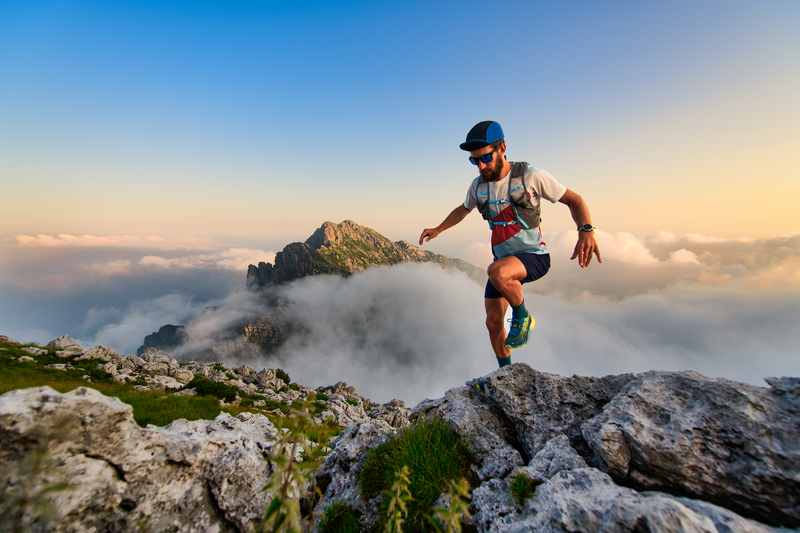
95% of researchers rate our articles as excellent or good
Learn more about the work of our research integrity team to safeguard the quality of each article we publish.
Find out more
ORIGINAL RESEARCH article
Front. Microbiol. , 16 February 2023
Sec. Infectious Agents and Disease
Volume 14 - 2023 | https://doi.org/10.3389/fmicb.2023.1129155
This article is part of the Research Topic Regulation of Virulence Attributes of Pathogenic Fungi and Management Strategies View all 6 articles
The Cdc14 phosphatase family is highly conserved in fungi. In Saccharomyces cerevisiae, Cdc14 is essential for down-regulation of cyclin-dependent kinase activity at mitotic exit. However, this essential function is not broadly conserved and requires only a small fraction of normal Cdc14 activity. Here, we identified an invariant motif in the disordered C-terminal tail of fungal Cdc14 enzymes that is required for full enzyme activity. Mutation of this motif reduced Cdc14 catalytic rate and provided a tool for studying the biological significance of high Cdc14 activity. A S. cerevisiae strain expressing the reduced-activity hypomorphic mutant allele (cdc14hm) as the sole source of Cdc14 proliferated like the wild-type parent strain but exhibited an unexpected sensitivity to cell wall stresses, including chitin-binding compounds and echinocandin antifungal drugs. Sensitivity to echinocandins was also observed in Schizosaccharomyces pombe and Candida albicans strains lacking CDC14, suggesting this phenotype reflects a novel and conserved function of Cdc14 orthologs in mediating fungal cell wall integrity. In C. albicans, the orthologous cdc14hm allele was sufficient to elicit echinocandin hypersensitivity and perturb cell wall integrity signaling. It also caused striking abnormalities in septum structure and the same cell separation and hyphal differentiation defects previously observed with cdc14 gene deletions. Since hyphal differentiation is important for C. albicans pathogenesis, we assessed the effect of reduced Cdc14 activity on virulence in Galleria mellonella and mouse models of invasive candidiasis. Partial reduction in Cdc14 activity via cdc14hm mutation severely impaired C. albicans virulence in both assays. Our results reveal that high Cdc14 activity is important for C. albicans cell wall integrity and pathogenesis and suggest that Cdc14 may be worth future exploration as an antifungal drug target.
CDC14 was originally identified in Saccharomyces cerevisiae as an essential gene required for the final stage of nuclear division (Culotti and Hartwell, 1971). The Cdc14 protein was subsequently characterized as a protein phosphatase that fulfilled its essential function by terminating, and reversing the effects of, mitotic cyclin-dependent kinase (Cdk) activity to trigger mitotic exit (Visintin et al., 1998). CDC14 is widely conserved in the major eukaryotic lineages, with the exception of angiosperm plants (Kerk et al., 2008; Ah-Fong and Judelson, 2011; DeMarco et al., 2020), and Cdc14 catalytic domain structure, activity and specificity are also broadly conserved (Gray et al., 2003; Bremmer et al., 2012; Kobayashi and Matsuura, 2017; DeMarco et al., 2020). For example, human CDC14 orthologs can complement cdc14 mutant phenotypes in S. cerevisiae and Schizosaccharomyces pombe (Li et al., 1997; Vázquez-Novelle et al., 2005).
Cdc14 is related to the dual-specificity phosphatase (DSP) subfamily of protein tyrosine phosphatases (PTP), sharing the invariant HCX5R catalytic motif and two-step catalytic mechanism involving a covalent phospho-enzyme intermediate (Taylor et al., 1997; Wang et al., 2004). Despite its clear evolutionary relationship to the DSP and PTP families, Cdc14 evolved a very strong preference for phosphoserine sites followed by the minimal sequence motif P-X-K (P-X-R to a lesser extent) in an ancestral eukaryote, making it functionally a phosphoserine phosphatase. This unique specificity for a PTP family member arose from an apparent duplication of the DSP domain, which created a novel substrate binding groove along a domain interface adjacent to the active site (Gray et al., 2003; Kobayashi and Matsuura, 2017; DeMarco et al., 2020). The tandem arrangement of DSP folds, and the specificity it imparts, are signature features of Cdc14 enzymes across eukaryotes. The broad conservation implies Cdc14 specificity must fill an essential niche in cellular regulation of most eukaryotic species that cannot be provided by other prominent protein phosphatases. One hypothesis, based on work in the oomycete Phytopthora infestans, is that Cdc14 co-evolved with eukaryotic flagella (Ah-Fong and Judelson, 2011), and subsequent studies have reported roles for Cdc14 enzymes in regulating aspects of cilia structure and function in vertebrates, including human cells (Clement et al., 2011; Imtiaz et al., 2018; Uddin et al., 2019). The fungal subkingdom Dikarya, which includes most fungal pathogens of plants and animals, represents a striking exception to the Cdc14-flagella correlation, as they have strictly retained CDC14 during evolution despite having lost flagella.
Within Dikarya, Cdc14 functional characterizations have been conducted in several species, including the model yeasts S. cerevisiae and S. pombe. Interestingly, the originally described essential function in terminating Cdk activity and triggering mitotic exit in S. cerevisiae is not widely conserved, even in other ascomycetes. In fact, CDC14 deletions in other fungal species often have modest impacts on vegetative cell division (Cueille et al., 2001; Trautmann et al., 2001; Wang et al., 2013; Li et al., 2015, 2018; Yang et al., 2018). Moreover, we and others found that the essential function in S. cerevisiae requires only a small fraction of natural Cdc14 expression (Taxis et al., 2009; Jungbluth et al., 2010; Powers and Hall, 2017). Cdc14 is an abundant phosphatase in S. cerevisiae, with protein levels comparable to subunits of PP2A phosphatases (Wang et al., 2015). The fact that a small fraction of expressed Cdc14 is sufficient for the essential function in S. cerevisiae implies that the majority of Cdc14 is necessary for other important cellular functions.
Cdc14 has been linked to regulation of diverse biological processes in model fungi, including centrosome duplication, mitotic spindle dynamics, chromosome segregation, DNA replication and repair, polarized growth, autophagy, and response to osmotic and genotoxic stresses (Manzano-López and Monje-Casas, 2020). The conservation of many of these functions remains uncertain but it is now becoming clear that a highly conserved function of fungal Cdc14 phosphatases is regulation of cytokinesis and septation at the end of the cell cycle. The S. pombe Cdc14 ortholog, Clp1, does not regulate mitotic exit but plays multiple roles ensuring correct cytokinesis and septation (Trautmann et al., 2001; Clifford et al., 2008). Blocking Cdc14 nuclear export in S. cerevisiae allowed normal mitotic exit regulation but resulted in cytokinesis defects (Bembenek et al., 2005). In the phytopathogenic fungi Fusarium graminearum and Magnaporthe oryzae, CDC14 deletion impaired septum formation and the coordination between nuclear division and cytokinesis, leading to multi-nucleated conidial and hyphal compartments (Li et al., 2015, 2018). Similar septation defects were observed after CDC14 deletion in another plant pathogen, Aspergillus flavus (Yang et al., 2018). Finally, in the opportunistic human pathogen Candida albicans, CDC14 deletion caused defective cell separation after cytokinesis and septation, resulting in connected chains of cells from failure to activate the Ace2 transcription factor (Clemente-Blanco et al., 2006). A number of Cdc14 substrates have been identified and implicated in cytokinesis and septation regulation in S. cerevisiae and S. pombe (Manzano-López and Monje-Casas, 2020b) but the relevant substrates remain unknown in most other fungal species.
Importantly, in studies of CDC14 deletion-associated phenotypes in phytopathogenic fungi, severe reductions in virulence were observed, even when vegetative growth was only marginally impacted (Li et al., 2015, 2018; Yang et al., 2018). The molecular mechanisms by which Cdc14 promotes plant infection are undefined. It is unknown if CDC14 is also important for virulence in animal fungal pathogens, although the CDC14 deletion in C. albicans impaired hyphal development, which is believed to be important for virulence (Lo et al., 1997; Brand, 2011). Collectively, these reports suggest that one or more conserved Cdc14 functions in fungi is generally important for host infection by pathogens.
To better understand fungal Cdc14 functions unrelated to mitotic exit that require high level expression in the model yeast S. cerevisiae, we sought a way to partially reduce Cdc14 activity in vivo. We focused on the poorly conserved, and seemingly disordered, C-terminal tail, which is dispensable for phosphatase activity and the essential mitotic exit function (Taylor et al., 1997). In some Cdc14 orthologs this region has regulatory phosphorylation sites and elements that control cellular localization (Bembenek et al., 2005; Wolfe et al., 2006; Li et al., 2014), but other specific functions have not been described. Of note, the isolated ScCdc14 catalytic domain lacking the C-terminal region exhibited reduced activity toward the generic phosphatase substrate para-nitrophenyl phosphate (pNPP) in an early characterization of Cdc14 enzyme activity (Taylor et al., 1997). We subsequently observed a similar phenomenon with truncated Cdc14 enzymes from F. graminearum (Li et al., 2015) and other fungal species (our unpublished data), suggesting that the C-terminal region does make a conserved contribution to full catalytic activity. Since the existing crystal structures of Cdc14 phosphatases lack the C-terminal tail (Gray et al., 2003; Kobayashi and Matsuura, 2017), it is unclear how this might occur, but it suggests that modulation of the C-terminus might allow creation of reduced activity hypomorphic alleles that are otherwise normal and could be used to study the functional importance of high level Cdc14 expression.
In this report, we identify a short, invariant motif in the disordered C-terminus of fungal Cdc14 enzymes that stimulates Cdc14 activity and is a likely target for in vivo Cdc14 regulation. We characterized mutations in this motif initially in S. cerevisiae, and then more extensively in C. albicans. The results revealed an unexpected new role for Cdc14 in promoting fungal cell wall integrity and demonstrated that high level Cdc14 expression is critically important for pathogenesis in C. albicans, suggesting Cdc14 might be an effective antifungal drug target.
