
Noophan, P. L., Figueroa, L. A., and Munakata-Marr, J. (2004). Nitrite oxidation inhibition by hydroxylamine: experimental and model evaluation. Water Sci. Technol. 50, 295–304.
Ouyang, L., Wang, K. J., Liu, X. Y., Wong, M. H., Hu, Z. L., Chen, H. R., et al. (2020). A study on the nitrogen removal efficacy of bacterium Acinetobacter tandoii MZ-5 from a contaminated river of Shenzhen, Guangdong Province, China. Biosour. Technol. 315:123888. doi: 10.1016/j.biortech.2020.123888
Ren, Y. X., Yang, L., and Liang, X. (2014). The characteristics of a novel heterotrophic nitrifying and aerobic denitrifying bacterium, Acinetobacter junii YB. Biosour. Technol. 171, 1–9. doi: 10.1016/j.biortech.2014.08.058
Wan, C. L., Yang, X., Lee, D. J., Du, M. A., Wan, F., and Chen, C. (2011). Aerobic denitrification by novel isolated strain using nitrite as nitrogen source. Biosour. Technol. 102, 7244–7248.
Wang, Q. K., and He, J. Z. (2020). Complete nitrogen removal via simultaneous nitrification and denitrification by a novel phosphate accumulating Thauera sp. strain SND5. Water Res. 185:116300. doi: 10.1016/j.watres.2020.116300
Wen, G., Wang, T., Li, K., Wang, H. Y., Wang, J. Y., and Huang, T. L. (2019). Aerobic denitrification performance of strain Acinetobacter johnsonii WGX-9 using different natural organic matter as carbon source: effect of molecular weight. Water Res. 164:114956. doi: 10.1016/j.watres.2019.114956
Xia, L., Li, X. M., Fan, W. H., and Wang, J. L. (2020). Heterotrophic nitrification and aerobic denitrification by a novel Acinetobacter sp. ND7 isolated from municipal activated sludge. Biosour. Technol. 301:122749.
Xie, F. X., Thiri, M., and Wang, H. (2021). Simultaneous heterotrophic nitrification and aerobic denitrification by a novel isolated Pseudomonas mendocina X49. Biosour. Technol. 319:124198.
Xing, C. Y., Fang, Y. C., Chen, X., Guo, J. S., Shen, Y., and Yan, P. (2020). Effect of hydroxylamine on community of ANAMMOX sludge. Environ. Sci. 41, 3365–3372.
Xu, N., Liao, M., Liang, Y. Q., Guo, J. W., Zhang, Y. H., Xie, X., et al. (2021). Biological nitrogen removal capability and pathways analysis of a novel low C/N ratio heterotrophic nitrifying and aerobic denitrifying bacterium (Bacillus thuringiensis strain WXN-23). Environ. Res. 195:110797. doi: 10.1016/j.envres.2021.110797
Yang, L., Wang, X. H., Cui, S., Ren, Y. X., Yu, J., Chen, N., et al. (2019). Simultaneous removal of nitrogen and phosphorous by heterotrophic nitrification-aerobic denitrification of a metal resistant bacterium Pseudomonas putida strain NP5. Biosour. Technol. 285:121360. doi: 10.1016/j.biortech.2019.121360
Yao, S., Ni, J. R., Ma, T., and Li, C. (2013). Heterotrophic nitrification and aerobic denitrification at low temperature by a newly isolated bacterium, Acinetobacter sp. HA2. Bioresour. Technol. 139, 80–86. doi: 10.1016/j.biortech.2013.03.189
Yarbrough, J. M., Rake, J. B., and Eagon, R. G. (1980). Bacterial inhibitory effects of Nitrite: inhibition of active transport, but not of group translocation, and of intracellular enzymes. Appl. Environ. Microbiol. 39, 831–834. doi: 10.1128/aem.39.4.831-834.1980
Ye, Q., Li, K. L., Li, Z. L., Xu, Y., He, T. X., Tang, W. H., et al. (2017). Heterotrophic nitrification-aerobic denitrification performance of strain Y-12 under low temperature and high concentration of inorganic nitrogen conditions. Water 9:835. doi: 10.3390/w9110835
Yu, Y., Li, M. X., Dai, X., Meng, F. H., Qi, X. J., Hou, J. Q., et al. (2019). In situ mature leachate treatment with hydroxylamine addition in the Aerobic-Anaerobic Recirculation Landfill. Sci. Total Environ. 696:134084. doi: 10.1016/j.scitotenv.2019.134084
Zekker, I., Kroon, K., Rikmann, E., Tenno, T., Tomingas, M., Vabamae, P., et al. (2012). Accelerating effect of hydroxylamine and hydrazine on nitrogen removal rate in moving bed biofilm reactor. Biodegradation 23, 739–749. doi: 10.1007/s10532-012-9549-6
Zhang, M. M., He, T. X., Chen, M. P., and Wu, Q. F. (2022). Ammonium and hydroxylamine can be preferentially removed during simultaneous nitrification and denitrification by Pseudomonas taiwanensis EN-F2. Biosour. Technol. 350:126912. doi: 10.1016/j.biortech.2022.126912
Zhang, X. X., Xia, Y. K., Wang, C. C., Li, J. J., Wu, P., Ma, L. P., et al. (2020). Enhancement of nitrite production via addition of hydroxylamine to partial denitrification (PD) biomass: functional genes dynamics and enzymatic activities. Biosour. Technol. 318:124274. doi: 10.1016/j.biortech.2020.124274
Zhang, Y., Wang, X. J., Wang, W. Q., Sun, Z. T., and Li, J. (2019). Investigation of growth kinetics and partial denitrification performance in strain Acinetobacter johnsonii under different environmental conditions. R. Soc. Open Sci. 6:191275. doi: 10.1098/rsos.191275
Zhao, B., An, Q., He, Y. L., and Guo, J. S. (2012). N2O and N2 production during heterotrophic nitrification by Alcaligenes faecalis strain NR. Biosour. Technol. 116, 379–385. doi: 10.1016/j.biortech.2012.03.113
Zhao, B., He, Y. L., Hughes, J., and Zhang, X. F. (2010). Heterotrophic nitrogen removal by a newly isolated Acinetobacter calcoaceticus HNR. Biosour. Technol. 101, 5194–5200. doi: 10.1016/j.biortech.2010.02.043
Keywords: nitrogen removal rate, HN-AD, enzyme activities, nitrogen balance, hydroxylamine and nitrite
Citation: Zhang M, He T, Wu Q and Chen M (2023) Efficient detoxication of hydroxylamine and nitrite through heterotrophic nitrification and aerobic denitrification by Acinetobacter johnsonii EN-J1. Front. Microbiol. 14:1130512. doi: 10.3389/fmicb.2023.1130512
Received: 23 December 2022; Accepted: 28 March 2023;
Published: 17 April 2023.
Edited by:
Haihan Zhang, Xi’an University of Architecture and Technology, ChinaReviewed by:
Jingbo Guo, Northeast Electric Power University, ChinaChengmei Liao, Nankai University, China
Copyright © 2023 Zhang, He, Wu and Chen. This is an open-access article distributed under the terms of the Creative Commons Attribution License (CC BY). The use, distribution or reproduction in other forums is permitted, provided the original author(s) and the copyright owner(s) are credited and that the original publication in this journal is cited, in accordance with accepted academic practice. No use, distribution or reproduction is permitted which does not comply with these terms.
*Correspondence: Tengxia He, dHhoZUBnenUuZWR1LmNu