- The Key Laboratory for Silviculture and Conservation of Ministry of Education, College of Forestry, Beijing Forestry University, Beijing, China
Cytospora chrysosperma is a destructive plant pathogenic fungus, which causes canker disease on numerous woody plants. However, knowledge concerning the interaction between C. chrysosperma and its host remains limited. Secondary metabolites produced by phytopathogens often play important roles in their virulence. Terpene cyclases (TC), polyketide synthases (PKS) and non-ribosomal peptide synthetases (NRPS) are the key components for the synthesis of secondary metabolites. Here, we characterized the functions of a putative terpene type secondary metabolite biosynthetic core gene CcPtc1 in C. chrysosperma, which was significantly up-regulated in the early stages of infection. Importantly, deletion of CcPtc1 greatly reduced fungal virulence to the poplar twigs and they also showed significantly reduced fungal growth and conidiation compared with the wild-type (WT) strain. Furthermore, toxicity test of the crude extraction from each strain showed that the toxicity of crude extraction secreted by ΔCcPtc1 were strongly compromised in comparison with the WT strain. Subsequently, the untargeted metabolomics analyses between ΔCcPtc1 mutant and WT strain were conducted, which revealed 193 significantly different abundant metabolites (DAMs) inΔCcPtc1 mutant compared to the WT strain, including 90 significantly downregulated metabolites and 103 significantly up-regulated metabolites, respectively. Among them, four key metabolic pathways that reported to be important for fungal virulence were enriched, including pantothenate and coenzyme A (CoA) biosynthesis. Moreover, we also detected significant alterations in a series of terpenoids, among which (+)-ar-turmerone, pulegone, ethyl chrysanthemumate, and genipin were significantly down-regulated, while cuminaldehyde and (±)-abscisic acid were significantly up-regulated. In conclusion, our results demonstrated that CcPtc1 acts as a virulence-related secondary metabolism factor and provides new insights into the pathogenesis of C. chrysosperma.
1. Introduction
Filamentous fungi are known to produce an abundant of bioactive secondary metabolites that involve in fungal transcription, development, intercellular communication, and virulence (Wu et al., 2012; Brakhage, 2013). Remarkably, many of secondary metabolites show a wide range of important applications in antiviral, antibiotic, antitumor, antihypercholesterolemic, and immunosuppressant activities as well as phytotoxic and mycotoxic activities (Bok and Keller, 2004; Saha et al., 2020). Phytopathogenic fungi will produce various mycotoxins during the interaction processes, which can promote the fungal colonization, induce plant cell death and cause disease (Wang et al., 2014), such as the T-toxin from Cochliobolus heterostrophus (Wu et al., 2012), deoxynivalenol (DON) from Fusarium spp. (Feizollahi and Roopesh, 2022), and aflatoxin produced by Aspergillus flavus (Yang et al., 2020). These secondary metabolites have been proven to be crucial for virulence and are generally synthesized by secondary metabolite biosynthetic gene clusters (SMBGCs) including the polyketide synthase type (PKS), non-ribosomal peptide synthetase type (NRPS), a dimethylallyl tryptophan synthetase type (DMATS) and terpene cyclase type (TC) (Rokas et al., 2018; Jia et al., 2019). It has been reported that the velvet family proteins (LaeA, VeA, and VelB) and cytochrome P450 related enzymes (CYP) are also contribution to the synthesis of secondary metabolites (Bok et al., 2006; Keller et al., 2006; Bayram et al., 2008).
To date, mycotoxins synthesized by SMBGCs have been implicated as crucial pathogenic factors for pathogens, such as T-toxin, Aflatoxin B1 (AFB1), cercosporin toxin, fusaoctaxin A, and botrydial (Deighton et al., 2001; Choquer et al., 2005; Siewers et al., 2005; Baker et al., 2006; Myung et al., 2012; Jia et al., 2019; Caceres et al., 2020). AFB1 is a toxic secondary metabolite produced by several fungal species belonging to the Flavi section of the Aspergillus genus (Caceres et al., 2016). Prevalence of the cercosporin toxin biosynthesis (CTB) gene clusters as virulence promoters in Cercospora sp. (Daub and Ehrenshaft, 2000; Choquer et al., 2005; Ebert et al., 2019). In addition, numerous studies have shown that the backbone genes of the SMBGCs (NRPS, PKS, and TC) are also strongly associate with the pathogenicity of pathogenic fungi, including Verticillium dahliae, Pyrenophora tritici-repentis, Diaporthe helianthi, Trichoderma virens, and C. heterostrophus (Baker et al., 2006; Dorrestein and Kelleher, 2006; Brakhage and Schroeckh, 2011; Crutcher et al., 2013; Ruocco et al., 2018; Rawlinson et al., 2019; Li et al., 2022). For instance, in Alternaria alternata, the production of HC and AM toxin, virulence factors (pathogen determinants that cause damage to the infected host), were affected when NRPS was deleted (Johnson et al., 2000). Moreover, PKS catalyzes the biosynthesis of polyketides, which consist of a large and diverse group of secondary metabolites, such as antibiotics, toxins, and melanin (Kim et al., 2005; Ruocco et al., 2018; Li et al., 2022). However, compared with the NRPS and PKS, there are relatively few functional studies on TC, especially in phytopathogenic fungi.
Generally, plant pathogenic fungi produce kinds of related compounds rather than a single toxin, and they are often differing in biological activity. Fusarium graminearum, the causal agent of Fusarium head blight, crown rot and seedling blight on wheat, produces several mycotoxins in the infected plants, including trichothecenes, zearalenone and fusaoctaxin A. Valsa mali, the pathogen of apple canker disease, can produce several toxins such as protocatechuic acid, p-hydroxybenzoic acid, p-hydroxyacetophenone, phloroglucinol, 3-(hydroxyphenyl) propionic acid, and isocoumarins, which play important roles in promoting the infection of V. mali on apple trees (Wang et al., 2014; Feng et al., 2020).
The necrotrophic phytopathogenic fungus Cytospora chrysosperma (Pers.) Fr is the causal agent of canker disease which causes serious stem damage in numerous woody plants annually, especially, leading to serious forestry, ecological and economic losses each year in northwest China (Adams et al., 2006; Kepley et al., 2015; Wang et al., 2015). Until now, several genes associated with pathogenicity were identified and functionally analyzed in C. chrysosperma (Wang and Wang, 2020; Han et al., 2021a,b; Wen et al., 2021). For instance, Sge1 homolog played important roles in fungal virulence and expression of effector genes in C. chrysosperma (Han et al., 2021b). In addition, two conserved MAPK members in C. chrysosperma, CcPmk1, and CcSlt2, were characterized as vital pathogenicity-related regulators, which regulate fungal virulence and expression of secondary metabolism gene clusters (Yu et al., 2019, 2022a; Xiong et al., 2021). Notably, C. chrysosperma is considered to be a necrotrophic fungus similar to V. mali which tend to kill its host cells rapidly (Biggs et al., 1983; Adams et al., 2006; Yin et al., 2015). Previous studies have shown that V. mali synthesizes a variety of toxins to promote the infection process (Wang et al., 2014; Feng et al., 2020; Zhang et al., 2022). However, the molecular mechanism and functions of SMBGCs and backbone genes, as well as their effects on pathogenicity, is scarcely reported in C. chrysosperma. Lately, a total of 68 secondary metabolite core genes were predicted in C. chrysosperma genome including NRPS and NRPS-like, PKS, terpene, hybrid SMB, and other SMB gene models (Xiong et al., 2021). Based on these findings, we believe that revealing the function of secondary metabolic genes is important for the exploration of the molecular pathogenesis of C. chrysosperma.
Our previous works revealed that the whole terpene type gene cluster (GME3317_g to GME3324_g) and NRPS-T1PKS hybrid type gene clusters (GME3437_g to GME3444_g) were all significantly down-regulated in the ΔCcPmk1 and ΔCcSlt2. Remarkably, the backbone gene CcPpns1 (GME3440_g) of gene cluster from GME3437_g to GME3444_g was important for fungal virulence (Yu et al., 2022b). In this study, we showed that CcPtc1, the backbone gene of terpene type gene cluster, markedly affected morphological development, pathogenicity, and toxic secondary metabolites of C. chrysosperma. Moreover, the secondary metabolites secreted by C. chrysosperma could cause cell death in the host plants. Toxicity tests also proved that absence of CcPtc1 caused markedly compromised toxicity of C. chrysosperma in comparison with wild-type (WT) strain. Furthermore, metabolomic analyses of the ΔCcPtc1 and WT displayed large quantities of different abundant metabolites (DAMs), many of which has been reported correlated with pathogenicity in phytopathogenic fungi, such as pantothenate and trehalose 6-phosphate. In addition, deletion of CcPtc1 also resulted in changes in terpenoids in C. chrysosperma. Collectively, the results increased our knowledge on the TC type secondary metabolism gene in fungal pathogenicity, which might provide new clues for the control strategies of canker disease.
2. Materials and methods
2.1. Fungal strains and cultivation conditions
The WT strain of C. chrysosperma (CFCC 89981) used in this study was preserved in the forest pathology laboratory of Beijing Forestry University (Fan et al., 2020). Strains used in this study were regularly cultured on potato dextrose agar medium (PDA; 20% potato extract, 2% glucose, and 1.5% agar) at 25°C. For DNA isolation and RNA isolation, mycelia were cultured in liquid potato dextrose broth medium (PDB; 20% potato extract and 2% glucose) for 2 days at 150 rpm, 25°C. Populus bark broth medium (PBB; 30% 1-year-old polar branch extract and 1% glucose) was used for the extraction of crude secondary metabolites.
2.2. Sequence and phylogenetic analysis of CcPtc1
The sequence of terpene type gene cluster (GME3317_g to GME3324_g) were acquired from the draft genome sequence of C. chrysosperma, which had been sequenced by our laboratory (NCBI GenBank accession number JAEQMF000000000). The genome sequences of the terpene type gene cluster and the corresponding CDS sequences were committed to the Gene Structure Display Server (GSDS)1 to analyze the number and alignment of introns and exons. TBtools (V0.66836) was used to visualize the relative expression level (Chen et al., 2020). The homologs of this terpene type gene cluster were searched in the genome of other microorganisms in the JGI database. The domain structures of terpene type gene cluster were annotated using the InterProScan tool.2 In addition, phylogenetic analysis was conducted with MEGA 10.0 software using the full-length protein sequences and neighbor-joining method with 1,000 bootstrap replications.
2.3. Targeted disruption of CcPtc1 and mutant complementation
The split marker method was used to construct the CcPtc1 gene deletion (ΔCcPtc1) mutants as previously described (Catlett et al., 2003; Goswami, 2012). According to this method, the upstream (∼1.3 kb) and downstream (∼1.2 kb) flanking sequences of CcPtc1 were amplified by primer pairs CcPtc1-5Ffor/CcPtc1-5Frev and CcPtc1-3Ffor/CcPtc1-3Frev, respectively (Supplementary Table 1). The hygromycin B resistance cassette (HPH) was amplified by specific primer pairs hygromycinfor and hygromycinrev, which included approximately 20 bp overlapped 5′ and 3′flanking sequences, respectively. Then, the resulting upstream and downstream fragments were fused with two-thirds of the hygromycin B resistance cassette by overlap PCR using primer pairs CcPtc1-5Ffor/HY-R and YG-F/CcPtc1-3Frev, respectively. The two overlapping fragments were directly transformed into protoplasts of the WT strain by using the PEG-mediated transformation, and the transformants were selected on TB3 agar medium supplemented with 20 μg/ml hygromycin B. All transformants were identified by PCR assays with the primer pairs External-CcPtc1for/External-CcPtc1rev and Internal-CcPtc1for/Internal-CcPtc1rev to screening the successful replacement transformants. In addition, to analyze homologous recombination events in the transformants, southern blotting analysis was performed with the DIG High Prime DNA Labeling and Detection Starter Kit I, following the manufacturer’s protocol (Roche, Germany). BglI was used to digest the genomic DNA extracted from the WT strain and the transformants. The probes were amplified by the primers ProbeHPHfor and ProbeHPHrev from HPH and the primers ProbeCcPtc1for and ProbeCcPtc1rev for CcPtc1.