All plasmids and strains used in this study are listed in Supplementary Tables S1, S2, respectively. All engineered strains were confirmed by PCR and DNA sequencing. S. cerevisiae strain YKA1038 expressing cdc14hm in W303 background was created using the delitto perfetto approach exactly as described (Storici and Resnick, 2006). The plasmid shuffle cdc14Δ strain HCY109 and the pRS314 plasmid expressing CDC14 from its natural promoter were gifts from Dr. Harry Charbonneau, Purdue University. To replace wild-type CDC14 in HCY109, cells were transformed with pRS314 expressing either cdc14hm or CDC14 followed by selection on 5-FOA to eliminate the URA3-based pRS316-CDC14 plasmid. C. albicans strain JC2712 was created by integration of CaURA3 at the NEUT5L locus in CAI4. JC2711 was created by deletion of both copies of CDC14 using the URA blaster method (Fonzi and Irwin, 1993) followed by integration of CaURA3 at the NEUT5L locus. C. albicans complementing strains expressing a single-copy of wild-type CDC14-3xHA (JC2721) or cdc14hm-3xHA (HCAL102) were created by transformation of a SalI and EcoRV fragment of pJC347 or pHLP661, respectively, into JC8 (CAI4 cdc14Δ/Δ) and selecting on medium lacking uridine. To create pJC347, the 3’ UTR of CDC14 (+250 to +536 bp after the STOP codon) was PCR-amplified with the addition of SacI and EcoRV sites to the 5′ and 3′ ends, respectively, and cloned into SacI and EcoRV-digested pFA-URA3 (Gola et al., 2003) to generate pFA-URA3-CDC14utr. Then, a synthetic DNA with SalI and BamHI sites added to the 5′ and 3′ ends, respectively, containing 400 bp of CDC14 promoter, the CDC14-3xHA ORF and a 3-UTR with the first 249 bp after the STOP codon of the CDC14 gene was cloned into the SalI and BamHI sites on pFA-URA3-CDC14utr. To create pHLP661, the cdc14hm mutation (encoding 414QPRK➔ 414AARA substitutions) was generated with the QuikChange Lightning site-directed mutagenesis kit (Agilent) using pJC347 as the template and confirmed by DNA sequencing.
Changes in URA3 marker expression are known to impact C. albicans virulence and hyphal differentiation (Lay et al., 1998; Sundstrom et al., 2002; Sharkey et al., 2005). Our strains all have the identical URA3 marker cassette from pFA-URA3 but integrated at 2 different genomic loci (NEUT5L and CDC14, see Table S2). For each locus we have strains with wild-type CDC14 and mutant cdc14 (either cdc14Δ/Δ or cdc14hm/Δ) to control for URA3 expression effects on observed phenotypes. In addition, we used qRT-PCR (see below for method details) to measure relative URA3 transcript levels in strains JC2712, JC2711, JC2721, and HCAL102 (Supplementary Figure S1). No statistically significant differences in transcript levels were observed between URA3 inserted at NEUT5L versus CDC14, demonstrating that all four strains can be compared without concern that observed phenotypes are influenced by locus-specific URA3 expression differences.
The yap1Δ and hog1Δ strains were obtained from the S. cerevisiae haploid deletion library (Horizon Discovery). The yen1Δ mus81Δ S. cerevisiae strain was a gift from Dr. Lorraine Symington, Columbia University. S. pombe strains were a gift from Dr. Kathleen Gould, Vanderbilt University. The cdc14-3 strain was a gift from Dr. Michael Weinreich, Van Andel Research Institute.
Escherichia coli expression plasmids for purification of recombinant Cdc14 enzymes were generated using the Gateway cloning system (Invitrogen). S. cerevisiae CDC14 coding sequence was amplified by PCR from an existing plasmid. C. albicans CDC14 coding sequence was amplified from a cDNA preparation to avoid its intron. Both were cloned into the pENTR/D-TOPO entry vector and then transferred to pDEST17 destination vector following the provided instructions for expression with an N-terminal 6xHis tag. Mutations to the QPRK motif of both were generated by QuikChange mutagenesis. Full coding sequences for all plasmids were verified by DNA sequencing.
Liquid S. cerevisiae and C. albicans cultures were grown in YPD medium (10 g/l yeast extract, 20 g/l peptone, 20 g/l glucose). YPD was supplemented with 40 mg/l adenine (YPAD) for S. cerevisiae W303 strains. Cultures were grown at 30°C with shaking at 225 rpm. S. pombe strains were grown in YES (5 g/l yeast extract, 30 g/l glucose, 225 mg/l adenine, histidine, leucine, uracil, and lysine) at 30°C with shaking at 225 rpm. E. coli were grown in 2xYT (16 g/l tryptone, 10 g/l yeast extract, and 5 g/l NaCl) at 37°C with shaking at 225 rpm. Agar was added to 20% (w/v) for growth on solid medium. For agar plate spotting assays, single colonies were grown to saturation. Strains were serially diluted in eightfold steps starting from OD600 = 1.0 and 5 μl of four consecutive dilutions were spotted on plates and grown at 30°C for 3–5 days. C. albicans cdc14Δ/Δ cultures were diluted one less time than other strains and spots therefore correspond to 8-fold higher absorbance; this was necessary to normalize colony density on untreated control plates. For hyphal induction on solid medium, C. albicans strains were streaked for single colonies on Spider media plates (10 g/l beef broth, 10 g/l mannitol, 2 g/l K2HPO4, 20 g/l agar) or YPD plates supplemented with 10% fetal bovine serum (FBS) (Atlanta Biologicals) and grown at 37°C for 5 days. For liquid hyphal induction, strains were grown overnight to saturation at 30°C and diluted to an OD600 = 0.1 in pre-warmed 37°C YPD containing 10% FBS. Cultures were then incubated at 37°C with shaking at 225 rpm.
Total protein extracts were prepared as described (Kushnirov, 2000; von der Haar, 2007) with subtle modifications. Briefly, 8 ml of mid-log phase cells were treated with 10% trichloroacetic acid and pelleted by centrifugation. Cell pellets were washed with 10 ml 70% ethanol and 2x with 1 ml water, then re-suspended in 1 ml 0.2 M NaOH and incubated 10 min on ice. Cells were pelleted, resuspended in 160 x OD600 μl loading dye (120 mM Tris–HCl pH 6.8, 4% sodium dodecyl sulfate, 0.02% bromophenol blue, 20% glycerol), heated at 95°C for 10 min, and lysates clarified by centrifugation at 16,000 x g. Proteins were separated on 10% tris-glycine SDS-PAGE gels, transferred to 0.45 μm nitrocellulose membranes (Bio-Rad) and probed overnight at 4°C with mouse anti-HA (1:5,000; Sigma-Aldrich, 12CA5), rabbit anti-PSTAIR (1:5,000; Millipore-Sigma, 06–923), rabbit anti-p44/42 MAPK (1:2,500; Cell Signaling Technology, 9102) or rabbit anti-G6PDH (1:5,000; Sigma-Aldrich, A9521). Secondary anti-mouse and anti-rabbit antibodies conjugated to horseradish peroxidase were from Jackson ImmunoResearch (115–035-003 or 111–035-003) and used at 1:10,000 dilution for 60 min at 4°C. Immunoblots were developed using Clarity Western ECL Substrate (Bio-Rad) and imaged on a ChemiDoc MP multimode imager (Bio-Rad).
6xHis-Cdc14 enzymes were purified from E. coli as previously described (DeMarco et al., 2020).
Activities toward varying concentrations of pNPP and synthetic phosphopeptides were assayed as previously described (DeMarco et al., 2020). Data were fit with the Michaelis-Menten equation in GraphPad Prism to determine steady-state kinetic parameters.
Cells were grown to mid-log phase (OD600 ~ 0.8) in YPD and, where indicated, treated with 50 ng/ml micafungin for 1 h prior to RNA extraction. RNA was isolated and purified using acid phenol as described (Serratore et al., 2018; Baker et al., 2022). Reverse transcription was performed using All-in-One 5X RT MasterMix (ABM). Primer Express 3.0 software was used to design primers and qRT-PCR was performed as previously described (Serratore et al., 2018; Baker et al., 2022) with at least three biological replicates. Data were analyzed using the comparative CT method (2-ΔΔCT). Internal controls were RDN18 (18S rRNA) for C. albicans and ACT1 for S. cerevisiae. All samples were normalized to an untreated wild-type strain. Primers used for all qRT-PCR analyses are listed in Supplementary Table S3.
Larvae of G. mellonella were purchased from waxworms.net (St. Marys, OH, United States) and infection assays were performed similar to previous reports (Cotter et al., 2000; Ames et al., 2017). Upon arrival, larvae were held at room temperature in the dark, without food for 24 h before injection. C. albicans strains were grown to saturation in YPD and cells washed three times with PBS, sonicated for 10 s, and counted using a Luna-FL automated cell counter (Logos Biosystems). Larvae (n ≥ 40 per strain) of similar size and color were injected with 6 μl of 2 × 105 cells into their lower left proleg using a Hamilton syringe. An additional group was injected with PBS to serve as a negative control. Injected larvae were incubated in petri dishes at 37°C in the dark for 5 days. Death was measured every 24 h based on the ability of the larvae to flip over after being placed on their backs. Survival data were plotted using the Kaplan–Meier survival curve and statistical analysis was performed using a log-rank Mantel–Cox test.
A mouse model of invasive C. albicans infection of immune-compromised individuals was based on several previously described experimental models (Abruzzo et al., 2000; Cheng et al., 2013; Segal and Frenkel, 2018). BALB/c mice (n = 8 per strain, 4 males and 4 females, 5-week-old) were acclimatized for 7 days upon arrival. On days 8 and 11, mice were rendered neutropenic by intraperitoneal (IP) doses of cyclophosphamide (Segal and Frenkel, 2018); 150 mg/kg on day 8 and 100 mg/kg on day 11. On day 12, 5 × 105 CFUs of a C. albicans strain (measured using a hemocytometer after staining with 0.4% trypan blue to rule out dead cells) in 0.2 ml PBS were administered via IP injection (Cheng et al., 2013). An additional group received cyclophosphamide but was not infected to serve as a negative control. Mice were monitored for morbidity every 6 h and were euthanized upon showing severe signs of illness, including weight loss (>20%), dyspnea, unresponsiveness, staggered gait, and inability to eat. On day 19, surviving mice were humanely euthanized through CO2 inhalation. Lungs, liver, spleen, and kidneys were collected from euthanized mice for histopathology. Survival data were plotted using the Kaplan–Meier survival curve and statistical analysis was performed using a log-rank Mantel–Cox test. Selection of 5 × 105 CFUs of each C. albicans strain was based on a pilot study performed using different doses (5 × 106 to 1 × 104 CFUs) of both wild-type and cdc14Δ/Δ strains.
