- 1State Key Laboratory of Animal Nutrition, Institute of Animal Sciences, Chinese Academy of Agricultural Sciences, Beijing, China
- 2College of Animal Sciences and Technology, Huazhong Agricultural University, Wuhan, China
- 3MoE Key Laboratory of Molecular Animal Nutrition, College of Animal Sciences, Zhejiang University, Hangzhou, China
Biochanin A (BCA), an isoflavone phytoestrogen, is a secondary metabolite produced mainly in leguminous plants. The objective of this study was to evaluate the effect of BCA on lactation performance, nitrogen metabolism, and the health of dairy goat. Thirty mid-lactation Saanen dairy goats were divided into three groups randomly: control, 2 g/d BCA group, and 6 g/d BCA group. After 36 days of feeding, 30 dairy goats were transferred to individual metabolic cages. Subsequently, milk yield, feed intake, total feces, and urine excretion were recorded and samples were collected continuously for 3 days. Blood and ruminal fluid samples were collected over the subsequent 4 days. Milk yield, milk protein, fat content, and the feed conversion ratio of dairy goat were significantly increased by the BCA treatment. The levels of serum 17β-estradiol, growth hormone, insulin-like growth factor 1, glutathione peroxidase activity, and total antioxidant capacity were also increased significantly by BCA, indicating that BCA enhanced the antioxidant capacity of dairy goat. Amino acid degradation was significantly inhibited, while the ammonia nitrogen content was reduced significantly by BCA. Total volatile fatty acids was significantly increased by BCA supplementation. In addition, the relative abundance of Verrucomicrobiota was decreased significantly. However, the growth of nitrogen metabolism and cellulolytic bacteria was significantly increased under BCA treatment, including Prevotella sp., Treponema sp., Ruminococcus flavefaciens, and Ruminobacter amylophilus. In conclusion, supplementation with BCA improved the milk production performance, nitrogen metabolism, rumen fermentation and antioxidant capacity, and regulated the rumen microbiome of dairy goat.
Introduction
Biochanin A (BCA), an isoflavone phytoestrogen with various functions (Wenjin et al., 2021), is a secondary metabolite produced mainly in leguminous plants such as red clover and chickpea (Křížová et al., 2019). The BCA content in red clover (Trifolium pratense L.) leaves during the flowering stage ranges from 4.57 to 6.86 mg/g dry matter (DM), with an average concentration of 5.76 mg/g DM, which accounts for approximately 40% of the total isoflavones (Lemeziene et al., 2015). Chickpea (Cicer arietinum L.) sprouts contain lower concentration of BCA than red clover, with a maximum concentration of 2.34 mg/g DM (Gao et al., 2015). Sonication of peanuts (Arachis hypogaea) in 80% aqueous ethanol yielded a BCA content of 0.964 mg/100 g DM (Chukwumah et al., 2009). Young plants of Astragalus glycyphyllos L. and Astragalus cicer L. had BCA contents of 8.81 and 11.4 mg/100 g DM, respectively (Butkute et al., 2018). Other natural sources of BCA include alfalfa (Kagan et al., 2015) and soybeans (Hu et al., 2021). Recent studies have demonstrated that BCA possesses numerous biological properties, such as anti-inflammatory, anti-microbial, and antioxidant activities (Jalaludeen et al., 2016; Sadri et al., 2017). Furthermore, BCA has been shown to be a potential drug for protection against osteoporosis (Lee and Choi, 2005), prevention and treatment of atherosclerotic cardiovascular disease (Yu et al., 2020), and alleviation of symptoms in postmenopausal women (Galal et al., 2018). Therefore, BCA is an important source of novel health foods and natural products.
Hyper-ammonia-producing bacteria (HAB) play a vital role in amino acid (AA) deamination in the rumen (Flythe and Kagan, 2010). In vitro, BCA was shown to possess antimicrobial activity against HAB isolated from the rumen fluid of bovines and caprines; however, it has to act synergistically with other heat-stable antibacterial compounds to inhibit HAB growth (Flythe and Kagan, 2010; Flythe et al., 2013). In addition, BCA exhibited selective antimicrobial activity against amylolytic and cellulolytic bacteria, and increased Lactobacilli, decreased gram-positive cocci, and directly inhibited the growth of Fibrobacter succinogenes S85, Ruminococcus flavefaciens 8, and Ruminococcus albus 8 (Harlow et al., 2017b,2018). These results were attributed to the enhancement of the activities of native rumen antimicrobials (e.g., bacteriocins) by BCA (Flythe et al., 2013; Harlow et al., 2017b). Studies have also shown that BCA can inhibit lactic acid production and increase lactate-utilizing bacteria and lactic acid metabolism, thereby ameliorating pH decline, promoting rumen fermentation, and increasing the concentrations of acetate, propionate, and total volatile fatty acids (VFAs) (Harlow et al., 2017b,2018). Furthermore, BCA could be a substitute for antibiotics, such as monensin, for reducing rumen acidosis (Harlow et al., 2017c,2021). Supplementing steers fed dried distillers’ grains (DDG) with BCA improved crude protein (CP) digestibility of DDG and increased average daily gain, as the addition of BCA decreased ammonia production and improved the quality of absorbed protein by inhibiting HAB (Flythe et al., 2013; Harlow et al., 2017a). Similar results were observed with red clover whereby the average daily gain depended on the interaction between BCA and other isoflavones (Harlow et al., 2020).
In our lab, it was observed that BCA inhibited the activity of proteolytic and ureolytic bacteria and improved the efficiency of microbial protein synthesis (Liu et al., 2020). Therefore, BCA is hypothesized to be a potential novel urease inhibitor. However, to date, there is limited information on the in vivo effects of BCA on dairy livestock. Therefore, the objective of this study was to evaluate the effects of BCA on production performance, health, and rumen microbiome of dairy goats.
