Corrigendum: Ethyl lauroyl arginate: an update on the antimicrobial potential and application in the food systems industry: a review
- 1College of Food and Bioengineering, Zhengzhou University of Light Industry, Zhengzhou, China
- 2Henan Key Laboratory of Cold Chain Food Quality and Safety Control, Zhengzhou, China
Ethyl lauroyl arginate (ELA), a cationic surfactant with low toxicity, displays excellent antimicrobial activity against a broad range of microorganisms. ELA has been approved as generally recognized as safe (GRAS) for widespread application in certain foods at a maximum concentration of 200 ppm. In this context, extensive research has been carried out on the application of ELA in food preservation for improving the microbiological safety and quality characteristics of various food products. This study aims to present a general review of recent research progress on the antimicrobial efficacy of ELA and its application in the food industry. It covers the physicochemical properties, antimicrobial efficacy of ELA, and the underlying mechanism of its action. This review also summarizes the application of ELA in various foods products as well as its influence on the nutritional and sensory properties of such foods. Additionally, the main factors influencing the antimicrobial efficacy of ELA are reviewed in this work, and combination strategies are provided to enhance the antimicrobial potency of ELA. Finally, the concluding remarks and possible recommendations for the future research are also presented in this review. In summary, ELA has the great potential application in the food industry. Overall, the present review intends to improve the application of ELA in food preservation.
Introduction
Food safety is a highly complex public health issue in the world, which leads to serious adverse health consequences and large economic losses. Among various contaminants, microorganisms are reported as an important factor affecting the safety of food products. Fresh produce and processed foods can be easily contaminated by various microorganisms including bacteria, molds, yeasts, and viruses across the whole food supply chain. Bacteria and viruses are the most common cause of food poisoning and can result in a myriad of symptoms, ranging from diarrhea syndromes, fever, and even death (Bintsis, 2017). The United States Department of Agriculture (USDA) estimated that 15 major pathogens (such as Campylobacter spp., Clostridium perfringens, Salmonella, and norovirus) led to roughly 8.9 million cases of illness and an economic cost of around 17.6 billion dollars in 2018 in the United States (United States Department of Agriculture, Economic Research Service, 2022). Some microorganisms can also cause food spoilage, resulting in deterioration of the nutritional and sensory properties of foods as well as significant economic losses (Ishangulyyev et al., 2019). Therefore, how to ensure the safety of food products is one of the most important issues in the food industry. The use of chemical antimicrobial agents is one of the best and the most effective methods of preserving foods. Food antimicrobial agents can inhibit the growth of or inactivate various spoilage and pathogenic microorganisms, thereby extending the shelf life of food products. Various chemical preservatives have been used commonly so far in the food industry, such as organic acids and their salts, nitrates, nitrites, and sulfur dioxide. However, an increasing number of studies suggest that long-term exposure to chemical antimicrobial agents may result in potential health risks (Javanmardi et al., 2019; Karwowska and Kononiuk, 2020), which has received high attention both from consumers and manufacturers. Therefore, numerous efforts have been made to develop alternative antimicrobial compounds with higher safety and efficacy.
Ethyl lauroyl arginate (ELA), also known as ethyl-Nα-lauroyl-Larginate, is an amino acid-based cationic surfactant, which is synthesized from L-arginine, lauric acid, and ethanol. ELA is considered one of the most potent antimicrobial substances among novel food additives for its broad-spectrum antimicrobial activity against a wide range of bacteria, yeasts, and filamentous fungi (Ma et al., 2020; Demircan and Özdestan Ocak, 2021). The use of ELA as a food preservative has been approved by the Food Drug Administration (FDA), the European Food Safety Agency (EFSA), and other countries. In this context, the application of ELA in various food products has been widely investigated in recent years. It is therefore important to develop a better understanding of the application of ELA in the food industry. So this article aims to provide a general overview of the physicochemical properties and antimicrobial activity of ELA as well as its underlying mechanism of action. Moreover, this review also summarizes the use of ELA to improve the microbiological safety, quality attributes, and shelf life of various food products such as fruit and vegetables, meat, poultry, and dairy products. Furthermore, a detailed comprehensive review is performed on the combination strategies to enhance the potency of ELA and the factors influencing its antimicrobial efficacy. Finally, concluding remarks and suggestions for further work are also presented.
Basis of ELA
Properties and synthesis of ELA
Ethyl lauroyl arginate hydrochloride (C20H40N4O3HCl, CAS NO. 60372–77-2) is a white hygroscopic powder with a melting point at 50.5°C to 58°C. ELA has a molecular weight of 421.023 g/mol and has good water solubility (greater than 247 g/kg at 20°Cl; EFSA, 2007). The pKa of ELA is at about 10–11 and the isoelectric point is above pH 12 (Czakaj et al., 2021). The structural formula is presented in Figure 1. As a surfactant, ELA exhibits self-assembly behavior and forms micelles above the critical micelle concentration (CMC). The CMC of ELA is determined to be 0.18–0.21% (w/v; Asker et al., 2008; Bai et al., 2018). ELA includes a wide range of surface tension values from 25.4 to 31.8 (Infante et al., 1984; Czakaj et al., 2021). ELA is also used to prepare oil-in-water (O/W) emulsion with a hydrophilic–lipophilic balance (HLB) value of 10.5 (Ma et al., 2020). ELA is stable for more than 2 years at room temperature when protected in a closed container. The half-life of ELA is greater than 1 year at pH 4, 57 d at pH 7, and 34 h at pH 9 during 25°C storage, indicating that the hydrolysis of ELA is accelerated in alkaline conditions (EFSA, 2007). However, ELA has a bitter taste above 50 ppm in food and beverage products (Zheng, 2014), which may adversely influence the taste and flavor of food products.
In 1984, ELA was first synthesized by the Higher Council of Scientific Research (CSIC) in Barcelona. It was then patented and commercialized by the Venta de Especialidades Químicas S.A. (VEDEQSA) company of LAMIRSA GROUP. ELA can be synthesized by the esterification reaction between L-arginine and ethanol, followed by the amidation reaction between the obtained ethyl arginine and lauroyl chloride in an aqueous medium under appropriate temperature (10–15°C) and pH conditions (6.7–6.9). After filtration and drying, the resultant ELA is recovered as the hydrochloride salt (EFSA, 2019).
Metabolism and toxicity of ELA
According to the in vivo and in vitro studies, ELA is rapidly converted to L-arginine ethyl ester via the cleavage of lauroyl side chain or Nα-lauroyl-L-arginine (LAS) via the loss of ethyl ester (Figure 2). The resulting intermediates are further hydrolyzed to form L-arginine, which is further metabolized to urea and ornithine. Ornithine is further converted to CO2 and urea. Lauric acid is a saturated fat widely found in many vegetable fats and can enter normal fatty acid metabolism. Alcohol can be degraded to CO2 and water via some normal metabolic processes (EFSA, 2007; Hawkins et al., 2009). These data suggest that ELA is primarily and rapidly metabolized in vivo.
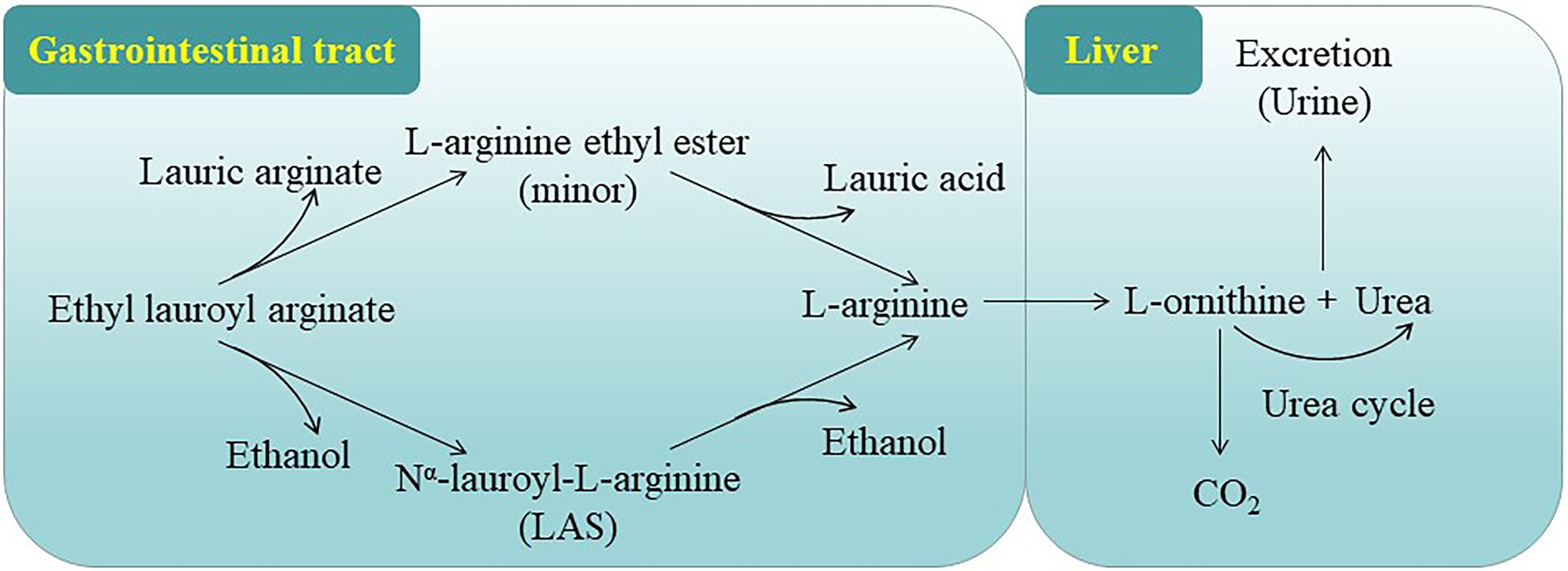
Figure 2. Proposed metabolic pathway of ELA (EFSA, 2007; Hawkins et al., 2009).
The potential toxicity of ELA has been well investigated. Based on two short-term toxicity studies, ELA has no effects on the white blood cells parameters of rats (EFSA, 2019). It has reported that highest dose ELA (15,000 mg/kg feed) had noted effects on the delay (average of 4 days) in vaginal opening in the female offspring (EFSA, 2019). However, the observed delay in vaginal opening is of no long-term toxicological relevance. The research data suggest that ELA has very low mammalian toxicity (Ruckman et al., 2004; EFSA, 2007). More toxicological metabolic investigations are reviewed by Ruckman et al. (2004). The lowest No Observed Adverse Effect Levels (NOAELs) of ELA were 47 and 56 mg/kg body weight (bw) per day for males and females, respectively (EFSA, 2019). The EFSA panel established an acceptable daily intake (ADI) of 0.5 mg/kg bw for ELA (EFSA, 2007). In June 2008, the Joint FAO/WHO Expert Committee on Food Additives (JECFA) established an ADI of 4 mg/kg bw for ethyl-Nα-lauroyl-l-arginate, the active ingredient of ELA (EFSA, 2019).
