- 1Department of Laboratory Medicine, Nanjing Drum Tower Hospital, The Affiliated Hospital of Nanjing University Medical School, Nanjing, Jiangsu, China
- 2Department of Acute Infectious Disease Control and Prevention, Jiangsu Provincial Center for Disease Control and Prevention, Nanjing, Jiangsu, China
Objective: To analyze the molecular epidemiology of carbapenem-resistant Enterobacter cloacae complex (CREC) by whole-genome sequencing and to explore its clinical characteristics.
Methods: Enterobacter cloacae complex isolates collected in a tertiary hospital during 2013–2021 were subjected to whole-genome sequencing to determine the distribution of antimicrobial resistance genes (ARGs), sequence types (STs), and plasmid replicons. A phylogenetic tree of the CREC strains was constructed based on the whole-genome sequences to analyze their relationships. Clinical patient information was collected for risk factor analysis.
Results: Among the 51 CREC strains collected, blaNDM-1 (n = 42, 82.4%) was the main carbapenem-hydrolyzing β-lactamase (CHβL), followed by blaIMP-4 (n = 11, 21.6%). Several other extended-spectrum β-lactamase-encoding genes were also identified, with blaSHV-12 (n = 30, 58.8%) and blaTEM-1B (n = 24, 47.1%) being the predominant ones. Multi-locus sequence typing revealed 25 distinct STs, and ST418 (n = 12, 23.5%) was the predominant clone. Plasmid analysis identified 15 types of plasmid replicons, among which IncHI2 (n = 33, 64.7%) and IncHI2A (n = 33, 64.7%) were the main ones. Risk factor analysis showed that intensive care unit (ICU) admission, autoimmune disease, pulmonary infection, and previous corticosteroid use within 1 month were major risk factors for acquiring CREC. Logistic regression analysis showed that ICU admission was an independent risk factor for CREC acquisition and was closely related with acquiring infection by CREC with ST418.
Conclusion: BlaNDM-1 and blaIMP-4 were the predominant carbapenem resistance genes. ST418 carrying BlaNDM-1 not only was the main clone, but also circulated in the ICU of our hospital during 2019–2021, which highlights the necessity for surveillance of this strain in the ICU. Furthermore, patients with risk factors for CREC acquisition, including ICU admission, autoimmune disease, pulmonary infection, and previous corticosteroid use within 1 month, need to be closely monitored for CREC infection.
1. Introduction
Enterobacter cloacae complex (ECC), which belongs to the family Enterobacteriaceae, is a common nosocomial pathogen responsible for several human infections, including bacteremia and respiratory tract, wound, and urinary tract infections (Maria et al., 2012; Jin et al., 2020). Currently, ECC includes Enterobacter cloacae, Enterobacter hormaechei, Enterobacter kobei, Enterobacter asburiae, Enterobacter cancerogenus, Enterobacter nimipressuralis, and Enterobacter mori. E. cloacae and E. hormaechei are the most frequently isolated from human clinical specimens (Sutton et al., 2018; Davin-Regli et al., 2019).
Carbapenems have been extensively used in clinic as a last choice for treating severe gram-negative bacterial infection owing to their broad-spectrum antibacterial activity and non-toxicity (Jin et al., 2018). In recent years, with the widespread use of carbapenem antibiotics, the emergence and spread of carbapenem-resistant Enterobacteriaceae (CRE) has posed a serious public health threat (Davin-Regli and Pages, 2015; Jiang et al., 2022). At present, carbapenem-resistant Enterobacter cloacae complex (CREC) is the third most common CRE following Escherichia coli and Klebsiella pneumoniae in China (Zhang et al., 2018; Hu et al., 2022). An increased incidence of CREC with clonal transmission and endemicity has been constantly reported in various regions (Jiang et al., 2022).
The main mechanism of carbapenem resistance in CREC is the production of carbapenem-hydrolyzing β-lactamases (CHβLs), among which KPC, NDM, VIM, IMP, and OXA-48 are the most prevalent ones. In addition, overexpression of the β-lactamase AmpC, the occurrence of extended-spectrum β-lactamases (ESBLs), such as TEM, CTX-M, and SHV, membrane-associated mechanisms, such as outer membrane permeability, and the overexpression of efflux pumps have been demonstrated to contribute to carbapenem resistance in ECC (Davin-Regli et al., 2019). The acquisition of the carbapenem resistance genes are most often transferred by mobile genetic elements (MGEs), such as plasmids and transposons, which can be easily transferred between different species (Dong et al., 2022). Globally, NDM is the main CHβL conferring carbapenem resistance in CREC. However, regional differences in the distribution of CREC CHβLs have been observed, with KPC being predominant in North America, OXA-48 and VIM being predominant in Europe, and NDM being the most prevalent in China (Annavajhala et al., 2019).
A multinational surveillance study based on multi-locus sequence typing (MLST) revealed substantial clonal diversity of CREC, and ST114, ST93, ST90, and ST78 were potential high-risk clones globally widespread in 37 countries (Peirano et al., 2018). Risk factor analysis for CREC acquisition has shown that ICU admission, prior antimicrobial exposure, invasive treatments, surgical procedures, and prolonged hospitalization are the main risk factors for CREC acquisition (Jiang et al., 2019; Li et al., 2019; Tetsuka et al., 2019; Tian et al., 2020). However, studies on a long-term continuous study of CREC has been poorly reported in China.
The present study was performed in Nanjing Drum Tower Hospital, a 3800-bed tertiary hospital. A total of 51 CREC strains collected during 2013–2021 were subjected to whole-genome sequencing (WGS) to analyze the distribution of antimicrobial resistance genes (ARGs), sequence types (STs), and plasmid replicons. Clinical patient information was collected to analyze risk factors for CREC acquisition. We attempted to systematically analyze the molecular epidemiology and clinical characteristics of CREC infection to provide evidence for effective control of nosocomial infection with CREC.
2. Materials and methods
2.1. Bacterial isolates
In total, 51 consecutive, non-duplicate CREC isolates were collected in Nanjing Drum Tower Hospital (the affiliated hospital of Nanjing University Medical School) during 2013–2021. All the strains were verified by Vitek 2.0 (BioMérieux, Marcy- l’Étoile, France) and matrix-associated laser desorption ionization–time of flight mass spectrometry (MALDI-TOF MS) (BioMérieux, Craponne, France). And these strains were confirmed as CREC by testing the susceptibilities to imipenem by Bauer et al. (1966) method according to the Clinical and Laboratory Standards Institute (CLSI) criteria (Humphries et al., 2021).
The strains were isolated from the following samples: sputum (n = 16, 31.4%), abdominal dropsy (n = 8, 15.7%), blood (n = 8, 15.7%) secretion (n = 7, 13.7%), urine (n = 6, 11.8%), catheter (n = 3, 5.9%), pleural effusion (n = 2, 3.9%), and bronchial alveolar lavage fluid (n = 1, 2.0%). The numbers of strains isolated in each year were as follows: 2013 (n = 1, 2.0%), 2014 (n = 2, 3.9%), 2015 (n = 4, 7.8%), 2016 (n = 2, 3.9%), 2017 (n = 5, 9.8%), 2018 (n = 7, 13.7%), 2019 (n = 8, 15.7%), 2020 (n = 9, 17.6%), and 2021 (n = 13, 25.5%). The CREC isolates were mostly collected from the ICU (n = 16, 30.8%), surgical ward (n = 7, 13.5%), cardiac surgery ward (n = 3, 5.8%), hematology ward (n = 3, 5.8%), and respiratory ward (n = 3, 5.8%).
