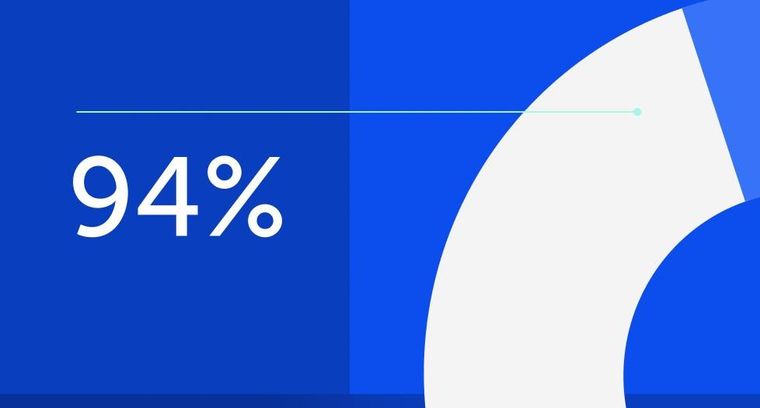
94% of researchers rate our articles as excellent or good
Learn more about the work of our research integrity team to safeguard the quality of each article we publish.
Find out more
REVIEW article
Front. Microbiol., 06 March 2023
Sec. Extreme Microbiology
Volume 14 - 2023 | https://doi.org/10.3389/fmicb.2023.1093018
This article is part of the Research TopicStudies on Life at the Energetic Edge – from Laboratory Experiments to Field-Based Investigations, Volume IIView all 11 articles
Extremely thermophilic methanogens in the Methanococci and heterotrophs in the Thermococci are common in deep-sea hydrothermal vents. All Methanococci use H2 as an electron donor, and a few species can also use formate. Most Methanococci have a coenzyme F420-reducing formate dehydrogenase. All Thermococci reduce S0 but have hydrogenases and produce H2 in the absence of S0. Some Thermococci have formate hydrogenlyase (Fhl) that reversibly converts H2 and CO2 to formate or an NAD(P)+-reducing formate dehydrogenase (Nfd). Questions remain if Methanococci or Thermococci use or produce formate in nature, why only certain species can grow on or produce formate, and what the physiological role of formate is? Formate forms abiotically in hydrothermal fluids through chemical equilibrium with primarily H2, CO2, and CO and is strongly dependent upon H2 concentration, pH, and temperature. Formate concentrations are highest in hydrothermal fluids where H2 concentrations are also high, such as in ultramafic systems where serpentinization reactions occur. In nature, Methanococci are likely to use formate as an electron donor when H2 is limiting. Thermococci with Fhl likely convert H2 and CO2 to formate when H2 concentrations become inhibitory for growth. They are unlikely to grow on formate in nature unless formate is more abundant than H2 in the environment. Nearly all Methanococci and Thermococci have a gene for at least one formate dehydrogenase catalytic subunit, which may be used to provide free formate for de novo purine biosynthesis. However, only species with a membrane-bound formate transporter can grow on or secrete formate. Interspecies H2 transfer occurs between Thermococci and Methanococci. This and putative interspecies formate transfer may support Methanococci in low H2 environments, which in turn may prevent growth inhibition of Thermococci by its own H2. Future research directions include understanding when, where, and how formate is used and produced by these organisms in nature, and how transcription of Thermococci genes encoding formate-related enzymes are regulated.
It was estimated that 40% of bacterial and archaeal global biomass is found in the rocky portion of the ocean crust below ocean sediments (Bar-On et al., 2018; Fleming and Wuertz, 2019). These microbes live in cracks and pores of the rocky subseafloor in the absence of sunlight and often in the absence of oxygen and rely on the gases, aqueous compounds (e.g., sulfide, sulfate, and nitrate), organic compounds, and minerals found locally for growth. In high-temperature anoxic environments, H2 is generally considered to be the primary electron donor and CO2 the primary carbon source for autotrophic metabolism. However, recently other electron donors and carbon sources such as formate have been considered as alternatives (Windman et al., 2007), especially in high pH environments where dissolved inorganic carbon precipitates as calcium carbonate and is largely unavailable to autotrophs (Lang et al., 2018; McGonigle et al., 2020; Brazelton et al., 2022). There are strong links between formate and H2 in hydrothermal environments and in the physiology of microbes that consume and produce formate and H2.
High-temperature microbes that use formate and H2 are examined herein, namely methanogens (in the class Methanococci and the class Methanopyri) and heterotrophs (in the class Thermococci). These organisms are found in deep-sea hydrothermal vents on or near tectonic plate boundaries – both mid-ocean ridges and subduction zones. Thermophiles and hyperthermophiles are defined as those organisms with optimal growth temperatures above 50°C and 80°C, respectively (Stetter, 2006). In this review, the term ‘extreme thermophile’ will be used to describe organisms with optimal growth temperatures above 65°C. Extremely thermophilic Methanococci and Thermococci are among the more cosmopolitan and well-studied microbes found in hydrothermal vent environments. All Methanococci and the marine hyperthermophile Methanopyrus kandleri (the sole member of the Methanopyri) use H2 and CO2 as energy and carbon sources to produce CH4, H2O, and biomass (Thauer et al., 2008). All Thermococci use peptides and sugars as carbon and energy sources and reduce zero-valent sulfur (S0) to a sulfide species or reduce 2 H+ to H2 in the absence of S0 (Wu et al., 2018). However, some extremely thermophilic Methanococci and Thermococci grow using formate as an energy source only or as both energy and carbon sources (Belay et al., 1986; Kim et al., 2010; Lim et al., 2014). This raises questions about which organisms can use formate, when they use formate in nature, and for what purpose. This review describes how formate and H2 are formed in hydrothermal vents, the concentrations of these compounds in pure hydrothermal fluids, the physiology of extremely thermophilic Methanococci and Thermococci as it relates to formate and H2 use, transcriptional regulation of formate dehydrogenase and hydrogenase genes, and suggests likely roles for formate use by these organisms in nature.
Deep-sea hydrothermal vents provide one of the best access points to the hydrothermally influenced portion of the rocky subseafloor and are ideal starting points for understanding biogeochemical processes in these regions of the crust. Some hydrothermal fluids rise through the crust undiluted, so-called “end-member hydrothermal fluid,” and exit the seafloor at temperatures generally above 300°C (Table 1). It can also mix with cold seawater on or below the seafloor creating habitats for extremely thermophilic anaerobes either within the host rock (e.g., basalt) or in metal sulfide mineral precipitates (e.g., black smoker chimneys). Most hydrothermal vent studies are focused on one of three types of sites: ultramafic sites along slow-to-ultraslow tectonic spreading centers, mafic sites along intermediate-to-fast spreading centers, and subduction-influenced sites near tectonic convergence zones (Figure 1).
Figure 1. Global map of the hydrothermal vents in Table 1. Hydrothermal vents hosted in ultramafic rock are shown in red; basalt, in black; and in subduction zones, in yellow-brown.
The host rock in mafic and ultramafic sites have high concentrations of MgO and FeO, but they differ in their silica content, with ultramafic rocks having silica concentrations less than 45% (by weight), while mafic rocks have concentrations above 45%. Most abiotic formation of H2 in hydrothermal vents occurs by hydrothermal alteration of the ultramafic rock peridotite (i.e., serpentinization) (Table 1). Serpentinization occurs in environments with limited magma supply where peridotite is present in the rock hosting hydrothermal circulation and is mostly associated with ultramafic sites. Olivine and orthopyroxene, the most abundant minerals in peridotite, are unstable under hydrothermal conditions, which causes dissolution-reprecipitation reactions and the formation of serpentine, magnetite, and H2 [e.g., 6 (Mg, Fe)2SiO4 + 7 H2O → 3(Mg, Fe)Si2O5(OH)4 + Fe3O4 + H2] (Klein et al., 2020). Methanococci and Thermococci are common in most ultramafic-influenced hydrothermal sites except at the Lost City hydrothermal vent field (Table 1). At Lost City, the high pH hydrothermal fluids formed by low temperature serpentinization lead to calcium carbonate precipitation and very low dissolved inorganic carbon concentrations. This likely hinders the growth of autotrophs such as Methanococci and Methanopyri unless they can grow on an aqueous carbon source such as formate.
Serpentinization is inhibited by silica and is thus less common in mafic and felsic rocks (felsic rocks are > 65% silica by weight). In mafic (basalt)-hosted hydrothermal systems, the oxidation of ferrous iron minerals, such as pyrrhotite to pyrite (FeS + H2S → FeS2 + H2) and magnetite to hematite (2 Fe3O4 + H2O → 3 Fe2O3 + H2), and weathering of the ocean crust by oxygen-depleted water in the root zone of a hydrothermal system are also significant sources of H2 in hydrothermal systems (Klein et al., 2020). H2 and H2S concentrations are controlled by chemical equilibrium between fluid and the pyrite-pyrrhotite-magnetite mineral assemblages present. Most H2 and H2S fluid compositions fall close to the metastable extension of pyrite-pyrrhotite equilibrium (Klein et al., 2020). H2 concentrations in mafic hydrothermal fluids also increase significantly following a volcanic eruption as circulating fluids interact with newly injected rock (Lilley et al., 2003; Seewald et al., 2003; Von Damm and Lilley, 2004). Mafic hydrothermal vent sites generally tend to have Thermococci and Methanococci present (Table 1), especially following volcanic eruptions (Holden et al., 1998; Huber et al., 2002; Meyer et al., 2013), but Methanococci can become rare or undetectable during quiescent periods between eruptions when H2 concentrations decrease or in low H2 hydrothermal vents (Ver Eecke et al., 2009, 2012).
In contrast, hydrothermal vents that form along volcanic arcs at convergent plate boundaries have host rock with hydrous minerals, silica accumulation in aging oceanic crust, and more felsic character, such as dacite and andesite. The hydrothermal fluids from these rocks tend to have lower pH and lower H2 (Table 1). While Thermococci are generally present at these sites, Methanococci tend to be rare or undetectable (Table 1) likely due to the very low H2 concentrations (Ver Eecke et al., 2012).
Other more minor abiotic H2 contributions in hydrothermal vents come from magmatic degassing at low hydrostatic pressures (e.g., shallow vent sites) and radiolysis of water (Klein et al., 2020). Biotic sources of H2 at extremely thermophilic temperatures by Thermococci are described in Section “4. H2 production by Thermococci.”
Hydrogen is used by extremely thermophilic Methanococci, specifically, the genera Methanocaldococcus (Topt 80–85°C), Methanotorris (Topt 75–88°C), Methanofervidicoccus (Topt 70°C), and Methanothermococcus (Topt 65°C), and in the Methanopyri, which consists solely of Methanopyrus kandleri (Topt 98°C) (Table 2).
Table 2. Growth characteristics of the classes Methanococci and Methanopyri and presence of genes for formate transport (FT), formate dehydrogenase (fdh), hydrogenases (eha, ehb, frh, vhu, hmd), and purine biosynthesis (purT, purP).