To generation the CcPtc1 gene complementation construct, a fragment containing the entire length of the CcPtc1 coding region along with native promoter sequence and terminator sequence was cloned from gDNA using the primer pair CcPtc1-Compfor/CcPtc1-Comprev. The resulting PCR products were co-transformed into protoplasts of the ΔCcPtc1-11 strains with a geneticin-resistant cassette. After that, we selected the transformants in TB3 medium supplemented with 40 μg/ml geneticin. Successful complementation was confirmed by PCR with the primer pair Internal-CcPtc1for/Internal-CcPtc1rev. The complementation strain was named ΔCcPtc1/PTC1 in this study. All primers used in gene deletion and complementation were listed in Supplementary Table 1.
2.4. Fungal growth and conidiation
To analyze the differences in vegetative growth and conidiation among the WT, deletion mutants, and complemented strains, each strain was inoculated on the PDA plates at 25°C in the dark. The growth and conidial formation were observed at 24, 48, and 60 h and 30 days post inoculation. Each experiment was replicated at least three times.
2.5. Pathogenicity assays
Healthy annual poplar twigs collected from the nursery garden in the Beijing forestry university were used for pathogenicity assay. Hyphal plugs of the WT, gene deletion mutants, and complemented strains were inoculated on the 20-cm-long twigs which scorched with a flat iron (5 mm in diameter). The inoculated twigs were incubated at 25°C for 5∼8 days under moist conditions and photographs were taken after 5 days. The experiments were repeated at least three times.
2.6. Extraction of crude extracts and toxicity tests
For analysis of metabolite production, the plugs of WT and ΔCcPtc1 were cultivated into PBB medium at 25°C and 150 rpm for 10 days. Culture filtrates of WT, ΔCcPtc1, and PBB medium were extracted with equal volume of ethyl acetate for three times, and the organic phase was saved. Organic phases were combined to obtain crude extracts of secondary metabolites. The organic phases were distilled using a rotary evaporator under 45°C and re-dissolved in 20 ml Dimethyl sulfoxide (DMSO). The phytotoxic activities of the crude extracts were tested on polar leaves using the leaf puncture method. PBB crude extract–treated and DMSO-treated samples were kept as control. Inoculated leaves were incubated in a petri dish under 25°C. The pictures were taken at 24 h after treatment. The experiment was performed with three biological replicates at least.
2.7. RNA extraction and quantitative RT-PCR
To analyze the expression levels of other genes among the terpene type gene cluster in the CcPtc1 deletion mutant, the WT, ΔCcPtc1-11 and ΔCcPtc1-14 strains were cultivated in PDB supplemented with sterilized poplar twigs at 25°C and 150 rpm for 48 h. Mycelium was harvested by Miracloth (Calbiochem). Then flash-frozen in liquid nitrogen and ground to powder. Total RNA was extracted from powder using RNA Easy Fast Plant Tissue Kit (TIANGEN, China) according to the manufacturer’s protocol. First-strand cDNA was prepared using ABScript II cDNA Fist-Strand Synthesis Kit (ABclonal, China).
Quantitative real-time PCR was performed with SuperReal PreMix Plus (ABclonal, China) on the Applied Biosystems 7500 Real-Time PCR system (Applied Biosystems). The CcActin gene of C. chrysosperma was used as an internal reference for all RT-qPCR experiments. Relative expression was calculated using the 2–Δ Δ Ct method. The experiment was performed in triplicate with three independent technical replicates each. All primers used in the present study were listed in Supplementary Table 1.
2.8. Analysis of metabolomics data
To prepare the metabolome samples, the WT and ΔCcPtc1 strains was simultaneously cultured in PBB medium with the same conditions as crude extraction assay. Each strain contained six repeats and generated twelve datasets.
All samples were placed in the EP tubes and resuspended with prechilled 80% methanol by well vortex. After whirled (melted on ice for 30 s), sonification (6 min), and centrifuged (5,000 rpm, 4°C for 1 min), the supernatant of all samples was freeze-dried and dissolved with 10% methanol. Finally, the solution was injected into the LC-MS/MS system for further analysis. UHPLC-MS/MS was used to analyze the composition of the WT and ΔCcPtc1 strains using a Vanquish UHPLC system (Thermo Fisher, Germany) coupled with an Orbitrap Q ExactiveTM HF mass spectrometer (Thermo Fisher, Germany) in Novogene Co., Ltd. (Beijing, China). Samples were injected onto a Hypesil Gold column (100 × 2.1 mm, 1.9 μm) with a 12-min linear gradient at a flow rate of 0.2 mL/min. Q ExactiveTM HF mass spectrometer was operated in positive/negative polarity mode with spray voltage of 3.5 kV, capillary temperature of 320°C, sheath gas flow rate of 35 psi and aux gas flow rate of 10 L/min, S-lens RF level of 60, Aux gas heater temperature of 350°C.
The raw data generate by UHPLC-MS/MS were processed using the Compound Discoverer 3.1 (CD3.1, Thermo Fisher) to perform peak alignment, peak picking, and quantitation for each metabolite. The KEGG database,3 Human Metabolome Database (HMDB) database,4 and LIPIDMaps database5 were used to annotate these metabolites. Principal components analysis (PCA) and Partial least squares discriminant analysis (PLS-DA) were performed at metaX. We applied univariate analysis (t-test) to calculate the statistical significance (p-value). Metabolites with Variable Importance in Projection (VIP) > 1, fold change (FC) > 1.2 or FC < 0.833, and p-value < 0.05 were considered to be DAMs. The functions of these metabolites and metabolic pathways were annotated using the KEGG database. Enrichment metabolic pathways of differential metabolites were selected with x/n > y/N and p < 0.05.
3. Results
3.1. Identification of terpene type gene cluster in C. chrysosperma
Our previous works found that two putative secondary metabolism related gene clusters (GME3437_g to GME3444_g and GME3317_g to GME3324_g) were regulated by the key pathogenicity factor CcPmk1 and CcSlt2. In this study, the backbone gene (GME3321_g) of gene cluster from GME3317_g to GME3324_g was functional characterized. GME3321_g contained a terpene cyclase-like 2 protein family domain (IPR 034686), therefore, we named it as CcPtc1 (Figure 1A). Additionally, GME3317_g, GME3322_g, and GME3323_g contains a conserved cytochrome P450 domain (IPR 001128), respectively (Figure 1A). The structure of the 8 genes among these terpene type gene cluster were characterized using the GSDS, which contained 0 to 7 introns (Supplementary Figure 1). In addition, we aimed to determine the putative homologs of this gene cluster in other fungal species. Therefore, the whole sequence of this gene cluster was used as queries to search through the genome sequences of Magnaporthe oryzae, V. dahliae, Botrytis cinerea, Cryphonectria parasitica, Saccharomyces cerevisiae, Sclerotinia sclerotiorum, and Fusarium species. Nevertheless, no significant hits were obtained in these fungal species.
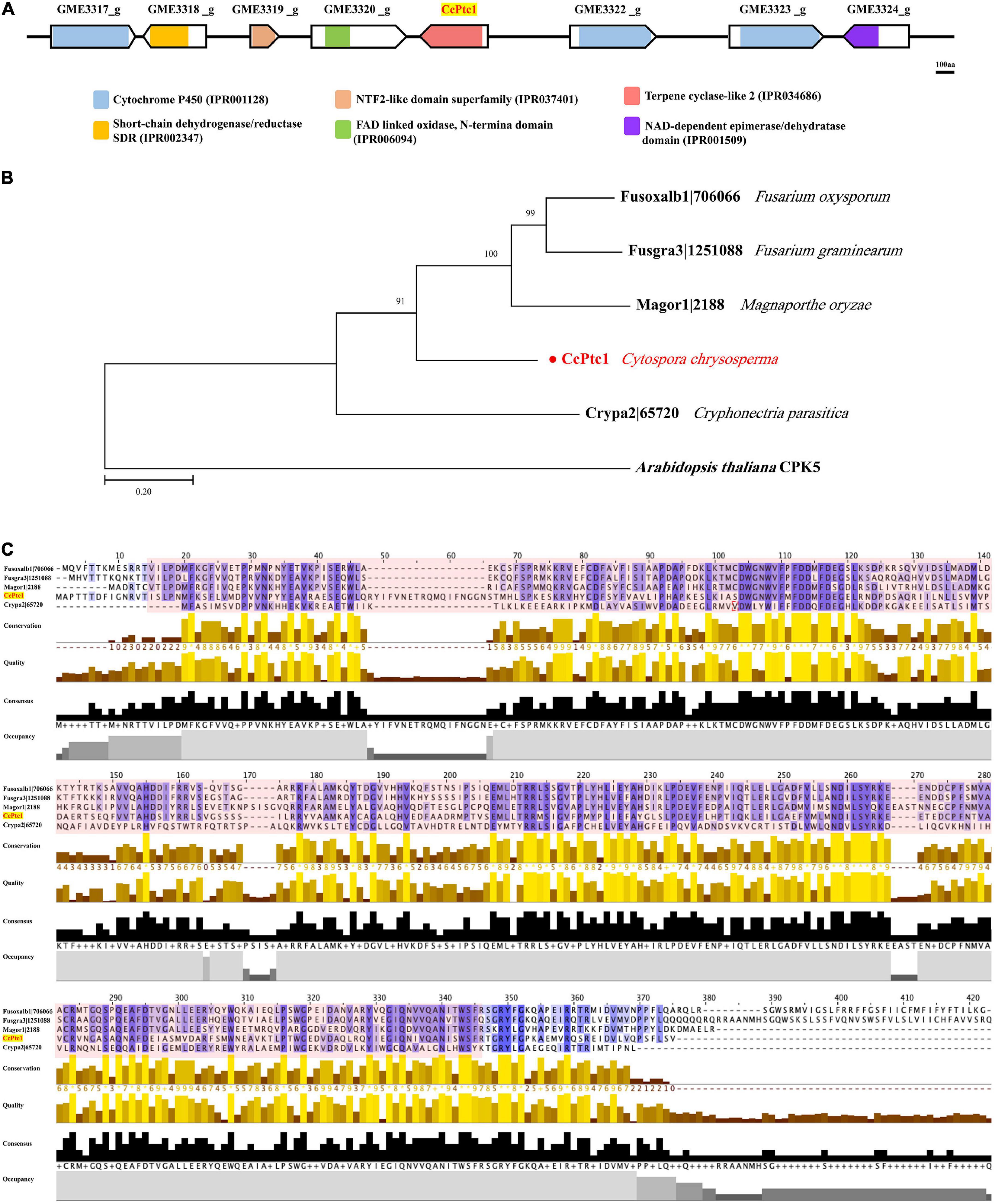
Figure 1. Domain and phylogenetic analysis of terpene type gene cluster (GME3317_g ∼ GME3324_g). (A) The line with the arrow indicates the scheme of the terpene type gene cluster (GME3317_g ∼ GME3324_g) proteins; domains were predicted using the InterProScan tool. Different color boxes indicate different domain categories. Bar = 100 aa. (B) Phylogenetic analysis of the backbone gene CcPtc1 homologs from four fungi. Bootstrap percentages over 50% are indicated at the nodes. The tree was rooted in outgroup taxon Arabidopsis thaliana. The detailed information of the orthologs is listed in Supplementary Table 2. (C) Multiple sequence alignment of terpene cyclase-like 2 protein from four fungi species. The protein domain (IPR 034686) is shadowed in red. The detailed information of the terpene cyclase-like 2 protein orthologs are listed in Supplementary Table 2.
Subsequently, we only identified the homologs of backbone gene CcPtc1 in other fungal species, and many homologs were found because of the conserved sequence of terpene cyclase-like 2 protein family domain. Homologos protein sequences of CcPtc1 were downloaded from four fungal species, including M. oryzae, Fusarium oxysporum, F. graminearum, and C. parasitica, to produce a phylogenetic tree (Figure 1B). The information of all these proteins were listed in Supplementary Table 2. Moreover, we analyzed the sequences and protein domain (IPR 034686) of terpene cyclase-like 2 proteins in these four fungi (Figure 1C). The overall amino acid identity of CcPtc1 to the Magor1| 2188, Fusoxalb1| 706066, and Fusgra3| 1251088 is with an identity of 44, 48, and 45%, respectively (Supplementary Table 2).