Materials and methods
Animal management
Animal experiments were approved by the Animal Care and Use Committee of the Institute of Animal Science of the Chinese Academy of Agricultural Sciences (approval no. IAS2020-97). Thirty mid-lactation health Saanen dairy goats (body weights of 63.5 ± 5.6 kg at 147 ± 3 d of lactation) from Weihe Dairy Farm (Qiangyang, China) were randomly divided into three groups (n = 10): basal diet group (control), basal diet with 2 g/d BCA per goat group (BL), and basal diet with 6 g/d BCA per goat group (BH). To make sure the 6 g/d group indeed ingested 6 g/d BCA a day per goat, BCA was mixed with a small amount of basic diet and fed to each goat separately every morning. After each goat had finished all the basic diet with BCA, the left diet would be given to them. The doses used were based on previous report (Harlow et al., 2017c). The duration of the experiment was 50 d in autumn (September–November), with 43 d permitted for diet acclimatization and 7 d allocated for sample collection. The dairy goats were placed in individual metabolic cages on day 36 of the acclimatization period. The goats were fed, ad libitum, a basal diet and total mixed diet (TMR), and were allowed approximately 5% orts twice a day at 08:00 and 14:30. Dairy goats were milked twice daily, at 07:00 and 18:00. The dietary ingredients (% DM) and chemical composition (% DM) of TMR are shown in Table 1.
Sample collection
During days 44–46 of the experimental period, the feed intake of each goat was recorded daily. Samples of TMR and orts were collected and stored at –20°C until further analysis. The dairy milk yields obtained from all the goats were recorded from day 44 to 46 of the experimental period. Milk samples were collected at 07:00 and 18:00 and mixed at a ratio of 1:1. A 40 mL subsample of milk was transferred to vials preserved with 2-bromo-2-nitropropane-1-3-diol and stored at 4°C for composition analysis. Approximately 80 mL subsamples, without any treatment, were stored in two 50 mL centrifuge tubes at –20°C. Urine and fecal samples were collected daily from days 44 to 46 of the experimental period. The urine was collected in a plastic container containing 200 mL of diluted sulfuric acid which was used to maintain the pH at <3. Urine volumes were recorded daily, with 5% of the urine samples stored in vials. Urine samples from each goat were mixed for three consecutive days and stored at –20°C until nitrogen balance and purine derivative analysis were performed. The total fecal output of each goat was collected into large metal nets that were placed under the metabolic cages and weighed daily. A 5% aliquot of each goat feces was transferred into self-sealed plastic bags daily, mixed for three consecutive days, and stored at –20°C until chemical analysis was performed.
On days 47 and 48 of the experimental period, approximately 1 h after the morning feed, blood samples were collected from the jugular vein using 10 mL vacutainer tubes containing sodium heparin. The tubes were immediately centrifuged at 3,500 × g at 4°C for 15 min to separate the plasma (Mendowski et al., 2020). The plasma samples were transferred to 2 mL centrifuge tubes to determine the plasma antioxidant capacity, biochemical parameters, and endocrine indices. On days 49 and 50, rumen fluid samples were collected through the ruminal cannula via suction using a hose 1 h after the morning feed (Mendowski et al., 2020). Rumen fluid was filtered through four layers of cheesecloth, and the pH was determined immediately. A 10 mL subsample of ruminal fluid was acidified immediately using 2 mL of metaphosphoric acid (25%, m/v) to determine the VFA, and ammonia-nitrogen (NH3-N) contents. A 40 mL subsample without metaphosphoric acid was collected into a 50 mL centrifuge tube and stored at –20°C for ruminal bacterial diversity analysis. All the samples of every goat and data point was included in the analysis.
Sample analysis
Samples of TMR, orts, and feces were dried at 65°C in a forced-air oven to a constant weight to measure the DM, CP, ether extract, acid detergent fiber (ADF), and neutral detergent fiber (NDF) contents by wet chemistry. The DM was determined using a forced-air oven (AOAC method 930.15). The nitrogen content was determined using a Kjeldahl auto-analyzer (VELP DK20, Usmate, Italy), and the CP was calculated as N × 6.25 (AOAC method 990.03). The ether extract content was determined using the AOAC International method 920.39 (AOAC method 920.39). The ADF and NDF contents were measured according to a previously described method (Van Soest et al., 1991). Milk subsamples preserved in 2-bromo-2-nitropropane-1-3-diol were analyzed for true protein, fat, lactose, and total milk solids using infrared spectroscopy (Foss FT 120, Denmark). The nitrogen content in the milk, fecal, and urine samples was determined using the Kjeldahl method (AOAC method 990.39). The NH3-N concentration in rumen fluids and urine was measured using a phenol-hypochlorite assay. The rumen AA contents were determined as previously described (Liu et al., 2020). In the rumen fluid preserved with metaphosphoric acid, the VFA content was analyzed using gas chromatography (Agilent 7890A, USA). The allantoin, xanthine, uric acid, and hypoxanthine contents in the urine samples were measured as previously described (Chen and Gomes, 1992).
The total protein, albumin, globulin, creatinine, and β-hydroxybutyric acid content and the activities of alanine and aspartate aminotransferase in the plasma of dairy goats were analyzed using a Beckman AU680 Automatic Biochemical Analyzer (Beckman Coulter Inc., FL, USA). The total AA content, NH3 concentration, glutathione peroxidase (GSH-Px), superoxide dismutase (SOD), catalase, and total antioxidant capacity (T-AOC) in plasma were quantified using total AA (A026-1-1), blood ammonia (A086-1), GSH-Px (A005-1), SOD (A001-3-2), catalase (A007-1-1), and T-AOC (A01502-1) assay kits from Nanjing Jiancheng Bioengineering Institute (Nanjing, China), respectively. The levels of growth hormone, prolactin, estradiol, and insulin in plasma were determined using a DFM-96 Gamma Radioimmunoassay Counter (Zhongheng Electromechanical Technology Development Co., Ltd, Hefei, China). Insulin-like growth factor 1 (IGF-1) was determined using a bovine IGF-1 ELISA kit (Shanghai Ketao Biotechnology Co. LTD, Shanghai, China) according to the manufacturer’s instructions.