2.2. Genomic DNA extraction, whole-genome sequencing, de novo assembly, scaffolding, and annotation
Fresh colonies of 51 CREC strains were picked from Luria–Bertani plates (Oxoid, UK) for genomic DNA extraction using the Ultraclean Microbial DNA Isolation Kit (MO BIO Laboratories, Carlsbad, CA, USA) according to the manufacturer’s recommendations. After a quality check, the genomic DNA samples were sent to Beijing Tiangen Biochemical Technology (Beijing, China) for sequencing on the MiSeq platform (Illumina, San Diego, CA, USA). After quality trimming (Qs ≥ 20), de novo assembly of the paired-end reads was performed using CLC Genomics Workbench v21.0.4 (Qiagen, Hilden, Germany). Scaffolding was performed using SSPACE standard version 3.0 and gaps within scaffolds were closed using GapFiller (Boetzer et al., 2011). The assembled genomes were submitted to NCBI for annotation.
2.3. Analysis of genomic epidemiology
Genomes of 51 CREC isolates have been uploaded to the NCBI BioProject repository1 with the accession number PRJNA868583 and are publicly available. MLST of strains retrieved from GenBank was performed using MLST v2.0.2 ARGs were identified using Resfinder v2.1.3 Antibiotic resistance plasmid genes were analyzed by uploading the genomes to PlasmidFinder v2.1.4
2.4. Phylogenetic analysis
The genome sequences of E. hormaechei strains were annotated using Prokka v1.14.6 (Seemann, 2014). The gff files produced by Prokka were used as input for genomic analysis using Roary v3.13.0, and SNP alignment sequences of 3,135 core genes were obtained (Page et al., 2015). The nucleotide substitution model was determined to be general time reversal model using jModelTest v2.0 (Darriba et al., 2012). A maximum-likelihood evolutionary tree was constructed using the RAxML-NG software v1.1.0 with bootstrapping set to 1000 (Kozlov et al., 2019) and was visualized using iTOL v6.5.8 Branches with bootstrap values of less than 50 were deleted (Letunic and Bork, 2021).
2.5. Clinical information collection
Among the 51 patients with CREC isolates, the clinical information of 50 ones were available to identify possible risk factors for CREC acquisition, whereas, clinical information of one patient with CREC was missing. Meanwhile, fifty isolates from patients with carbapenem-susceptible Enterobacter cloacae (CSEC) collected during the same time period and in the same wards and anatomical sites were included as a control group. The following factors were analyzed: patient demographics, underlying diseases and acquired infections, invasive procedures during hospital stay, and treatments within 1 month (Tetsuka et al., 2019; Tian et al., 2020; Oka et al., 2022). The study was approved by the Ethics Committee of Nanjing Drum Tower Hospital (approval No. 2022-049-2) and dispensed the need to obtain signed informed consents on account of the absence of any intervention.
2.6. Statistical analysis
The SPSS software (v25.0) was conducted for statistical analysis. The results of univariate analysis were reported as P-values by using the Chi-square test. To identify independent risk factors for CREC acquisition, significant variables with P < 0.10 in univariate analysis were enrolled in the logistic regression model for multivariate analysis (Tian et al., 2020). P < 0.05 was considered statistically significant. McNemar-Bowker test was used to analyze the distribution consistency between ARGs and plasmid replicons. P-value > 0.05 was taken as the consistency between ARGs and plasmid replicons.
3. Results
3.1. ECC species identified
Among the 51 ECC isolates, 44 (86.3%) isolates were identified as Enterobacter hormaechei, three (5.9%) as Enterobacter kobei, two (3.9%) as Enterobacter cloacae, and the two (3.9%) as Enterobacter asburiae (Figure 1A).
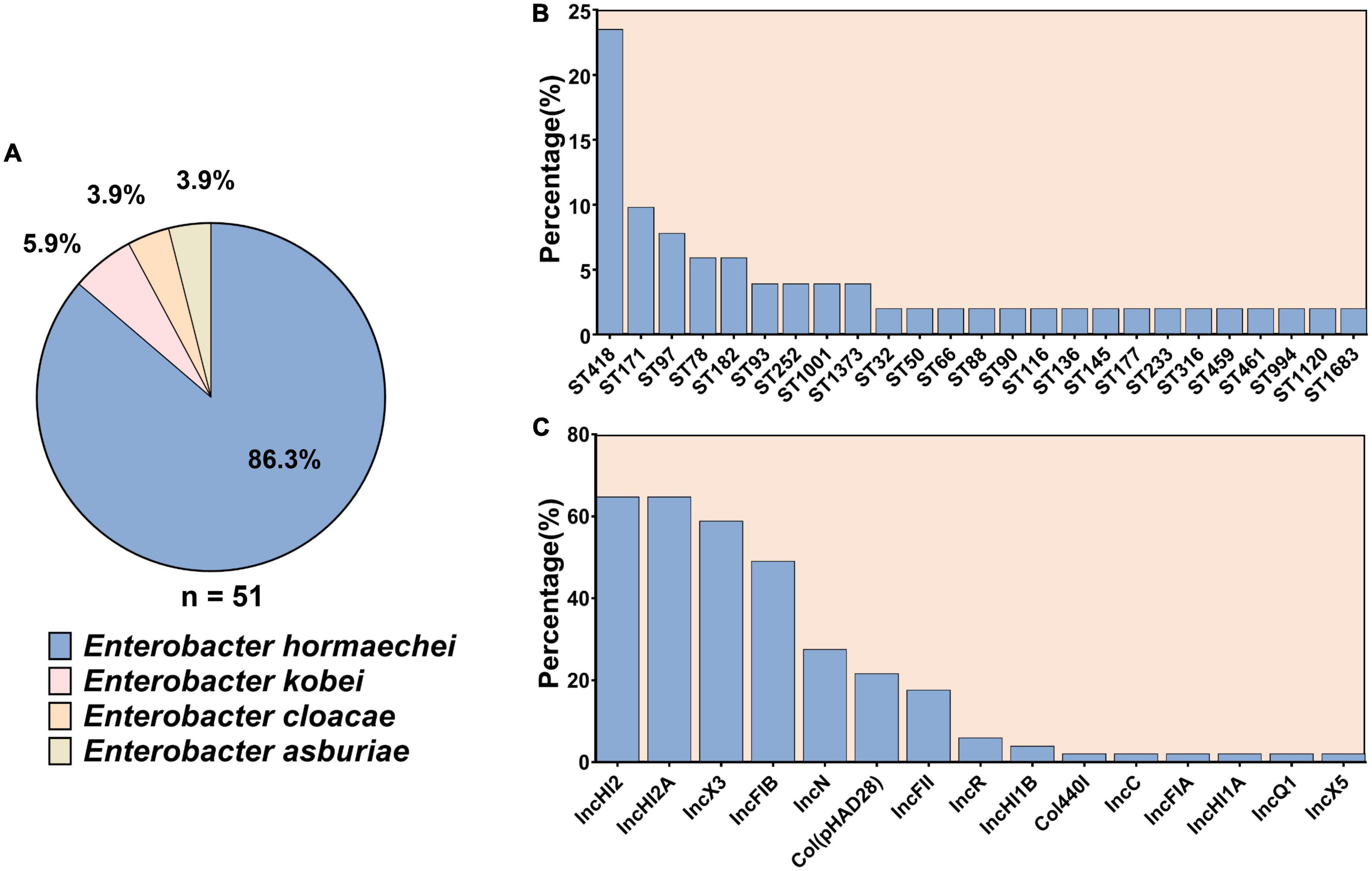
Figure 1. The subspecies and sequence types of 51 carbapenem-resistant Enterobacter cloacae complex as well as the distribution of plasmid replicons among them. (A) The subspecies of 51 carbapenem-resistant Enterobacter cloacae complex. (B) Sequence types of the 51 carbapenem-resistant Enterobacter cloacae complex strains. (C) Plasmid replicons among the 51 carbapenem-resistant Enterobacter cloacae complex.
