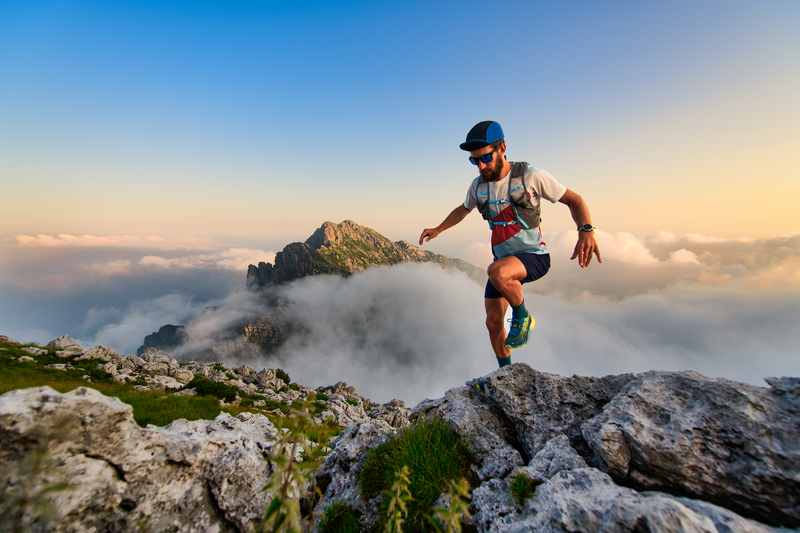
94% of researchers rate our articles as excellent or good
Learn more about the work of our research integrity team to safeguard the quality of each article we publish.
Find out more
ORIGINAL RESEARCH article
Front. Microbiol. , 09 May 2023
Sec. Microorganisms in Vertebrate Digestive Systems
Volume 14 - 2023 | https://doi.org/10.3389/fmicb.2023.1117905
Functional constipation (FC) is a high morbidity gastrointestinal disease for which dysfunction in the enteric nervous system is a major pathogenesis mechanism. To enhance our understanding of the involvement of intestinal microbiota and its metabolites in the pathogenesis of FC, we conducted a shotgun metagenomic sequencing analysis of gut microbiota and serum short-chain fatty acids (SCFAs) analysis in 460 Chinese women with different defecation frequencies. We observed that the abundance ofFusobacterium_varium, a butyric acid-producing bacterium, was positively correlated (P = 0.0096) with the frequency of defecation; however, the concentrations of serum butyric acid was negatively correlated (P = 3.51E-05) with defecation frequency. These results were verified in an independent cohort (6 patients with FC and 6 controls). To further study the effects of butyric acid on intestinal nerve cells, we treated mouse intestinal neurons in vitro with various concentrations of butyrate (0.1, 0.5, 1, and 2.5 mM). We found that intestinal neurons treated with 0.5 mM butyrate proliferated better than those in the other treatment groups, with significant differences in cell cycle and oxidative phosphorylation signal pathways. We suggest that the decreased butyrate production resulting from the reduced abundance of Fusobacterium in gut microbiota affects the proliferation of intestinal neurons and the energy supply of intestinal cells. However, with FC disease advancing, the consumption and excretion of butyric acid reduce, leading to its accumulation in the intestine. Moreover, the accumulation of an excessively high amount of butyric acid inhibits the proliferation of nerve cells and subsequently exacerbates the disease.
Functional constipation (FC) is one of the most common gastrointestinal diseases in clinical practice. The incidence rate of FC is approximately 14–17%, increasing with age (Bharucha et al., 2013; Bharucha and Wald, 2019). Until now, FC is believed to be related to low intestinal motility and poor lifestyle habits; however, its specific pathogenesis remains unclear (Aziz et al., 2020). The prevalence of FC in female patients is about 1.5 times higher than that in men, mainly because the drastic changes in hormone levels in women during menstruation and pregnancy affect the transportation of the small intestine and colon (Bharucha et al., 2013). Therefore, studying the pathogenesis of FC among female patients and identifying effective treatments to improve constipation is of great research value and social significance.
The enteric nervous system (ENS) plays a crucial role in the intestine. Their complex neural networks dominate and control intestinal function (Deb et al., 2020; Camilleri, 2021; Saldana-Morales et al., 2021; Vicentini et al., 2021). Previous studies have shown that the volume of colonic Cajal stromal cells in some patients with FC is significantly reduced, and the neuronal structure in the colonic circular smooth muscle layer is also reduced (He et al., 2000; Lyford et al., 2002; Tong et al., 2004), suggesting that the reduction of these cells may play an important role in the pathophysiology of chronic constipation. However, the specific mechanisms underlying neuronal decrease are unclear.
The human gut tract contains bacteria, archaea, viruses, and other microorganisms. Their metabolites include vitamins, short-chain fatty acids (SCFAs), secondary bile acids, choline metabolites, phenols, terpenoids, lipids, and hormones (Agus et al., 2021; Xiao et al., 2021). Normal microbiota and the external environment form a microecosystem that affects intestinal function through metabolites to resist external infections and improve autoimmunity.
Currently, SCFAs, secondary bile acids, and choline metabolites are considered to be the most important metabolites that regulate intestinal function. Because SCFAs can only be produced via glycolysis by anaerobic bacteria in the human intestine (Morrison and Preston, 2016), they are the most widely studied gut microbiota-derived metabolites. SCFAs are the main energy source for intestinal cells and are involved in various physiological functions within the host (Martin-Gallausiaux et al., 2021). In the ENS, SCFAs can regulate enteric neuronal survival and stimulate neurogenesis, but the specific mechanism is unclear (Vicentini et al., 2021).
Previous studies have shown that improving gut microbiota composition can alleviate FC. For example, common probiotics improve the intestinal microenvironment, enhance intestinal peristalsis and alleviate FC by increasing the concentration of 5-HT and SCFAs in the intestine (Miller et al., 2017; Martoni et al., 2019; Lewis et al., 2020; Wang et al., 2020). Fecal transplantation has also been used to treat patients with FC and achieved good efficacy (Yang et al., 2021; Zhang et al., 2021). However, our understanding of the involvement of gut microbiota and its metabolites in the pathophysiology of FC is still lacking.
Consequently, in this study, we performed a shotgun metagenomic sequencing analysis of gut microbiota and serum SCFAs analysis in 460 Chinese menopausal women with different defecation frequencies to explore the relationship between intestinal microbiota and their metabolites, intestinal peristalsis, and FC and subsequently validated the most significant findings in an independent sample. Furthermore, we performed in vitro cell experiments to verify the effect of butyrates on the proliferation of intestinal nerve cells.
