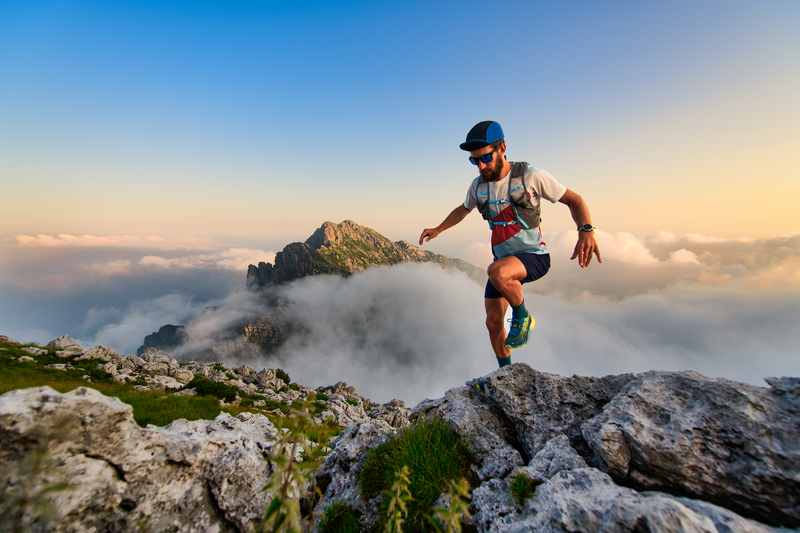
95% of researchers rate our articles as excellent or good
Learn more about the work of our research integrity team to safeguard the quality of each article we publish.
Find out more
ORIGINAL RESEARCH article
Front. Microbiol. , 19 January 2023
Sec. Microbiotechnology
Volume 14 - 2023 | https://doi.org/10.3389/fmicb.2023.1117835
Whisky lactone is a naturally occurring fragrance compound in oak wood and is widely used as a sensory additive in food products. However, safe and efficient methods for the production of its individual enantiomers for applications in the food industry are lacking. The aim of this study was to develop an efficient and highly stereoselective process for the synthesis of individual enantiomeric forms of whisky lactones. The proposed three-step method involves (1) column chromatography separation of a diastereoisomeric mixture of whisky lactone, (2) chemical reduction of cis-and trans-whisky lactones to corresponding syn-and anti-diols, and (3) microbial oxidation of racemic diols to individual enantiomers of whisky lactone. Among various bacteria in the genera Dietzia, Gordonia, Micrococcus, Rhodococcus, and Streptomyces, R. erythropolis DSM44534 and R. erythropolis PCM2150 effectively oxidized anti-and syn-3-methyl-octane-1,4-diols (1a-b) to corresponding enantiomerically pure cis-and trans-whisky lactones, indicating high alcohol dehydrogenase activity. Bio-oxidation catalyzed by whole cells of these strains yielded enantiomerically pure isomers of trans-(+)-(4S,5R) (2a), trans-(−)-(4R,5S) (2b), and cis-(+)-(4R,5R) (2d) whisky lactones. The optical density of bacterial cultures and the impact of the use of acetone powders as catalysts on the course of the reaction were also evaluated. Finally, the application of R. erythropolis DSM44534 in the form of an acetone powder generated the enantiomerically enriched cis-(−)-(4S,5S)-isomer (2c) from the corresponding syn-diol (1b). The newly developed method provides an improved approach for the synthesis of chiral whisky lactones.
Whisky lactone is a crucial ingredient in aged alcoholic beverages, such as whisky, cognac, and brandy (Maga, 1996) and a fragrance ingredient in various foods (e.g., sweet and baked foods) and beverages. It is also used as a repellent against mosquitoes and flies (Suzukt et al., 1992). Whisky lactone was first identified in 1970 by Suomalainen and Nykanen (Suomalainen and Nykanen, 1970) as a single compound in many alcohols in oak barrels; therefore, it is commonly named oak lactone. Masuda and Nishimura (Masuda and Nishimura, 1971) later discovered that two diastereoisomers can be isolated from oak wood species. Then, in 1989, Gunther and Mosandl separated four whisky lactone stereoisomers. Mixtures of isomers have been described as reminiscent of coconut, while cis-isomers have been characterized as woody and earthy and trans-isomers as celery-like. It should be noted that in nature, oak wood contains only trans-(+)-(4S,5R) and cis-(−)-(4S,5S) whisky lactone isomers (Abbott et al., 1995).
Several processes for trans-and cis-isomers of whisky lactone synthesis have been described (Ito et al., 1996; Brenna et al., 2001; Armstrong et al., 2009; Jiang et al., 2010; Pisani et al., 2012; Boratyński et al., 2013, 2014, 2018; Xie et al., 2017). However, these processes are based on multi-stage chemical synthesis using metal catalysts and organic solvents. Despite their wide use, metal-based catalysts are often harmful to the environment. A method for obtaining lactone stereoisomers with non-metallic catalysts (Xie et al., 2017) has also been described; however, the multistep approach was characterized by a relatively low conversion. Therefore, safe methods for the production of each stereoisomers of whisky lactone that fulfill green chemistry requirements are needed. Several biocatalytic pathways lead to optically active stereoisomers of whisky lactone. For example, alcohol dehydrogenase isolated from horse liver (HLADH) enantioselectively oxidizes racemic syn- and anti-3-methyloctane-1,4-diols (Boratyński et al., 2014). Another method uses whole cells of Beauveria bassiana AM278 and Pycnidiella resinae KCH50 for the lactonization of γ-oxo acids (Boratyński et al., 2013). Alternatively, the trans-(+)-(4S,5R) enantiomer of whisky lactone is obtained by reduction of the corresponding γ-oxo acids catalyzed by baker’s yeast (Brenna et al., 2001).
The chirality of chemical compounds is very important in biological processes. In the case of the production of chiral drugs, enantiomers may interact differently with individual metabolic systems (Beck, 2002). Enantiomers can also evoke different aroma sensations or have a different odor intensity (expressed as the odor threshold). Since the properties of individual enantiomers can vary substantially, it is important to develop methods for obtaining enantiomerically pure compounds. One such method is biotransformation involving whole microbial cells (bacteria, yeast, or fungi) or isolated enzymes (Nagy et al., 2006; Marie et al., 2011; Chreptowicz et al., 2016). This method can be used to generate optically active compounds that occur naturally and are difficult to obtain by chemical methods (Braga and Belo, 2016).