3.2. The expression of terpene type gene cluster is induced during the early infection process
To investigate the expression of terpene type gene cluster during infection processes, we collected the expression data for these cluster genes from our previous transcriptome data of the initial infection process [0 and 1 days post-inoculation (dpi)] in poplar branches (Li et al., 2021). The expression levels of genes in this terpene type gene cluster were shown in Figure 2A, and half of the genes (4/8) were significantly upregulated at 1 dpi compared to 0 dpi. We then analyzed the expression levels of these cluster genes during the middle and late C. chrysosperma infection process (7 dpi and 15 dpi) of poplar branches. As shown in Supplementary Figure 2, most of the genes (7/8) were significantly upregulated at 7 dpi compared to 0 dpi, and 3 out of 8 genes were significantly upregulated at 15 dpi compared to 0 dpi. Notably, the expression of the backbone gene (CcPtc1) was significantly up-regulated during the whole infection processes.
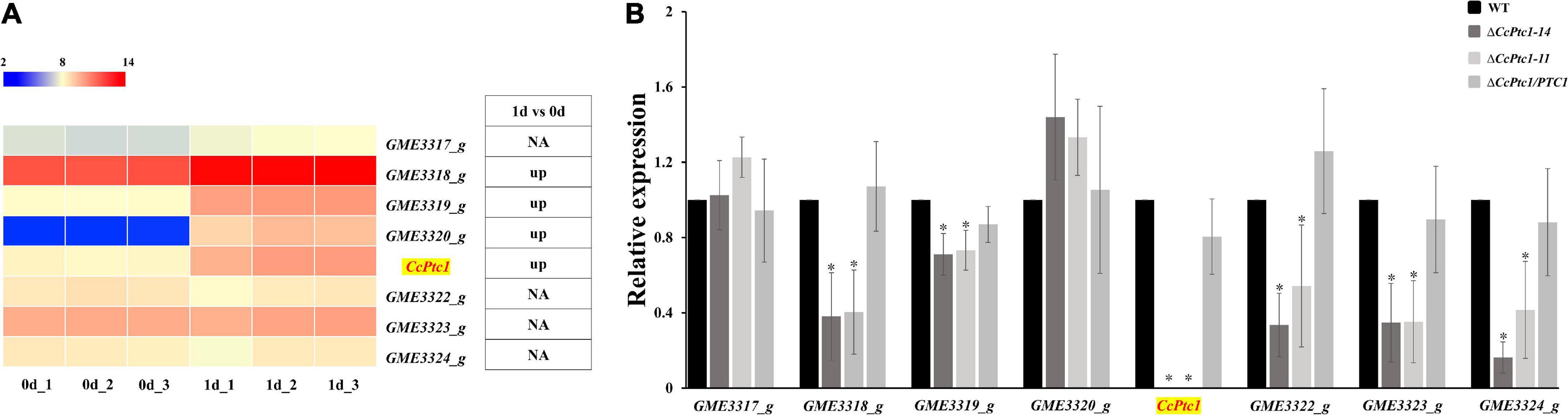
Figure 2. The expression patterns of terpene type gene cluster at the initial infection stages. (A) The heatmap shows the expression data of the genes in the cluster at the initial stages of infection. The original fragments per kilobase per million (FPKM) values of the cluster genes were transformed by log2. The color scale ranging from blue to red indicates increasing expression levels. The differentially expressed genes (|log2foldchange| ≥ 1, p-value < 0.05) are indicated. The “up” represents significantly up regulated. (B) Expression of terpene type gene cluster in wild-type (WT) and CcPtc1 deficient mutants. RT-PCR was used to determine the cluster genes expression levels in the WT and two ΔCcPtc1 deletion mutant strains on PDB (supplemented with sterilized poplar twigs to mimic the states of infection) at 2 dpi. The CcActin gene was used as the reference gene. The error bars represent the standard error based on three independent biological replicates with three technical replicates each. The data were analyzed using one way ANOVA and Duncan’s range test. The asterisks indicate significant differences (p-value < 0.05).
In order to reveal the functions of CcPtc1 during the infection processes, we generated two CcPtc1 deletion mutants ΔCcPtc1-11 and ΔCcPtc1-14, which had been confirmed by the PCR and Southern blot analysis (Supplementary Figures 3A, B). For the complementation of ΔCcPtc1, a fragment containing the native promoter and the CcPtc1 coding sequence was isolated from genomic DNA and transformed into the ΔCcPtc1-11 strain and screened by PCR (Supplementary Figure 3C). One complemented strain (ΔCcPtc1/PTC1) and two mutant strains (ΔCcPtc1-11 and ΔCcPtc1-14) were used for subsequent phenotypic analyses.
To determine the effects of CcPtc1 on the other genes among its cluster (GME3317_g to GME3324_g), we analyzed the expression levels of these genes in the WT and ΔCcPtc1 strains (Figure 2B) in mimetic infection process. The qRT-PCR assays show that, 5 out of 7 genes in this cluster exhibited significantly reduced expression levels in the CcPtc1 deletion mutants compared with those in the WT strain. These results suggest that this terpene type gene cluster, especially the backbone genes CcPtc1, may play important roles in the fungal pathogenicity of C. chrysosperma.
3.3. CcPtc1 is indispensable for hyphal growth and conidiation
To determine the potential roles of CcPtc1 in C. chrysosperma vegetative growth, we cultivated the WT, CcPtc1 deletion mutants and complemented strains on PDA plates at 25°C for 60 h. Development phenotypic analysis showed that ΔCcPtc1 exhibited an apparent smaller colony diameter compared with the WT, and the growth defects of the knockout strains were restored in complementation strain (Figures 3A, B). Compared with the WT and ΔCcPtc1/PTC1 strains, the CcPtc1 deletion mutant showed approximately 17.13% reduction in hyphal growth on PDA plates.
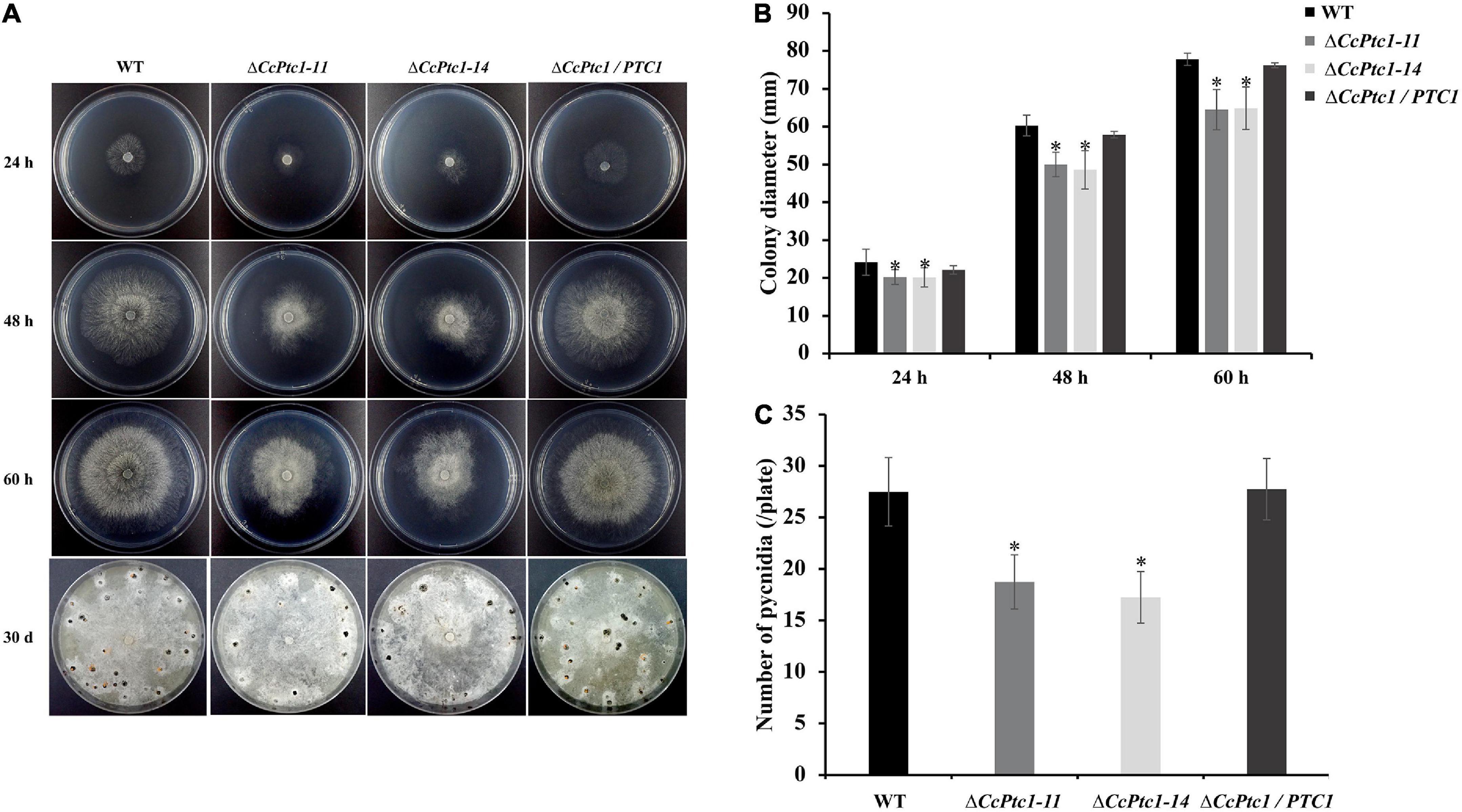
Figure 3. Growth and conidiation phenotype of Cytospora chrysosperma wild-type (WT) and ΔCcPtc1. (A) Colony morphologies and pycnidia formation of the WT, mutant, and complemented strains after 24, 48, and 60 h and 30 days of growth on PDA plates. (B) Bar chart showing the colony diameter strains on PDA plates. (C) Quantification of pycnidia production in four strains based on three independent experiments. In bar graphs, error bars represent the standard error based on three independent biological replicates with three technical replicates each. The data were analyzed using Duncan’s range test. The asterisks indicate significant differences (p-value < 0.05). All pathogenicity experiments were performed three times.
To investigate whether CcPtc1 contributed to conidiation, we then calculated the number of pycnidium produced by the WT strain and CcPtc1 deletion mutants. The results suggest that ΔCcPtc1 produced significantly decreased amount of pycnidia compared to WT strain on PDA after 30 days (a reduction of approximately 34.5%) (Figures 3A, C). Taken together, these results suggest that CcPtc1 is required for conidiation and fungal growth.
3.4. CcPtc1 is required for fungal virulence
As mentioned above, the expression level of CcPtc1 was significantly increased during the early infection stages. Thus, it prompted us to explore the roles of CcPtc1 in fungal virulence. We performed a pathogenicity test on detached poplar twigs with inoculating mycelial plugs of the WT, CcPtc1 deletion mutants, and complemented strains. As shown in Figure 4A, poplar twigs inoculated with WT strains exhibited severely typical symptoms at 4∼7 dpi, while the poplar twigs inoculated with ΔCcPtc1 deletion mutants showed significantly reduced lesion areas (over 70% reduction). In addition, the complemented strain ΔCcPtc1/PTC1 displayed a comparable lesion size as the WT strain (Figures 4A, B). Overall, these results demonstrate that the CcPtc1 contributes to fungal virulence during the infection process.