Overall, 518 perimenopausal and postmenopausal female volunteers were recruited in the Third Affiliated Hospital of Southern Medical University, Guangzhou, in November 2017, according to the inclusion and exclusion criteria in this study. We collected peripheral blood and fecal samples from these volunteers, performed metagenomic sequencing, and targeted the detection of serum SCFAs. After excluding individuals with missing data on one of the multi-omics data, we obtained multi-omics data from 460 Chinese Han menopausal women for the subsequent analysis. The participants were divided into the following four groups based on defecation frequency: group 1: >2 times/day, group 2: 1 time/day, group 3: 1 time/2 days, and group 4: <1 time/3 days. Referring to Rome IV for the diagnostic of FC, group 4 was identified as patients with FC, group 3 could be considered as high-risk people for FC, and groups 1 and 2 were low-risk people. There were no significant differences in age, menopausal age, and body mass index (BMI) among the four groups (Table 1 and Supplementary Table 1). However, since there were only 10 participants in group 4 and the data dispersion was high, we also combined groups 3 and 4 into one FC high-risk (FCHR) group for some of the subsequent analyses.
We performed gut microbiota profiling in the four groups through shotgun metagenomic sequencing. After data quality control and filtering, the average number of microbes obtained from each sample was 10,720. For subsequent analyses, we focused on 625 bacterial species whose relative abundance was greater than 0.01% (Supplementary Table 2). As shown in Figure 1A, at the phylum level, groups 1 and 2 had higher abundances of Bacteroides, Fusobacteria, and Proteobacteria than groups 3 and 4, while groups 3 and 4 had a higher abundance of Firmicutes. Similarly, at the genus level, groups 1 and 2 had higher Bacteroidaceae_ Bacteroides and lower Firmicutes levels than groups 3 and 4 (Supplementary Figure 1). These results indicated that there were significant differences in the composition of gut microbiota among people with different defecation frequencies. Moreover, we conducted α and β diversity analyses of intestinal microorganisms and observed that there were differences in biodiversity among the four groups. Shannon and Simpson indices and the principal coordinates analysis (PCoA) based on Bray Curtis distances showed that the biodiversity of groups 1 and 2 was lower than that of groups 3 and 4, and there were statistically significant differences between groups 1 and 3, as well as groups 2 and 3 (Figures 1B–D).
Figure 1. Significant bacterial structural differences between low- and high-risk groups of FC based on metagenomic data analysis. (A) The compositions and relative abundances of intestinal microorganisms in the four groups were different. (B) Shannon diversity in four groups. P = 1.00E-07 for the comparison between groups 1 and 3, P = 3.60E-05 for the comparison between groups 2 and 3. (C) Simpson diversity in four groups. P = 2.90E-05 for the comparison between groups 1 and 3, P = 7.95E-04 for the comparison between groups 2 and 3. (D) Principal coordinate analysis, P = 0.002 for the comparison between groups 1 and 3, P = 0.002 for the comparison between group 2 and 3. (E) The top 10 different bacteria species among the four groups obtained via Deseq2. In the comparison between groups 1 and 2, Fusobacterium (FC = 1.34, P = 0.0004). s_Fusobacterium_varium (FC = 1.32, P = 0.0038). s_Akkermansia_muciniphila (FC = –2.19, P = 2.62E-06), a positive FC indicates a decrease in relative abundance in the latter group of comparisons, and a negative FC indicates an increase in relative abundance in the latter group of comparisons. In the comparison between groups 2 and 3, Fusobacterium (FC = 2.64, P = 2.54E-17). s_Fusobacterium_varium (FC = 3.32, P = 5.61E-21). In the comparison between groups 3 and 4, Acetobacter (FC = 2.22, P = 6.52E-05). Akkermansia (FC = 2.05, P = 0.017). s_Akkermansia_sp._CAG:344 (FC = 3.79, P = 6.67E-06), FC = log2 fold change, A positive FC indicates a decrease in relative abundance in the latter group compared between the two groups, and a negative FC indicates an increase in relative abundance in the latter group compared between the two groups.
To clarify the correlation between the type of bacteria and defecation frequency, we used DESeq2 to compare the bacterial operational taxonomic units (OTUs) among the four groups and obtained the top 10 bacteria with the largest fold change. The results showed that Fusobacterium was the differential bacteria with the greatest fold change in groups 1 and 2, as well as groups 2 and 3, while s_Fusobacterium_varium was the only bacteria that coexisted in the two differential lists. In groups 1 and 2, we also found an increase in the relative abundance of s_Akkermansia_muciniphila, which may lead to intestinal damage. In groups 3 and 4, we found that Acetobacter and Akkermansia were the top two differential bacteria with a decrease in relative abundance, among which s_Akkermansia_sp._CAG:344 was the bacteria with the largest fold change (Figure 1E and Supplementary Table 4). Therefore, comparing the FC low- and high-risk groups, we observed that Fusobacterium, a butyric acid-producing bacterium, was the key differential bacterium in groups 1, 2, and 3 (Supplementary Figure 3). The abundances of s_Fusobacterium_varium and s_Fusobacterium_mortiferum in the population were positively correlated with the frequency of defecation (Supplementary Figure 4). In contrast, we found no significant difference in other butyrate-producing bacteria and methanogens such as Faecalibacterium_prausnitzii, butyrateimonas_virosa, Lactobacillus, Bifidobacterium, and methanogenic in the four groups (Supplementary Table 6). Similar results were also obtained when comparing the FCHR group with groups 1 and 2 (Supplementary Figure 5).
We compared the serum SCFA levels among the four FC groups and found that butyric acid was significantly different between groups 1 and 2, 1 and 3, and 1 or 2 and the FCHR group (Table 2). In addition, serum butyric acid levels were negatively correlated with the frequency of defecation (Figure 2B). These results suggested that serum SCFAs concentration, especially butyric acid, may be closely related to intestinal defecation function.
Figure 2. Correlation analysis between the abundance of carbohydrate metabolism pathway, defecation frequency, and serum butyric acid concentration. The abundance of carbohydrate metabolism pathway in gut microbiota was (A) positively correlated (P = 0.013) with defecation frequency but (C) negatively correlated (P = 0.036) with serum butyric acid levels across the four groups. (B) Defecation frequency was negatively correlated (P = 3.51E-05) with serum butyric acid levels across the four groups. There was no significant correlation between the abundance of carbohydrate metabolism pathway and serum butyric acid concentration in (D) group 1 (P = 0.105), (E) group 2 (P = 0.373), (F) group 3 (P = 0.314), and (G) group 4 (P = 0.984).
We further explored the potential differences of bacterial metabolic pathways in different groups to identify gut microbiota functional capacity associated with FC. After quality control and host gene removal, clean reads were mapped to 1,048,576 unigenes in the integrated gene catalog (Homo_sapiens.GRCh38.dna_sm.chromosome.chr.fa). The differential metabolic pathway analysis [based on Kyoto Encyclopedia of Genes and Genomes (KEGG) level 2 modules] detected eight differential metabolic pathways between groups 2 and 3 (Table 3), and similar results were obtained when comparing the FCHR group with groups 1 and 2 (Supplementary Table 7). In group 3 and the FCHR group, the abundance of metabolic pathways for cofactors and vitamins metabolism, xenobiotics biodegradation and metabolism, and carbohydrate metabolism decreased, whereas pathways for translation, cell growth and death, and replication and repair increased (Table 3 and Supplementary Table 7). These differential metabolic pathways suggested that with an advancing degree of FC disease, the metabolic ability of intestinal microbes to utilize and metabolize exogenous substances decreased; however, the rate of the renewal of microbes increased.