The genus Rhodococcus (phylum Actinobacteria) includes Gram-positive, non-motile aerobic bacteria (Alvarez, 2019). Bacteria in this genus have been isolated from soil, groundwater, marine sediments, and diseased and healthy animals and plants (Larkin et al., 2006). Only a few species are pathogenic, e.g., R. equi (a cause of foal pneumonia) and R. fascians (a cause of leafy gall disease). Various Rhodococcus strains have been used as biocatalysts for the degradation of natural organic compounds as well as xenobiotics (Kim et al., 2018). These bacteria show, inter alia, the ability to biodegrade short-and long-chain alkanes and aromatic, heterocyclic, and polycyclic compounds (Larkin et al., 2005). They are characterized by high metabolic diversity, indicating high tolerance against a wide range of substrates and solvents (Liang et al., 2019). For this reason, the use of Rhodococcus strains in the bioremediation of organic pollutants from petroleum, like o-xylene, has been investigated (Kim et al., 2002, 2010). The degradation of lignins via R. jostii RHA1 can lead to the production of vanillin, a valuable flavor compound (Ahmad et al., 2011). Rhodococcus members are also able to carry out the desulfurization reaction and therefore can degrade sulfur-containing compounds found in fossil fuels, like benzothiophene or dibenzothiophene (Khairy et al., 2015). Rhodococcus has a wide range of enzymatic activities and is therefore a biocatalyst of choice in various biotransformation processes involving alcohol dehydrogenases (ADHs), oxidases, monooxygenases, dioxygenases, reductases, etc. (Stampfer et al., 2002; Nikodinovic et al., 2006; Kim et al., 2013; Ewing et al., 2015; Nolte and Urlacher, 2015; Biermann et al., 2016; Müller et al., 2016; Wu and Li, 2018; Sheldon and Brady, 2019).
The aim of this study was to develop a biocatalytic method of obtaining industrially valuable optically active whisky lactones. Established methods are limited and usually characterized by low stereoselectivity. Herein, selected bacterial strains were tested for the bio-oxidation of anti- and syn-3-methyloctane-1,4-diols (1a-b). Two strains of R. erythropolis with high ADH activity stereoselectively catalyzed biotransformation, yielding highly enantioenriched whisky lactone isomers. The newly described method is a cost-efficient strategy for the asymmetric synthesis of each stereoisomer of whisky lactones.
Dietzia maris PCM2292, Gordonia bronchialis PCM2167, Gordonia rubripertincta PCM2144, Micrococcus luteus PCM525, Rhodococcus coprophilus PCM2174, Rhodococcus erythropolis PCM2150, Rhodococcus rhodnii PCM2157, Rhodococcus rhodochrous PCM909, Rhodococcus ruber PCM2166, Rhodococcus ruber PCM2171, Rhodococcus ruber PCM2216, and Streptomyces griseus subsp. griseus PCM2331 were purchased from the Polish Academy of Sciences. Dietzia sp. DSM44016 and Rhodococcus erythropolis DSM44534 were purchased from the German Collection of Microorganisms and Cell Cultures. Biocatalysts were maintained at 4°C on PCM medium agar slants and were then transferred into conical flasks with PCM medium containing sodium chlorine (6 g), glucose (20 g), casein (2 g), bacteriological peptone (10 g), and yeast extract (2 g) dissolved in distilled water (1 L) at 25°C and pH 5.5.
A diastereoisomeric mixture of whisky lactones, nicotinamide adenine dinucleotide (NAD+), nicotinamide adenine dinucleotide phosphate (NADP+), flavin mononucleotide (FMN), glutamate dehydrogenase (GDH), LiAlH4, and PCM medium ingredients was purchased from Sigma-Aldrich Chemical Co. (St. Louis, MO, USA).
A commercially available diastereoisomeric mixture of cis/trans-whisky lactones was separated by column chromatography to obtain individual trans (0.430 g) and cis (0.500 g)-whisky lactones, which were subsequently chemically reduced to corresponding anti-(0.380 g) and syn-3-methyl-octane-1,4-diol (0.456 g) diols (1a-b). This procedure has been described by us in detail (Hernik et al., 2021). The NMR and IR spectra of lactones and diols are as follows:
Trans-whisky lactone (2a-b) 1H NMR (600 MHz, CDCl3) δ: 0.91 (t, J = 7.2 Hz, 3H, CH3-4′); 1.13 (d, J = 6.5 Hz, 3H, CH3-4); 1.32–1.42 (m, 3H, CH2-3′, one of CH2-2′); 1.50 (m, 1H, one of CH2-2′); 1.60 (m, 1H, one of CH2-1′); 1.68 (m, 1H, one of CH2-1′); 2.15–2.25 (m, 2H, one of CH2-3, H-4); 2.66 (m, 1H, one of CH2-3); 4.00 (td, J = 7.9, 4.0 Hz, 1H, H-5); 13C NMR (150 MHz, CDCl3): δ 13.89 (C-4′), 17.49 (CH3-4), 22.49 (C-3′), 27.85 (C-2′), 33.70 (C-1′), 36.08 (C-4), 37.13 (C-3), 87.46 (C-5), 176.61 (C-2); IR (film, cm−1): 1787 (s), 1222 (s), 1187 (s).
Cis-whisky lactone (2c-d) 1H NMR (600 MHz,CDCl3) δ: 0.91 (t, J = 7.3 Hz, 3H, CH3-4′); 1.00 (d, J = 7.0 Hz, 3H, CH3-4); 1.29–1.40 (m, 3H, CH2-3′, one of CH2-2′); 1.45–1.54 (m, 2H, one of CH2-2′, one of CH2-1′); 1.65 (m, 1H, one of CH2-1′); 2.18 (dd, J = 17.0, 4.0 Hz, 1H, one of CH2-3); 2.57 (m, 1H, H-4); 2.67 (dd, J = 17.0, 7.8 Hz, 1H, one of CH2-3); 4.42 (ddd, J = 10.1, 5.6, 4.1 Hz, 1H, H-5); 13C NMR (150 MHz, CDCl3): δ 13.82 (CH3-4), 13.90 (C-4′), 22.51 (C-3′), 28.03 (C-2′), 29.57 (C-4), 33.01 (C-1′), 37.56 (C-3), 83.70 (C-5), 176.94 (C-2); IR (film, cm−1): 1787 (s), 1,219 (m), 1,180 (s).
