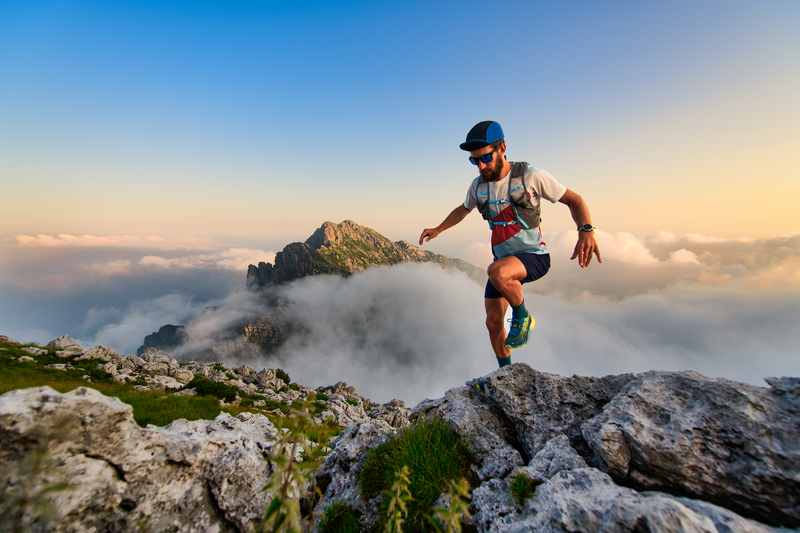
95% of researchers rate our articles as excellent or good
Learn more about the work of our research integrity team to safeguard the quality of each article we publish.
Find out more
REVIEW article
Front. Microbiol. , 15 February 2023
Sec. Microbe and Virus Interactions with Plants
Volume 14 - 2023 | https://doi.org/10.3389/fmicb.2023.1122947
This article is part of the Research Topic Molecular Mechanisms of Evasion of Plant Immunity by Microbes View all 6 articles
Plants, as sessile organisms, are constantly exposed to pathogens in nature. Plants rely on physical barriers, constitutive chemical defenses, and sophisticated inducible immunity to fight against pathogens. The output of these defense strategies is highly associated with host development and morphology. Successful pathogens utilize various virulence strategies to colonize, retrieve nutrients, and cause disease. In addition to the overall defense-growth balance, the host-pathogen interactions often lead to changes in the development of specific tissues/organs. In this review, we focus on recent advances in understanding the molecular mechanisms of pathogen-induced changes in plants’ development. We discuss that changes in host development could be a target of pathogen virulence strategies or an active defense strategy of plants. Current and ongoing research about how pathogens shape plant development to increase their virulence and causes diseases could give us novel views on plant disease control.
In nature, plants and their pathogens are in a continuous coevolutionary arms race (Burdon and Thrall, 2009). Plants, as sessile organisms, are attacked by various pathogens including bacteria, fungi, oomycetes, and nematodes. They evolved efficient constitutive and inducible innate immune responses to detect pathogens and defend themselves from disease (Bigeard et al., 2015). In general, plants are resistant to most pathogens because of preformed physical barriers (e.g., cuticle and cell wall; Hamann, 2012), and constitutive chemical defenses (e.g., antimicrobial compounds; Osbourn, 1996). These physical barriers are often dependent on the developmental stages of a plant host and/or present on specific organs (Smith, 2001; Ingram and Nawrath, 2017; Wolf, 2022). For example, secondary cell walls are strengthened in fully mature tissues (Zhong and Ye, 2015; Meents et al., 2018); cuticle layers are thicker in adult plants (Yeats and Rose, 2013; Budke and Goffinet, 2016; Ingram and Nawrath, 2017).
Two intertwined inducible immune responses protect plants from invading pathogens. On the cell surface, a group of membrane anchored or associated pattern recognition receptors (PRRs) recognize conserved microbial elicitors, including bacterial flagellin (Hayashi et al., 2001), elongation factor Tu (EF-Tu; Zipfel et al., 2006), and fungal cell wall components like chitin and polysaccharides (Kaku et al., 2006; Yin et al., 2016), which triggers a collection of immune responses called pathogen-associated molecular patterns (PAMP)-triggered immunity (PTI; Bigeard et al., 2015; Tang et al., 2017; Saijo et al., 2018). Successful pathogens can secrete effector proteins, hormone mimics, toxins, and other small molecules to compromise the host plants immune system, or alter other cellular processes (Ngou et al., 2022). Many effectors can increase pathogen virulence by countering PTI, which causes effector triggered susceptibility (ETS; Guttman et al., 2006; Jones and Dangl, 2006; Kwi-Mi et al., 2008). The functions of pathogen effectors have been widely discussed and reviewed (Deslandes and Rivas, 2012; Dou and Zhou, 2012; Howden and Huitema, 2012; Ngou et al., 2022). Within a cell, nucleotide binding-leucine-rich repeat (NLR) proteins act as intracellular receptors to detect effectors and activate effector-triggered immunity (ETI; Jones et al., 2016). PTI components are required for the full activation of ETI, and ETI also enhances PTI responses (Naveed et al., 2020; Tena, 2021; Yuan et al., 2021; Chang et al., 2022). Both PTI and ETI can induce a series of immune responses, such as stomata closure (Zhang et al., 2008; Sawinski et al., 2013; Yuan et al., 2021), reactive oxygen species burst (O’Brien et al., 2012), the hypersensitive response (Mur et al., 2008), the production of antimicrobial compounds and defense-related proteins (Bednarek, 2012), and mitogen-activated protein kinases (MAPKs) cascades activation with ETI often manifests in the faster and stronger forms (Meng and Zhang, 2013).
Given the essential function of PTI and ETI in defense, components of these immune signaling pathways are under tight spatial-temporal regulation. Plants balance development and defense to ensure resource allocation, quick adaptation to changing environment, and eventually successful reproduction (Huot et al., 2014). Mis-regulation of their expression level or function may lead to hyper-activation of immune response and eventually stunted growth or cell death (Li et al., 2020). Arabidopsis mutants with constitutively activated defense suffer from autoimmune symptoms including dwarfism and lesion formation (Rodriguez et al., 2016; van Wersch et al., 2016). High disease resistance in some crop varieties can also be associated with reduction in yield, a phenomenon known as yield penalty (Brown, 2002). Spatiotemporal regulation of key immune components is essential to prevent misfiring. For example, the response to bacterial derived elicitor flg22, a 22 amino acid peptide derived from flagellin, is confined to the root cap and transition/elongation zone in Arabidopsis (Emonet et al., 2021). Such immune response is further gated by a co-incident wound signaling to ensure the proper development of root in a microbial-rich environment (Zhou et al., 2020). Defense responses can also be differentially activated at distinct stages of host development. In Arabidopsis and tobacco, the old plants accumulate more Salicylic Acid (SA) and exhibit stronger SA responses than young plants (Carella et al., 2014; Wilson et al., 2017). Similar age-dependent resistance is associated with early seedling development, maturation of individual organs, or flowering (Kus et al., 2002; Rusterucci et al., 2005; Wilson et al., 2017). Taken together, plant development and immune response are highly coordinated by intrinsic molecular networks.