The total DNA of rumen microorganisms was extracted using the hexadecyltrimethylammonium bromide method and measured using Nanodrop One (Thermo Scientific, MA, USA). The sequencing library was constructed using the MGIEasy kit (BGI, China) and the BGISEQ-500, with read lengths of 2 × 100 base pairs (bp), was used to sequence the libraries. Quality control analysis of the original data was performed using the TrimGalore software to remove low-quality reads with lengths less than 50 bp and average base mass less than 20 (Mehdipour et al., 2020). The relevant data were then compared with the host (sheep, alfalfa, corn, and soybean) database using BM Tagger to remove the host data (Uritskiy et al., 2018). The sequence was then annotated with the species using the Kraken 2 software (Kopylova et al., 2012) and the GTDB-r89 database (Waschulin et al., 2022). The relative abundance of species was analyzed by principal component analysis and the diversity analysis was performed using MicrobiomeAnalyst (Chong et al., 2020). The species composition and community structure of the rumen microbiome were tested for significance using linear discriminant analysis effect size analysis.
Calculations and statistical analysis
Feed conversion ratio was calculated as previously described (Sears et al., 2020) using the following equation:
Feed conversion ratio (%) = 3.5% FCM/DM intake
3.5% FCM = [0.4324 × milk yield (kg)] + [16.216 × milk fat yield (kg)],
where FCM = fat-corrected milk; DM = dry matter.
Data were analyzed using the GLM model of SAS v.9.4 (SAS Institute Inc., Cary, NC, USA) as follows:
where Yij = dependent variable; μ = overall mean; Bi = fixed effect of random block (i = 1 to 5); Tj = fixed effect of BCA level (0, 2, or 6 g); and εij = experimental error.
Differences among treatments were considered significant at P < 0.05, based on Tukey’s multiple comparisons of SAS v.9.4 (SAS Institute Inc., Cary, NC, USA). Results were expressed as least squares means and the means ± standard error of the mean (SEM).
Results
Intake, milk yield and composition, and feed conversion ratio
Milk yield in BH was 25.34%, which was significantly greater than that in control group (P = 0.04), however no significant difference was observed between the control and BL groups (P = 0.70; Table 2). Milk protein and total solid contents were increased significantly in BH by 9.73 and 6.38% (P < 0.01), respectively. The feed conversion ratios of dairy goats in BL and BH were increased significantly by 34.92 and 31.75% (P < 0.01), respectively. However, body weight, dry matter intake (DMI), milk fat, and lactose contents were not significantly affected by BCA supplementation.
Rumen fermentation parameters
As shown in Table 3, the total VFA, acetate, propionate, butyrate, valerate, and isovalerate contents in the rumen of dairy goats were increased significantly with BCA supplementation (P < 0.05). However, the pH and NH3-N concentration in the rumen were decreased significantly with BCA supplementation (P < 0.05).
Antioxidant capacity and endocrine indexes in plasma
The GSH-Px activity in plasma was significantly increased with the increase in BCA treatment (Table 4), with GSH-Px in BL increasing by 14.83% and BH increasing by 44.81% (P = 0.02). The T-AOC in BH was significantly higher (P = 0.03) than that in BL. However, SOD and catalase activities were not significantly affected by BCA supplementation. Furthermore, the total protein, albumin, globulin, uric acid, creatinine, and β-hydroxybutyric acid contents, in addition to the alanine and aspartate aminotransferase activities, were not significantly affected by BCA supplementation (Table 5).
The levels of 17β-estradiol and growth hormone in the plasma of dairy goats in the BH group were significantly higher than those in the BL group (P < 0.05; Table 6). The IGF-1 content was significantly increased by BCA supplementation (P < 0.05). Compared with control, the IGF-1 content in BL and BH was increased by 28.26 and 26.79%, respectively. However, plasma prolactin and insulin levels were not significantly affected by BCA supplementation.
Rumen microbiome
Rumen microbial diversity was not significantly affected by BCA supplementation (Figure 1A). However, the relative abundance of microbial flora was influenced by BCA supplementation (Figure 1B). The quantity of Verrucomicrobiota bacteria was significantly decreased, while that of nitrogen metabolism and cellulolytic bacteria was significantly increased, including Prevotella sp., Treponema sp., Ruminococcus flavefaciens, and Ruminobacter amylophilus (P < 0.05; Figure 1C). Thus, BCA regulated the flora of the rumen microbiome, promoting nitrogen metabolic efficiency in the rumen of dairy goats.