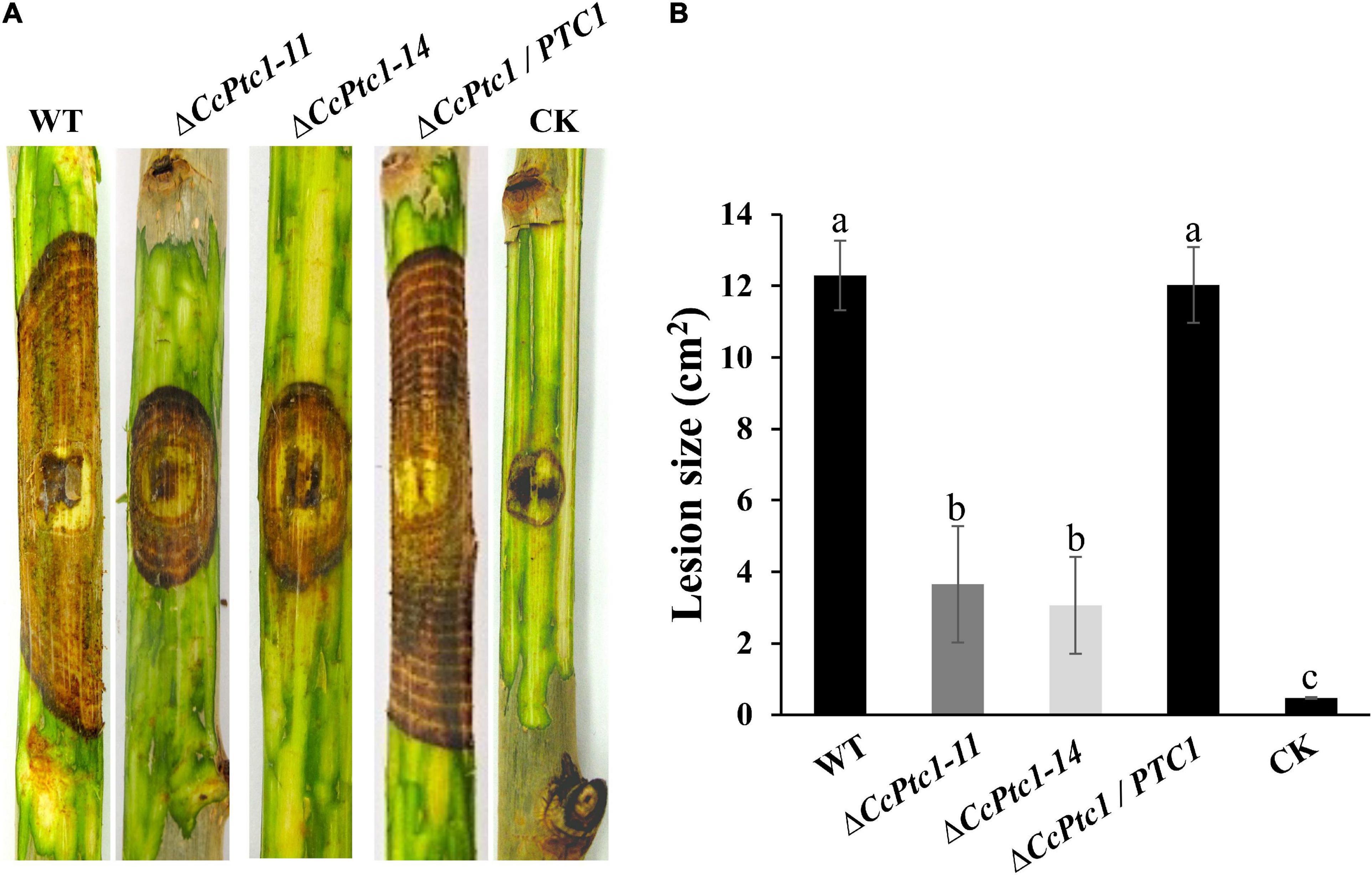
Figure 4. Infection phenotype of Cytospora chrysosperma wild-type (WT) and ΔCcPtc1. (A) Pathogenicity test of ΔCcPtc1 on detached poplar twigs. WT, CcPtc1 deletion strain, complemented strain ΔCcPtc1/PTC1 and CK (PDA plugs) were inoculated onto detached twigs of poplar and incubated at 25°C. Typical twigs were photographed at 7 dpi. (B) The bar chart shows average lesion size of twigs quantified at 7 dpi and means ± SD. CK means inoculating PDA plugs after scalding. The error bars represent the standard errors based on three independent biological replicates with three technical replicates each. The data were analyzed using Duncan’s range test. The different letters indicate significant differences from the WT strain (p-value < 0.05).
3.5. CcPtc1 affects the production of toxic secondary metabolites in C. chrysosperma
CcPtc1 was predicted as the backbone gene of the secondary metabolite gene cluster and it was required for fungal virulence, thus we speculated whether the impaired pathogenicity may result from the reduced production of secondary metabolites. To investigate the role of CcPtc1 in the production of secondary metabolites, toxicity tests were conducted. We collected culture filtrates of WT strain, ΔCcPtc1 strain, and uninoculated medium to obtain crude extracts of secondary metabolites. Toxicity of these crude secondary metabolites was tested on poplar leaves.
Infiltration the crude extracts of WT and ΔCcPtc1 into poplar leaves with leaf puncture method produced a brown ovoid region of necrosis which extend over the entire infiltration zone at 24 h after treatment (Figure 5A). On the contrary, the DMSO solvent and uninoculated medium control did not produce any necrotic symptom. Although necrotic symptoms were similar across WT and CcPtc1 deletion mutants, crude secondary metabolites from WT were more effective than those from ΔCcPtc1, the area of the necrotic lesions inoculated with the ΔCcPtc1 was reduced by approximately 50.3% compared with the WT strain (Figures 5A, B).
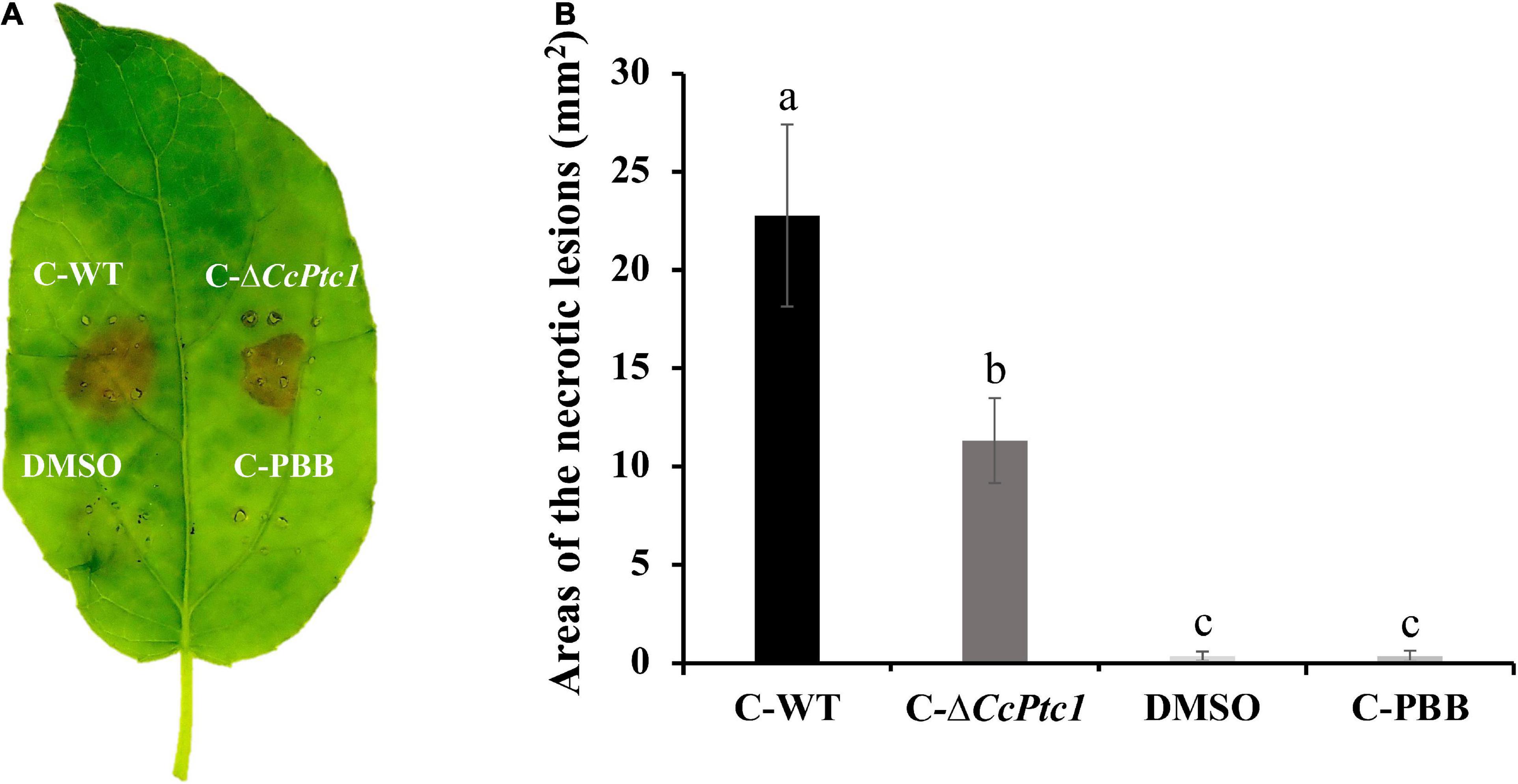
Figure 5. Detection of crude secondary metabolites produced by wild-type (WT; Cytospora chrysosperma) and ΔCcPtc1 in infected poplar tissues. (A) Representative images and measurements of lesions on poplar leaves infected by indicated crude secondary metabolites at 24 h. C-WT, crude secondary metabolites of WT; C-PBB, crude extracts of PBB medium; C-ΔCcPtc1, crude secondary metabolites of ΔCcPtc1. DMSO and crude extracts of PBB were used as controls. (B) Bar graphs show the statistical analysis of at least three biological replicates, means ± SD are shown. The different letters indicates a significant difference (p-value < 0.05) based on one-way ANOVA followed by Duncan’s range test.
These findings verify that C. chrysosperma was likely to produce toxic secondary metabolites which cause damage on host plant tissue, and deletion of CcPtc1 may lead to reduction toxicity of secondary metabolites.
3.6. Metabolomics analysis between the wild type and the CcPtc1 deletion mutant
To clarify the differences in metabolites after the deletion of CcPtc1, six biological individuals for WT or ΔCcPtc1 strain were analyzed by mass spectrometry-based metabolomics, respectively. High Pearson correlation values between the quality control samples were obtained, indicating high data stability during LC-MS/MS analysis (Figure 6A). The markedly separation between WT group and ΔCcPtc1 group was validated by PCA with the first principal component (PC1) value of 29.52% and the second principal component (PC2) accounted for 11.64% of the variation (Figure 6B). The PCA analysis revealed that the metabolites of ΔCcPtc1 and WT strains were significantly different.
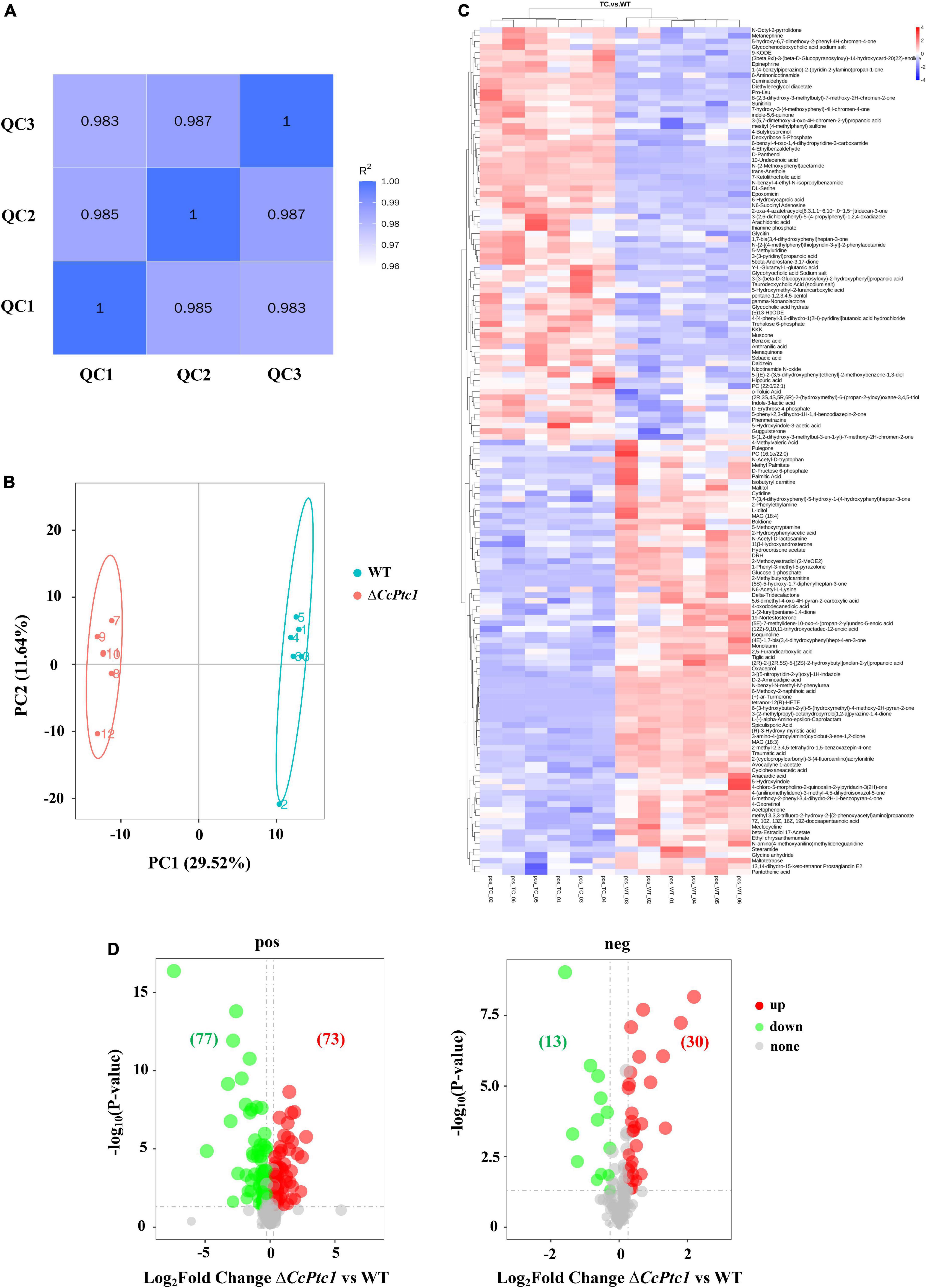
Figure 6. Quantity analyses of metabolomic data between wild-type (WT) group and ΔCcPtc1 group. (A) In positive polarity mode, pearson correlation of three quality control (QC) samples during the LC-MS/MS analysis. (B) In positive polarity mode, score plot (PC1 vs. PC2) of principal component analysis (PCA) of metabolites extracted from ΔCcPtc1 compared to those from WT strain. The green circle indicates WT group, whereas the red circle indicates ΔCcPtc1 group. (C) Heat map of detected metabolites in positive polarity mode. Red and blue colors indicate increase and decrease of metabolite levels relative to the median metabolite level, respectively. (D) The volcano map of metabolites profile in ΔCcPtc1 compared to those in WT strain. The increased metabolites were marked in red dots, whereas the decreased metabolites were marked in green dots. The significantly changed metabolites were defined as Variable Importance in Projection (VIP) > 1, FC (fold change) > 1.2 or p-value < 0.05, and FC < 0.833.