On the other side, pathogens can utilize multiple strategies to colonize plants, obtain nutrients, enhance susceptibility, and cause disease (Stavrinides et al., 2008). Pathogen infection often affects the growth and developmental processes of the host (Kwi-Mi et al., 2008). An interesting example is the formation of various gall structures when a plant is colonized by insects or fungi (Harris and Pitzschke, 2020). Most plant-formed galls are pathogens’ feeding sites to accommodate infection including some of the most studied pathogens Rhodococcus fascians, Pseudomonas savastanoi, rust fungi, Agrobacterium tumefaciens, root cyst nematodes, and gall midges (Harris and Pitzschke, 2020). Pathogen-induced changes in plant development can be an outcome of the pathogen’s virulence strategies or the active plant defense strategy upon pathogens infection. Here, we review recent advances in understanding the molecular signaling of pathogen-triggered changes in the development of specific tissues/organs and discuss the potential impact of these developmental changes in host-pathogen interactions. Excellent reviews on the overall balance between defense and growth/biomass can be found here (Huot et al., 2014; Figueroa-Macías et al., 2021; He et al., 2022). Here, we listed various aspects of pathogen effects on plant growth and development as shown in Table 1.
Table 1. Examples of pathogen-induced changes in plant growth and development with known molecular mechanisms.
Phytohormones are multifaceted regulators of plant development and defense. Pathogens can regulate plants’ growth and development as a virulence strategy by mimicking phytohormones or altering plants hormone signaling. Plant-parasitic nematodes colonize and reprogram root cells (Siddique et al., 2015). Cyst and root-knot nematodes penetrate plant roots and migrate to a competent cell in the vascular cylinder to induce a feeding cell complex. Cyst nematodes induce the syncytium by dissolving cell-wall of neighboring cells and fusing their protoplasts (Zhang et al., 2017). Root-knot nematodes recruit the progenitor cell, induce cell enlarge and repeated mitosis without cytokinesis, and eventually form a giant cell (Jagdale et al., 2021). Thus, reprogramming host cell differentiation is an essential virulence strategy for nematode survival. Auxin has been detected in secretions from root-knot nematode, Meloidogyne incognita and cyst nematode, Heterodera schachtii (Oosterbeek et al., 2021). It is suggested that nematode-secreted auxin at the feeding sites trigger local auxin accumulation and induce cell fate reprogramming (Niebel et al., 1994; Siddique et al., 2015). In addition, nematodes use effectors to manipulate auxin signaling. An effector protein 19C07 secreted by H. schachtii can target the Arabidopsis LAX3 auxin import protein to increase the auxin influx onto feeding sites (Lee et al., 2011); LAX3 can trigger the cell wall hydrolysis to stimulate syncytium development and allows the lateral root to emerge (Swarup et al., 2008; Lee et al., 2011). Another effector 10A07 interacts with a plant kinase [interacting plant kinase: (IPK) and the auxin regulator protein INDOLEACETIC ACID-INDUCED16 (IAA16)] in Arabidopsis, preventing it from repressing auxin response genes. Transgenic plant expressed 10A07 displayed stunted shoot sand roots phenotype and more susceptible to nematode infection (Hewezi et al., 2015).
Nematodes also secrete plant peptide hormone (PPH) mimics to shape plant development and facilitate pathogen parasitism (Chen et al., 2015; Ronald and Joe, 2018). Multiple classes of PPH effector mimics have been documented from nematodes including clavata3/embryo surrounding region (CLE)-like, C-terminally encoded peptide (CEP)-like, and inflorescence deficient in abscission (IDA)-like peptides (Ronald and Joe, 2018). CLE-like peptide mimics share sequence homology with plants CLEs, which regulate shoot, root, and vascular meristem maintenance (Lu et al., 2009; Yamaguchi et al., 2016; Ronald and Joe, 2018). Plant A-type CLEs can bind to CLAVATA1 (CLV1)/CLV2 heterodimer and suppress the apical meristem activity and promote cell differentiation (Ito et al., 2006), while B-type plant CLEs can bind to a tracheary element differentiation inhibitory factor (TDIF)-receptor (TDR) and suppress tracheary elements differentiation and promote cell division (Whitford et al., 2008). Nematodes encode both A-type and B-type CLE-like peptide mimics (Replogle et al., 2011; Guo et al., 2017). These CLE-like peptide effectors are secreted and packed into secretory granules and then delivered to the cytoplasm of host root cells through their stylet (Katsir et al., 2011; Mitchum et al., 2012). The peptide mimics then undergoes post-translational modification and interacts with plant CLEs receptor complex, including CLV1, CLV2, and BARELY ANY MERISTEMs and TDR to induce massive cell proliferation and feeding cells formation (Chen et al., 2015; Yamaguchi et al., 2016). CEP-like peptide effector mimics that identified from Meloidogyne and CEPs mediate the nitrogen-demand signaling, nodulation, and lateral root development in plants (Taleski et al., 2018). Plant CEPs peptides can move from xylem vessels to the shoots and bind to the leucine-rich repeat receptors, CEPR1 and CEPR2 (Tabata and Sawa, 2014), which induces the CEP downstream 1/2 (CEPD1/2) to upregulate the nitrogen transporter NRT2.1 (Ota et al., 2020). Over-expression of CEP displays phenotype with reduced root cell proliferation and primary root elongation, and increased lateral root development (Ohkubo et al., 2017). Nematode CEPs were also found to upregulate NRT2.1 and reduce primary root length. Eves-Van Den Akker and colleagues suggested that nematodes CEPs limit the expansion of feeding sites to prevent draining excessive nutrient from plants and kill host plants (Eves-Van Den Akker et al., 2016).
Bacterial pathogens also manipulate auxin signaling to alter root development (Kong et al., 2020). Wound caused by emerging lateral roots can be an entry point of bacterial pathogens including Pseudomonas syringae pv. tomato strain DC3000 (Pto DC3000; Kong et al., 2020). Interestingly, Pto DC3000 infection strongly triggered lateral root formation. Auxin response factor 7 (ARF7) and ARF19 are required for the Pto DC3000-induced lateral root formation. SA, a key phytohormone against biotrophic pathogens, can suppress lateral root formation, presumably blocking bacteria entrance. Arabidopsis SA deficit mutants show enhanced DC3000-induced lateral root development. ARF7, a well-known regulator of lateral root development, antagonizes the expression of SA marker genes and promotes lateral root development (Kong et al., 2020). These observations indicate an antagonistic interaction between ARF7-regulated auxin signaling and SA signaling in governing lateral root formation, a potential entrance of pathogens into Arabidopsis (Kong et al., 2020). It is speculated that Pto DC3000 can manipulate auxin signaling to promote entrance during infection. The virulence factor triggers this developmental change is still unclear (Kong et al., 2020).
Arabidopsis infected by R. fascians, a gram-positive phytopathogenic bacterium, displays a spectrum of developmental phenotypes including a narrow lamina, serrated leaf margin, and an uneven leaf surface (Manes et al., 2004; Depuydt et al., 2009). One cellular change associated with these developmental phenotypes is the neoplastic outgrowth in the infected tissue caused by excessive mitotic cell division (Depuydt et al., 2009). R. fascians employs multiple strategies to keep infected leaves at an undifferentiated stage. For example, Class-I KNOX genes (KNOTTED-like homeobox, KNAT), required for maintaining the undifferentiated status of cells in the shoot apical meristem, are induced in symptomatic leaves (Depuydt et al., 2008). Constitutional expression of KNOX in plants led to the reduction of gibberellic acids (GA) activity and induction of cytokinin (CK) levels. R. fascians infection modulates the plant CK metabolism and activates the CK biosynthesis via Arabidopsis response regulators 5/cytokinin 5 (ARR5/CK5) signaling, resulting in small and narrow leaf blades and serrated leaf margins (Depuydt et al., 2008). In addition, R. fascians can recruit the CYCLIN D3/RETINOBLASTOMA RELATED (CYCD3/RBR) pathway to stimulate G1-to-S transition and promote proliferation over differentiation (Depuydt et al., 2008, 2009). It is suggested that R. fascians infection stimulated the two major cell cycle checkpoints transition, which plays a critical role in symptom development. Consequently, with the cell cycle checkpoints manipulation and hormonal signaling regulation, the infected leaves will reach a state of eternal youth. Although it is unclear how R. fascians benefits from undifferentiated cells, studies on age-related disease resistance suggest that pre-mature tissues tend to be more susceptible to pathogen infection (Ficke et al., 2002; Calonnec et al., 2018; Mansfeld et al., 2020). Premature cucumber and strawberries are more susceptible to phytophthora and fungal pathogens (Asalf et al., 2014; Mansfeld et al., 2020). It is reasonable to speculate that R. fascians may use multiple strategies to suppress the host ontogenetic resistance by keeping infected leaves at a young stage.