3.2. Distribution of antimicrobial resistance genes
Various genes conferring resistance to 12 antimicrobial agents were identified in the 51 CREC isolates (Figure 2).
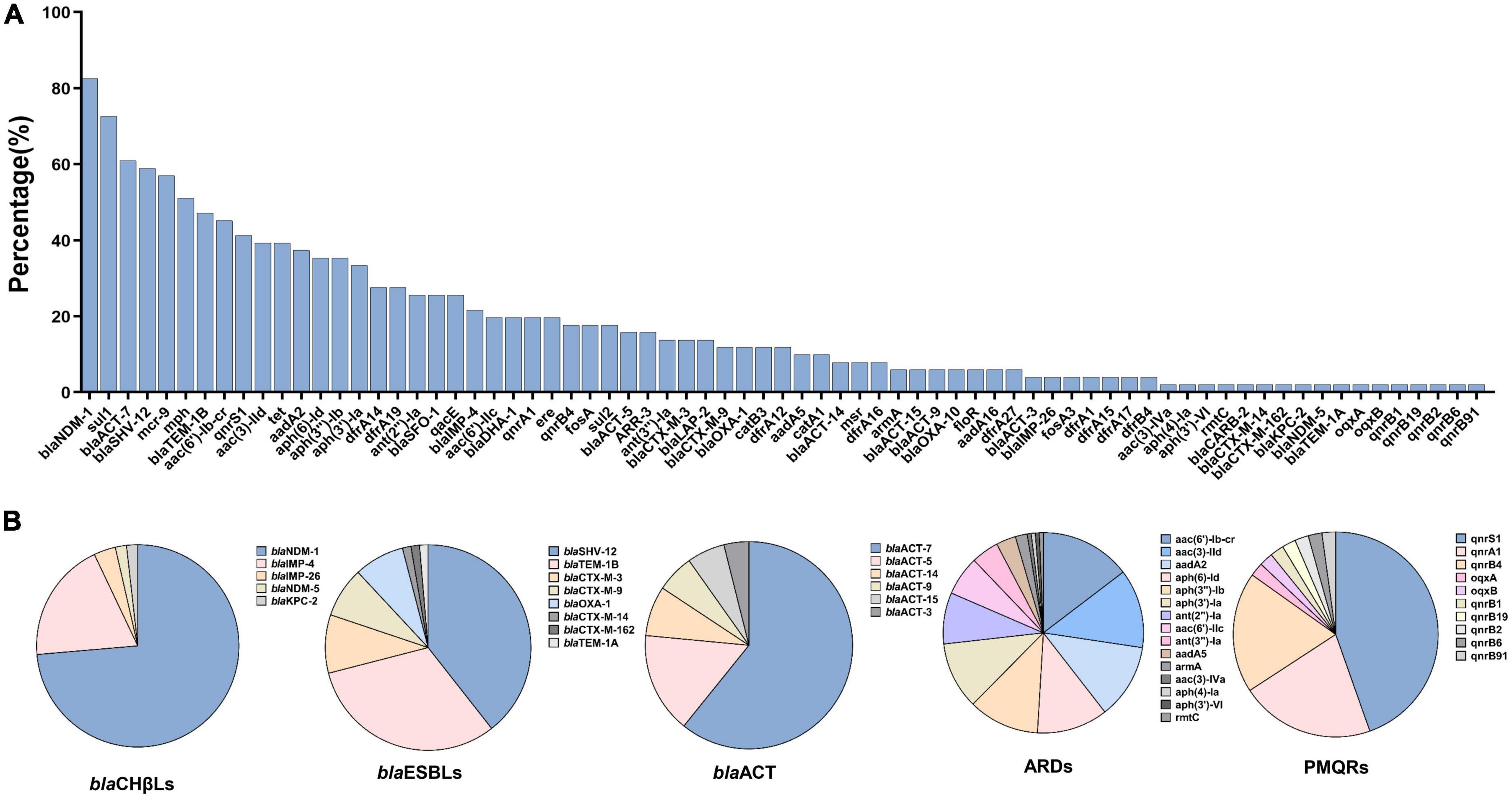
Figure 2. Antimicrobial resistance genes and the detailed subtypes detected among 51 carbapenem-resistant Enterobacter cloacae complex isolates. (A) Distribution of antimicrobial resistance genes ranked by the percentages. (B) The detailed subtypes of some predominant resistance genes. blaCHβLs, carbapenem-hydrolyzing β-lactamases; blaESBLs, extended-spectrum β-lactamases; ARDs, aminoglycoside resistant determinants; PMQRs, plasmid mediated quinolone resistance genes.
Five blaCHβls were identified; blaNDM-1 (n = 42, 82.4%) and blaIMP-4 (n = 11, 21.6%) were the predominant ones, followed by blaIMP-26 (n = 2, 3.9%), blaNDM-5 (n = 1, 2.0%), and blaKPC-2 (n = 1, 2.0%). Of note, co-existence of blaNDM-1 and blaIMP-4 was detected in six isolates (EC6949, EC13079, EC13224, EC13343, EC15480, and EC16542). Co-existence of blaNDM-1 and blaKPC-2 was detected in one isolate, EC13565. As for blaESBLs, blaSHV-12 (n = 30, 58.8%), blaTEM-1B (n = 24, 47.1%), blaCTX-M-3 (7, 13.7%), blaCTX-M-9 (n = 6, 11.8%), blaOXA-1 (n = 6, 11.8%) and blaCTX-M-14, blaCTX-M-162, and blaTEM-1A (n = 1, 2.0% each) were identified (Figure 2B).
All 51 ECC isolates carried blaACT with blaACT-7 being the predominant one (Figure 2). Of note, mobile colistin resistance (mcr)-9 (29/51, 56.9%) was highly prevalent among the CREC isolates in this study (Figure 2A). Co-existence of mcr-9 and blaNDM-1, blaIMP-4, blaIMP-26, or KPC-2 was identified in more than 50% of the CREC isolates (Supplementary Table 1).
3.3. STs of the 51 CREC strains
The 51 CREC isolates were assigned to 25 STs. ST418 (12/51, 23.5%) was the most prevalent, followed by ST171 (5/51, 9.8%), ST97 (4/51, 7.8%), ST78 (3/51, 5.9%), ST182 (3/51, 5.9%), ST93 (2/51, 3.9%), ST252 (2/51, 3.9%), ST1001 (2/51, 3.9%), and ST1373 (2/51, 3.9%). The remaining STs were only detected in one isolate (Figure 1B).
