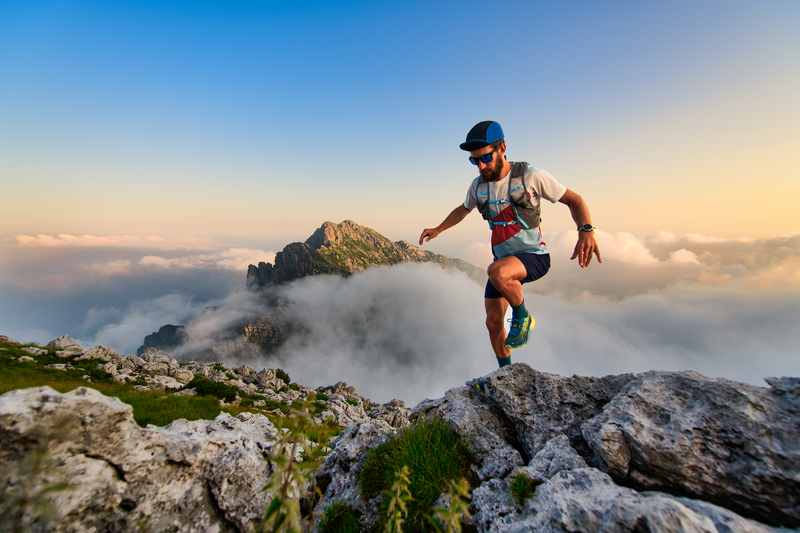
95% of researchers rate our articles as excellent or good
Learn more about the work of our research integrity team to safeguard the quality of each article we publish.
Find out more
ORIGINAL RESEARCH article
Front. Microbiol. , 22 March 2023
Sec. Microbiotechnology
Volume 14 - 2023 | https://doi.org/10.3389/fmicb.2023.1122078
This article is part of the Research Topic Engineering Microalgal Chassis Cells View all 15 articles
Cyanobacteria have raised great interest in biotechnology, e.g., for the sustainable production of molecular hydrogen (H2) using electrons from water oxidation. However, this is hampered by various constraints. For example, H2-producing enzymes compete with primary metabolism for electrons and are usually inhibited by molecular oxygen (O2). In addition, there are a number of other constraints, some of which are unknown, requiring unbiased screening and systematic engineering approaches to improve the H2 yield. Here, we introduced the regulatory [NiFe]-hydrogenase (RH) of Cupriavidus necator (formerly Ralstonia eutropha) H16 into the cyanobacterial model strain Synechocystis sp. PCC 6803. In its natural host, the RH serves as a molecular H2 sensor initiating a signal cascade to express hydrogenase-related genes when no additional energy source other than H2 is available. Unlike most hydrogenases, the C. necator enzymes are O2-tolerant, allowing their efficient utilization in an oxygenic phototroph. Similar to C. necator, the RH produced in Synechocystis showed distinct H2 oxidation activity, confirming that it can be properly matured and assembled under photoautotrophic, i.e., oxygen-evolving conditions. Although the functional H2-sensing cascade has not yet been established in Synechocystis yet, we utilized the associated two-component system consisting of a histidine kinase and a response regulator to drive and modulate the expression of a superfolder gfp gene in Escherichia coli. This demonstrates that all components of the H2-dependent signal cascade can be functionally implemented in heterologous hosts. Thus, this work provides the basis for the development of an intrinsic H2 biosensor within a cyanobacterial cell that could be used to probe the effects of random mutagenesis and systematically identify promising genetic configurations to enable continuous and high-yield production of H2via oxygenic photosynthesis.
The anthropogenic emission of greenhouse gases like carbon dioxide (CO2) derived from the usage of fossil resources is regarded as the major driver of climate change. To tackle this issue, new approaches need to be supplied toward a CO2-neutral society and economy. Molecular hydrogen (H2) is generally believed to be an ideal candidate as a future energy carrier due to its high energy density and greenhouse gas emission-free usage. Industrially, H2 is, however, still mainly obtained via steam reforming of natural gases, therefore relying on fossil resources and leading to a considerable greenhouse gas footprint (Howarth and Jacobson, 2021).
Biotechnological H2 production using microorganisms as whole-cell biocatalysts offers the advantage of a sustainable process based on renewable resources. These biological H2 formation routes encompass anaerobic fermentation using organic compounds as electron donors in, e.g., chemotrophic Clostridium and Enterobacter species or phototrophic sulfur and non-sulfur bacteria, as well as oxygenic photosynthesis using algae and cyanobacteria (Mahidhara et al., 2019). Approaches based on oxygenic photosynthesis appear most promising as they rely on electrons that have been obtained from light-dependent oxidation of water. Cyanobacteria are the only prokaryotes capable of this process. Great effort has been made to optimize H2 production within cyanobacterial models such as the unicellular strain Synechocystis sp. PCC 6803 (hereafter referred to as Synechocystis). However, the breakthrough to enable continuous H2 production in whole-cell cyanobacterial catalysts has not been achieved yet. Currently, it suffers from low yields and rates as well as the prototypical molecular oxygen (O2) sensitivity of the enzymes involved in the formation of H2, namely hydrogenases or nitrogenases. Hydrogenases are metalloenzymes that perform the reversible splitting of H2 into protons and electrons. They are grouped based on the composition of their active site into nickel-iron [NiFe]-, iron–iron [FeFe]-, and iron [Fe]- or Hmd-hydrogenases (Lubitz et al., 2014). Previous studies tackled, for instance, the catalytic performance of H2 production by introducing highly active, heterologous [FeFe]-hydrogenases into Synechocystis (Berto et al., 2011; Wegelius et al., 2018) or by fusing the endogenous [NiFe]-hydrogenase to photosystem I for a direct electron transfer from photosynthesis (Appel et al., 2020). The O2 sensitivity has been addressed, for example, by introducing a heterologous O2-tolerant [NiFe]-hydrogenase (Lupacchini et al., 2021). Another strategy enabling a continuous hydrogenase activity would be the spatial separation from O2, e.g., through the encapsulation in synthetic microcompartments as demonstrated in Escherichia coli (hereafter referred to as E. coli) (Li et al., 2020). Moreover, metabolic engineering might target the redirecting of electron flows from competing pathways, like respiration and nitrate assimilation, to H2 evolution (Baebprasert et al., 2011). Nevertheless, further research and alternative approaches are required to overcome the known as well as yet unknown limitations and to make photosynthesis-driven H2 production amenable for biotechnological applications in the future (Bühler et al., 2021). In this regard, biosensors that respond to H2 in an easily detectable way could help to enable, e.g., a systematic screening of mutant libraries and the selection of those that are beneficial for H2 production.
Synthetic biosensors based on engineered bacterial cells, that respond to certain input stimuli with a desired output signal, can be designed by harnessing natural signal transduction systems to drive the expression of a reporter gene (Zhang et al., 2015; Ni et al., 2021). Also cyanobacteria have already been used as hosts to implement such cascades, e.g., for the intracellular sensing of heavy metals (Lacey et al., 2019; Patyi et al., 2021), O2 (Immethun et al., 2016), or toluene (Inaba et al., 2018). Natural H2-responsive systems were described in Bradyrhizobium japonicum (B. japonicum) (Black et al., 1994; van Soom et al., 1997, 1999), Rhodobacter capsulatus (R. capsulatus) (Dischert et al., 1999; Elsen et al., 2003), and Cupriavidus necator (also known as Ralstonia eutropha) H16 (hereafter referred to as C. necator) (Lenz et al., 1997; Lenz and Friedrich, 1998). The purple non-sulfur bacterium R. capsulatus has already been engineered to follow H2 production in co-cultivated green algae (Wecker et al., 2011). However, such a co-cultivation approach impedes the use as a tool for efficient mutant screening and is not feasible in the case of cyanobacteria as most bacterial strains do not grow in cyanobacterial growth media. In the long-term, a cyanobacterial biosensor strain that directly responds to intracellularly evolved H2 appears promising to use it as platform for a systematic optimization of H2 production within the same cell.
Cupriavidus necator has become the model organism for H2 oxidation in presence of O2. As a true “Knallgas” bacterium it can utilize H2 as sole electron donor and O2 as terminal electron acceptor. For this purpose, it uses O2-tolerant [NiFe]-hydrogenases. C. necator contains even four [NiFe]-hydrogenases that are O2-tolerant, among them the soluble NAD+-reducing (SH) and membrane-bound hydrogenase (MBH) as well as the regulatory hydrogenase (RH) (Lenz et al., 2015). The RH is a cytoplasmic enzyme and has a comparably simple structure composed of the two hydrogenase subunits, HoxB and HoxC (Pierik et al., 1998; Kleihues et al., 2000; Bernhard et al., 2001). The catalytic Ni-Fe center is coordinated by four cysteines to the protein matrix of the HoxC subunit (Winter et al., 2004). The active site iron carries three diatomic ligands, two cyanides (CN−) and one carbon monoxide (CO) (Pierik et al., 1998). Incorporation of the active site into the RH requires a set of seven auxiliary maturases, which are encoded by the hypA1B1F1CDEX genes (Buhrke et al., 2001; Bürstel et al., 2016). The RH serves as H2 sensor in combination with a two-component regulatory system and transmits the H2 input signal via the histidine kinase HoxJ to the response regulator HoxA, which functions as transcriptional activator for hydrogenase gene expression (Zimmer et al., 1995; Lenz et al., 1997; Schwartz et al., 1998; Figure 1A). Unlike canonical two-component systems, transcriptional activation is mediated by the unphosphorylated form of HoxA, and HoxJ phosphorylates/inactivates HoxA in the absence of H2 (Lenz and Friedrich, 1998). Notably, the phosphorylating activity of HoxJ is knocked out in its parental stain C. necator H16. To restore the native activity of HoxJ, a specific amino acid exchange is required, resulting in a functional HoxJ(S422G), also referred to as HoxJ* (Lenz and Friedrich, 1998). HoxJ* and the RH form the ternary H2-sensing complex (Buhrke et al., 2004). Production of active RH has already been established in E. coli (Lenz et al., 2007), very recently even under aerobic conditions (Fan et al., 2022).