Phytoplasmas are a group of obligate phloem bacterial pathogens that can be transmitted among its host plants by insect vectors (Sugio et al., 2011b). Phytoplasmas infected plants display phloem necrosis, witches’ broom, phyllody, and dwarfism (Hogenhout et al., 2008, 2009; Rashid et al., 2018; Omenge et al., 2021). Recent studies revealed that such developmental changes are induced by phytoplasmas effectors (Hogenhout et al., 2008; Hogenhout and Loria, 2008; Sugio et al., 2011a,b; Bertaccini et al., 2019; Furch et al., 2021; Omenge et al., 2021).
Witches’ broom and dwarfism associated with phytoplasma infection can be induced by single effectors. Transient expression of phytoplasma virulence effector, tengu-su inducer (TENGU), secreted by Onion Yellows phytoplasma strain Mild (OY-M), in Nicotiana benthamiana phenocopied witches’ broom and dwarfism symptoms (Hoshi et al., 2009). TENGU localizes in parenchyma, meristem tissues, and the apical buds. Transgenic TENGU plants showed downregulated auxin-responsive genes in microarray analysis, including Auxin/Indole-3-Acetic Acid (AUX/IAA) family genes IAA29, and IAA7/AUX2, small auxin-induced RNA (SAUR) family genes SAUR_AC1, and Gretchen Hagen 3 (GH3) family genes GH3.5/WES1, which suggested that TENGU can inhibit auxin-related signaling and alter plants development (Hoshi et al., 2009). In addition, TENGU can interfere with the jasmonic acid biosynthesis to cause plant sterility without floral malformations (Minato et al., 2014). Auxin response factors, ARF6 and ARF8, promote floral maturation by activating Jasmonic Acid (JA) synthesis or by decreasing JA degradation (Nagpal et al., 2005). The expression of ARF6 and ARF8 were significantly decreased in both transgenic TENGU-plants and phytoplasma-infected plants. Consequently, JA level was decreased in TENGU-transgenic buds (Minato et al., 2014). Thus, TENGU hijack multiple nodes of auxin signaling to manipulate flower development.
Phytoplasma effectors also contribute to the phyllody phenotype (Bertaccini, 2007). The molecular mechanism of flower abnormalities induced by phytoplasma infection was first documented in tomatoes (Pracros et al., 2006; Sugio et al., 2011a,b). Stolbur phytoplasma infected tomato showed virescence, phyllody, sepal hypertrophy, and aborted reproductive organs (Pracros et al., 2006). Expressing SAP54, an effector of Aster Yellows phytoplasma strain Witches’ Broom (AY-WB), was sufficient to induce phyllody in Arabidopsis (Aurin et al., 2020). Further studies showed that SAP54 alters the host plant reproductive and floral development by degrading a group of type II MADS-domain transcription factors (MTFs) regulating the floral transition and floral organ development (Jagdale et al., 2021). Arabidopsis radiation-sensitive-23 (RAD23) family proteins, RAD23C, and RAD23D, physically interact with SAP54 and are required for the degradation of host MTFs and phytoplasma-induced phyllody (MacLean et al., 2014). Aster leafhopper Macrosteles quadrilineatus has oviposition preference for plants with green leaf-like flowers induced by SAP54 (MacLean et al., 2011; Aurin et al., 2020). It is proposed that SAP54-induced phyllody facilitates the transmission of AY-WB.
Another phytoplasma AY-WB secreted effector protein 11 (SAP11) alters shoot proliferation and leaf shape changes (Pecher et al., 2019). Arabidopsis transgenic plant expressing SAP11 displays large curly leaves and overproduces axillary stems (Martín-Trillo and Cubas, 2010; Sugio et al., 2011a,b). SAP11 interacts with and destabilizes Arabidopsis TCP [TEOSINTE BRANCHED, CYCLOIDEA, PROLIFERATING CELL NUCLEAR ANTIGEN FACTOR1 (PCF1), and PCF2] transcription factors including TEOSINTE BRANCHED1, PROLIFERATING CELL FACTORS 1 and 2, TCP2, TCP7, TCP13, and CYCLOIDEA transcription factors (Martín-Trillo and Cubas, 2010; Sugio et al., 2011a,b). TCP transcription factors function to suppress excessive growth of leaf and shoot branching during normal growth (Dhaka et al., 2017). Although it is unclear how the altered leaf morphology and branching number benefit phytoplasma, SAP11 can also suppress JA biosynthesis to enhance the survival and reproduction of the insect vectors (Sugio et al., 2011a). JA is a major phytohormone that is involved in the defense against the AY-WB leafhopper vector M. quadrilineatus and M. quadrilineatus can produce about 60% more progeny on AY-WB-infected plants with the decreased JA expression (Sugio et al., 2011a). The transgenic Arabidopsis plants expressing SAP11 accumulate less JA. The destabilized Arabidopsis TCPs by SAP11 can reduce the lipoxygenase (LOX) genes expression, which leads to reduced JA synthesis (Sugio et al., 2011a). SAP11 homologs are reported to alter the development as well in other plant species including maize, wheat, and coconut (Pecher et al., 2019). Maize Bushy Stunt Phytoplasma (MBSP), a SAP11 homolog that mainly infects maize, can also bind the helix–loop–helix region of the TCP domain, and destabilize the TEOSINTE BRANCHED 1/CYCLOIDEA (TB1/CYC) TCPs. SAP11 homolog of MBSP can induce axillary branching like the AY-WB SAP11, while preventing the female inflorescence development and inducing the tassels feminization (Pecher et al., 2019). Since all the disease symptoms induced by the phytoplasmas generate more young and green tissues, phytoplasma insect vectors have preference on the young and green tissues for feeding and laying eggs (Hogenhout et al., 2008; Hogenhout and Loria, 2008). It is speculative that phytoplasma induced developmental changes are part of the pathogen’s virulence strategy to enhance their fitness. Phytoplasmas generates effectors that target plant development processes resulting in generating more young vegetative tissue (witches’ broom and phyllody, etc.) or prolonging the plant host lifespan. The young vegetative tissue attracts more insect sectors, which helps the transmission of phytoplasmas. Phytoplasmas, as a biotrophic pathogen, benefits from the prolonged lifespan of its plant host, which increases the phytoplasmas fitness. Another speculation is that phytoplasmas modulate the plants’ development to regulate the hormone production or alter the plants defense hormone signaling like JA, thus increasing the susceptibility of the host, which improves fitness (Sugio et al., 2011b).