3.4. Plasmid replicons in the 51 CREC strains
Fifteen types of plasmid replicons were identified in 98% (50/51) of the isolates: IncHI2 (n = 33, 64.7%) and IncHI2A (n = 33, 64.7%) were the most prevalent ones, followed by IncX3 (n = 30, 58.8%), IncFIB (n = 25, 49.0%), IncN (n = 14, 27.5%), and Col (pHAD28) (n = 11, 21.6%). The remaining plasmid replicons were relatively less common (Figure 1C). No plasmid replicons were found in isolate EC15999.
Statistical analysis on the distribution consistency between ARGs and plasmid replicons in the CREC isolates are shown in Table 1. There was a consistency in the distribution of blaIMP and IncN, and the consistent distribution was also observed between blaSHV-12 and IncHI2, IncHI2A, IncX3, or IncFIB. Moreover, consistency distribution between mcr-9 and IncHI2, IncHI2A, IncX3, or IncFIB was also found.

Table 1. Distribution of plasmid replicons and antimicrobial resistance genes in the 51 carbapenem-resistant Enterobacter cloacae complex (CREC) isolates.
3.5. Phylogeny of E. hormaechei
The phylogenetic tree of the 44 E. hormaechei isolates displayed genetic diversity, and 17 clades were distinguished (Figure 3). Ten ST418 clones formed the largest clade, indicating clonal dissemination of ST418 strains in our hospital during 2019–2021. In addition, we found clonal dissemination of ST97, ST78, and ST171 clones.
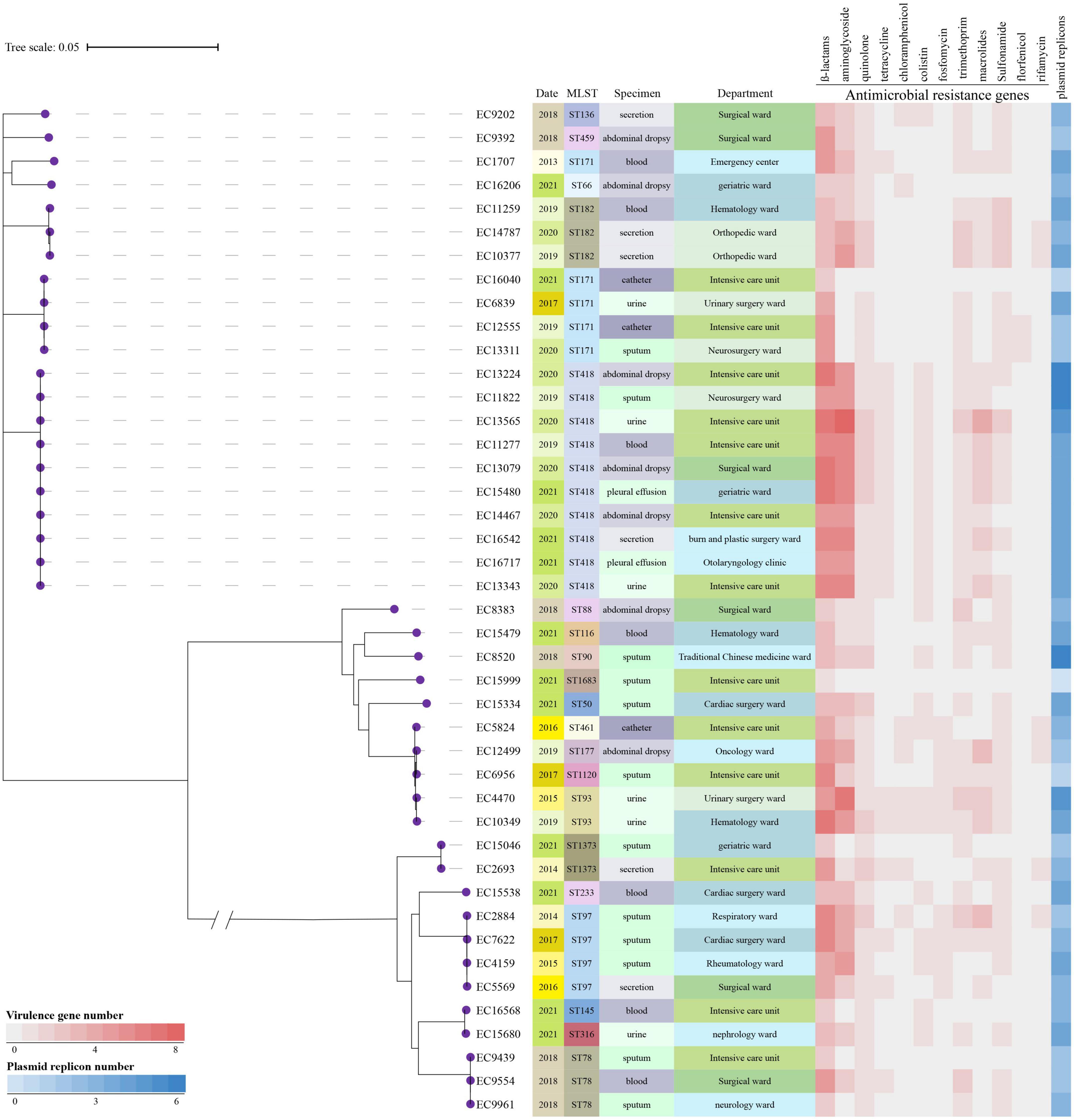
Figure 3. Phylogenetic tree and heatmap of the 44 Enterobacter hormaechei isolates. Phylogenetic tree was constructed based on single nucleotide polymorphism alignment of genomes. MLST, multi-locus sequence typing.
3.6. Risk factor analysis
The results of the comparison of clinical variables between the CREC and CSEC group are presented in Table 2. Intensive care unit (ICU) admission, autoimmune disease, pulmonary infection, and previous corticosteroid use within 1 month were the main risk factors for CREC acquisition. Multivariate analysis revealed that ICU admission (P = 0.008) was an independent risk factor for CREC acquisition (Table 3). The results shown in Table 4 indicate that ICU admission was significantly (P = 0.045) correlated with CREC acquisition with ST418 as compared to CREC acquisition with non-ST418 strains.