The whole genome sequences of 10 extremely thermophilic Methanococci plus M. kandleri were analyzed for known hydrogenases (see Supplementary materials). All 11 of the Methanococci and Methanopyri in the genome survey have at least one of the following hydrogenase genes (see Greening et al., 2016 for a review): (1) eha and ehb operons, which encode for membrane-bound multimeric hydrogenases that couple H2 oxidation to ferredoxin reduction and are H+/Na+ driven for anaplerotic (Eha) and anabolic (Ehb) purposes (Porat et al., 2006; Lie et al., 2012); (2) an frh operon, which encodes for a soluble complex that directly couples H2 oxidation to coenzyme F420 reduction (Hendrickson and Leigh, 2008); (3) an hmd gene, which encodes a soluble methylenetetrahydromethanopterin dehydrogenase that couples oxidation of H2 to the reduction of methenyltetrahydromethanopterin in the archaeal Wood-Ljungdahl CO2 fixation pathway (Hendrickson and Leigh, 2008); and (4) a vhu operon, which encodes for soluble heterodisulfide reductase-linked complexes that bifurcate electrons from H2 to heterodisulfide (coenzyme M-coenzyme B) and ferredoxin (Kaster et al., 2011). These hydrogenases are described and listed in Figure 2, Table 2, and Supplementary Table 1. Coenzyme F420, ferredoxin, coenzyme M, and coenzyme B are soluble electron carriers in methanogens (Thauer et al., 2008). Extremely thermophilic Methanococci and Methanopyri will often have two or three copies of the genes encoding these enzymes (Table 2 and Supplementary Table 1).
Figure 2. Formate dehydrogenase (Fdh), hydrogenase, and formate transporter proteins and their reactions that are found in Methanococci and Methanopyri. Fdh catalyzes the following formate oxidation reactions: cytoplasmic reduction of coenzyme F420 (F420) and cytoplasmic reduction of coenzyme M (CoM), coenzyme B (CoB), and ferredoxin (Fd). The hydrogenases catalyze the following H2 oxidation reactions: membrane-bound reduction of Fd (Eha), cytoplasmic reduction of F420 (Frh), cytoplasmic reduction of methenyl-tetrahydromethanopterin (CH-H4MPT) (Hmd), and cytoplasmic reduction of CoM, CoB, and Fd. F420, Fd, CoM, and CoB are cytoplasmic electron carriers. Methenyl-H4MPT is an intermediate of the Wood-Ljungdahl CO2 fixation pathway. Created with BioRender.com.
The growth of natural assemblages of extremely thermophilic Methanococci in hydrothermal vent fluids from Axial Seamount is largely dependent on H2 availability and temperature (Topçuoğlu et al., 2016). The Monod kinetic half-saturation value (Ks) for growth of extremely thermophilic methanogens was 27–66 μM with maximum methane production rates of 24–43 fmol CH4 produced cell–1 h–1 (Ver Eecke et al., 2012; Stewart et al., 2019). Methanocaldococcus jannaschii and Methanothermococcus thermolithotrophicum were shown to grow by interspecies H2 transfer when grown in co-culture with Thermococcus celer, Thermococcus stetteri, and Pyrococcus furiosus (Bonch-Osmolovskaya and Stetter, 1991). When M. jannaschii was grown in monoculture at high (80–83 μM) and low (15–27 μM) H2 concentrations and in co-culture with the hyperthermophilic H2 producer Thermococcus paralvinellae (representing very low H2 flux), growth and cell-specific CH4 production rates decreased with decreasing H2 availability (Topçuoğlu et al., 2019). However, the number of cells produced per mole of CH4 produced (i.e., cell yield) increased six-fold with decreasing H2 indicating increased growth efficiency when growth was limited by H2 (Topçuoğlu et al., 2019). Relative to high H2 concentrations, isotopic fractionation of CO2 to CH4 was 16‰ larger for cultures grown at low H2 concentrations and 45–56‰ larger in co-culture suggesting reversal of the Wood-Ljungdahl pathway during methanogenesis with low H2 flux (Valentine et al., 2004; Topçuoğlu et al., 2019). While all four types of hydrogenases were synthesized by M. jannaschii with high and low H2 flux, transcript levels of hmd and eha decreased with decreasing H2 availability (Topçuoğlu et al., 2019).
Hydrogen is produced by Thermococci, specifically, the genera Thermococcus (Topt 75–90°C), Palaeococcus (Topt 83°C), and Pyrococcus (Topt 96–105°C) (Table 3).
Table 3. Growth characteristics of the class Thermococci and presence of genes for formate transport (FT), formate dehydrogenase operons (fhl, nfd), and individuals (fdhA) with neighboring hydrogenase operons, individual hydrogenase operons (mbh, sh, frh, codh), and purine biosynthesis (purT, purP).
The whole genome sequences of 30 Thermococci were analyzed for known hydrogenases (see Supplementary materials). All 30 Thermococci analyzed have at least one of the following hydrogenase operons: (1) An mbh operon, which encodes for a membrane-bound hydrogenase that couples oxidation of ferredoxin to H2 evolution with concomitant H+/Na+ translocation across the membrane using antiporters (Sapra et al., 2003); (2) an sh operon, which encodes for a soluble sulfhydrogenase that couples oxidation of H2 oxidation to the reduction of NAD(P)+ (Van Haaster et al., 2008); (3) an frh operon, which encodes for cytoplasmic coenzyme F420 reducing-type hydrogenase that oxidizes H2 and passes electrons to a thioredoxin reductase (Jung et al., 2020); and (4) a codh operon, which encodes for a membrane-bound hydrogenase that couples oxidation of CO to H2 evolution with concomitant H+/Na+ translocation across the membrane using antiporters (Bae et al., 2012; Moon et al., 2012). These hydrogenases are described and listed in Figure 3, Table 3, and Supplementary Table 2.
Figure 3. Formate dehydrogenase, hydrogenase, and formate transporter proteins and their reactions that are found in Thermococci. Formate hydrogenlyase (Fhl) catalyzes membrane-bound oxidation of formate to H2 and CO2. NAD(P)H: formate dehydrogenase (Nfd) catalyzes cytoplasmic oxidation of formate coupled with reduction of NAD(P)+. The hydrogenases catalyze the following reactions: membrane-bound oxidation of ferredoxin (Fd) coupled with H2 production (Mbh), cytoplasmic H2 oxidation coupled with reduction of NAD(P)+ (Sh), and cytoplasmic H2 oxidation (Frd) coupled with reduction of thioredoxin reductase (TrxR). Fd and NAD(P)H are cytoplasmic electron carriers. TrxR is part of the redox cascade for sulfur response regulation using SurR. Created with BioRender.com.
All Thermococci have at least one mbh operon and all but one have at least one sh operon (Table 3). These enzymes are the core hydrogenases for Thermococci (Schut et al., 2012; Boyd et al., 2014). Twelve of the 30 Thermococci in the survey have frh operons. Five of the 30 Thermococci have codh operons. It was shown that the growth of Thermococcus sp. strain AM4 and Thermococcus onnurineus can be supported by CO with concomitant H2 production (Sokolova et al., 2004; Bae et al., 2012; Moon et al., 2012), although the physiological role of this enzyme in Thermococcus is yet to be determined for growth in its natural environment.
In Thermococci, the reduction of S0 is the preferred route for electron disposal over the reduction of H+ to H2. In P. furiosus, the presence of S0 in growth media resulted in decreases in Mbh and Sh hydrogenase specific activities, each by an order of magnitude (Adams et al., 2001). There was an immediate downregulation of mbh and an upregulation of mbs (membrane-bound sulfane reductase) (Wu et al., 2018) and nsr (NAD(P)H:S0 reductase) in P. furiosus when S0 was added to growth medium (Schut et al., 2001, 2007). A sulfur response regulator protein (SurR) was identified as the transcription factor regulating hydrogenase and sulfur responsive genes (Lipscomb et al., 2009, 2017). The proposed model suggests that SurR contains a redox-active cysteine disulfide that can reduce S0 to H2S (Yang et al., 2010). SurR is reduced in a redox cascade involving NAD(P)H-dependent thioredoxin reductase (TrxR) and protein disulfide oxidoreductase (Pdo) as the electron donors (Lim et al., 2017). In the absence of S0, SurR remains reduced, binds to GTTn3AAC(n5GTT), promotes the transcription of mbh and sh genes, and represses the expression of mbs and nsr genes (Lipscomb et al., 2009). Thermococci with the Frh hydrogenase also can reduce TrxR using H2 as the electron donor (Jung et al., 2020).
Abiotic formation of formate, carbon monoxide, methane, and hydrocarbons in hydrothermal vents is of interest as potential growth substrates for microbes. Methane and hydrocarbons in vents were suggested to form through Fischer-Tropsch type reactions [(2n + 1)H2 + nCO → CnH2n+2 + nH2O] or leach from fluid inclusions in plutonic rocks (Berndt et al., 1996; Horita and Berndt, 1999; McCollom and Seewald, 2001; McDermott et al., 2015). In contrast to hydrocarbons, there is a strong thermodynamic drive toward rapid C-H-O equilibrium in hydrothermal fluids within hours to days. Kinetic barriers preclude the formation of CH4 in this equilibrium (Shock, 1990). This permits the creation of metastable formate species (H2 + CO2 ↔ HCOOH), CO (HCOOH ↔ CO + H2O), formaldehyde (HCOOH + H2 ↔ CH2O + H2O), and methanol (CH2O + H2 ↔ CH3OH) through the sequential reduction of CO2 using H2 as the reductant (Seewald et al., 2006).
The abundance of formate in chemical equilibrium with dissolved inorganic carbon is strongly dependent on H2 concentration, pH, and temperature (McCollom and Seewald, 2003; Seewald et al., 2006). In a gold-titanium reaction cell, HCOO– was formed from CO2 at 300°C and 350 bar in less than 24 h from H2 generated from hydrothermal alteration of olivine serving as the reductant (McCollom and Seewald, 2001). In a separate study, incubation of a 175 mmol/kg HCOOH solution at 300°C and 350 bar in the gold reaction cell led to near complete conversion to H2 and CO2 within 48 h, CO reached 0.83 mmol/kg, and HCOO– + HCOOH (or ΣHCOOH) decreased to 0.38 mmol/kg (Seewald et al., 2006). Reducing the temperature to 200°C and then to 150°C each led to an increase in ΣHCOOH, a decrease in CO, and C-H-O equilibrium within 115 h and 71 h, respectively. Injection of 172 mmol/kg CO led to production of H2, ΣCO2, and ΣHCOOH, and decreasing CO. Alkaline conditions favored the formation of HCOOH, HCO3–, and CO32– (Seewald et al., 2006). Therefore, the abundance of formate, CO, and CH3OH in seafloor hydrothermal systems will be regulated by the residence times of fluids in reactions zones, and physical and chemical conditions in the subsurface environments.