Carbohydrate metabolism was closely associated with butyric acid metabolism, and the correlation analysis across the four groups showed that the frequency of defecation was positively correlated with the abundance of carbohydrate metabolism pathway, but carbohydrate metabolism was negatively correlated to the concentration of serum butyric acid (Figures 2A, C). However, there was no significant correlation between serum butyric acid concentration and the abundance of carbohydrate metabolism pathways within each individual group (Figures 2D–G). These results showed that butyric acid production capacity directly affects the concentration of butyric acid absorbed into the blood in the intestine, potentially affecting the frequency of defecation.
To further validate our findings, we recruited an independent sample of 12 premenopausal women, including 6 patients with FC and 6 healthy controls (HC), in Huai Hua City, Hunan Province, China. There were no significant differences in age and BMI between the FC and HC groups (Table 4). Metagenomics next-generation sequencing (mNGS) and targeted SCFAs were used to evaluate fecal and serum samples. MicrobiomeAnalyst (Chong et al., 2020) was used for analysis after obtaining the filtered data. We compared the structural composition of the intestinal microbiota of 460 people with that of the 12 people. The results showed that there were differences in the structure of intestinal microbiota between the two groups (Figures 3A, B). At the gate level, although the structure of the intestinal microbiota of the two groups was similar, there were significant differences. Among 460 people, the top five in relative abundance were Bacteroides, Clostridium, Eubacterium, Firmicutes, and Roseburia, while among the 12 people were Bacteroides, Prevotella, Escherichia, Faecalibacterium. Moreover, the Simpson and Shannon indices of the 12 people were significantly lower than that of the 460 people. This difference in intestinal microbiota structure was attributed to geographic differences (He et al., 2018).
Figure 3. Significant bacterial structural differences between HC and FC groups were found based on metagenomic data analysis. (A,B) The relative abundance of intestinal microbiota in phyla and genus levels of the two groups. (C) Alpha diversity analysis, Simpson, P = 0.52, Shannon, P = 0.87. (D) PCoA, P = 0.71. (E) The LDA distribution diagram analysis showed a clear alteration of the microbiota characterized by higher s_Fusobacterium ulcerans levels in the HC group (LDA score >2.5). In the comparison between groups HC and FC, we found g_Fusobacterium (LDAscore = –3.74, P = 0.025), s_Fusobacterium_ulcerans (LDAscore = –3.64, P = 0.037), s_Fusobacterium_mortiferum (LDAscore = –3.05, P = 0.025), s_Lachnospiraceae_bacterium_GAM79 (LDAscore = 3.64, P = 0.038), s_Bacteroides_sp_4_1_36 (LDAscore = 3.25, P = 0.025), s_Bacteroides_fluxus (LDAscore = 3.11, P = 0.01), s_Bacteroides_sp_ AM54_2NS (LDAscore = 2.82, P = 0.01). A positive LDAscore indicates a decrease in relative abundance in the HC group, and a negative LDAscore indicates an increase in relative abundance in the HC group. LDAscore, linear discriminant analysis score. A positive LDAscore indicates an increase in relative abundance in the FC group compared with the HC group, and a negative LDAscore indicates a decrease in relative abundance in the FC group compared with the HC group.
The validation population analysis results showed that the Shannon and Simpson indices and PCoA were not significantly different between the two groups (Figures 3D, E). The results of differential intestinal microbes obtained by linear discriminant analysis (LDA) showed that at the genus level, only the relative abundance of g_Fusobacterium decreased in the FC group compared with the HC group (LDAscore = −3.74, P = 0.025). At the species level, s_Fusobacterium_ulcerans was the most significantly reduced bacteria (LDAscore = −3.64, P = 0.037), and s_Fusobacterium_mortiferum (LDAscore = −3.05, P = 0.025) was also at the top. Several other species also showed higher relative abundance in the HC group, including s_Lachnospiraceae_bacterium_GAM79, s_Bacteroides_sp_4_1_36, s_Bacteroides_fluxus, and s_Bacteroides_sp_AM54_2NS (Figure 3E and Supplementary Table 5). Among the tested serum SCFAs, only butyric acid showed a significant difference (P = 0.002) between the FC and HC groups (Table 5).
Overall, we obtained similar results by analyzing two groups of women with different living places and ages; the abundance of Fusobacterium in the intestine was positively correlated with the frequency of defecation; however, serum butyric acid was the opposite.
Butyrate plays an important role in intestinal energy supply, nerve signal transduction, and maintenance of intestinal homeostasis (Soret et al., 2010; Su et al., 2021). To study whether butyrate affects the proliferation of intestinal nerve cells, we isolated intestinal nerve cells from C57BL/6 mice, and according to a previous study (Su et al., 2021), we chose five different butyric acid concentrations (0, 0.1, 0.5, 1, and 2.5 mM) to culture the cells for 72 h (Figure 4 and Supplementary Figure 2). We found that the nerve cells cultured in 0.5 mM butyrate proliferated significantly faster and showed a better growth state than those cultured under other conditions (Figures 4B, C). These results demonstrated that the proliferation of intestinal nerve cells was directly affected by butyrate concentration.
Figure 4. Butyrate affects intestinal nerve cell proliferation in vitro. (A) The nerve cells were cultured with different concentrations of butyrate (0, 0.1, 0.5, 1, and 2.5 mM) for 72 h and stained with nerve-specific antibody Tuj1 and DAPI. (B,C) Cells cultured in 0.5 mM butyrate showed significantly higher cell proliferation rate (assessed by OD450) and area coverage than cells cultured under all the other conditions. *P < 0.05, ***P < 0.001.
To further investigate the specific mechanism underlying the observed differences in intestinal nerve cell proliferation under different butyrate concentrations, we performed RNA-seq on mouse intestinal nerve cells after culturing them with various concentrations of butyrate (0, 0.5, and 2.5 mM) for 72 h. Compared with the 0.5 mM group, 1,029 genes were significantly up-regulated and 323 genes were down-regulated in the 0 mM group; whereas, compared with the 2.5 mM group, 794 genes were up-regulated and 124 genes were down-regulated in Figures 5A, B.