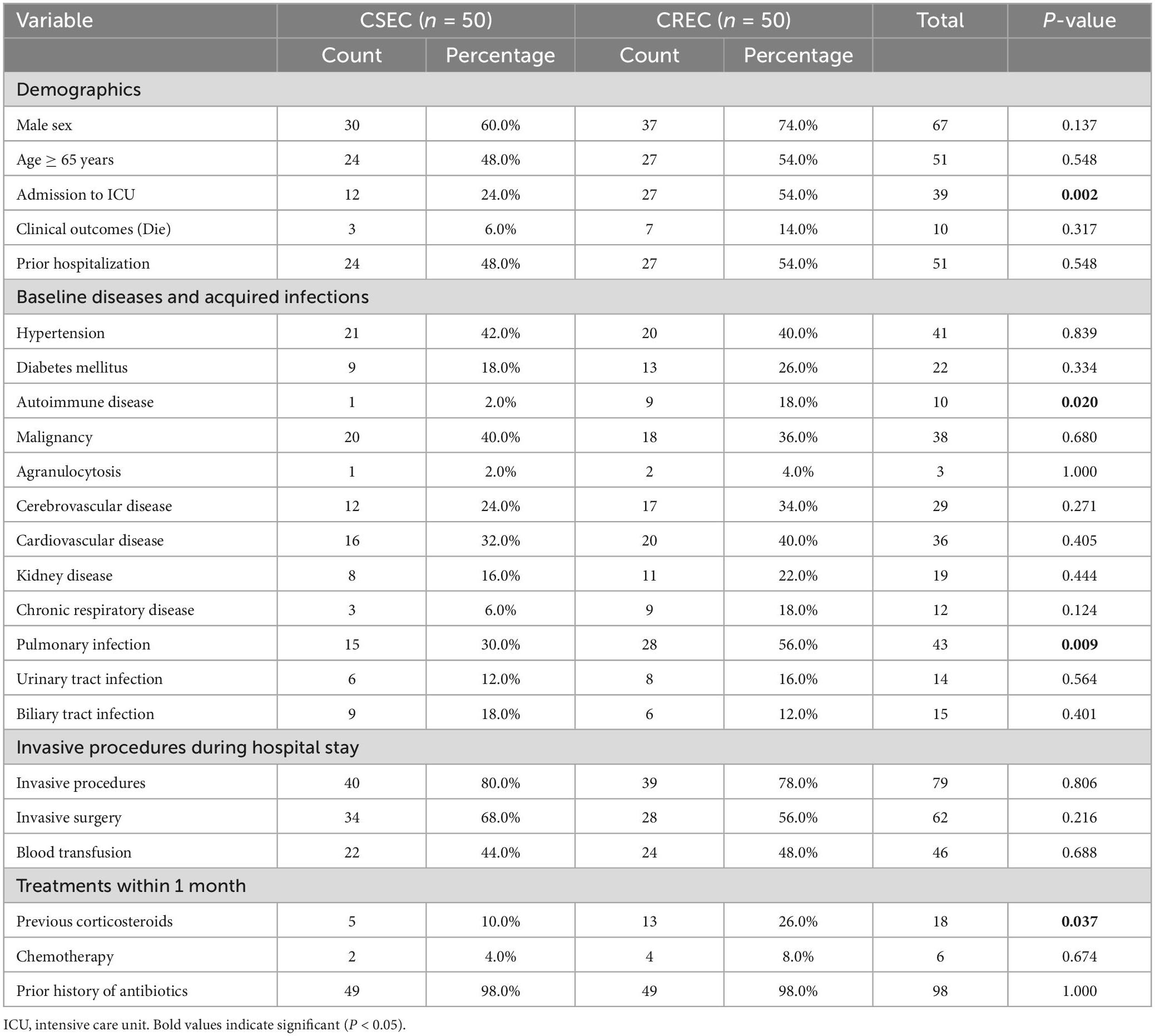
Table 2. Univariate analysis of the risk factors for acquiring carbapenem-susceptible Enterobacter cloacae complex (CSEC) and carbapenem-resistant Enterobacter cloacae complex (CREC).

Table 3. Multivariate analysis of risk factors for carbapenem-susceptible Enterobacter cloacae complex (CSEC) and carbapenem-resistant Enterobacter cloacae complex (CREC).
4. Discussion
The wide spread of the CREC poses a serious public health threat. Therefore, it is urgent to characterize the molecular epidemiology of CREC and risk factors for CREC acquisition to provide more information for guiding the formulation and implementation of infection control measures. In this study, we used WGS technology to analyze the genomic characteristics of clinical CREC isolates collected over a 9-year period in a tertiary hospital in Southeast China. To the best of our knowledge, this is the first study to analyze the genomic epidemiology and potential risk factors for acquisition of CREC isolates collected in Jiangsu Province, China.
CHβLs have increasingly spread globally over the last decades (Poirel et al., 2007). In our study, blaNDM-1 was the predominant blaCHβL, which is in accordance with the results of a multicenter study conducted in 11 Chinese cities that provided evidence that blaNDM-1 is the main CHβL conferring resistance to carbapenem (Zhao et al., 2019), although we also identified blaIMP as a major blaCHβL. Both blaNDM and blaIMP are widely disseminated among bacteria (Tzouvelekis et al., 2012). BlaIMP-4 is frequently detected in ECC strains, whereas blaIMP-26 has been rarely reported in ECC strains (Jin et al., 2020) since it was first reported in a clinical carbapenem-resistant Pseudomonas aeruginosa isolate in Singapore in 2010 (Koh et al., 2010). Notably, five E. hormaechei isolates co-carrying blaNDM-1 and blaIMP-4 were found in the clonally disseminated clone ST418, indicating that infection control measures should be implemented to prevent further spread. No strains were found to carry blaVIM-1, which is the most common blaCHβL in Southern European countries (Villa et al., 2014). BlaSHV-12 and blaTEM-1B were the predominant blaESBLs in our study which may result from the co-occurrence of these genes among the same mobile elements such as plasmids or integron, whereas CTX-M-producers are the most frequent in Latin American countries (Seki et al., 2013). Of note, all the 51 ECC isolates carried blaACT, which is a plasmid AmpC β-lactamase gene widely disseminated among Enterobacteriaceae. In addition to the constitutive expression of AmpC β-lactamase, the frequent occurrence of blaACT among these strains may lead to decreased susceptibility to cephalosporins (Davin-Regli et al., 2019).
Notably, mcr-9 was highly prevalent among the CREC isolates in this study. Albeit there was a distribution consistency between mcr-9 and IncHI2, IncHI2A, IncX3, and IncFIB, mcr-9 and these plasmids were not in the same contigs among isolates (Supplementary Table 2), so it is difficult to confirm the connection between mcr-9 and any inc type. Furthermore, as a recently emerging colistin resistance determinants, mcr-9 was identified firstly in a clinical Salmonella enterica isolate in the USA in 2019 (Carroll et al., 2019). Thereafter, the high prevalence of mcr-9 worldwide has been revealed by ongoing discoveries, posing potential threat to public health (Li et al., 2020). Although isolates harboring mcr-9 were still susceptible to colistin, active monitoring should be implemented since inducible resistance to colistin has been reported after induction of mcr-9 expression using colistin (Ling et al., 2020). Co-existence of mcr-9 and blaCHβLs within single CREC isolates as observed in our study has been reported previously. An E. hormaechei isolate co-carried mcr-9 and blaVIM-4 or blaNDM-1, but on different plasmids (Yuan et al., 2019; Soliman et al., 2020), and a clinic E. cloacae complex strain co-harbored mcr-9 and blaIMP-1 (Kananizadeh et al., 2020). In the current study, co-existence of mcr-9 and blaNDM-1, bla IMP-4, bla IMP-26, or KPC-2 was identified in more than 50% of the CREC isolates, highlighting the potential of these bacteria to develop colistin resistance. Therefore, active surveillance for mcr-9 is necessary to control further spread.