Here, 725 metabolites (data have been de-duplicated) were detected with varied abundance in both strains including 550 metabolites in positive polarity mode (Figure 6C) and 175 metabolites in negative polarity mode (data not shown). Among them, the abundance of 193 metabolites were significantly changed including 90 metabolites significantly decreased their abundance and 103 metabolites significantly increased their abundance (Figure 6D). According to KEGG, HMDB, or LIPID MAPS databases, these 193 DAMs were categorized to the following major classes: biosynthesis of secondary metabolites (14 annotations), nucleosides, nucleotides, and analogs (4 annotations), organic acids and derivatives (14 annotations, 1 annotation was included in biosynthesis of secondary metabolites), carbohydrates and carbohydrate conjugates (10 annotations, 1 annotation was included in biosynthesis of secondary metabolites), and lipids and lipid-like molecules (38 annotations, 4 annotations were included in biosynthesis of secondary metabolites) (Figure 7A and Supplementary Table 3). Importantly, lipid and organic acids were the category that enriched by most of DAMs in response to CcPtc1 deletion. These observations suggest that different abundant metabolites are resulted from CcPtc1 deletion and might be the agents for CcPtc1 to modulate fungal phenotypes.
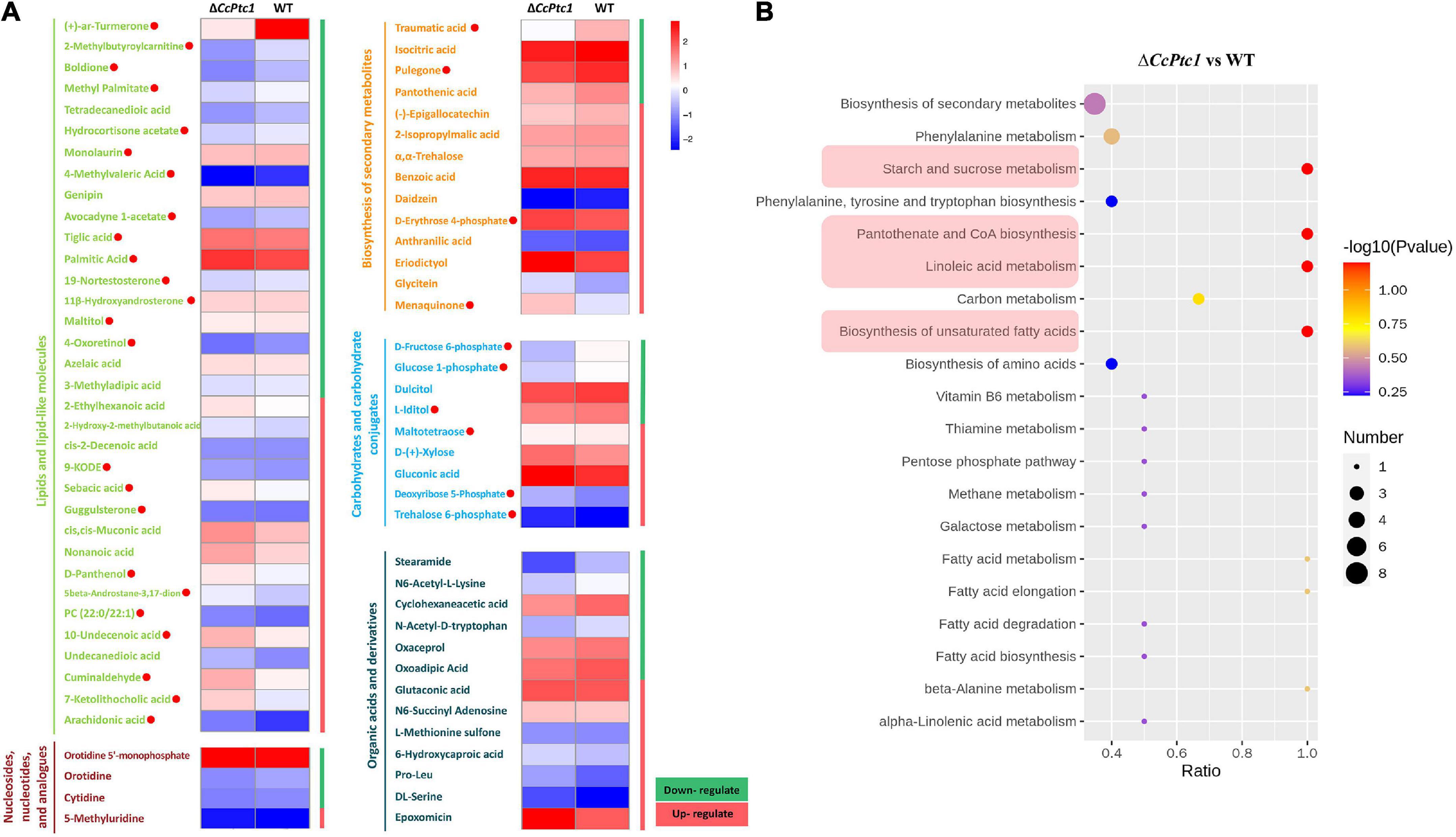
Figure 7. Metabolites enrichment data among wild-type (WT) and ΔCcPtc1. (A) Differential metabolites can be divided into five categories: Lipids and lipid-like molecules, Nucleosides, nucleotides, and analogs, Biosynthesis of secondary metabolites, Carbohydrates and carbohydrate conjugates and Organic acids and derivatives. Red dots represent lipid (27 metabolites, 3 was included in biosynthesis of secondary metabolites) and carbohydrates metabolites (7 metabolites, 1 was included in biosynthesis of secondary metabolites) that were significantly enriched in the positive mode. (B) Metabolic pathway enrichment analyses. Four metabolic pathways with lowest p-value were shadowed in red. The scatterplot showed the enriched KEGG pathway of differentially changed metabolites.
3.7. Variations in metabolic profiles in the absence of CcPtc1
Functionally annotation of the 193 DAMs revealed that four metabolic pathways of the C. chrysosperma were identified including starch and sucrose metabolism, linoleic acid metabolism, pantothenate and coenzyme A (CoA) biosynthesis, and biosynthesis of unsaturated fatty acids (Figure 7B).
The schematic map of significant enrichment metabolic pathways and changes level of key metabolites identified in the mycelia culture filtrates was shown in Figure 8. The key metabolites included trehalose 6-phosphate, D-Fructose 6-phosphate, arachidonic acid, 9-KODE, palmitic acid, pantothenate, and pantothenol were significantly influenced by CcPtc1 (Figure 8). As shown in Figure 8, deletion of CcPtc1 resulted in a decrease in the content of D-Fructose 6-phosphate, pantothenate and palmitic acid, and an increase in the content of trehalose 6-phosphate, 9-KODE, arachidonic acid, and pantothenol.
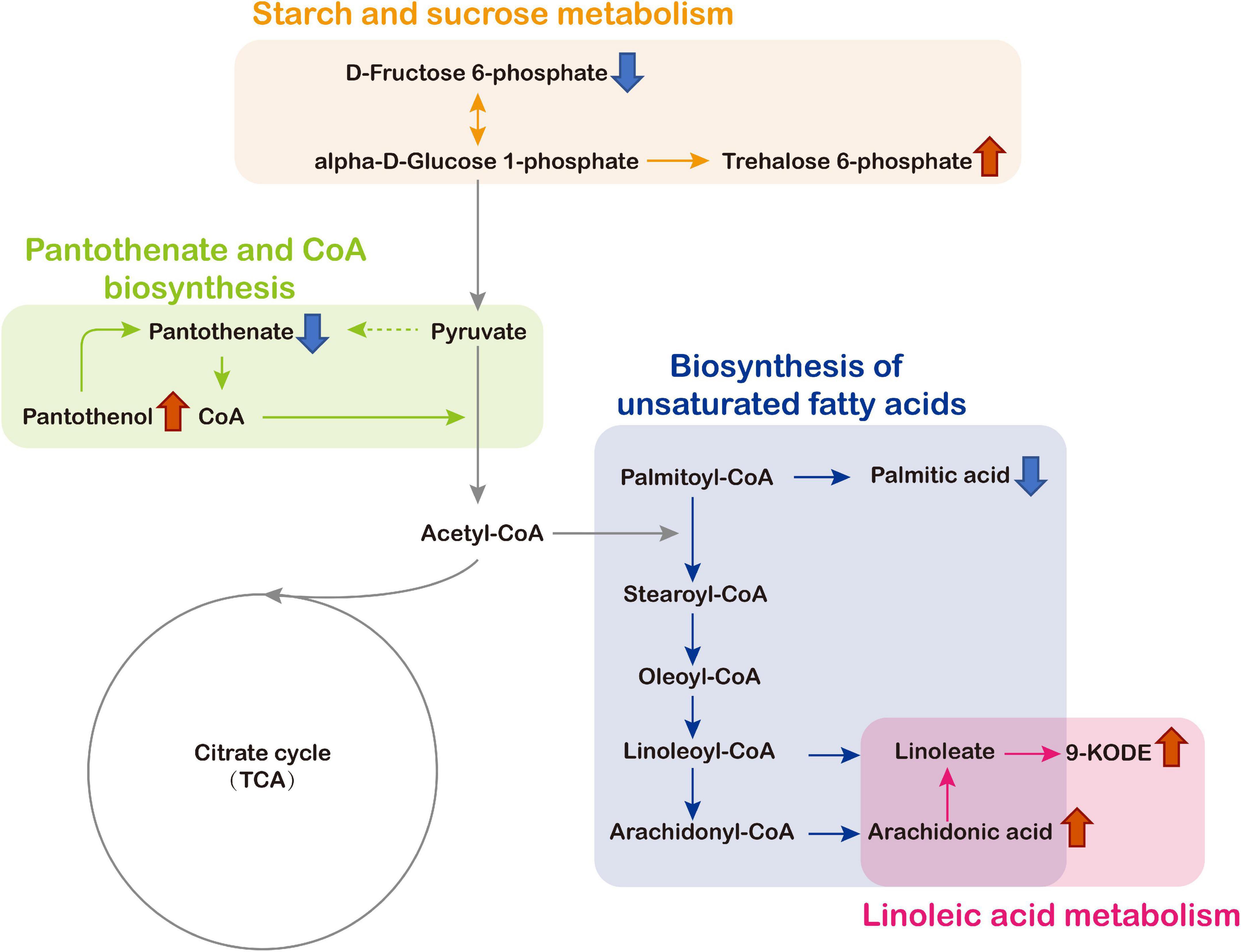
Figure 8. Alterations of metabolite levels in Cytospora chrysosperma induced by deletion of CcPtc1 and schematic biosynthetic pathways map of these metabolites according to the KEGG database. Red and blue arrows denote, respectively, higher and lower levels of metabolites in C. chrysosperma. TCA, tricarboxylic acid.