Lungs, kidneys, and livers from euthanized mice were formalin-fixed, and transferred to 70% ethanol on the next day. Tissues were embedded in paraffin, sectioned, and stained with hematoxylin and eosin (H&E) at the Purdue Histology Research Laboratory for histopathological examination. Tissues were examined by a board-certified veterinary anatomic pathologist, histological features were evaluated, and representative tissue sections were photographed. Fungal pathogens were visualized and evaluated for morphology using periodic acid Schiff (PAS) staining.
Samples were prepared based on a previously published protocol (Wright, 2000) with minor modifications. Day 1: Samples were fixed by adding cell culture to an equal volume of 5% glutaraldehyde in 0.1 M sodium cacodylate buffer. After 5 min cells were pelleted by centrifugation, resuspended in 2.5% glutaraldehyde in the same buffer, and fixed overnight at 4°C. Day 2: Cells were rinsed and resuspended in 2% aqueous potassium permanganate for 1 h, then rinsed until the solution cleared. Cells were then embedded in agarose, en bloc stained in 1% aqueous uranyl acetate for 1 h and rinsed. Day 3: Samples were dehydrated with a graded series of ethanol, then transferred into a 2:1 mixture of ethanol and LR White resin and rotated for 2 h. They were then transferred into a 1:1 mixture of ethanol and LR White resin and rotated overnight. Day 4: Samples were moved into pure LR White resin, rotated for 2 h, then placed under house vacuum. The resin was changed three times throughout the day and the samples remained under vacuum overnight. Day 5: Samples were embedded in LR White resin in gelatin capsules and polymerized at 60°C for 24 h. Thin sections were cut on a Leica UC6 ultramicrotome and stained with 4% uranyl acetate and lead citrate. Images were acquired on a FEI Tecnai T12 electron microscope equipped with a tungsten source operated at 80 kV.
The observation that the isolated catalytic domains of multiple fungal Cdc14 orthologs have reduced enzyme activity raised the possibility of creating hypomorphic mutants to test the importance of full Cdc14 activity. Using S. cerevisiae Cdc14 (ScCdc14), we set out to identify regions of the ~200 amino acid C-terminal tail that specifically contribute to enzyme activity. We purified full length ScCdc14(1–551), the catalytic domain truncation ScCdc14(1–374) and a truncation of intermediate length, ScCdc14(1–449) (Figure 1A; Supplementary Figure S2A) and compared activity under steady-state conditions. Consistent with prior reports (Taylor et al., 1997; Li et al., 2015), ScCdc14(1–374) reaction rate toward pNPP was greatly reduced compared to ScCdc14(1–551) (Figure 1B). ScCdc14(1–449) activity was nearly identical to ScCdc14(1–551), demonstrating that the sequence between amino acids 374 and 449 is required for full activity. We used ScCdc14(1–449) to represent full, or “wild-type,” activity in subsequent experiments. The same analysis with a biologically-relevant phosphopeptide substrate derived from a known ScCdc14 substrate site in Yen1 (Eissler et al., 2014) revealed a similar large reduction in kcat for ScCdc14(1–374) compared to ScCdc14(1–449), indicating that the difference in activity is not substrate-dependent (Figure 1C).
Figure 1. A conserved motif in Cdc14’s disordered C-terminal tail is required for full catalytic activity. (A) Domain map of Cdc14. DSPn and DSPc are the N-and C-terminal dual-specificity phosphatase domains, respectively. CX5R is the invariant PTP family catalytic motif. Sites of truncations and the invariant QPRK motif are indicated in the disordered C-terminus following DSPc. (B) Steady-state kinetic analyses with full length ScCdc14 (1–551) and the indicated truncation variants toward pNPP yielded kcat values of 0.91 (1–551), 0.80 (1–449), and 0.04 (1–374) sec−1. (C) Same as (B) with phosphopeptide substrate HT[pS]PIKSIG, yielding kcat values of 1.21 (1–449) and 0.01 (1–374) sec−1. (D) Multiple sequence alignment of fungal Cdc14 orthologs from the indicated species was generated by Clustal Omega using default settings. Only a portion of the C-terminal region showing the invariant QPRK motif is shown. (E) Steady-state kinetic analyses comparing wild-type ScCdc14 and QPRK motif mutants towards pNPP; kcat values were 0.02 (1–449QARA) and 0.03 (1–449APRK) sec−1. (F) Same as (E) with the phosphopeptide substrate; kcat values were 0.02 (1–449QARA) and 0.03 (1–449APRK) sec−1. The ScCdc14 (1–449) data in panels (E,F) are identical to panel (C). All kinetic data in panels (B,C,E,F) are averages of at least 3 independent trials with standard deviation error bars. Fit lines and kcat values were generated in GraphPad Prism using the Michaelis–Menten function.
A multiple sequence alignment of diverse fungal Cdc14 orthologs revealed a single, short invariant sequence within the otherwise poorly conserved C-terminus (Figure 1D) that was not observed in animal Cdc14 sequences (Supplementary Figure S3). Part of this sequence, QPRK, strongly resembles the optimal substrate recognition motif of Cdc14 enzymes, SPxK (“x” is preferentially a basic amino acid) (Bremmer et al., 2012; Eissler et al., 2014; Kobayashi and Matsuura, 2017), suggesting it could influence catalysis by interacting with the active site as a pseudosubstrate. Furthermore, this motif is within residues 374–449 required for full activity. We substituted Ala either for the Pro and Lys residues, analogous to two critical positions for Cdc14 substrate recognition, or for the Gln, since this residue would occupy the pSer binding pocket if the motif were acting as a pseudosubstrate. The resulting ScCdc14(1-449QARA) and ScCdc14(1-449APRK) variants exhibited kcat decreases similar to ScCdc14(1–374) with both pNPP (Figure 1E) and phosphopeptide (Figure 1F) substrates. Mutation of QPRK did not affect substrate specificity as activity was still dependent on the same major substrate features as wild-type Cdc14 enzymes (Supplementary Figure S2B). We conclude the QPRK motif makes a significant contribution to enzyme turnover rate and is responsible for the observed rate difference between catalytic domain truncations and full-length enzymes.
The strict conservation of the QPRK motif across the fungal kingdom suggests it is biologically important. To characterize its biological significance, we generated a haploid S. cerevisiae strain expressing the hypomorphic cdc14QARA allele (hereafter called cdc14hm) as the sole source of Cdc14 from the natural CDC14 locus using the delitto perfetto approach (Storici and Resnick, 2006). In liquid culture, the mutant strain grew at a similar rate to the wild-type parental strain at low cell density but slowed somewhat as cell density increased and ultimately terminated at a slightly lower optical density (Supplementary Figure S4A). Thus, the essential mitotic exit function was not disrupted by the reduction in Cdc14 catalytic rate, consistent with our prior observations that it requires a small fraction of normal Cdc14 activity (Powers and Hall, 2017). We performed agar plate spotting assays in the presence of a variety of cellular stress conditions to determine if reducing Cdc14 activity sensitizes cells to stress. cdc14hm colony size was slightly smaller than wild-type on untreated control plates but otherwise grew similarly and cdc14hm was no more sensitive than wild-type to genotoxic, oxidative, and osmotic stresses (Figure 2A; Supplementary Figures S4B–D). The temperature-sensitive cdc14-3 allele was previously shown to impart some sensitivity to calcofluor white (CFW), a chitin-binding compound that causes cell wall stress (Breitkreutz et al., 2010). We confirmed this observation and found that cdc14hm exhibited strong sensitivity to CFW (Figure 2B) and another chitin-binding compound, Congo red (Supplementary Figure S4E). To determine if this sensitivity was unique to chitin binding chemicals we performed spotting assays in the presence of echinocandin drugs, which cause cell wall stress by inhibiting synthesis of the major cell wall polymer (1,3)-β-D-glucan (Sucher et al., 2009). cdc14hm was acutely sensitive to both micafungin and caspofungin compared to wild-type (Figures 2C,D). cdc14hm was also sensitive to growth at high temperature, another condition that generates cell wall stress (Fuchs and Eleftherios, 2009; Supplementary Figure S4F). Cell wall stress sensitivity was observed when cdc14hm, but not CDC14, was expressed from a CEN plasmid in a cdc14Δ background (Supplementary Figure S4G). Sensitivity in cdc14hm was complemented by transformation with a CEN plasmid expressing wild-type CDC14 (Supplementary Figure S4H), suggesting it is a recessive loss of function phenotype caused by the reduced activity. Micafungin sensitivity was rescued in the presence of sorbitol, an osmotic stabilizer known to suppress cell wall integrity defects (Torres et al., 1991), suggesting that reduced Cdc14 activity compromises cell wall integrity (Figure 2E).
Figure 2. QPRK mutation causes sensitivity to cell wall stress in S. cerevisiae. (A–E) Cultures of the indicated S. cerevisiae strains were serially diluted and spotted on YPAD agar plates containing indicated concentrations of (A) methyl methanesulfonate (MMS), (B) calcofluor white (CFW), (C) micafungin (MF), (D) caspofungin (CF), and (E) MF or MF + sorbitol. “#1” and “#2” refer to independent isolates of cdc14hm. yen1Δ mus81Δ is a positive control for MMS sensitivity (Ho et al., 2010). All plates were incubated at 30°C for 72 h. Images are representative of three biological replicates. “Wild-type” is W303. (F) Immunoblot detecting phosphorylated, active form of Slt2 (pSlt2) from cell lysates of mid-log phase cells with anti-p44/42 MAP kinase antibody. pSlt2 signals were corrected based on the G6PDH (glucose-6-phosphate dehydrogenase) load control and then normalized to the untreated wild-type sample to generate the Relative pSlt2 value, which is an average of two independent trials.
Cell wall damage activates the cell wall integrity (CWI) signaling pathway (Levin, 2011). Activation of the CWI pathway can be monitored by immunoblotting for the phosphorylated CWI MAP kinase, Slt2. We compared phospho-Slt2 levels in log phase wild-type and cdc14hm cultures in the absence and presence of micafungin. cdc14hm showed a 3-fold increase in phospho-Slt2 in the absence of micafungin, but both wild-type and cdc14hm strains showed similar Slt2 activation after brief treatment with micafungin (Figure 2F). We also monitored expression of FKS2, which encodes a catalytic subunit of (1,3)-β-D-glucan synthase that is induced by CWI signaling during cell wall stress (Mazur et al., 1995; Zhao et al., 1998; Jung and Levin, 1999). Consistent with the pSlt2 result, FKS2 transcript level was twofold higher in cdc14hm compared to the isogenic wild-type strain in the absence of micafungin (Supplementary Figure S4I). These results are consistent with a cell wall integrity defect, but mostly normal CWI signaling, in cdc14hm S. cerevisiae cells.
To test if Cdc14-dependent sensitivity to cell wall stress is conserved in other fungal species, we performed spotting assays with CDC14 deletion strains of S. pombe and C. albicans. S. pombe clp1Δ exhibited clear sensitivity to caspofungin, but not calcofluor white, presumably due to the lack of chitin in S. pombe cell walls (Pérez et al., 2018; Figure 3A). C. albicans cdc14Δ/Δ exhibited strong sensitivity to calcofluor white, caspofungin, and micafungin (Figure 3B). These observations suggest that Cdc14 makes a broadly conserved contribution to fungal cell wall integrity.