Legal aspects of ELA use in foods
In September 2005, FDA presented the No Objection Letter for ELA to be a generally recognized as safe (GRAS) compound and used as an antimicrobial agent in different types of foods at levels up to 200 ppm (FDA, 2005). In addition, the USDA approved the use of ELA in meat and poultry products at up to 200 ppm (United States Department of Agriculture, Food Safety and Inspection Service, 2022). In the European Union, ELA was evaluated for safety by the EFSA in April 2007 at the 39th EFSA evaluated Codex Committee on Food Additives and Contaminants (CCFAC). EFSA assigned the E 243 number for ELA in 2013. In May 2014, the Commission Regulation (EU) No 506/2014 was published, authoring the use of ELA as a preservative in certain heat-treated meat products. In August 2014, ELA was approved in Canada as a preservative in various foods. At present, ELA is currently authorized as a food preservative in other countries, such as Australia, New Zealand, Mexico, Colombia, Chile, Israel and Turkey, United Arab Emirates, and Vietnam, at a maximum concentration of 200 ppm (EFSA, 2019; Motta et al., 2020).
Antimicrobial activity and mechanism of ELA
ELA has attracted increasing interest due to its better antimicrobial activity against bacteria, yeasts, and molds.
Antibacterial and antibiofilm activity of ELA
Previous work had shown that ELA is active against various food spoilage and pathogenic bacteria. Table 1 summarizes the minimum inhibitory concentrations (MICs) and minimum bactericidal concentrations (MBCs) of ELA against different bacteria. The diverse MIC and MBC values of ELA are observed in the literature (Table 1) as a consequence of differences in the strains and serotypes of bacteria tested, the methods used, medium composition, and so on. According to Table 1, Gram-negative bacteria tend to be more resistant to ELA than Gram-positive ones (Infante et al., 1984; Rodríguez et al., 2004; Becerril et al., 2013; Suksathit and Tangwatcharin, 2013). Gram-negative bacteria are surrounded by an external membrane primarily composed of lipopolysaccharides and phospholipids, which acts as a permeability barrier against external toxic compounds (Miller, 2016). Gram-positive bacteria are more sensitive to ELA due to lacking the additional protection afforded by the outer membrane.
Biofilms are defined as microbial communities attached to a surface and encased in a matrix of extracellular polymeric secretions. Cells in a biofilm demonstrate much greater resistance to several acute environmental stressors (Galié et al., 2018). After treatment with ELA (50, 100, and 200 μg/ml) for 2 h, L. monocytogenes, S. Enteritidis and S. Typhimurium in biofilms on stainless steel and rubber surfaces were reduced by up to 7 and 3.5 log10 CFU/cm2, respectively (Sadekuzzaman et al., 2017). Similar results were obtained by Fu et al. (2017a) and Fernández et al. (2018). In addition, Kim et al. (2017) speculated that the antibiofilm activity of ELA against Pseudomonas aeruginosa might be attributed to its iron chelation activity and blocking effect on the iron signals associated with the biofilm development.
Antifungal activity of ELA
Yeasts and molds can cause various degrees of deterioration and decomposition of foods, such as grains, nuts, meat, milk, fruits, and vegetables. According to literature, ELA displays strong antifungal activities against various yeasts and molds (Table 2). Typically, the MIC values of ELA against Saccharomyces cerevisiae, Candida albicans, and Zygosaccharomyces bailii were 35, 112.5, and 62.5 μg/ml, respectively (Loeffler et al., 2014). Similarly, ELA showed in vitro antifungal potential against Botrytis cinerea, Alternaria alternate, Penicillium italicum, and Penicillium digitatum with a MIC value of 400, 200, 400, and 400 μg/ml, respectively (Li et al., 2018). It should be pointed out, however, that yeasts and molds exhibit significantly greater resistance to ELA than bacterial cells based upon the MIC values in Tables 1, 2, which may be due to the differences in the structure and chemical composition of cell walls.
Possible antimicrobial mechanism of ELA
Although the antimicrobial mechanism of ELA has not been fully deciphered, microbial cell membranes are thought to be the main target of ELA. As a cationic surfactant, ELA can damage cell membranes, leading to the disruption of cell membranes, the lose of membrane potential, and leakage of cellular components (Figure 3). Pattanayaiying et al. (2014) investigated the effects of ELA on the morphological and ultrastructural features of E. coli O157:H7, L. monocytogenes, and Brochothrix thermosphacta cells by scanning electron microscopy and transmission electron microscopy. The results showed that ELA caused remarkable changes in the ultrastructure and morphology of bacterial cells, such as distorted and dimpled E. coli O157: H7 cells and the intracytoplasmic coagulation of B. thermosphacta cells. In another work, Coronel-León et al. (2016) studied the influences of ELA on the membrane potential and permeability of bacterial cells by flow cytometry. After ELA treatment at 1 × MIC, the percentage of bisoxonol-positive Yersinia enterocolitica and Lactobacillus plantarum cells were increased to 1.8 and 0.3%, respectively, significantly higher than that of the control cells (0.6 and 0.1%, respectively), suggesting the depolarization of the membrane. The percentages of propidium iodide-positive Y. enterocolitica and L. plantarum cells increased to 97.8 and 99.6%, respectively, significantly higher than that of the control cells (0.7 and 0.01%, respectively), after exposure to ELA at 1 × MIC, indicating the significant increase in cell membrane permeability (Coronel-León et al., 2016). As results of cell membrane disruption, ELA causes releases of intracellular contents such as potassium (Rodríguez et al., 2004; Coronel-León et al., 2016), proteins (Xu et al., 2018; Zhao et al., 2022a), nucleic acids (Xu et al., 2018; Yang et al., 2019), and ATP (Ma et al., 2016c), and disrupt normal cellular metabolism, thereby leading to cell death. Meanwhile, ELA may bind to the cellular components with negative charges after entry into the microbial cells, thereby affecting the normal metabolic function. For instance, Ma et al. (2016c) observed a strong interaction between ELA and bacterial DNA, a negatively charged polymer, through electrostatic attraction and hydrophobic interaction.
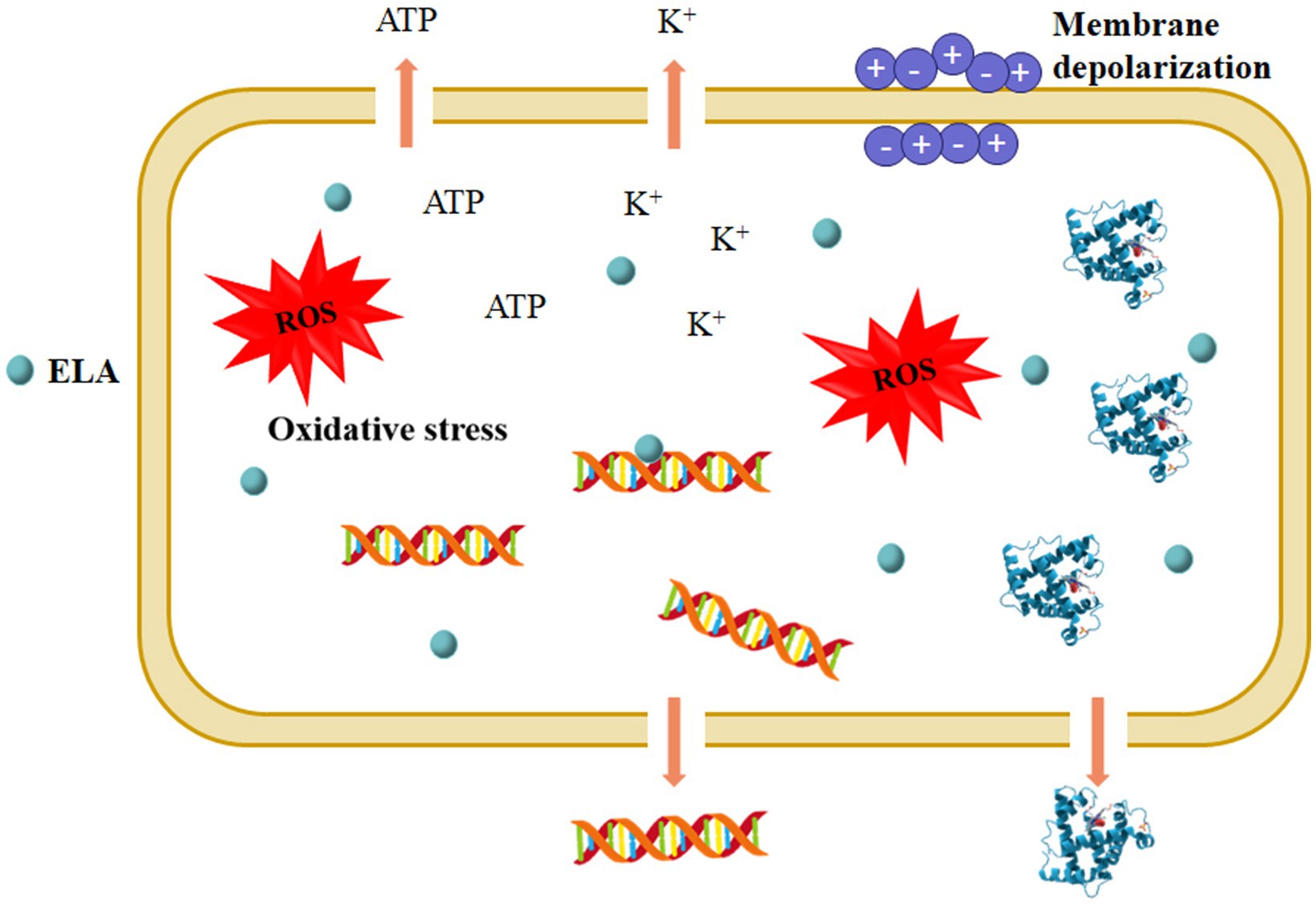
Figure 3. Proposed mechanisms underlying the antimicrobial action of ELA. ROS, reactive oxygen species.
The generation of intracellular reactive oxygen species (ROS) is thought to be involved in the antimicrobial action of ELA (Figure 3). As revealed by Yang et al. (2019), the co-administration of antioxidants (such as glutathione and ascorbic acid) effectively suppressed the inactivation efficacy of ELA against E. coli O157:H7 and L. innocua, suggesting that oxidative stress was directly associated with ELA-induced microbial inactivation. Excessive ROS can cause irreversible oxidative damage to cellular components (such as DNA, proteins, and lipids) and activate signaling pathways, leading to the disruption of normal cellular functions and ultimately cell death. However, the pathways of ELA-induced ROS generation in microbial cells are still not clear, and more detailed research is required.
In summary, the antimicrobial effect of ELA is mainly related to membrane damage and oxidative stress (Figure 3). On the whole, the present research is mainly focused on the influences of ELA on the structure and function of microbial cells. While multiomics-based analyses (i.e., transcriptomics, proteomics, and metabolomics) should be used to elucidate the underlying molecular mechanisms for the action of ELA. Additionally, the molecular dynamics simulation can be used to explore the membrane binding and disruption mechanisms of ELA (Velasco-Bolom et al., 2018).