Figure 5. Mechanism of butyrate on the proliferation of intestinal nerve cells. (A,B) Volcano plots show up-regulated and down-regulated genes of 0.5 mM/0 mM and 2.5 mM/0.5 mM, fold change > 1. (C,E) Top 20 of the functional and pathway analysis by go biological process and KEGG of 0.5 mM/0 mM. (D,F) Top 20 of the function and pathway analysis by go biological process and KEGG of 2.5 mM/0.5 mM. (G–I) Top-ranked signaling pathways related to cell proliferation in GSEA analysis of 0.5 mM/0 mM comparison group. Cell cycle and apoptosis were up-regulated, and the metabolism of xenobiology by cytochrome P450 was downregulated. (J,K) Top-ranked signaling pathways related to cell proliferation in GSEA analysis of 2.5 mM/0.5 mM comparison group. Oxidative phosphorylation was up-regulated, and the cell cycle was down-regulated.
We performed gene ontology (GO), KEGG, and Gene Set Enrichment Analysis (GSEA) enrichment analyses on these differentially expressed genes (DEGs). Compared with the 0.5 mM group, the GO biological process enriched in the 0 mM group was related to cell cycle, DNA repair, and apoptotic process (Figure 5C), while the biological process enriched in the 2.5 mM group was related to cell cycle, cellular response to DNA damage stimulus, and oxidative phosphorylation (Figure 5D). KEGG and GSEA analyses showed that 21 pathways were up-regulated and 48 pathways were down-regulated in 0 mM group. Among them, cell cycle, DNA replication, and apoptosis were up-regulated, and cytochrome P450 was down-regulated in xenobiology (Figures 5E, G–I) and Supplementary Table 7). Forty-one pathways were up-regulated, and four pathways were down-regulated in the 2.5 mM group. Among them, oxidative phosphorylation, glutathione metabolism, and NAFLD were up-regulated, and cell cycle and regenerative recombination were down-regulated (Figures 5F, J, K and Supplementary Table 8). These are key pathways closely related to cell proliferation.
Therefore, although lack of butyrate and high concentration of butyrate inhibited the proliferation of nerve cells, they were caused by different mechanisms. The possible mechanism of lack of butyrate may be cell cycle disorder and subsequent apoptosis, while the high concentration of butyrate may be a series of cell damage and cell cycle inhibition caused by increased oxidative phosphorylation.
By studying female intestinal microbes and their metabolites and in vitro evaluation of butyrate effects on nerve cells, we believe that the reduction of Fusobacterium may be one of the causes of constipation. The experiment showed that butyrate with proper concentration could provide a better environment for the proliferation of nerve cells. Therefore, the maintenance of intestinal butyrate concentration is essential for intestinal function.
Intestinal microbes are closely associated with intestinal functions. Many studies have shown that there are many butyrate-producing bacteria in the gut, including F. prausnitzii and B. virosa (Thompson et al., 1992; Hertel et al., 2021). In this study, the abundant distribution of F. prausnitzii and B. virosa in the whole study population was not different; hence, Fusobacterium may play a more vital role than those two butyrate-producing bacteria in patients with FC.
In this study, we observed that the diversity of intestinal microbes was negatively correlated with the frequency of defecation. This result is consistent with the finding from a recent study where gut microbiota diversity and species of Prevotella and Bacteroides were significantly decreased in patients with chronic constipation (Dan et al., 2020). Constipation destroys the intestinal microenvironment, reduces the abundance of dominant microorganisms such as Prevotella and Bacteroides, and increases the less abundant harmful microorganisms, such as Escherichia and Enterobacteriaceae, thus leading to increased gut microbial diversity.
Firmicutes and Bacteroidetes are the core microbiota of the human gut, both of which participate in many physiological activities. Previous studies have suggested that the relative abundance ratio of Firmicutes/Bacteroidetes (F/B) in adults should be maintained within a normal range because either too high or too low has been associated with the diseases (Magne et al., 2020). Jeffery et al. (2020) reported that the F/B value could differentiate subtypes of patients with irritable bowel syndrome (IBS) in clinical practice (Stojanov et al., 2020). Liu et al. (2018) found that the F/B value of patients with liver cirrhosis was higher than that of the healthy control group. Moreover, we found that in the four groups of samples, F/B gradually increased with the increase in population FC risk, indicating the potential of abnormal F/B values as a clinical warning indicator for FC. Prevotella is also a dominant bacterium in the gut. Recent studies have reported that Prevotella can utilize polysaccharides to produce succinic acid, which enhances the immune response of antigen-specific T cells and protects host health by binding to the succinic acid receptor GPR91 on the surface of dendritic cells (Rubic et al., 2008). Additionally, inflammation caused by immune deficiency is one of the most common symptoms in FC (Rosa et al., 2023); consequently, we speculate that this is related to a decrease in the relative abundance of Prevotella.
This study showed a seemingly paradoxical result—the abundance of Fusobacterium, a butyrate-producing bacterium in the intestine, was decreased in patients with FC, whereas the serum butyric acid concentration was increased in patients with FC. In addition, the blood concentrations of other SCFAs, such as valeric acid, acetic acid, and caproic acid, were also increased (though not statistically significant) with the decrease in defecation frequency. However, similar results have been reported in studies for FC and Parkinson’s disease (PD, approximately 40–80% of patients with PD exhibit constipation or prolonged colonic transit) (Shin et al., 2020; Tian et al., 2021; Chen et al., 2022; Yang et al., 2022). For instance, Tian et al. (2021) observed a relative decrease in SCFA-producing bacteria in intestinal microbiota in patients with constipation, and Yang et al. (2022) found that patients with PD and constipation had lower levels of SCFAs in fecal samples but higher SCFA levels in plasma than in that of patients with PD without constipation. A possible explanation is that the reduction of intestinal peristalsis and defecation frequency in patients with constipation leads to decreased SCFAs consumption and excretion in the gut, which in turn results in more SCFAs are accumulated in the intestine and absorbed into the blood, particularly with an increased intestinal permeability (Tian et al., 2021).
Nerve cells play important roles in the intestine. The ENS in most areas of the intestine comprises two main ganglion layers as follows: the intermuscular and submucosal ganglions, which contain many types of intestinal neurons and glial cells. Axons from the ENS and extrinsic neurons innervate most layers of the intestinal wall and regulate several intestinal functions. One of the main pathogenesis mechanisms of FC is the dysfunction of the colonic smooth muscle nerve, which leads to slow intestinal peristalsis and causes disease (Tong et al., 2004; Deb et al., 2020; Camilleri, 2021; Saldana-Morales et al., 2021). Studies have confirmed that SCFAs play a crucial role in the ENS, including providing energy for the ENS and participating in regulating colon transport by controlling the increase of 5-HT concentration (Lin et al., 2021). However, there is no study on the relationship between SCFAs and the proliferation of the intestinal nervous system. Our research shows that an appropriate concentration of butyrate can effectively promote the proliferation of mouse intestinal nerve cells. Both low and high concentrations of butyrate can affect the normal cell cycle and damage the repair of intestinal nerve cells, possibly through different mechanisms.