Anti-3-methyloctane-1,4-diol (1a) 1H NMR (600 MHz,CDCl3) δ: 0.87 (d, J = 6.8 Hz, 3H, CH3-3); 0.89 (t, J = 7.1 Hz, 3H, CH3-8); 1.22–1.36 (m, 3H, one of CH2-6, CH2-7); 1.38–1.46 (m, 3H, CH2-5, one of CH2-6); 1.50 (m, 1H, one of CH2-2); 1.67–1.77 (m, 2H, one of CH2-2, H-3); 2.81 i 3.00 (two s, 2H, 2xOH); 3.55 (m, 1H, H-4); 3.62 (ddd, J = 10.9, 7.1, 5.0 Hz, 1H, one of CH2-1); 3.73 (ddd, J = 10.9, 6.4, 5.0 Hz, 1H, one of CH2-1); 13C NMR (150 MHz, CDCl3) δ: 13.89 (CH3-3), 14.12 (C-8), 22.79 (C-7), 28.70 (C-6), 33.35 (C-5), 35.99 (C-3), 36.20 (C-2), 60.65 (C-1), 74.97 (C-4); IR (film, cm−1): 3342 (s), 1475 (m), 1,395 (m), 1065 (m), 1,018 (m).
Syn-3-methyloctane-1,4-diol (1b) 1H NMR (600 MHz, CDCl3) δ: 0,89 (t, J = 7.1 Hz, 3H, CH3-8); 0.92 (d, J = 6.8 Hz, 3H, CH3-3); 1.23–1.36 (m, 3H, one of CH2-6, CH2-7); 1.37–1.51 (m, 3H, CH2-5, one of CH2-6); 1.56 (m, 1H, one of CH2-2); 1.62–1.70 (m, 2H, one of CH2-2, H-3);3.13 (s, 2H, 2xOH); 3.38 (ddd, J = 8.4, 5.5, 3.3 Hz, 1H, H-4); 3.59 (ddd, J = 11.4, 6.9, 5.1 Hz, 1H, one of CH2-1); 3.72 (ddd, J = 11.4, 6.7, 5.0 Hz, 1H, one of CH2-1); 13C NMR (150 MHz, CDCl3) δ: 14.12 (C-8), 16.60 (CH3-3), 22.81 (C-7), 28.06 (C-6), 34.14 (C-5), 35.26 (C-2), 36.43 (C-3), 60.31 (C-1), 75.82 (C-4); IR (film, cm−1): 3333 (s), 1,480 (s), 1386 (s), 1069 (s), 1018 (s).
Twenty-four well MTPs were sterilized at 121°C and 1 atm. Then, 4 mL of sterile PCM medium was added to each well of the MTP. Holes were inoculated with 0.2 mL of pre-prepared cultures of bacteria at OD600 = 0.3 and shaken (200 rpm) for 24 h at 22°C. Then, 0.002 g of the substrate (anti-3-methyloctane-1,4-diol (1a) or syn-3-methyloctane-1,4-diol (1b)) dissolved in 0.1 mL of acetone was added to every well. For simple extraction, ethyl acetate (0.7 mL) was added to the samples (1 mL) and shaken for 5 min at 200 rpm in 2 mL Eppendorf tubes. The organic phase was transferred to a vial and dehydrated by anhydrous MgSO4. Then, it was filtered through a paper filter to a GC vial. Biotransformation was controlled after 6, 24, and 48 h on the GC. Control experiments were also performed in which microorganisms were cultured on the medium without the addition of substrate to check metabolites.
Forty milliliters of PCM medium were added to 100 mL tapered flasks and then sterilized at 121°C at a pressure of 1 atm. The medium was inoculated with 0.5 mL of pre-culture of bacteria at OD600 = 0.3. The prepared bacterial cultures were placed for 3 days at 22°C and shaken at 150 rpm. Then, 0.01 g of the substrate (anti-3-methyloctane-1,4-diol (1a) or syn-3-methyloctane-1,4-diol (1b)) dissolved in 0.5 mL of acetone water was added to each of the flasks. For simple extraction, ethyl acetate (5 mL) was added to the samples (10 mL) and shaken for 5 min at 200 rpm in Falcon tubes. The organic phase was transferred to a vial and dehydrated by anhydrous MgSO4. Then, it was filtered through a filter paper to a GC vial. Biotransformation was controlled after 3 and 7 days on the GC.
Eighty milliliters of PCM medium were placed in 250 mL Erlenmeyer flask and sterilized at 121°C for 15 min. The medium was inoculated with 5 mL of preprepared cultures of bacteria at OD600 = 0.3. Erlenmeyer flasks with bacterial cultures were placed for 3 days at 22°C and shaken at 150 rpm. After incubation for 3 days, 0.05 g of the substrate (anti-3-methyloctane-1,4-diol (1a) or syn-3-methyloctane-1,4-diol (1b)) dissolved in 2 mL of acetone was added to the culture. Samples were extracted after 24, 48, and 72 h and evaluated by GC.
Five hundred milliliters of a 3-day bacterial culture were centrifuged at 12,000× g for 10 min at 4°C and the medium was separated from the bacterial cells. Cells were suspended in acetone (−20°C), centrifuged at 12,000× g for 10 min at 4°C, and the acetone was removed. This process was repeated three times and finally the cells were dried for 1 h to obtain dry and non-sticky acetone powders.
Twenty-four well MTPs were sterilized at 121°C at a pressure of 1 atm. Then, 0.1 g of acetone powder was added with 3 mL of phosphate buffer (pH = 8.0) to each well of the MTP. Into each well, 0.002 g of NAD+ or NADP+ as coenzymes and 0.004 g of FMN or 0.001 g GDH as coenzyme regeneration agents were added in 0.1 ml of phosphate buffer (pH = 8.0). Subsequently, 0,010 g of the substrate (anti-3-methyloctane-1,4-diol (1a) or syn-3-methyloctane-1,4-diol (1b)) dissolved in 0.1 mL of acetone was added to every well and the MTP was shaken (200 rpm) at 22°C. For simple extraction, ethyl acetate (0.7 mL) was added to the samples (1 mL) and shaken for 5 min at 200 rpm in 2 mL Eppendorf tubes. The organic phase was transferred to a vial and dehydrated by anhydrous MgSO4. Then, it was filtered through a filter paper to a GC vial. Biotransformation was controlled after 6, 18, 42, and 66 h on the GC.