During host-pathogen interaction, plants may actively reprogram their growth and development as a defense strategy. Cauline leaves of Arabidopsis were shed after infected by Pto DC3000 with a functional type III secretion, which is proposed as a defense mechanism to limit pathogen invasion (Patharkar et al., 2017). Leaf abscission as an active defense only occurs when bacteria physically contact the cauline leaf abscission zone. Regulators of abscission in normal development such as HAESA/HAESA-like 2, INFLORESCENCE DEFICIENT IN ABSCISSION, and NEVERSHED were required for the leaf abscission under bacterial infection, indicating that a normal developmental machinery was reprogrammed during plant-microbe interaction. SA may serve as a link between bacterial sensing and leaf shedding since SA-deficient mutants fail to shed cauline leaves upon infection (Patharkar et al., 2017). Several other plant species show abscission of infected organs in response to pathogens as well. For example, tomatoes shed their leaves in response to P. syringae and powdery mildew infection (Lim and Kunkel, 2005; Kissoudis et al., 2016), suggesting that shedding infected organs might be a common defense mechanism via reprogramming a developmental process.
Plants can also change the timing of their developmental progression as a defense strategy. Arabidopsis seed germination was arrested in the presence of Pseudomonas aeruginosa (Chahtane et al., 2018). l-2-Amino-4-methoxy-trans-3-butenoic acid (AMB) is a non-proteinogenic amino acid toxin for prokaryotes and eukaryotes produced by P. aeruginosa. Interestingly, upon the detection of AMB, plants induced DELLA proteins in seeds, leading to a DELLA-dependent but GA-independent arrest of seed germination. In this process, germination repressor ABI5 was also over-accumulated (Chahtane et al., 2018). Since arrested seed germination has no clear benefit to the bacterial pathogen, it is speculated that the delay of germination in the presence of P. aeruginosa is to avoid deleterious seedling infection.
In another case, accelerated flowering together with increase branches were observed in Arabidopsis challenged with three different pathogen species including two bacterial pathogens, P. syringae and Xanthomonas campestris, and an oomycete, Peronospora parasitica (Korves and Bergelson, 2003). Korves suggested that these similar developmental changes in susceptible Arabidopsis were the general developmental responses to pathogen infection that may affect tolerance of and/or resistance to disease (Korves and Bergelson, 2003). It is noteworthy that plants gain age-related resistance (ARR) during shoot maturation including flowering (Wilson et al., 2013; Lyons et al., 2015; Hu and Yang, 2019). For example, Nicotiana tabacum was found to gain resistance to pathogen P. parasitica upon flowering (Wyatt and Kuc, 1992). Thus, pathogen-induced flowering could be a defense mechanism to activate ARR.
Stomata is one of the natural openings for many pathogens to enter plant hosts (Melotto et al., 2006, 2008). The regulation of stomata opening is a battlefield of host and pathogens. On one side, plants motivate immune responses to close stomata upon pathogen detection, while pathogens use hormone mimics or effector proteins to open stomata (Gimenez-Ibanez et al., 2014). Interestingly, the battle is not only limited to mature stomata but extends to the early stage of stomata differentiation. Upon P. syringae pv. tomato (Pto DC3000) infection, Arabidopsis reduces stomatal density by 20% in the subsequently developed new leaves with many epidermal pavement cells (Dutton et al., 2019). It is speculated that such a reduction serves as a defense mechanism to decrease bacteria entry. The mechanism of pathogen-induced reduction of stomatal density remains unknown. Dutton suggested that the stomatal reduction is possibly mediated by components of plant immune system such as the AZI protein, a potential systemic signal, flagellin receptor, and SA biosynthesis (Dutton et al., 2019). Given that Pto DC3000 infection could trigger lateral root emergence (Kong et al., 2020), plant host and pathogens are in an arms race to create or limit entrance.
Here, we summarize the current understanding of pathogen-triggered changes in plant development (Figure 1). Recent progress in studying pathogens’ virulence strategies, such as function of effectors, have helped to distinguish whether altered host development is a consequence of pathogen virulence or active host defense. However, it is still challenging to elucidate how a pathogen-induced developmental change benefits the causal agent. A deeper investigation of pathogen physiology and life cycle is valuable to fill the gap. Although our understanding of the plant development-defense tradeoff is accumulating (Huot et al., 2014; He et al., 2022), further dissecting the molecular details of host development-defense crosstalk will contribute to understanding how developmental changes can be used as a defense tool.
Figure 1. Pathogens triggered changes in plants development. Pathogen-triggered development changes in plants can be results of active defense strategies, including accelerating flowering time, shed its cauline leaves, reduced the stomatal density, and arrest seed germination. Pathogens’ virulence strategies also target host development. Such changes can be achieved by effectors, such as phytoplasma effector induced phyllody and witches’ broom, or hormone mimics including neoplastic growth induced by Rhodococcus fascians and giant cell/syncytium formed during nematode infection. The Arabidopsis model was cited from Illustrations, Plant (2017): Shoot illustrations. Figshare. Collection. https://doi.org/10.6084/m9.figshare.c.3701035.v13.
FK and LY conceptualized and wrote the manuscript. All authors contributed to the article and approved the submitted version.
This project is supported by NIH R35GM143067 to LY.
We thank all the members of Yang lab for helpful discussions and critical reading and reviews of this manuscript.
The authors declare that the research was conducted in the absence of any commercial or financial relationships that could be construed as a potential conflict of interest.
All claims expressed in this article are solely those of the authors and do not necessarily represent those of their affiliated organizations, or those of the publisher, the editors and the reviewers. Any product that may be evaluated in this article, or claim that may be made by its manufacturer, is not guaranteed or endorsed by the publisher.
Asalf, B., Gadoury, D. M., Tronsmo, A. M., Seem, R. C., Dobson, A., Peres, N. A., et al. (2014). Ontogenic resistance of leaves and fruit, and how leaf folding influences the distribution of powdery mildew on strawberry plants colonized by Podosphaera aphanis. Phytopathology 104, 954–963. doi: 10.1094/phyto-12-13-0345-r
Aurin, M. B., Haupt, M., Gorlach, M., Rumpler, F., and Theissen, G. (2020). Structural requirements of the phytoplasma effector protein SAP54 for causing homeotic transformation of floral organs. Mol. Plant-Microbe Interact. 33, 1129–1141. doi: 10.1094/MPMI-02-20-0028-R
Bednarek, P. (2012). Chemical warfare or modulators of defence responses—the function of secondary metabolites in plant immunity. Curr. Opin. Plant Biol. 15, 407–414. doi: 10.1016/j.pbi.2012.03.002
Bertaccini, A. (2007). Phytoplasmas: diversity, taxonomy, and epidemiology. Front. Biosci. J. Virtual 12, 673–689. doi: 10.2741/2092
Bertaccini, A., Oshima, K., Maejima, K., and Namba, S. (2019). “Phytoplasma effectors and pathogenicity factors. Phytoplasma Pffectors and pathogenicity factors” in Phytoplasmas: Plant Pathogenic Bacteria – III. eds. A. Bertaccini, K. Oshima, M. Kube, and G. Rao(Singapore: Springer), 17–34.