Application of ELA as a food preservative
ELA has been widely studied to extend the microbiological shelf-life and the quality characteristics of various food categories, such as meat and meat products, fruits and vegetables, dairy products, and aquatic products. Similar to other antimicrobial agents, ELA has the possibility of being directly added into the food samples or being incorporated into an active packaging (Figure 4).
Meat and meat products
Microbial contamination of meat and meat products is a global health issue, which leads to foodborne illnesses and food poisoning (Heredia and García, 2018). As an ingredient on the GRAS list, ELA has been approved by the FDA for use in meat and poultry products up to a maximum level of 200 ppm (FDA, 2005). In Europe, ELA is currently authorized in heat-treated meat products, smoked sausages and liver paste up to the level of 160 mg/kg food (EFSA, 2019).
ELA can be applied to meat and meat products by direct addition, spray, dip, or brush (Table 3). As reported by Peng et al. (2021), the ELA supplement at 0.1 or 0.3 g/kg effectively inhibited the growth of bacteria in yak sausage during 15 days of storage at 0–4°C. Meanwhile, ELA also resulted in significant reductions in drip loss, cooking loss, total volatile basic nitrogen (TVB-N) contents, and pH values of yak sausage during the 15 day storage period. Previous studies showed that ELA-spray treatment can effectively inactivate or prevent the growth of microorganisms on meat and meat products, such as ground beef (Dias-Morse et al., 2014), chicken meat (Hawkins et al., 2016), and ham products (Taormina and Dorsa, 2009a; Lavieri et al., 2014).
Antimicrobial polymer films loaded with ELA have been also designed and applied to meat and meat products (Theinsathid et al., 2012; Higueras et al., 2013; Guo et al., 2014; Muriel-Galet et al., 2015; Kashiri et al., 2019; Hassan and Cutter, 2020; Tirloni et al., 2021). For instance, Higueras et al. (2013) investigated the antimicrobial activity of chitosan-5% ELA film against mesophiles, psychrophiles, Pseudomonas spp., colifoms, lactic acid bacteria on fresh chicken breast fillets during storage at 4°C for up to 8 days. The results showed that chitosan-5% ELA film produced 1.78–5.81 log reduction of the tested bacterial species. Similar findings were shown by Hassan and Cutter (2020) that the composite antimicrobial film containing 0.5, 1.0%, or 2.5% ELA also could control foodborne pathogens associated with muscle foods effectively including raw beef, raw chicken breast, and ready-to-eat turkey breast. Besides, it has been showed that ELA is more effective when combined with other antimicrobials, such as potassium lactate, and sodium diacetate (Martin et al., 2009; Porto-Fett et al., 2010; Stopforth et al., 2010), and bacteriophage (Yang et al., 2017).
Fruits and vegetables
Fresh fruits and vegetables can be contaminated with harmful microorganisms, which continue to be an important source of foodborne disease outbreaks. ELA has been studied as antimicrobial for treating fresh produce (Table 4), such as apples (Shen et al., 2021), strawberries (Li et al., 2021), cantaloupes (Ma et al., 2016c), bell peppers (Li et al., 2020), lettuce (Huang and Nitin, 2017; Nübling et al., 2017b), and spinach (Ruengvisesh et al., 2015; Zhao et al., 2022b). As reported, the E. coli O157:H7 and L. innocua cells on lettuce leaves decreased by more than 3-log after washing with ELA solution (0.1% w/w) for 20 min with shaking at 200 rpm (Huang and Nitin, 2017). Moreover, the quality attributes of fresh produce were maintained well after ELA treatment (Ma et al., 2016c; Huang and Nitin, 2017; Nübling et al., 2017a; Li et al., 2020). In addition, ELA can also effectively inactivate foodborne pathogens in the washing water (Nübling et al., 2017b; Zhao et al., 2022b). For instance, after washing endive in water containing 100 mg/l of ELA at 4 or 45°C for 2 min, the total aerobic mesophilic count in the process water of the pilot plant decreased by 2.7- and more than 4-log, respectively (Nübling et al., 2017b). Therefore, ELA may reduce the chance of cross-contamination while washing fruits and vegetables.
In addition, ELA also could effectively inactivate biofilms formed on fruits and vegetables (Sadekuzzaman et al., 2017; Fu et al., 2017a,b). As reported by Sadekuzzaman et al. (2017), 200 μg/ml of ELA reduced E. coli O157:H7, L. monocytogenes, S. Enteritidis, and S. Typhimurium biofilms up to 1.5 log10 CFU/cm2 on the lettuce leaf surface (p < 0.01). Fu et al. (2017a) also made a similar observation that the viable bacterial of 12 h- and 24 h-old E. coli O157:H7 biofilm on cantaloupe rind decreased by 1.74- and 1.21-log, respectively, after ELA treatment (2 mg/ml) at 22°C for 5 min.
Dairy products
Dairy products, such as milk, cheese, and butter, can be contaminated with harmful bacteria, resulting in serious health risk. Soni et al. (2010) reported that the population of L. monocytogenes in whole milk or skim milk with initial counts of 4 log10 CFU/mL was reduced by approximately 1-log with 200 μg/ml of ELA for 24 h. After treatment with 800 μg/ml of ELA for 24 h, L. monocytogenes counts in the samples were reduced to nondetectable levels, and there was no subsequent regrowth of L. monocytogenes after their extended storage at 4°C for up to 15 d. Similar findings were also revealed in queso fresco cheese (Soni et al., 2010, 2012). For queso fresco cheese treated with 200 or 800 μg/ml of ELA, the L. monocytogenes populations decreased by 1.2- and 3.0-log within 24 h at 4°C, respectively. During the 28 d storage at 4°C, L. monocytogenes counts in untreated controls increased from the initial 4 log10 CFU/g to 8.3 log10 CFU/g. In the same condition, the overall growth of L. monocytogenes decreased by 0.3 to 2.6-log for cheese with 200 μg/ml of ELA and by 2.3 to 5.0-log for samples with 800 μg/ml of ELA. As reported by Woodcock et al. (2009), the aerobic plate count in unflavored milk with ELA (125, 170, or 200 μg/ml) remained below the regulatory limit of 20,000 CFU/ml for grade ‘A’ pasteurized milk during 21 days of storage at 6°C. However, the antimicrobial activity of ELA added to chocolate milk was reduced compared to unflavored milk, which might be due to the stabilizers (e.g., carrageenan) in the chocolate powder. The combinations of ELA with other antimicrobial agents were also used in dairy products such as essential oils (Ma et al., 2013), ε-polylysine, and nisin (Martínez-Ramos et al., 2020). According to Ma et al. (2013), the combination of ELA with cinnamon leaf oil or eugenol exhibited synergistic against L. monocytogenes in 2% reduced-fat milk. In the work of Martínez-Ramos et al. (2020), a synergistic interaction was observed for ELA and ε-polylysine against L. monocytogenes in queso fresco cheese.
Aquatic products
Aquatic products, like any food item, can be contaminated with a variety of bacteria and viruses capable of causing disease in consumers (Iwamoto et al., 2010). The potential of ELA to improve the microbial safety and quality of aquatic products has been assessed in several studies (Soni et al., 2014; Zhuang et al., 2020). For instance, Soni et al. (2014) determined the efficacy of ELA for the inactivation of L. monocytogenes inoculated on cold-smoked salmon. The results indicated that the salmon treated with ELA (200 ppm) individually for 24 h at 4°C were able to achieve a 2.2 log reduction of L. monocytogenes. In recent work, Zhuang et al. (2020) assessed the effects of ELA on microbiota, quality, and biochemical changes of largemouth bass fillets during storage. The fillets were immersed in sterile water and 0.1% (w/v) ELA solution for 10 min, respectively, and then were stored at 4°C. The total viable counts (TVC) of ELA-treated samples were significantly lower than that of the control during the storage up to 11 d, suggesting that ELA was effective in the washing/cleaning of aquatic products. Meanwhile, ELA also attenuated effectively the changes in the color, TVB-N, ammonia concentration, and biogenic amines of chill-stored largemouth bass fillets. In addition, edible films incorporated with ELA were also used for aquatic products preservation. Demircan and Özdestan Ocak (2021) found that edible chitosan film coating with ELA (0.1%, w/v) significantly inhibits bacterial growth and biogenic amines formation of mackerel fillets during storage at 2°C. Similarly, active starch-gelatin films with ELA could effectively inhibit the microbial growth in marinated salmon and extend the product shelf life in terms of microbial spoilage (Moreno et al., 2017).
Other food products
The antimicrobial effect of ELA has been investigated with other food products. Chen et al. (2015) investigated the antimicrobial activity of ELA against Salmonella in peanut paste at different fat concentrations. For peanut paste with different water activities (aw) of 1.0 and 0.7, 5,000 ppm of ELA reduced the population of S. Tennessee in low fat (< 5%) peanut paste by 0.92- and 4.08-log, respectively, after 5-day storage at 25°C. In addition, the counts of S. Tennessee in low fat (< 5%) peanut paste with aw 0.5 and 0.3 were reduced to undetectable levels after 24 h with 5,000 ppm of ELA. The efficacy of ELA is also negatively affected by the fat concentration. For peanut paste with 50% fat, ELA at 5,000 ppm caused a 1.58-log reduction in 5 days compared with the control (Chen et al., 2015). In contrast, the spray application of 0.2% ELA and 200 ppm peracetic acid did not cause significant reductions in the aerobic plate count or E. coli/coliform counts of California walnuts (Frelka and Harris, 2015).
Active food packaging
In recent decades, various antimicrobial substances have been wildly incorporated into food packaging systems to extend the shelf life of food products. Data from the literature indicate that ELA has been utilized to prepare active antimicrobial food packaging materials. Generally, ELA is incorporated into petroleum-based polymers (such as polyethylene and ethylene vinyl alcohol copolymers; Muriel-Galet et al., 2015; Manso et al., 2021) and biopolymers such as poly-γ-glutamic acid (Gamarra-Montes et al., 2018), polylactic acid, chitosan, pullulan, starch, and zein (Table 5). According to previous studies, ELA-incorporated food packaging materials exhibit excellent antimicrobial activity. For example, Muriel-Galet et al. (2015) prepared ethylene vinyl alcohol copolymers (EVOH) films containing ELA at 5% or 10% (w/w). All the ELA-incorporated films displayed antimicrobial capability against L. monocytogenes and E. coli both in vitro and in inoculated ready-to-eat surimi sticks. On the 10th day of storage at 4°C, L. monocytogenes and E. coli on samples wrapped in EVOH films with 10% ELA decreased by >3.25- and > 2.32- log, respectively.