The separation of the diastereoisomeric mixture of cis/trans-whisky lactones and chemical reduction of whisky lactones to corresponding diols were controlled by thin layer chromatography, using aluminum foil plates coated with silica gel. Compounds were detected by spraying the plates with 1% Ce(SO4)2 and 2% H3[P(Mo3O10)4] in 10% H2SO4. Gas chromatography (GC, FID, carrier gas H2) was carried out using the Agilent Technologies 7,890 N (GC System, Santa Clara, CA, USA). Enantiomeric excesses of the products were determined on the chiral column Cyclosil-B (30 m × 0.25 mm × 0.25 μm; Agilent Technologies) according to the following temperature program: 80°C, 160°C (3°C/min), 250°C (20°C/min) (3 min). Samples (2 μL) were injected at a split ratio of 9:1; the carrier gas flow rate was 1 ml/min. The total run time was 34.0 min. Retention times (t) were established as follows: tR = 20.74 min for trans-(+)-(4S,5R) (2a), tR = 21.05 min for trans-(−)-(4R,5S) (2b), tR = 22.42 min for cis-(−)-(4S,5S) (2c), tR = 22.54 min for cis-(+)-(4R,5R) (2d) (Supplementary Figure S1). The substrates were determined on the chiral column CP-Chirasil L-Val (25 m × 0.25 mm × 0.12 μm; Agilent Technologies) according to the following temperature program: 80°C, 165°C (3°C/min), 200°C (20°C/min) (1 min). Samples (2 μL) were injected with a split ratio of 9:1; the flow of carrier gas was 1 mL/min. The total run time was 31.0 min. Retention times (tR) were established as follows: tR = 18.553 for anti-3-methyloctane-1,4-diol (1a), tR = 18.630 min for syn-3-methyloctane-1,4-diol (1b). The structures of the compounds were confirmed on the basis of 1H NMR and 13C NMR, which were recorded for CDCl3 solutions using a Bruker Advance DRX 600 (600 MHz) spectrometer (Billerica, MA, USA). IR spectra were determined using the FTIR Thermo-Mattson IR 300 Spectrometer. Optical rotations were measured on a Jasco P-2000 Polarimeter.
All experiments were performed in triplicate, and mean values are presented. For all comparisons, differences were not significant, as determined by Student’s t-tests. Additionally, the standard deviations were calculated for the conversion rate and percentages of whisky lactone isomers are shown in the tables. Statistical analyses were performed using Past 4.02.
Fourteen bacterial strains in the genera Dietzia, Gordonia, Micrococcus, Rhodococcus, and Streptomyces were selected for screening-scale transformation in microtiter plates (MTPs) to obtain enantiomerically pure trans- and cis-whisky lactones (2a–d) from corresponding diols (1a–b). These microorganisms were chosen on the basis of our previous research focused on the sustainable management of oleo industry by-products in the microbial synthesis of whisky lactones (Hernik et al., 2021). Owing to the modest enantioselectivity and low yield in these previous semi-preparative-scale processes, our goal was to develop an efficient, and highly stereoselective process for the synthesis of individual enantiomeric forms of whisky lactones. Proposed by us three-step method involves (1) column chromatography separation of a diastereoisomeric mixture of whisky lactones, (2) chemical reduction of trans- and cis-whisky lactones (2a-d) (de > 99% determined by GC) with LiAlH4 to give the corresponding syn-and anti-diol, respectively (1a-b) (de > 99% determined by GC), and (3) microbial oxidation of racemic diols to individual enantiomers of whisky lactone (Scheme 1).
Scheme 1. Overview of three steps in the chemo-enzymatic synthesis of individual whisky lactone enantiomers.
Initial biotransformations were carried out in MTPs. This allowed us a rapid selection of microorganisms with high ADH activity, responsible for the stereoselective oxidation of racemic diols to corresponding chiral lactones (Boratyński et al., 2013, 2016, 2020).
In oxidation of anti-3-methyloctane-1,4-diol (1a) the highest enantiomeric excess of trans-(+)-(4S,5R) isomer (2a) (ee = 96%) was detected after 24 h of transformation with Dietzia sp. DSM44016. In bio-oxidation with R. erythropolis DSM44534, R. rhodnii PCM2157, and R. ruber PCM 2166 for 24 h, enantiomeric excesses of trans-(+)-(4S,5R) isomer (2a) were lower (ee = 70–80%). It is worth mentioning that the opposite enantiomerically enriched trans-(−)-(4R, 5S) isomer (2b) (ee = 73%) was detected after 48 h of transformation with R. erythropolis PCM2150. The enantiomerically pure cis-(+)-(4R,5R) isomer (2d) (ee > 99%) was obtained by biotransformation with four strains: Dietzia sp. DSM44016, R. erythropolis DSM44534, R. erythropolis PCM2150, and R. ruber PCM2166. In bio-oxidation with the remaining strains, enantiomeric excesses of trans- and cis-whisky lactones were substantially lower than those for these four strains; using S. griseus subsp. griseus PCM2331, D. maris PCM2292, and R. ruber PCM2216, no conversion was observed (Table 1).
In biotransformations with syn-3-methyloctane-1,4-diol (1b) enantiomerically pure trans-(+)-(4S,5R) lactone (2a) was produced by Dietzia sp. DSM44016 and R. erythropolis PCM2150 after 24 h. On the other hand, enantiomerically pure cis-(+)-(4R,5R) whisky lactone (2d) was obtained with R. erythropolis DSM44534 and R. erythropolis PCM2150. For oxidation with M. luteus PCM525, R. erythropolis DSM44534, and R. rhodnii PCM2157, only cis-isomers of whisky lactone were formed. M. luteus PCM525 delivered cis-(−)-(4S,5S) (2c) with the highest enantiomeric excess (ee = 70%). In biotransformations with S. griseus subsp. griseus PCM2331, R. coprophilus PCM2174, R. rhodochrous PCM909, R. ruber PCM2171, and G. rubripertincta PCM2144, substantially lower enantiomeric excesses were observed than those achieved with other strains. No substrate conversion was detected using D. maris PCM2292 and R. ruber PCM2216 (Table 2).
Surprisingly, the enantiomerically pure or highly enriched trans-and cis-whisky lactones dominantly formed by selected microorganisms: Dietzia sp. DSM44016, R. erythropolis DSM44534, R. erythropolis PCM2150, and R. ruber PCM2166, indicate on dynamic kinetic resolution processes in performed biotransformations. Based on this preliminary results carried out in MTPs with anti-and syn-diols, aforementioned five microorganisms were selected for subsequent analyses.