Moreover, to explore the changes of terpenoids in ΔCcPtc1 mutants, we further analyzed the metabolomic data and identified 17 terpenoid related metabolites (Supplementary Table 4), which could be categorized into four major categories: diterpenoids, monoterpenoids, sesquiterpenoids, and terpene glycosides. Among the 17 terpene metabolites detected, the number of monoterpenoids and sesquiterpenoids was the largest, of which 9 metabolites belonged to monoterpenoids and 5 metabolites belonged to sesquiterpenoids (Figure 9A and Supplementary Table 4). Further analysis revealed that 6 terpenoid related metabolites were significantly alterations in the ΔCcPtc1 compared to the WT, including down-regulated metabolites, (+)-ar-turmerone, pulegone, ethyl chrysanthemumate and genipin, and up-regulated metabolites cuminaldehyde and (±)-abscisic acid (Figure 9A).
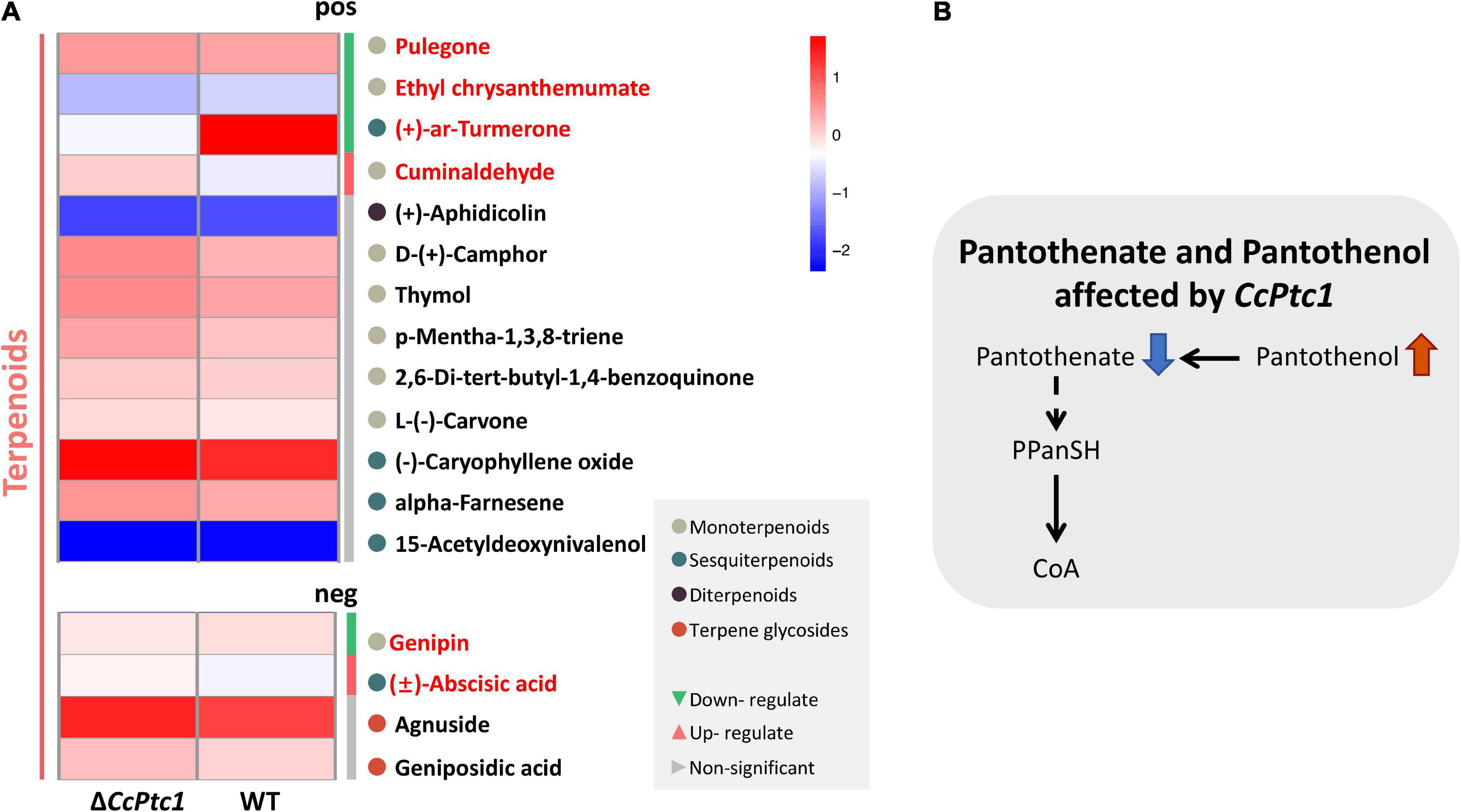
Figure 9. Metabolomic analysis. (A) The heatmap showing the full range of detected terpenoids. The red font indicates significantly enriched terpenoids. (B) A proposed model for the pantothenate and coenzyme A (CoA) mechanism of Cytospora chrysosperma. PPanSH:4′-Phosphopantetheine. The red upward arrows indicate upregulation, and the blue downward arrows indicate downregulation.
3.8. Pantothenate biosynthesis regulated by CcPtc1 in C. chrysosperma
We focused on the biosynthesis of pantothenate and CoA which are significantly enriched in positive polarity mode. Remarkably, we discovered that early intermediates of the pantothenate and CoA biosynthesis pathway (pantothenate and pantothenol) were significant changed in ΔCcPtc1: the amounts of pantothenate was significant down-regulated, and in contrast, the amounts of pantothenol was remarkably up-regulated compared to the WT strain. Furthermore, the ΔCcPtc1 strain showed significant perturbations of pantothenate. Previous studies have showed that pantothenate plays dominant role in regulation of carbohydrate, lipid, and nucleic acid metabolism. As shown in Figure 6 and Supplementary Table 3, the lipid metabolites accounted for the largest number (27 out of 79) (Figure 6 and Supplementary Table 3). Besides, a certain amount of carbohydrates metabolites was also enriched (7/79) (Figure 6 and Supplementary Table 3). Importantly, the lipid (16/27) and carbohydrate (4/7) metabolites were generally reduced in CcPtc1 mutant. It is suggested that deletion of CcPtc1 could reduce the accumulation of pantothenate, which resulted in a significant changed in the content of lipid and carbohydrates metabolites (Figure 9B).
In this study, we found that CcPtc1 markedly affected fungal development, pathogenicity, and toxic secondary metabolites in C. chrysosperma. Taken together, these results shows that CcPtc1 acts as a crucial virulence factor.
4. Discussion
Plant pathogenic fungi are usually capable of secreting multifarious secondary metabolites (also known as natural products), including mycotoxins and secretory proteinaceous toxins, which subvert plant defense responses and contribute to the pathogenicity on plant hosts (Howlett, 2006). We characterized the main functions of the backbone gene CcPtc1 of a terpene type secondary metabolism gene cluster (GME3317_g to GME3324_g), which was required for fungal growth, conidial development, biosynthesis of secondary metabolites in C. chrysosperma. We further performed untargeted metabolomic analysis of the WT and ΔCcPtc1, which revealed that CcPtc1 was involved in the starch and sucrose metabolism, pantothenate and CoA biosynthesis, linoleic acid metabolism and biosynthesis of unsaturated fatty acids. Collectively, the results suggest that CcPtc1 is an important pathogenic factor of C. chrysosperma.
Secondary metabolites secreted by some fungal pathogens often play an important role in triggering these responses (Howlett, 2006). Among them, terpenoids account for a large proportion of secondary metabolites, and the reactions catalyzed by TC are largely responsible for the chemical diversity of terpene natural products (Dickschat, 2016). In this study, we explored the function of a putative terpene cyclase, CcPtc1, and confirmed its ability to regulate pathogenicity, fungal development, and toxic secondary metabolites in C. chrysosperma. Although there are few reports on TC, this gene still plays an important role in fungal biological processes. For example, Fusarium mycotoxin biosynthetic genes discovered to date included a TC gene cluster for trichothecenes (Desjardins and Proctor, 2007). Moreover, in the biocontrol fungus T. virens, the experimental evidence demonstrates that putative terpene cyclase vir4 and vir cluster was involved in the synthesis of volatile terpene compounds (Crutcher et al., 2013). As a consequence, we believe that the study of TC function is a promising direction not only in the study of pathogenic mechanism in C. chrysosperma, but also for filamentous plant fungi. Future investigation of the specific functions of the genes in this cluster will help us understand the assembly line of secondary metabolites synthesized by this cluster and further elucidate the molecular strategies used by C. chrysosperma for successful invasion.
Previous research found secondary metabolic pathways were usually capable of regulating the fungal developmental program not only including hyphae development, but also sexual fruiting-body formation (Bayram et al., 2008; Jiang et al., 2011). Meanwhile, a relationship between mycotoxin production and sporulation in several mycotoxigenic has been demonstrated: In Fusarium verticillioides, knocked out gene FCC1 (for Fusarium cyclin C1) result in reduced production of conidia and fumonisin B1 biosynthesis (Shim and Woloshuk, 2001). Earlier studies suggest that deletion of laeA and veA genes in A. alternata, respectively, strongly reduced sporulation and greatly compromised mycotoxin production during pathogenesis of tomato fruits and in vitro (Estiarte et al., 2016). Consistent with these findings, biosynthesis of toxic secondary metabolites and sporulation in ΔCcPtc1 showed reduced compared with WT strains in C. chrysosperma. Thus, the results suggest that terpene cyclase-like 2 protein CcPtc1 plays an important role in the development of C. chrysosperma. Furthermore, although the effect of secondary metabolic genes on sporulation was not conserved, based on the previous articles and our results, we deduced that there was some connection between fungal toxin output and the sporulation process.
As mentioned earlier, necrotrophic pathogens tend to kill host cells rapidly. To date, several reports have demonstrated that secondary metabolite biosynthetic genes and the toxins they synthesize are the primary weapons of necrotrophic pathogens. For instance, deletion of FgVEA led to inhibition in aerial hyphae formation, virulence and deoxynivalenol (DON) biosynthesis in F. graminearum (Jiang et al., 2011). Another well-known toxin botrydial, produced by botrydial biosynthetic genes of B. cinerea could induce chlorosis and collapse of French bean tissue (Deighton et al., 2001; Siewers et al., 2005). Currently in V. mali, there have been many secondary metabolic genes that play a role in the infection process, such as VmLaeA, VmVeA, and VmVelB (Wu et al., 2018; Feng et al., 2020). In this study, we showed that secondary metabolite backbone gene CcPtc1 markedly affected virulence and toxic secondary metabolites in the necrotrophic fungus C. chrysosperma. In addition, we showed that C. chrysosperma was likely to secrete a series of metabolites to damage the host tissue, and absence of CcPtc1 would affect production of metabolites in C. chrysosperma. Further, combining previous studies with our findings, it is reasonable to speculate that toxins synthesized by secondary metabolic genes play an important role in the infestation process of necrotrophic pathogens.