One of the major pathways down-regulated in nerve cells by the lack of butyrate is the metabolism of xenobiology by cytochrome P450. This pathway is involved in regulating various cell signal transduction pathways and normal homeostasis that are crucial to the cell cycle (Navarro-Mabarak et al., 2018; Wang and Yao, 2021). When the CYP450 pathway is out of balance, the cycle of nerve cells will be disrupted, and the expression of cell cycle-related pathways and apoptosis-related pathways will be up-regulated, eventually leading to apoptosis (Wang and Yao, 2021). Another vital protein is the aryl hydrocarbon receptor (AHR), which can bind with various ligands to activate intracellular signals. Some studies have reported that a reduction of AHR expression will lead to a slowdown of intestinal peristalsis (Zapletal et al., 2017; Obata et al., 2020), while the expression of AHR in the 0 mM group decreased significantly (Supplementary Table 11). This finding may be the main factor leading to slow intestinal peristalsis in patients with FC.
Excessively high butyrate concentration mainly leads to the up-regulation of the neuronal oxidative phosphorylation pathway and down-regulation of cell cycle and endogenous recombination pathways. The increased activity of the oxidative phosphorylation pathway will lead to the increase of reactive oxygen species (ROS) and neuronal apoptosis rate (Rose et al., 2018; Singh et al., 2019). It has been reported that a high butyrate concentration can inhibit the cell cycle and hinder the repair ability of DNA Double Strand Break (DSB) (Li, 2017), and it is consistent with our results. Butyrate can also change gene expression and prevent cell proliferation by inhibiting the chromatin remodeling activity of histone deacetylases (HDACs) (Koprinarova et al., 2011; Zeng et al., 2020). In our study, we found that HDAC1 was down-regulated in both 0 mM and 2.5 mM groups, suggesting that its abnormal expression may be associated with nerve cell proliferation disorders (Supplementary Table 11).
The different mechanisms of nerve cell proliferation disorders caused by low and high concentrations of butyrate may explain the contradiction that the abundance of butyrate-producing bacteria in the intestine was positively correlated with the frequency of defecation; however, serum butyrate level is negatively correlated with the frequency of defecation. We speculate that when the abundance of butyric acid-producing bacteria in the intestine reduces, butyrate metabolism decreases, hindering intestinal nerve cell proliferation and slowing down intestinal peristalsis. This may be one of the reasons for the occurrence and initial development of FC. When constipation occurs due to the reduction of butyrate consumption in the intestine, the concentration accumulation increases and inhibits the proliferation of nerve cells, resulting in the continuous impairment of intestinal function, and the absorption of butyrate into the blood also increases correspondingly.
This study has some limitations. First, we did not define the range of butyrate concentration in the intestines of normal people, which needs to be supplemented by subsequent studies. Second, this study was conducted on Chinese women, and thus the results may not be generalized to other populations.
In conclusion, we found that reducing butyric acid-producing bacteria in the gut may cause intestinal nerve cell dysplasia and insufficient energy supply of intestinal cells, resulting in reduced frequency of defecation and increased risk of constipation. Moreover, this showed that high concentrations of butyric acid block the repair of the ENS, making the condition worse. Therefore, maintaining an appropriate concentration of butyrate in the intestine of human constipation patients may also improve the intestinal nervous system, thereby improving constipation. This finding is also a future direction for the treatment of constipation.
In this study, we collected a total of 530 volunteers from two regions as follows: the experimental and validation groups. Both groups used the same inclusion criteria, sample collection criteria, and sample storage conditions to ensure the reliability of the results. Notably, serum and fecal samples were collected in both groups. Among them, 518 female samples were collected from the Third Affiliated Hospital of Southern Medical University in Guangzhou, Guangdong Province, China, in 2017, and 12 samples were collected from the First Affiliated Hospital of Hunan Medical College in Huaihua, Hunan Province, China, in 2020. This study was approved by the Third Affiliated Hospital of Southern Medical University and was performed in accordance with the principles of the Helsinki Declaration II. The details of the research design have been described in previous studies (Gong et al., 2021; Greenbaum et al., 2022). The inclusion criteria included participants that were: 1. older than 40 years old; 2. who had lived in the sample collection area for more than 3 months.
People with a history of uterus, ovary, and gastrointestinal surgery; serious cardiovascular and cerebrovascular diseases; diabetes; liver disease; gastrointestinal disease; and other diseases related to intestinal microorganisms were excluded from the present study. Each participant signed a written informed consent form before participating in the study. Subsequently, we assessed epidemiological information on age, medical history, family history, physical activity, alcohol use, diet habits, smoking history, defecation frequency, and medical history, among others, using a questionnaire. The Rome IV criteria for functional constipation was used for evaluating gastrointestinal symptoms. Finally, 472 samples with complete information were obtained for follow-up research through screening.
Furthermore, 3 ml of peripheral blood samples were collected from each participant after overnight fasting for at least 8 h, and we isolated serum and extracted genomic DNA from the blood. According to the instruction, feces were collected at the hospital or home and delivered immediately at low temperatures. Fecal samples were obtained for DNA extraction and metagenomic sequencing. According to the manufacturer’s instructions, fecal DNA was extracted using the E.Z.N.A.® Stool DNA Kit (D4015-02, Omega, Inc., USA), and blood DNA was extracted using a SolPure DNA kit (Magen, China). Each sample was frozen at −80°C in a freezer.
The fecal samples collected from the experimental (518) and validation (12) groups in this study were sequenced using a shotgun metagenomic sequencing approach, and serum samples were analyzed by gas chromatography-mass spectrometry. The details of the data generation and quality control processes of these samples have been described previously (Lv et al., 2021).
MicrobiomeAnalyst1 (Chong et al., 2020) was used to comprehensively analyze the metagenomic data, including diversity analysis, DEseq2, and Linear Discriminant Analysis Effect Size (LEfSe). Finally, we obtained α, β diversity and intergroup differences of intestinal microorganisms. The Kruskal–Wallis test was used for SCFA analysis.
We purchased C57BL/6 mice from Hunan SJA Laboratory Animal Co., Ltd. (Changsha, China), and the 4–6 weeks old C57BL/6 mice were euthanized by intraperitoneal injection of pentobarbital sodium. The intestines were removed and cleaned using Hanks’ balanced salt solution (HBSS; ThermoFisher, USA). After stripping the intestinal mucosa with tweezers, a cell suspension was obtained by digestion and separation with 1 mg/ml collagenase 4 (C4-BIOC, Sigma-Aldrich, Germany). The cells were cultured in Neurobasal A medium specifically designed for neural cells for 48 hours. Each 50 ml of complete medium consists of 47.5 ml Neurobasic A medium (10888022, ThermoFisher, USA), 1 ml B-27 (50×) (17504044, ThermoFisher, USA), 500 μl fetal bovine serum (M3942-500ML, Sigma, USA), 500 μl 200 mM L-glutamine (ThermoFisher, USA), 50 μl glial cell-derived neurotrophin (HY-F0003, MCE, China) and 500 μl antibiotics (100×) (15140122, ThermoFisher, USA). The isolation methods and steps for mouse intestinal primary nerve cells have been previously described (Ye et al., 2020). Animal care and experiments were conducted in accordance with the Guidelines for Animal Experimentation, Central South University, Changsha, Hunan, China, and were approved by the Animal Care and Use Committee of the University.