In the oxidation of anti-3-methyloctane-1,4-diol (1a) with R. erythropolis PCM2150, R. erythropolis DSM44534, and Dietzia sp. DSM44016, complete conversion (conv. = 100%) was observed after 6, 24, and 48 h, respectively. Oxidation with R. erythropolis PCM2150 afforded enantiomerically pure trans-(−)-(4R, 5S) (2b) (25%, ee = 99%) and cis-(+)-(4R,5R) (2d) (75%, ee = 99%) whisky lactone isomers after 48 h. In biotransformations conducted with R. erythropolis DSM44534, enantiomerically pure trans-(−)-(4R, 5S) (2b) (82%, ee = 99%) and cis-(+)-(4R,5R) (2d) (18%, ee = 99%) enantiomers were obtained after 48 h. After 72 h with the same strain, only the trans-(−)-(4R, 5S) (2b) isomer (100%, ee = 99%) was obtained. During transformation with Dietzia sp. DSM44016 after 72 h, enantiomerically enriched trans-(−)-(4R, 5S) (2b) (87%, ee = 54%) and enantiomerically pure cis-(+)-(4R,5R) (13%, ee = 99%) whisky lactone isomer (2d) were detected. In the course of biotransformation with R. erythropolis DSM44534 and Dietzia sp. DSM44016, trans-(+)-(4S,5R) (2a) enantiomer formation was relatively high, while trans-(−)-(4R, 5S) (2b) enantiomer became dominant over time. This trend was consistent with those in biotransformations in MTP, in which the formation of the trans-(+)-(4S, 5R) isomer (2a) in the initial stage was also observed. In addition, during biotransformation with R. erythropolis DSM44534 and R. erythropolis PCM2150, the same enantiomers of whisky lactone formed but in different amounts. Biotransformation with R. ruber PCM2166 showed a lower conversion rate (69%), and enantiomerically enriched trans-(+)-(4S,5R) (2a) (58%, ee = 64%) and cis-(+)-(4R,5R) (2d) (11%, ee = 72%) whisky lactones were acquired. The transformation with R. ruber PCM2157 showed very low conversion (5%) after 72 h (Table 3).
During the biotransformation of syn-3-methyloctane-1,4-diol (1b) with R. erythropolis DSM2150, R. erythropolis DSM44534, and Dietzia sp. DSM44016, the conversion rate was analogous to those for the transformation of anti-diol (1a). With R. erythropolis DSM2150, after 24 h, enantiomerically pure trans-(+)-(4S, 5R) (2a) (31%, ee = 99%) and cis-(+)-(4R,5R) (2d) (69%, ee = 99%) enantiomers of whisky lactone were obtained. After 48 h with the same strain, only the cis-(+)-(4R,5R) (2d) (100%, ee = 99%) isomer was acquired. In bio-oxidation with R. erythropolis DSM44534, after 24 h, only cis-(+)-(4R,5R) (2d) (100%, ee = 99%) whisky lactone was obtained. With Dietzia sp. DSM44016, after 48 h, enantiomerically enriched trans-(+)-(4S, 5R) (2a) (34%, ee = 94%) and cis-(+)-(4R,5R) (2d) (66%, ee = 97%) isomers formed. In biotransformation with R. ruber PCM2166, after 72 h, enantiomerically pure trans-(+)-(4S, 5R) (2a) enantiomer (11%, ee = 99%) and enantiomerically enriched cis-(+)-(4R,5R) (2d) (66%, ee = 97%) whisky lactone were obtained. Biotransformation with M. luteus PCM525 and R. ruber PCM2157 showed low conversion rates, while no conversion was observed with G. bronchialis PCM2167 (Table 4).
Based on preliminary screening experiments for the preparative biotransformation of anti- and syn-3-methyl-octane-1,4-diols (1a–b) R. erythropolis DSM2150 and R. erythropolis DSM44534 were selected.
The effect of the biomass concentration based on optical density (OD600 = 0.3, 0.5, and 1.0) during the course of biotransformation was evaluated. In a comparison of results obtained after 24, 48, and 72 h, no significant differences were detected in the enantiomeric excess of lactones and the conversion of diols. For this reason, preparative biotransformations were performed at OD600 = 1.0.
During biotransformation with anti-3-methyloctane-1,4-diol (1a), the time needed for conversion was substantially longer than that for biotransformation at a smaller scale. Moreover, the formation of byproducts was observed. In bio-oxidation with R. erythropolis DSM44534, after 144 h, enantiomerically pure trans-(−)-(4R,5S) (2b) ( = −96.3 (c = 0.25, CH3OH, ee = 99%, yield = 22%); ref. = −97.0 (c = 0.34, CH3OH, ee = 99%) (Wilkinson et al., 2004)) and cis-(+)-(4R,5R) (2d) ( = +79.4 (c = 0.2, CH3OH, ee = 99%, yield = 8%); ref. = +79.0 (c = 0.5, CH3OH, ee = 99%) (Wilkinson et al., 2004)) whisky lactones were detected (Table 5).
The conversion of syn-3-methyloctane-1,4-diol (1b) was fastest during bio-oxidation with R. erythropolis PCM2150 (after 18 h). With R. erythropolis DSM44534, complete conversion required 42 h. As a result of biotransformation catalyzed by R. erythropolis PCM2150, after 42 h, enantiomerically enriched trans-(+)-(4S,5R) (2a) ( = +98.1 (c = 0.2, CH3OH, ee = 97%, yield = 14%); ref. = +97.0 (c = 0.34, CH3OH, ee = 99%) (Wilkinson et al., 2004)) and optically pure cis-(+)-(4R,5R) (2d) ( = +78.1 (c = 0.15, CH3OH, ee = 99%, yield = 60%); ref. = +79.0 (c = 0.5, CH3OH, ee = 99%) (Wilkinson et al., 2004)) whisky lactones were obtained. During bio-oxidation with R. erythropolis PCM44534, after 42 h, enantiomerically enriched trans-(+)-(4S,5R) (2a) ( = +94.8 (c = 0.1, CH3OH, ee = 90%, yield = 28%); ref. = +97.0 (c = 0.34, CH3OH, ee = 99%) and pure cis-(+)-(4R,5R) (2d) ( = +79.9 (c = 0.2, CH3OH, ee = 99%, yield = 40%); ref. = +79.0 (c = 0.5, CH3OH, ee = 99%) (Wilkinson et al., 2004)) whisky lactones were obtained (Table 6).
By comparing the results from the submerged preparative biotransformations described herein with our previous results obtained by solid-state fermentation (Boratyński et al., 2020), processes conducted in the SmF were characterized by the substantially greater and faster conversion of diols to corresponding whisky lactones. Moreover, the bio-oxidation carried out in SmF afforded enantiomerically pure lactones on a preparative scale, which could not be obtained in preparative SSF biotransformations. Among fourteen bacteria tested, bio-oxidation by R. erythropolis DSM44534 and R. erythropolis PCM2150 showed the highest efficiency and stereoselectivity, yielding trans-(+)-(4S,5R) (2a), trans-(−)-(4R,5S) (2b) and cis-(+)-(4R,5R) (2d) whisky lactones.