Figure 1. H2-dependent signal transduction in C. necator and its potential utilization for the design of a cyanobacterial H2 sensor strain that could be used as platform for the improvement of sustainable H2 production via oxygenic photosynthesis. (A) H2-responsive signal cascade in C. necator that enables the utilization of molecular hydrogen (H2) when other (favored) energy sources are absent. Signal transduction involves the regulatory hydrogenase (RH) module HoxBC as H2 sensor (with very low catalytic activity for H2 oxidation), the histidine kinase HoxJ and the response regulator HoxA, which promotes transcription of genes for two oxygen-tolerant hydrogenases [a soluble NAD+-reducing hydrogenase (SH) or a membrane-bound hydrogenase (MBH)]. These hydrogenases show high activity toward H2 oxidation and are used for energy-conservation. (B) Potential application of this H2-responsive signal cascade to drive reporter gene expression in cyanobacteria. This would enable optical detection of H2 that was produced by other hydrogenases using electrons obtained by light-driven water oxidation. This screening platform could facilitate strain engineering with diverse strategies, e.g., improving hydrogenase properties by (repeated) rational design or random mutagenesis, toward an improved photosynthetic H2 production. SH, soluble NAD+-reducing hydrogenase; RH, regulatory hydrogenase.
In this study, we introduced the H2-sensing module of the C. necator RH, i.e., HoxB and HoxC, into Synechocystis. For this purpose, synthetic operons for the structural and accessory genes were designed for expression in Synechocystis. Heterologously produced and catalytically active RH was extracted from photoautotrophically grown cells that continuously evolve O2. Furthermore, as proof of concept, we introduced functional HoxJ* and HoxA into E. coli to modulate the expression of a reporter gene fused to a HoxA-responsive heterologous promoter. Our study provides the basis for further engineering of a cyanobacterial H2 biosensor strain that might enable a systematic screening of genetic setups and the selection of those beneficial for H2 production by the host-specific or other introduced hydrogenases (Figure 1B).
Escherichia coli strains DH5α or JM109 were grown at 37°C either on agar-solidified LB medium or in LB liquid medium supplemented with 5 g L−1 NaCl under continuous shaking at 200 rpm. To select for the presence of certain plasmids the medium was supplemented with 100 μg mL−1 ampicillin, 35 μg mL−1 chloramphenicol, or 50 μg mL−1 spectinomycin. C. necator (obtained from the German Collection of Microorganisms and Cell Cultures, DSMZ) was grown at 37°C in LB liquid medium supplemented with 2.5 g L−1 NaCl under continuous shaking at 200 rpm. Synechocystis was cultivated in yBG11 (Shcolnick et al., 2007) liquid medium under continuous shaking at 150 rpm, or BG11 (Stanier et al., 1979) solidified with 1.5% (w/v) Bacto agar (Becton Dickinson) and supplemented with 3 g L−1 Na2S2O3. The cyanobacterial growth media were buffered with 10–50 mM HEPES to pH 7.2. Photoautotrophic growth conditions were set to 30°C, ambient CO2, constant light illumination with 50 μmol photons m−2 s−1, and 75% (v/v) humidity. For the selection of mutants, the media were supplemented with 10 μg mL−1 chloramphenicol, 20 μg mL−1 spectinomycin, or 50 μg mL−1 kanamycin. A non-motile, glucose tolerant strain of Synechocystis, originally received from Martin Hagemann (Rostock University, Germany), was used as the wild type (WT). The mutant Synechocystis(Δhox) that is devoid of the endogenous [NiFe]-hydrogenase was obtained from Kirsten Gutekunst (Kassel University, Germany). In particular, hoxEFUYH (sll1220-sll1226) have been replaced by a kanamycin resistance cassette (Appel et al., 2020).
In silico work was performed using the software Geneious (Biomatters). Genetic constructs were generated through standard molecular cloning procedures and maintained on plasmids in E. coli DH5α. DNA processing and recombination were performed using FastDigest restriction endonucleases (Thermo Scientific), T4 DNA ligase (Thermo Scientific), and FastAP thermosensitive alkaline phosphatase (Thermo Scientific) following the manufacturer’s instructions. The obtained constructs were verified by Sanger sequencing. Information about used oligonucleotides and plasmids is given in Supplementary Tables S1, S2.
The sequences for the design of synthetic operons encoding the H2-sensing complex and the corresponding maturases were obtained from the megaplasmid pHG1 of C. necator (Schwartz et al., 2003). The hoxJ sequence was modified to code for a variant exhibiting an amino acid substitution from serine to glycine at position 422, denoted as HoxJ* (Lenz and Friedrich, 1998). The hoxC sequence was altered to instead encode the variant HoxC(D15H) (Gebler et al., 2007). The gene sequences were codon-usage optimized for Synechocystis using the web-based tool JCat (Grote et al., 2005). The inducible promoters PrhaBAD (Behle et al., 2020) and PnrsB (Englund et al., 2016) were fused to the hox and hyp operons, respectively. The constructs were also equipped with unique restriction endonuclease sites flanking each operon, effective ribosome binding sites (RBS*, Heidorn et al., 2011) upstream of each ORF, as well as standardized transcription terminators (BioBrick BBa_B0015, Registry of Standard Biological Parts, 2003). Tag-encoding sequences were added to the 3′ ends of the open reading frames of hoxB (3xFLAG-tag), hoxJ* (3xFLAG-tag) and hypX (Strep-tag), respectively. The synthetic operons were chemically synthesized (Eurofins Genomics) and provided on plasmids, denoted as pHox2 and pHyp. For the maintenance in Synechocystis, the synthetic operons were transferred, either separately or combined, into the plasmid pSHDY_PrhaBAD::mVenus_PJ23119::rhaS (Behle et al., 2020) via XbaI/BcuI and BcuI/PstI sites, respectively. Thereby, the PrhaBAD::mVenus cassette was replaced. The resulting plasmids were named pHySe_Hox and pHySe_Hox_Hyp (Figure 2). The full sequences including annotations are provided in the Supplementary Data.
Figure 2. Scheme of synthetic operons for the expression of C. necator genes encoding the H2-sensing HoxBCJ* complex and its associated maturases in Synechocystis. (A) Structure of the plasmid pSHDY_PrhaBAD::mVenus_PJ23119::rhaS (herein referred to as pSHDY). It can be maintained in Synechocystis, contains a spectinomycin selection marker (SmR) for selection and enables L-rhamnose (Rha)-inducible reporter gene expression via PrhaBAD by featuring a cassette for the constitutive expression of rhaS (Behle et al., 2020). (B) Structure of the pHySe plasmids. The hox and hyp genes are organized in synthetic operons with a transcription terminator (Term.) at the 3′ ends. Their polycistronic transcription is driven by PrhaBAD and the nickel-ion (Ni2+)-responsive PnrsB (Englund et al., 2016), respectively. Each gene is interconnected by a ribosome binding site, RBS* (Heidorn et al., 2011). (C) Verification of recombinant Synechocystis strains. A Synechocystis parental strain lacking the endogenous hydrogenase was transformed using either pSHDY, pHySe_Hox, or pHySe_Hox_Hyp. Four selected clones (cl.1–4) were analyzed by colony PCR with primers that specifically targeted regions of the particular vector, i.e., S17 and S31 for pSHDY, S22 and S31 for pHySe_Hox, as well as S30 and S31 for pHySe_Hox_Hyp. The respective pure plasmid DNA served as positive control (pos. control). A PCR reaction lacking a template was used as negative control (neg. control).