Figure 3. Cdc14-dependent cell wall stress sensitivity is conserved in other fungal species. Liquid cultures of the indicated S. pombe (A) and C. albicans (B) strains were serially diluted and spotted on YES or YPD agar plates, respectively, containing the indicated concentrations of micafungin (MF), caspofungin (CF), or calcofluor white (CFW). CLP1 is the S. pombe homolog of CDC14. (C) Steady-state kinetic analysis of CaCdc14 (1–427) and CaCdc14 (1–427AARA) using the Yen1 phosphopeptide substrate. kcat values were 0.092 (1–427) and 0.015 (1–427AARA) sec−1. Data are means from three independent trials and error bars are standard deviations. Liquid cultures of the indicated C. albicans strains were serially diluted and spotted on YPD agar plates containing the indicated concentrations of micafungin (D) or caspofungin (E). Plates were incubated at 30°C for 3–5 days (until mature colonies were visible in the wild-type strain). Images are representative of three independent trials.
We next tested if the QPRK motif functions similarly in C. albicans Cdc14 (CaCdc14) and if mutating it is sufficient to cause cell wall stress sensitivity, as in S. cerevisiae. We purified recombinant truncated CaCdc14 (residues 1–427, which retains the QPRK motif), as full-length protein was insoluble, and created a variant with Ala substitutions at the Gln, Pro, and Lys positions of the QPRK motif (Supplementary Figure S5A). CaCdc14(1–427) exhibited the same major substrate preferences as ScCdc14 and other Cdc14 orthologs (Supplementary Figure S5B), consistent with its identical active site composition (Supplementary Figure S3). Steady-state kinetic analyses with the Yen1 phosphopeptide substrate revealed a 6-fold reduction in kcat for CaCdc14(1–427AARA) compared to CaCdc14(1–427) (Figure 3C). Thus, the QPRK motif makes a similar, albeit somewhat weaker, contribution to CaCdc14 phosphatase activity.
To study the biological significance of the QPRK motif in C. albicans we complemented cdc14Δ/Δ with either cdc14hm-3xHA (where cdc14hm encodes the QPRK➔AARA substitutions) or CDC14-3xHA (Supplementary Figure S5C). cdc14hm growth rate was intermediate between the wild-type and cdc14Δ/Δ strains, consistent with a partial loss of activity and function (Supplementary Figure S5D). In agar plate spotting assays cdc14hm/Δ displayed hypersensitivity to a range of echinocandin concentrations, similar to cdc14Δ/Δ, whereas CDC14/Δ behaved like the CDC14/CDC14 wild-type parent strain (Figures 3D,E). Thus, echinocandin hypersensitivity requires only a partial reduction in Cdc14 activity. As in S. cerevisiae, hypersensitivity was specific to cell wall stress as cdc14Δ/Δ and cdc14hm/Δ were mostly insensitive to genotoxic, oxidative, and osmotic stress (Supplementary Figure S5E).
We next tested if CWI signaling was altered in Cdc14-deficient C. albicans strains, similar to S. cerevisiae. We used the same anti-p44/42 MAPK antibody used to detect the phosphorylated, active form of the CWI MAP kinase and Slt2 ortholog, Mkc1, by immunoblotting. In the absence of added stress, liquid cultures of both cdc14Δ/Δ and cdc14hm/Δ displayed an equal elevation in active Mkc1 compared to CDC14/CDC14 (Figures 4A,B). Interestingly, CDC14/Δ cells displayed an intermediate elevation in phospho–Mkc1 signal, suggesting that even CDC14 haplo-insufficiency can cause some chronic cell wall abnormality. The reason for this is unclear, but it suggests that CWI is particularly sensitive to Cdc14 activity level in C. albicans. In contrast to the basal state, when cultures were briefly challenged with micafungin, activated Mkc1 was significantly lower in both cdc14Δ/Δ and cdc14hm/Δ compared to CDC14/CDC14 and CDC14/Δ (Figures 4A,B). This suggests that Cdc14 activity may also play a role in normal CWI pathway activation in response to certain cell wall stress signals.
Figure 4. Reduced Cdc14 activity causes elevated CWI signaling and perturbs cell wall gene expression in C. albicans. (A) Phosphorylation of Mkc1 (pMkc1) was detected in whole cell extracts from mid-log phase cultures of the indicated C. albicans strains by anti-p44/p42 MAP kinase immunoblotting. Saturated cultures were back-diluted to OD600 = 0.01 and grown to mid-log phase. A portion of the untreated culture was collected and the remainder was treated with 50 ng/ml micafungin (MF) for 30 min. Anti-PSTAIR is a loading control. (B) pMkc1 chemiluminescence signals from (A) were normalized to PSTAIR and plotted as a fold-increase above the untreated wild-type signal. Values are means from three independent experiments and error bars are standard deviations. Unpaired T-tests assuming equal variance comparing pMkc1 level in each strain to wild-type were used to determine statistical significance – *p < 0.05; ns, not significant (p > 0.05). (C–F) Relative mRNA levels of selected cell wall stress-responsive genes in mid-log phase cultures of C. albicans strains either untreated (−) or treated (+) with 50 ng/ml MF for 1 h were measured by qRT-PCR. Transcript levels were normalized to RDN18 and set relative to the untreated wild-type sample. Data are averages of at least five biological trials and error bars are standard deviations. Outliers were identified by Grubbs test and removed if detected. Unpaired T-tests assuming equal variance were used to compare (1) the basal (untreated) expression in each strain with wild-type (†p < 0.05) and (2) untreated with MF-treated samples of each strain. If a significant increase was observed with MF treatment, then the average fold-increase was added above the bar and an additional T-test was performed to determine if fold-increase was significantly different than wild-type fold-increase (*p < 0.05). ns, not significant (p > 0.05).
To test if Cdc14 activity affects gene expression downstream of Mkc1 we used qRT-PCR to measure transcript levels of several cell wall stress-responsive genes (Ibe and Munro, 2021) in the absence and presence of micafungin. Consistent with the phospho-Mkc1 immunoblotting results, ECM331, PGA13, and PGA31 transcripts were significantly elevated in cdc14Δ/Δ and/or cdc14hm/Δ strains compared to CDC14/CDC14 and CDC14/Δ in the absence of micafungin, although CRH11 showed no change (Figures 4C–F). Interestingly, after brief micafungin treatment cdc14Δ/Δ cells failed to induce expression of any of the four genes and the fold-induction in cdc14hm/Δ was significantly reduced compared to wild-type strains. This effect was not due to the genes already being maximally induced since three out of four transcript levels were lower in micafungin-treated cdc14Δ/Δ and cdc14hm/Δ compared to CDC14/CDC14 and CDC14/Δ. Thus, Cdc14 activity is important for expression of at least some cell wall stress-responsive genes, and our results collectively imply a complex relationship between Cdc14 activity and cell wall integrity signaling in C. albicans (see section Discussion).
We used TEM to look for overt differences in cell wall structure between wild-type and Cdc14-deficient C. albicans yeast form cells that could explain the observed cell wall integrity phenotypes. The most striking difference was the structure of the septum in dividing cells. In CDC14/CDC14 and CDC14/Δ cells the primary septum was uniformly visible as a bright, straight band bisecting the bud neck, with thin layers of secondary septum on either side (Figures 5A,B), as expected (Meitinger and Pereira, 2016). Relatively normal primary septa were also observed in some cdc14hm/Δ and cdc14Δ/Δ cells, however we frequently observed primary septa with abnormal morphologies in these strains that were never observed in wild-type cells (Figures 5C,D). The primary septa were often discontinuous, non-linear, forked, or deviated from the bud neck. Moreover, the secondary septa in cdc14hm/Δ and cdc14Δ/Δ were often thickened, and in some cases appeared to lack primary septa altogether. These secondary septa were reminiscent of previously described remedial septa that form in the absence of primary septum assembly (Walker et al., 2013). From the TEM analysis we conclude that high Cdc14 activity is critical for proper septum assembly during cell division.
Figure 5. Reduced Cdc14 activity impairs C. albicans septum assembly and cell separation. (A–D) Transmission electron micrographs of C. albicans yeast form cells of the indicated strains undergoing septation in mid-log phase liquid cultures. PS, primary septum; SS, secondary septum. (E) Light microscopy images of log phase cultures of the indicated strains.
Previous characterization of cdc14Δ/Δ C. albicans revealed defects in separation of yeast form cells following cell division, resulting in formation of large cell clumps (Clemente-Blanco et al., 2006). This phenotype was linked to regulation of the Ace2 transcription factor that controls expression of cell separation genes following cytokinesis and septation. In liquid cultures cdc14hm/Δ also formed large cell clumps that were indistinguishable from cdc14Δ/Δ, whereas CDC14/Δ did not (Figure 5E). The clumps were dispersed by sonication, consistent with normal completion of cytokinesis, but incomplete dissolution of septa. In asynchronously growing cultures we detected similar reductions in the transcript levels of Ace2 target genes in cdc14hm/Δ and cdc14Δ/Δ by qRT-PCR (Supplementary Figure S6). This suggests that high Cdc14 activity is also needed to fully activate Ace2. Thus, CaCdc14 appears to play critical roles in both the assembly and dissolution of the septum during yeast form cell division. Septum defects could, in principle, contribute to the impaired cell wall integrity of Cdc14-deficient cells.
Candida albicans cdc14Δ/Δ was also previously reported to be defective in hyphal differentiation and invasive growth on agar plates (Clemente-Blanco et al., 2006). We therefore tested if cdc14hm/Δ also exhibits these phenotypes. On Spider medium plates, cdc14Δ/Δ and cdc14hm/Δ were completely unable to develop filamentous colonies and exhibited very limited agar invasion (Figure 6A). Microscopic analysis of cells extracted from the Spider agar after washing of the plate surface revealed that even invasive cells exhibited a severe reduction in the number and length of hyphae compared to strains with wild-type CDC14 (Figure 6B). Similar lack of invasion and formation of hyphae by cdc14Δ/Δ and cdc14hm/Δ were also observed on YPD-serum agar plates (Supplementary Figures S7A,B). In liquid cultures, hyphal differentiation differences between the strains were more subtle. Both cdc14Δ/Δ and cdc14hm/Δ exhibited hyper-polarized growth consistent with hyphae, but at 2 h after serum induction their elongated cells were shorter on average and displayed more pseudohyphal character than those of the wild-type CDC14 strains (Supplementary Figure S7C). We conclude that normal hyphal development also requires high CDC14 activity.
Figure 6. Reduced Cdc14 activity impairs hyphal development on solid medium. (A) The indicated C. albicans strains were grown on Spider medium agar plates at 37°C for 5 days. Plates were imaged before and after vigorous washing of unattached cells off the surface with water. After washing, agar plugs were removed and cross sections were imaged to illustrate depth of invasive growth. (B) Pieces of the agar plugs from (A) were manually ground into small pieces, which were then mounted on a microscopy slide, compressed under a cover slip, and images of embedded cells captured by DIC microscopy.