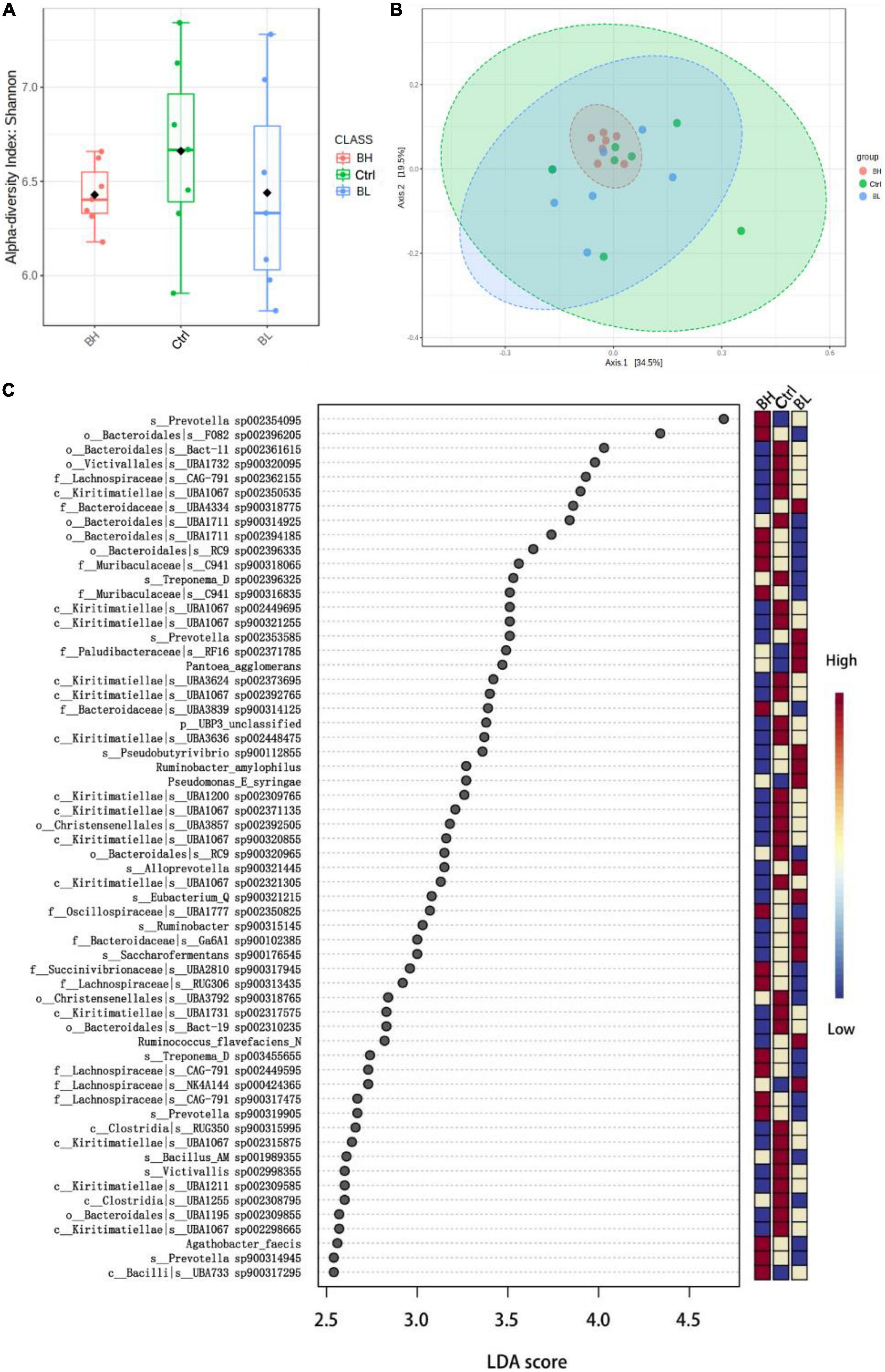
Figure 1. Effect of biochanin A (BCA) on the structure of rumen microbiome of dairy goats (n = 7, mean ± SEM). (A) Diversity (Shannon index) analysis of rumen microbiome. (B) Principal component analysis of rumen microbiome. (C) Linear discriminant analysis (LDA) Effect Size analysis of rumen microbial differential flora in genus level. Ctrl, control group; BL, low level BCA group; BH, high level BCA group.
Discussion
Currently, few studies have investigated the influence of BCA on the growth and production performance of dairy goats and cows. Supplementation of steers with BCA (6.3 g/d) exhibited an increase in the average daily gain. The reduction in ammonia concentration was attributed to BCA, which inhibited HAB fermentation of AA deamination (Harlow et al., 2017c). Steers supplemented with 0.91 kg of red clover hay (equivalent to 0.9–1.4 g BCA) and 1.51 kg DDG also exhibited daily gain (Harlow et al., 2020). When the grass silage was replaced with red and white clover silage, the DMI was increased by 1.2 and 1.3 kg, respectively, and the milk yield was increased by 1.5 and 2.2 kg/d, respectively (Steinshamn, 2010). In this study, milk yield and composition were increased when dairy goats were fed BCA. Similarly, supplementation with flavonoid-rich plant extracts improved the milk yield of dairy cows; however, flavonoids from different sources have different effects (Gessner et al., 2015; Zhan et al., 2017). Alfalfa flavonoid extract increased milk lactose synthesis and secretion by reducing the milk fat and protein content (Zhan et al., 2017). However, soy isoflavone supplementation reduced milk production (Kasparovska et al., 2016). These varying results could be caused by extracts from different sources containing different types and amounts of flavonoids. The expression of milk protein and lactose synthesis-related genes were inhibited by BCA and genistein, however, they were upregulated by formononetin and daidzein, and the metabolite of BCA, p-ethylphenol (Tsugami et al., 2017).
The nitrogen intake of dairy goats was not significantly affected by BCA addition; however, urine secretion was increased with BCA addition. Urinary nitrogen excretion remained constant. BCA could delay the rate of urea hydrolysis in the rumen, thereby allowing for more urea to be used by rumen microorganisms for microbial protein synthesis. Furthermore, BCA reduced the degradation of proteins and AAs in the rumen by inhibiting the activity of proteolytic bacteria (Liu et al., 2020) and hyper-ammonia-producing bacteria (Harlow et al., 2017c). BCA supplementation increased milk yield, indicating that dietary N utilization efficiency was improved (Harlow et al., 2017c; Pereira et al., 2020). Fewer non-degradable rumen proteins increased protein utilization and produced more milk protein (Morales et al., 2010). In another study, the acetate, propionate, and total VFA contents increased, and pH was decreased by BCA supplementation, which affected cellulose fermentation by rumen cellulolytic bacteria (Harlow et al., 2018). Similar results were obtained when BCA was added to a corn diet fermented by amylolytic bacteria; BCA increased the activity of lactic acid-utilizing bacteria and reduced the starch degradation rate (Harlow et al., 2021). However, the VFA content remained unchanged when no dietary supplements were added to the artificial medium (Liu et al., 2020). In contrast to these studies, all the VFA contents were increased by BCA in this study, which may be caused by different fermentation substrates (Harlow et al., 2020, 2021). In addition, the total VFAs and propionate contents in the rumen were also increased by replacing corn silage with different proportions of red clover (Castro-Montoya et al., 2018). Thus, the increase in VFAs by BCA was dependent on the fermentation substrate.