Plasmids of the pFO series and derivatives of pSB1A2_Ptrc1O (Huang et al., 2010), all of which harbor various combinations of expression cassettes for the genes hoxA, hoxJ* and sfgfp, were generated via the Gibson assembly procedure (Gibson et al., 2009). For this, respective sequences were amplified via PCR using primers with 5′ extensions to create homologous overhangs for the desired assembly with other DNA fragments. The PSH promoter, including the 5′ untranslated upstream region of hoxF (Zimmer et al., 1995; Schwartz et al., 1998), was amplified from genomic DNA of C. necator using primer pair P17/P18. Together with the sfgfp reporter gene and a downstream BioBrick BBa_B0015 transcription terminator, which were generated through PCR with primers P16/Sam_102 from pSEVA351-sfgfp (Opel et al., 2022), it was used for the assembly of pFO6 utilizing KpnI-treated pSEVA351 (Martínez-García et al., 2020) as vector. The hoxA gene was amplified from gDNA of C. necator using P25 that additionally contained the ribosome binding site BioBrick BBa_B0034 (Registry of Standard Biological Parts, 2003) as 5′ extension and P26 fusing a sequence encoding a hexahistidine-tag at the 3′ end of the gene. The linear fragment was used for the assembly with BcuI-cut pSB1A2, yielding pSB1A2_Ptrc1O-hoxA. pSB1A2_Ptrc1O-hoxAD55A has a substitution at the codon coding for the amino acid at position 55 of HoxA from 5′-GAT-3′ (Asp) to 5’-GCC-3′ (Ala). It was generated with the DpnI-digested product from an inverted PCR, taking pSB1A2_Ptrc1O-hoxA as template and the primer pair P52/P53, as well as the homologous overhangs-supplying HoxA(D55A) double-stranded DNA fragment. The Ptrc1O-hoxA and Ptrc1O-hoxAD55A constructs were PCR-amplified using primers P49 and P50 from either pSB1A2_Ptrc1O-hoxA or pSB1A2_Ptrc1O-hoxAD55A, thereby fused to a 3’sequence encoding a Strep-tag II, instead of the His-tag, to be each inserted into BcuI-linearized pSEVA351, yielding pFO25 and pFO26, respectively. Analogously, these two synthetic gene constructs were inserted into BcuI-cut pFO6, which resulted in pFO27 and pFO28, respectively. To obtain pFO45 and pFO46, pHox2 was first subjected to an inverted PCR using P83 and P84, thereby deleting hoxBFLAG and hoxCD15H as well as the particular upstream situated RBS*. This was followed by AQUA cloning, creating pHox5 that encodes the PrhaBAD::hoxJ*FLAG cassette. The latter was excised by restriction with XbaI and inserted into XbaI-linearized pFO27 and pFO28, yielding pFO45 and pFO46, respectively. Sequences of the pFO series are provided in the Supplementary Data.
Synechocystis WT as well as Synechocystis(Δhox) parental cells were made electro-competent and transformed via electroporation as described previously (Brandenburg et al., 2021). Plasmid-harboring strains were selected on BG11 agar plates containing appropriate antibiotics. Plasmid presence was verified by colony PCR using suitable primers and the GoTaq MasterMix (Promega) according to the manufacturer’s instructions. Recombinant E. coli JM109 strains were generated by electroporation of electro-competent cells via standard procedures.
For the isolation of RNA, Synechocystis cells were grown until reaching an OD750 ~ 0.8. The cultures were subsequently supplemented with final concentrations of 0.1% (w/v) L-rhamnose and 5 μM NiSO4. After 24 h, cells were harvested by rapid vacuum filtration applying sterilized polyether sulfone filters (pore size 0.8 μm, PALL). RNA isolation was performed as described previously (Bolay et al., 2022). The RNA samples were treated with RNase-free DNase I (Thermo Scientific) according to the manufacturer’s instructions. Afterwards, cDNA was generated by applying the high-capacity cDNA reverse transcription kit (Thermo Scientific) as given in the manufacturer’s instructions. A total of ~0.4 ng cDNA were used as template for quantitative PCR. Amplification of specific regions within either the rnpB gene or hypX were performed using the GoTaq MasterMix (Promega) according to the manufacturer’s instructions and primer pairs rnpB_114F/rnpB_226R and S30/P88, respectively (Supplementary Table S1).
Synechocystis cells were grown in presence of elevated CO2 concentration of 2% (v/v) to an OD750 of ~2. To induce expression of the hox and hyp genes the medium was supplemented with final concentrations (f.c.) of 0.2% (w/v) L-rhamnose and 5 μM NiSO4. In addition, 17 μM (f.c.) ferric ammonium citrate was added to foster hydrogenase maturation similar to previous reports (Lupacchini et al., 2021). Samples were collected by centrifugation after 24 and 48 h. Cells were resuspended in 750 μL TBS lysis buffer (100 mM Tris, 150 mM NaCl, 1 mM PMSF, pH 7.5) and transferred to 2 mL Precellys tubes (Bertin), together with a mixture of glass beads (Sartorius) of 0.09–0.15, 0.17–0.18, and 0.5 mm diameter. Cell disruption was performed using a Precellys Evolution homogenizer (Bertin) equipped with a Cryolys cooling system (Bertin) for 4 × 30 s at 10.000 rpm with 30 s interim breaks for cooling. The samples were subsequently separated in supernatant (soluble extract) and sediment (crude extract) by centrifugation and subjected to protein concentration determination using a Bradford dye reagent ready-to-use solution (Thermo Scientific) according to the manufacturer’s instructions. Cell suspensions of recombinant E. coli JM109 strains were analogously treated to obtain soluble protein extracts. Those cells were beforehand cultivated as described for GFP fluorescence determination, but using 0.5% (w/v) D-glucose instead of glycerol. Protein separation was performed via SDS-PAGE using a total amount of 20 μg protein for each sample. For immunodetection via Western blots the separated proteins were transferred to nitrocellulose membranes of 0.45 μm pore size (GVS), followed by hybridization with either a Strep-Tactin horse radish peroxidase (HRP) (IBA Lifesciences GmbH) or a monoclonal ANTI-FLAG M2-Peroxidase conjugate (Sigma-Aldrich) according to the manufacturer’s instructions. Chemiluminescence was detected by using the substrate solutions WesternSure PREMIUM Chemiluminescent (LI-COR) or WesterBright ECL (advansta) and the Fusion FX7 EDGE V0.7 imaging system (VILBER), following the manufacturer’s instructions.
Pre-cultivation of Synechocystis was performed as described above and expression of heterologous genes was induced by 0.1% (w/v) L-rhamnose and/or 2.5 μM NiSO4. After 48 h, cells were harvested and disrupted analogously but using an alternative lysis buffer (5% (v/v) glycerol, 50 mM KPO4, 1 mM PMSF, pH 8). A Synechocystis strain harboring the hoxFUYHW genes encoding the SH from C. necator (Lupacchini et al., 2021) served as control. Approximately 400 μg of soluble proteins were separated via native PAGE and subjected to in-gel staining as previously described (Lupacchini et al., 2021) with few modifications. These concerned the supplementation of 90 μM phenazine methosulfate, additionally to 800 μM NAD+ and 60 μM nitro blue tetrazolium (NBT) in an H2-saturated activity buffer (50 mM Tris, pH 8). Furthermore, the incubation time was increased from ~0.5 h to ~2.5 h. Hydrogenase activity, i.e., the release of electrons from H2 oxidation, is indicated via a step-wise reduction of the electron transfer mediators NAD+ and/or phenazine methosulfate and the colorimetric dye nitro blue tetrazolium, which finally results in a visible precipitation of formazan (Ponti et al., 1978). The gels were subsequently decolorized from the remaining loading dye in activity buffer overnight. Afterwards, presence of HoxB and HoxJ* proteins was confirmed by blotting the same polyacrylamide gel and hybridizing the membrane with antibodies against the attached 3xFLAG-tag as described above.
Recombinant Synechocystis strains containing plasmids pFO25 (negative control), pFO6 (PSH::sfgfp), pFO27 (PSH::sfgfp + Ptrc1O::hoxA), or pFO28 (PSH::sfgfp + Ptrc1O::hoxAD55A) were analyzed in vivo regarding GFP fluorescence. The detection was performed as described previously (Opel et al., 2022). GFP fluorescence determination in E. coli JM109 was conducted for recombinant strains harboring the following plasmids: pSEVA351 (negative control), pFO27 (PSH::sfgfp + Ptrc1O::hoxA), pFO45 (PSH::sfgfp + Ptrc1O::hoxA + PrhaBAD::hoxJ*), and pFO46 (PSH::sfgfp + Ptrc1O:: hoxAD55A + PrhaBAD::hoxJ*). Single colonies from selective LB agar plates were picked to inoculate liquid LB medium pre-cultures that were grown for ~18 h at 37°C. 1% (v/v) of these suspensions were taken to inoculate second pre-cultures using M9* medium, supplemented with 0.001% (w/v) thiamine, 2 mM MgSO4, 0.4% (v/v) glycerol, US* trace elements solution, and buffered to pH 7.2. The M9* pre-cultures were incubated for ~24 h at 37°C. A volume of 100 μL of these cell suspensions were added to 10 mL M9* medium in baffled shake flasks, and the cells were further cultivated at 30°C instead, due to the temperature sensitivity of HoxA (Zimmer et al., 1995). These main cultures were supplemented with 10 μM IPTG and/or 0.2% L-rhamnose after 8 h, followed by another 16 h of cultivation. For GFP fluorescence determination, samples were diluted to an OD600 of ~0.5 with TBS buffer (100 mM Tris, 150 mM NaCl, pH 7.5) in a final volume of 1,200 μL. Technical triplicates (each 200 μL) were transferred into an opaque black flat microtiter 96-well-plate (Nunc), followed by fluorescence measurements using an Infinite 200 PRO microplate reader (Tecan; gain: 123, integration time: 20 μs, excitation bandwidth: 9 nm, emission bandwidth: 20 nm, z-position: 2000 μm, 25 flashes) and excitation/emission wavelengths of 485 nm/520 nm, respectively. Furthermore, the same sample was used to measure the absorption at 600 nm in a transparent flat microtiter 96-well-plate (Nunc), also using the Infinite 200 PRO microplate reader (bandwidth: 9 nm, 25 flashes). The blank of the TBS buffer background was subtracted from the values detected for the cell suspensions. The fluorescence intensities were finally normalized by division of respective OD600 values.