Hyphal differentiation is thought to be important for C. albicans virulence (Lo et al., 1997; Kadosh, 2019), suggesting that reduced Cdc14 activity might compromise pathogenesis. We tested this idea in two established animal models of invasive candidiasis, the larvae of the wax moth Galleria mellonella (Cotter et al., 2000; Fuchs et al., 2010; MacCallum, 2011; Segal and Frenkel, 2018), and immunosuppressed mice (Hohl, 2014). In G. mellonella, cdc14Δ/Δ and cdc14hm/Δ exhibited dramatically reduced virulence compared to CDC14/CDC14 and CDC14/Δ (Figure 7A). In mice the virulence defect associated with reduced Cdc14 was even more dramatic. An initial titration of fungal dose revealed cdc14Δ/Δ to be almost completely avirulent even at the highest fungal titer (Supplementary Figure S8A,B). At the minimum CDC14/CDC14 dose required for complete mortality, cdc14hm/Δ failed to kill any mice and, surprisingly, CDC14/Δ resulted in 50% mortality, killing only the female mice, suggesting CDC14 heterozygosity is sufficient to impact virulence and that there may be a gender bias in sensitivity to Cdc14 deficiency (Figure 7B).
Figure 7. Loss or reduction of Cdc14 function impairs pathogenesis of C. albicans. (A) G. mellonella larvae or (B) immunosuppressed BALB/c mice were infected with 2 × 105 or 5 × 105 C. albicans cells of the indicated strains, respectively, or mock-uninfected. p-values were determined using a log-rank (Mantel–Cox) test comparing the survival distribution of hosts infected with wild-type C. albicans to each experimental strain. (C–E) Histopathological analysis of tissue sections from mouse organs harvested after completion of experiment in (B). In (C) fungal cells were stained with periodic acid-Schiff (PAS) in representative tissue sections to illustrate morphology. In (D,E) liver and lung tissues, respectively, were stained with H&E. All images were acquired at 200x magnification, except the CDC14/Δ sample in (D), which was imaged at 20x to illustrate the necrotic lesion with surrounding inflammation. The cdc14Δ/Δ liver section in (D) illustrates one of the rare lesions [associated with the cluster of fungal cells shown in (C)] found in mice infected with cdc14 mutant strains. In (D,E) the following shock-related lesions (lacking invading fungal cells) in mice infected with CDC14/CDC14 and CDC14/Δ strains are labeled: endothelial leakage characterized by hepatic (D) or pulmonary alveolar (E) edema (a), multifocal hemorrhage and congestion (b), and intravascular microthrombi (c). Recruited inflammatory cells (d) and necrotic lesions (e) are also labeled. H&E-stained liver and lung sections of an uninfected mouse can be found in Supplementary Figure S8C for comparison.
Histological analysis of mouse organs following infection revealed extensive colonization by predominantly hyphal cells of CDC14/CDC14 and CDC14/Δ strains, resulting in inflammation and necrosis, as expected (Figures 7C–E; Supplementary Figure S8C). In contrast, minimal colonization and necrosis were observed after infection by cdc14hm/Δ and cdc14Δ/Δ strains and the rare fungal cells that were detected were almost completely yeast form, consistent with the hyphal differentiation defects observed on agar plates. In mice infected with wild-type C. albicans, organs like the liver and lungs showed evidence of shock prior to appearance of fungal cells. Lesions consistent with shock included endothelial leakage characterized by edema fluid, multifocal hemorrhage and congestion, and occasional intravascular microthrombi (Figures 7D,E). These were not observed in the organs of mice infected with cdc14hm/Δ or cdc14Δ/Δ, raising the possibility that Cdc14 promotes secretion of soluble factors that contribute to vascular damage. These results show that high Cdc14 activity is critically important for C. albicans pathogenesis.
Our results identify Cdc14 phosphatase as a novel virulence factor in C. albicans and reveal a new and conserved contribution of Cdc14 to fungal cell wall integrity. Importantly, partial loss of Cdc14 activity through mutation of a conserved catalytic enhancer element in the disordered C-terminal region was sufficient to render C. albicans hypersensitive to cell wall stress, and impair septation, hyphal development, and pathogenesis. Since C. albicans virulence depends on hyphal differentiation (Lo et al., 1997; Brand, 2011; Kadosh, 2019), the pathogenesis phenotype could be explained by the hyphal defect of Cdc14-deficient strains. However, CaCdc14 function in septation and cell wall integrity may contribute to virulence as well, a possibility that will require future testing. We speculate that the observed cell wall integrity and septation defects reflect a general importance of high level Cdc14 expression across fungi.
Cdc14 has been implicated in regulation of septation and cell separation previously. In S. cerevisiae Cdc14 promotes delivery of the chitin synthase Chs2, which synthesizes the primary septum, to the bud neck after completion of mitosis (Chin et al., 2012). It also regulates actomyosin ring assembly (Miller et al., 2015) and the ingression progression complex that coordinates actomyosin ring contraction and membrane ingression with Chs2-dependent septum assembly (Meitinger et al., 2010; Palani et al., 2012; Foltman et al., 2016). Mutations in the Mitotic Exit Network, which activates ScCdc14, lead to septum structural defects (Meitinger et al., 2010). In the phytopathogenic fungi Fusarium graminearum, Magnaporthe oryzae, and Aspergillus flavus, CDC14 deletion reduces the number of septa in conidia and vegetative hyphae and in M. oryzae perturbs correct localization of appressoria-delimiting septa (Li et al., 2015, 2018; Yang et al., 2018). Despite the evidence that Cdc14 is important for normal septation, our results appear to be the first to show that Cdc14 deficiency causes structural defects in primary septa. Cdc14 is also important for dissolving septa to allow cell separation. ScCdc14 dephosphorylates and activates Ace2 to induce cell separation genes (Brace et al., 2011; Powers et al., 2017), and preventing ScCdc14 nuclear export or reducing Cdc14 level via inducible degradation resulted in cell separation failure (Bembenek et al., 2005; Powers and Hall, 2017). Cdc14 is also needed to activate Ace2 to promote cell separation in C. albicans (Clemente-Blanco et al., 2006). Septation and cell separation defects could be a primary cause of the cell wall stress sensitivity observed in Cdc14-deficient C. albicans, although direct evidence of such a causal relationship is still missing.
In addition to causing a cell wall structural defect, our results suggest that Cdc14 deficiency impacts CWI signaling in C. albicans in a complex way. Despite the elevated CWI pathway activation in unstressed Cdc14-deficient strains (including heterozygous CDC14/Δ), cdc14Δ/Δ and cdc14hm/Δ exhibited reduced stimulation of phospho-Mkc1 following micafungin treatment. This suggests that Cdc14 deficiency both causes cell wall damage and also plays a role in activating the response to this damage. Consistent with this idea, Cdc14-deficient strains displayed modest up-regulation of a subset of known cell wall stress-responsive genes in the absence of added stress (in line with elevated phospho-Mkc1) but were defective in stimulating expression of these genes in response to micafungin exposure. The extent of this defect correlated with the severity of Cdc14 deficiency; cdc14Δ/Δ showed no micafungin-stimulated expression of the four cell wall stress-responsive genes we tested. These results are somewhat difficult to reconcile but are most consistent with Cdc14 influencing CWI signaling at steps upstream and downstream of Mkc1. Consequently, the cell wall stress sensitivity phenotype could be explained by a Cdc14-dependent cell wall structural defect, a deficiency in the CWI signaling response to the cell wall defect, or both. The fact that CDC14/Δ cells exhibited increased basal phospho-Mkc1 but normal CWI signaling and transcriptional response after micafungin treatment reinforces the idea that Cdc14 functions in multiple capacities to promote cell wall integrity that are differentially sensitive to its activity level. We surveyed a small subset of cell wall stress-responsive genes, and a complete picture of how Cdc14 impacts the response to cell wall stress will ultimately require global approaches like RNA-seq.
Cdc14 was previously linked to several fungal stress pathways. S. pombe Clp1 and ScCdc14 are released from the nucleolus and activated in response to genotoxic stress (Diaz-Cuervo and Bueno, 2008; Broadus and Gould, 2012; Villoria et al., 2017). A global phosphatase and kinase interaction network analysis revealed multiple interactions of ScCdc14 with stress signaling MAP kinases (Breitkreutz et al., 2010) and other studies have reported connections between Cdc14 and the osmotic stress response mediated by the Hog1 MAP kinase (Chasman et al., 2014; Tognetti et al., 2020). In the phytopathogen A. flavus, a cdc14Δ strain exhibited growth delays in the presence of chitin-binding compounds and osmotic stress (Yang et al., 2018). In the entomopathogen Beauveria bassiana, cdc14Δ displayed modest sensitivity to multiple stresses, including the chitin-binding Congo red (Wang et al., 2013). These studies imply that fungal Cdc14 may play diverse roles in facilitating fungal stress responses, although we emphasize that cdc14hm/Δ C. albicans with partial loss of Cdc14 activity exhibited a very specific sensitivity to cell wall stress. An area for future investigation will be determining if the cell wall stress hypersensitivity of Cdc14-deficient strains is related to the virulence defect. Specific mutations that affect Cdc14 function in cell wall integrity would facilitate this and could be enabled by a thorough understanding of Cdc14 regulation during cell division, in response to cell wall stress, and during hyphal development.
Our results reinforce the fact that different functions of Cdc14 require different levels of activity. In S. cerevisiae the essential mitotic exit function requires a small fraction of normal Cdc14 activity (Powers and Hall, 2017). Why would functions in cytokinesis and cell wall integrity require much higher Cdc14 activity? In S. cerevisiae the essential function is fulfilled by nuclear Cdc14 (Bembenek et al., 2005) whereas cytokinesis functions require cytoplasmic Cdc14 generated by the Mitotic Exit Network kinases (Sanchez-Diaz et al., 2012). One possibility is that cytoplasmic functions require more enzyme because of the greater volume relative to the nucleus, which effectively dilutes Cdc14 concentration. In C. albicans Cdc14 localization is also cell-cycle regulated. It is primarily nuclear prior to mitosis, then appears at the spindle pole bodies during early mitosis, and finally becomes cytoplasmic and localizes to the bud neck in late mitosis (Clemente-Blanco et al., 2006). A second possibility is that the mitotic exit function requires minimal activity to trigger a positive feedback system (López-Avilés et al., 2009), whereas the cytokinesis, septation, and cell wall integrity functions depend on bulk substrate dephosphorylation that necessitates higher Cdc14 concentration. A third possibility is that Cdc14 has high affinity for mitotic exit substrates that must be dephosphorylated relatively early after Cdc14 activation and lower affinity for substrates involved in later processes like cytokinesis and septation, resulting in a need for higher Cdc14 activity to achieve their dephosphorylation. There is evidence that substrate affinity does influence the order of Cdc14 substrate dephosphorylation during mitotic exit (Kataria et al., 2018), providing some support for this possibility.