Bigeard, J., Colcombet, J., and Hirt, H. (2015). Signaling mechanisms in pattern-triggered immunity (PTI). Mol. Plant 8, 521–539. doi: 10.1016/j.molp.2014.12.022
Brown, J. K. (2002). Yield penalties of disease resistance in crops. Curr. Opin. Plant Biol. 5, 339–344. doi: 10.1016/s1369-5266(02)00270-4
Budke, J. M., and Goffinet, B. (2016). Comparative cuticle development reveals taller sporophytes are covered by thicker calyptra cuticles in mosses. Front. Plant Sci. 7:832. doi: 10.3389/fpls.2016.00832
Burdon, J. J., and Thrall, P. H. (2009). Coevolution of plants and their pathogens in natural habitats. Science 324, 755–756. doi: 10.1126/science.1171663
Calonnec, A., Jolivet, J., Vivin, P., and Schnee, S. (2018). Pathogenicity traits correlate with the susceptible vitis vinifera leaf physiology transition in the biotroph fungus Erysiphe necator: an adaptation to plant ontogenic resistance. Front. Plant Sci. 9:1808. doi: 10.3389/fpls.2018.01808
Carella, P., Wilson, D. C., and Cameron, R. K. (2014). Some things get better with age: differences in salicylic acid accumulation and defense signaling in young and mature Arabidopsis. Front. Plant Sci. 5:775. doi: 10.3389/fpls.2014.00775
Chahtane, H., Nogueira Fuller, T., Allard, P. M., Marcourt, L., Ferreira Queiroz, E., Shanmugabalaji, V., et al. (2018). The plant pathogen Pseudomonas aeruginosa triggers a DELLA-dependent seed germination arrest in Arabidopsis. eLife 7:e37082. doi: 10.7554/eLife.37082
Chang, M., Chen, H., Liu, F., and Fu, Z. Q. (2022). PTI and ETI: convergent pathways with diverse elicitors. Trends Plant Sci. 27, 113–115. doi: 10.1016/j.tplants.2021.11.013
Chen, S., Lang, P., Chronis, D., Zhang, S., De Jong, W. S., Mitchum, M. G., et al. (2015). In planta processing and glycosylation of a nematode CLAVATA3/ENDOSPERM SURROUNDING REGION-like effector and its interaction with a host CLAVATA2-like receptor to promote parasitism. Plant Physiol. 167, 262–272. doi: 10.1104/pp.114.251637
Depuydt, S., De Veylder, L., Holsters, M., and Vereecke, D. (2009). Eternal youth, the fate of developing Arabidopsis leaves upon Rhodococcus fascians infection. Plant Physiol. 149, 1387–1398. doi: 10.1104/pp.108.131797
Depuydt, S., Doležal, K., Van Lijsebettens, M., Moritz, T., Holsters, M., and Vereecke, D. (2008). Modulation of the hormone setting by Rhodococcus fascians results in ectopic KNOX activation in Arabidopsis. Plant Physiol. 146, 1267–1281. doi: 10.1104/pp.107.113969
Deslandes, L., and Rivas, S. (2012). Catch me if you can: bacterial effectors and plant targets. Trends Plant Sci. 17, 644–655. doi: 10.1016/j.tplants.2012.06.011
Dhaka, N., Bhardwaj, V., Sharma, M. K., and Sharma, R. (2017). Evolving tale of TCPs: new paradigms and old lacunae. Front. Plant Sci. 8:479. doi: 10.3389/fpls.2017.00479
Dou, D., and Zhou, J. M. (2012). Phytopathogen effectors subverting host immunity: different foes, similar battleground. Cell Host Microbe 12, 484–495. doi: 10.1016/j.chom.2012.09.003
Dutton, C., Horak, H., Hepworth, C., Mitchell, A., Ton, J., Hunt, L., et al. (2019). Bacterial infection systemically suppresses stomatal density. Plant Cell Environ. 42, 2411–2421. doi: 10.1111/pce.13570
Emonet, A., Zhou, F., Vacheron, J., Heiman, C. M., Denervaud Tendon, V., Ma, K. W., et al. (2021). Spatially restricted immune responses are required for maintaining root meristematic activity upon detection of bacteria. Curr. Biol. 31, 1012–1028.e7. doi: 10.1016/j.cub.2020.12.048
Eves-Van Den Akker, S., Lilley, C. J., Yusup, H. B., Jones, J. T., and Urwin, P. E. (2016). Functional C-TERMINALLY ENCODED PEPTIDE (CEP) plant hormone domains evolved de novo in the plant parasite Rotylenchulus reniformis. Mol. Plant Pathol. 17, 1265–1275. doi: 10.1111/mpp.12402
Ficke, A., Gadoury, D. M., and Seem, R. C. (2002). Ontogenic resistance and plant disease management: a case study of grape powdery mildew. Phytopathology 92, 671–675. doi: 10.1094/phyto.2002.92.6.671
Figueroa-Macías, J. P., García, Y. C., Núñez, M., Díaz, K., Olea, A. F., and Espinoza, L. (2021). Plant growth-defense trade-offs: molecular processes leading to physiological changes. Int. J. Mol. Sci. 22:693. doi: 10.3390/ijms22020693
Furch, A. C. U., Theissen, G., and Zimmermann, M. R. (2021). New phytoplasma effector: 50 shades of green. Cell Host Microbe 29, 1601–1603. doi: 10.1016/j.chom.2021.10.008
Gimenez-Ibanez, S., Boter, M., Fernández-Barbero, G., Chini, A., Rathjen, J. P., and Solano, R. (2014). The bacterial effector HopX1 targets JAZ transcriptional repressors to activate jasmonate signaling and promote infection in Arabidopsis. PLoS Biol. 12:e1001792. doi: 10.1371/journal.pbio.1001792
Guo, X., Wang, J., Gardner, M., Fukuda, H., Kondo, Y., Etchells, J. P., et al. (2017). Identification of cyst nematode B-type CLE peptides and modulation of the vascular stem cell pathway for feeding cell formation. PLoS Pathog. 13:e1006142. doi: 10.1371/journal.ppat.1006142
Guttman, D. S., Gropp, S. J., Morgan, R. L., and Wang, P. W. (2006). Diversifying selection drives the evolution of the type III secretion system pilus of Pseudomonas syringae. Mol. Biol. Evol. 23, 2342–2354. doi: 10.1093/molbev/msl103
Hamann, T. (2012). Plant cell wall integrity maintenance as an essential component of biotic stress response mechanisms. Front. Plant Sci. 3:77. doi: 10.3389/fpls.2012.00077
Harris, M. O., and Pitzschke, A. (2020). Plants make galls to accommodate foreigners: some are friends, most are foes. New Phytol. 225, 1852–1872. doi: 10.1111/nph.16340
Hayashi, F., Smith, K. D., Ozinsky, A., Hawn, T. R., Yi, E. C., Goodlett, D. R., et al. (2001). The innate immune response to bacterial flagellin is mediated by toll-like receptor 5. Nature 410, 1099–1103. doi: 10.1038/35074106
He, Z., Webster, S., and He, S. Y. (2022). Growth-defense trade-offs in plants. Curr. Biol. 32, R634–r639. doi: 10.1016/j.cub.2022.04.070
Hewezi, T., Juvale, P. S., Piya, S., Maier, T. R., Rambani, A., Rice, J. H., et al. (2015). The cyst nematode effector protein 10A07 targets and recruits host posttranslational machinery to mediate its nuclear trafficking and to promote parasitism in Arabidopsis. Plant Cell 27, 891–907. doi: 10.1105/tpc.114.135327
Hogenhout, S. A., and Loria, R. (2008). Virulence mechanisms of gram-positive plant pathogenic bacteria. Curr. Opin. Plant Biol. 11, 449–456. doi: 10.1016/j.pbi.2008.05.007
Hogenhout, S. A., Oshima, K., Ammar el, D., Kakizawa, S., Kingdom, H. N., and Namba, S. (2008). Phytoplasmas: bacteria that manipulate plants and insects. Mol. Plant Pathol. 9, 403–423. doi: 10.1111/j.1364-3703.2008.00472.x