To functionally produce the RH of C. necator in Synechocystis, we rationally designed two operons in silico that were generated via chemical synthesis and inserted it into a vector that can be maintained in Synechocystis (Figures 2A,B). In case of the active site-containing HoxC subunit, we made use of the amino acid exchange variant HoxC(D15H), which, in contrast to the native protein, supports H2-dependent growth of C. necator at an O2 level of up to 10% (Gebler et al., 2007). The resulting gene cluster for the biosynthesis of the H2-sensing complex comprised hoxB, hoxCD15H, and hoxJ*. For proper maturation of the catalytic [NiFe] center we also utilized the corresponding accessory genes hypA1B1F1CDEFX (Buhrke et al., 2001; Bürstel et al., 2016). In C. necator, these genes are organized in an operon structure with partially overlapping open reading frames (Schwartz et al., 2003). To ensure their correct expression in the cyanobacterial target organism, we altered the spatial organization by linking each hox and hyp gene by an artificial spacer region, resulting in separate translational units (Figure 2B). Furthermore, the synthetic ribosome binding site RBS* which functions in Synechocystis (Heidorn et al., 2011), was introduced upstream of every single gene to enable efficient translation initiation. For facile detection of the proteins, sequences encoding either a 3xFLAG-tag or a Strep-tag were fused to hoxB, hoxJ*, and hypX (Figure 2B). The hoxJ* and hypX genes were chosen because they are the dorsal genes of each particular operon. Detection of both proteins is considered representative of upstream gene expression.
All protein-coding sequences were codon-usage optimized for translation in Synechocystis. To drive the polycistronic transcription of hox and hyp gene clusters, we used the L-rhamnose-inducible promoter PrhaBAD from E. coli (Behle et al., 2020) and the nickel ion (Ni2+)-dependent promoter PnrsB of Synechocystis (Englund et al., 2016), respectively. Thus, this setup permits a selective induction as well as a tight and tunable transcription of the synthetic gene constructs PrhaBAD::hoxBFLAGCD15HJ*FLAG (hox operon) and PnrsB::hypA1B1F1CDEFXStrep (hyp operon) in Synechocystis. Moreover, both operons were equipped with insulating transcription terminators at their 3′ end.
The constructs were inserted into the replicative vector pSHDY_PrhaBAD::mVenus _PJ23119-rhaS (hereafter referred to as pSHDY), which encodes the heterologous transcriptional regulator RhaS that enables rhamnose-inducible gene expression in Synechocystis (Behle et al., 2020). The PrhaBAD::mVenus cassette present in this pSHDY construct (Figure 2A) was replaced with the synthetic hox operon resulting in the plasmid pHySe_Hox. Subsequently, the hyp operon was inserted downstream to obtain the plasmid pHySe_Hox_Hyp (Figure 2B). The resulting plasmids pHySe_Hox and pHySe_Hox_Hyp, as well as the precursor construct pSHDY, were used individually for the transformation of Synechocystis. For transformation, a strain devoid of the endogenous [NiFe]-hydrogenase, designated Synechocystis(Δhox) (Appel et al., 2020), was used to prevent subsequent cross-reactions with the RH activity. Plasmid presence was verified in all obtained clones (Figure 2C).
C-terminal linkage with 3x-FLAG (HoxB & HoxJ*) or Strep-tags (HypX), enabled protein detection by commercially available antibodies targeting the corresponding tag. The Synechocystis strain harboring pHySe_Hox_Hyp was grown in the presence of L-rhamnose and Ni2+ to trigger hox and hyp gene expression, respectively (see section “Materials and methods” for specific inducer concentrations). To confirm heterologous gene expression, we performed immunoblotting to detect HoxB and HoxJ* using protein extracts of samples collected 24 and 48 h after induction. In addition to the crude extract, we also analyzed the soluble protein fraction obtained by centrifugation. In fact, distinct bands were detected, and their intensity increased according to the induction time. The bands represent HoxB-FLAG and HoxJ*-FLAG, as no signal was detected in the same size range in the Synechocystis wild-type strain (WT) (Figure 3). Consistent with the expected cytoplasmic localization of the RH, a strong signal for HoxB-FLAG was observed in the soluble extracts. However, the signal associated with HoxJ*-FLAG, which is also thought to be soluble, was predominantly present in the crude extract indicating partial protein misfolding or membrane association. Nevertheless, even though the signal was quite weak, a significant part was also found in the soluble fraction, in particular 48 h after induction. Thus, both fusion proteins were specifically detected, confirming the expression of the corresponding genes.
Figure 3. HoxJ* and HoxB from C. necator are synthesized in Synechocystis. Western blot for the detection of HoxJ*-FLAG and (~54 kDa) HoxB-FLAG (~40 kDa) fusion proteins. Samples were taken from Synechocystis(Δhox) that harbored the plasmid pHySe_Hox_Hyp, 24 and 48 h after induction with 0.2% (w/v) L-rhamnose and 5 μM NiSO4. Protein extract of a Synechocystis wild type (WT) strain served as negative control. CE, crude extract; SE, soluble extract.
In case of HypX-Strep, however, no specific signal was observed in cells containing pHySe_Hox_Hyp (not shown), indicating either no translation or protein instability. To verify that the synthetic hyp operon is at least transcribed, we extracted total RNA from the same strain and performed classical reverse transcriptase (RT)-PCR targeting hypX. The reversely transcribed copy DNA (cDNA) for hypX was only detected in the Synechocystis strain containing pHySe_Hox_Hyp but not in the WT (Figure 4). That the band obtained is indeed a result of cDNA amplification of a hypX transcript was verified by a parallel RNA sample that was not treated with reverse transcriptase and consequently showed no bands for hypX and the housekeeping gene rnpB. As hypX is situated at the 3′ end of the synthetic gene cluster, we assume, that transcription of the upstream situated hyp genes also occurred. However, this analysis did not confirm the translation of the hyp gene transcripts. Nevertheless, sufficient synthesis of the maturation apparatus for the RH could be assumed, as indicated by the subsequent analysis (see below).
Figure 4. The polycistronic hyp mRNA is being transcribed in Synechocystis. PCR amplification of hypX or the housekeeping gene rnpB from cDNA that has been prepared from RNA isolates of Synechocystis wild type (control) or a strain harboring pHySe_Hox_Hyp. Samples in lanes denoted ‘RT +’ were treated with reverse transcriptase beforehand, while those indicated ‘RT −’ were not.
To investigate if the recombinant gene expression indeed results in the formation of active H2-sensing RH, we analyzed the H2 oxidation activity in soluble extracts of photoautotrophically grown cells of Synechocystis strains harboring the plasmids pSHDY, pHySe_Hox, and pHySe_Hox_Hyp. To this end, we used an in-gel activity assay under an H2 atmosphere (Buhrke et al., 2004), which has also been used recently to confirm the activity of hydrogenases in Synechocystis (Lupacchini et al., 2021). The recombinant strains were cultivated in the presence of different inducer combinations to achieve independent expression of the hoxBCJ* genes (L-rhamnose) and the hypA1B1F1CDEX operon (Ni2+). Soluble protein extracts were prepared and subjected to native polyacrylamide gel electrophoresis. Strikingly, in-gel H2 oxidation activity was detected only for Synechocystis(pHySe_Hox_Hyp) induced with both L-rhamnose and Ni2+ (Figure 5A, In-gel staining panel). The resulting activity bands were located at the same positions as the bands in the immunoblot analysis (also based on the native gel), showing HoxB-FLAG in complex with HoxC as the hydrogenase core module, and potentially HoxJ*-FLAG (Figure 5A, Western Blot panel). As the production of both HoxB-FLAG and HoxJ*-FLAG has been confirmed by a previous Western blot (Figure 3), a separate detection of both proteins has not been performed in this case. No H2 oxidation activity was detected in the corresponding native gel, when the Hyp proteins required to produce catalytically active RH were absent, either due to the lack of the hyp genes (in case of strain pHySe_Hox) or the inducer Ni2+ (in case of strain pHySe_Hox_Hyp) (Figure 5A). Thus, expression of both the hox and hyp gene clusters is required to obtain detectable H2 oxidation activity for the H2-sensing RH in Synechocystis. While most [NiFe]-hydrogenases are inactivated by traces of O2 (Shafaat et al., 2013), the RH activity has shown to be O2-tolerant (Ash et al., 2015). Remarkably, the RH activity was observed in extracts of O2-evolving photoautotrophically grown Synechocystis cells, indicating that both the maturation process and the catalytic activity of the RH occurred in the presence of O2.
Figure 5. RH-mediated H2 oxidation in Synechocystis. (A) Recombinant Synechocystis(Δhox) strains lacking the endogenous hydrogenase and containing either pSHDY, pHySe_Hox, or pHySe_Hox_Hyp were grown photoautotrophically under aerobic conditions in the presence of the inducers, i.e., 0.1% (w/v) L-rhamnose (Rha) and/or 2.5 μM Ni2+ (indicated by +/−). Soluble proteins were separated via native PAGE and subjected to in-gel H2-dependent formazan staining to localize the H2-sensing complex (upper panel), as well as immunological detection of the HoxB-FLAG and HoxJ*-FLAG proteins (middle panel). A denaturing SDS-PAGE was conducted as loading control (lower panel). (B) In-gel activity of cells incubated with the given inducers using different amounts of Rha in % (w/v). A Synechocystis strain recombinantly producing the highly active NAD+-reducing soluble hydrogenase from C. necator (SH) served as positive control.