Regardless of the true explanation, it appears clear that Cdc14 activity levels in C. albicans and S. cerevisiae are physiologically important. Even heterozygous CDC14/cdc14Δ cells exhibited some phenotypes, most notably the reduction in virulence in the mouse infection assay. We speculate that the invariant QPRK motif in the C-terminus of fungal Cdc14 enzymes exists to provide a mechanism for regulating Cdc14 activity level around a critical threshold. Although the need for this mechanism is still unclear, results from both S. cerevisiae and S. pombe support its existence. A C-terminal Cdk phosphorylation site in ScCdc14 was previously shown to inhibit enzyme activity by an unknown mechanism (Li et al., 2014). This site happens to be adjacent to the QPRK motif and also appears to be essentially invariant in fungi (although its exact position relative to QPRK varies, see alignment in Figure 1D). In S. pombe Clp1 phosphorylation by Cdk1 at C-terminal sites (including the one adjacent to its QPRK motif) also reduces enzymatic activity and is required for normal kinetics of mitotic progression (Wolfe et al., 2006). Thus, it appears likely that phosphoregulation of QPRK motif function is a mechanism for controlling overall Cdc14 activity level in fungal species. It will be interesting to test if phosphorylation adjacent to the QPRK motif of CaCdc14 also regulates enzyme activity and if dephosphorylation is required to promote CaCdc14 function in septation, hyphal development, and the response to cell wall stress.
While a number of substrates of ScCdc14 and SpCdc14 involved in regulation of cytokinesis and/or septation have been identified (Mocciaro and Schiebel, 2010; Meitinger et al., 2012; Chen et al., 2013; Kuilman et al., 2015), it remains unknown what substrates of CaCdc14 must be dephosphorylated to promote normal septum assembly, cell wall integrity, and hyphal differentiation. Understanding how Cdc14 influences these processes will require identification of the relevant direct substrates. A few candidate substrates that may be relevant to our observed phenotypes exist. Evidence suggests that Ace2 is a CaCdc14 substrate likely related to the observed cell separation defects in Cdc14-deficient strains (Clemente-Blanco et al., 2006). A previous proteomic study of CaCdc14 interacting proteins and candidate substrates did not identify proteins with obvious connections to septum assembly but did identify Ace2, the chitinase Cht4, and a couple components of the actomyosin contractile ring (Kaneva et al., 2019), although these were not validated as substrates. The Nim1 kinase, Gin4, which helps organize the septin scaffold at the bud neck that is crucial for proper septation and cytokinesis is dephosphorylated by CaCdc14 (Au Yong et al., 2016) and this could potentially be related to the septum structure and cell wall integrity defects observed here. Still, our current knowledge of Cdc14 substrates in C. albicans is very limited and it is likely that CaCdc14 dephosphorylates multiple targets that influence cell wall integrity, septum assembly, and pathogenesis.
The observation that partial reduction in Cdc14 activity severely impairs pathogenesis in animal models of invasive candidiasis suggests that Cdc14 might be a useful antifungal drug target where even modest therapeutic inhibition of activity could successfully combat infections. Moreover, the fact that reduced Cdc14 activity sensitizes C. albicans to echinocandin drugs raises the possibility that Cdc14 inhibitors could be valuable for combating clinical echinocandin resistance. We argued previously that the Cdc14 active site should be amenable to specific inhibitor development (DeMarco et al., 2020). High resolution structural information on substrate recognition by the fungal Cdc14 active site is available (Kobayashi and Matsuura, 2017), and despite sharing a common catalytic mechanism with PTPs, Cdc14 possesses a strict and unusual substrate specificity that could be mimicked for effective and highly selective inhibitor development. In animals, deletion of CDC14 has little impact on cell division and development (Saito et al., 2004; Berdougo et al., 2008; Mocciaro et al., 2010) and the possibility of successful therapeutic intervention by partial inhibition of Cdc14 activity further minimizes the likelihood of deleterious effects on human cells. The Cdc14 active site region is invariant across the fungal kingdom (DeMarco et al., 2020) and therefore inhibitors developed to target CaCdc14 would likely be effective at inhibiting Cdc14 orthologs from many other pathogens. For this reason, it will be useful in the future to determine if Cdc14 is similarly required for infection by other human pathogens.
The original contributions presented in the study are included in the article/Supplementary material, further inquiries can be directed to the corresponding author.
The animal study was reviewed and approved by Purdue University Institutional Animal Care and Use Committee.
MH conceived the study. MH, KM, SB, JC-B, SN, and AA designed portions of the study. KM and MH designed and wrote the manuscript. SB, JC-B, AA, and SN contributed manuscript sections and helped edit the manuscript. KM, AA, KB, SH, AD, NN, AK, GL, KA, AE-M, JC-B, and MH performed the experiments and/or provided essential reagents. KM, AA, KB, SH, AD, SN, JC-B, SB, and MH analyzed and interpreted results. All authors have approved the final manuscript version.
This study was supported by grants from the National Institutes of Health AI136995 (SB) and NIFA 1007570 (SB). This work was funded in part by a grant from the Purdue University AgSEED program (MH and SB). MH was supported, in part, by the Indiana Clinical and Translational Sciences Institute funded, in part by Award Number UL1TR002529 from the National Institutes of Health, National Center for Advancing Translational Sciences, Clinical and Translational Sciences Award. The content is solely the responsibility of the authors and does not necessarily represent the official views of the National Institutes of Health. JC-B was supported by grants PID2020-118109RB-I00 funded by MCIN/AEI/10.13039/501100011033 and GR21117 of the Regional Government of Extremadura and the European Regional Development Fund. AE-M is the recipient of a pre-doctoral fellowship from the Junta de Extremadura, financed by the European Social Fund (ESF), Ref. PD18066.
We thank Kathleen Gould (Vanderbilt University), Harry Charbonneau (Purdue University), Michael Weinreich (Van Andel Research Institute), and Lorraine Symington (Columbia University) for strains and plasmids. We thank Chris Gilpin and Laurie Muller of the Purdue Life Sciences Microscopy Facility for assistance preparing TEM samples and collecting TEM data. We also thank the Purdue University Animal Resource Facility and Histology Research laboratory for their contributions to this research. Payment for this article was generously provided, in part, by the Purdue University Libraries Open Access Publishing Fund.
The authors declare that the research was conducted in the absence of any commercial or financial relationships that could be construed as a potential conflict of interest.
All claims expressed in this article are solely those of the authors and do not necessarily represent those of their affiliated organizations, or those of the publisher, the editors and the reviewers. Any product that may be evaluated in this article, or claim that may be made by its manufacturer, is not guaranteed or endorsed by the publisher.
The Supplementary material for this article can be found online at: https://www.frontiersin.org/articles/10.3389/fmicb.2023.1129155/full#supplementary-material
Abruzzo, G. K., Gill, C. J., Flattery, A. M., Li, K., Claire, L., Smith, J. G., et al. (2000). Efficacy of the echinocandin caspofungin against disseminated aspergillosis and candidiasis in cyclophosphamide-induced immunosuppressed mice. Antimicrob. Agents Chemother. 44, 2310–2318. doi: 10.1128/AAC.44.9.2310-2318.2000
Ah-Fong, A. M., and Judelson, H. S. (2011). New role for Cdc14 phosphatase: localization to basal bodies in the oomycete phytophthora and its evolutionary coinheritance with eukaryotic flagella. PLoS One 6:e16725. doi: 10.1371/journal.pone.0016725
Ames, L., Duxbury, S., Pawlowska, B., Ho, H., Haynes, K., and Bates, S. (2017). Galleria mellonella as a host model to study Candida glabrata virulence and antifungal efficacy. Virulence 8, 1909–1917. doi: 10.1080/21505594.2017.1347744
Au Yong, J. Y., Wang, Y.-M., and Wang, Y. (2016). The Nim1 kinase Gin4 has distinct domains crucial for septin assembly, phospholipid binding and mitotic exit. J. Cell Sci. 129, 2744–2756. doi: 10.1242/jcs.183160
Baker, K. M., Hoda, S., Saha, D., Gregor, J. B., Georgescu, L., Serratore, N. D., et al. (2022). The Set1 histone H3K4 methyltransferase contributes to azole susceptibility in a species-specific manner by differentially altering the expression of drug efflux pumps and the Ergosterol gene pathway. Antimicrob. Agents Chemother. 66:20. doi: 10.1128/aac.02250-21
Bembenek, J., Kang, J., Kurischko, C., Li, B., Raab, J. R., Belanger, K. D., et al. (2005). Crm1-mediated nuclear export of Cdc14 is required for the completion of cytokinesis in budding yeast. Cell Cycle 4, 961–971. doi: 10.4161/cc.4.7.1798
Berdougo, E., Nachury, M. V., Jackson, P. K., and Jallepalli, P. V. (2008). The nucleolar phosphatase Cdc14B is dispensable for chromosome segregation and mitotic exit in human cells. Cell Cycle 7, 1184–1190. doi: 10.4161/cc.7.9.5792
Brace, J., Hsu, J., and Weiss, E. L. (2011). Mitotic exit control of the Saccharomyces cerevisiae Ndr/LATS kinase Cbk1 regulates daughter cell separation after cytokinesis. Mol. Cell. Biol. 31, 721–735. doi: 10.1128/MCB.00403-10
Brand, A. (2011). Hyphal growth in human fungal pathogens and its role in virulence. Int. J. Microbiol. 2012:517529. doi: 10.1155/2012/517529