In our previous study, whisky lactone enantiomers were obtained by the microbial whole-cell reduction of γ-oxoacids (Boratyński et al., 2013). The trans-(+)-(4S,5R) (2a) enantiomer was obtained (ee = 99%) as the only product of the biotransformation catalyzed by Didymosphaeria igniaria KCH6651, Laetiporus sulphurens AM525, Chaetomium sp. KCH6670, and Saccharomyces cerevisiae AM464. However, during the biotransformation of the same γ-oxoacid by Beauveria bassiana AM278 and Pycnidiella resinae KCH50, a mixture of trans-(+)-(4S, 5R) (2a) (ee = 99%) and cis-(−)-(4S,5S) (2c) (ee = 45–77%) isomers was obtained. During enzymatic reactions catalyzed by the alcohol dehydrogenases HLADH and PADH I, enantiomerically enriched trans-(−)-(4R, 5S) (2b) and cis-(+)-(4R,5R) (2d) isomers were obtained (ee = 27–82%). In a previously described method of obtaining the trans-(+)-(4S, 5R) (2a) enantiomer (ee = 99%) by lactonization biocatalyzed by baker’s yeast, the efficiency on a preparative scale was 38% (Brenna et al., 2001). Compared to these results, trans-(+)-(4S,5R) (2a), trans-(−)-(4R,5S) (2b), and cis-(+)-(4R,5R) (2d) enantiomers of whisky lactone (ee = 97–99%) were obtained by the newly developed approach.
Since the enantiomerically pure cis-(−)-(4S,5S) (2c) whisky lactone was not obtained, oxidation was carried out with acetone powders prepared from selected bacteria (Dietzia sp. DSM44016, R. erythropolis DSM44534, and R. erythropolis PCM2150). For enzymatic transformation, NAD+ and NADP+ as coenzymes and FMN and GDH for coenzyme regeneration were selected. Only NADP+ and GDH were suitable for biotransformations (Schenkels and Duine, 2000).
In all biotransformations of anti-3-methyloctane-1,4-diol (1a), only trans-whisky lactone isomers formed. In bio-oxidation with acetone powders from Dietzia sp. DSM44016, after 66 h, trans-(+)-(4S,5R) (2a) whisky lactone was detected (ee = 74%) with 100% conversion. Transformation with acetone powder from R. erythropolis DSM44534 after 42 h led to the trans-whisky lactone isomer trans-(−)-(4R,5S) (2b) (ee = 87%) with 85% conversion. It is worth noting that when using acetone powders from Dietzia sp. DSM44016 and R. erythropolis PCM2150, during the biotransformation of anti-diol (1a), an opposite trans-(+)-(4S,5R) (2a) isomer formed in the screening-scale whole-cell biotransformations. In transformations of syn-3-methyloctane-1,4-diol (1a) with acetone powders from Dietzia sp. DSM44016, R. erythropolis DSM44534, and R. erythropolis PCM2150, conversion was complete after 66 h. Oxidation with R. erythropolis DSM44534 acetone powders after 42 h afforded the highest enantiomeric excess (ee = 86%) of cis-(−)-(4S,5S) (2c) isomer, instead of cis-(+)-(4R,5R) (2d), which was obtained in whole-cell biotransformations with these bacteria. Biotransformations with acetone powders were characterized by lower and slower conversion than those in screening-scale bio-oxidation involving whole cells from the same strains. Additionally, in transformations with acetone powders, only one isomer always formed; when anti-diol (1a) was added as substrate, trans-whisky lactone formed, while syn-diol (1b) produced cis-whisky lactone. The application of acetone powders in biotransformations is often used to increase the stability of enzymes and improve enantioselectivity. Additionally, these biocatalysts frequently increase the yield and benefit from simple storage and use. For instance, the use of acetone powders from Geotrichum candidum by Nakamura and Madsuda (Nakamura and Matsuda, 1998) increased the enantioselectivity and efficiency of the reduction of ketones to alcohols and generated products with the opposite configuration. However, we did not observe a benefit of this biocatalyst over whole cell oxidation.
A chemo-enzymatic three-step method for obtaining whisky lactone isomers was developed. This method combined the separation of a diastereoisomeric mixture of whisky lactone isomers by column chromatography followed by chemical reduction to corresponding racemic diols. The latter are submitted to microbial oxidation to obtain each stereoisomer of whisky lactone. Among bacteria from different genera, R. erythropolis DSM44534 and R. erythropolis PCM2150 effectively oxidized anti- and syn-3-methyloctane-1,4-diols (1a-b) to corresponding whisky lactones, indicating high ADH activity. Bio-oxidation carried out on a preparative scale yielded enantiomerically pure isomers of trans-(+)-(4S,5R) (2a), trans-(−)-(4R,5S) (2b) and cis-(+)-(4R,5R) (2d) whisky lactones. In addition, it was developed that acetone powders prepared from selected bacteria could be used to generate enantiomerically enriched cis-(−)-(4S,5S) (2c) whisky lactone isomers, although the reactions were characterized by lower conversion. Based on the obtained results, it was noticed that the dynamic kinetic resolution processes are probably involved in the described whole cells transformations. Therefore, further studies are currently ongoing on wider portfolio of substrates to confirm the mechanism that occurs during this process.
The original contributions presented in the study are included in the article/Supplementary material, further inquiries can be directed to the corresponding authors.
DH and FB: conceptualization, formal analysis, methodology, resources, and writing – original draft. DH: funding acquisition and investigation. FB and EB: supervision. DH and ES: visualization. TO, EB, and FG: writing – review and editing. All authors have read and agreed to the published version of the manuscript.
This research and APC were funded by the project “UPWR 2.0: International and Interdisciplinary Program of Development of Wrocław University of Environmental and Life Sciences,” co-financed by the European Social Fund under the Operational Program Knowledge Education Development, under contract No. POWR.03.05.00-00-Z062/18 of 4 June 2019.
We would like to thank Editage for providing the editing service.
The authors declare that the research was conducted in the absence of any commercial or financial relationships that could be construed as a potential conflict of interest.
All claims expressed in this article are solely those of the authors and do not necessarily represent those of their affiliated organizations, or those of the publisher, the editors and the reviewers. Any product that may be evaluated in this article, or claim that may be made by its manufacturer, is not guaranteed or endorsed by the publisher.
The Supplementary material for this article can be found online at: https://www.frontiersin.org/articles/10.3389/fmicb.2023.1117835/full#supplementary-material
Abbott, N., Puech, J. L., Bayonove, C., and Baumes, R. (1995). Determination of the aroma threshold of the cis and trans racemic forms of b-methyl-c-octalactone by gas chromatography-sniffing analysis. Am. J. Enol. Vitic. 46, 292–294.