The RH activity in Synechocystis is comparatively low, as demonstrated by the long incubation time of ~2.5 h required to obtain detectable bands derived from H2-dependent NBT reduction in the activity gel. A Synechocystis control strain containing the highly active SH from C. necator (Lupacchini et al., 2021) showed significantly stronger signal intensities after ~0.5 h already. The low signal strength of the RH in Synechocystis could not be increased by enhanced L-rhamnose levels, suggesting a saturation at 0.05% (w/v) rhamnose and consequently no limitation of the RH structural proteins (Figure 5B). Altogether, these results demonstrate for the first time the functional production of a recombinant regulatory hydrogenase with low H2 turnover activity in a cyanobacterium.
The final goal is to couple the functional RH with the associated kinase HoxJ* and the cognate response regulator HoxA to establish an H2-sensing signal transduction cascade in Synechocystis. In C. necator, HoxA positively controls the transcription of the genes encoding the SH and the MBH through binding to the promoters PSH and PMBH, respectively (Zimmer et al., 1995; Schwartz et al., 1998). The HoxA activity is modulated by HoxJ*-mediated phosphorylation, with transcriptional activation by HoxA in its non-phosphorylated state (Lenz and Friedrich, 1998). To drive HoxA-mediated gene expression in Synechocystis, the hoxA gene was set under control of the IPTG-inducible Ptrc1O promoter, which has been shown to provide sufficient constitutive expression due to the lack of the lac repressor LacI in the cyanobacterial host (Huang et al., 2010). Furthermore, a sfgfp reporter gene encoding the superfolder green fluorescent protein (Pédelacq et al., 2006) (hereafter referred to as GFP) was fused to the HoxA-dependent promoter PSH (Figure 6A). The synthetic gene constructs PSH::sfgfp and Ptrc1O::hoxA were introduced into Synechocystis WT, either separately or combined on a replicative plasmid. However, no significant GFP fluorescence beyond background activity was detected in any strain carrying the reporter gene construct alone or in combination with Ptrc1O::hoxA (Figure 6B). An inactivation of HoxA by unspecific phosphorylation could be excluded because the GFP fluorescence was similar in a reporter strain containing the phosphorylation-insensitive variant HoxA(D55A) instead of HoxA. This variant cannot be phosphorylated at the crucial aspartate at position 55 and has been shown to be always active (Lenz and Friedrich, 1998). Although several attempts have been made to improve hoxA expression, e.g., by using different promoters as well as codon-usage optimized gene variants, HoxA-dependent sfgfp expression has not yet been achieved in Synechocystis (not shown).
Figure 6. GFP fluorescence of E. coli and Synechocystis representing transcriptional regulation via HoxA and its modulation by HoxJ*. (A) Genetic setup of the generated strains. Expression of hoxA was driven by the IPTG-dependent lac promoter derivative Ptrc1O (Huang et al., 2010). The expression of the hoxJ* gene, fused to a FLAG-tag encoding sequence, was performed using the L-rhamnose (Rha)-inducible promoter PrhaBAD (Behle et al., 2020). HoxA-dependent expression of a reporter was achieved by fusion of a sfgfp gene to the cognate promoter of the NAD+-reducing, soluble hydrogenase gene cluster of C. necator, PSH (Zimmer et al., 1995; Schwartz et al., 1998). HoxJ* in turn modulates HoxA-dependent transcriptional regulation (Lenz and Friedrich, 1998). (B,C,E) Whole-cell GFP fluorescence of recombinant Synechocystis or E. coli JM109 strains harboring the synthetic PSH::sfgfp reporter gene construct in combination with different genetic information shown in panel A. Treatment with inducers IPTG and/or Rha prior to the measurements is indicated by ‘+.’ Detected fluorescence was normalized to OD750 (panel B) or OD600 (panels C and E) and is given in arbitrary units (a.u.). The particular negative controls (neg. control) contained a plasmid without reporter gene cassette. Data are the mean ± SD of at least two biological replicates (clones) each measured in technical triplicates. (D) Western blot confirming the presence of HoxJ*-FLAG (~54 kDa) in soluble protein extracts of E. coli JM109 harboring a PrhaBAD::hoxJ*FLAG cassette versus an empty vector neg. control.
However, to validate the general functionality of our genetic constructs and to establish HoxA and HoxJ*-dependent gene expression in a heterologous system, we introduced the same replicative plasmids harboring Ptrc1O::hoxA and PSH::sfgfp into E. coli JM109. As this host contains LacI, hoxA expression is IPTG-inducible. Indeed, a ~ 5-fold higher GFP fluorescence was detected in cells grow in presence of IPTG compared to cells grown in absence of IPTG (Figure 6C). Under non-induced conditions, the GFP fluorescence was similar to the background autofluorescence of control cells harboring the empty vectors. Thus, in contrast to Synechocystis, the expected activity of HoxA to promote reporter gene expression via the PSH promoter was confirmed in E. coli.
Moreover, another synthetic gene construct encoding HoxJ* was included in the study to demonstrate the modulation of HoxA activity. HoxJ*-mediated phosphorylation of HoxA is expected to inactivate the response regulator (Lenz and Friedrich, 1998; Buhrke et al., 2004). A gene encoding HoxJ* carrying a C-terminal FLAG-tag (HoxJ*FLAG) was set under control of the L-rhamnose-inducible promoter PrhaBAD, and the resulting plasmid was introduced into the strain already harboring the Ptrc1O::hoxA construct. In general, this promoter could also be used in Synechocystis (Behle et al., 2020). Again, IPTG-induced hoxA gene expression resulted in GFP fluorescence. Remarkably, a significant decrease in GFP fluorescence was observed in cells that were grown in presence of both IPTG and L-rhamnose (Figure 6C). This correlates well with the exclusive detection of HoxJ* in protein extracts of cells grown in presence of L-rhamnose and carrying the respective gene construct (Figure 6D). The GFP level decreased to 50% compared to values obtained with strains either lacking the hoxJ* gene or that do not sufficiently express hoxJ* due to the absence of L-rhamnose. HoxA(D55A), which cannot be inactivated by HoxJ* through phosphorylation (Lenz and Friedrich, 1998), was again included as a control. As expected, a higher reporter signal was obtained with the corresponding strain in the presence of both IPTG an L-rhamnose than with the strain expressing wild-type hoxA as well as hoxJ* (Figure 6C). This result also suggests that the response regulator in E. coli JM109 is not inactivated by unspecific phosphorylation. Thus, for HoxA, an effective ~30% reduction in reporter signal strength was achieved by HoxJ* compared to HoxA(D55A), demonstrating the desired modulation. Moreover, the HoxJ*-mediated decrease of HoxA-dependent GFP fluorescence was further tunable by different amounts of L-rhamnose (Figure 6E).
H2-based signal transduction cascades are considered being widespread, as the corresponding genes have been detected in many available genomes and metagenomes (Greening et al., 2016). Nevertheless, the biochemical and molecular mechanisms of H2 sensing have been studied in only a few representative bacteria to date. Ever since, these systems have been engineered and coupled with reporters. For example, C. necator has been engineered regarding a HoxBCJA-dependent expression of lacZ encoding β-galactosidase as reporter (Lenz and Friedrich, 1998). In principle, such recombinant strains could be utilized for the detection of H2 synthesized by other microbes, e.g., by co-cultivation or agar overlay assays on petridish basis (Wecker and Ghirardi, 2014). Similar to C. necator, the H2-sensing system of R. capsulatus consists of four proteins: HupUV which form the H2-sensing hydrogenase, the histidine kinase HupT, and the transcriptional regulator HupR that finally activates expression of an energy-generating uptake hydrogenase (Dischert et al., 1999; Elsen et al., 2003). This system has also been developed into a biosensor to screen large libraries of H2-producing nitrogenase variants in R. capsulatus directly (Barahona et al., 2016). Moreover, the corresponding strain has also been used as whole-cell biosensor to track H2 production in co-cultivated green algae (Wecker et al., 2011). However, in the case of cyanobacteria, such a co-cultivation approach is not feasible since most bacterial strains do not grow in cyanobacterial growth media lacking an organic carbon source.
In order to monitor H2 evolution within cyanobacterial cells, however, a signal transduction cascade must be transferred to the corresponding strain. This would allow in vivo screening of H2 evolution and the optimization of strains carrying, e.g., alternative hydrogenases (Figure 1). For example, Wecker et al. used engineered R. capsulatus strains that monitor H2 by reporter fluorescence to track the activity of a recombinant H2-evolving hydrogenase from Clostridium acetobutylicum. Only low H2 production has been detected, but the system potentially enables further screening and hydrogenase evolution approaches (Wecker et al., 2017). We successfully implemented two parts of the four-part H2-responsive signal transduction cascade from C. necator in the cyanobacterium Synechocystis. This is considered as a first step toward a synthetic cyanobacterial H2 biosensor that could be used analogously to previous reports (Wecker et al., 2017). A potential application would be, for instance, to optimize the H2 evolution activities of heterologously produced O2-tolerant, energy-converting hydrogenases, one of which was successfully implemented in Synechocystis recently (Lupacchini et al., 2021).