Breitkreutz, A., Choi, H., Sharom, J. R., Boucher, L., Neduva, V., Larsen, B., et al. (2010). A global protein kinase and phosphatase interaction network in yeast. Science 328, 1043–1046. doi: 10.1126/science.1176495328/5981/1043
Bremmer, S. C., Hall, H., Martinez, J. S., Eissler, C. L., Hinrichsen, T. H., Rossie, S., et al. (2012). Cdc14 phosphatases preferentially dephosphorylate a subset of cyclin-dependent kinase (Cdk) sites containing phosphoserine. J. Biol. Chem. 287, 1662–1669. doi: 10.1074/jbc.M111.281105
Broadus, M. R., and Gould, K. L. (2012). Multiple protein kinases influence the redistribution of fission yeast Clp1/Cdc14 phosphatase upon genotoxic stress. Mol. Biol. Cell 23, 4118–4128. doi: 10.1091/mbc.E12-06-0475
Chasman, D., Ho, Y.-H., Berry, D. B., Nemec, C. M., MacGilvray, M. E., Hose, J., et al. (2014). Pathway connectivity and signaling coordination in the yeast stress-activated signaling network. Mol. Syst. Biol. 10:759. doi: 10.15252/msb.20145120
Chen, J. S., Broadus, M. R., McLean, J. R., Feoktistova, A., Ren, L., and Gould, K. L. (2013). Comprehensive proteomics analysis reveals new substrates and regulators of the fission yeast clp1/cdc14 phosphatase. Mol. Cell. Proteomics 12, 1074–1086. doi: 10.1074/mcp.M112.025924M112.025924
Cheng, S., Clancy, C. J., Xu, W., Schneider, F., Hao, B., Mitchell, A. P., et al. (2013). Profiling of Candida albicans gene expression during intra-abdominal candidiasis identifies biologic processes involved in pathogenesis. J. Infect. Dis. 208, 1529–1537. doi: 10.1093/infdis/jit335
Chin, C. F., Bennett, A. M., Ma, W. K., Hall, M. C., and Yeong, F. M. (2012). Dependence of Chs2 ER export on dephosphorylation by cytoplasmic Cdc14 ensures that septum formation follows mitosis. Mol. Biol. Cell 23, 45–58. doi: 10.1091/mbc.e11-05-0434
Clement, A., Solnica-Krezel, L., and Gould, K. L. (2011). The Cdc14B phosphatase contributes to ciliogenesis in zebrafish. Development 138, 291–302. doi: 10.1242/dev.055038138/2/291
Clemente-Blanco, A., Gonzalez-Novo, A., Machin, F., Caballero-Lima, D., Aragon, L., Sanchez, M., et al. (2006). The Cdc14p phosphatase affects late cell-cycle events and morphogenesis in Candida albicans. J. Cell Sci. 119, 1130–1143. doi: 10.1242/jcs.02820
Clifford, D. M., Wolfe, B. A., Roberts-Galbraith, R. H., McDonald, W. H., Yates, J. R., and Gould, K. L. (2008). The Clp1/Cdc14 phosphatase contributes to the robustness of cytokinesis by association with anillin-related Mid1. J. Cell Biol. 181, 79–88. doi: 10.1083/jcb.200709060
Cotter, G., Doyle, S., and Kavanagh, K. (2000). Development of an insect model for the in vivo pathogenicity testing of yeasts. Pathog. Dis. 27, 163–169. doi: 10.1111/j.1574-695X.2000.tb01427.x
Cueille, N., Salimova, E., Esteban, V., Blanco, M., Moreno, S., Bueno, A., et al. (2001). Flp1, a fission yeast orthologue of the s. cerevisiae CDC14 gene, is not required for cyclin degradation or rum1p stabilisation at the end of mitosis. J. Cell Sci. 114, 2649–2664. doi: 10.1242/jcs.114.14.2649
Culotti, J., and Hartwell, L. H. (1971). Genetic control of the cell division cycle in yeast. 3. Seven genes controlling nuclear division. Exp. Cell Res. 67, 389–401. doi: 10.1016/0014-4827(71)90424-1
DeMarco, A. G., Milholland, K. L., Pendleton, A. L., Whitney, J. J., Zhu, P., Wesenberg, D. T., et al. (2020). Conservation of Cdc14 phosphatase specificity in plant fungal pathogens: implications for antifungal development. Sci. Rep. 10:12073. doi: 10.1038/s41598-020-68921-3
Diaz-Cuervo, H., and Bueno, A. (2008). Cds1 controls the release of Cdc14-like phosphatase Flp1 from the nucleolus to drive full activation of the checkpoint response to replication stress in fission yeast. Mol. Biol. Cell 19, 2488–2499. doi: 10.1091/mbc.E07-08-0737E07-08-0737
Eissler, C. L., Mazon, G., Powers, B. L., Savinov, S. N., Symington, L. S., and Hall, M. C. (2014). The Cdk/Cdc14 module controls activation of the Yen1 Holliday junction resolvase to promote genome stability. Mol. Cell 54, 80–93. doi: 10.1016/j.molcel.2014.02.012
Foltman, M., Molist, I., Arcones, I., Sacristan, C., Filali-Mouncef, Y., Roncero, C., et al. (2016). Ingression progression complexes control extracellular matrix remodelling during cytokinesis in budding yeast. PLoS Genet. 12:e1005864. doi: 10.1371/journal.pgen.1005864
Fonzi, W. A., and Irwin, M. Y. (1993). Isogenic strain construction and gene mapping in Candida albicans. Genetics 134, 717–728. doi: 10.1093/genetics/134.3.717
Fuchs, B. B., and Eleftherios, M. (2009). Our paths might cross: the role of the fungal cell wall integrity pathway in stress response and cross talk with other stress response pathways. Eukaryot. Cell 8, 1616–1625. doi: 10.1128/EC.00193-09
Fuchs, B. B., O’Brien, E., El Khoury, J. B., and Mylonakis, E. (2010). Methods for using Galleria mellonella as a model host to study fungal pathogenesis. Virulence 1, 475–482. doi: 10.4161/viru.1.6.12985
Gola, S., Martin, R., Walther, A., Dünkler, A., and Wendland, J. (2003). New modules for PCR-based gene targeting in Candida albicans: rapid and efficient gene targeting using 100 bp of flanking homology region. Yeast 20, 1339–1347. doi: 10.1002/yea.1044
Gray, C. H., Good, V. M., Tonks, N. K., and Barford, D. (2003). The structure of the cell cycle protein Cdc14 reveals a proline-directed protein phosphatase. EMBO J. 22, 3524–3535. doi: 10.1093/emboj/cdg348
Ho, C. K., Mazon, G., Lam, A. F., and Symington, L. S. (2010). Mus81 and Yen1 promote reciprocal exchange during mitotic recombination to maintain genome integrity in budding yeast. Mol. Cell 40, 988–1000. doi: 10.1016/j.molcel.2010.11.016
Hohl, T. M. (2014). Overview of vertebrate animal models of fungal infection. J. Immunol. Methods 410, 100–112. doi: 10.1016/j.jim.2014.03.022
Ibe, C., and Munro, C. A. (2021). Fungal cell wall proteins and signaling pathways form a cytoprotective network to combat stresses. J. Fungi 7:739. doi: 10.3390/jof7090739
Imtiaz, A., Belyantseva, I. A., Beirl, A. J., Fenollar-Ferrer, C., Bashir, R., Bukhari, I., et al. (2018). CDC14A phosphatase is essential for hearing and male fertility in mouse and human. Hum. Mol. Genet. 27, 780–798. doi: 10.1093/hmg/ddx440
Jung, U. S., and Levin, D. E. (1999). Genome-wide analysis of gene expression regulated by the yeast cell wall integrity signalling pathway. Mol. Microbiol. 34, 1049–1057. doi: 10.1046/j.1365-2958.1999.01667.x
Jungbluth, M., Renicke, C., and Taxis, C. (2010). Targeted protein depletion in Saccharomyces cerevisiae by activation of a bidirectional degron. BMC Syst. Biol. 4:176. doi: 10.1186/1752-0509-4-176
Kadosh, D. (2019). Regulatory mechanisms controlling morphology and pathogenesis in Candida albicans. Host microbe interact. Fungi • host-microbe interact. Parasitology 52, 27–34. doi: 10.1016/j.mib.2019.04.005
Kaneva, I. N., Sudbery, I. M., Dickman, M. J., and Sudbery, P. E. (2019). Proteins that physically interact with the phosphatase Cdc14 in Candida albicans have diverse roles in the cell cycle. Sci. Rep. 9:6258. doi: 10.1038/s41598-019-42530-1
Kataria, M., Mouilleron, S., Seo, M. H., Corbi-Verge, C., Kim, P. M., and Uhlmann, F. (2018). A PxL motif promotes timely cell cycle substrate dephosphorylation by the Cdc14 phosphatase. Nat. Struct. Mol. Biol. 25, 1093–1102. doi: 10.1038/s41594-018-0152-3
Kerk, D., Templeton, G., and Moorhead, G. B. (2008). Evolutionary radiation pattern of novel protein phosphatases revealed by analysis of protein data from the completely sequenced genomes of humans, green algae, and higher plants. Plant Physiol. 146, 351–367. doi: 10.1104/pp.107.111393
Kobayashi, J., and Matsuura, Y. (2017). Structure and dimerization of the catalytic domain of the protein phosphatase Cdc14p, a key regulator of mitotic exit in Saccharomyces cerevisiae. Protein Sci. 26, 2105–2112. doi: 10.1002/pro.3244
Kuilman, T., Maiolica, A., Godfrey, M., Scheidel, N., Aebersold, R., and Uhlmann, F. (2015). Identification of Cdk targets that control cytokinesis. EMBO J. 34, 81–96. doi: 10.15252/embj.201488958
Kushnirov, V. V. (2000). Rapid and reliable protein extraction from yeast. Yeast 16, 857–860. doi: 10.1002/1097-0061(20000630)16:9<857::AID-YEA561>3.0.CO;2-B
Lay, J., Henry, K. L., Clifford, J., Bulawa, C. E., Becker, J. M., and Yigal, K. (1998). Altered expression of selectable marker URA3 in gene-disrupted Candida albicans strains complicates interpretation of virulence studies. Infect. Immun. 66, 5301–5306. doi: 10.1128/IAI.66.11.5301-5306.1998
Levin, D. E. (2011). Regulation of cell wall biogenesis in Saccharomyces cerevisiae: the cell wall integrity signaling pathway. Genetics 189, 1145–1175. doi: 10.1534/genetics.111.128264
Li, C., Cao, S., Zhang, C., Zhang, Y., Zhang, Q., Xu, J.-R., et al. (2018). MoCDC14 is important for septation during conidiation and appressorium formation in Magnaporthe oryzae. Mol. Plant Pathol. 19, 328–340. doi: 10.1111/mpp.12523
Li, Y., Cross, F. R., and Chait, B. T. (2014). Method for identifying phosphorylated substrates of specific cyclin/cyclin-dependent kinase complexes. Proc. Natl. Acad. Sci. U. S. A. 111, 11323–11328. doi: 10.1073/pnas.1409666111
Li, L., Ernsting, B. R., Wishart, M. J., Lohse, D. L., and Dixon, J. E. (1997). A family of putative tumor suppressors is structurally and functionally conserved in humans and yeast. J. Biol. Chem. 272, 29403–29406. doi: 10.1074/jbc.272.47.29403
Li, C., Melesse, M., Zhang, S., Hao, C., Wang, C., Zhang, H., et al. (2015). FgCDC14 regulates cytokinesis, morphogenesis, and pathogenesis in Fusarium graminearum. Mol. Microbiol. 98, 770–786. doi: 10.1111/mmi.13157
Lo, H.-J., Köhler, J. R., DiDomenico, B., Loebenberg, D., Cacciapuoti, A., and Fink, G. R. (1997). Nonfilamentous C. albicans mutants are avirulent. Cells 90, 939–949. doi: 10.1016/S0092-8674(00)80358-X
López-Avilés, S., Kapuy, O., Novák, B., and Uhlmann, F. (2009). Irreversibility of mitotic exit is the consequence of systems-level feedback. Nature 459, 592–595. doi: 10.1038/nature07984
MacCallum, D. M. (2011). Hosting infection: experimental models to assay Candida virulence. Int. J. Microbiol. 2012:363764. doi: 10.1155/2012/363764