Primary nerve cells were plated in 96 well plates with 1,000 cells/well. After culturing with 0.1, 0.5, 1, and 2.5 mM butyrate (B5887, Sigma-Aldrich, Germany) for 72 h, 10 μl Cell Counting Kit-8 (CCK-8, New Cell & Molecular Biotech, China) was added to each well. After 2 hours, absorbance values at 450 nm were examined by a microplate reader (Bio-TEK Instrument, USA).
The cells were fixed with paraformaldehyde, permeabilized with 0.5% Triton X-100 (85111, Thermo, USA), diluted in phosphate-buffered saline (PBS) buffer for 20 min at room temperature, and then blocked with goat serum. Fixed cells were incubated with Tuj-1 (bsm-52323r, BIOSs, China, 1:100 diluted with PBS) at 4°C overnight and then incubated with a 1:200 fluorescent dye-labeled secondary antibody (s0001, Affinity, China) at 37°C for 1 h. Samples were examined and photographed using a fluorescence microscope (Olympus, Tokyo, Japan). Four photos at different locations were randomly selected, and the cell area was measured using the ImageJ software.
Total RNA was extracted from mouse intestinal nerve cells using TRIzol reagent (15596018, Invitrogen, USA), and each group was prepared with three parallel replicates. All the RNA samples were sent to BGI Corporation (Shenzhen, China) for RNA-seq analysis via a BGISEQ-500 sequencer. They were averagely generating about 1.19 Gb bases per sample. The average mapping ratio with reference genome was 93.99%; 18,514 genes were identified. The pathway analysis for DEGs was performed based on the KEGG database. The data were analyzed on the Dr. Tom network platform of BGI.
Differences between two or more groups were analyzed using the independent samples t-test. When variances were not homogeneous, the data were analyzed using the Kruskal–Wallis test. All tests were performed using the SPSS 21 software. The correlation analysis of defecation frequency, serum butyric acid concentration, bacterial OTUs relative abundance, and the metabolic pathway was evaluated using the Spearman correlation coefficient. Statistical significance was set at P < 0.05.
The datasets presented in this study can be found in online repositories. The names of the repository/repositories and accession number(s) can be found below: BioProject, PRJNA950204.
This study was approved by the Third Affiliated Hospital of Southern Medical University and was performed in accordance with the principles of the Helsinki Declaration II. The patients/participants provided their written informed consent to participate in this study. This animal study was reviewed and approved by Animal Care and experiments were conducted in accordance with the Guidelines for Animal Experimentation, Central South University, Changsha, Hunan, China, and were approved by the Animal Care and Use Committee of the university.
LW, W-QL, XL, H-JT, R-PQ, and P-PL contributed to the data curation. LW, W-QL, and H-ML participated in the formal analysis. H-WD and H-MX contributed to the project conception, design, and initiation. LW and J-TY participated in the design and performance of the in vitro experiments. JS, H-WD, and H-MX supervised the study. LW contributed in the writing of the original draft. LW, XL, HS, H-WD, and H-MX participated in the writing of the review and editing. All authors contributed to the article and approved the submitted version.
This study was supported by the National Key R&D Program of China (2017YFC1001100) and the Construction Project of the Center of Reproductive Health, Central South University (164990007). H-WD and HS were partially supported by grants from the National Institutes of Health (U19AG055373, R01AR069055, R01AG061917, and R01AG068232).
We thank Jiangwan Group for supporting the “Construction Project of the Center of Reproductive Health.” Gut metagenomics sequencing data can be requested from H-MX.
The authors declare that the research was conducted in the absence of any commercial or financial relationships that could be construed as a potential conflict of interest.
All claims expressed in this article are solely those of the authors and do not necessarily represent those of their affiliated organizations, or those of the publisher, the editors and the reviewers. Any product that may be evaluated in this article, or claim that may be made by its manufacturer, is not guaranteed or endorsed by the publisher.
The Supplementary Material for this article can be found online at: https://www.frontiersin.org/articles/10.3389/fmicb.2023.1117905/full#supplementary-material
Agus, A., Clément, K., and Sokol, H. (2021). Gut microbiota-derived metabolites as central regulators in metabolic disorders. Gut 70, 1174–1182. doi: 10.1136/gutjnl-2020-323071
Aziz, I., Whitehead, W., Palsson, O., Törnblom, H., and Simrén, M. (2020). An approach to the diagnosis and management of Rome IV functional disorders of chronic constipation. Expert. Rev. Gastroenterol. Hepatol. 14, 39–46. doi: 10.1080/17474124.2020.1708718
Bharucha, A., and Wald, A. (2019). Chronic constipation. Mayo Clin. Proc. 94, 2340–2357. doi: 10.1016/j.mayocp.2019.01.031
Bharucha, A., Pemberton, J., and Locke, G. (2013). American gastroenterological association technical review on constipation. Gastroenterology 144, 218–238. doi: 10.1053/j.gastro.2012.10.028
Camilleri, M. (2021). Gastrointestinal motility disorders in neurologic disease. J. Clin. Invest. 131:e143771. doi: 10.1172/JCI143771
Chen, S., Chen, C., Liao, H., Lin, Y., Wu, Y., Liou, J., et al. (2022). Association of fecal and plasma levels of short-chain fatty acids with gut microbiota and clinical severity in patients with Parkinson disease. Neurology 98, e848–e858. doi: 10.1212/WNL.0000000000013225
Chong, J., Liu, P., Zhou, G., and Xia, J. (2020). Using microbiomeanalyst for comprehensive statistical, functional, and meta-analysis of microbiome data. Nat. Protoc. 15, 799–821. doi: 10.1038/s41596-019-0264-1
Dan, Z., Mao, X., Liu, Q., Guo, M., Zhuang, Y., Liu, Z., et al. (2020). Altered gut microbial profile is associated with abnormal metabolism activity of autism spectrum disorder. Gut Microbes. 11, 1246–1267. doi: 10.1080/19490976.2020.1747329