In this study, rumen ammonia nitrogen concentration was linearly decreased with increasing BCA. The hydrolysis rate of urea was decreased, which ultimately resulted in the inhibition of AA degradation by BCA (Liu et al., 2020). The inhibition of AA degradation by BCA was mainly achieved by inhibiting the growth of hyper-ammonia-producing bacteria, which ferment AAs into ammonia in the rumen (Harlow et al., 2017c). Hyper-ammonia bacterial growth was reduced not inhibited by BCA through the enhancement, by rumen microorganisms, of antibacterial activity of bacteriocin, which reduced the ammonia nitrogen content rather than exerting an inhibitory effect, thereby indicating that BCA had synergistic antibacterial activity (Flythe et al., 2013). Crude red clover phenolic extracts also inhibited the growth of hyper-ammonia-producing bacteria (Clostridium sticklandii SR) (Kagan and Flythe, 2012), increased bypass protein, and promoted the by reducing protein nitrogen loss (Harlow et al., 2017c). Therefore, BCA promoted growth of steers rumen fermentation and increased microbial protein production. In addition, the SOD, GSH-Px, and catalase are the main antioxidant enzymes in animals. Serum GSH-Px activity reflected oxidative stress and contributed to oxidative defense in animals (Tuzun et al., 2002). The activities of SOD and GSH-Px were enhanced by BCA through inhibiting nicotinamide adenine dinucleotide phosphate and malondialdehyde production (Yu et al., 2019). In addition, BCA prevented oxidative stress by enhancing the total antioxidant status and the levels of SOD and catalase (Jalaludeen et al., 2016; Sadri et al., 2017). Isoflavone BCA is activating a novel nuclear factor erythroid 2-related factor 2-antioxidant response element activator and can protect against oxidative damage (Yu et al., 2019). Flavonoid extracts containing BCA had a similar effect on improving the antioxidant capacity of livestock and poultry. In chickens supplemented with soybean isoflavone, increased T-AOC and SOD levels in the plasma were reported (Jiang et al., 2007). Dietary supplementation with flavonoids from Scutellaria baicalensis Georgi enhanced the antioxidative ability of broilers (Liao et al., 2018). The activities of SOD and GSH-Px were also increased by feeding alfalfa flavone extract to dairy cows (Zhan et al., 2017). The GSH-Px activity was increased with an increase in BCA supplementation, indicating that BCA can improve the antioxidant performance of dairy goats by enhancing T-AOC and the activities of antioxidant enzymes.
Plasma biochemical parameters reflected the metabolism of nutrients and health conditions of the animal body (Wang et al., 2011). No differences were observed in the total protein, albumin, globulin, uric acid, creatinine, and β-hydroxybutyric acid contents, suggesting that BCA had no effect on the health of dairy goats. Alanine and aspartate aminotransferase are vital indices of liver function, reflecting the permeability and metabolic function of liver cells (Li et al., 2017). Increases in alanine and aspartate aminotransferase activities were caused by damage to the integrity of the hepatic membrane architecture (Kotyzova et al., 2013). In this study, although there is no significant difference, aspartate aminotransferase activity had a decreased trend in the BH group, indicating that a high dose of BCA may have a hepatoprotective effect in dairy goats, and this trend was also observed in a previous study in rats (Breikaa et al., 2013). PUN and NH3-N were the final protein metabolites that reflected AA metabolism (Li et al., 2017). PUN concentrations reached a peak approximately 2 h after feeding, then gradually decreased, reverting to normal levels at 6 h (Sinclair et al., 2012). A similar trend was observed in the NH3-N concentrations, which may have resulted from the decreased hepatic clearance of NH3-N (Ludden et al., 2000). Soybean isoflavone, an isoflavone phytoestrogen, is a physiological regulator of the reproductive and nutritional processes of the body through the neuroendocrine system to improve the production performance of animals (Jiang et al., 2007). Isoflavone promoted the development of mammary glands, increased milk yield, and accelerated animal growth by inducing changes in endogenous hormone levels in blood, such as prolactin, growth hormone, and IGF-1 (Tsugami et al., 2017). In this study, BCA treatment significantly increased the levels of 17β-estradiol, growth hormone, and IGF-1. Similarly, the levels of 17β-estradiol, growth hormone, and prolactin in the plasma of mid-lactation dairy cows were also increased by soybean isoflavone daidzein (Tsugami et al., 2017). These hormones can regulate the lactation of dairy goats and the structural development of mammary gland, and estradiol and IGF-1 can regulate mammary epithelial cells by binding to estrogen receptor and maintaining its expression (Tsugami et al., 2017). However, there are limited reports on the effects of BCA on the endocrine system of animals; therefore, further studies are required to explore the underlying mechanisms.
In this study, the flora structure of the rumen microbiome was modulated by BCA supplementation, which promoted nitrogen metabolic efficiency in the rumen. Similarly, in another study, the abundance of Bacteroidetes was increased by isoflavone-enriched feed, resulting in a high nitrogen metabolic efficiency in the rumen (Kasparovska et al., 2016). Rumen microbial diversity was not significantly affected, whereas the abundance of rumen microbiota was significantly affected by red clover isoflavones (Melchior et al., 2018). These results indicated that the nitrogen metabolic efficiency in the rumen was improved by BCA treatment by modulating the structure of the rumen microbiome. In this study, the flora of nitrogen metabolism and cellulolytic bacteria in rumen was regulated by BCA, and these rumen microbiota can promote nitrogen metabolic efficiency of dairy goats, resulting in enhancing feed conversion and increasing VFA production. As we know, acetate and butyrate are the precursors of milk fat, and propionate is the precursor of lactose in milk. Therefore, the increased VFA led to the improvement in milk production. In addition, reduced ammonia can be used by rumen microbes, and the increase of microbial protein can increase the milk protein, also leading to the increased feed conversion and VFA production. Additionally, in health dairy goats, the improvement in antioxidative capacity can enhance their resistance of pathogen and diseases, which is beneficial to the health of goats. Moreover, unchanged plasma biochemical parameters indicated that BCA had no side effects on the immune and health of dairy goats. The altered endocrine indexes indicated BCA can increased the production performance of dairy goat through hormone to some extent, including 17β-estradiol, GH, and IGF-1.