Hogenhout, S. A., Van der Hoorn, R. A. L., Terauchi, R., and Kamoun, S. (2009). Emerging concepts in effector biology of plant-associated organisms. Mol. Plant-Microbe Interact. 22, 115–122. doi: 10.1094/mpmi-22-2-0115
Hoshi, A., Oshima, K., Kakizawa, S., Ishii, Y., Ozeki, J., Hashimoto, M., et al. (2009). A unique virulence factor for proliferation and dwarfism in plants identified from a phytopathogenic bacterium. Proc. Natl. Acad. Sci. U. S. A. 106, 6416–6421. doi: 10.1073/pnas.0813038106
Howden, A. J., and Huitema, E. (2012). Effector-triggered post-translational modifications and their role in suppression of plant immunity. Front. Plant Sci. 3:160. doi: 10.3389/fpls.2012.00160
Hu, L., and Yang, L. (2019). Time to fight: molecular mechanisms of age-related resistance. Phytopathology 109, 1500–1508. doi: 10.1094/phyto-11-18-0443-rvw
Huang, W., MacLean, A. M., Sugio, A., Maqbool, A., Busscher, M., Cho, S. T., et al. (2021). Parasitic modulation of host development by ubiquitin-independent protein degradation. Cell 184, 5201–5214.e5212. doi: 10.1016/j.cell.2021.08.029
Huot, B., Yao, J., Montgomery, B. L., and He, S. Y. (2014). Growth-defense tradeoffs in plants: a balancing act to optimize fitness. Mol. Plant 7, 1267–1287. doi: 10.1093/mp/ssu049
Ingram, G., and Nawrath, C. (2017). The roles of the cuticle in plant development: organ adhesions and beyond. J. Exp. Bot. 68, 5307–5321. doi: 10.1093/jxb/erx313
Ito, Y., Nakanomyo, I., Motose, H., Iwamoto, K., Sawa, S., Dohmae, N., et al. (2006). Dodeca-CLE peptides as suppressors of plant stem cell differentiation. Science 313, 842–845. doi: 10.1126/science.1128436
Jagdale, S., Rao, U., and Giri, A. P. (2021). Effectors of root-knot nematodes: an arsenal for successful parasitism. Front. Plant Sci. 12:800030. doi: 10.3389/fpls.2021.800030
Janik, K., Mithofer, A., Raffeiner, M., Stellmach, H., Hause, B., and Schlink, K. (2017). An effector of apple proliferation phytoplasma targets TCP transcription factors-a generalized virulence strategy of phytoplasma?. Mol. Plant Pathol. 18, 435–442. doi: 10.1111/mpp.12409
Jones, J. D., and Dangl, J. L. (2006). The plant immune system. Nature 444, 323–329. doi: 10.1038/nature05286
Jones, J. D., Vance, R. E., and Dangl, J. L. (2016). Intracellular innate immune surveillance devices in plants and animals. Science 354:aaf6395. doi: 10.1126/science.aaf6395
Kaku, H., Nishizawa, Y., Ishii-Minami, N., Akimoto-Tomiyama, C., Dohmae, N., Takio, K., et al. (2006). Plant cells recognize chitin fragments for defense signaling through a plasma membrane receptor. Proc. Natl. Acad. Sci. U. S. A. 103, 11086–11091. doi: 10.1073/pnas.0508882103
Katsir, L., Davies, K. A., Bergmann, D. C., and Laux, T. (2011). Peptide signaling in plant development. Curr. Biol. 21, R356–R364. doi: 10.1016/j.cub.2011.03.012
Kissoudis, C., Sunarti, S., van de Wiel, C., Visser, R. G., van der Linden, C. G., and Bai, Y. (2016). Responses to combined abiotic and biotic stress in tomato are governed by stress intensity and resistance mechanism. J. Exp. Bot. 67, 5119–5132. doi: 10.1093/jxb/erw285
Kong, X., Zhang, C., Zheng, H., Sun, M., Zhang, F., Zhang, M., et al. (2020). Antagonistic interaction between auxin and SA signaling pathways regulates bacterial infection through lateral root in Arabidopsis. Cell Rep. 32:108060. doi: 10.1016/j.celrep.2020.108060
Korves, T. M., and Bergelson, J. (2003). A developmental response to pathogen infection in Arabidopsis. Plant Physiol. 133, 339–347. doi: 10.1104/pp.103.027094
Kus, J. V., Zaton, K., Sarkar, R., and Cameron, R. K. (2002). Age-related resistance in Arabidopsis is a developmentally regulated defense response to Pseudomonas syringae. Plant Cell 14, 479–490. doi: 10.1105/tpc.010481
Kwi-Mi, C., Kadunari, I., Naoyuki, U., and Masao, T. (2008). New perspectives on plant defense responses through modulation of developmental pathways. Mol. Cell 26, 107–112. doi: 10.14348/.1970.0.0
Lee, C., Chronis, D., Kenning, C., Peret, B., Hewezi, T., Davis, E. L., et al. (2011). The novel cyst nematode effector protein 19C07 interacts with the Arabidopsis auxin influx transporter LAX3 to control feeding site development. Plant Physiol. 155, 866–880. doi: 10.1104/pp.110.167197
Li, P., Lu, Y. J., Chen, H., and Day, B. (2020). The lifecycle of the plant immune system. CRC Crit. Rev. Plant Sci. 39, 72–100. doi: 10.1080/07352689.2020.1757829
Lim, M. T., and Kunkel, B. N. (2005). The Pseudomonas syringae avrRpt2 gene contributes to virulence on tomato. Mol. Plant-Microbe Interact. 18, 626–633. doi: 10.1094/mpmi-18-0626
Lu, S. W., Chen, S., Wang, J., Yu, H., Chronis, D., Mitchum, M. G., et al. (2009). Structural and functional diversity of CLAVATA3/ESR (CLE)-like genes from the potato cyst nematode Globodera rostochiensis. Mol. Plant-Microbe Interact. 22, 1128–1142. doi: 10.1094/mpmi-22-9-1128
Lyons, R., Rusu, A., Stiller, J., Powell, J., Manners, J. M., and Kazan, K. (2015). Investigating the association between flowering time and defense in the Arabidopsis thaliana-Fusarium oxysporum interaction. PLoS One 10:e0127699. doi: 10.1371/journal.pone.0127699
MacLean, A. M., Orlovskis, Z., Kowitwanich, K., Zdziarska, A. M., Angenent, G. C., Immink, R. G., et al. (2014). Phytoplasma effector SAP54 hijacks plant reproduction by degrading MADS-box proteins and promotes insect colonization in a RAD23-dependent manner. PLoS Biol. 12:e1001835. doi: 10.1371/journal.pbio.1001835
MacLean, A. M., Sugio, A., Makarova, O. V., Findlay, K. C., Grieve, V. M., Toth, R., et al. (2011). Phytoplasma effector SAP54 induces indeterminate leaf-like flower development in Arabidopsis plants. Plant Physiol. 157, 831–841. doi: 10.1104/pp.111.181586
Maejima, K., Iwai, R., Himeno, M., Komatsu, K., Kitazawa, Y., Fujita, N., et al. (2014). Recognition of floral homeotic MADS domain transcription factors by a phytoplasmal effector, phyllogen, induces phyllody. Plant J. 78, 541–554. doi: 10.1111/tpj.12495
Manes, C. L. D. O., Beeckman, T., Ritsema, T., Van Montagu, M., Goethals, K., and Holsters, M. (2004). Phenotypic alterations in Arabidopsis thaliana plants caused by Rhodococcus fascians infection. J. Plant Res. 117, 139–145. doi: 10.1007/s10265-003-0138-y
Mansfeld, B. N., Colle, M., Zhang, C., Lin, Y. C., and Grumet, R. (2020). Developmentally regulated activation of defense allows for rapid inhibition of infection in age-related resistance to Phytophthora capsici in cucumber fruit. BMC Genomics 21:628. doi: 10.1186/s12864-020-07040-9