Although there are various reports on the food packaging materials with ELA (Table 5), most of which are confined to the laboratory, due to the absence of suitable large-scale manufacturing processes for continuous production. The electrospinning process has been regarded as one suitable method for the large-scale production of long and continuous nanofibers. In several recent studies, the electrospinning technique was used to prepare polymer nanofibers with ELA. Deng et al. (2018) prepated chitosan/poly(ethylene oxide /ELA) composite nanofibrous films via electrospinning, which displayed excellent antimicrobial activity against E. coli and Staphylococcus aureus. In a recent work of Li et al. (2021), the authors prepared polylactic acid/ELA composite nanofibrous films via electrospinning. According to the results of the disc diffusion assay, the active films exhibited outstanding antimicrobial activity against E. coli O157:H7, S. aureus, and Botrytis cinerea. Meanwhile, the active films with ELA could also effectively extend the shelf-life of strawberries at 25°C. Summarily, considerable attention should be devoted to the large-scale production and practical application of active food packaging with ELA.
Disinfection of food-contact surfaces
Food contact surfaces are considered to be the primary source of microbial contamination within food processing areas. Another potential application for ELA is the decontamination of food processing surfaces and equipment. Saini et al. (2013) investigated the efficacy of ELA against L. monocytogenes on polished stainless steel coupons. After exposure to ELA at 100 μg/ml for 5 and 10 min, L. monocytogenes with an initial level of 4 log10 CFU/coupon decreased by 1.38- and 2.57-log, respectively. Similar findings were observed in bacterial biofilms on food contact surfaces, such as stainless steel, rubber (Sadekuzzaman et al., 2017), and glass (Fu et al., 2017a; Fernández et al., 2018). As previously reported by Sadekuzzaman et al. (2017), the populations of E. coli O157:H7, L. monocytogenes, S. Enteritidis and S. Typhimurium in biofilms grown on stainless steel decreased from 6.0, 7.2, 5.4, and 5.1 log10 CFU/cm2 to values below the detection limit, respectively, after ELA treatment at 200 μg/ml for 2 h. Significant biofilm reduction in rubber surface was also observed for all the tested strains after ELA treatment (50–200 μg/ml) for 2 h.
Combination of ELA with other technologies
Studies have indicated that ELA alone may not be sufficient for food preservation due to the influences of food constituents and treatment conditions (such as pH and temperature). The combination of ELA and other hurdles has been highlighted as a feasible strategy to enhance microbial inactivation by additive or synergistic effects. Some combined approaches have already proved successful in this regard, such as methylparaben (Li et al., 2018), nisin and ε-polylysine (Martínez-Ramos et al., 2020), essential oils or their constituents (Manrique et al., 2017), mild heat and ultraviolet (Yang et al., 2019), and high hydrostatic pressure (Seemeen, 2011).
ELA with antibacterial agents
Several benefits have be resulted from the combination of ELA with other antibacterial agents, such as methylparaben (Li et al., 2018; Loeffler et al., 2020), ε-polylysine (Kozak et al., 2017; Martínez-Ramos et al., 2020), nisin (Pattanayaiying et al., 2014; Soni et al., 2014), potassium lactate and sodium diacetate (Martin et al., 2009), organic acid salts (Luchansky et al., 2005; Suksathit and Tangwatcharin, 2013), and peracetic acid (Shen et al., 2021).
Parabens, also known as para-hydroxybenzoic acid esters, are a class of antimicrobial preservatives allowed for use in foods. It is proven that ELA exhibits higher antibacterial and antifungal activities in combination with methylparaben (Li et al., 2018; Loeffler et al., 2020). As reported by Loeffler et al. (2020), the MIC values of ELA against L. innocua and P. fluorescens in nutrient broth with 2% bovine serum albumin (BSA) were 300 and 200 μg/ml, respectively. However, the MIC values of ELA against L. innocua or P. fluorescens decreased to 175 and 125 μg/ml, respectively, when combined with 0.1% methylparaben in the same testing condition. Similar findings were also obtained by Li et al. (2018) that ELA combined with methylparaben displayed enhanced antifungal activity. For instance, the MIC values of ELA and methylparaben against P. italicum were 400 and 800 μg/ml, respectively; while the MIC for ELA was reduced to 50 μg/ml in the presence of methylparaben at 200 μg/ml (Li et al., 2018).
ε-polylysine, a naturally antimicrobial cationic peptide, is commercially used as a safe food preservative globally. Martínez-Ramos et al. (2020) evaluated the antimicrobial combinations of ELA and ε-polylysine on L. monocytogenes growth in queso fresco. For the cheeses treated with ε-polylysine (250 μg/g) and ELA (200 μg/g), the L. monocytogenes population increased by approximately 1.5-log stored for 28 d at 4°C, lower than the control samples (~3 log10 CFU/g growth from the initial inoculum). Similar findings showed that the combined applications of ELA with ε-polylysine resulted in a significant reduction of L. monocytogenes in whole milk (Kozak et al., 2018) and Salmonella on sterile filter paper (Benli et al., 2011).
Nisin is a polypeptide bacteriocin and is commonly used as a food preservative. Pattanayaiying et al. (2014) investigated the effects of ELA and nisin, alone or in combination on the survival of bacteria. For instance, the population of E. coli O157:H7 decreased by 4.45-log after ELA treatment at 0.2 mg/ml for 6 h, while the bacterial cells were not inhibited by nisin at 320 AU/ml alone. Interestingly, the E. coli O157:H7 population decreased by approximately 7.16-log within the first hour of the combination treatment with ELA and nisin. Similarly, L. monocytogenes in cold-smoked salmon was reduced from 3.5 log CFU/cm2 to an undetectable level after the treatment of ELA (200 μg/ml) and nisin (500 μg/ml; Soni et al., 2014). The enhanced antimicrobial activity of ELA combined with nisin may be probably due to the formation of membrane channels and leakage of intracellular constituents such as potassium and phosphate ions (Pattanayaiying et al., 2014).
ELA with essential oils
Essential oils are complex mixture of plant volatile compounds, which posse broad-spectrum antimicrobial activity. The antimicrobial activity of ELA combined with essential oils or their constituents was assessed in previous work (Ma et al., 2013, 2016a; Manrique et al., 2017). In a previous study, ELA (5 mg/l), cinnamon essential oil (200 mg/l), or EDTA (500 mg/l) did not exhibit antibacterial activity against E. coli O157:H7, S. Enteritidis, and L. monocytogenes in tryptic soy broth (TSB; Ma et al., 2016a). In contrast, the population of E. coli O157:H7, S. Enteritidis, and L. monocytogenes decreased by 4.70-, 5.01-, and 1.71-log, respectively, after the combined treatment of 5 mg/l ELA, 500 mg/l EDTA and 200 mg/l cinnamon essential oil (Ma et al., 2016a). Similarly, ELA combined with cinnamon essential oil exhibited much greater antibacterial activity against L. monocytogenes in 2% reduced-fat milk during 48 h of incubation at 21°C (Ma et al., 2013). Manrique et al. (2017) investigated the bacterial inactivation after the simultaneous or sequential application of ELA and eugenol. The simultaneous exposure of ELA and eugenol was found to be the most effective to inactivate Staphylococcus carnosus and L. innocua.
However, the application of essential oils as antibacterial agents is limited by their low water solubility, high volatility, and low long-term stability. The encapsulation of essential oils in nanoemulsions is an effective approach to overcome these limitations. As a cationic surfactant, ELA is widely used to prepare essential oil nanoemulsions. Chang et al. (2015) found that ELA addition substantially increased the antimicrobial efficacy of thyme oil nanoemulsions against Zygosaccharomyces bailii, an acid-resistant spoilage yeast. On the other hand, Ma et al. (2016b) reported that mixing ELA with lecithin could improve the physical properties of nanoemulsions based on thymol-eugenol mixtures. Nonetheless, the presence of nanosized lipid droplets in thyme oil-in-water nanoemulsions reduced the antifungal activity of ELA, which might be due to the partitioning of ELA between the lipid droplet surfaces and the yeast cell surfaces (Ziani et al., 2011). Therefore, future studies should concentrate on the interactions of surfactants and lipid droplets in essential oils nanoemulsions, which may help to improve the design of more effective antimicrobial delivery systems.
ELA with mild heat
Recently, mild heat-based hurdles have been applied as novel food decontamination techniques. ELA combined with mild heating demonstrates synergistic antibacterial activity (Yang et al., 2019; Juneja et al., 2020). As reported by Yang et al. (2019), E. coli in sterile phosphate buffered saline (PBS) decreased by approximately 5-log after being treated with ELA (15 μg/ml) and mild heat (55°C) for 4 min. In contrast, ELA or mild heat alone demonstrated no significant effects on E. coli inactivation within 4 min of treatment. Significant synergistic inactivation of L. innocua was also observed after the combination treatment with ELA and mild heat under the same experimental condition.
Similar findings were also reported by Juneja et al. (2020), who examined the efficacy of ELA to reduce the L. monocytogenes population in ground beef following sous-vide processing at different temperatures. The D-values obtained from the Weibull model ranged from 43.74 to 4.47 min at 55–62.5°C. With the addition of ELA at 3 mg/g, the D-values at 55 to 62.5°C determined by the Weibull model were 22.71 and 1.60 min. ELA in beef increased the sensitivity of L. monocytogenes to sous-vide treatment, thereby extending the shelf-life and improving the product quality.
ELA with non-thermal technologies
Non-thermal technologies, such as ultraviolet (UV) light, high hydrostatic pressure (HHP), ultrasound, cold plasma, and pulsed electric field, have been used in the sanitization of food products (Wu et al., 2020). These non-thermal technologies are operated at normal temperature conditions and with very short times, which helps to improve the sensorial and nutritional quality of foods. Several studies have reported the synergistic efficacy of ELA combined with non-thermal technologies, such as UV light (Yang et al., 2019) and HHP (Seemeen, 2011).
UV light possesses excellent germicidal properties against various microbial pathogens and has already been applied in the food industries. Yang et al. (2019) evaluated the antibacterial activity of ELA in combination with UVA. ELA treatment (15 μg/ml) alone inactivated 2 logs of E. coli O157:H7 in sterile PBS after 30 min of incubation, while UVA (320 to 400 nm) alone produced no significant inactivation of O157:H7 cells. In contrast, the E. coli O157:H7 population was decreased by approximately 6.5-log after the combined treatment with ELA and UVA for 30 min. A similar phenomenon was observed in L. innocua after ELA treatment alone or in combination with UVA. The authors also proposed that the synergistic action of ELA and UVA might be due to the enhanced oxidative stress and exacerbated membrane damage (Yang et al., 2019). HHP is an emerging non-thermal process technique and can inactivate harmful microorganisms in foods by intense pressure. Seemeen (2011) studied the effects of ELA and HPP on the shelf-life of ready-to-eat cooked chicken breast roast during storage at 4°C for 16 weeks. Aerobic plate counts (APCs) of chicken breast roast samples only decreased by 0.5- and 0.05-log, respectively, after exposure to ELA (200 μg/ml) or HHP (450 MPa for 1 min) alone followed by storage at 4°C for 16 weeks. For samples treated with HPP and ELA at 450 MPa for 1 min, APC decreased by 2.67-log. Therefore, ELA combined with HPP is an efficient method for extending the microbial shelf-life of the ready-to-eat sliced chicken breast roast.