Conclusion
Milk production performance, nitrogen metabolism, and the feed conversion ratio of dairy goats were improved by BCA supplementation. Rumen AA degradation were inhibited, whereas VFAs were increased with BCA supplementation, indicating that rumen fermentation was promoted. Antioxidant performance (GSH-Px activity and T-AOC) and endocrine hormones levels (prolactin, growth hormone, and IGF-1) were also increased by BCA supplementation. The relative abundance of rumen microbiota were also affected by BCA supplementation, such as decreased Verrucomicrobiota and increased nitrogen metabolism and cellulolytic bacteria, including Prevotella sp., Treponema sp., Ruminococcus flavefaciens, and Ruminobacter amylophilus. Thus, dairy goat supplemented with BCA at a concentration of 6 g/day per goat can improve its performance and health status.
Data availability statement
The original contributions presented in this study are publicly available. This data can be found here: https://nmdc.cn/resource/genomics/project/detail/NMDC10018266.
Ethics statement
This animal study was reviewed and approved by Animal Care and Use Committee of the Institute of Animal Science of the Chinese Academy of Agricultural Sciences (approval no. IAS2020-97).
Author contributions
YL, JW, and SZ conceived and designed the experiments. QX and YL wrote and prepared the original draft. QX, WD, NZ, JW, and SZ edited the manuscript. QX, JW, and SZ critically reviewed the manuscript. All authors reviewed and approved the final manuscript.
Funding
This study was supported by grants from the National Key R&D Program of China (No. 2022YFD1301004), National Center of Technology Innovation for Dairy (2021-NCTID-2), the State Key Laboratory of Animal Nutrition (2004DA125184G2206), and Key Laboratory of Molecular Animal Nutrition of Zhejiang University (KLMAN202101 and KLMAN202205).
Conflict of interest
The authors declare that the research was conducted in the absence of any commercial or financial relationships that could be construed as a potential conflict of interest.
Publisher’s note
All claims expressed in this article are solely those of the authors and do not necessarily represent those of their affiliated organizations, or those of the publisher, the editors and the reviewers. Any product that may be evaluated in this article, or claim that may be made by its manufacturer, is not guaranteed or endorsed by the publisher.
References
Breikaa, R., Algandaby, M., El-Demerdash, E., and Abdel-Naim, A. (2013). Biochanin a protects against acute carbon tetrachloride-induced hepatotoxicity in rats. Biosci. Biotechnol. Biochem. 77, 909–916. doi: 10.1271/bbb.120675
Butkute, B., Dagilyte, A., Benetis, R., Padarauskas, A., Ceseviciene, J., Olsauskaite, V., et al. (2018). Mineral and phytochemical profiles and antioxidant activity of herbal material from two temperate Astragalus species. Biomed Res. Int. 2018:6318630. doi: 10.1155/2018/6318630
Castro-Montoya, J., Witzig, M., Rahman, M., Westreicher-Kristen, E., and Dickhoefer, U. (2018). In vitro rumen fermentation, microbial protein synthesis and composition of microbial community of total mixed rations replacing maize silage with red clover silage. J. Anim. Physiol. Anim. Nutr. 102, 1450–1463. doi: 10.1111/jpn.12970
Chen, X., and Gomes, M. (1992). Estimation of microbial protein supply to sheep and cattle based on urinary excretion of purine derivatives – an overview of technical details. Aberdeen: Rowett Research lnstitute.
Chong, J., Liu, P., Zhou, G., and Xia, J. (2020). Using microbiomeanalyst for comprehensive statistical, functional, and meta-analysis of microbiome data. Nat. Protoc. 15, 799–821. doi: 10.1038/s41596-019-0264-1
Chukwumah, Y., Walker, L., Verghese, M., and Ogutu, S. (2009). Effect of frequency and duration of ultrasonication on the extraction efficiency of selected isoflavones and trans-resveratrol from peanuts (Arachis hypogaea). Ultrason. Sonochem. 16, 293–299. doi: 10.1016/j.ultsonch.2008.07.007
Flythe, M., and Kagan, I. (2010). Antimicrobial effect of red clover (Trifolium pratense) phenolic extract on the ruminal hyper ammonia-producing bacterium, Clostridium sticklandii. Curr. Microbiol. 61, 125–131. doi: 10.1007/s00284-010-9586-5
Flythe, M., Harrison, B., Kagan, I., Klotz, J., and Aiken, G. (2013). Antimicrobial activity of red clover (Trifolium pratense L.) extract on caprine hyper-ammonia-producing bacteria. Agric. Food Anal. Bacteriol. 3, 176–185.