Ahmad, M., Roberts, J. N., Hardiman, E. M., Singh, R., Eltis, L. D., and Bugg, T. D. H. (2011). Identification of DypB from Rhodococcus jostii RHA1 as a lignin peroxidase. Biochemistry 50, 5096–5107. doi: 10.1021/bi101892z
Armstrong, A., Ashraff, C., Chung, H., and Murtagh, L. (2009). Oxidative rearrangement of 2-alkoxy-3,4-dihydro-2H-pyrans: Stereocontrolled synthesis of 4,5-cis-disubstituted tetrahydrofuranones including whisky and cognac lactones and crobarbatic acid. Tetrahedron 65, 4490–4504. doi: 10.1016/j.tet.2009.04.013
Beck, G. (2002). Synthesis of chiral drug substances. Synlett 2002, 0837–0850. doi: 10.1055/s-2002-31890
Biermann, M., Gruß, H., Hummel, W., and Gröger, H. (2016). Guerbet alcohols: from processes under harsh conditions to synthesis at room temperature under ambient pressure. ChemCatChem 8, 895–899. doi: 10.1002/cctc.201501241
Boratyński, F., Dancewicz, K., Paprocka, M., Gabryś, B., and Wawrzeńczyk, C. (2016). Chemo-enzymatic synthesis of optically active γ-and δ-decalactones and their effect on aphid probing, feeding and settling behavior. PLoS One 11:e0146160. doi: 10.1371/journal.pone.0146160
Boratyński, F., Pannek, J., Walczak, P., Janik-Polanowicz, A., Huszcza, E., Szczepańska, E., et al. (2014). Microbial alcohol dehydrogenase screening for enantiopure lactone synthesis: down-stream process from microtiter plate to bench bioreactor. Process Biochem. 49, 1637–1646. doi: 10.1016/j.procbio.2014.06.019
Boratyński, F., Smuga, M., and Wawrzeńczyk, C. (2013). Lactones 42. Stereoselective enzymatic/microbial synthesis of optically active isomers of whisky lactone. Food Chem. 141, 419–427. doi: 10.1016/j.foodchem.2013.02.106
Boratyński, F., Szczepańska, E., De Simeis, D., Serra, S., and Brenna, E. (2020). Bacterial biotransformation of oleic acid: new findings on the formation of γ-dodecalactone and 10-ketostearic acid in the culture of Micrococcus luteus. Molecules 25:3024. doi: 10.3390/molecules25133024
Boratyński, F., Szczepańska, E., Grudniewska, A., Skalny, B., and Olejniczak, T. (2018). A novel approach for microbial synthesis of enantiomerically pure whisky lactones based on solid-state fermentation. Molecules 23:659. doi: 10.3390/molecules23030659
Braga, A., and Belo, I. (2016). Biotechnological production of gamma-decalactone, a peach like aroma, by Yarrowia lipolytica. World J. Microbiol. Biotechnol. 32:169. doi: 10.1007/s11274-016-2116-2
Brenna, E., Dei Negri, C., Fuganti, C., and Serra, S. (2001). Baker's yeast-mediated approach to (−)-cis-and (+)-trans-Aerangis lactones. Tetrahedron Asymmetry 12, 1871–1879. doi: 10.1016/S0957-4166(01)00314-7
Chreptowicz, K., Wielechowska, M., Główczyk-Zubek, J., Rybak, E., and Mierzejewska, J. (2016). Production of natural 2-phenylethanol: from biotransformation to purified product. Food Bioprod. Process. 100, 275–281. doi: 10.1016/j.fbp.2016.07.011
Ewing, T. A., Fraaije, M. W., and van Berkel, W. J. H. (2015). “Oxidation using alcohol oxidases” in Biocatalysis in Organic Synthesis 3. eds. K. Faber, W.-D. Fessner, and N. J. Turner (Stuttgart: Georg Thieme Verlag KG), 157–186.
Hernik, D., Pannek, J., Szczepańska, E., Olejniczak, T., and Boratyński, F. (2021). Bacterial whole cells synthesis of whisky lactones in a solid-state fermentation bioreactor prototype. Catalysts 11:320. doi: 10.3390/catal11030320
Ito, K., Yoshitake, M., and Katsuki, T. (1996). Chiral bipyrindine and biquinoline ligands: their asymmetric synthesis and application to the synthesis of trans-whisky lactone. Tetrahedron 52, 3905–3920. doi: 10.1016/S0040-4020(96)00058-0
Jiang, X., Fu, C., and Ma, S. (2010). A concise synthesis of (−)- and (+)-trans-whisky lactones. Eur. J. Org. Chem. 2010, 687–693. doi: 10.1002/ejoc.200901058
Khairy, H., Wübbeler, J. H., and Steinbüchel, A. (2015). Biodegradation of the organic disulfide 4,40-Dithiodibutyric acid by Rhodococcus spp. Appl. Environ. Microbiol. 81, 8294–8306. doi: 10.1128/AEM.02059-15
Kim, D., Choi, K. Y., Yoo, M., Zylstra, G. J., and Kim, E. (2018). Biotechnological potential of Rhodococcus biodegradative pathways. J. Microbiol. Biotechnol. 28, 1037–1051. doi: 10.4014/jmb.1712.12017
Kim, S. H., Han, H. Y., Lee, Y. J., Kim, C. W., and Yang, J. W. (2010). Effect of electrokinetic remediation on indigenous microbial activity and community within diesel contaminated soil. Sci. Total Environ. 408, 3162–3168. doi: 10.1016/j.scitotenv.2010.03.038
Kim, D., Kim, Y. S., Kim, S. K., Kim, S. W., Zylstra, G. J., Kim, Y. M., et al. (2002). Monocyclic aromatic hydrocarbon degradation by Rhodococcus sp. strain DK17. Appl. Environ. Microbiol. 68, 3270–3278. doi: 10.1128/AEM.68.7.3270-3278.2002
Kim, D., Yoo, M., Choi, K. Y., Kang, B. S., and Kim, E. (2013). Characterization and engineering of an o-xylene dioxygenase for biocatalytic applications. Bioresour. Technol. 145, 123–127. doi: 10.1016/j.biortech.2013.03.034
Larkin, M. J., Kulakov, L. A., and Allen, C. C. R. (2005). Biodegradation and Rhodococcus—Masters of catabolic versatility. Curr. Opin. Biotechnol. 16, 282–290. doi: 10.1016/j.copbio.2005.04.007
Larkin, M. J., Kulakov, L. A., and Allen, C. C. R. (2006). Biodegradation by members of the genus Rhodococcus: biochemistry, physiology, and genetic adaptation. Adv. Appl. Microbiol. 59, 1–29. doi: 10.1016/S0065-2164(06)59001-X