Formate is also generated across a pH gradient of more than three pH units using a mineral precipitate bridge at the interface of two fluids (Hudson et al., 2020). This may be relevant to the formation of formate on the early Earth or possibly in extraterrestrial oceans where high pH serpentinized fluids are emitted into an acid ocean. Under standard conditions, the generation of formate from H2 and CO2 is not thermodynamically favorable. However, H2 in synthetic alkaline vent fluid (pH 12.3) passed electrons to dissolved CO2 in a synthetic acid ocean (pH 3.9) at 25°C through a Fe(Ni)S mineral interface generating 1.5 μM HCOO– in the ocean fluid (Hudson et al., 2020). Isotopic labeling showed that protonation occurred using H2O on the ocean side of the interface, not H2 on the vent side. Weakening the pH gradient led to decreased concentrations of HCOO– produced. Nickel in the precipitate is a crucial part of the reduction mechanism as HCOO– yield dropped below detection without Ni in the ocean precipitation fluid.
There have been very few measurements of formate in natural hydrothermal fluids due in part to the analytical difficulty of measuring formate at low concentrations (Schink et al., 2017). Formate has been measured mostly at sites with high H2 concentrations such as at the Lost City, Von Damm, and Piccard hydrothermal vent sites and were 36–669 μM (Table 1). Formate and H2 were also measured at Snake Pit and TAG hydrothermal vents, which are mafic hydrothermal vents on the Mid-Atlantic Ridge, where formate concentrations were 1–2 nM and H2 concentrations were 0.08–2.4 μM (Konn et al., 2022). At ultramafic sites, formate concentrations are generally 10–100 fold lower than that of H2 at the same site (Lang et al., 2010; McDermott et al., 2015) while at mafic sites the formate concentration is often more than 1,000 fold lower than the H2 concentration (McDermott et al., 2018; Konn et al., 2022).
Methanocaldococcus jannaschii was shown to incorporate 14C-formate into biomass during growth (Sprott et al., 1993), which may be used in part for de novo purine biosynthesis. Inosine monophosphate (IMP) is a precursor for adenine and guanine synthesis for purine biosynthesis and is made from ribose-5-phosphate (Figure 4). In most organisms, the pathway intermediates glycinamide-ribose-5-phosphate (GAR) and aminoimidazole carboxamide-ribose-5-phosphate (AICAR) are formylated using N10-formyl-tetrahydrofolate as the formyl donor. However, the genes for these enzymes are absent in Methanococci and Methanopyri and are replaced with genes that encode for enzymes that use free formate and energy from ATP to formylate their substrates (White, 1997; Brown et al., 2011). These enzymes are formylglycinamide-ribose-5-phosphate synthetase (PurT) and forminido imidazole carboxamide-ribose-5-phosphate synthetase (PurP) (Figure 4). M. jannaschii was shown to have PurP activity and that it produced free 13C-formate in the cell when incubated with H2 and H13CO3 (Ownby et al., 2005). Herein, a genome survey of the eleven extremely thermophilic methanogens showed that all the organisms have homologs for purP and all but M. kandleri have homologs for purT (Table 2 and Supplementary Table 1). This suggests that these organisms have a mechanism for formate synthesis.
Figure 4. Biochemical pathway for de novo purine biosynthesis using free formate as the source of the formyl group (after Brown et al., 2011).
Nine of the 11 Methanococci and Methanopyri genomes have genes that encode for a cytoplasmic formate dehydrogenase (Table 2 and Supplementary Table 1). Formate dehydrogenases catalyze the reversible oxidation of formate to CO2 using various electron acceptors. The catalytic α subunit (FdhA) contains tungsten, selenocysteine, and a (Fe4-S4) cluster as cofactors while the β subunit (FdhB) contains three (Fe4-S4) clusters (Niks and Hille, 2019). FdhAB in Methanococci and Methanopyri is homologous to two formate dehydrogenases in the mesophilic methanogen Methanococcus maripaludis, also a Methanococci, that use coenzyme F420 as their redox partner (Figure 2; Wood et al., 2003; Lupa et al., 2008). M. maripaludis grows hydrogenotrophically on H2 and CO2 but also grows on formate in their absence (Jones et al., 1983b). When fdhA1 was mutated in M. maripaludis, the organism was unable to grow on formate and formate dehydrogenase activity in cell extracts was undetectable (Lupa et al., 2008). Observations with hydrogenase mutants in M. maripaludis suggest that coenzyme F420 is an intermediate in formate-to-H2 conversion (Lupa et al., 2008). An M. maripaludisΔfdhA1ΔfdhA2 double mutant grown in purine-free defined medium grew as well as the wild-type strain suggesting that formate dehydrogenase is not essential for de novo purine biosynthesis (Wood et al., 2003). The absence of fdhAB genes in Methanocaldococcus infernus and Methanofervidicoccus abyssi (Table 2 and Supplementary Table 1) also supports the idea that formate dehydrogenase is not essential for purine biosynthesis. However, it is likely that H2 and coenzyme F420 are electron donors for formate production and can help meet the cellular demand for formate for purine biosynthesis.
The formate dehydrogenase (FdhA1B1) from M. maripaludis also forms an enzyme complex with heterodisulfide reductase, the soluble hydrogenase Vhu, and formylmethanofuran dehydrogenase (Figure 2; Costa et al., 2010). It was necessary for the organism’s growth on formate but not on H2 (Costa et al., 2010). Therefore, in addition to coenzyme F420 reduction, this formate dehydrogenase also oxidizes formate to reduce the heterodisulfide coenzyme M-coenzyme B and ferredoxin through electron bifurcation. Coenzyme M, coenzyme B, and ferredoxin are cytoplasmic electron carriers in these methanogens. Expression of the second formate dehydrogenase gene (fdhA2) in M. maripaludis increased when cells were grown under H2 limited conditions but was unchanged under formate limited conditions (Costa et al., 2013) and was not required for growth on formate (Lupa et al., 2008) suggesting that this isoenzyme may have a separate physiological function.
For extremely thermophilic methanogens, it appears that a formate transporter is required for growth on formate. Formate transporters import or export formate across the cytoplasmic membrane and require co-translocation of a H+ (Figure 2). Three thermophilic methanogens in our survey (Methanotorris formicicus, Methanothermococcus okinawensis, and Methanothermococcus thermolithotrophicus) grew on formate in the absence of H2 and CO2 but not any of the other methanogens examined (Table 2). Each of these methanogens that grew on formate has a gene that encodes for a membrane-bound formate transporter (fdhC) in its genome, which is absent in all other methanogens examined, except for Methanocaldococcus fervens which was not tested for growth on formate (Table 2 and Supplementary Table 1). M. maripaludis has an fdhC gene in an operon with fdhA1B1 (Sattler et al., 2013). In M. fervens and M. okinawensis, the formate transporter gene fdhC appears to be in the same operon as fdhAB suggesting they are co-transcribed (Supplementary Table 1).
Like Methanococci, all Thermococci lack the enzymes that use N10-formyl-tetrahydrofolate as the formyl donor for de novo purine biosynthesis (Brown et al., 2011). Instead, most Thermococci use formate-dependent enzymes (PurT and PurP) for de novo purine biosynthesis (Figure 4, Table 3, and Supplementary Table 2). Therefore, they depend on a source of free formate in the cell for de novo synthesis. However, some Thermococcus species (T. paralvinellae, T. barophilus CH5, T. onnurineus, and T. gorgonarius) lack most or all the genes for the purine biosynthesis pathway (Brown et al., 2011) and likely rely on environmental sources of purines.
All 30 Thermococci genomes have at least one copy of the gene that encodes for the catalytic α subunit of formate dehydrogenase (FdhA) either in the form of formate hydrogenlyase, NAD(P)+-dependent formate dehydrogenase, or the catalytic subunit alone (Table 3 and Supplementary Table 2). The phylogeny of FdhA in extremely thermophilic Methanococci, Methanopyri, and Thermococci showed one clade for Methanococci and Methanopyri and five clades among the Thermococci (Figure 5). In Thermococci, hydrogenase operons often flank fdhA-containing operons in the genome (Figure 6 and Supplementary Table 2) suggesting a close association between formate and H2 in these organisms. In Groups 1 and 2 in Figure 5, fdhA was encoded in an operon with a formate transporter gene. For Group 1, in nearly all instances, the fdhA-containing operon was immediately downstream from an frh operon and immediately upstream from one or two mbh operons on the same DNA strand suggesting that they may be co-transcribed (Figure 6). In Group 1A, fdhA was encoded in a formate hydrogenlyase (fhl) operon (Kim et al., 2010; Topçuoğlu et al., 2018; Le Guellec et al., 2021; Table 3; Supplementary Table 2). This enzyme reversibly couples formate oxidation to H2 evolution on the cytoplasmic membrane with concomitant H+/Na+ translocation across the membrane via antiporter modules (Figure 4; Kim et al., 2010; Lim et al., 2014). In Group 1B, fdhA was encoded in a NAD(P)+-dependent formate dehydrogenase (nfd) operon (Figure 6). This soluble enzyme catalyzes the reversible oxidation of formate using NAD(P)+ or ferredoxin as its redox partner (Le Guellec et al., 2021; Yang et al., 2022; Figure 4). In Group 2, fdhA was encoded in an nfd operon but neighbored an sh operon in the genome instead of frh and mbh operons (Figure 6). These nfd and sh operons are transcribed in opposite directions from the same intergenic spacer region.
Figure 5. Phylogenetic tree based on catalytic subunit alpha (FdhA) for the various formate dehydrogenases found in extremely thermophilic Methanococci, Methanopyri, and Thermococci. The phylogeny of FdhA was inferred by using a maximum likelihood method and Jones-Taylor-Thornton (JTT) matrix-based modeling (Jones et al., 1992). After 1000 bootstrap constructions, the tree with the highest log likelihood (–31,270.37) is shown, with values next to nodes indicating the percentage of reconstructions in which the topology was preserved (values < 70% are omitted for clarity). There were a total of 736 positions in the final dataset. Branch lengths are to scale and indicate the number of substitutions per site. GenBank/EMBL/DDBJ open reading frame numbers are included in parentheses. Evolutionary analyses were conducted in MEGA11 (Tamura et al., 2021). Clade associations with operon arrangements on the genomes and the presence of a formate transporter or putative regulatory elements are shown.
Figure 6. Operon and gene maps for Thermococci containing operons for formate hydrogenlyase (fhl), NAD(P)H: formate dehydrogenase (nfd), membrane hydrogenase (mbh), soluble hydrogenase (sh), and F420-reducing-like hydrogenase (frh) (Groups 1–4) and genes for the catalytic subunit of formate dehydrogenase (fdhA), and purine biosynthesis (pur) (Group 5). Also shown are the locations of SurR binding sites (S), the tetR gene for transcriptional regulation, and the operons containing a formate transporter (FT) gene. The top scale bar is for the Group 1–4 operons; the bottom scale bar, the genes for Group 5.
The fdhA from Groups 3 and 4 are in fhl operons that lack a formate transporter gene. In Group 3, the fhl operon was next to an sh operon (Figure 6). These fhl and sh operons are transcribed in opposite directions from the same intergenic spacer region. In Group 4, the fhl operon did not neighbor any hydrogenase operons in the genome, and in Group 5 the fdhA gene was the only formate dehydrogenase-related gene present in the genome (Figure 6). Often these solo genes in Group 5 are near the purine biosynthesis genes in genome sequences (Supplementary Table 2). In T. sibiricus, nearly all the genes for de novo purine biosynthesis (purFCMTEDPSQL) and fdhA are next to each other in the genome, although they are not all on the same DNA strand (Figure 6). In these organisms, it is unknown if fdhA alone encodes for a functional formate dehydrogenase or what the redox partner is for this putative enzyme. However, it is plausible that it might be used to produce formate for purine biosynthesis when other formate dehydrogenases and formate transport proteins are absent.