The diverse STs identified in our study indicated the genetic diversity of the ECC isolates, which was confirmed by phylogenetic analysis. Notably, the main clonally disseminated CREC strains, including ST418, ST171, ST97, and ST78, differed from the most widespread STs among global CREC isolates in 37 countries (ST114, ST93, ST90, and ST78) (Peirano et al., 2018) and from the primary epidemic ST120 clone in Henan Province, China (Liu et al., 2015). Of note, this is the first report of the spread of CREC ST418 clones during 2019–2021 in our hospital. These epidemic clones frequently co-carried blaNDM-1, blaSHV-12, and blaTEM-1B. Similarly, a study conducted in the Chinese cities Shenzhen and Dongguan indicated that ST418, which produces NDM-1 carbapenemase, is the main epidemic CREC strain (Jin et al., 2018), highlighting the necessity of active surveillance. To date, E. hormaechei ST182 was the main host for blaIMP-15 (Canada-Garcia et al., 2022); E. hormaechei ST50, ST66 and ST145 have been found to be VIM-producer in France (Emeraud et al., 2022); E. hormaechei ST90 isolates carrying the blaOXA-436 gene were suspected to be the source of the outbreak in Denmark (Raun-Petersen et al., 2022); And co-occurrence of E. hormaechei ST177 carrying blaNDM-1 and blaKPC-2 has been reported in China (Yang et al., 2018).
In Northeast USA, CREC ST171 carried blaKPC-3 on the IncFIA plasmid (Gomez-Simmonds et al., 2018), and stable uptake of the IncFIA plasmid helped ST171 to proliferate successfully throughout this area, as isolates lacking this plasmid were rare (Annavajhala et al., 2019). Although CREC ST78 clones have primarily been found in Northeast USA (Gomez-Simmonds et al., 2018), ST78 did not exhibit the same rapid clonal proliferation as ST171. Globally, CREC ST78 appears to be associated with various plasmid backbones, conferring its unique ability to acquire multidrug-resistance genes (Annavajhala et al., 2019). In Japan, ST78 isolates were found to carry blaIMP-1 on class 1 integrons encoded on multiple plasmids, such as IncHI2, IncW, and IncFIB (Aoki et al., 2018). Given the various plasmids in ST78, these strains may rapidly develop resistance to various antimicrobial agents under selective pressure. To the best of our knowledge, CREC ST252, ST1001, ST93, ST1373, ST32, ST88, ST136, ST233, ST316, ST459, ST461, ST994, ST1120, and ST1683 have not been previously reported.
Admission to the ICU was an independent risk factor of CREC acquisition in our study, which is in accordance with the finding of a study conducted in Southwest China (Tian et al., 2020), confirming the risk of ICU admission for CREC acquisition. Further analysis showed that ICU admission was also associated with the acquisition of endemic CREC-ST418 clones, which may be because endemic CREC ST418 was the main clone circulating in the ICU in our study. This was the first time we combined molecular typing with risk factor analysis.
This study had several limitations. First, although the strains were collected during 2013–2021, the sample size was relatively small and may not fully reflect the molecular epidemiology of CREC strains. Second, the study was performed in a single hospital, and the results cannot be generalized to other institutions.
In conclusion, blaNDM-1 and blaIMP-4 were the main blaCHβLs in our CREC isolates, and blaSHV-12 and blaTEM-1B were the major co-carried blaESBLs. ST418 was the predominant ST, followed by ST171 and ST97, all of which displayed clonal dissemination. Plasmid replicons were diverse, with IncHI2, IncHI2A, and IncX3 being the most frequent replicons. As the wide spread of CREC, early detection and surveillance to prevent the emergence and further spread of antibiotic resistance are urgently needed.
Data availability statement
The datasets presented in this study can be found in online repositories. The names of the repository/repositories and accession number(s) can be found in the article/Supplementary material.
Ethics statement
The studies involving human participants were reviewed and approved by the Ethics Committee of Nanjing Drum Tower Hospital (Approval No. 2022-049-2). Written informed consent from the participants’ legal guardian/next of kin was not required to participate in this study in accordance with the national legislation and the institutional requirements.
Author contributions
MH performed the bioinformatics analysis and wrote the manuscript. CL interpreted the data on the ARGs and plasmid replicons and revised the manuscript. HX sorted the data and helped with manuscript writing. JZ and YZ interpreted the data on the ARGs and plasmid replicons. CCL performed the statistical analysis. HS and XC designed the study and revised the manuscript. All authors read and approved the final manuscript.
Funding
This work was supported by the National Natural Science Foundation of China (81902124 and 82002205) and by funding for Clinical Trials from the Affiliated Drum Tower Hospital, Medical School of Nanjing University (2021-LCYJ-PY-06).
Acknowledgments
We are very grateful to Tianshe Li for providing technical help on data sorting and bioinformatic analysis. We thank editage (www.editage.cn) for English language editing.
Conflict of interest
The authors declare that the research was conducted in the absence of any commercial or financial relationships that could be construed as a potential conflict of interest.
Publisher’s note
All claims expressed in this article are solely those of the authors and do not necessarily represent those of their affiliated organizations, or those of the publisher, the editors and the reviewers. Any product that may be evaluated in this article, or claim that may be made by its manufacturer, is not guaranteed or endorsed by the publisher.
Author disclaimer
The views expressed in this article are those of the authors and do not necessarily reflect the official policy or position of the Department of Laboratory Medicine, Nanjing Drum Tower Hospital, the affiliated Hospital of Nanjing University Medical School, and the Clinical Research Center, the Second Hospital of Nanjing, Nanjing University of Chinese Medicine, Nanjing, China.
Supplementary material
The Supplementary Material for this article can be found online at: https://www.frontiersin.org/articles/10.3389/fmicb.2023.1127948/full#supplementary-material
Footnotes
- ^ https://www.ncbi.nlm.nih.gov/bioproject/PRJNA868583
- ^ https://cge.food.dtu.dk/services/MLST/
- ^ https://cge.food.dtu.dk/services/ResFinder/
- ^ https://cge.food.dtu.dk/services/PlasmidFinder/
References
Annavajhala, M. K., Gomez-Simmonds, A., and Uhlemann, A. C. (2019). Multidrug-Resistant Enterobacter cloacae complex emerging as a global, diversifying threat. Front. Microbiol. 10:44. doi: 10.3389/fmicb.2019.00044
Aoki, K., Harada, S., Yahara, K., Ishii, Y., Motooka, D., Nakamura, S., et al. (2018). Molecular characterization of IMP-1-Producing Enterobacter cloacae complex isolates in Tokyo. Antimicrob. Agents Chemother. 62:e02091–17. doi: 10.1128/AAC.02091-17
Bauer, A. W., Kirby, W. M., Sherris, J. C., and Turck, M. (1966). Antibiotic susceptibility testing by a standardized single disk method. Am. J. Clin. Pathol. 45, 493–496.