In this study, untargeted metabolomics analyses revealed that pantothenate and CoA biosynthesis pathway was enriched. In pantothenate and CoA biosynthesis pathway, we identified two metabolites (pantothenate and pantothenol), and pantothenate was significantly down-regulated in ΔCcPtc1 compared with the WT strain. Previous studies have showed that pantothenate (vitamin B5) is not only an essential metabolite for all biological systems, but also the precursor of the indispensable cofactor CoA (Alberts and Vagelos, 1966; Webb et al., 2004; Leonardi et al., 2005). Pantothenate plays dominant role in abundant biological processes, including carbohydrate, lipid, and nucleic acid metabolism. These reports were consistent with our metabolome results (Figure 6). Here, we found that the majority of DAMs were lipid, while a portion was carbohydrates. Therefore, we deduced that the level of pantothenate affects the process of toxin production in C. chrysosperma by influencing the metabolism of lipid and carbohydrates. In addition, we also found that pantothenate and pantothenate biosynthetic pathway shows critical impacts on virulence in Histoplasma capsulatum (Garfoot et al., 2014), A. fumigatus (Dietl et al., 2018) and Mycobacterium tuberculosis (MTB) (Sambandamurthy et al., 2002), and also necessary for the growth of bacterial (Srinivas et al., 2012; He et al., 2018; Yao et al., 2018). These results were identical to the defect of fungal development and pathogenicity in ΔCcPtc1, which suggest that CcPtc1 possibly affected virulence and hyphae development by partial regulating the content of downstream metabolites in C. chrysosperma. Moreover, pantothenol (Dexpanthenol) has been reported to be capable of acting as a substrate for pantothenate kinase in MTB to produce 4′-phosphopantothenol and eventually affects the biosynthesis of CoA (Kumar et al., 2007). Our data indicated that the content of panthenol was significantly up-regulated in ΔCcPtc1 compared with the WT strain. Therefore, we hypothesized that, CcPtc1 might affects the biological reaction using CoA as cofactor by affecting the early intermediates of CoA synthesis, pantothenate and pantothenol (Figure 9B).
In the present study, we also found that another metabolite associated with fungal pathogenicity, trehalose 6-phosphate (T6P), accumulated significantly in the starch and sucrose metabolism pathway pathway. T6P is the key intermediate of the trehalose biosynthesis pathway in plants and yeast (Lunn et al., 2014; Figueroa and Lunn, 2016). Previous studies have determined that the accumulation of T6P has cytotoxic effects in different species (Puttikamonkul et al., 2010; Song et al., 2014; Korte et al., 2016). Additionally, T6P has been verified to have important impacts on the fungal virulence and development of filamentous fungal plant pathogens such as Magnaporthe grisea (Wilson et al., 2007), M. oryzae (Chen et al., 2021), and Stagonospora nodorum (Lowe et al., 2009). In the plant pathogenic fungus M. grisea, T6P synthase (Tps1) is a central regulator for regulating the pentose phosphate pathway and intracellular levels of NADPH, and responsible for fungal virulence (Wilson et al., 2007). Deletion of MoTPS2 result in significantly intracellular accumulation of T6P, which leading to defects in the hyphae development and pathogenicity of the M. oryzae (Chen et al., 2021). Our data demonstrated that T6P was significantly accumulated in the ΔCcPtc1 mutant compared with the WT strain. Correspondingly, the ΔCcPtc1 mutant showed reduced pathogenicity and impaired growth. In C. chrysosperma, whether the accumulation of T6P is related to the defected pathogenicity and the affected hyphal growth of the ΔCcPtc1 needs to be further verified.
As mentioned above, we also enriched some of organic acids and derivatives. Compared to the WT group, a total of 13 differentially abundant organic acids and derivatives metabolites were identified in ΔCcPtc1 group, among which seven upregulated and six downregulated. Fungal pathogens are capable to be classified into two categories, acidic and alkaline fungi by whether fungal pathogens can acidify or alkalize the host’s environment by secreting organic acids or ammonia (Prusky and Lichter, 2008). Organic acids are essential in the invasion and pathogenesis of acidic fungi. Acidic fungi can acidify the host’s environment by secreting organic acids to enhancing their infectivity. Not only that, organic acid molecules secreted by acidic fungi plays a pivotal role in the activation of virulence factors and enhancement of pathogenicity of certain fungi (Jiao et al., 2022). Several studies have shown that organic acids such as citric, oxalic, and gluconic acids are participates in numerous pathogenic processes and important for fungal pathogenicity. Notably, supportive evidence of oxalic acid (OA) acting as a vital virulence factor in C. chrysosperma has been proved. Meanwhile it was substantialized that acidification of host environment was a necessary condition for pathogenic of C. chrysosperma (Wang and Wang, 2020). The metabolomic data provided evidence that CcPtc1 played an influential role to some of the organic acids and derivatives. However, whether the effect of CcPtc1 on the pathogenicity of C. chrysosperma was related to the downstream organic acids and derivatives needs to be further verified.
In summary, CcPtc1 was one of the terpene cyclase-like 2 protein family members identified in C. chrysosperma, which was important for virulence, mycotoxin production and development.
Data availability statement
The datasets presented in this study can be found in online repositories. The names of the repository/repositories and accession number(s) can be found in the article/Supplementary material.
Author contributions
DX, CT, and YY designed the experiments. YY, LY, and XQ performed the experiments and the data analyses. YY prepared the figures and wrote the manuscript. All authors contributed to the article and approved the submitted version.
Funding
This work was supported by the National Key Research and Development Program (2022YFD1401003), the Fundamental Research Funds for the Central Universities (2021ZY15), and the National Natural Science Foundation of China (31800540).
Conflict of interest
The authors declare that the research was conducted in the absence of any commercial or financial relationships that could be construed as a potential conflict of interest.
Publisher’s note
All claims expressed in this article are solely those of the authors and do not necessarily represent those of their affiliated organizations, or those of the publisher, the editors and the reviewers. Any product that may be evaluated in this article, or claim that may be made by its manufacturer, is not guaranteed or endorsed by the publisher.
Supplementary material
The Supplementary Material for this article can be found online at: https://www.frontiersin.org/articles/10.3389/fmicb.2023.1084828/full#supplementary-material
Footnotes
- ^ http://gsds.cbi.pku.edu.cn/
- ^ http://www.ebi.ac.uk/interpro/
- ^ https://www.genome.jp/kegg/pathway.html
- ^ https://hmdb.ca/metabolites
- ^ http://www.lipidmaps.org/
References
Adams, G. C., Roux, J., and Wingfield, M. J. (2006). Cytospora species (Ascomycota, Diaporthales, Valsaceae) introduced and native pathogens of trees in South Africa. Aust. Plant Pathol. 35, 521–548.
Alberts, A. W., and Vagelos, P. R. (1966). Acyl carrier protein. 8. Studies of acyl carrier protein and coenzyme A in Escherichia coli pantothenate or betaalanine auxotrophs. J. Biol. Chem. 241, 5201–5204.
Baker, S. E., Kroken, S., Inderbitzin, P., Asvarak, T., Li, B. Y., Shi, L., et al. (2006). Two polyketide synthase-encoding genes are required for biosynthesis of the polyketide virulence factor, T-toxin, by Cochliobolus heterostrophus. Mol. Plant Microbe Interact. 19, 139–149. doi: 10.1094/MPMI-19-0139
Bayram, O., Krappmann, S., Ni, M., Bok, J. W., Helmstaedt, K., Valerius, O., et al. (2008). VelB/VeA/LaeA complex coordinates light signal with fungal development and secondary metabolism. Science 320, 1504–1506. doi: 10.1126/science.1155888
Biggs, A. R., Davis, D. D., and Merrill, W. (1983). Histopathology of cankers on populus caused by Cytospora chrysosperma. Can. J. Bot. 61, 563–574.
Bok, J. W., and Keller, N. P. (2004). LaeA, a regulator of secondary metabolism in Aspergillus spp. Eukaryot. Cell 3, 527–535. doi: 10.1128/EC.3.2.527-535.2004
Bok, J. W., Hoffmeister, D., Maggio-Hall, L. A., Murillo, R., Glasner, J. D., and Keller, N. P. (2006). Genomic mining for Aspergillus natural products. Chem. Biol. 13, 31–37. doi: 10.1016/j.chembiol.2005.10.008
Brakhage, A. A. (2013). Regulation of fungal secondary metabolism. Nat. Rev. Microbiol. 11, 21–32. doi: 10.1038/nrmicro2916
Brakhage, A. A., and Schroeckh, V. (2011). Fungal secondary metabolites - strategies to activate silent gene clusters. Fungal Genet. Biol. 48, 15–22. doi: 10.1016/j.fgb.2010.04.004
Caceres, I., El Khoury, R., Medina, A., Lippi, Y., Naylies, C., Atoui, A., et al. (2016). Deciphering the anti-aflatoxinogenic properties of eugenol using a large-scale q-PCR approach. Toxins (Basel) 8:123. doi: 10.3390/toxins8050123
Caceres, I., Khoury, A. A., Khoury, R. E., Lorber, S., Oswald, I. P., Khoury, A. E., et al. (2020). Aflatoxin biosynthesis and genetic regulation: A review. Toxins (Basel) 12:150. doi: 10.3390/toxins12030150
Catlett, N. L., Lee, B.-N., Yoder, O. C., and Turgeon, B. G. (2003). Split-marker recombination for efficient targeted deletion of fungal genes. Fungal Genet. Rep. 50, 9–11. doi: 10.4148/1941-4765.1150
Chen, C., Chen, H., Zhang, Y., Thomas, H. R., Frank, M. H., He, Y., et al. (2020). TBtools: An Integrative toolkit developed for interactive analyses of big biological data. Mol. Plant 13, 1194–1202. doi: 10.1016/j.molp.2020.06.009
Chen, X., Abubakar, Y. S., Yang, C., Wang, X., Miao, P., Lin, M., et al. (2021). Trehalose phosphate synthase complex-mediated regulation of trehalose 6-phosphate homeostasis is critical for development and pathogenesis in Magnaporthe oryzae. mSystems 6:e0046221. doi: 10.1128/mSystems.00462-21
Choquer, M., Dekkers, K. L., Chen, H. Q., Cao, L., Ueng, P. P., Daub, M. E., et al. (2005). The CTB1 gene encoding a fungal polyketide synthase is required for cercosporin biosynthesis and fungal virulence of Cercospora nicotianae. Mol. Plant Microbe Interact. 18, 468–476. doi: 10.1094/MPMI-18-0468
Crutcher, F. K., Parich, A., Schuhmacher, R., Mukherjee, P. K., Zeilinger, S., and Kenerley, C. M. (2013). A putative terpene cyclase, vir4, is responsible for the biosynthesis of volatile terpene compounds in the biocontrol fungus Trichoderma virens. Fungal Genet. Biol. 56, 67–77. doi: 10.1016/j.fgb.2013.05.003
Daub, M. E., and Ehrenshaft, M. (2000). The photoactivated cercospora toxin cercosporin: Contributions to plant disease and fundamental biology. Annu. Rev. Phytopathol. 38, 461–490. doi: 10.1146/annurev.phyto.38.1.461