Under defined growth conditions, 11 of the 30 Thermococci strains analyzed either oxidized added formate as an energy source (plus trace levels of organic compounds as a carbon source) and produced H2 (Kim et al., 2010; Topçuoğlu et al., 2018) or secreted formate when grown on organic compounds in the presence of high background H2 and the absence of added formate (Hensley et al., 2016; Topçuoğlu et al., 2018; Le Guellec et al., 2021). These 11 strains are the only Thermococci in the survey that have a formate transporter gene (Table 3). The other 19 Thermococci lack this gene and were unable to grow on formate or secrete formate (Kim et al., 2010; Le Guellec et al., 2021). Therefore, it appears that a formate transporter is required for Thermococci to secrete formate or, like Methanococci, for growth of Thermococci on formate. The presence of a formate transporter gene or transcript should be a criterion when determining if Methanococci or Thermococci are potentially using or producing formate in their natural habitat.
The standard Gibbs energy for interconversion between formate and H2 + CO2 is small; therefore, the direction of the reaction is highly dependent upon the relative concentrations of formate and H2 in the environment (Schink et al., 2017; Le Guellec et al., 2021). Le Guellec et al. (2021) calculated that CO2 reduction to formate using H2 is thermodynamically more favorable than formate oxidation to H2 and CO2 at Lost City, Von Damm, Rainbow, Lucky Strike, Snake Pit, and Ashadze 1 hydrothermal vent sites based on relative formate and H2 concentrations in hydrothermal fluids. The physiological response of Thermococcus is in keeping with this idea. Growth of T. paralvinellae on a sugar or peptides when sparged with H2 led to higher levels of fhl1 expression and higher formate secretion relative to cultures sparged with N2 (Topçuoğlu et al., 2018). It was concluded that fhl and nfd expression in Thermococci is primarily for the purpose of ameliorating H2 inhibition rather than for growth on formate (Topçuoğlu et al., 2019; Le Guellec et al., 2021). Thermococci would require an environment where formate concentrations exceed H2 concentrations to grow on formate. The formate produced by Thermococci may supplement the growth of Methanococci even when Thermococci produce H2, as is observed with fermenter-methanogen relationships in mesophilic environments (Schink et al., 2017).
Formate consumption in Methanococci is closely associated with H2 use in the cell. Therefore, a question that arises is whether formate or H2 regulates fdhAB expression in these organisms. The thermophilic methanogen Methanobacterium thermoformicicum grows on H2 and CO2 as well as separately on formate. It has a formate transporter gene (fdhC) directly upstream of its formate dehydrogenase genes (fdhAB) (Nolling and Reeve, 1997). Transcripts of fdhCAB were present in M. thermoformicicum at all growth stages when grown on formate. When grown on H2 and CO2, fdhCAB transcripts were barely detectable in early exponential growth phase but increased dramatically as cells approached late exponential growth phase in a closed batch system when H2 became more limiting. Similarly, fdh expression in M. maripaludis was controlled by the presence of H2 and not formate (Wood et al., 2003). Using fdhC-lacZ gene fusions, β-galactosidase activity increased in M. maripaludis cells grown on H2 and CO2 as they approached late exponential growth phase, again when H2 became limiting. When grown on formate, β-galactosidase activity was higher in cells with N2 and CO2 in the headspace relative to those with H2 and CO2 in the headspace. β-galactosidase activity increased in cells grown on formate plus H2 and CO2 after the H2 and CO2 was replaced mid-growth phase with N2 and CO2.
In M. maripaludis, genes for a putative response regulator and a histidine kinase are directly upstream of fdhC, which is three genes upstream of fdhA1B1 and part of a putative five-gene operon (Sattler et al., 2013). Random mutagenesis showed that disruption of this putative response regulator led to slower growth of M. maripaludis on formate relative to the wild type. It also led to increased fdhA1 transcriptional abundance regardless of whether H2 and CO2 or formate was the growth substrate. Impairment of derepression of the fdhC-fdhA1B1 operon is a plausible explanation (Sattler et al., 2013). Therefore, H2 present at high concentrations may interact with the histidine kinase and activate the response regulator in a two-component regulatory system that represses fdhC-fdhA1B1 expression, which is derepressed when H2 levels are low or absent.
Very little is known about transcriptional regulation of the fhl and nfd operons in Thermococci. Group 1 Thermococci genomes (Figure 6) encode syntenic frh, either fhl or nfd, and mbh operons with a formate transporter gene encoded in the fhl or nfd operon (Figure 6). These frh, fhl, nfd, and mbh operons each have GTTn3AAC(n5GTT) in their promoter region just upstream of BRE/TATA RNA polymerase binding sites suggesting they are also regulated and promoted by the sulfur response regulator protein SurR (see Section “4.2. Growth of Thermococci with and without S0”). Furthermore, Frh was shown to oxidize H2 and reduce TrxR (Jung et al., 2020), which reduces SurR via Pdo, suggesting that it might serve as a regulatory hydrogenase that promotes frh, fhl, nfd, and mbh expression when H2 concentrations increase in the cell. Therefore, like Methanococci, H2 abundance appears to regulate formate use in Thermococci. A remaining question is whether formate also regulates gene expression in Thermococci. In T. paralvinellae, expression of the Group 1A fhl operon containing the formate transporter gene increased when cells were grown on formate relative to growth on maltose or peptides while expression of mbh either remained unchanged or decreased (Topçuoğlu et al., 2018). This suggests that in addition to SurR regulation, formate either directly or indirectly regulates gene expression in T. paralvinellae as well. Validation and the mechanism of this putative regulation is yet to be determined.
None of the promoter regions for the nfd, fhl, or sh operons in Groups 2–4 had a SurR nucleotide binding sequence. All but one of the Group 4 fhl operons have a gene encoding for a TetR/AcrR family transcriptional regulator that is ∼350 nucleotides upstream of and transcribed in the same direction as the fhl operon (Supplementary Table 2). TetR/AcrR family transcriptional regulators are one-component systems where a single protein contains both a sensory domain and a DNA-binding domain (Cuthbertson and Nodwell, 2013). They are widely associated with antibiotic resistance and the regulation of genes encoding small molecule exporters and are usually encoded alongside target operons (Colclough et al., 2019). In T. paralvinellae, expression of the Group 4 fhl operon decreased when cells were grown on formate relative to growth on maltose or peptides (Topçuoğlu et al., 2018). The mechanism for regulation of Group 2–5 formate dehydrogenase-related genes is unknown.
Formate and H2 are linked both in hydrothermal vent environments and in the metabolisms of extremely thermophilic Methanococci and Thermococci. Methanococci prefer H2 oxidation to formate oxidation but appear to switch to the latter when H2 is limiting. Similarly, Thermococci appear to prefer H2 production to formate production but switch to the latter when H2 is excessive and inhibitory. H2 is typically far more abundant than formate in hydrothermal vent fluids suggesting that in high H2 environments formate is unlikely to be used by Methanococci and Methanopyri for growth. However, in hydrothermal environments that are very low H2 environments but rich in organic compounds, Thermococci may produce H2 and formate that are then used to support the growth of extremely thermophilic methanogens. Understanding where, when, and how formate is used by extreme thermophiles in nature is largely unknown and an area of future research. Furthermore, our understanding of transcriptional regulation of fhl and nfd in Thermococci is nascent. A key question is if and how formate influences gene expression, especially in concert with SurR regulation of hydrogenases and sulfur responsive genes.
JH and HS contributed to the conceptualization, original draft preparation, review, and editing of the manuscript. JH conducted bioinformatic analyses and data compilation. Both authors read and agreed to the published version of the manuscript.
This research was provided by the NASA Exobiology grant 80NSSC21K1240 and USDA National Institute of Food and Agriculture grant MAS00550 to JH.
We thank Briana Kubik, Gema Garcia, and Gabriella Rizzo for their constructive comments, and the reviewers for their helpful suggestions.
The authors declare that the research was conducted in the absence of any commercial or financial relationships that could be construed as a potential conflict of interest.
All claims expressed in this article are solely those of the authors and do not necessarily represent those of their affiliated organizations, or those of the publisher, the editors and the reviewers. Any product that may be evaluated in this article, or claim that may be made by its manufacturer, is not guaranteed or endorsed by the publisher.
The Supplementary Material for this article can be found online at: https://www.frontiersin.org/articles/10.3389/fmicb.2023.1093018/full#supplementary-material
Adams, M. W. W., Holden, J. F., Lal Menon, A., Schut, G. J., Grunden, A. M., Hou, C., et al. (2001). Key role for sulfur in peptide metabolism and in regulation of three hydrogenases in the hyperthermophilic archaeon Pyrococcus furiosus. J. Bacteriol. 183, 716–724. doi: 10.1128/JB.183.2.716-724.2001
Anderson, R. E., Beltrán, M. T., Hallam, S. J., and Baross, J. A. (2013). Microbial community structure across fluid gradients in the Juan de Fuca ridge hydrothermal system. FEMS Microbiol. Ecol. 83, 324–339. doi: 10.1111/j.1574-6941.2012.01478.x
Atomi, H., Fukui, T., Kanai, T., Morikawa, M., and Imanaka, T. (2004). Description of Thermococcus kodakaraensis sp. nov., a well studied hyperthermophilic archaeon previously reported as Pyrococcus sp. KOD1. Archaea 1, 263–267.
Bae, S. S., Kim, T. W., Lee, H. S., Kwon, K. K., Kim, Y. J., Kim, M.-S., et al. (2012). H2 production from CO, formate, or starch using the hyperthermophilic archaeon, Thermococcus onnurineus. Biotechnol. Lett. 34, 75–79. doi: 10.1007/s10529-011-0732-3
Bae, S. S., Kim, Y. J., Yang, S. H., Lim, J. K., Jeon, J. H., Lee, H. S., et al. (2006). Thermococcus onnurineus sp. nov., a hyperthermophilic archaeon isolated from a deep-sea hydrothermal vent area at the pacmanus Field. J. Microbiol. Biotechnol. 16, 1826–1831.