ELA with bacteriophages
Bacteriophages (phages) are bacterial viruses and can be used as narrow-spectrum antibacterials in food production for various advantages such as low inherent toxicity and no adverse environmental impact (Lewis and Hill, 2020). At present, some phage cocktails are available commercially and are currently used as either food additives or GRAS, such as ListShield™ and PhageGuard Listex™ P100. ELA does not affect the antibacterial activity of bacteriophages because phage particle is mainly composed of a protein molecule embedded in a capsid (Yang et al., 2017). The antibacterial activity of LEA combined with bacteriophages was investigated in several studies (Soni et al., 2012, 2014; Sukumaran et al., 2015; Yang et al., 2017).
According to previous studies (Yang et al., 2017), the counts of surviving L. monocytogenes on chicken breast decreased by 0.07- and 0.06-log, respectively, after a single round of treatment with bacteriophages (ListShield) or 100 mg/kg of ELA and storage at 4°C for 3 days. After the combined treatment with 100 mg/kg of ELA and bacteriophages, 0.43-log reduction was observed for L. monocytogenes on the chicken breast after storage at 4°C for 3 days. Moreover, the combined treatment with ELA and bacteriophage did not significantly affect the surface color parameters, sensory properties, pH, and thiobarbituric acid reactive substances (TBARS) content of chicken breasts (Yang et al., 2017). ELA combined with bacteriophages was also used for the preservation of queso fresco cheese (Soni et al., 2012) and cold-smoked salmon (Soni et al., 2014). As revealed by Soni et al. (2014), L. monocytogenes cells in cold-smoked salmon were reduced from 3.5 log10 CFU/cm2 to an undetectable level within 24 h after the combined treatments of ELA (200 ppm) with bacteriophage P100 (Listex™ P100, 108 PFU/cm2). Further studies are still needed to reveal the complex mechanisms underlying combined treatment with ELA and bacteriophages.
Factors affecting the antimicrobial activity of ELA
Though the antimicrobial of ELA has been already approved in previous publications, its effectiveness in practical application is still challenged by the treatment conditions and the natural specific characteristics of foods. Previous research shows that the antimicrobial efficacy of ELA is influenced by many factors, such as its concentration, exposure time, the particular properties of the microorganisms targeted, temperature, pH, and the characteristics of the treatment medium or foods.
ELA concentration and treatment time
Generally, ELA exhibits enhanced antimicrobial activity at high concentrations. After ELA treatment at 0.001 and 0.01% with peracetic acid (80 ppm) for 30 s, L. monocytogenes with an initial population of 7.06 log10 CFU/mL decreased by 1.48- and more than 5- log reduction, respectively (Shen et al., 2021). The anti-biofilm potential of ELA is also significantly enhanced with increasing concentration (Sadekuzzaman et al., 2017; Fu et al., 2017a). ELA displays higher antimicrobial activity with increasing exposure time (Becerril et al., 2013; Shen et al., 2021). For instance, L. innocua in broth medium decreased by 2.5- and 4- log after exposure to 25 μg/ml of ELA for 2 and 4 min, respectively (Becerril et al., 2013). Similarly, L. monocytogenes incubated on apples decreased by 2.48- and 2.58-log after the combined treatment of ELA (0.05%) and peracetic acid (80 ppm) for 30 s and 2 min, respectively (Shen et al., 2021).
Characteristics of microorganisms
The antimicrobial activity of ELA is influenced by the types, status, and population of microorganisms. As seen in Tables 1, 2, different microorganisms show various sensitivity to ELA. For instance, the MICs and MBCs of ELA against fungi are generally higher than that against bacteria, which may due to the different chemical composition and structures of their cell walls. Loeffler et al. (2014) assessed the antimicrobial efficacy of ELA against S. cerevisiae, C. albicans, and Z. bailii. S. cerevisiae was the most sensitive strain to ELA with a MIC value of 35 μg/ml (112.5 μg/ml for C. albicans and 62.5 μg/ml for Z. bailii). The antimicrobial efficacy of ELA is also affected by the serotypes of microorganisms. For instance, the MIC of ELA was 0.004% for L. monocytogenes 10403S (serotype 1/2a) and was 0.005% for L. monocytogenes 2045 (serotype 4b) at 37°C (Lingbeck et al., 2014). In addition, the specific physiological status of microbial cells (such as susceptibility and resistance, tolerance, persistence, and biofilm) may also affect the antimicrobial activity of ELA. For example, the antimicrobial activity of ELA is significantly affected by the biofilm growth age. After exposure to ELA at 80 μg/ml for 5 min, the viable bacterial of 2 h- and 24 h-old E. coli O157:H7 biofilms on cover glass decreased by 2.65- and 0.63- log, respectively (Fu et al., 2017a).
Temperature
Temperature affects the antimicrobial activity of ELA. In the work of Lingbeck et al. (2014), Listeria and Salmonella were treated with ELA and then incubated at different temperatures for 24 h (4, 10, or 37°C for Listeria and 10, 25, or 37°C for Salmonella). The results showed that ELA exhibited stronger antibacterial activity when used at a higher incubation temperature. For example, the MICs of ELA against Salmonella were 0.072% at 10°C, 0.035% at 25°C, and 0.02% at 37°C, respectively. These results may be due to the changes in cellular structures and compositions of bacteria at different incubation temperatures, which further affect bacterial survival to environmental stresses (Cebrián et al., 2008). Similar findings were also reported by Taormina and Dorsa (2009b) that ELA was more effective to inactivate L. monocytogenes at 23°C (decreased more than 5.48 log) than at 4.4°C (decreased by 4.11 log) after only 5 min of exposure time. In addition, Yang et al. (2019) reported that ELA combined with mild heat (55°C) exhibited enhanced antibacterial activity against E. coli O157:H7 and L. innocua. Therefore, the combination of ELA and mild heat represents a promising strategy to eliminate microorganisms in foods. Finally, it should also point out that high temperatures may accelerate the hydrolysis of ELA. So ELA cannot be used at too high temperatures.
pH
ELA has been shown to maintain antimicrobial activity over a wide pH range from 3 to 7, which may be used as an antibacterial agent for a wide range of food products. However, low or high pH may result in more extensive hydrolysis of ELA, thereby resulting in a decrease in its antibacterial activity. According to previous studies, ELA is easily decomposed under basic conditions. The half-life of ELA is greater than 1 year at pH 4, 57 d at pH 7, and 34 h at pH 9 during 25°C storage (EFSA, 2007), suggesting its decomposition by base-catalyzed hydrolysis. Therefore, special attention should be paid to the pH of food products for the practical use of ELA.
Food matrices and components
ELA may interact with other components within foods and beverages, such as starch (Ma et al., 2013), proteins (Loeffler et al., 2020), polysaccharides (Loeffler et al., 2014), and lipids (Ziani et al., 2011; Magrinyà et al., 2015), causing a significant decrease in its antimicrobial activity. In general, many studies confirm that the concentration of ELA required to inactivate microorganisms in foods is higher than that needed for in vitro tests. Ma et al. (2013) reported that ELA effectively inhibited L. monocytogenes in TSB with a MIC of 11.8 μg/ml. However, potato starch at 2–5% (w/v) in TSB increased the MIC of ELA to 93.8–187.5 μg/ml. In addition, L. monocytogenes in 2% reduced-fat milk were only decreased by 1.02-log from the initial count of 7.31 log10 CFU/mL after incubation with 375 μg/ml of ELA at 32°C for 24 h (Ma et al., 2013). Similarly, the MIC values of ELA against L. innocua and P. fluorescens in nutrient broth (NB) were remarkably increased by 4–13 fold in the presence of BSA, whey protein isolate, or soy protein hydrolysate (Loeffler et al., 2020). Some additives used in foods and beverages also may affect the antimicrobial activity of ELA. In the work of Loeffler et al. (2014), the authors investigated the antimicrobial efficacy of ELA in the presence of xanthan and λ-carrageenan, two anionic polysaccharides used widely in beverages. The MIC values of ELA against S. cerevisiae, C. albicans, and Z. bailii were increased significantly with the increasing polysaccharide concentration (Loeffler et al., 2014). Chen et al. (2015) found that the fat concentration of peanut paste negatively impacted the antimicrobial efficacy of ELA. Magrinyà et al. (2015) also revealed that the antimicrobial efficacy of ELA was decreased with increasing fat addition (0 to 15 wt.%). These results might be due to that more ELA was present at the interface between water and fat, therefore, leading to the decreased antimicrobial effect. However, when the fat content increased from 15 to 50 wt.%, more ELA might again be present in the aqueous phase, causing a dramatic increase in the antimicrobial activity of ELA (Magrinyà et al., 2015).
Food matrices and components may protect microorganisms from the washing or disinfection treatment of ELA. In addition, ELA can form complexes with some charged food components (such as proteins and polysaccharides) by electrostatic interaction (Loeffler et al., 2014, 2020). Food ingredients with no charge, e.g., starch, may reduce the antimicrobial activity of ELA by increasing the viscosity, thereby limiting access of ELA to microorganisms cells (Ma et al., 2013). Furthermore, foods are characterized by a higher amount of available nutrients compared to in vitro assays, which may enable microorganisms to repair cellular damage and maintain homeostasis, leading to a decreased sensitivity if exposed to ELA.
Conclusions and future perspectives
ELA exerts strong antimicrobial activity against a wide range of food pathogens and spoilage microorganisms. The antimicrobial action of ELA may be mainly attributed to its amphiphilic structure, membrane damage, and oxidative stress. As a promising antimicrobial agent, ELA has been widely exploited to improve the safety and quality of foods. Nevertheless, more and further studies are still required. The antibacterial mechanisms of ELA should be further elucidated with multi-omics techniques and molecular dynamics simulation. The lower antimicrobial efficacy of ELA is observed when in foods and beverages. Thus, much more work is needed to understand the interactions between ELA and the various components of foods. Also, more attention should be paid to the combination of ELA with other existing antimicrobials or technologies in food processing to improve the antibacterial efficacy of ELA. ELA-based antimicrobial packaging has been recognized as a promising form of active food packaging and an emerging technology, while more special attentions should be devoted to its commercial application.
Author contributions
YfM and YqM: writing – review and editing. DZ and SW: writing original – draft. LC: review and editing. QX: conceptualization and supervision. All authors reviewed the manuscript, contributed to the article, and approved the submitted version.
Funding
The work is financially supported by the Natural Science Foundation of Henan Province (No. 212300410090) and the Collaborative Innovation Special Project of Zhengzhou (No. 2021ZDPY0201).
Conflict of interest
The authors declare that the research was conducted in the absence of any commercial or financial relationships that could be construed as a potential conflict of interest.
Publisher’s note
All claims expressed in this article are solely those of the authors and do not necessarily represent those of their affiliated organizations, or those of the publisher, the editors and the reviewers. Any product that may be evaluated in this article, or claim that may be made by its manufacturer, is not guaranteed or endorsed by the publisher.