Martín-Trillo, M., and Cubas, P. (2010). TCP genes: a family snapshot ten years later. Trends Plant Sci. 15, 31–39. doi: 10.1016/j.tplants.2009.11.003
Meents, M. J., Watanabe, Y., and Samuels, A. L. (2018). The cell biology of secondary cell wall biosynthesis. Ann. Bot. 121, 1107–1125. doi: 10.1093/aob/mcy005
Melotto, M., Underwood, W., and He, S. Y. (2008). Role of stomata in plant innate immunity and foliar bacterial diseases. Annu. Rev. Phytopathol. 46, 101–122. doi: 10.1146/annurev.phyto.121107.104959
Melotto, M., Underwood, W., Koczan, J., Nomura, K., and He, S. Y. (2006). Plant stomata function in innate immunity against bacterial invasion. Cells 126, 969–980. doi: 10.1016/j.cell.2006.06.054
Meng, X., and Zhang, S. (2013). MAPK cascades in plant disease resistance signaling. Annu. Rev. Phytopathol. 51, 245–266. doi: 10.1146/annurev-phyto-082712-102314
Minato, N., Himeno, M., Hoshi, A., Maejima, K., Komatsu, K., Takebayashi, Y., et al. (2014). The phytoplasmal virulence factor TENGU causes plant sterility by downregulating of the jasmonic acid and auxin pathways. Sci. Rep. 4:7399. doi: 10.1038/srep07399
Mitchum, M. G., Wang, X., Wang, J., and Davis, E. L. (2012). Role of nematode peptides and other small molecules in plant parasitism. Annu. Rev. Phytopathol. 50, 175–195. doi: 10.1146/annurev-phyto-081211-173008
Mur, L. A., Kenton, P., Lloyd, A. J., Ougham, H., and Prats, E. (2008). The hypersensitive response; the centenary is upon us but how much do we know? J. Exp. Bot. 59, 501–520. doi: 10.1093/jxb/erm239
Nagpal, P., Ellis, C. M., Weber, H., Ploense, S. E., Barkawi, L. S., Guilfoyle, T. J., et al. (2005). Auxin response factors ARF6 and ARF8 promote jasmonic acid production and flower maturation. Development 132, 4107–4118. doi: 10.1242/dev.01955
Naveed, Z. A., Wei, X., Chen, J., Mubeen, H., and Ali, G. S. (2020). The PTI to ETI continuum in phytophthora-plant interactions. Front. Plant Sci. 11:593905. doi: 10.3389/fpls.2020.593905
Ngou, B. P. M., Ding, P., and Jones, J. D. G. (2022). Thirty years of resistance: Zig-zag through the plant immune system. Plant Cell 34, 1447–1478. doi: 10.1093/plcell/koac041
Niebel, A., Gheysen, G., and Van Montagu, M. (1994). Plant-cyst nematode and plant-root-knot nematode interactions. Parasitol. Today 10, 424–430. doi: 10.1016/0169-4758(94)90172-4
O’Brien, J. A., Daudi, A., Butt, V. S., and Bolwell, G. P. (2012). Reactive oxygen species and their role in plant defence and cell wall metabolism. Planta 236, 765–779. doi: 10.1007/s00425-012-1696-9
Ohkubo, Y., Tanaka, M., Tabata, R., Ogawa-Ohnishi, M., and Matsubayashi, Y. (2017). Shoot-to-root mobile polypeptides involved in systemic regulation of nitrogen acquisition. Nat. Plants 3:17029. doi: 10.1038/nplants.2017.29
Omenge, K. M., Rumpler, F., Kathalingam, S. S., Furch, A. C. U., and Theissen, G. (2021). Studying the function of phytoplasma effector proteins using a chemical-inducible expression system in transgenic plants. Int. J. Mol. Sci. 22. doi: 10.3390/ijms222413582
Oosterbeek, M., Lozano-Torres, J. L., Bakker, J., and Goverse, A. (2021). Sedentary plant-parasitic nematodes alter auxin homeostasis via multiple strategies. Front. Plant Sci. 12:668548. doi: 10.3389/fpls.2021.668548
Osbourn, A. E. (1996). Preformed antimicrobial compounds and plant defense against fungal attack. Plant Cell 8, 1821–1831. doi: 10.1105/tpc.8.10.1821
Ota, R., Ohkubo, Y., Yamashita, Y., Ogawa-Ohnishi, M., and Matsubayashi, Y. (2020). Shoot-to-root mobile CEPD-like 2 integrates shoot nitrogen status to systemically regulate nitrate uptake in Arabidopsis. Nat. Commun. 11:641. doi: 10.1038/s41467-020-14440-8
Patharkar, O. R., Gassmann, W., and Walker, J. C. (2017). Leaf shedding as an anti-bacterial defense in Arabidopsis cauline leaves. PLoS Genet. 13:e1007132. doi: 10.1371/journal.pgen.1007132
Pecher, P., Moro, G., Canale, M. C., Capdevielle, S., Singh, A., MacLean, A., et al. (2019). Phytoplasma SAP11 effector destabilization of TCP transcription factors differentially impact development and defence of Arabidopsis versus maize. PLoS Pathog. 15:e1008035. doi: 10.1371/journal.ppat.1008035
Pracros, P., Renaudin, J., Eveillard, S., Mouras, A., and Hernould, M. (2006). Tomato flower abnormalities induced by stolbur phytoplasma infection are associated with changes of expression of floral development genes. Mol. Plant-Microbe Interact. 19, 62–68. doi: 10.1094/mpmi-19-0062
Rashid, U., Bilal, S., Bhat, K. A., Shah, T. A., Wani, T. A., Bhat, F. A., et al. (2018). Phytoplasma effectors and their role in plant-insect interaction. Int. J. Curr. Microbiol. App. Sci. 7, 1136–1148. doi: 10.20546/ijcmas.2018.702.141
Replogle, A., Wang, J., Bleckmann, A., Hussey, R. S., Baum, T. J., Sawa, S., et al. (2011). Nematode CLE signaling in Arabidopsis requires CLAVATA2 and CORYNE. Plant J. 65, 430–440. doi: 10.1111/j.1365-313X.2010.04433.x
Rodriguez, E., El Ghoul, H., Mundy, J., and Petersen, M. (2016). Making sense of plant autoimmunity and 'negative regulators'. FEBS J. 283, 1385–1391. doi: 10.1111/febs.13613
Ronald, P., and Joe, A. (2018). Molecular mimicry modulates plant host responses to pathogens. Ann. Bot. 121, 17–23. doi: 10.1093/aob/mcx125
Rusterucci, C., Zhao, Z., Haines, K., Mellersh, D., Neumann, M., and Cameron, R. K. (2005). Age-related resistance to Pseudomonas syringae pv. Tomato is associated with the transition to flowering in Arabidopsis and is effective against Peronospora parasitica. Physiol. Mol. Plant Pathol. 66, 222–231. doi: 10.1016/j.pmpp.2005.08.004
Saijo, Y., Loo, E. P., and Yasuda, S. (2018). Pattern recognition receptors and signaling in plant-microbe interactions. Plant J. 93, 592–613. doi: 10.1111/tpj.13808
Sawinski, K., Mersmann, S., Robatzek, S., and Bohmer, M. (2013). Guarding the green: pathways to stomatal immunity. Mol. Plant-Microbe Interact. 26, 626–632. doi: 10.1094/MPMI-12-12-0288-CR
Siddique, S., Radakovic, Z. S., De La Torre, C. M., Chronis, D., Novak, O., Ramireddy, E., et al. (2015). A parasitic nematode releases cytokinin that controls cell division and orchestrates feeding site formation in host plants. Proc. Natl. Acad. Sci. U. S. A. 112, 12669–12674. doi: 10.1073/pnas.1503657112
Smith, L. G. (2001). Plant cell division: building walls in the right places. Nat. Rev. Mol. Cell Biol. 2, 33–39. doi: 10.1038/35048050