Bar-On, Y. M., Phillips, R., and Milo, R. (2018). The biomass distribution on Earth. Proc. Natl. Acad. Sci. U.S.A. 115, 6506–6511. doi: 10.1073/pnas.1711842115
Baumberger, T., Früh-Green, G. L., Thorseth, I. H., Lilley, M. D., Hamelin, C., Bernasconi, S. M., et al. (2016). Fluid composition of the sediment-influenced loki’s castle vent field at the ultra-slow spreading arctic mid-ocean ridge. Geochim. Cosmochim. Acta 187, 156–178. doi: 10.1016/j.gca.2016.05.017
Belay, N., Sparling, R., and Daniels, L. (1986). Relationship of formate to growth and methanogenesis by Methanococcus thermolithotrophicus. Appl. Environ. Microbiol. 52, 1080–1085. doi: 10.1128/aem.52.5.1080-1085.1986
Belkin, S., Wirsen, C. O., and Jannasch, H. W. (1985). Biological and abiological sulfur reduction at high temperatures. Appl. Environ. Microbiol. 49, 1057–1061. doi: 10.1128/aem.49.5.1057-1061.1985
Berndt, M. E., Allen, D. E., and Seyfried, W. E. Jr. (1996). Reduction of CO2 during serpentinization of olivine at 300°C and 500 bar. Geology 24, 351–354. doi: 10.1130/0091-76131996024<0351:ROCDSO<2.3.CO;2
Birrien, J.-L., Zeng, X., Jebbar, M., Cambon-Bonavita, M.-A., Quérellou, J., Oger, P., et al. (2011). Pyrococcus yayanosii sp. nov., an obligate piezophilic hyperthermophilic archaeon isolated from a deep-sea hydrothermal vent. Int. J. Syst. Evol. Microbiol. 61, 2827–2831. doi: 10.1099/ijs.0.024653-0
Bonch-Osmolovskaya, E. A., and Stetter, K. O. (1991). Interspecies hydrogen transfer in cocultures of thermophilic Archaea. System. Appl. Microbiol. 14, 205–208.
Boyd, E. S., Schut, G. J., Adams, M. W. W., and Peters, J. W. (2014). Hydrogen metabolism and the evolution of biological respiration. Microbe 9, 361–367.
Brazelton, W. J., McGonigle, J. M., Motamedi, S., Pendleton, H. L., Twing, K. I., Miller, B. C., et al. (2022). Metabolic strategies shared by basement residents of the lost city hydrothermal field. Appl. Environ. Microbiol. 88:e0092922. doi: 10.1128/aem.00929-22
Brazelton, W. J., Schrenk, M. O., Kelley, D. S., and Baross, J. A. (2006). Methane- and sulfur-metabolizing microbial communities dominate the lost city hydrothermal field ecosystem. Appl. Environ. Microbiol. 72, 6257–6270. doi: 10.1128/AEM.00574-06
Brown, A. M., Hoopes, S. L., White, R. H., and Sarisky, C. A. (2011). Purine biosynthesis in archaea: Variations on a theme. Biol. Direct 6:63. doi: 10.1186/1745-6150-6-63
Burggraf, S., Fricke, H., Neuner, A., Kristjansson, J., Rouvier, P., Mandelco, L., et al. (1990). Methanococcus igneus sp. nov., a novel hyperthermophilic methanogen from a shallow submarine hydrothermal system. System. Appl. Microbiol. 13, 263–269. doi: 10.1016/s0723-2020(11)80197-9
Callac, N., Oger, P., Lesongeur, F., Rattray, J. E., Vannier, P., Michoud, G., et al. (2016). Pyrococcus kukulkanii sp. nov., a hyperthermophilic, piezophilic archaeon isolated from a deep-sea hydrothermal vent. Int. J. Syst. Evol. Microbiol. 66, 3142–3149. doi: 10.1099/ijsem.0.001160
Canganella, F., Jones, W. J., Gambacorta, A., and Antranikian, G. (1998). Thermococcus guaymasensis sp. nov. and Thermococcus aggregans sp. nov., two novel thermophilic archaea isolated from the Guaymas basin hydrothermal vent site. Int. J. Syst. Bacteriol. 48, 1181–1185. doi: 10.1099/00207713-48-4-1181
Colclough, A. L., Scadden, J., and Blair, J. M. A. (2019). TetR-family transcription factors in gram-negative bacteria: Conservation, variation and implications for efflux-mediated antimicrobial resistance. BMC Genomics 20:731. doi: 10.1186/s12864-019-6075-5
Costa, K. C., Wong, P. M., Wang, T., Lie, T. J., Dodsworth, J. A., Swanson, I., et al. (2010). Protein complexing in a methanogen suggests electron bifurcation and electron delivery from formate to heterodisulfide reductase. Proc. Natl. Acad. Sci. U.S.A. 107, 11050–11055. doi: 10.1073/pnas.1003653107
Costa, K. C., Yoon, S. H., Pan, M., Burn, J. A., Baliga, N. S., and Leigh, J. A. (2013). Effects of H2 and formate on growth yield and regulation of methanogenesis in Methanococcus maripaludis. J. Bacteriol. 195, 1456–1462. doi: 10.1128/JB.02141-12
Courtine, D., Vince, E., Maignien, L., Philippon, X., Gayet, N., Shao, Z., et al. (2021). Thermococcus camini sp. nov., a hyperthermophilic and piezophilic archaeon isolated from a deep-sea hydrothermal vent at the mid-atlantic ridge. Int. J. Syst. Evol. Microbiol. 71:004853. doi: 10.1099/ijsem.0.004853
Cuthbertson, L., and Nodwell, J. R. (2013). The TETR family of regulators. Microbiol. Mol. Biol. Rev. 77, 440–475. doi: 10.1128/MMBR.00018-13
Dalmasso, C., Oger, P., Seva, G., Courtine, D., L’Harison, S., Garlaschelli, A., et al. (2016). Thermococcus piezophilus sp. nov., a novel hyperthermophilic and piezophilic archaeon with a broad pressure range for growth, isolated from a deepest hydrothermal vent at the mid-Cayman rise. Syst. Appl. Microbiol. 39, 440–444. doi: 10.1016/j.syapm.2016.08.003
Ding, K., Seyfried, W. E. Jr., Zhang, Z., Tivey, M. K., Von Damm, K. L., and Bradley, A. M. (2005). The in situ pH of hydrothermal fluids at mid-ocean ridges. Earth Planet. Sci. Lett. 237, 167–174. doi: 10.1016/j.epsl.2005.04.041
Duffaud, G. D., d’Hennezel, O. B., Peek, A. S., Reysenbach, A.-L., and Kelly, R. M. (1998). Isolation and characterization of Thermococcus barossii, sp. nov., a hyperthermophilic archaeon isolated from a hydrothermal vent flange formation. System. Appl. Microbiol. 21, 40–49.
Erauso, G., Reysenbach, A.-L., Godfroy, A., Meunier, J. R., Crump, B., Partensky, F., et al. (1993). Pyrococcus abyssi sp. nov., a new hyperthermophilic archaeon isolated from a deep-sea hydrothermalvent. Arch. Microbiol. 160, 338–349.
Fiala, G., and Stetter, K. O. (1986). Pyrococcus furiosus sp. nov. Represents a novel genus of marine heterotrophic archaebacteria growing optimally at 100°C. Arch. Microbiol. 145, 56–61.
Fleming, H.-C., and Wuertz, S. (2019). Bacteria and archaea on earth and their abundance in biofilms. Nat. Rev. Microbiol. 17, 247–260. doi: 10.1038/s41579-019-0158-9
Flores, G. E., Campbell, J. H., Kirshtein, J. D., Meneghin, J., Podar, M., Steinberg, J. I., et al. (2011). Microbial community structure of hydrothermal deposits from geochemically different vent fields along the mid-atlantic ridge. Environ. Microbiol. 13, 2158–2171. doi: 10.1111/j.1462-2920.2011.02463.x
Flores, G. E., Shakya, M., Meneghin, J., Yang, Z. K., Seewald, J. S., Wheat, C. G., et al. (2012). Inter-field variability in the microbial communities of hydrothermal vent deposits from a back-arc basin. Geobiology 10, 333–346. doi: 10.1111/j.1472-4669.2012.00325.x
Fortunato, C. S., Larson, B., Butterfield, D. A., and Huber, J. A. (2018). Spatially distinct, temporally stable microbial populations mediate biogeochemical cycling at and below the seafloor in hydrothermal vent fluids. Environ. Microbiol. 20, 769–784. doi: 10.1111/1462-2920.14011
Gallant, R. M., and Von Damm, K. L. (2006). Geochemical controls on hydrothermal fluids from the Kairei and Edmond vent fields, 23°-25°S, central Indian ridge. Geochem. Geophys. Geosyst. 7:Q06018. doi: 10.1029/2005GC001067
Gamo, T., Masuda, H., Yamanaka, T., Okamura, K., Ishibashi, J., Nakayama, E., et al. (2004). Discovery of a new hydrothermal venting site in the southernmost Mariana Arc: Al-rich hydrothermal plumes and white smoler activity associated with biogenic methane. Geochem. J. 38, 527–534. doi: 10.2343/geochemj.38.527
González, J. M., Kato, C., and Horikoshi, K. (1995). Thermococcus peptonophilus sp. nov., a fast-growing, extremely thermophilic archaebacterium isolated from deep-sea hydrothermal vents. Arch. Microbiol. 164, 159–164.
González, J. M., Masuchi, Y., Robb, F. T., Ammerman, J. W., Maeder, D. L., Yanagibayashi, M., et al. (1998). Pyrococcus horikoshii sp. nov., a hyperthermophilic archaeon isolated from a hydrothermal vent at the okinawa trough. Extremophiles 2, 123–130. doi: 10.1007/s007920050051
Gorlas, A., Croce, O., Oberto, J., Gauliard, E., Forterre, P., and Marguet, E. (2014). Thermococcus nautili sp. nov., a hyperthermophilic archaeon isolated from a hydrothermal deep-sea vent. Int. J. Syst. Evol. Microbiol. 64, 1802–1810. doi: 10.1099/ijs.0.060376-0
Greening, C., Biswas, A., Carere, C. R., Jackson, C. J., Taylor, M. C., Stott, M. B., et al. (2016). Genomic and metagenomic surveys of hydrogenase distribution indicate H2 is a widely utilized energy source for microbial growth and survival. ISME J. 10, 761–777. doi: 10.1038/ismej.2015.153
Grote, R., Li, L., Tamaoka, J., Kato, C., Horikoshi, K., and Antranikian, G. (1999). Thermococcus siculi sp. nov., a novel hyperthermophilic archaeon isolated from a deep-sea hydrothermal vent at the Mid-Okinawa Trough. Extremophiles 3, 55–62.
Han, Y., Gonnella, G., Adam, N., Schippers, A., Burkhardt, L., Kurtz, S., et al. (2018). Hydrothermal chimneys host habitat-specific microbial communities: Analogues for studying the possible impact of mining seafloor massive sulfide deposits. Sci. Rep. 8:10386. doi: 10.1038/s41598-018-28613-5
Hendrickson, E. L., and Leigh, J. A. (2008). Roles of coenzyme F420-reducing hydrogenases and hydrogen- and F420-dependent methlenetetrahydromethanopterin dehydrogenases in reduction of F420 and production of hydrogen during methanogenesis. J. Bacteriol. 190, 4818–4821. doi: 10.1128/JB.00255-08
Hensley, S. A., Moreira, E., and Holden, J. F. (2016). Hydrogen production and enzyme activities in the hyperthermophile Thermococcus paralvinellae grown on maltose, tryptone, and agricultural waste. Front. Microbiol. 7:167. doi: 10.3389/fmicb.2016.00167
Holden, J. F., Summit, M., and Baross, J. A. (1998). Thermophilic and hyperthermophilic microorganisms in 3-30°C hydrothermal fluids following a deep-sea volcanic eruption. FEMS Microbiol. Ecol. 25, 33–41. doi: 10.1111/j.1574-6941.1998.tb00458.x
Holden, J. F., Takai, K., Summit, M., Bolton, S., Zyskowski, J., and Baross, J. A. (2001). Diversity among three novel groups of hyperthermophilic deep-sea Thermococcus species from three sites in the Northeastern Pacific ocean. FEMS Microbiol. Ecol. 36, 51–60.