Deb, B., Prichard, D. O., and Bharucha, A. E. (2020). Constipation and fecal incontinence in the elderly. Curr. Gastroenterol. Rep. 22:54. doi: 10.1007/s11894-020-00791-1
Gong, R., Xiao, H., Zhang, Y., Zhao, Q., Su, K., Lin, X., et al. (2021). Identification and functional characterization of metabolites for bone mass in peri- and postmenopausal Chinese women. J. Clin. Endocrinol. Metab. 106, e3159–e3177. doi: 10.1210/clinem/dgab146
Greenbaum, J., Lin, X., Su, K., Gong, R., Shen, H., Shen, J., et al. (2022). Integration of the human gut microbiome and serum metabolome reveals novel biological factors involved in the regulation of bone mineral density. Front. Cell Infect. Microbiol. 12:853499. doi: 10.3389/fcimb.2022.853499
He, C., Burgart, L., Wang, L., Pemberton, J., Young-Fadok, T., Szurszewski, J., et al. (2000). Decreased interstitial cell of Cajal volume in patients with slow-transit constipation. Gastroenterology 118, 14–21. doi: 10.1016/s0016-5085(00)70409-4
He, Y., Wu, W., Zheng, H., Li, P., McDonald, D., Sheng, H., et al. (2018). Regional variation limits applications of healthy gut microbiome reference ranges and disease models. Nat. Med. 24, 1532–1535. doi: 10.1038/s41591-018-0164-x
Hertel, J., Heinken, A., Martinelli, F., and Thiele, I. (2021). Integration of constraint-based modeling with fecal metabolomics reveals large deleterious effects of Fusobacterium spp. on community butyrate production. Gut Microbes. 13, 1–23. doi: 10.1080/19490976.2021.1915673
Jeffery, I. B., Das, A., O’Herlihy, E., Coughlan, S., Cisek, K., Moore, M., et al. (2020). Differences in fecal microbiomes and metabolomes of people with vs without irritable bowel syndrome and bile acid malabsorption. Gastroenterology 158, 1016.e8–1028.e8. doi: 10.1053/j.gastro.2019.11.301
Koprinarova, M., Botev, P., and Russev, G. (2011). Histone deacetylase inhibitor sodium butyrate enhances cellular radiosensitivity by inhibiting both DNA nonhomologous end joining and homologous recombination. DNA Repair 10, 970–977. doi: 10.1016/j.dnarep.2011.07.003
Lewis, E. D., Antony, J., Crowley, D., Piano, A., Bhardwaj, R., Tompkins, T., et al. (2020). Efficacy of Lactobacillus paracasei HA-196 and Bifidobacterium longum R0175 in alleviating symptoms of irritable bowel syndrome (IBS): A randomized placebo-controlled study. Nutrients 12:1159. doi: 10.3390/nu12041159
Li, C. (2017). Flow cytometry analysis of cell cycle and specific cell synchronization with butyrate. Methods Mol. Biol. 1524, 149–159. doi: 10.1007/978-1-4939-6603-5_9
Lin, X., Liu, Y., Ma, L., Ma, X., Shen, L., Ma, X., et al. (2021). Constipation induced gut microbiota dysbiosis exacerbates experimental autoimmune encephalomyelitis in C57BL/6 mice. J. Transl. Med. 19:317. doi: 10.1186/s12967-021-02995-z
Liu, Y., Jin, Y., Li, J., Zhao, L., Li, Z., Xu, J., et al. (2018). Small bowel transit and altered gut microbiota in patients with liver cirrhosis. Front. Physiol. 9:470. doi: 10.3389/fphys.2018.00470
Lv, W., Lin, X., Shen, H., Liu, H., Qiu, X., Li, B., et al. (2021). Human gut microbiome impacts skeletal muscle mass via gut microbial synthesis of the short-chain fatty acid butyrate among healthy menopausal women. J. Cachexia Sarcopenia Muscle 12, 1860–1870. doi: 10.1002/jcsm.12788
Lyford, G., He, C., Soffer, E., Hull, T., Strong, S., Senagore, A., et al. (2002). Pan-colonic decrease in interstitial cells of Cajal in patients with slow transit constipation. Gut 51, 496–501. doi: 10.1136/gut.51.4.496
Magne, F., Gotteland, M., Gauthier, L., Zazueta, A., Pesoa, S., Navarrete, P., et al. (2020). The firmicutes/bacteroidetes ratio: A relevant marker of gut dysbiosis in obese patients? Nutrients 12:1474. doi: 10.3390/nu12051474
Martin-Gallausiaux, C., Marinelli, L., Blottière, H., Larraufie, P., and Lapaque, N. (2021). SCFA: Mechanisms and functional importance in the gut. Proc. Nutr. Soc. 80, 37–49. doi: 10.1017/S0029665120006916
Martoni, C., Evans, M., Chow, C., Chan, L., and Leyer, G. (2019). Impact of a probiotic product on bowel habits and microbial profile in participants with functional constipation: A randomized controlled trial. J. Dig. Dis. 20, 435–446. doi: 10.1111/1751-2980.12797
Miller, L., Ouwehand, A., and Ibarra, A. (2017). Effects of probiotic-containing products on stool frequency and intestinal transit in constipated adults: Systematic review and meta-analysis of randomized controlled trials. Ann. Gastroenterol. 30, 629–639. doi: 10.20524/aog.2017.0192
Morrison, D., and Preston, T. (2016). Formation of short chain fatty acids by the gut microbiota and their impact on human metabolism. Gut Microbes. 7, 189–200. doi: 10.1080/19490976.2015.1134082
Navarro-Mabarak, C., Camacho-Carranza, R., and Espinosa-Aguirre, J. (2018). Cytochrome P450 in the central nervous system as a therapeutic target in neurodegenerative diseases. Drug Metab. Rev. 50, 95–108. doi: 10.1080/03602532.2018.1439502
Obata, Y., Castaño, Á, Boeing, S., Bon-Frauches, A., Fung, C., Fallesen, T., et al. (2020). Neuronal programming by microbiota regulates intestinal physiology. Nature 578, 284–289. doi: 10.1038/s41586-020-1975-8
Rosa, C., Altomare, A., Terrigno, V., Carbone, F., Tack, J., Cicala, M., et al. (2023). Constipation-predominant irritable bowel syndrome (IBS-C): Effects of different nutritional patterns on intestinal dysbiosis and symptoms. Nutrients 15:1647. doi: 10.3390/nu15071647
Rose, S., Bennuri, S., Davis, J., Wynne, R., Slattery, J., Tippett, M., et al. (2018). Butyrate enhances mitochondrial function during oxidative stress in cell lines from boys with autism. Transl. Psychiatry 8:42. doi: 10.1038/s41398-017-0089-z
Rubic, T., Lametschwandtner, G., Jost, S., Hinteregger, S., Kund, J., Carballido-Perrig, N., et al. (2008). Triggering the succinate receptor GPR91 on dendritic cells enhances immunity. Nat. Immunol. 9, 1261–1269. doi: 10.1038/ni.1657