Galal, A., Mohamed, A., Khater, S., and Metwally, M. (2018). Beneficial role of biochanin A on cutaneous and renal tissues of ovariectomized rats treated with anastrozole. Life Sci. 201, 9–16. doi: 10.1016/j.lfs.2018.03.037
Gao, Y., Yao, Y., Zhu, Y., and Ren, G. (2015). Isoflavone content and composition in chickpea (Cicer arietinum L.) sprouts germinated under different conditions. J. Agric. Food Chem. 63, 2701–2707. doi: 10.1021/jf5057524
Gessner, D., Koch, C., Romberg, F., Winkler, A., Dusel, G., Herzog, E., et al. (2015). The effect of grape seed and grape marc meal extract on milk performance and the expression of genes of endoplasmic reticulum stress and inflammation in the liver of dairy cows in early lactation. J. Dairy Sci. 98, 8856–8868. doi: 10.3168/jds.2015-9478
Harlow, B., Flythe, M., and Aiken, G. (2017b). Effect of biochanin A on corn grain (Zea mays) fermentation by bovine rumen amylolytic bacteria. J. Appl. Microbiol. 122, 870–880. doi: 10.1111/jam.13397
Harlow, B., Flythe, M., Kagan, I., and Aiken, G. (2017c). Biochanin A (an isoflavone produced by red clover) promotes weight gain of steers grazed in mixed grass pastures and fed dried-distillers’, Grains. Crop Sci. 57, 506–514. doi: 10.2135/cropsci2016.07.0590
Harlow, B., Aiken, G., Klotz, J., and Flythe, M. (2017a). Biochanin A mitigates rumen microbial changes associated with a sub-acute ruminal acidosis challenge. J. Anim. Sci. 95:263. doi: 10.2527/asasann.2017.536
Harlow, B., Flythe, M., and Aiken, G. (2018). Biochanin A improves fibre fermentation by cellulolytic bacteria. J. Appl. Microbiol. 124, 58–66. doi: 10.1111/jam.13632
Harlow, B., Flythe, M., Kagan, I., Goodman, J., Klotz, J., and Aiken, G. (2020). Isoflavone supplementation, via red clover hay, alters the rumen microbial community and promotes weight gain of steers grazing mixed grass pastures. PLoS One 15:e0229200. doi: 10.1371/journal.pone.0229200
Harlow, B., Flythe, M., Klotz, J., Harmon, D., and Aiken, G. (2021). Effect of biochanin A on the rumen microbial community of Holstein steers consuming a high fiber diet and subjected to a subacute acidosis challenge. PLoS One 16:e0253754. doi: 10.1371/journal.pone.0253754
Hu, K., Shi, X., Xu, D., Laborda, P., Wu, G., Liu, F., et al. (2021). Antibacterial mechanism of Biochanin A and its efficacy for the control of Xanthomonas axonopodis pv. glycines in soybean. Pest Manag. Sci. 77, 1668–1673. doi: 10.1002/ps.6186
Jalaludeen, A., Ha, W., Lee, R., Kim, J., Do, J., Park, C., et al. (2016). Biochanin a ameliorates arsenic-induced hepatoand hematotoxicity in rats. Molecules 21:69. doi: 10.3390/molecules21010069
Jiang, Z., Jiang, S., Lin, Y., Xi, P., Yu, D., and Wu, T. (2007). Effects of soybean isoflavone on growth performance, meat quality, and antioxidation in male broilers. Poult. Sci. 86, 1356–1362. doi: 10.1093/ps/86.7.1356
Kagan, I., and Flythe, M. (2012). Factors affecting the separation and bioactivity of red clover (Trifolium pratense) extracts assayed against Clostridium sticklandii, a ruminal hyper ammonia-producing bacterium. Nat. Prod. Commun. 7, 1605–1608. doi: 10.1177/1934578X1200701217
Kagan, I., Goff, B., and Flythe, M. (2015). Soluble phenolic compounds in different cultivars of red clover and alfalfa, and their implication for protection against proteolysis and ammonia production in ruminants. Nat. Prod. Commun. 10, 1263–1267. doi: 10.1177/1934578X1501000732
Kasparovska, J., Pecinkova, M., Dadakova, K., Krizova, L., Hadrova, S., Lexa, M., et al. (2016). Effects of isoflavone-enriched feed on the rumen microbiota in dairy cows. PLoS One 11:e0154642. doi: 10.1371/journal.pone.0154642
Kopylova, E., Noé, L., and Touzet, H. (2012). SortMeRNA: Fast and accurate filtering of ribosomal RNAs in metatranscriptomic data. Bioinformatics 28, 3211–3217. doi: 10.1093/bioinformatics/bts611
Kotyzova, D., Bludovska, M., and Eybl, V. (2013). Differential influences of various arsenic compounds on antioxidant defense system in liver and kidney of rats. Environ. Toxicol. Pharmacol. 36, 1015–1021. doi: 10.1016/j.etap.2013.09.003
Křížová, L., Dadáková, K., Kašparovská, J., and Kašparovský, T. (2019). Isoflavones. Molecules 24:1076. doi: 10.3390/molecules24061076
Lee, K., and Choi, E. (2005). Biochanin A stimulates osteoblastic differentiation and inhibits hydrogen peroxide-induced production of inflammatory mediators in MC3T3-E1 cells. Biol. Pharm. Bull. 28, 1948–1953. doi: 10.1248/bpb.28.1948
Lemeziene, N., Padarauskas, A., Butkute, B., Ceseviciene, J., Taujenis, L., Norkeviciene, E., et al. (2015). The concentration of isoflavones in red clover (Trifolium pratense L.) at flowering stage. Zemdirbyste 102, 443–448. doi: 10.13080/z-a.2015.102.057
Li, Y., Wang, Z., Yang, H., Xu, L., Xie, Y., Jin, S., et al. (2017). Effects of dietary fiber on growth performance, slaughter performance, serum biochemical parameters, and nutrient utilization in geese. Poult. Sci. 96, 1250–1256. doi: 10.3382/ps/pew385
Liao, X., Wen, Q., Zhang, L., Lu, L., Zhang, L., and Luo, X. (2018). Effect of dietary supplementation with flavonoid from Scutellaria baicalensis Georgi on growth performance, meat quality and antioxidative ability of broilers. J. Integr. Agric. 17, 1165–1170. doi: 10.1016/S2095-3119(17)61803-3
Liu, S., Zhang, Z., Hailemariam, S., Zheng, N., Wang, M., Zhao, S., et al. (2020). Biochanin a inhibits ruminal nitrogen-metabolizing bacteria and alleviates the decomposition of amino acids and urea in vitro. Animals 10:368. doi: 10.3390/ani10030368
Ludden, P., Harmon, D., Huntington, G., Larson, B., and Axe, D. (2000). Influence of the novel urease inhibitor N-(n-butyl) thiophosphoric triamide on ruminant nitrogen metabolism: II. Ruminal nitrogen metabolism, diet digestibility, and nitrogen balance in lambs. J. Anim. Sci. 78, 188–198. doi: 10.2527/2000.781188x