Boetzer, M., Henkel, C. V., Jansen, H. J., Butler, D., and Pirovano, W. (2011). Scaffolding pre-assembled contigs using SSPACE. Bioinformatics 27, 578–579. doi: 10.1093/bioinformatics/btq683
Canada-Garcia, J. E., Grippo, N., de Arellano, E. R., Bautista, V., Lara, N., Navarro, A. M., et al. (2022). Phenotypic and molecular characterization of IMP-producing Enterobacterales in Spain: Predominance of IMP-8 in Klebsiella pneumoniae and IMP-22 in Enterobacter roggenkampii. Front. Microbiol. 13:1000787. doi: 10.3389/fmicb.2022.1000787
Carroll, L. M., Gaballa, A., Guldimann, C., Sullivan, G., Henderson, L. O., and Wiedmann, M. (2019). Identification of novel mobilized colistin resistance gene MCR-9 in a multidrug-resistant, colistin-susceptible Salmonella enterica serotype typhimurium isolate. mBio 10:e00853–19. doi: 10.1128/mBio.00853-19
Darriba, D., Taboada, G. L., Doallo, R., and Posada, D. (2012). jModelTest 2: More models, new heuristics and parallel computing. Nat. Methods 9:772. doi: 10.1038/nmeth.2109
Davin-Regli, A., Lavigne, J. P., and Pages, J. M. (2019). Enterobacter spp.: Update on taxonomy, clinical aspects, and emerging antimicrobial resistance. Clin. Microbiol. Rev. 32:e00002–19. doi: 10.1128/CMR.00002-19
Davin-Regli, A., and Pages, J. M. (2015). Enterobacter aerogenes and Enterobacter cloacae; versatile bacterial pathogens confronting antibiotic treatment. Front. Microbiol. 6:392. doi: 10.3389/fmicb.2015.00392
Dong, X., Zhu, M., Li, Y., Huang, D., Wang, L., Yan, C., et al. (2022). Whole-Genome Sequencing-Based Species Classification, Multilocus Sequence Typing, and Antimicrobial Resistance Mechanism Analysis of the Enterobacter cloacae Complex in Southern China. Microbiol. Spectr. 10:e02160–22. doi: 10.1128/spectrum.02160-22
Emeraud, C., Petit, C., Gauthier, L., Bonnin, R. A., Naas, T., and Dortet, L. (2022). Emergence of VIM-producing Enterobacter cloacae complex in France between 2015 and 2018. J. Antimicrob. Chemother. 77, 944–951. doi: 10.1093/jac/dkab471
Gomez-Simmonds, A., Annavajhala, M. K., Wang, Z., Macesic, N., Hu, Y., Giddins, M. J., et al. (2018). Genomic and Geographic Context for the Evolution of High-Risk Carbapenem-Resistant Enterobacter cloacae Complex Clones ST171 and ST78. mBio 9, 542–518. doi: 10.1128/mBio.00542-18
Hu, K., Zhang, J., Zou, J., Zeng, L., Li, J., Wang, J., et al. (2022). Molecular characterization of NDM-1-producing carbapenem-resistant E. cloacae complex from a tertiary hospital in Chongqing, China. Front. Cell Infect. Microbiol. 12:935165. doi: 10.3389/fcimb.2022.935165
Humphries, R., Bobenchik, A. M., Hindler, J. A., and Schuetz, A. N. (2021). Overview of Changes to the Clinical and Laboratory Standards Institute Performance Standards for Antimicrobial Susceptibility Testing, M100, 31st Edition. J. Clin. Microbiol. 59:e00213–21. doi: 10.1128/JCM.00213-21
Jiang, Y., Jia, X., and Xia, Y. (2019). Risk factors with the development of infection with tigecycline- and carbapenem-resistant Enterobacter cloacae. Infect. Drug Resist. 12, 667–674. doi: 10.2147/IDR.S189941
Jiang, Y., Yang, S., Deng, S., Lu, W., Huang, Q., and Xia, Y. (2022). Epidemiology and resistance mechanisms of tigecycline- and carbapenem-resistant Enterobacter cloacae in Southwest China: A 5-year retrospective study. J. Glob. Antimicrob. Resist. 28, 161–167. doi: 10.1016/j.jgar.2022.01.005
Jin, C., Zhang, J., Wang, Q., Chen, H., Wang, X., Zhang, Y., et al. (2018). Molecular Characterization of Carbapenem-Resistant Enterobacter cloacae in 11 Chinese Cities. Front. Microbiol. 9:1597. doi: 10.3389/fmicb.2018.01597
Jin, C., Zhou, F., Cui, Q., Qiang, J., and An, C. (2020). Molecular Characteristics of Carbapenem-Resistant Enterobacter cloacae in a Tertiary Hospital in China. Infect. Drug Resist. 13, 1575–1581. doi: 10.2147/IDR.S254056
Kananizadeh, P., Oshiro, S., Watanabe, S., Iwata, S., Kuwahara-Arai, K., Shimojima, M., et al. (2020). Emergence of carbapenem-resistant and colistin-susceptible Enterobacter cloacae complex co-harboring blaIMP-1 and mcr-9 in Japan. BMC Infect. Dis. 20:282. doi: 10.1186/s12879-020-05021-7
Koh, T. H., Khoo, C. T., Tan, T. T., Arshad, M. A., Ang, L. P., Lau, L. J., et al. (2010). Multilocus sequence types of carbapenem-resistant Pseudomonas aeruginosa in Singapore carrying metallo-beta-lactamase genes, including the novel bla(IMP-26) gene. J. Clin. Microbiol. 48, 2563–2564. doi: 10.1128/JCM.01905-09
Kozlov, A. M., Darriba, D., Flouri, T., Morel, B., and Stamatakis, A. (2019). RAxML-NG: A fast, scalable and user-friendly tool for maximum likelihood phylogenetic inference. Bioinformatics 35, 4453–4455. doi: 10.1093/bioinformatics/btz305
Letunic, I., and Bork, P. (2021). Interactive Tree Of Life (iTOL) v5: An online tool for phylogenetic tree display and annotation. Nucleic Acids Res. 49, W293–W296. doi: 10.1093/nar/gkab301
Li, S., Guo, F. Z., Zhao, X. J., Wang, Q., Wang, H., An, Y. Z., et al. (2019). Impact of individualized active surveillance of carbapenem-resistant enterobacteriaceae on the infection rate in intensive care units: A 3-year retrospective study in a teaching hospital of People’s Republic of China. Infect. Drug Resist. 12, 1407–1414. doi: 10.2147/IDR.S201644
Li, Y., Dai, X., Zeng, J., Gao, Y., Zhang, Z., and Zhang, L. (2020). Characterization of the global distribution and diversified plasmid reservoirs of the colistin resistance gene mcr-9. Sci. Rep. 10:8113. doi: 10.1038/s41598-020-65106-w
Ling, Z., Yin, W., Shen, Z., Wang, Y., Shen, J., and Walsh, T. R. (2020). Epidemiology of mobile colistin resistance genes mcr-1 to mcr-9. J. Antimicrob. Chemother. 75, 3087–3095. doi: 10.1093/jac/dkaa205
Liu, C., Qin, S., Xu, H., Xu, L., Zhao, D., Liu, X., et al. (2015). New Delhi Metallo-beta-Lactamase 1(NDM-1), the Dominant Carbapenemase Detected in Carbapenem-Resistant Enterobacter cloacae from Henan Province, China. PLoS One 10:e0135044. doi: 10.1371/journal.pone.0135044
Maria, L. M., Floriana, G., and Stefania, S. (2012). Enterobacter cloacae complex: Clinical impact and emerging antibiotic resistance. Future Microbiol. 7, 887–902. doi: 10.2217/fmb.12.61