Horita, J., and Berndt, M. E. (1999). Abiogenic methane formation and isotopic fractionation under hydrothermal conditions. Science 285, 1055–1057. doi: 10.1126/science.285.5430.1055
Hou, J., Sievert, S. M., Wang, Y., Seewald, J. S., Natarajan, V. P., Wang, F., et al. (2020). Microbial succession during the transition from active to inactive stages of deep-sea hydrothermal vent sulfide chimneys. Microbiome 8:102. doi: 10.1186/s40168-020-00851-8
Huber, H., Thomm, M., König, H., Thies, G., and Stetter, K. O. (1982). Methanococcus thermolithotrophicus, a novel thermophilic lithotrophic methanogen. Arch. Microbiol. 132, 47–50. doi: 10.1007/BF00690816
Huber, J. A., Butterfield, D. A., and Baross, J. A. (2002). Temporal changes in archaeal diversity and chemistry in a mid-ocean ridge subseafloor habitat. Appl. Environ. Microbiol. 68, 1585–1594. doi: 10.1128/AEM.68.4.1585-1594.2002
Huber, R., Stöhr, J., Hohenhaus, S., Rachel, R., Burggraf, S., Jannasch, H. W., et al. (1995). Thermococcus chitonophagus sp. nov., a novel, chitin-degrading, hyperthermophilic archaeum from a deep-sea hydrothermal vent environment. Arch. Microbiol. 164, 255–264.
Hudson, R., de Graaf, R., Strandoo Rodin, M., Ohno, A., Lane, N., McGlynn, S. E., et al. (2020). CO2 reduction driven by a pH gradient. Proc. Natl. Acad. Sci. U.S.A. 117, 22873–22879. doi: 10.1073/pnas.2002659117
Jaeschke, A., Jørgensen, S. L., Bernasconi, S. M., Pedersen, R. B., Thorseth, I. H., and Früh-Green, G. L. (2012). Microbial diversity of loki’s castle black smokers at the arctic mid-ocean ridge. Geobiology 10, 548–561. doi: 10.1111/gbi.12009
Jeanthon, C., L’Haridon, S., Reysenbach, A. L., Corre, E., Vernet, M., Messner, P., et al. (1999). Methanococcus vulcanius sp. nov., a novel hyperthermophilic methanogen isolated from east pacific rise, and identification of Methanococcus sp. DSM 4213T as Methanococcus fervens sp. nov. Int. J. Syst. Microbiol. 49, 583–589. doi: 10.1099/00207713-49-2-583
Jeanthon, C., L’Haridon, S., Reysenbach, A. L., Vernet, M., Messner, P., Sleytr, U. B., et al. (1998). Methanococcus infernus sp. nov., a novel hyperthermophilic lithotrophic methanogen isolated from a deep-sea hydrothermal vent. Int. J. Syst. Bacteriol. 48, 913–919. doi: 10.1099/00207713-48-3-913
Jolivet, E., Corre, E., L’Harison, S., Forterre, P., and Prieur, D. (2004). Thermococcus marinus sp. nov. and Thermococcus radiotolerans sp. nov., two hyperthermophilic archaea from deep-sea hydrothermal vents that resist ionizing radiation. Extremophiles 8, 219–227. doi: 10.1007/s00792-004-0380-9
Jolivet, E., L’Haridon, S., Corre, E., Forterre, P., and Prieur, D. (2003). Thermococcus gammatolerans sp. nov., a hyperthermophilic archaeon from a deep-sea hydrothermal vent that resists ionizing radiation. Int. J. Syst. Evol. Microbiol. 53, 847–851. doi: 10.1099/ijs.0.02503-0
Jones, D. T., Taylor, W. R., and Thornton, J. M. (1992). The rapid generation of mutation data matrices from protein sequences. Comput. Appl. Biosci. 8, 275–282.
Jones, W. J., Paynter, M. J. B., and Gupta, R. (1983b). Characterization of Methanococcus maripaludis sp. nov., a new methanogen isolated from salt marsh sediment. Arch. Microbiol. 135, 91–97. doi: 10.1007/BF00408015
Jones, W. J., Leigh, J. A., Mayer, F., Woese, C. R., and Wolfe, R. S. (1983a). Methanococcus jannaschii sp. nov., an extremely thermophilic methanogen from a submarine hydrothermal vent. Arch. Microbiol. 136, 254–261. doi: 10.1007/BF00425213
Jung, H.-C., Lim, J. K., Yang, T.-J., Kang, S. G., and Lee, H. S. (2020). Direct electron transfer between the frhAGB-encoded hydrogenase and thioredoxin reductase in the nonmethanogenic archaeon Thermococcus onnurineus NA1. Appl. Environ. Microbiol. 86, e2630–e2619. doi: 10.1128/AEM.02630-19
Kaster, A.-K., Moll, J., Parey, K., and Thauer, R. K. (2011). Coupling of ferredoxin and heterodisulfide reduction via electron bifurcation in hydrogenotrophic methanogenic archaea. Proc. Natl. Acad. Sci. U.S.A. 108, 2981–2986. doi: 10.1073/pnas.1016761108
Kim, Y. J., Lee, H. S., Kim, E. S., Bae, S. S., Lim, J. K., Matsumi, R., et al. (2010). Formate-driven growth coupled with H2 production. Nature 467, 352–355. doi: 10.1038/nature09375
Klein, F., Tarnas, J. D., and Bach, W. (2020). Abiotic sources of molecular hydrogen on Earth. Elements 16, 19–24. doi: 10.2138/gselements.16.1.19
Kobayashi, T., Kwak, Y. S., Akiba, T., Kudo, T., and Horikoshi, K. (1994). Thermococcus profundus sp. nov., a new hyperthermophilic archaeon isolated from a deep-sea hydrothermal vent. Syst. Appl. Microbiol. 17, 232–236.
Konn, C., Donval, J. P., Guyader, V., Germain, Y., Alix, A.-S., Roussel, E., et al. (2022). Extending the dataset of fluid geochemistry of the menez Gwen, lucky strike, rainbow, tag and snake pit hydrothermal vent fields: Investigation of temporal stability and organic contribution. Deep Sea Res. 179:103630. doi: 10.1016/j.dsr.2021.103630
Kormas, K. A., Tivey, M. K., Von Damm, K., and Teske, A. (2006). Bacterial and archaeal phylotypes associated with distinct mineralogical layers of a white smoker spire from a deep-sea hydrothermal vent site (9°N, east pacific rise). Environ. Microbiol. 8, 909–920. doi: 10.1111/j.1462-2920.2005.00978.x
Kumagai, H., Nakamura, K., Toki, T., Morishita, T., Okino, K., Ishibashi, J.-I., et al. (2008). Geological background of the Kairei and Edmond hydrothermal fields along the central Indian ridge: Implications of their vent fluids’ distinct chemistry. Geofluids 8, 239–251. doi: 10.1111/j.1468-8123.2008.00223.x
Kurr, M., Huber, R., König, H., Jannasch, H. W., Fricke, H., Trincone, A., et al. (1991). Methanopyrus kandleri, gen. and sp. nov., represents a novel group of hyperthermophilic methanogens, growing at 110°C. Arch. Microbiol. 156, 239–247. doi: 10.1007/BF00262992
Lang, S. Q., Butterfield, D. A., Schulte, M., Kelley, D. S., and Lilley, M. D. (2010). Elevated concentrations of formate, acetate and dissolved organic carbon found at the lost city hydrothermal field. Geochim. Cosmochim. Acta 74, 941–952. doi: 10.1016/j.gca.2009.10.045
Lang, S. Q., Früh-Green, G. L., Bernasconi, S. M., Brazelton, W. J., Schrenk, M. O., and McGonigle, J. M. (2018). Deeply-sourced formate fuels sulfate reducers but not methanogens at lost city hydrothermal field. Sci. Rep. 8:755. doi: 10.1038/s41598-017-19002-5
Lang, S. Q., Früh-Green, G. L., Bernasconi, S. M., Lilley, M. D., Proskurowski, G., Méhay, S., et al. (2012). Microbial utilization of abiogenic carbon and hydrogen in a serpentinite-hosted system. Geochim. Cosmochim. Acta 92, 82–99. doi: 10.1016/j/gca.2012.06.006
Le Guellec, S., Leroy, E., Courtine, D., Godfroy, A., and Roussel, E. G. (2021). H2-dependent formate production by hyperthermophilic thermococcales: An alternative to sulfur reduction for reducing-equivalents disposal. ISME J. 15, 3423–3436. doi: 10.1038/s41396-021-01020-x
Lie, T. J., Costa, K. C., Lupa, B., Korpole, S., Whitman, W. B., and Leigh, J. A. (2012). Essential anaplerotic role for the energy-converting hydrogenase eha in hydrogenotrophic methanogenesis. Proc. Natl. Acad. Sci. U.S.A. 109, 15473–15478. doi: 10.1073/pnas.1208779109
Lilley, M. D., Butterfield, D. A., Lupton, J. E., and Olson, E. J. (2003). Magmatic events can produce rapid changes in hydrothermal vent chemistry. Nature 422, 878–881. doi: 10.1038/nature01569
Lim, J. K., Jung, H.-C., Kang, S. G., and Lee, H. S. (2017). Redox regulation of SurR by protein disulfide oxidoreductase in Thermococcus onnurineus NA1. Extremophiles 21, 491–498. doi: 10.1007/s00792-017-0919-1
Lim, J. K., Kim, Y. J., Yang, J.-A., Namirimu, T., Yang, S.-H., Park, M.-J., et al. (2020). Thermococcus indicus sp. nov., a Fe(III)-reducing hyperthermophilic archaeon isolated from the onnuri vent field of the central Indian ocean ridge. J. Microbiol. 58, 260–267. doi: 10.1007/s12275-020-9424-9
Lim, J. K., Mayer, F., Kang, S. G., and Müller, V. (2014). Energy conservation by oxidation of formate to carbon dioxide and hydrogen via a sodium ion current in a hyperthermophilic archaeon. Proc. Natl. Acad. Sci. U.S.A. 111, 11497–11502. doi: 10.1073/pnas.1407056111
Lin, T. J., Ver Eecke, H. C., Breves, E. A., Dyar, M. D., Jamieson, J. W., Hannington, M. D., et al. (2016). Linkages between mineralogy, fluid chemistry, and microbial communities within hydrothermal chimneys from the endeavour segment, Juan de Fuca ridge. Geochem. Geophys. Geosyst. 17, 300–323. doi: 10.1002/2015GC006091
Lipscomb, G. L., Keese, A. M., Cowart, D. M., Schut, G. J., Thomm, M., Adams, M. W. W., et al. (2009). SurR: A transcriptional activator and repressor controlling hydrogen and elemental sulfur metabolism in Pyrococcus furiosus. Mol. Microbiol. 71, 332–349. doi: 10.1111/j.1365-2958.2008.06525.x
Lipscomb, G. L., Schut, G. J., Scott, R. A., and Adams, M. W. W. (2017). SurR is a master regulator of the primary electron flow pathways in the order thermococcales. Mol. Microbiol. 104, 869–881. doi: 10.1111/mmi.13668
Lupa, B., Hendrickson, E. L., Leigh, J. A., and Whitman, W. B. (2008). Formate-dependent H2 production by the mesophilic methanogen Methanococcus maripaludis. Appl. Environ. Microbiol. 74, 6584–6590. doi: 10.1128/AEM.01455-08
Marteinsson, V. T., Birrien, J.-L., Reysenbach, A.-L., Vernet, M., Marie, D., Gambacorta, A., et al. (1999). Thermococcus barophilus sp. nov., a new barophilic and hyperthermophilic archaeon isolated under high hydrostatic pressure from a deep-sea hydrothermal vent. Int. J. Syst. Bacteriol. 49, 351–359.