References
Abdel-Naeem, H. H. S., Zayed, N. E. R., and Mansour, H. A. (2021). Effect of chitosan and lauric arginate edible coating on bacteriological quality, deterioration criteria, and sensory attributes of frozen stored chicken meat. LWT-Food Sci. Technol. 150:111928. doi: 10.1016/j.lwt.2021.111928
Asker, D., Weiss, J., and McClements, D. (2008). Analysis of the interactions of a cationic surfactant (lauric arginate) with an anionic biopolymer (pectin): isothermal titration calorimetry, light scattering, and microelectrophoresis. Langmuir 25, 116–122. doi: 10.1021/la803038w
Bai, L., Xiang, W. C., Huan, S. Q., and Rojas, O. J. (2018). Formulation and stabilization of concentrated edible oil-in-water emulsions based on electrostatic complexes of a food-grade cationic surfactant (ethyl lauroyl arginate) and cellulose nanocrystals. Biomacromolecules 19, 1674–1685. doi: 10.1021/acs.biomac.8b00233
Becerril, R., Manso, S., Nerin, C., and Gómez-Lus, R. (2013). Antimicrobial activity of lauroyl arginate ethyl (LAE), against selected food-borne. Food Control 32, 404–408. doi: 10.1016/j.foodcont.2013.01.003
Bechstein, D. V., Popp, J., Sudhaus-Joern, N., and Krischek, C. (2019). Effect of ethyl-lauroyl-arginate hypochloride in combination with high hydrostatic pressure processing on the microbial load and physico-chemical characteristics of minced and portioned chicken breast meat. Poult. Sci. 98, 966–976. doi: 10.3382/ps/pey427
Benli, H., Sanchez-Plata, M. X., and Keeton, J. T. (2011). Efficacy of epsilon-polylysine, lauric arginate, or acidic calcium sulfate applied sequentially for Salmonella reduction on membrane filters and chicken carcasses. J. Food Prot. 74, 743–750. doi: 10.4315/0362-028X.JFP-10-463
Bintsis, T. (2017). Foodborne pathogens. AIMS Microbiol 3, 529–563. doi: 10.3934/microbiol.2017.3.529
Cebrián, G., Sagarzazu, N., Pagán, R., Condón, S., and Mañas, P. (2008). Resistance of Escherichia coli grown at different temperatures to various environmental stresses. J. Appl. Microbiol. 105, 271–278. doi: 10.1111/j.1365-2672.2008.03745.x
Chang, Y. H., McLandsborough, L., and McClements, D. J. (2015). Fabrication, stability and efficacy of dual-component antimicrobial nanoemulsions: essential oil (thyme oil) and cationic surfactant (lauric arginate). Food Chem. 172, 298–304. doi: 10.1016/j.foodchem.2014.09.081
Chen, W., Golden, D. A., Critzer, F. J., and Davidson, P. M. (2015). Antimicrobial activity of cinnamaldehyde, carvacrol, and lauric arginate against Salmonella Tennessee in a glycerol-sucrose model and peanut paste at different fat concentrations. J. Food Prot. 78, 1488–1495. doi: 10.4315/0362-028X.JFP-14-599
Coronel-León, J., López, A., Espuny, M. J., Beltran, M. T., Molinos-Gómez, A., Rocabayera, X., et al. (2016). Assessment of antimicrobial activity of Nα-lauroyl arginate ethylester (LAE®) against Yersinia enterocolitica and Lactobacillus plantarum by flow cytometry and transmission electron microscopy. Food Control 63, 1–10. doi: 10.1016/j.foodcont.2015.10.050
Czakaj, A., Jarek, E., Krzan, M., and Warszyński, P. (2021). Ethyl lauroyl arginate, an inherently multicomponent surfactant system. Molecules 26:5894. doi: 10.3390/molecules26195894
Demircan, B., and Özdestan Ocak, Ö. (2021). The effects of ethyl lauroyl arginate and lemon essential oil added edible chitosan film coating on biogenic amines formation during storage in mackerel fillets. J. Food Process. Preserv. 45:e15454. doi: 10.1111/jfpp.15454
Deng, L. L., Taxipalati, M., Zhang, A. P., Que, F., Wei, H. W., Feng, F. Q., et al. (2018). Electrospun chitosan/poly(ethylene oxide)/lauric arginate nanofibrous film with enhanced antimicrobial activity. J. Agric. Food Chem. 66, 6219–6226. doi: 10.1021/acs.jafc.8b01493
Dias-Morse, P., Pohlman, F. W., Williams, J., and BrownJr, A. H. (2014). Single or multiple decontamination interventions involving lauric arginate on beef trimmings to enhance microbial safety of ground beef. Prof. Anim. Sci. 30, 477–484. doi: 10.15232/pas.2014-01334
EFSA (2007). Opinion of the scientific panel on food additives, flavourings, processing aids and materials in contact with food on a request from the commission related to an application on the use of ethyl lauroyl arginate as a food additive. EFSA J. 5:511. doi: 10.2903/j.efsa.2007.511
EFSA (2019). Scientific opinion on the safety of ethyl lauroyl arginate (E243) as a food additive in the light of the new information provided and the proposed extension of use. EFSA J. 17:5621. doi: 10.2903/j.efsa.2019.5621
Fernández, C. E., Aspiras, M., Dodds, M. W., González-Cabezas, C., and Rickard, A. H. (2018). Combinatorial effect of magnolia bark extract and ethyl lauroyl arginate against multi-species oral biofilms: food additives with the potential to prevent biofilm-related oral diseases. J. Funct. Foods 47, 48–55. doi: 10.1016/j.jff.2018.05.012
Frelka, J. C., and Harris, L. J. (2015). Evaluation of microbial loads and the effects of antimicrobial sprays in postharvest handling of California walnuts. Food Microbiol. 48, 133–142. doi: 10.1016/j.fm.2014.10.015
Fu, Y. Z., Deering, A. J., Bhunia, A. K., and Yao, Y. (2017a). Biofilm of Escherichia coli O157:H7 on cantaloupe surface is resistant to lauroyl arginate ethyl and sodium hypochlorite. Int. J. Food Microbiol. 260, 11–16. doi: 10.1016/j.ijfoodmicro.2017.08.008
Fu, Y. Z., Deering, A. J., Bhunia, A. K., and Yao, Y. (2017b). Pathogen biofilm formation on cantaloupe surface and its impact on the antibacterial effect of lauroyl arginate ethyl. Food Microbiol. 64, 139–144. doi: 10.1016/j.fm.2016.12.020
Galié, S., Garcia-Gutiérrez, C., Miguélez, E. M., Villar, C. J., and Lombó, F. (2018). Biofilms in the food industry: health aspects and control methods. Front. Microbiol. 9:898. doi: 10.3389/fmicb.2018.00898
Gamarra-Montes, A., Missagia, B., Morató, J., and Muñoz-Guerra, S. (2018). Antibacterial films made of ionic complexes of poly(γ-glutamic acid) and ethyl lauroyl arginate. Polymers 10:21. doi: 10.3390/polym10010021
Guo, M. M., Jin, T. Z., Wang, L. X., Scullen, O. J., and Sommers, C. H. (2014). Antimicrobial films and coatings for inactivation of Listeria innocua on ready-to-eat deli Turkey meat. Food Control 40, 64–70. doi: 10.1016/j.foodcont.2013.11.018
Hassan, A. H. A., and Cutter, C. N. (2020). Development and evaluation of pullulan-based composite antimicrobial films (CAF) incorporated with nisin, thymol and lauric arginate to reduce foodborne pathogens associated with muscle foods. Int. J. Food Microbiol. 320:108519. doi: 10.1016/j.ijfoodmicro.2020.108519
Hawkins, D. R., Rocabayera, X., Ruckman, S., Segret, R., and Shaw, D. (2009). Metabolism and pharmacokinetics of ethyl Nα-lauroyl-L-arginate hydrochloride in human volunteers. Food Chem. Toxicol. 47, 2711–2715. doi: 10.1016/j.fct.2009.07.028
Hawkins, J. L., Vimini, B., Schwarz, J. G., Nichols, P., and Parveen, S. (2016). Application of antimicrobial agents via commercial spray cabinet to inactivate Salmonella on skinless chicken meat. J. Food Prot. 79, 569–573. doi: 10.4315/0362-028X.JFP-15-248
Heredia, N., and García, S. (2018). Animals as sources of food-borne pathogens: a review. Anim Nutr. 4, 250–255. doi: 10.1016/j.aninu.2018.04.006
Higueras, L., López-Carballo, G., Hernández-Muñoz, P., Gavara, R., and Rollini, M. (2013). Development of a novel antimicrobial film based on chitosan with LAE (ethyl-Nα-dodecanoyl-l-arginate) and its application to fresh chicken. Int. J. Food Microbiol. 165, 339–345. doi: 10.1016/j.ijfoodmicro.2013.06.003
Huang, K., and Nitin, N. (2017). Enhanced removal of Escherichia coli O157:H7 and Listeria innocua from fresh lettuce leaves using surfactants during simulated washing. Food Control 79, 207–217. doi: 10.1016/j.foodcont.2017.03.032
Infante, M. R., Erra, P., Juliá, R., and Prats, M. (1984). Surface active molecules: preparation and properties of long chain Nα-acyl-l-α-amino-ω-guanidine alkyl acid derivatives. Int. J. Cosmet. Sci. 6, 275–282. doi: 10.1111/j.1467-2494.1984.tb00385.x
Ishangulyyev, R., Kim, S., and Lee, S. H. (2019). Understanding food loss and waste—why are we losing and wasting food? Foods. 8:297. doi: 10.3390/foods8080297
Iwamoto, M., Ayers, T., Mahon, B. E., and Swerdlow, D. L. (2010). Epidemiology of seafood-associated infections in the United States. Clin. Microbiol. Rev. 23, 399–411. doi: 10.1128/CMR.00059-09
Javanmardi, F., Rahmani, J., Ghiasi, F., Hashemi Gahruie, H., and Mousavi, K. A. (2019). The association between the preservative agents in foods and the risk of breast cancer. Nutr. Cancer 71, 1229–1240. doi: 10.1080/01635581.2019.1608266
Juneja, V. K., Osoria, M., Tiwari, U., Xu, X. R., Golden, C. E., Mukhopadhyay, S., et al. (2020). The effect of lauric arginate on the thermal inactivation of starved Listeria monocytogenes in sous-vide cooked ground beef. Food Res. Int. 134:109280. doi: 10.1016/j.foodres.2020.109280
Karwowska, M., and Kononiuk, A. (2020). Nitrates/nitrites in food—risk for nitrosative stress and benefits. Antioxidants. 9:241. doi: 10.3390/antiox9030241
Kashiri, M., López-Carballo, G., Hernández-Muñoz, P., and Gavara, R. (2019). Antimicrobial packaging based on a LAE containing zein coating to control foodborne pathogens in chicken soup. Int. J. Food Microbiol. 306:108272. doi: 10.1016/j.ijfoodmicro.2019.108272
Kim, T. S., Ham, S. Y., Park, B. B., Byun, Y., and Park, H. D. (2017). Lauroyl arginate ethyl blocks the iron signals necessary for Pseudomonas aeruginosa biofilm development. Front. Microbiol. 8:970. doi: 10.3389/fmicb.2017.00970
Kozak, S. M., Brown, S. R. B., Bobak, Y., and D’Amico, D. J. (2018). Control of Listeria monocytogenes in whole milk using antimicrobials applied individually and in combination. J. Dairy Sci. 101, 1889–1900. doi: 10.3168/jds.2017-13648
Kozak, S. M., Margison, K. M., and D’Amico, D. J. (2017). Synergistic antimicrobial combinations inhibit and inactivate Listeria monocytogenes in neutral and acidic broth systems. J. Food Prot. 80, 1266–1273. doi: 10.4315/0362-028X.JFP-17-035
Lavieri, N. A., Sebranek, J. G., Brehm-Stecher, B. F., Cordray, J. C., Dickson, J. S., Horsch, A. M., et al. (2014). Investigating the control of Listeria monocytogenes on a ready-to-eat ham product using natural antimicrobial ingredients and postlethality interventions. Foodborne Pathog. Dis. 11, 462–467. doi: 10.1089/fpd.2013.1702