Stavrinides, J., McCann, H. C., and Guttman, D. S. (2008). Host-pathogen interplay and the evolution of bacterial effectors. Cell. Microbiol. 10, 285–292. doi: 10.1111/j.1462-5822.2007.01078.x
Sugio, A., Kingdom, H. N., MacLean, A. M., Grieve, V. M., and Hogenhout, S. A. (2011a). Phytoplasma protein effector SAP11 enhances insect vector reproduction by manipulating plant development and defense hormone biosynthesis. Proc. Natl. Acad. Sci. U. S. A. 108, E1254–E1263. doi: 10.1073/pnas.1105664108
Sugio, A., MacLean, A. M., Kingdom, H. N., Grieve, V. M., Manimekalai, R., and Hogenhout, S. A. (2011b). Diverse targets of phytoplasma effectors: from plant development to defense against insects. Annu. Rev. Phytopathol. 49, 175–195. doi: 10.1146/annurev-phyto-072910-095323
Swarup, K., Benková, E., Swarup, R., Casimiro, I., Péret, B., Yang, Y., et al. (2008). The auxin influx carrier LAX3 promotes lateral root emergence. Nat. Cell Biol. 10, 946–954. doi: 10.1038/ncb1754
Tabata, R., and Sawa, S. (2014). Maturation processes and structures of small secreted peptides in plants. Front. Plant Sci. 5:311. doi: 10.3389/fpls.2014.00311
Taleski, M., Imin, N., and Djordjevic, M. A. (2018). CEP peptide hormones: key players in orchestrating nitrogen-demand signalling, root nodulation, and lateral root development. J. Exp. Bot. 69, 1829–1836. doi: 10.1093/jxb/ery037
Tang, D., Wang, G., and Zhou, J. M. (2017). Receptor kinases in plant-pathogen interactions: more than pattern recognition. Plant Cell 29, 618–637. doi: 10.1105/tpc.16.00891
van Wersch, R., Li, X., and Zhang, Y. (2016). Mighty dwarfs: arabidopsis autoimmune mutants and their usages in genetic dissection of plant immunity. Front. Plant Sci. 7:1717. doi: 10.3389/fpls.2016.01717
Wang, N., Li, Y., Chen, W., Yang, H. Z., Zhang, P. H., and Wu, Y. F. (2018). Identification of wheat blue dwarf phytoplasma effectors targeting plant proliferation and defence responses. Plant Pathology 67, 603–609. doi: 10.1111/ppa.12786
Whitford, R., Fernandez, A., De Groodt, R., Ortega, E., and Hilson, P. (2008). Plant CLE peptides from two distinct functional classes synergistically induce division of vascular cells. Proc. Natl. Acad. Sci. U. S. A. 105, 18625–18630. doi: 10.1073/pnas.0809395105
Wilson, D. C., Carella, P., Isaacs, M., and Cameron, R. K. (2013). The floral transition is not the developmental switch that confers competence for the Arabidopsis age-related resistance response to Pseudomonas syringae pv. Tomato. Plant Mol. Biol. 83, 235–246. doi: 10.1007/s11103-013-0083-7
Wilson, D. C., Kempthorne, C. J., Carella, P., Liscombe, D. K., and Cameron, R. K. (2017). Age-related resistance in arabidopsis thaliana involves the MADS-domain transcription factor SHORT VEGETATIVE PHASE and direct action of salicylic acid on Pseudomonas syringae. Mol. Plant-Microbe Interact. 30, 919–929. doi: 10.1094/mpmi-07-17-0172-r
Wolf, S. (2022). Cell wall signaling in plant development and defense. Annu. Rev. Plant Biol. 73, 323–353. doi: 10.1146/annurev-arplant-102820-095312
Wyatt, S., and Kuc, J. (1992). The effect of leaf age, flowering, and senescence on the resistance of tobacco to blue mold. Phytopathology 80:1000.
Yamaguchi, Y. L., Ishida, T., and Sawa, S. (2016). CLE peptides and their signaling pathways in plant development. J. Exp. Bot. 67, 4813–4826. doi: 10.1093/jxb/erw208
Yeats, T. H., and Rose, J. K. (2013). The formation and function of plant cuticles. Plant Physiol. 163, 5–20. doi: 10.1104/pp.113.222737
Yin, H., Du, Y., and Dong, Z. (2016). Chitin oligosaccharide and chitosan oligosaccharide: two similar but different plant elicitors. Front. Plant Sci. 7:522. doi: 10.3389/fpls.2016.00522
Yuan, M., Ngou, B. P. M., Ding, P., and Xin, X. F. (2021). PTI-ETI crosstalk: an integrative view of plant immunity. Curr. Opin. Plant Biol. 62:102030. doi: 10.1016/j.pbi.2021.102030
Zhang, W., He, S. Y., and Assmann, S. M. (2008). The plant innate immunity response in stomatal guard cells invokes G-protein-dependent ion channel regulation. Plant J. 56, 984–996. doi: 10.1111/j.1365-313X.2008.03657.x
Zhang, L., Lilley, C. J., Imren, M., Knox, J. P., and Urwin, P. E. (2017). The complex cell wall composition of syncytia induced by plant parasitic cyst nematodes reflects both function and host plant. Front. Plant Sci. 8:1087. doi: 10.3389/fpls.2017.01087
Zhong, R., and Ye, Z. H. (2015). Secondary cell walls: biosynthesis, patterned deposition and transcriptional regulation. Plant Cell Physiol. 56, 195–214. doi: 10.1093/pcp/pcu140
Zhou, F., Emonet, A., Dénervaud Tendon, V., Marhavy, P., Wu, D., Lahaye, T., et al. (2020). Co-incidence of damage and microbial patterns controls localized immune responses in roots. Cells 180, 440–453.e18. doi: 10.1016/j.cell.2020.01.013
Zhou, J., Ma, F., Yao, Y., Deng, M., Chen, M., Zhang, S., et al. (2021). Jujube witches’ broom phytoplasma effectors SJP1 and SJP2 induce lateral bud outgrowth by repressing the ZjBRC1-controlled auxin efflux channel. Plant Cell Environ. 44, 3257–3272. doi: 10.1111/pce.14141
Keywords: plant-pathogen interaction, plant development, virulence, fitness, disease symptom
Citation: Kong F and Yang L (2023) Pathogen-triggered changes in plant development: Virulence strategies or host defense mechanism? Front. Microbiol. 14:1122947. doi: 10.3389/fmicb.2023.1122947
Received: 13 December 2022; Accepted: 25 January 2023;
Published: 15 February 2023.
Edited by:
Jinliang Liu, College of Plant Sciences, Jilin University, ChinaReviewed by:
Yiming Wang, Nanjing Agricultural University, ChinaCopyright © 2023 Kong and Yang. This is an open-access article distributed under the terms of the Creative Commons Attribution License (CC BY). The use, distribution or reproduction in other forums is permitted, provided the original author(s) and the copyright owner(s) are credited and that the original publication in this journal is cited, in accordance with accepted academic practice. No use, distribution or reproduction is permitted which does not comply with these terms.
*Correspondence: Li Yang, ✉ bGkueWFuZzFAdWdhLmVkdQ==
Disclaimer: All claims expressed in this article are solely those of the authors and do not necessarily represent those of their affiliated organizations, or those of the publisher, the editors and the reviewers. Any product that may be evaluated in this article or claim that may be made by its manufacturer is not guaranteed or endorsed by the publisher.
Research integrity at Frontiers
Learn more about the work of our research integrity team to safeguard the quality of each article we publish.