Oka, K., Matsumoto, A., Tetsuka, N., Morioka, H., Iguchi, M., Ishiguro, N., et al. (2022). Clinical characteristics and treatment outcomes of carbapenem-resistant Enterobacterales infections in Japan. J. Glob. Antimicrob. Resist. 29, 247–252. doi: 10.1016/j.jgar.2022.04.004
Page, A. J., Cummins, C. A., Hunt, M., Wong, V. K., Reuter, S., Holden, M. T., et al. (2015). Roary: Rapid large-scale prokaryote pan genome analysis. Bioinformatics 31, 3691–3693. doi: 10.1093/bioinformatics/btv421
Peirano, G., Matsumura, Y., Adams, M. D., Bradford, P., Motyl, M., Chen, L., et al. (2018). Genomic Epidemiology of Global Carbapenemase-Producing Enterobacter spp., 2008-2014. Emerg. Infect. Dis. 24, 1010–1019. doi: 10.3201/eid2406.171648
Poirel, L., Pitout, J. D., and Nordmann, P. (2007). Carbapenemases: Molecular diversity and clinical consequences. Future Microbiol. 2, 501–512. doi: 10.2217/17460913.2.5.501
Raun-Petersen, C., Toft, A., Nordestgaard, M. M., Holm, A., Overballe-Petersen, S., Hammerum, A. M., et al. (2022). Investigation of an Enterobacter hormaechei OXA-436 carbapenemase outbreak: When everything goes down the drain. Infect. Prev. Pract. 4:100228. doi: 10.1016/j.infpip.2022.100228
Seemann, T. (2014). Prokka: Rapid prokaryotic genome annotation. Bioinformatics 30, 2068–2069. doi: 10.1093/bioinformatics/btu153
Seki, L. M., Pereira, P. S., de Souza Conceicao, M., Souza, M. J., Marques, E. A., Carballido, J. M., et al. (2013). Molecular epidemiology of CTX-M producing Enterobacteriaceae isolated from bloodstream infections in Rio de Janeiro, Brazil: Emergence of CTX-M-15. Braz. J. Infect. Dis. 17, 640–646. doi: 10.1016/j.bjid.2013.03.012
Soliman, A. M., Maruyama, F., Zarad, H. O., Ota, A., Nariya, H., Shimamoto, T., et al. (2020). Emergence of a Multidrug-Resistant Enterobacter hormaechei Clinical Isolate from Egypt Co-Harboring mcr-9 and blaVIM-4. Microorganisms 8:595. doi: 10.3390/microorganisms8040595
Sutton, G. G., Brinkac, L. M., Clarke, T. H., and Fouts, D. E. (2018). Enterobacter hormaechei subsp. hoffmannii subsp. nov., Enterobacter hormaechei subsp. xiangfangensis comb. nov., Enterobacter roggenkampii sp. nov., and Enterobacter muelleri is a later heterotypic synonym of Enterobacter asburiae based on computational analysis of sequenced Enterobacter genomes. F1000Res. 7:521. doi: 10.12688/f1000research.14566.2
Tetsuka, N., Hirabayashi, A., Matsumoto, A., Oka, K., Hara, Y., Morioka, H., et al. (2019). Molecular epidemiological analysis and risk factors for acquisition of carbapenemase-producing Enterobacter cloacae complex in a Japanese university hospital. Antimicrob. Resist. Infect. Control 8:126. doi: 10.1186/s13756-019-0578-3
Tian, X., Huang, C., Ye, X., Jiang, H., Zhang, R., Hu, X., et al. (2020). Carbapenem-Resistant Enterobacter cloacae Causing Nosocomial Infections in Southwestern China: Molecular Epidemiology, Risk Factors, and Predictors of Mortality. Infect. Drug Resist. 13, 129–137. doi: 10.2147/IDR.S234678
Tzouvelekis, L. S., Markogiannakis, A., Psichogiou, M., Tassios, P. T., and Daikos, G. L. (2012). Carbapenemases in Klebsiella pneumoniae and other Enterobacteriaceae: An evolving crisis of global dimensions. Clin. Microbiol. Rev. 25, 682–707. doi: 10.1128/CMR.05035-11
Villa, J., Viedma, E., Branas, P., Orellana, M. A., Otero, J. R., and Chaves, F. (2014). Multiclonal spread of VIM-1-producing Enterobacter cloacae isolates associated with In624 and In488 integrons located in an IncHI2 plasmid. Int. J. Antimicrob. Agents 43, 451–455. doi: 10.1016/j.ijantimicag.2014.02.006
Yang, B., Feng, Y., McNally, A., and Zong, Z. (2018). Occurrence of Enterobacter hormaechei carrying bla(NDM-1) and bla(KPC-2) in China. Diagn. Microbiol. Infect. Dis. 90, 139–142. doi: 10.1016/j.diagmicrobio.2017.10.007
Yuan, Y., Li, Y., Wang, G., Li, C., Xiang, L., She, J., et al. (2019). Coproduction Of MCR-9 And NDM-1 By Colistin-Resistant Enterobacter hormaechei Isolated From Bloodstream Infection. Infect. Drug Resist. 12, 2979–2985. doi: 10.2147/IDR.S217168
Zhang, Y., Wang, Q., Yin, Y., Chen, H., Jin, L., Gu, B., et al. (2018). Epidemiology of Carbapenem-Resistant Enterobacteriaceae Infections: Report from the China CRE Network. Antimicrob. Agents Chemother. 62:e01882–17. doi: 10.1128/AAC.01882-17
Zhao, Y., Li, C., Zhang, J., Fu, Y., Hu, K., Su, S., et al. (2019). The in vitro activity of polymyxin B and tigecycline alone and combination with other antibiotics against carbapenem-resistant Enterobacter cloacae complex isolates, including high-risk clones. Ann. Transl. Med. 7:779. doi: 10.21037/atm.2019.11.33
Keywords: Enterobacter cloacae complex, carbapenem resistance, whole-genome sequencing, resistant determinants, sequence types, plasmid replicons, clinical characteristics
Citation: Han M, Liu C, Xie H, Zheng J, Zhang Y, Li C, Shen H and Cao X (2023) Genomic and clinical characteristics of carbapenem-resistant Enterobacter cloacae complex isolates collected in a Chinese tertiary hospital during 2013–2021. Front. Microbiol. 14:1127948. doi: 10.3389/fmicb.2023.1127948
Received: 20 December 2022; Accepted: 06 February 2023;
Published: 21 February 2023.
Edited by:
Maria Jorge Campos, Polytechnic Institute of Leiria, PortugalReviewed by:
Nikolaos Strepis, Erasmus Medical Center, NetherlandsGongli Zong, Shandong First Medical University, China
Guerin Francois, Centre Hospitalier Universitaire (CHU) de Rennes, France
Copyright © 2023 Han, Liu, Xie, Zheng, Zhang, Li, Shen and Cao. This is an open-access article distributed under the terms of the Creative Commons Attribution License (CC BY). The use, distribution or reproduction in other forums is permitted, provided the original author(s) and the copyright owner(s) are credited and that the original publication in this journal is cited, in accordance with accepted academic practice. No use, distribution or reproduction is permitted which does not comply with these terms.
*Correspondence: Xiaoli Cao, Y2FvLXhpYW8tbGlAMTYzLmNvbQ==; Han Shen,
c2hlbmhhbjEwMzY2QHNpbmEuY29t
†These authors have contributed equally to this work and share first authorship