Lewis, R., and Hill, C. (2020). Overcoming barriers to phage application in food and feed. Curr. Opin. Biotechnol. 61, 38–44. doi: 10.1016/j.copbio.2019.09.018
Li, Y., Deng, L. L., Xu, X. H., and Feng, F. Q. (2020). Effect of compound Nα-lauroyl-L-arginate ethylester preservative on the preservation of green bell pepper. Food Sci. 41, 201–206. doi: 10.7506/spkx1002-6630-20190617-178
Li, T., Liu, Y. X., Qin, Q. X., Zhao, L., Wang, Y. T., Wu, X. M., et al. (2021). Development of electrospun films enriched with ethyl lauroyl arginate as novel antimicrobial food packaging materials for fresh strawberry preservation. Food Control 130:108371. doi: 10.1016/j.foodcont.2021.108371
Li, Y., Xu, X. H., Li, Y., and Feng, F. Q. (2018). Antibacterial activity of Nα-lauroyl-L-arginate ethylester against five pathogenic microorganisms of fruits and vegetables preservative on the preservation of green bell pepper. Food Mach. 34, 127–131+193. doi: 10.13652/j.issn.1003-5788.2018.07.027
Lingbeck, J. M., Cordero, P., O'Bryan, C. A., Johnson, M. G., Ricke, S. C., and Crandall, P. G. (2014). Temperature effects on the antimicrobial efficacy of condensed smoke and lauric arginate against Listeria and Salmonella. J. Food Prot. 77, 934–940. doi: 10.4315/0362-028X.JFP-13-459
Loeffler, M., McClements, D., McLandsborough, L., Terjung, N., Chang, Y., and Weiss, J. (2014). Electrostatic interactions of cationic lauric arginate with anionic polysaccharides affect antimicrobial activity against spoilage yeasts. J. Appl. Microbiol. 117, 28–39. doi: 10.1111/jam.12502
Loeffler, M., Schwab, V., Terjung, N., Weiss, J., and McClements, D. J. (2020). Influence of protein type on the antimicrobial activity of LAE alone or in combination with methylparaben. Foods. 9:270. doi: 10.3390/foods9030270
Luchansky, J., Call, J., Hristova, B., Rumery, L., Yoder, L., and Oser, A. (2005). Viability of Listeria monocytogenes on commercially-prepared hams surface treated with acidic calcium sulfate and lauric arginate and stored at 4 °C. Meat Sci. 71, 92–99. doi: 10.1016/j.meatsci.2005.04.006
Ma, Q. M., Davidson, P. M., Critzer, F., and Zhong, Q. X. (2016a). Antimicrobial activities of lauric arginate and cinnamon oil combination against foodborne pathogens: improvement by ethylenediaminetetraacetate and possible mechanisms. LWT-Food Sci. Technol. 72, 9–18. doi: 10.1016/j.lwt.2016.04.021
Ma, Q. M., Davidson, P. M., and Zhong, Q. X. (2013). Antimicrobial properties of lauric arginate alone or in combination with essential oils in tryptic soy broth and 2% reduced fat milk. Int. J. Food Microbiol. 166, 77–84. doi: 10.1016/j.ijfoodmicro.2013.06.017
Ma, Q. M., Davidson, P. M., and Zhong, Q. X. (2016b). Nanoemulsions of thymol and eugenol co-emulsified by lauric arginate and lecithin. Food Chem. 206, 167–173. doi: 10.1016/j.foodchem.2016.03.065
Ma, Q. M., Davidson, P. M., and Zhong, Q. X. (2020). Properties and potential food applications of lauric arginate as a cationic antimicrobial. Int. J. Food Microbiol. 315:108417. doi: 10.1016/j.ijfoodmicro.2019.108417
Ma, Q. M., Zhang, Y., Critzer, F., Davidson, P. M., and Zhong, Q. X. (2016c). Quality attributes and microbial survival on whole cantaloupes with antimicrobial coatings containing chitosan, lauric arginate, cinnamon oil and ethylenediaminetetraacetic acid. Int. J. Food Microbiol. 235, 103–108. doi: 10.1016/j.ijfoodmicro.2016.07.030
Magrinyà, N., Terjung, N., Loeffler, M., Gibis, M., Bou, R., and Weiss, J. (2015). Influence of fat addition on the antimicrobial activity of sodium lactate, lauric arginate and methylparaben in minced meat. Int. J. Food Microbiol. 215, 86–94. doi: 10.1016/j.ijfoodmicro.2015.08.017
Manrique, Y., Gibis, M., Schmidt, H., and Weiss, J. (2017). Influence of application sequence and timing of eugenol and lauric arginate (LAE) on survival of spoilage organisms. Food Microbiol. 64, 210–218. doi: 10.1016/j.fm.2017.01.002
Manso, S., Wrona, M., Salafranca, J., Nerín, C., Alfonso, M. J., and Caballero, M. Á. (2021). Evaluation of new antimicrobial materials incorporating ethyl lauroyl arginate or silver into different matrices, and their safety in use as potential packaging. Polymers 13:355. doi: 10.3390/polym13030355
Martin, E., Griffis, C., Vaughn, K., O'Bryan, C., Friedly, E., Marcy, J., et al. (2009). Control of Listeria monocytogenes by lauric arginate on frankfurters formulated with or without lactate/diacetate. J. Food Sci. 74, M237–M241. doi: 10.1111/j.1750-3841.2009.01196.x
Martínez-Ramos, A. R., Ibarra-Sánchez, L. A., Amaya-Llano, S. L., and Miller, M. J. (2020). Evaluation of combinations of nisin, lauric arginate, and ε-polylysine to control Listeria monocytogenes in queso fresco. J. Dairy Sci. 103, 11152–11162. doi: 10.1016/j.ijfoodmicro.2012.01.010
Miller, S. I. (2016). Antibiotic resistance and regulation of the gram-negative bacterial outer membrane barrier by host innate immune molecules. MBio 7, e01541–e01516. doi: 10.1128/mBio.01541-16
Moore, A., Nannapaneni, R., Kiess, A., and Sharma, C. S. (2017). Evaluation of USDA approved antimicrobials on the reduction of Salmonella and Campylobacter in ground chicken frames and their effect on meat quality. Poult. Sci. 96, 2385–2392. doi: 10.3382/ps/pew497
Moreno, O., Atarés, L., and Chiralt, A. (2017). Active starch-gelatin films for shelf-life extension of marinated salmon. LWT-Food Sci. Technol. 84, 189–195. doi: 10.1016/j.lwt.2017.05.005
Moreno, O., Atarés, L., Chiralt, A., Cruz-Romero, M. C., and Kerry, J. (2018). Starch-gelatin antimicrobial packaging materials to extend the shelf life of chicken breast fillets. LWT-Food Sci. Technol. 97, 483–490. doi: 10.1016/j.lwt.2018.07.005
Motta, J.F.G., Ribeiro-santos, R., Guimarães, M.C., and Moura, L. De A. G., Vitorazi, L. and Melo, N. R.De. (2020). Nα-lauroyl-l-arginine ethyl ester monohydrochloride, an antimicrobial agent and its use: a review. Res., Soc. Dev. 9:e6059108996. doi: 10.33448/rsd-v9i10.8996
Muriel-Galet, V., López-Carballo, G., Gavara, R., and Hernández-Muñoz, P. (2015). Antimicrobial effectiveness of lauroyl arginate incorporated into ethylene vinyl alcohol copolymers to extend the shelf-life of chicken stock and surimi sticks. Food Bioprocess Technol. 8, 208–217. doi: 10.1007/s11947-014-1391-x
Nair, D. V., Nannapaneni, R., Kiess, A., Mahmoud, B., and Sharma, C. S. (2014). Antimicrobial efficacy of lauric arginate against Campylobacter jejuni and spoilage organisms on chicken breast fillets. Poult. Sci. 93, 2636–2640. doi: 10.3382/ps.2013-03858
Nübling, S., Hagele, F., Wohlt, D., Graf, B., Schweiggert, R. M., Carle, R., et al. (2017a). Effects of Quillaja saponaria extract and Nα-lauroyl-L-arginine ethyl ester on reducing selected foodborne pathogens in vitro and maintaining quality of fresh-cut endive (Cichorium endivia L.) at pilot plant scale. Food Control 73, 393–400. doi: 10.1016/j.foodcont.2016.08.029
Nübling, S., Wohlt, D., Saile, N., Weiss, A., and Schmidt, H. (2017b). Antimicrobial effect of lauroyl arginate ethyl on Escherichia coli O157:H7 and Listeria monocytogenes on red oak leaf lettuce. Eur. Food Res. Technol. 243, 879–887. doi: 10.1007/s00217-016-2802-1
Otero, V., Becerril, R., Santos, J. A., Rodríguez-Calleja, J. M., Nerín, C., and García-López, M. L. (2014). Evaluation of two antimicrobial packaging films against Escherichia coli O157:H7 strains in vitro and during storage of a Spanish ripened sheep cheese (Zamorano). Food Control 42, 296–302. doi: 10.1016/j.foodcont.2014.02.022
Pattanayaiying, R., H-Kittikun, A., and Cutter, C. N. (2014). Effect of lauric arginate, nisin Z, and a combination against several food-related bacteria. Int. J. Food Microbiol. 188, 135–146. doi: 10.1016/j.ijfoodmicro.2014.07.013
Pattanayaiying, R., H-Kittikun, A., and Cutter, C. N. (2015). Incorporation of nisin Z and lauric arginate into pullulan films to inhibit foodborne pathogens associated with fresh and ready-to-eat muscle foods. Int. J. Food Microbiol. 207, 77–82. doi: 10.1016/j.ijfoodmicro.2015.04.045
Peng, M. L., Hao, G., Tang, S. H., and Li, S. (2021). Preservative lauroyl arginine ethyl ester on the quality of yak sausage during storage. Food Ferment. Indus. 47, 176–182. doi: 10.13995/j.cnki.11-1802/ts.026130
Porto-Fett, A., Campano, S., Smith, J., Oser, A., Shoyer, B., Call, J., et al. (2010). Control of Listeria monocytogenes on commercially-produced frankfurters prepared with and without potassium lactate and sodium diacetate and surface treated with lauric arginate using the sprayed lethality in container (SLIC®) delivery method. Meat Sci. 85, 312–318. doi: 10.1016/j.meatsci.2010.01.020
Rodríguez, E., Seguer, J., Rocabayera, X., and Manresa, A. (2004). Cellular effects of monohydrochloride of L-arginine, N-lauroyl ethylester (LAE) on exposure to Salmonella typhimurium and Staphylococcus aureus. J. Appl. Microbiol. 96, 903–912. doi: 10.1111/j.1365-2672.2004.02207.x
Ruckman, S. A., Rocabayera, X., Borzelleca, J. F., and Sandusky, C. B. (2004). Toxicological and metabolic investigations of the safety of Nα-Lauroyl-L-arginine ethyl ester monohydrochloride (LAE). Food Chem. Toxicol. 42, 245–259. doi: 10.1016/j.fct.2003.08.022
Ruengvisesh, S., Loquercio, A., Castell-Perez, E., and Taylor, T. M. (2015). Inhibition of bacterial pathogens in medium and on spinach leaf surfaces using plant-derived antimicrobials loaded in surfactant micelles. J. Food Sci. 80, M2522–M2529. doi: 10.1111/1750-3841.13085
Sadekuzzaman, M., Yang, S., Kim, H. S., Mizan, M. F. R., and Ha, S. D. (2017). Evaluation of a novel antimicrobial (lauric arginate ester) substance against biofilm of Escherichia coli O157:H7, Listeria monocytogenes, and Salmonella spp. Int. J. Food Sci. Technol. 52, 2058–2067. doi: 10.1111/ijfs.13484
Saini, J. K., Barrios, M. A., Marsden, J. L., Getty, K. J., and Fung, D. Y. (2013). Efficacy of antimicrobial lauric arginate against Listeria monocytogenes on stainless steel coupons. Adv. Appl. Microbiol. 3, 65–68. doi: 10.4236/aim.2013.31010
Seemeen, S. (2011). Effects of high pressure processing and ethyl lauroyl arginate on the shelf-life of ready-to-eat sliced chicken breast roast. Master diss., Massey University.