According to the current model, H2-sensing requires continuous H2 activation, i.e., H2 binding, H2 cleavage, as well as the corresponding proton and electron transfer (Lenz et al., 2015). Protein–protein complex formation with HoxJ* is if course also required (Buhrke et al., 2004; Löscher et al., 2010). Thus, continuous H2 oxidation is a prerequisite for H2-sensing. While the H2 oxidation activity of RH itself has been shown to be O2 insensitive (Buhrke et al., 2005), the signal transduction process is sensitive to high O2 levels. In this study, we therefore used a variant of the RH large subunit with an amino acid exchange near the active site, HoxC(D15H) (Gebler et al., 2007). The turnover rate of the native RH is almost two orders of magnitude lower than that of energy-conserving standard [NiFe]-hydrogenases (Bernhard et al., 2001). The H2 oxidation activity of the HoxBC(D15H) variant is indeed another two orders of magnitude lower, which explains the weak signals observed in the in-gel activity assay (Figure 5). However, it mediated native-like H2 signal transduction in vivo and supported H2-dependent growth of C. necator even at 10% O2, where signal transduction by the native RH was shown to be impaired (Gebler et al., 2007). The active-site containing HoxC subunit of RH also lacks a C-terminal extension that is typical for standard [NiFe]-hydrogenases and is proteolytically cleaved after insertion of the catalytic center (Kleihues et al., 2000). The comparatively simple structure, the lack of need for proteolytic quality control, the low H2 consumption, and of course the O2-tolerant H2-sensing ability make the RH an ideal candidate for a synthetic cyanobacterial H2 biosensor.
Here, we demonstrated that the recombinant RH in Synechocystis is synthesized in a catalytically active form, as evidenced by the H2 oxidation activity detected in protein extracts. Moreover, the cell extracts were obtained from photoautotrophically grown, O2-evolving cells. This shows that both the biocatalyst RH and its maturation machinery function properly under aerobic conditions in Synechocystis. In addition to the structural hox genes, the co-expression of the accessory hypA1B1F1CDEX genes of C. necator was required to achieve active RH. Synechocystis contains six endogenous Hyp proteins, likewise denoted HypA-F, that are responsible for the maturation of the bidirectional [NiFe]-hydrogenase of this organism (Hoffmann et al., 2006). However, Synechocystis lacks a homolog of the C. necator HypX. Based on our results, we cannot conclude whether all Hyp components or rather a reduced set from C. necator are necessary to achieve H2 oxidation activity of the RH in Synechocystis. The Synechocystis Hyp proteins may at least partially compensate for the maturation of O2-tolerant [NiFe]-hydrogenases from C. necator in the absence of the corresponding heterologous assembly apparatus. Notably, the NAD+-reducing [NiFe]-hydrogenase from C. necator was functionally produced in Synechocystis, without co-expression of the associated hyp genes (Lupacchini et al., 2021). However, it has been suggested that the C. necator Hyp proteins may be required for full SH activity as they have an amino acid sequence identity with the Synechocystis homologs of only 50–67%. Furthermore, HypX is required for aerobic maturation of the [NiFe]-hydrogenases of C. necator (Bürstel et al., 2016; Schulz et al., 2020).
The transfer of signal-responsive components into heterologous hosts usually includes a promoter and the associated transcriptional regulator (Fernandez-López et al., 2015; Sonntag et al., 2020; Ni et al., 2021). Our objective was to transfer the H2-sensing module (HoxBC) and the associated two-component regulatory system (HoxJ* and HoxA) to a cyanobacterial species to establish an H2-dependent transcriptional response. This has not yet been achieved in Synechocystis, presumably due to the absence of the minor sigma factor σ54 in cyanobacteria (Riaz-Bradley, 2019), which is required for the transcriptional activation of PSH and PMBH in C. necator (Zimmer et al., 1995; Schwartz et al., 1998). In addition, the DNA-bending integration host factor (IHF) may participate in hydrogenase promotor activation in C. necator (Zimmer et al., 1995; Schwartz et al., 1998). Accordingly, the introduction of this heterologous sigma factor or promoter engineering in Synechocystis should be pursued, which was beyond the scope of this study. However, as a proof of principle, we introduced HoxA and HoxJ* into E. coli to demonstrate the transcriptional regulation of a sfgfp reporter gene fused to PSH. Specific HoxA-dependent GFP fluorescence was detected, likely related to the presence of σ54 in E. coli (Jones et al., 1994). Our data are consistent with previous findings on functional, HoxA-controlled expression of a reporter gene fused to PSH in E. coli (Schwartz et al., 1998). Moreover, as expected, the kinase activity of HoxJ* clearly modulated the HoxA-dependent GFP fluorescence in E. coli, leading to a decreased reporter signal. Overall, we now have all genetic elements in hand to eventually assemble a functional H2 biosensor with optical readout in a cyanobacterium. This could ultimately be used to monitor cyanobacterial H2 production, e.g., to enable evolutionary or high-throughput screening approaches to improve hydrogenase properties as well as to circumvent existing constraints.
The original contributions presented in the study are included in the article/Supplementary material, further inquiries can be directed to the corresponding author.
SK designed the study. FO, MAI, and IW constructed the plasmids. FO and IW performed the gene expression and RH activity analyses in Synechocystis. FO performed the GFP reporter assays. SL contributed to RH activity determination and experimental expertise for the in-gel assays. LL and OL contributed methodology and know-how on O2-tolerant hydrogenases. FO and SK wrote the manuscript with contributions from all co-authors. All authors contributed to the article and approved the submitted version.
This project was initiated by a grant of the Max-Buchner Foundation to SK (MBFSt-Kennziffer: 3714). We acknowledge the use of the facilities of the Centre for Biocatalysis (MiKat) at the Helmholtz Centre for Environmental Research, which is supported by European Regional Development Funds (EFRE, Europe funds Saxony). We also acknowledge the use of the facilities of H2Saxony. This project (Nr. 100361842) is financed from funds of the European Regional Development Fund (EFRE) and co-financed by means of taxation based on the budget adopted by the representatives of the Landtag of Saxony.
The authors thank Jens Appel (University of Kassel, Germany) for the fruitful discussions at the beginning of the project.
The authors declare that the research was conducted in the absence of any commercial or financial relationships that could be construed as a potential conflict of interest.
All claims expressed in this article are solely those of the authors and do not necessarily represent those of their affiliated organizations, or those of the publisher, the editors and the reviewers. Any product that may be evaluated in this article, or claim that may be made by its manufacturer, is not guaranteed or endorsed by the publisher.
The Supplementary material for this article can be found online at: https://www.frontiersin.org/articles/10.3389/fmicb.2023.1122078/full#supplementary-material
Appel, J., Hueren, V., Boehm, M., and Gutekunst, K. (2020). Cyanobacterial in vivo solar hydrogen production using a photosystem I–hydrogenase (PsaD-HoxYH) fusion complex. Nat. Energy 5, 458–467. doi: 10.1038/s41560-020-0609-6
Ash, P. A., Liu, J., Coutard, N., Heidary, N., Horch, M., Gudim, I., et al. (2015). Electrochemical and infrared spectroscopic studies provide insight into reactions of the NiFe regulatory hydrogenase from Ralstonia eutropha with O2 and CO. J. Phys. Chem. B 119, 13807–13815. doi: 10.1021/acs.jpcb.5b04164
Baebprasert, W., Jantaro, S., Khetkorn, W., Lindblad, P., and Incharoensakdi, A. (2011). Increased H2 production in the cyanobacterium Synechocystis sp. strain PCC 6803 by redirecting the electron supply via genetic engineering of the nitrate assimilation pathway. Metab. Eng. 13, 610–616. doi: 10.1016/j.ymben.2011.07.004
Barahona, E., Jiménez-Vicente, E., and Rubio, L. M. (2016). Hydrogen overproducing nitrogenases obtained by random mutagenesis and high-throughput screening. Sci. Rep. 6:38291. doi: 10.1038/srep38291
Behle, A., Saake, P., Germann, A. T., Dienst, D., and Axmann, I. M. (2020). Comparative dose-response analysis of inducible promoters in cyanobacteria. ACS Synth. Biol. 9, 843–855. doi: 10.1021/acssynbio.9b00505
Bernhard, M., Buhrke, T., Bleijlevens, B., de Lacey, A. L., Fernandez, V. M., Albracht, S. P., et al. (2001). The H(2) sensor of Ralstonia eutropha. Biochemical characteristics, spectroscopic properties, and its interaction with a histidine protein kinase. J. Biol. Chem. 276, 15592–15597. doi: 10.1074/jbc.M009802200
Berto, P., D'Adamo, S., Bergantino, E., Vallese, F., Giacometti, G. M., and Costantini, P. (2011). The cyanobacterium Synechocystis sp. PCC 6803 is able to express an active FeFe-hydrogenase without additional maturation proteins. Biochem. Biophys. Res. Commun. 405, 678–683. doi: 10.1016/j.bbrc.2011.01.095
Black, L. K., Fu, C., and Maier, R. J. (1994). Sequences and characterization of hupU and hupV genes of Bradyrhizobium japonicum encoding a possible nickel-sensing complex involved in hydrogenase expression. J. Bacteriol. 176, 7102–7106. doi: 10.1128/jb.176.22.7102-7106.1994
Bolay, P., Schlüter, S., Grimm, S., Riediger, M., Hess, W. R., and Klähn, S. (2022). The transcriptional regulator RbcR controls ribulose-1,5-bisphosphate carboxylase/oxygenase (RuBisCO) genes in the cyanobacterium Synechocystis sp. PCC 6803. New Phytol. 235, 432–445. doi: 10.1111/nph.18139
Brandenburg, F., Theodosiou, E., Bertelmann, C., Grund, M., Klähn, S., Schmid, A., et al. (2021). Trans-4-hydroxy-L-proline production by the cyanobacterium Synechocystis sp. PCC 6803. Metab. Eng. Commun. 12:e00155. doi: 10.1016/j.mec.2020.e00155
Bühler, K., Bühler, B., Klähn, S., Krömer, J. O., Dusny, C., and Schmid, A. (2021). “11 biocatalytic production of white hydrogen from water using cyanobacteria” in Photosynthesis: Biotechnological applications with microalgae. ed. M. Rögner (Berlin, Boston: DE GRUYTER), 279–306.