Saldana-Morales, F., Kim, D., Tsai, M., and Diehl, G. (2021). Healthy intestinal function relies on coordinated enteric nervous system, immune system, and epithelium responses. Gut Microbes. 13, 1–14. doi: 10.1080/19490976.2021.1916376
Shin, C., Lim, Y., Lim, H., and Ahn, T. (2020). Plasma short-chain fatty acids in patients with parkinson’s disease. Mov. Disord. 35, 1021–1027. doi: 10.1002/mds.28016
Singh, A., Kukreti, R., Saso, L., and Kukreti, S. (2019). Oxidative stress: A key modulator in neurodegenerative diseases. Molecules 24:1583. doi: 10.3390/molecules24081583
Soret, R., Chevalier, J., De Coppet, P., Poupeau, G., Derkinderen, P., Segain, J., et al. (2010). Short-chain fatty acids regulate the enteric neurons and control gastrointestinal motility in rats. Gastroenterology 138, 1772–1782. doi: 10.1053/j.gastro.2010.01.053
Stojanov, S., Berlec, A., and Štrukelj, B. (2020). The influence of probiotics on the firmicutes/bacteroidetes ratio in the treatment of obesity and inflammatory bowel disease. Microorganisms 8:1715. doi: 10.3390/microorganisms8111715
Su, M., He, Y., Xue, S., Yu, J., Ren, X., Huang, N., et al. (2021). Butyric acid alleviated chronic intermittent hypoxia-induced lipid formation and inflammation through up-regulating HuR expression and inactivating AMPK pathways. Biosci. Rep. 41:BSR20203639. doi: 10.1042/BSR20203639
Thompson, J., Nguyen, N., and Robrish, S. (1992). Sucrose fermentation by Fusobacterium mortiferum ATCC 25557: Transport, catabolism, and products. J. Bacteriol. 174, 3227–3235. doi: 10.1128/jb.174.10.3227-3235.1992
Tian, H., Chen, Q., Yang, B., Qin, H., and Li, N. (2021). Analysis of gut microbiome and metabolite characteristics in patients with slow transit constipation. Dig. Dis. Sci. 66, 3026–3035. doi: 10.1007/s10620-020-06500-2
Tong, W., Liu, B., Zhang, L., Zhang, S., and Lei, Y. (2004). Decreased interstitial cells of Cajal in the sigmoid colon of patients with slow transit constipation. Int. J. Colorectal. Dis. 19, 467–473. doi: 10.1007/s00384-003-0577-x
Vicentini, F., Keenan, C., Wallace, L., Woods, C., Cavin, J., Flockton, A., et al. (2021). Intestinal microbiota shapes gut physiology and regulates enteric neurons and glia. Microbiome 9:210. doi: 10.1186/s40168-021-01165-z
Wang, J., and Yao, S. (2021). Roles of gut microbiota and metabolites in pathogenesis of functional constipation. Evid. Based Complement. Alternat. Med. 2021:5560310. doi: 10.1155/2021/5560310
Wang, J., Bai, X., Peng, C., Yu, Z., Li, B., Zhang, W., et al. (2020). Fermented milk containing Lactobacillus casei Zhang and Bifidobacterium animalis ssp. lactis V9 alleviated constipation symptoms through regulation of intestinal microbiota, inflammation, and metabolic pathways. J. Dairy Sci. 103, 11025–11038. doi: 10.3168/jds.2020-18639
Xiao, L., Liu, Q., Luo, M., and Xiong, L. (2021). Gut microbiota-derived metabolites in irritable bowel syndrome. Front. Cell Infect. Microbiol. 11:729346. doi: 10.3389/fcimb.2021.729346
Yang, B., Tian, H., Ye, C., Lin, Z., Zhao, D., Ma, C., et al. (2021). The efficacy and safety of fecal microbiota transplantation combined with biofeedback for mixed constipation: A retrospective cohort study. Front. Med. 8:746990. doi: 10.3389/fmed.2021.746990
Yang, X., Ai, P., He, X., Mo, C., Zhang, Y., Xu, S., et al. (2022). Parkinson’s disease is associated with impaired gut-blood barrier for short-chain fatty acids. Mov. Disord. 37, 1634–1643. doi: 10.1002/mds.29063
Ye, L., Li, G., Goebel, A., Raju, A., Kong, F., Lv, Y., et al. (2020). Caspase-11-mediated enteric neuronal pyroptosis underlies Western diet-induced colonic dysmotility. J. Clin. Invest. 130, 3621–3636. doi: 10.1172/JCI130176
Zapletal, O., Tylichová, Z., Neča, J., Kohoutek, J., Machala, M., Milcová, A., et al. (2017). Butyrate alters expression of cytochrome P450 1A1 and metabolism of benzo[a]pyrene via its histone deacetylase activity in colon epithelial cell models. Arch. Toxicol. 91, 2135–2150. doi: 10.1007/s00204-016-1887-4
Zeng, H., Safratowich, B., Wang, T., Hamlin, S., and Johnson, L. (2020). Butyrate inhibits deoxycholic-acid-resistant colonic cell proliferation via cell cycle arrest and apoptosis: A potential pathway linking dietary fiber to cancer prevention. Mol. Nutr. Food Res. 64:e1901014. doi: 10.1002/mnfr.201901014
Keywords: functional constipation, metagenomics, Fusobacterium, butyrate, enteric nervous system
Citation: Wang L, Lv W-Q, Yang J-T, Lin X, Liu H-M, Tan H-J, Quan R-P, Long P-P, Shen H, Shen J, Deng H-W and Xiao H-M (2023) Enteric nervous system damage caused by abnormal intestinal butyrate metabolism may lead to functional constipation. Front. Microbiol. 14:1117905. doi: 10.3389/fmicb.2023.1117905
Received: 03 January 2023; Accepted: 24 April 2023;
Published: 09 May 2023.
Edited by:
Hsiuying Wang, National Yang Ming Chiao Tung University, TaiwanReviewed by:
Arpita Aditya, University of Maryland, College Park, United StatesCopyright © 2023 Wang, Lv, Yang, Lin, Liu, Tan, Quan, Long, Shen, Shen, Deng and Xiao. This is an open-access article distributed under the terms of the Creative Commons Attribution License (CC BY). The use, distribution or reproduction in other forums is permitted, provided the original author(s) and the copyright owner(s) are credited and that the original publication in this journal is cited, in accordance with accepted academic practice. No use, distribution or reproduction is permitted which does not comply with these terms.
*Correspondence: Hong-Wen Deng, aGRlbmcyQHR1bGFuZS5lZHU=; Hong-Mei Xiao, aG14aWFvQGNzdS5lZHUuY24=
Disclaimer: All claims expressed in this article are solely those of the authors and do not necessarily represent those of their affiliated organizations, or those of the publisher, the editors and the reviewers. Any product that may be evaluated in this article or claim that may be made by its manufacturer is not guaranteed or endorsed by the publisher.
Research integrity at Frontiers
Learn more about the work of our research integrity team to safeguard the quality of each article we publish.