Sharma, C., Ates, A., Joseph, P., Nannapaneni, R., and Kiess, A. (2013). Reduction of Salmonella in skinless chicken breast fillets by lauric arginate surface application. Poult. Sci. 92, 1419–1424. doi: 10.1111/j.1750-3841.2009.01196.x
Shen, X. Y., Cong, J., Mugendi, J., Hanrahan, I., and Zhu, M. J. (2021). Synergistic effects of lauric arginate and peracetic acid in reducing Listeria monocytogenes on fresh apples. Front. Microbiol. 12:641034. doi: 10.3389/fmicb.2021.641034
Soni, K. A., Desai, M., Oladunjoye, A., Skrobot, F., and Nannapaneni, R. (2012). Reduction of Listeria monocytogenes in queso fresco cheese by a combination of listericidal and listeriostatic GRAS antimicrobials. Int. J. Food Microbiol. 155, 82–88. doi: 10.1016/j.ijfoodmicro.2012.01.010
Soni, K. A., Nannapaneni, R., Schilling, M. W., and Jackson, V. (2010). Bactericidal activity of lauric arginate in milk and Queso Fresco cheese against Listeria monocytogenes cold growth. J. Dairy Sci. 93, 4518–4525. doi: 10.3168/jds.2010-3270
Soni, K. A., Shen, Q., and Nannapaneni, R. (2014). Reduction of Listeria monocytogenes in cold-smoked salmon by bacteriophage P100, nisin and lauric arginate, singly or in combinations. Int. J. Food Sci. Technol. 49, 1918–1924. doi: 10.1111/ijfs.12581
Stopforth, J., Visser, D., Zumbrink, R., Van Dijk, L., and Bontenbal, E. (2010). Control of Listeria monocytogenes on cooked cured ham by formulation with a lactate-diacetate blend and surface treatment with lauric arginate. J. Food Prot. 73, 552–555. doi: 10.4315/0362-028X-73.3.552
Suksathit, S., and Tangwatcharin, P. (2013). Activity of organic acid salts in combination with lauric arginate against Listeria monocytogenes and Salmonella Rissen. ScienceAsia 39, 346–355. doi: 10.2306/scienceasia1513-1874.2013.39.346
Sukumaran, A. T., Nannapaneni, R., Kiess, A., and Sharma, C. S. (2015). Reduction of Salmonella on chicken meat and chicken skin by combined or sequential application of lytic bacteriophage with chemical antimicrobials. Int. J. Food Microbiol. 207, 8–15. doi: 10.1016/j.ijfoodmicro.2015.04.025
Taormina, P. J., and Dorsa, W. J. (2009a). Inactivation of Listeria monocytogenes on hams shortly after vacuum packaging by spray application of lauric arginate. J. Food Prot. 72, 2517–2523. doi: 10.4315/0362-028X-72.12.2517
Taormina, P. J., and Dorsa, W. J. (2009b). Short-term bactericidal efficacy of lauric arginate against Listeria monocytogenes present on the surface of frankfurters. J. Food Prot. 72, 1216–1224. doi: 10.4315/0362-028X-72.6.1216
Theinsathid, P., Visessanguan, W., Kruenate, J., Kingcha, Y., and Keeratipibul, S. (2012). Antimicrobial activity of lauric arginate-coated polylactic acid films against Listeria monocytogenes and Salmonella Typhimurium on cooked sliced ham. J. Food Sci. 77, M142–M149. doi: 10.1111/j.1750-3841.2011.02526.x
Tirloni, E., Bernardi, C., and Stella, S. (2021). Ethyl lauroyl arginate (LAE): antimicrobial activity of LAE-coated film for the packaging of raw beef and pork. J. Food Qual. 2021, 1–7. doi: 10.1155/2021/6643717
United States Department of Agriculture Economic Research Service. (2022) Cost estimates of foodborne illnesses. Available online: https://www.ers.usda.gov/data-products/cost-estimates-of-foodborne-illnesses/ Accessed 20 March 2022
United States Department of Agriculture, Food Safety and Inspection Service. (2022). Safe and suitable ingredients used in the production of meat, poultry and egg products. FSIS directive 7120.1, Revision 56. Available at: https://www.fsis.usda.gov/policy/fsis-directives/7120.1
Velasco-Bolom, J. L., Corzo, G., and Garduño-Juárez, R. (2018). Molecular dynamics simulation of the membrane binding and disruption mechanisms by antimicrobial scorpion venom-derived peptides. J. Biomol. Struct. Dyn. 36, 2070–2084. doi: 10.1080/07391102.2017.1341340
Woodcock, N. H., Hammond, B. H., Ralyea, R. D., and Boor, K. J. (2009). Nα-Lauroyl-l-arginine ethylester monohydrochloride reduces bacterial growth in pasteurized milk. J. Dairy Sci. 92, 4207–4210. doi: 10.3168/jds.2009-2150
Wu, D., Forghani, F., Daliri, E. B. M., Li, J., Liao, X. Y., Liu, D. H., et al. (2020). Microbial response to some nonthermal physical technologies. Trends Food Sci. Technol. 95, 107–117. doi: 10.1016/j.tifs.2019.11.012
Xu, X. H., Jiang, Z. L., Feng, F. Q., and Lu, R. R. (2018). Mechanisms of Nα-lauroyl arginate ethyl ester against Penicillium digitatum and Pectobacterium carotovorum subsp. carotovorum. J. Food Sci. Technol. 55, 3675–3682. doi: 10.1007/s13197-018-3296-6
Yang, X., Rai, R. W., Huu, C. N., and Nitin, N. (2019). Synergistic antimicrobial activity by light or thermal treatment and lauric arginate: membrane damage and oxidative stress. Appl. Environ. Microbiol. 85, e01033–e01019. doi: 10.1128/AEM.01033-19
Yang, S., Sadekuzzaman, M., and Ha, S. D. (2017). Treatment with lauric arginate ethyl ester and commercial bacteriophage, alone or in combination, inhibits Listeria monocytogenes in chicken breast tissue. Food Control 78, 57–63. doi: 10.1016/j.foodcont.2017.02.021
Zhao, D. B., Wang, S. D., Hu, Y. S., Liu, X., Tao, J., Sagratini, G., et al. (2022a). Insight into the antibacterial activity of lauric arginate against Escherichia coli O157:H7: membrane disruption and oxidative stress. LWT-Food Sci. Technol. 162:113449. doi: 10.1016/j.lwt.2022.113449
Zhao, D. B., Wang, S. D., Wang, J. Z., Wu, D., Liu, X., Niu, L. Y., et al. (2022b). Effects of lauroyl arginate ethyl (LAE) on pathogen inactivation and quality attributes of spinach leaves. J. Food Meas. Charact. 17, 706–715. doi: 10.1007/s11694-022-01661-2
Zheng, Z. X. (2014). Ingredient technology for food preservation. Ind. Biotechnol. 10, 28–33. doi: 10.1089/ind.2013.0023
Zhuang, S., Li, Y., Hong, H., Liu, Y. Y., Shu, R., and Luo, Y. K. (2020). Effects of ethyl lauroyl arginate hydrochloride on microbiota, quality and biochemical changes of container-cultured largemouth bass (Micropterus salmonides) fillets during storage at 4 °C. Food Chem. 324:126886. doi: 10.1016/j.foodchem.2020.126886
Keywords: ethyl lauroyl arginate, antimicrobial efficacy, decontamination, food, mechanism
Citation: Ma Y, Ma Y, Chi L, Wang S, Zhang D and Xiang Q (2023) Ethyl lauroyl arginate: An update on the antimicrobial potential and application in the food systems: a review. Front. Microbiol. 14:1125808. doi: 10.3389/fmicb.2023.1125808
Edited by:
Huhu Wang, Nanjing Agricultural University, ChinaReviewed by:
Magdalena Olszewska, University of Warmia and Mazury in Olsztyn, PolandHongkun Xue, Hebei University, China
Copyright © 2023 Ma, Ma, Chi, Wang, Zhang and Xiang. This is an open-access article distributed under the terms of the Creative Commons Attribution License (CC BY). The use, distribution or reproduction in other forums is permitted, provided the original author(s) and the copyright owner(s) are credited and that the original publication in this journal is cited, in accordance with accepted academic practice. No use, distribution or reproduction is permitted which does not comply with these terms.
*Correspondence: Qisen Xiang, eGlhbmdxaXNlbjIwMDZAMTYzLmNvbQ==; Yunfang Ma, MjAxMzgwNEB6enVsaS5lZHUuY24=
†These authors have contributed equally to this work