McCliment, E. A., Voglesonger, K. M., O’Day, P. A., Dunn, E. E., Holloway, J. R., and Cary, S. C. (2006). Colonization of nascent, deep-sea hydrothermal vents by a novel archaeal and nanoarchaeal assemblage. Environ. Microbiol. 8, 114–125. doi: 10.1111/j.1462-2920.2005.00874.x
McCollom, T. M., and Seewald, J. S. (2001). A reassessment of the potential for reduction of dissolved CO2 to hydrocarbons during serpentinization of olivine. Geochim. Cosmochim. Acta 65, 3769–3778. doi: 10.1016/S0016-7037(01)00655-X
McCollom, T. M., and Seewald, J. S. (2003). Experimental constraints on the hydrothermal reactivity of organic acids and acid anions: I. Formic acid and formate. Geochim. Cosmochim. Acta 67, 3625–3644. doi: 10.1016/S0016-7037(03)00136-4
McDermott, J. M., Seewald, J. S., German, C. R., and Sylva, S. P. (2015). Pathways for abiotic organic synthesis at submarine hydrothermal fields. Proc. Natl. Acad. Sci. U.S.A. 112, 7668–7672. doi: 10.1073/pnas.1506295112
McDermott, J. M., Sylva, S. P., Ono, S., German, C. R., and Seewald, J. S. (2018). Geochemistry of fluids from Earth’s deepest ridge-crest hot-springs: Piccard hydrothermal field, mid-Cayman rise. Geochim. Cosmochim. Acta 228, 95–118. doi: 10.1016/j.gca.2018.01.021
McGonigle, J. M., Lang, S. Q., and Brazelton, W. J. (2020). Genomic evidence for formate metabolism by chloroflexi as the key to unlocking deep carbon in lost city microbial ecosystems. Appl. Environ. Microbiol. 86, e2583–e2519. doi: 10.1128/AEM.02583-19
Meyer, J. L., Akerman, N. H., Proskurowski, G., and Huber, J. A. (2013). Microbiological characterization of post-eruption “snowblower” vents at axial seamount, Juan de Fuca ridge. Front. Microbiol. 4:153. doi: 10.3389/fmicb.2013.00153
Miroshnichenko, M. L., Gongadze, G. M., Rainey, F. A., Kostyukova, A. S., Lysenko, A. M., Chernyh, N. A., et al. (1998). Thermococcus gorgonarius sp. nov. and Thermococcus pacificus sp. nov: Heterotrophic extremely thermophilic archaea from New Zealand submarine hot vents. Int. J. Syst. Bacteriol. 48, 23–29. doi: 10.1099/00207713-48-1-23
Miroshnichenko, M. L., Hippe, H., Stackebrandt, E., Kostrikina, N. A., Chernyh, N. A., Jeanthon, C., et al. (2001). Isolation and characterization of Thermococcus sibiricus sp. nov. From a western Siberia high-temperature oil reservoir. Extremophiles 5, 85–91. doi: 10.1007/s007920100175
Moon, Y.-J., Kwon, J., Yun, S.-H., Lim, H. L., Kim, M.-S., Kang, S. G., et al. (2012). Proteome analyses of hydrogen-producing hyperthermophilic archaeon Thermococcus onnurineus NA1 in different one-carbon substrate culture conditions. Mol. Cell. Proteomics 11:M111.015420. doi: 10.1074/mcp.M111.015420
Nakagawa, T., Takai, K., Suzuki, Y., Hirayama, H., Konno, U., Tsunogai, U., et al. (2006). Geomicrobiological exploration and characterization of a novel deep-sea hydrothermal system at the TOTO caldera in the Mariana volcanic Arc. Environ. Microbiol. 8, 37–49. doi: 10.1111/j.1462-2920.2005.00884.x
Niks, D., and Hille, R. (2019). Molybdenum- and tungsten-containing formate dehydrogenases and formylmethanofuran dehydrogenases: Structure, mechanism, and cofactor insertion. Protein Soc. 28, 111–122. doi: 10.1002/pro.3498
Nolling, J., and Reeve, J. N. (1997). Growth- and substrate-dependent transcription of the formate dehydrogenase (fdhCAB) operon in Methanobacterium thermoformicicum Z-245. J. Bacteriol. 179, 899–908. doi: 10.1128/jb.179.3.899-908.1997
Ownby, K., Xu, H., and White, R. H. (2005). A Methanocaldococcus jannaschii archaeal signature gene encodes for a 5-formaminoimidazole-4-carboxamide-1-β-D-ribofuranosyl 5’-monophosphate synthetase. J. Biol. Chem. 280, 10881–10887. doi: 10.1074/jbc.M413937200
Pagé, A., Tivey, M. K., Stakes, D. S., and Reysenbach, A.-L. (2008). Temporal and spatial archaeal colonization of hydrothermal vent deposits. Environ. Microbiol. 10, 874–884. doi: 10.1111/j.1462-2920.2007.01505.x
Perner, M., Kuever, J., Seifert, R., Pape, T., Koschinsky, A., Schmidt, K., et al. (2007). The influence of ultramafic rocks on microbial communities at the logatchev hydrothermal field, located 15°N on the Mid-Atlantic Ridge. FEMS Microbiol. Ecol. 16, 97–109. doi: 10.1111/j.1574-6941.2007.00325.x
Pledger, R. J., and Baross, J. A. (1989). Characterization of an extremely thermophilic archaebacterium from a black smoker polychaete (Paralvinella sp.) at the Juan de Fuca ridge. System. Appl. Microbiol. 12, 249–256.
Porat, I., Kim, W., Hendrickson, E. L., Xia, Q., Zhang, Y., Wang, T., et al. (2006). Disruption of the operon encoding Ehb hydrogenase limits anabolic CO2 assimilation in the archaeon Methanococcus maripaludis. J. Bacteriol. 188, 1373–1380. doi: 10.1128/JB.188.4.1373-1380.2006
Reveillaud, J., Reddington, E., McDermott, J., Algar, C., Meyer, J. L., Sylva, S., et al. (2016). Subseafloor microbial communities in hydrogen-rich vent fluids from hydrothermal systems along the mid-Cayman rise. Environ. Microbiol. 18, 1970–1987. doi: 10.1111/1462-2920.13173
Reysenbach, A.-L., St. John, E., Meneghin, J., Flores, G. E., Podar, M., Dombrowski, N., et al. (2020). Complex subsurface hydrothermal fluid mixing at a submarine arc volcano supports distinct and highly diverse microbial communities. Proc. Natl. Acad. Sci. U.S.A. 117, 32627–32638. doi: 10.1073/pnas.2019021117
Sakai, S., Takaki, Y., Miyazaki, M., Ogawara, M., Yanagawa, K., Miyazaki, J., et al. (2019). Methanofervidicoccus abyssi gen. nov., sp. nov., a hydrogenotrophic methanogen, isolated from a hydrothermal chimney in the mid-cayman spreading center, the Caribbean sea. Int. J. Syst. Evol. Microbiol. 69, 1225–1230. doi: 10.1099/ijsem.0.003297
Sapra, R., Bagramyan, K., and Adams, M. W. W. (2003). A simple energy-conserving system: Proton reduction coupled to proton translocation. Proc. Natl. Acad. Sci. U.S.A. 100, 7545–7550. doi: 10.1073/pnas.1331436100
Sattler, C., Wolf, S., Fersch, J., Goetz, S., and Rother, M. (2013). Random mutagenesis identifies factors involved in formate-dependent growth of the methanogenic archaeon Methanococcus maripaludis. Mol. Genet. Genomics 288, 413–424. doi: 10.1007/s00438-013-0756-6
Schink, B., Montag, D., Keller, A., and Müller, N. (2017). Hydrogen or formate: Alternative key players in methanogenic degradation. Environ. Microbiol. Rep. 9, 189–202. doi: 10.1111/1758-2229.12524
Schrenk, M. O., Kelley, D. S., Bolton, S. A., and Baross, J. A. (2004). Low archaeal diversity linked to subseafloor geochemical processes at the lost city hydrothermal field, mid-atlantic ridge. Environ. Microbiol. 6, 1086–1095. doi: 10.1111/j.1462-2920.2004.00650.x
Schut, G. J., Bridger, S. L., and Adams, M. W. W. (2007). Insights into the metabolism of elemental sulfur by the hyperthermophilic archaeon Pyrococcus furiosus: Characterization of a coenzyme A-dependent NAD(P)H sulfur oxidoreductase. J. Bacteriol. 189, 4431–4441. doi: 10.1128/JB.00031-07
Schut, G. J., Nixon, W. J., Lipscomb, G. L., Scott, R. A., and Adams, M. W. W. (2012). Mutational analyses of the enzymes involved in the metabolism of hydrogen by the hyperthermophilic archaeon Pyrococcus furiosus. Front. Microbiol. 3:163. doi: 10.3389/fmicb.2012.00163
Schut, G. J., Zhou, J., and Adams, M. W. W. (2001). DNA microarray analysis of the hyperthermophilic archaeon Pyrococcus furiosus: Evidence for a new type of sulfur-reducing enzyme complex. J. Bacteriol. 183, 7027–7036.