Liang, Y., Jiao, S., Wang, M., Yu, H., and Shen, Z. (2019). Overexpression of epoxide hydrolase in Rhodococcus ruber with high robustness for the synthesis of chiral epichlorohydrin. Process Biochem. 79, 49–56. doi: 10.1016/j.procbio.2018.12.023
Maga, J. A. (1996). Oak lactones in alcoholic beverages. Food Rev. Int. 12, 105–130. doi: 10.1080/87559129609541069
Marie, L., Gori, K., Agerlin, M., Jespersen, L., and Arneborg, N. (2011). Flavour compound production by Yarrowia lipolytica, Saccharomyces cerevisiae and Debaryomyces hansenii in a cheese-surface model. Int. Dairy J. 21, 970–978. doi: 10.1016/j.idairyj.2011.06.005
Masuda, M., and Nishimura, K. (1971). Branched nonalactones from some Quercus species. Phytochemistry 10, 1401–1402. doi: 10.1016/S0031-9422(00)84355-1
Müller, C. A., Weingartner, A. M., Dennig, A., Ru, A. J., Gröger, H., and Schwaneberg, U. (2016). A whole cell biocatalyst for double oxidation of cyclooctane. J. Ind. Microbiol. Biotechnol. 43, 1641–1646. doi: 10.1007/s10295-016-1844-5
Nagy, V., Toke, E. R., Keong, L. C., Szatzker, G., Ibrahim, D., Omar, I. C., et al. (2006). Kinetic resolutions with novel, highly enantioselective fungal lipases produced by solid state fermentation. J. Mol. Catal. B Enzym. 39, 141–148. doi: 10.1016/j.molcatb.2006.01.012
Nakamura, K., and Matsuda, T. (1998). Asymmetric reduction of ketones by the acetone powder of Geotrichum candidum. J. Org. Chem. 63, 8957–8964. doi: 10.1021/jo9812779
Nikodinovic, J., Dinges, J. M., Bergmeier, S. C., McMills, M. C., Wright, D. L., and Priestley, N. D. (2006). Resolution of methyl nonactate by Rhodococcus erythropolis under aerobic and anaerobic conditions. Org. Lett. 8, 443–445. doi: 10.1021/ol052739p35
Nolte, J. C., and Urlacher, V. B. (2015). “Cytochrome P450 in the oxidation of alkenes” in Biocatalysis in Organic Synthesis 3. eds. K. Faber, W.-D. Fessner, and N. J. Turner (Stuttgart: Georg Thieme Verlag KG), 21–63.
Pisani, L., Superchi, S., D’Elia, A., Scafato, P., and Rosini, C. (2012). Synthetic approach toward cis-disubstituted γ-and δ-lactones through enantioselective dialkylzinc addition to aldehydes: application to the synthesis of optically active flavors and fragrances. Tetrahedron 68, 5779–5784. doi: 10.1016/j.tet.2012.05.028
Schenkels, P., and Duine, J. A. (2000). Nicotinoprotein (NADH-containing) alcohol dehydrogenase from Rhodococcus erythropolis DSM 1069: an effcient catalyst for coenzyme-independent oxidation of a broad spectrum of alcohols and the interconversion of alcohols and aldehydes. Microbiology 146, 775–785. doi: 10.1099/00221287-146-4-775
Sheldon, R. A., and Brady, D. (2019). Broadening the scope of biocatalysis in sustainable organic synthesis. ChemSusChem 12, 2859–2881. doi: 10.1002/cssc.201900351
Stampfer, W., Kosjek, B., Moitzi, C., Kroutil, W., and Faber, K. (2002). Biocatalytic asymmetric hydrogen transfer. Angew. Chem. Int. Ed. 41, 1014–1017. doi: 10.1002/1521-3773(20020315)41:6<1014::aid-anie1014>3.0.co;2-6
Suomalainen, H., and Nykanen, L. (1970). Investigation into the aroma of alcoholic beverages. Naeringsmiddelindustrien 23, 15–30.
Suzukt, Y., Mori, W., Ishizone, H., Naito, K., and Honda, T. (1992). Concise enantiospecific syntheses of (+)-Eldanolide and (−)-cis-whisky lactone. Tetrahedron Lett. 33, 4931–4932. doi: 10.1016/S0040-4039(00)61237-6
Wilkinson, K. L., Elsey, G. M., Prager, R. H., Tanaka, T., and Sefon, M. A. (2004). Precursors to oak lactone. Part 2: synthesis, separation and cleavage of several β-D-glucopyranosides of 3-methyl-4-hydroxyoctanoic acid. Tetrahedron 60, 6091–6100. doi: 10.1016/j.tet.2004.05.070
Wu, S., and Li, Z. (2018). Whole-cell cascade biotransformations for one-pot multistep organic synthesis. ChemCatChem 10, 2164–2178. doi: 10.1002/cctc.201701669
Keywords: biotransformation, fragrances, Rhodococcus erythropolis, lactones, oxidation, enantioselectivity
Citation: Hernik D, Gatti F, Brenna E, Szczepańska E, Olejniczak T and Boratyński F (2023) Stereoselective synthesis of whisky lactone isomers catalyzed by bacteria in the genus Rhodococcus. Front. Microbiol. 14:1117835. doi: 10.3389/fmicb.2023.1117835
Received: 06 December 2022; Accepted: 03 January 2023;
Published: 19 January 2023.
Edited by:
Deniz Yildirim, Çukurova University, TürkiyeCopyright © 2023 Hernik, Gatti, Brenna, Szczepańska, Olejniczak and Boratyński. This is an open-access article distributed under the terms of the Creative Commons Attribution License (CC BY). The use, distribution or reproduction in other forums is permitted, provided the original author(s) and the copyright owner(s) are credited and that the original publication in this journal is cited, in accordance with accepted academic practice. No use, distribution or reproduction is permitted which does not comply with these terms.
*Correspondence: Dawid Hernik, ✉ ZGF3aWQuaGVybmlrQHVwd3IuZWR1LnBs; Filip Boratyński, ✉ ZmlsaXAuYm9yYXR5bnNraUB1cHdyLmVkdS5wbA==
Disclaimer: All claims expressed in this article are solely those of the authors and do not necessarily represent those of their affiliated organizations, or those of the publisher, the editors and the reviewers. Any product that may be evaluated in this article or claim that may be made by its manufacturer is not guaranteed or endorsed by the publisher.
Research integrity at Frontiers
Learn more about the work of our research integrity team to safeguard the quality of each article we publish.