Manzano-López, J., and Monje-Casas, F. (2020). The multiple roles of the Cdc14 phosphatase in cell cycle control. Int. J. Mol. Sci. 21:709. doi: 10.3390/ijms21030709
Mazur, P., Morin, N., Baginsky, W., El-Sherbeini, M., Clemas, J. A., Nielsen, J. B., et al. (1995). Differential expression and function of two homologous subunits of yeast 1,3-beta-D-glucan synthase. Mol. Cell. Biol. 15, 5671–5681. doi: 10.1128/MCB.15.10.5671
Meitinger, F., Palani, S., and Pereira, G. (2012). The power of MEN in cytokinesis. Cell Cycle 11, 219–228. doi: 10.4161/cc.11.2.18857
Meitinger, F., and Pereira, G. (2016). “Visualization of cytokinesis events in budding yeast by transmission electron microscopy” in Yeast Cytokinesis: Methods and Protocols. eds. A. Sanchez-Diaz and P. Perez (New York, NY: Springer New York), 87–95. doi: 10.1007/978-1-4939-3145-3_7
Meitinger, F., Petrova, B., Lombardi, I. M., Bertazzi, D. T., Hub, B., Zentgraf, H., et al. (2010). Targeted localization of Inn1, Cyk3 and Chs2 by the mitotic-exit network regulates cytokinesis in budding yeast. J. Cell Sci. 123, 1851–1861. doi: 10.1242/jcs.063891
Miller, D. P., Hall, H., Chaparian, R., Mara, M., Mueller, A., Hall, M. C., et al. (2015). Dephosphorylation of Iqg1 by Cdc14 regulates cytokinesis in budding yeast. Mol. Biol. Cell 26, 2913–2926. doi: 10.1091/mbc.E14-12-1637
Mocciaro, A., Berdougo, E., Zeng, K., Black, E., Vagnarelli, P., Earnshaw, W., et al. (2010). Vertebrate cells genetically deficient for Cdc14A or Cdc14B retain DNA damage checkpoint proficiency but are impaired in DNA repair. J. Cell Biol. 189, 631–639. doi: 10.1083/jcb.200910057
Mocciaro, A., and Schiebel, E. (2010). Cdc14: a highly conserved family of phosphatases with non-conserved functions? J. Cell Sci. 123, 2867–2876. doi: 10.1242/jcs.074815
Palani, S., Meitinger, F., Boehm, M. E., Lehmann, W. D., and Pereira, G. (2012). Cdc14-dependent dephosphorylation of Inn1 contributes to Inn1-Cyk3 complex formation. J. Cell Sci. 125, 3091–3096. doi: 10.1242/jcs.106021
Pérez, P., Cortés, J. C. G., Cansado, J., and Ribas, J. C. (2018). Fission yeast cell wall biosynthesis and cell integrity signalling. Cell Surf. 4, 1–9. doi: 10.1016/j.tcsw.2018.10.001
Powers, B. L., and Hall, M. C. (2017). Re-examining the role of Cdc14 phosphatase in reversal of Cdk phosphorylation during mitotic exit. J. Cell Sci., jcs.201012 130, 2673–2681. doi: 10.1242/jcs.201012
Powers, B. L., Hall, H., Charbonneau, H., and Hall, M. C. (2017). A substrate trapping method for identification of direct Cdc14 phosphatase targets. Methods Mol. Biol. 1505, 119–132. doi: 10.1007/978-1-4939-6502-1_10
Saito, R. M., Perreault, A., Peach, B., Satterlee, J. S., and van den Heuvel, S. (2004). The CDC-14 phosphatase controls developmental cell-cycle arrest in C. elegans. Nat. Cell Biol. 6, 777–783. doi: 10.1038/ncb1154
Sanchez-Diaz, A., Nkosi, P. J., Murray, S., and Labib, K. (2012). The mitotic exit network and Cdc14 phosphatase initiate cytokinesis by counteracting CDK phosphorylations and blocking polarised growth. EMBO J. 31, 3620–3634. doi: 10.1038/emboj.2012.224
Segal, E., and Frenkel, M. (2018). Experimental in vivo models of candidiasis. J. Fungi 4:21. doi: 10.3390/jof4010021
Serratore, N. D., Baker, K. M., Macadlo, L. A., Gress, A. R., Powers, B. L., Atallah, N., et al. (2018). A novel sterol-signaling pathway governs azole antifungal drug resistance and hypoxic gene repression in Saccharomyces cerevisiae. Genetics 208, 1037–1055. doi: 10.1534/genetics.117.300554
Sharkey, L. L., Liao, W., Ghosh, A. K., and Fonzi, W. A. (2005). Flanking direct repeats of hisG alter URA3 marker expression at the HWP1 locus of Candida albicans. Microbiology 151, 1061–1071. doi: 10.1099/mic.0.27487-0
Storici, F., and Resnick, M. A. (2006). The delitto perfetto approach to in vivo site-directed mutagenesis and chromosome rearrangements with synthetic oligonucleotides in yeast. Methods Enzymol. 409, 329–345. doi: 10.1016/S0076-6879(05)09019-1
Sucher, A. J., Chahine, E. B., and Balcer, H. E. (2009). Echinocandins: the newest class of antifungals. Ann. Pharmacother. 43, 1647–1657. doi: 10.1345/aph.1M237
Sundstrom, P., Cutler, J. E., and Staab, J. F. (2002). Reevaluation of the role of HWP1 in systemic candidiasis by use of Candida albicans strains with selectable marker URA3 targeted to the ENO1 locus. Infect. Immun. 70, 3281–3283. doi: 10.1128/IAI.70.6.3281-3283.2002
Taxis, C., Stier, G., Spadaccini, R., and Knop, M. (2009). Efficient protein depletion by genetically controlled deprotection of a dormant N-degron. Mol. Syst. Biol. 5:267. doi: 10.1038/msb.2009.25
Taylor, G. S., Liu, Y., Baskerville, C., and Charbonneau, H. (1997). The activity of Cdc14p, an oligomeric dual specificity protein phosphatase from Saccharomyces cerevisiae, is required for cell cycle progression. J. Biol. Chem. 272, 24054–24063. doi: 10.1074/jbc.272.38.24054
Tognetti, S., Jimenez, J., Vigano, M., Duch, A., Queralt, E., de Nadal, E., et al. (2020). Hog1 activation delays mitotic exit via phosphorylation of Net1. Proc. Natl. Acad. Sci. U. S. A. 117, 8924–8933. doi: 10.1073/pnas.1918308117
Torres, L., Martín, H., García-Saez, M. I., Arroyo, J., Molina, M., Sánchez, M., et al. (1991). A protein kinase gene complements the lytic phenotype of Saccharomyces cerevisiae lyt2 mutants. Mol. Microbiol. 5, 2845–2854. doi: 10.1111/j.1365-2958.1991.tb01993.x
Trautmann, S., Wolfe, B. A., Jorgensen, P., Tyers, M., Gould, K. L., and McCollum, D. (2001). Fission yeast Clp1p phosphatase regulates G2/M transition and coordination of cytokinesis with cell cycle progression. Curr. Biol. 11, 931–940. doi: 10.1016/s0960-9822(01)00268-8
Uddin, B., Partscht, P., Chen, N.-P., Neuner, A., Weiß, M., Hardt, R., et al. (2019). The human phosphatase CDC14A modulates primary cilium length by regulating centrosomal actin nucleation. EMBO Rep. 20:e46544. doi: 10.15252/embr.201846544
Vázquez-Novelle, M. D., Esteban, V., Bueno, A., and Sacristán, M. P. (2005). Functional homology among human and fission yeast Cdc14 phosphatases. J. Biol. Chem. 280, 29144–29150. doi: 10.1074/jbc.M413328200
Villoria, M. T., Ramos, F., Duenas, E., Faull, P., Cutillas, P. R., and Clemente-Blanco, A. (2017). Stabilization of the metaphase spindle by Cdc14 is required for recombinational DNA repair. EMBO J. 36, 79–101. doi: 10.15252/embj.201593540
Visintin, R., Craig, K., Hwang, E. S., Prinz, S., Tyers, M., and Amon, A. (1998). The phosphatase Cdc14 triggers mitotic exit by reversal of Cdk-dependent phosphorylation. Mol. Cell 2, 709–718. doi: 10.1016/S1097-2765(00)80286-5
von der Haar, T. (2007). Optimized protein extraction for quantitative proteomics of yeasts. PLoS One 2:e1078. doi: 10.1371/journal.pone.0001078
Walker, L. A., Lenardon, M. D., Preechasuth, K., Munro, C. A., and Gow, N. A. R. (2013). Cell wall stress induces alternative fungal cytokinesis and septation strategies. J. Cell Sci. 126, 2668–2677. doi: 10.1242/jcs.118885
Wang, W.-Q., Bembenek, J., Gee, K. R., Yu, H., Charbonneau, H., and Zhang, Z.-Y. (2004). Kinetic and mechanistic studies of a cell cycle protein phosphatase Cdc14. J. Biol. Chem. 279, 30459–30468. doi: 10.1074/jbc.M402217200
Wang, M., Herrmann, C. J., Simonovic, M., Szklarczyk, D., and Mering, C. (2015). Version 4.0 of PaxDb: protein abundance data, integrated across model organisms, tissues, and cell-lines. Proteomics 15, 3163–3168. doi: 10.1002/pmic.201400441
Wang, J., Liu, J., Hu, Y., Ying, S.-H., and Feng, M.-G. (2013). Cytokinesis-required Cdc14 is a signaling hub of asexual development and multi-stress tolerance in Beauveria bassiana. Sci. Rep. 3:3086. doi: 10.1038/srep03086
Wolfe, B. A., McDonald, W. H., Yates, J. R., and Gould, K. L. (2006). Phospho-regulation of the Cdc14/Clp1 phosphatase delays late mitotic events in S. pombe. Dev. Cell 11, 423–430. doi: 10.1016/j.devcel.2006.07.016
Wright, R. (2000). Transmission electron microscopy of yeast. Microsc. Res. Tech. 51, 496–510. doi: 10.1002/1097-0029(20001215)51:6<496::AID-JEMT2>3.0.CO;2-9
Yang, G., Hu, Y., Fasoyin, O. E., Yue, Y., Chen, L., Qiu, Y., et al. (2018). The Aspergillus flavus phosphatase CDC14 regulates development, aflatoxin biosynthesis and pathogenicity. Front. Cell. Infect. Microbiol. 8:141. doi: 10.3389/fcimb.2018.00141
Keywords: Cdc14, phosphatase, cell wall integrity, Candida albicans, echinocandins, septation, pathogenesis, hyphal differentiation
Citation: Milholland KL, AbdelKhalek A, Baker KM, Hoda S, DeMarco AG, Naughton NH, Koeberlein AN, Lorenz GR, Anandasothy K, Esperilla-Muñoz A, Narayanan SK, Correa-Bordes J, Briggs SD and Hall MC (2023) Cdc14 phosphatase contributes to cell wall integrity and pathogenesis in Candida albicans. Front. Microbiol. 14:1129155. doi: 10.3389/fmicb.2023.1129155
Received: 21 December 2022; Accepted: 26 January 2023;
Published: 16 February 2023.
Edited by:
Miguel Cacho Teixeira, University of Lisbon, PortugalReviewed by:
Slawomir Milewski, Gdansk University of Technology, PolandCopyright © 2023 Milholland, AbdelKhalek, Baker, Hoda, DeMarco, Naughton, Koeberlein, Lorenz, Anandasothy, Esperilla-Muñoz, Narayanan, Correa-Bordes, Briggs and Hall. This is an open-access article distributed under the terms of the Creative Commons Attribution License (CC BY). The use, distribution or reproduction in other forums is permitted, provided the original author(s) and the copyright owner(s) are credited and that the original publication in this journal is cited, in accordance with accepted academic practice. No use, distribution or reproduction is permitted which does not comply with these terms.
*Correspondence: Mark C. Hall, ✉ bWNoYWxsQHB1cmR1ZS5lZHU=
Disclaimer: All claims expressed in this article are solely those of the authors and do not necessarily represent those of their affiliated organizations, or those of the publisher, the editors and the reviewers. Any product that may be evaluated in this article or claim that may be made by its manufacturer is not guaranteed or endorsed by the publisher.
Research integrity at Frontiers
Learn more about the work of our research integrity team to safeguard the quality of each article we publish.