Buhrke, T., Bleijlevens, B., Albracht, S. P., and Friedrich, B. (2001). Involvement of hyp gene products in maturation of the H(2)-sensing NiFe hydrogenase of Ralstonia eutropha. J. Bacteriol. 183, 7087–7093.2001, doi: 10.1128/JB.183.24.7087-7093.2001
Buhrke, T., Lenz, O., Krauss, N., and Friedrich, B. (2005). Oxygen tolerance of the H2-sensing NiFe hydrogenase from Ralstonia eutropha H16 is based on limited access of oxygen to the active site. J. Biol. Chem. 280, 23791–23796. doi: 10.1074/jbc.M503260200
Buhrke, T., Lenz, O., Porthun, A., and Friedrich, B. (2004). The H2-sensing complex of Ralstonia eutropha: interaction between a regulatory NiFe hydrogenase and a histidine protein kinase. Mol. Microbiol. 51, 1677–1689. doi: 10.1111/j.1365-2958.2003.03933.x
Bürstel, I., Siebert, E., Frielingsdorf, S., Zebger, I., Friedrich, B., and Lenz, O. (2016). CO synthesized from the central one-carbon pool as source for the iron carbonyl in O2-tolerant NiFe-hydrogenase. Proc. Natl. Acad. Sci. U. S. A. 113, 14722–14726. doi: 10.1073/pnas.1614656113
Dischert, W., Vignais, P. M., and Colbeau, A. (1999). The synthesis of Rhodobacter capsulatus HupSL hydrogenase is regulated by the two-component HupT/HupR system. Mol. Microbiol. 34, 995–1006. doi: 10.1046/j.1365-2958.1999.01660.x
Elsen, S., Duché, O., and Colbeau, A. (2003). Interaction between the H2 sensor HupUV and the histidine kinase HupT controls HupSL hydrogenase synthesis in Rhodobacter capsulatus. J. Bacteriol. 185, 7111–7119. doi: 10.1128/JB.185.24.7111-7119.2003
Englund, E., Liang, F., and Lindberg, P. (2016). Evaluation of promoters and ribosome binding sites for biotechnological applications in the unicellular cyanobacterium Synechocystis sp. PCC 6803. Sci. Rep. 6:36640. doi: 10.1038/srep36640
Fan, Q., Caserta, G., Lorent, C., Zebger, I., Neubauer, P., Lenz, O., et al. (2022). High-yield production of catalytically active regulatory NiFe-hydrogenase from Cupriavidus necator in Escherichia coli. Front. Microbiol. 13:894375. doi: 10.3389/fmicb.2022.894375
Fernandez-López, R., Ruiz, R., La Cruz, F.De, and Moncalián, G. (2015). Transcription factor-based biosensors enlightened by the analyte. Front. Microbiol. 6,:648. doi: 10.3389/fmicb.2015.00648
Gebler, A., Burgdorf, T., de Lacey, A. L., Rüdiger, O., Martinez-Arias, A., Lenz, O., et al. (2007). Impact of alterations near the NiFe active site on the function of the H(2) sensor from Ralstonia eutropha. FEBS J. 274, 74–85. doi: 10.1111/j.1742-4658.2006.05565.x
Gibson, D. G., Young, L., Chuang, R.-Y., Venter, J. C., Hutchison, C. A., and Smith, H. O. (2009). Enzymatic assembly of DNA molecules up to several hundred kilobases. Nat. Methods 6, 343–345. doi: 10.1038/NMETH.1318
Greening, C., Biswas, A., Carere, C. R., Jackson, C. J., Taylor, M. C., Stott, M. B., et al. (2016). Genomic and metagenomic surveys of hydrogenase distribution indicate H2 is a widely utilised energy source for microbial growth and survival. ISME J. 10, 761–777. doi: 10.1038/ismej.2015.153
Grote, A., Hiller, K., Scheer, M., Munch, R., Nortemann, B., Hempel, D. C., et al. (2005). JCat: a novel tool to adapt codon usage of a target gene to its potential expression host. Nucleic Acids Res. 33, W526–W531. doi: 10.1093/nar/gki376
Heidorn, T., Camsund, D., Huang, H.-H., Lindberg, P., Oliveira, P., Stensjö, K., et al. (2011). Synthetic biology in cyanobacteria engineering and analyzing novel functions. Meth Enzymol. 497, 539–579. doi: 10.1016/B978-0-12-385075-1.00024-x
Hoffmann, D., Gutekunst, K., Klissenbauer, M., Schulz-Friedrich, R., and Appel, J. (2006). Mutagenesis of hydrogenase accessory genes of Synechocystis sp. PCC 6803. Additional homologues of hypA and hypB are not active in hydrogenase maturation. FEBS J. 273, 4516–4527. doi: 10.1111/j.1742-4658.2006.05460.x
Howarth, R. W., and Jacobson, M. Z. (2021). How green is blue hydrogen? Energy Sci. Eng. 9, 1676–1687. doi: 10.1002/ese3.956
Huang, H.-H., Camsund, D., Lindblad, P., and Heidorn, T. (2010). Design and characterization of molecular tools for a synthetic biology approach towards developing cyanobacterial biotechnology. Nucleic Acids Res. 38, 2577–2593. doi: 10.1093/nar/gkq164
Immethun, C. M., Ng, K. M., DeLorenzo, D. M., Waldron-Feinstein, B., Lee, Y.-C., and Moon, T. S. (2016). Oxygen-responsive genetic circuits constructed in Synechocystis sp. PCC 6803. Biotechnol. Bioeng. 113, 433–442. doi: 10.1002/bit.25722
Inaba, Y., Morioka, R., Junaid, M., Shiraiwa, Y., and Suzuki, I. (2018). Development of engineered sensor perceiving gaseous toluene signal in the cyanobacterium Synechocystis sp. PCC 6803. J. Appl. Phycol. 30, 71–78. doi: 10.1007/s10811-017-1277-1
Jones, D. H., Franklin, F. C., and Thomas, C. M. (1994). Molecular analysis of the operon which encodes the RNA polymerase sigma factor sigma 54 of Escherichia coli. Microbiology 140, 1035–1043. doi: 10.1099/13500872-140-5-1035
Kleihues, L., Lenz, O., Bernhard, M., Buhrke, T., and Friedrich, B. (2000). The H2 sensor of Ralstonia eutropha is a member of the subclass of regulatory NiFe hydrogenases. J. Bacteriol. 182, 2716–2724. doi: 10.1128/JB.182.10.2716-2724.2000
Lacey, R. F., Ye, D., and Ruffing, A. M. (2019). Engineering and characterization of copper and gold sensors in Escherichia coli and Synechococcus sp. PCC 7002. Appl. Microbiol. Biotechnol. 103, 2797–2808. doi: 10.1007/s00253-018-9490-7
Lenz, O., and Friedrich, B. (1998). A novel multicomponent regulatory system mediates H2 sensing in Alcaligenes eutrophus. Proc. Natl. Acad. Sci. U. S. A. 95, 12474–12479. doi: 10.1073/pnas.95.21.12474
Lenz, O., Lauterbach, L., Frielingsdorf, S., and Friedrich, B. (2015). “4 oxygen-tolerant hydrogenases and their biotechnological potential” in Biohydrogen. ed. M. Rögner (Berlin, München, Boston: DE GRUYTER), 61–69.