Mehdipour, P., Marhon, S., Ettayebi, I., Chakravarthy, A., Hosseini, A., Wang, Y., et al. (2020). Epigenetic therapy induces transcription of inverted SINEs and ADAR1 dependency. Nature 588:169. doi: 10.1038/s41586-020-2844-1
Melchior, E., Smith, J., Schneider, L., Mulliniks, J., Bates, G., McFarlane, Z., et al. (2018). Effects of red clover isoflavones on tall fescue seed fermentation and microbial populations in vitro. PLoS One 13:e0201866. doi: 10.1371/journal.pone.0201866
Mendowski, S., Chapoutot, P., Chesneau, G., Ferlay, A., Enjalbert, F., Cantalapiedra-Hijar, G., et al. (2020). Effects of pretreatment with reducing sugars or an enzymatic cocktail before extrusion of fava bean on nitrogen metabolism and performance of dairy cows. J. Dairy Sci. 103, 396–409. doi: 10.3168/jds.2019-17286
Morales, E., Adarve, G., Alcaide, E., and Sampelayo, M. (2010). Nitrogen and energy utilization in lactating dairy goats fed diets with different legume seeds. J. Anim. Physiol. Anim. Nutr. 94, 659–664. doi: 10.1111/j.1439-0396.2009.00953.x
Pereira, A., Moura, D., Whitehouse, N., and Brito, A. (2020). Production and nitrogen metabolism in lactating dairy cows fed finely ground field pea plus soybean meal or canola meal with or without rumen-protected methionine supplementation. J. Dairy Sci. 103, 3161–3176. doi: 10.3168/jds.2019-17186
Sadri, H., Goodarzi, M., Salemi, Z., and Seifi, M. (2017). Antioxidant effects of biochanin a in streptozotocin induced diabetic rats. Braz. Arch. Biol. Technol. 60, 1–10. doi: 10.1590/1678-4324-2017160741
Sears, A., Gonzalez, O., Alberto, A., Young, A., de Souza, J., Relling, A., et al. (2020). Effect of feeding a palmitic acid-enriched supplement on production responses and nitrogen metabolism of mid-lactating Holstein and Jersey cows. J. Dairy Sci. 103, 8898–8909. doi: 10.3168/jds.2020-18232
Sinclair, L., Blake, C., Griffin, P., and Jones, G. (2012). The partial replacement of soyabean meal and rapeseed meal with feed grade urea or a slow-release urea and its effect on the performance, metabolism and digestibility in dairy cows. Animal 6, 920–927. doi: 10.1017/S1751731111002485
Steinshamn, H. (2010). Effect of forage legumes on feed intake, milk production and milk quality–a review. Anim. Sci. Pap. Rep. 28, 195–206.
Tsugami, Y., Matsunaga, K., Suzuki, T., Nishimura, T., and Kobayashi, K. (2017). Isoflavones and their metabolites influence the milk component synthesis ability of mammary epithelial cells through prolactin/STAT5 signaling. Mol. Nutr. Food Res. 61:1700156. doi: 10.1002/mnfr.201700156
Tuzun, A., Erdil, A., Inal, V., Aydin, A., Bagci, S., Yesilova, Z., et al. (2002). Oxidative stress and antioxidant capacity in patients with inflammatory bowel disease. Clin. Biochem. 35, 569–572. doi: 10.1016/S0009-9120(02)00361-2
Uritskiy, G., DiRuggiero, J., and Taylor, J. (2018). MetaWRAP-a flexible pipeline for genome-resolved metagenomic data analysis. Microbiome 6:158. doi: 10.1186/s40168-018-0541-1
Van Soest, P., Robertson, J., and Lewis, B. (1991). Methods for dietary fiber, neutral detergent fiber, and nonstarch polysaccharides in relation to animal nutrition. J. Dairy Sci. 74, 3583–3597. doi: 10.3168/jds.S0022-0302(91)78551-2
Wang, J., Wu, C., and Feng, J. (2011). Effect of dietary antibacterial peptide and zinc-methionine on performance and serum biochemical parameters in piglets. Czech J. Anim. Sci. 56, 30–36. doi: 10.17221/341/2009-CJAS
Waschulin, V., Borsetto, C., James, R., Newsham, K., Donadio, S., Corre, C., et al. (2022). Biosynthetic potential of uncultured Antarctic soil bacteria revealed through long-read metagenomic sequencing. ISME J. 16, 101–111. doi: 10.1038/s41396-021-01052-3
Wenjin, S., Yangdong, Z., Xiang, L., Yufeng, D., and Qingbiao, X. (2021). Understanding the functional activity of polyphenols using omics-based approaches. Nutrients 13:3953. doi: 10.3390/nu13113953
Yu, C., Zhang, P., Lou, L., and Wang, Y. (2019). Perspectives regarding the role of biochanin a in humans. Front. Pharmacol. 10:793. doi: 10.3389/fphar.2019.00793
Yu, X., Chen, J., Deng, W., Xu, X., Liu, Q., Shi, M., et al. (2020). Biochanin a mitigates atherosclerosis by inhibiting lipid accumulation and inflammatory response. Oxid. Med. Cell. Longev. 2020:8965047. doi: 10.1155/2020/8965047
Keywords: antioxidant capacity, biochanin A, dairy goat, lactation performance, rumen microbiome, ruminal fermentation
Citation: Xu Q, Li Y, Du W, Zheng N, Wang J and Zhao S (2023) Effect of dietary biochanin A on lactation performance, antioxidant capacity, rumen fermentation and rumen microbiome of dairy goat. Front. Microbiol. 14:1101849. doi: 10.3389/fmicb.2023.1101849
Received: 18 November 2022; Accepted: 23 January 2023;
Published: 06 February 2023.
Edited by:
Guan Yang, City University of Hong Kong, Hong Kong SAR, ChinaReviewed by:
Yang Xiao, Mayo Clinic, United StatesJéssica Araújo, Federal University of Sergipe, Brazil
Copyright © 2023 Xu, Li, Du, Zheng, Wang and Zhao. This is an open-access article distributed under the terms of the Creative Commons Attribution License (CC BY). The use, distribution or reproduction in other forums is permitted, provided the original author(s) and the copyright owner(s) are credited and that the original publication in this journal is cited, in accordance with accepted academic practice. No use, distribution or reproduction is permitted which does not comply with these terms.
*Correspondence: Jiaqi Wang, amlhcWl3YW5nQHZpcC4xNjMuY29t; Shengguo Zhao,
emhhb3NoZW5nZ3VvMTk4NEAxNjMuY29t
†These authors have contributed equally to this work