Seewald, J. S., Zolotov, M. Y., and McCollom, T. (2006). Experimental investigation of single carbon compounds under hydrothermal conditions. Geochim. Cosmochim. Acta 70, 446–460. doi: 10.1016/j.gca.2005.09.002
Seewald, J., Cruse, A., and Saccocia, P. (2003). Aqueous volatiles in hydrothermal fluids from the main endeavour field, northern Juan de Fuca ridge: Temporal variability following earthquake activity. Earth Planet. Sci. Lett. 216, 575–590. doi: 10.1016/S0012-821X(03)00543-0
Shock, E. L. (1990). Geochemical constraints on the origin of organic compounds in hydrothermal systems. Orig. Life Evol. Biosph. 20, 331–367. doi: 10.1007/BF01581580
Sokolova, T. G., Jeanthon, C., Kostrikina, N. A., Chernyh, N. A., Lebedinsky, A. V., Stackebrandt, E., et al. (2004). The first evidence of anaerobic CO oxidation coupled with H2 production by a hyperthermophilic archaeon isolated from a deep-sea hydrothermal vent. Extremophiles 8, 317–323. doi: 10.1007/s00792-004-0389-0
Sprott, G. D., Ekiel, I., and Patel, G. B. (1993). Metabolic pathways in Methanococcus jannaschii and other methanogenic bacteria. Appl. Environ. Microbiol. 59, 1092–1098. doi: 10.1128/AEM.59.4.1092-1098.1993
Stetter, K. O. (2006). History of discovery of the first hyperthermophiles. Extremophiles 10, 357–362. doi: 10.1007/s00792-006-0012-7
Stewart, L. C., Algar, C. K., Fortunato, C. S., Larson, B. I., Vallino, J. J., Huber, J. A., et al. (2019). Fluid geochemistry, local hydrology, and metabolic activity define methanogen community size and composition in deep-sea hydrothermal vents. ISME J. 13, 1711–1721. doi: 10.1038/s41396-019-0382-3
Takai, K., Gamo, T., Tsunogai, U., Nakayama, N., Hirayama, H., Nealson, K. H., et al. (2004b). Geochemical and microbiological evidence for a hydrogen-based, hyperthermophilic subsurface lithoautotrophic microbial ecosystem (HyperSLiME) beneath an active deep-sea hydrothermal field. Extremophiles 8, 269–282. doi: 10.1007/s00792-004-0386-3
Takai, K., Inoue, A., and Horikoshi, K. (2002). Methanothermococcus okinawensis sp. nov., a thermophilic, methane-producing archaeon isolated from a Western Pacific deep-sea hydrothermal vent system. Int. J. Syst. Evol. Microbiol. 52, 1089–1095. doi: 10.1099/00207713-52-4-1089
Takai, K., Nealson, K. H., and Horikoshi, K. (2004a). Methanotorris formicicus sp. nov., a novel extremely thermophilic, methane-producing archaeon isolated from a black smoker chimney in the central Indian ridge. Int. J. Syst. Evol. Microbiol. 54, 1095–1100. doi: 10.1099/ijs.0.02887-0
Takai, K., Nunoura, T., Horikoshi, K., Shibuya, T., Nakamura, K., Suzuki, Y., et al. (2009). Variability in microbial communities in black smoker chimneys at the NW caldera vent field, brothers volcano, kermadec Arc. Geomicrobiol. J. 26, 252–269. doi: 10.1080/01490450903304949
Takai, K., Nunoura, T., Ishibashi, J., Lupton, J., Suzuki, R., Hamasaki, H., et al. (2008). Variability in the microbial communities and hydrothermal fluid chemistry at the newly discovered mariner hydrothermal field, southern Lau basin. J. Geophys. Res. 113:G02031. doi: 10.1029/2007JG000636
Tamura, K., Stecher, G., and Kumar, S. (2021). MEGA11: Molecular evolutionary genetics analysis version 11. Mol. Biol. Evol. 38, 3022–3027. doi: 10.1093/molbev/msab120
Thauer, R. K., Kaster, A.-K., Seedorf, H., Buckel, W., and Hedderich, R. (2008). Methanogenic archaea: Ecologically relevant differences in energy conservation. Nat. Rev. Microbiol. 6, 579–591. doi: 10.1038/nrmicro1931
Topçuoğlu, B. D., Meydan, C., Nguyen, T. B., Lang, S. Q., and Holden, J. F. (2019). Growth kinetics, carbon isotope fractionation, and gene expression in the hyperthermophile Methanocaldococcus jannaschii during hydrogen-limited growth and interspecies hydrogen transfer. Appl. Environ. Microbiol. 85, e180–e119. doi: 10.1128/AEM.00180-19
Topçuoğlu, B. D., Meydan, C., Orellana, R., and Holden, J. F. (2018). Formate hydrogenlyase and formate secretion ameliorate H2 inhibition in the hyperthermophilic archaeon Thermococcus paralvinellae. Environ. Microbiol. 20, 949–957. doi: 10.1111/1462-2920.14022
Topçuoğlu, B. D., Stewart, L. C., Morrison, H. G., Butterfield, D. A., Huber, J. A., and Holden, J. F. (2016). Hydrogen limitation and syntrophic growth among natural assemblages of thermophilic methanogens at deep-sea hydrothermal vents. Front. Microbiol. 7:1240. doi: 10.3389/fmicb.2016.01240
Valentine, D. L., Chidthaisong, A., Rice, A., Reeburgh, W. S., and Tyler, S. C. (2004). Carbon and hydrogen isotope fractionation by moderately thermophilic methanogens. Geochim. Cosmochim. Acta 68, 1571–1590. doi: 10.1016/j.gca.2003.10.012
Van Haaster, D. J., Silva, P. J., Hagedoorn, P.-L., Jongejan, J. A., and Hagen, W. R. (2008). Reinvestigation of the steady-state kinetics and physiological function of the soluble NiFe-hydrogenase I of Pyrococcus furiosus. J. Bacteriol. 190, 1584–1587. doi: 10.1128/JB.01562-07
Ver Eecke, H. C., Akerman, N. H., Huber, J. A., Butterfield, D. A., and Holden, J. F. (2013). Growth kinetics and energetics of a deep-sea hyperthermophilic methanogen under varying environmental conditions. Environ. Microbiol. Rep. 5, 665–671. doi: 10.1111/1758-2229.12065
Ver Eecke, H. C., Butterfield, D. A., Huber, J. A., Lilley, M. D., Olson, E. J., Roe, K. K., et al. (2012). Hydrogen-limited growth of hyperthermophilic methanogens at deep-sea hydrothermal vents. Proc. Natl. Acad. Sci. U.S.A. 109, 13674–13679. doi: 10.1073/pnas.1206632109
Ver Eecke, H. C., Kelley, D. S., and Holden, J. F. (2009). Abundances of hyperthermophilic autotrophic Fe(III) oxide reducers and heterotrophs in hydrothermal sulfide chimneys of the Northeastern Pacific ocean. Appl. Environ. Microbiol. 75, 242–245. doi: 10.1128/AEM.01462-08
Von Damm, K. L., and Lilley, M. D. (2004). “Diffuse flow hydrothermal fluids from 9° 50’ N east pacific rise: Origin, evolution and biogeochemical controls,” in The subseafloor biosphere at mid-ocean ridges, eds W. S. D. Wilcock, E. F. DeLong, D. S. Kelley, and S. C. Cary (Washington, DC: American Geophysical Union Press), 245–268.
Von Damm, K. L., Edmond, J. M., Measures, C. I., and Grant, B. (1985). Chemistry of submarine hydrothermal solutions at Guaymas basin, gulf of California. Geochim. Cosmochim. Acta 49, 2221–2237. doi: 10.1016/0016-7037(85)90223-6
White, R. H. (1997). Purine biosynthesis in the domain archaea without folates or modified folates. J. Bacteriol. 179, 3374–3377. doi: 10.1128/jb.179.10.3374-3377.1997
Windman, T., Zolotova, N., Schwandner, F., and Shock, E. L. (2007). Formate as an energy source for microbial metabolism in chemosynthetic zones of hydrothermal ecosystems. Astrobiology 7, 873–890. doi: 10.1089/ast.2007.0127
Wood, G. E., Haydock, A. K., and Leigh, J. A. (2003). Function and regulation of the formate dehydrogenase genes of the methanogenic archaeon Methanococcus maripaludis. J. Bacteriol. 185, 2548–2554. doi: 10.1128/JB.185.8.2548-2554.2003
Wu, C.-H., Schut, G. J., Poole, F. II, Haja, D. K., and Adams, M. W. W. (2018). Characterization of membrane-bound sulfane reductase: A missing link in the evolution of modern day respiratory complexes. J. Biol. Chem. 293, 16687–16696. doi: 10.1074/jbc.RA118.005092
Yang, H., Lipscomb, G. L., Keese, A. M., Schut, G. J., Thomm, M., Adams, M. W. W., et al. (2010). SurR regulates hydrogen production in Pyrococcus furiosus by a sulfur-dependent redox switch. Mol. Microbiol. 77, 1111–1122. doi: 10.1111/j.1365-2958.2010.07275.x
Yang, J., Lee, S. H., Ryu, J. Y., Lee, H. S., and Kang, S. G. (2022). A novel NADP-dependent formate dehydrogenase from the hyperthermophilic archaeon Thermococcus onnurineus NA1. Front. Microbiol. 13:844735. doi: 10.3389/fmicb.2022.844735
Zeng, X., Zhang, X., Jiang, L., Alain, K., Jebbar, M., and Shao, Z. (2013). Palaeococcus pacificus sp. nov., an archaeon from deep-sea hydrothermal sediment. Int. J. Syst. Evol. Microbiol. 63, 2155–2159. doi: 10.1099/ijs.0.044487-0
Zhao, H., Wood, A. G., Widdel, F., and Bryant, M. P. (1988). An extremely thermophilic Methanococcus from a deep-sea hydrothermal vent and its plasmid. Arch. Microbiol. 150, 178–183. doi: 10.1007/BF00425159
Zhao, W., Zeng, X., and Xiao, X. (2015). Thermococcus eurythermalis sp. nov., a conditional piezophilic, hyperthermophilic archaeon with a wide temperature range for growth, isolated from an oil-immersed chimney in the Guaymas basin. Int. J. Syst. Evol. Microbiol. 65, 30–35. doi: 10.1099/ijs.0.067942-0
Keywords: formate, hydrogen, hydrothermal vent, hyperthermophiles, formate dehydrogenase, hydrogenase, Thermococci, Methanococci
Citation: Holden JF and Sistu H (2023) Formate and hydrogen in hydrothermal vents and their use by extremely thermophilic methanogens and heterotrophs. Front. Microbiol. 14:1093018. doi: 10.3389/fmicb.2023.1093018
Received: 08 November 2022; Accepted: 20 February 2023;
Published: 06 March 2023.
Edited by:
Mark Alexander Lever, The University of Texas at Austin, United StatesReviewed by:
Stefan M. Sievert, Woods Hole Oceanographic Institution, United StatesCopyright © 2023 Holden and Sistu. This is an open-access article distributed under the terms of the Creative Commons Attribution License (CC BY). The use, distribution or reproduction in other forums is permitted, provided the original author(s) and the copyright owner(s) are credited and that the original publication in this journal is cited, in accordance with accepted academic practice. No use, distribution or reproduction is permitted which does not comply with these terms.
*Correspondence: James F. Holden, amhvbGRlbkB1bWFzcy5lZHU=
Disclaimer: All claims expressed in this article are solely those of the authors and do not necessarily represent those of their affiliated organizations, or those of the publisher, the editors and the reviewers. Any product that may be evaluated in this article or claim that may be made by its manufacturer is not guaranteed or endorsed by the publisher.
Research integrity at Frontiers
Learn more about the work of our research integrity team to safeguard the quality of each article we publish.