Lenz, O., Strack, A., Tran-Betcke, A., and Friedrich, B. (1997). A hydrogen-sensing system in transcriptional regulation of hydrogenase gene expression in Alcaligenes species. J. Bacteriol. 179, 1655–1663. doi: 10.1128/jb.179.5.1655-1663.1997
Lenz, O., Zebger, I., Hamann, J., Hildebrandt, P., and Friedrich, B. (2007). Carbamoylphosphate serves as the source of CN(−), but not of the intrinsic CO in the active site of the regulatory NiFe-hydrogenase from Ralstonia eutropha. FEBS Lett. 581, 3322–3326. doi: 10.1016/j.febslet.2007.06.027
Li, T., Jiang, Q., Huang, J., Aitchison, C. M., Huang, F., Yang, M., et al. (2020). Reprogramming bacterial protein organelles as a nanoreactor for hydrogen production. Nat. Commun. 11:5448. doi: 10.1038/s41467-020-19280-0
Löscher, S., Gebler, A., Stein, M., Sanganas, O., Buhrke, T., Zebger, I., et al. (2010). Protein-protein complex formation affects the Ni-Fe and Fe-S centers in the H2-sensing regulatory hydrogenase from Ralstonia eutropha H16. ChemPhysChem 11, 1297–1306. doi: 10.1002/cphc.200901007
Lubitz, W., Ogata, H., Rüdiger, O., and Reijerse, E. (2014). Hydrogenases. Chem. Rev. 114, 4081–4148. doi: 10.1021/cr4005814
Lupacchini, S., Appel, J., Stauder, R., Bolay, P., Klähn, S., Lettau, E., et al. (2021). Rewiring cyanobacterial photosynthesis by the implementation of an oxygen-tolerant hydrogenase. Metab. Eng. 68, 199–209. doi: 10.1016/j.ymben.2021.10.006
Mahidhara, G., Burrow, H., Sasikala, C., and Ramana, C. V. (2019). Biological hydrogen production: molecular and electrolytic perspectives. World J. Microbiol. Biotechnol. 35:116. doi: 10.1007/s11274-019-2692-z
Martínez-García, E., Goñi-Moreno, A., Bartley, B., McLaughlin, J., Sánchez-Sampedro, L., Pascual del Pozo, H., et al. (2020). SEVA 3.0: an update of the standard European vector architecture for enabling portability of genetic constructs among diverse bacterial hosts. Nucleic Acids Res. 48, D1164–D1170. doi: 10.1093/nar/gkz1024
Ni, C., Dinh, C. V., and Prather, K. L. J. (2021). Dynamic control of metabolism. Annu. Rev. Chem. Biomol. Eng. 12, 519–541. doi: 10.1146/annurev-chembioeng-091720-125738
Opel, F., Siebert, N. A., Klatt, S., Tüllinghoff, A., Hantke, J. G., Toepel, J., et al. (2022). Generation of synthetic shuttle vectors enabling modular genetic engineering of cyanobacteria. ACS Synth. Biol. 11, 1758–1771. doi: 10.1021/acssynbio.1c00605
Patyi, G., Hódi, B., Solymosi, D., Vass, I., and Kós, P. B. (2021). Increased sensitivity of heavy metal bioreporters in transporter deficient Synechocystis PCC 6803 mutants. PLoS One 16:e0261135. doi: 10.1371/journal.pone.0261135
Pédelacq, J.-D., Cabantous, S., Tran, T., Terwilliger, T. C., and Waldo, G. S. (2006). Engineering and characterization of a superfolder green fluorescent protein. Nat. Biotechnol. 24, 79–88. doi: 10.1038/nbt1172
Pierik, A. J., Schmelz, M., Lenz, O., Friedrich, B., and Albracht, S. P. (1998). Characterization of the active site of a hydrogen sensor from Alcaligenes eutrophus. FEBS Lett. 438, 231–235. doi: 10.1016/S0014-5793(98)01306-4
Ponti, V., Dianzani, M. U., Cheeseman, K., and Slater, T. F. (1978). Studies on the reduction of nitroblue tetrazolium chloride mediated through the action of NADH and phenazine methosulphate. Chem. Biol. Interact. 23, 281–291. doi: 10.1016/0009-2797(78)90090-x
Registry of Standard Biological Parts (2003). Available at: http://parts.igem.org (Accessed October 2022).
Riaz-Bradley, A. (2019). Transcription in cyanobacteria: a distinctive machinery and putative mechanisms. Biochem. Soc. Trans. 47, 679–689. doi: 10.1042/BST20180508
Schulz, A.-C., Frielingsdorf, S., Pommerening, P., Lauterbach, L., Bistoni, G., Neese, F., et al. (2020). Formyltetrahydrofolate Decarbonylase synthesizes the active site CO ligand of O2-tolerant NiFe hydrogenase. J. Am. Chem. Soc. 142, 1457–1464. doi: 10.1021/jacs.9b11506
Schwartz, E., Gerischer, U., and Friedrich, B. (1998). Transcriptional regulation of Alcaligenes eutrophus hydrogenase genes. J. Bacteriol. 180, 3197–3204. doi: 10.1128/JB.180.12.3197-3204.1998
Schwartz, E., Henne, A., Cramm, R., Eitinger, T., Friedrich, B., and Gottschalk, G. (2003). Complete nucleotide sequence of pHG1: a Ralstonia eutropha H16 megaplasmid encoding key enzymes of H2-based Lithoautotrophy and Anaerobiosis. J. Mol. Biol. 332, 369–383. doi: 10.1016/S0022-2836(03)00894-5
Shafaat, H. S., Rüdiger, O., Ogata, H., and Lubitz, W. (2013). NiFe hydrogenases: a common active site for hydrogen metabolism under diverse conditions. Biochim. Biophys. Acta 1827, 986–1002. doi: 10.1016/j.bbabio.2013.01.015
Shcolnick, S., Shaked, Y., and Keren, N. (2007). A role for mrgA, a DPS family protein, in the internal transport of Fe in the cyanobacterium Synechocystis sp. PCC 6803. Biochim. Biophys. Acta 1767, 814–819. doi: 10.1016/j.bbabio.2006.11.015
Sonntag, C. K., Flachbart, L. K., Maass, C., Vogt, M., and Marienhagen, J. (2020). A unified design allows fine-tuning of biosensor parameters and application across bacterial species. Metab. Eng. Commun 11:e00150. doi: 10.1016/j.mec.2020.e00150
Stanier, R. Y., Deruelles, J., Rippka, R., Herdman, M., and Waterbury, J. B. (1979). Generic assignments, strain histories and properties of pure cultures of cyanobacteria. Microbiology 111, 1–61. doi: 10.1099/00221287-111-1-1
van Soom, C., de Wilde, P., and Vanderleyden, J. (1997). HoxA is a transcriptional regulator for expression of the hup structural genes in free-living Bradyrhizobium japonicum. Mol. Microbiol. 23, 967–977. doi: 10.1046/j.1365-2958.1997.2781648.x
van Soom, C., Lerouge, I., Vanderleyden, J., Ruiz-Argüeso, T., and Palacios, J. M. (1999). Identification and characterization of hupT, a gene involved in negative regulation of hydrogen oxidation in Bradyrhizobium japonicum. J. Bacteriol. 181, 5085–5089. doi: 10.1128/JB.181.16.5085-5089.1999
Wecker, M. S. A., Beaton, S. E., Chado, R. A., and Ghirardi, M. L. (2017). Development of a Rhodobacter capsulatus self-reporting model system for optimizing light-dependent, FeFe-hydrogenase-driven H2 production. Biotechnol. Bioeng. 114, 291–297. doi: 10.1002/bit.26076
Wecker, M. S. A., and Ghirardi, M. L. (2014). High-throughput biosensor discriminates between different algal H2-photoproducing strains. Biotechnol. Bioeng. 111, 1332–1340. doi: 10.1002/bit.25206
Wecker, M. S., Meuser, J. E., Posewitz, M. C., and Ghirardi, M. L. (2011). Design of a new biosensor for algal H2 production based on the H2-sensing system of Rhodobacter capsulatus. Int. J. Hydrog. Energy 36, 11229–11237. doi: 10.1016/j.ijhydene.2011.05.121
Wegelius, A., Khanna, N., Esmieu, C., Barone, G. D., Pinto, F., Tamagnini, P., et al. (2018). Generation of a functional, semisynthetic FeFe-hydrogenase in a photosynthetic microorganism. Energy Environ. Sci. 11, 3163–3167. doi: 10.1039/c8ee01975d
Winter, G., Buhrke, T., Jones, A. K., and Friedrich, B. (2004). The role of the active site-coordinating cysteine residues in the maturation of the H2-sensing NiFe hydrogenase from Ralstonia eutropha H16. Arch. Microbiol. 182, 138–146. doi: 10.1007/s00203-004-0680-6
Zhang, J., Jensen, M. K., and Keasling, J. D. (2015). Development of biosensors and their application in metabolic engineering. Curr. Opin. Chem. Biol. 28, 1–8. doi: 10.1016/j.cbpa.2015.05.013
Zimmer, D., Schwartz, E., Tran-Betcke, A., Gewinner, P., and Friedrich, B. (1995). Temperature tolerance of hydrogenase expression in Alcaligenes eutrophus is conferred by a single amino acid exchange in the transcriptional activator HoxA. J. Bacteriol. 177, 2373–2380. doi: 10.1128/jb.177.9.2373-2380.1995
Keywords: sensing and signaling, biotechnological hydrogen, regulatory hydrogenase, biosensor, synthetic biology, cyanobacteria
Citation: Opel F, Itzenhäuser MA, Wehner I, Lupacchini S, Lauterbach L, Lenz O and Klähn S (2023) Toward a synthetic hydrogen sensor in cyanobacteria: Functional production of an oxygen-tolerant regulatory hydrogenase in Synechocystis sp. PCC 6803. Front. Microbiol. 14:1122078. doi: 10.3389/fmicb.2023.1122078
Received: 12 December 2022; Accepted: 22 February 2023;
Published: 22 March 2023.
Edited by:
Xuefeng Lu, Qingdao Institute of Bioenergy and Bioprocess Technology (CAS), ChinaReviewed by:
Peter Lindblad, Uppsala University, SwedenCopyright © 2023 Opel, Itzenhäuser, Wehner, Lupacchini, Lauterbach, Lenz and Klähn. This is an open-access article distributed under the terms of the Creative Commons Attribution License (CC BY). The use, distribution or reproduction in other forums is permitted, provided the original author(s) and the copyright owner(s) are credited and that the original publication in this journal is cited, in accordance with accepted academic practice. No use, distribution or reproduction is permitted which does not comply with these terms.
*Correspondence: Stephan Klähn, stephan.klaehn@ufz.de
Disclaimer: All claims expressed in this article are solely those of the authors and do not necessarily represent those of their affiliated organizations, or those of the publisher, the editors and the reviewers. Any product that may be evaluated in this article or claim that may be made by its manufacturer is not guaranteed or endorsed by the publisher.
Research integrity at Frontiers
Learn more about the work of our research integrity team to safeguard the quality of each article we publish.