Deighton, N., Muckenschnabel, I., Colmenares, A. J., Collado, I. G., and Williamson, B. (2001). Botrydial is produced in plant tissues infected by Botrytis cinerea. Phytochemistry 57, 689–692. doi: 10.1016/s0031-9422(01)00088-7
Desjardins, A. E., and Proctor, R. H. (2007). Molecular biology of Fusarium mycotoxins. Int. J. Food Microbiol. 119, 47–50. doi: 10.1016/j.ijfoodmicro.2007.07.024
Dickschat, J. S. (2016). Bacterial terpene cyclases. Nat. Prod. Rep. 33, 87–110. doi: 10.1039/c5np00102a
Dietl, A. M., Meir, Z., Shadkchan, Y., Osherov, N., and Haas, H. (2018). Riboflavin and pantothenic acid biosynthesis are crucial for iron homeostasis and virulence in the pathogenic mold Aspergillus fumigatus. Virulence 9, 1036–1049. doi: 10.1080/21505594.2018.1482181
Dorrestein, P. C., and Kelleher, N. L. (2006). Dissecting non-ribosomal and polyketide biosynthetic machineries using electrospray ionization Fourier-Transform mass spectrometry. Nat. Prod. Rep. 23, 893–918. doi: 10.1039/b511400b
Ebert, M. K., Spanner, R. E., de Jonge, R., Smith, D. J., Holthusen, J., Secor, G. A., et al. (2019). Gene cluster conservation identifies melanin and perylenequinone biosynthesis pathways in multiple plant pathogenic fungi. Environ. Microbiol. 21, 913–927. doi: 10.1111/1462-2920.14475
Estiarte, N., Lawrence, C. B., Sanchis, V., Ramos, A. J., and Crespo-Sempere, A. (2016). LaeA and VeA are involved in growth morphology, asexual development, and mycotoxin production in Alternaria alternata. Int. J. Food Microbiol. 238, 153–164. doi: 10.1016/j.ijfoodmicro.2016.09.003
Fan, X. L., Bezerra, J. D. P., Tian, C. M., and Crous, P. W. (2020). Cytospora (Diaporthales) in China. Persoonia 45, 1–45. doi: 10.3767/persoonia.2020.45.01
Feizollahi, E., and Roopesh, M. S. (2022). Mechanisms of deoxynivalenol (DON) degradation during different treatments: A review. Crit. Rev. Food Sci. Nutr. 62, 5903–5924. doi: 10.1080/10408398.2021.1895056
Feng, Y., Yin, Z., Wu, Y., Xu, L., Du, H., Wang, N., et al. (2020). LaeA controls virulence and secondary metabolism in apple canker pathogen Valsa mali. Front. Microbiol. 11:581203. doi: 10.3389/fmicb.2020.581203
Figueroa, C. M., and Lunn, J. E. (2016). A tale of two sugars: Trehalose 6-phosphate and sucrose. Plant Physiol. 172, 7–27. doi: 10.1104/pp.16.00417
Garfoot, A. L., Zemska, O., and Rappleye, C. A. (2014). Histoplasma capsulatum depends on de novo vitamin biosynthesis for intraphagosomal proliferation. Infect. Immun. 82, 393–404. doi: 10.1128/IAI.00824-13
Goswami, R. S. (2012). Targeted gene replacement in fungi using a split-marker approach. Methods Mol. Biol. 835, 255–269. doi: 10.1007/978-1-61779-501-5_16
Han, Z., Xiong, D., Xu, Z., Liu, T., and Tian, C. (2021a). The Cytospora chrysosperma virulence effector CcCAP1 mainly localizes to the plant nucleus to suppress plant immune responses. mSphere 6:e00883-20. doi: 10.1128/msphere.00883-20
Han, Z., Yu, R., Xiong, D., and Tian, C. (2021b). A Sge1 homolog in Cytospora chrysosperma governs conidiation, virulence and the expression of putative effectors. Gene 778:145474. doi: 10.1016/j.gene.2021.145474
He, W., Hu, S., Du, X., Wen, Q., Zhong, X. P., Zhou, X., et al. (2018). Vitamin B5 reduces bacterial growth via regulating innate immunity and adaptive immunity in mice infected with Mycobacterium tuberculosis. Front. Immunol. 9:365. doi: 10.3389/fimmu.2018.00365
Howlett, B. J. (2006). Secondary metabolite toxins and nutrition of plant pathogenic fungi. Curr. Opin. Plant Biol. 9, 371–375. doi: 10.1016/j.pbi.2006.05.004
Jia, L. J., Tang, H. Y., Wang, W. Q., Yuan, T. L., Wei, W. Q., Pang, B., et al. (2019). A linear nonribosomal octapeptide from Fusarium graminearum facilitates cell to-cell invasion of wheat. Nat. Commun. 10:922. doi: 10.1038/s41467-019-08726-9
Jiang, J., Liu, X., Yin, Y., and Ma, Z. (2011). Involvement of a velvet protein FgVeA in the regulation of asexual development, lipid and secondary metabolisms and virulence in Fusarium graminearum. PLoS One 6:e28291. doi: 10.1371/journal.pone.0028291
Jiao, W., Liu, X., Li, Y., Li, B., Du, Y., Zhang, Z., et al. (2022). Organic acid, a virulence factor for pathogenic fungi, causing postharvest decay in fruits. Mol. Plant Pathol. 23, 304–312. doi: 10.1111/mpp.13159
Johnson, R. D., Johnson, L., Itoh, Y., Kodama, M., Otani, H., and Kohmoto, K. (2000). Cloning and characterization of a cyclic peptide synthetase gene from Alternaria alternata apple pathotype whose product is involved in AM-toxin synthesis and pathogenicity. Mol. Plant Microbe Interact. 13, 742–753. doi: 10.1094/mpmi.2000.13.7.742
Keller, N., Bok, J., Chung, D., Perrin, R. M., and Keats Shwab, E. (2006). LaeA, a global regulator of Aspergillus toxins. Med. Mycol. 44(Suppl. 1), S83–S85. doi: 10.1080/13693780600835773
Kepley, J. B., Reeves, F. B., Jacobi, W. R., and Adams, G. C. (2015). Species associated with cytospora canker on Populus tremuloides. Mycotaxon 130, 783–805. doi: 10.5248/130.783
Kim, Y. T., Lee, Y. R., Jin, J., Han, K. H., Kim, H., Kim, J. C., et al. (2005). Two different polyketide synthase genes are required for synthesis of zearalenone in Gibberella zeae. Mol. Microbiol. 58, 1102–1113. doi: 10.1111/j.1365-2958.2005.04884.x
Korte, J., Alber, M., Trujillo, C. M., Syson, K., Koliwer-Brandl, H., Deenen, R., et al. (2016). Trehalose-6-phosphate-mediated toxicity determines essentiality of OtsB2 in Mycobacterium tuberculosis in vitro and in mice. PLoS Pathog. 12:e1006043. doi: 10.1371/journal.ppat.1006043
Kumar, P., Chhibber, M., and Surolia, A. (2007). How pantothenol intervenes in Coenzyme-A biosynthesis of Mycobacterium tuberculosis. Biochem. Biophys. Res. Commun. 361, 903–909. doi: 10.1016/j.bbrc.2007.07.080
Leonardi, R., Zhang, Y. M., Rock, C. O., and Jackowski, S. (2005). Coenzyme A: Back in action. Prog. Lipid Res. 44, 125–153. doi: 10.1016/j.plipres.2005.04.001
Li, H., Wang, D., Zhang, D. D., Geng, Q., Li, J. J., Sheng, R. C., et al. (2022). A polyketide synthase from Verticillium dahliae modulates melanin biosynthesis and hyphal growth to promote virulence. BMC Biol. 20:125. doi: 10.1186/s12915-022-01330-2
Li, X., Xiong, D., and Tian, C. (2021). Genome-wide identification, phylogeny and transcriptional profiling of SNARE genes in Cytospora chrysosperma. J. Phytopathol. 169, 471–485. doi: 10.1111/jph.13003
Lowe, R. G., Lord, M., Rybak, K., Trengove, R. D., Oliver, R. P., and Solomon, P. S. (2009). Trehalose biosynthesis is involved in sporulation of Stagonospora nodorum. Fungal Genet. Biol. 46, 381–389. doi: 10.1016/j.fgb.2009.02.002
Lunn, J. E., Delorge, I., Figueroa, C. M., Van Dijck, P., and Stitt, M. (2014). Trehalose metabolism in plants. Plant J. 79, 544–567. doi: 10.1111/tpj.12509
Myung, K., Zitomer, N. C., Duvall, M., Glenn, A. E., Riley, R. T., and Calvo, A. M. (2012). The conserved global regulator VeA is necessary for symptom production and mycotoxin synthesis in maize seedlings by Fusarium verticillioides. Plant Pathol. 61, 152–160. doi: 10.1111/j.1365-3059.2011.02504.x
Prusky, D., and Lichter, A. (2008). Mechanisms modulating fungal attack in post-harvest pathogen interactions and their control. Eur. J. Plant Pathol. 121, 281–289. doi: 10.1007/s10658-007-9257-y
Puttikamonkul, S., Willger, S. D., Grahl, N., Perfect, J. R., Movahed, N., Bothner, B., et al. (2010). Trehalose 6-phosphate phosphatase is required for cell wall integrity and fungal virulence but not trehalose biosynthesis in the human fungal pathogen Aspergillus fumigatus. Mol. Microbiol. 77, 891–911. doi: 10.1111/j.1365-2958.2010.07254.x
Rawlinson, C., See, P. T., Moolhuijzen, P., Li, H., Moffat, C. S., Chooi, Y. H., et al. (2019). The identification and deletion of the polyketide synthase-nonribosomal peptide synthase gene responsible for the production of the phytotoxic triticone A/B in the wheat fungal pathogen Pyrenophora tritici-repentis. Environ. Microbiol. 21, 4875–4886. doi: 10.1111/1462-2920.14854
Rokas, A., Wisecaver, J. H., and Lind, A. L. (2018). The birth, evolution and death of metabolic gene clusters in fungi. Nat. Rev. Microbiol. 16, 731–744. doi: 10.1038/s41579-018-0075-3
Ruocco, M., Baroncelli, R., Cacciola, S. O., Pane, C., Monti, M. M., Firrao, G., et al. (2018). Polyketide synthases of Diaporthe helianthi and involvement of DhPKS1 in virulence on sunflower. BMC Genom. 19:27. doi: 10.1186/s12864-017-4405-z
Saha, P., Ghosh, S., and Roy-Barman, S. (2020). MoLAEA regulates secondary metabolism in Magnaporthe oryzae. mSphere 5:e00936-19. doi: 10.1128/mSphere.00936-19
Sambandamurthy, V. K., Wang, X., Chen, B., Russell, R. G., Derrick, S., Collins, F. M., et al. (2002). A pantothenate auxotroph of Mycobacterium tuberculosis is highly attenuated and protects mice against tuberculosis. Nat. Med. 8, 1171–1174. doi: 10.1038/nm765
Shim, W. B., and Woloshuk, C. P. (2001). Regulation of fumonisin B(1) biosynthesis and conidiation in Fusarium verticillioides by a cyclin-like (C-type) gene, FCC1. Appl. Environ. Microbiol. 67, 1607–1612. doi: 10.1128/aem.67.4.1607-1612.2001
Siewers, V., Viaud, M., Jimenez-Teja, D., Collado, I. G., Gronover, C. S., Pradier, J. M., et al. (2005). Functional analysis of the cytochrome P450 monooxygenase gene bcbot1 of Botrytis cinerea indicates that botrydial is a strain-specific virulence factor. Mol. Plant Microbe Interact. 18, 602–612. doi: 10.1094/mpmi-18-0602
Song, X. S., Li, H. P., Zhang, J. B., Song, B., Huang, T., Du, X. M., et al. (2014). Trehalose 6-phosphate phosphatase is required for development, virulence and mycotoxin biosynthesis apart from trehalose biosynthesis in Fusarium graminearum. Fungal Genet. Biol. 63, 24–41. doi: 10.1016/j.fgb.2013.11.005
Srinivas, A., Rahul, K., Ramaprasad, E. V. V., Sasikala, C., and Ramana, C. V. (2012). Rhodovulum bhavnagarense sp. nov., a phototrophic alphaproteobacterium isolated from a pink pond. Int. J. Syst. Evol. Microbiol. 62(Pt 10), 2528–2532. doi: 10.1099/ijs.0.036152-0
Wang, C., Li, C., Li, B., Li, G., Dong, X., Wang, G., et al. (2014). Toxins produced by Valsa mali var. mali and their relationship with pathogenicity. Toxins (Basel) 6, 1139–1154. doi: 10.3390/toxins6031139
Wang, Y. L., Lu, Q., Decock, C., Li, Y. X., and Zhang, X. Y. (2015). Cytospora species from Populus and Salix in China with C. davidiana sp. nov. Fungal Biol. 119, 420–432. doi: 10.1016/j.funbio.2015.01.005
Wang, Y., and Wang, Y. (2020). Oxalic acid metabolism contributes to full virulence and pycnidial development in the poplar canker fungus Cytospora chrysosperma. Phytopathology 110, 1319–1325. doi: 10.1094/phyto-10-19-0381-r
Webb, M. E., Smith, A. G., and Abell, C. (2004). Biosynthesis of pantothenate. Nat. Prod. Rep. 21, 695–721. doi: 10.1039/b316419p
Wen, D., Yu, L., Xiong, D., and Tian, C. (2021). Genome-wide identification of bZIP transcription factor genes and functional analyses of two members in Cytospora chrysosperma. J. Fungi (Basel) 8:34. doi: 10.3390/jof8010034
Wilson, R. A., Jenkinson, J. M., Gibson, R. P., Littlechild, J. A., Wang, Z. Y., and Talbot, N. J. (2007). Tps1 regulates the pentose phosphate pathway, nitrogen metabolism and fungal virulence. EMBO J. 26, 3673–3685. doi: 10.1038/sj.emboj.7601795
Wu, D., Oide, S., Zhang, N., Choi, M. Y., and Turgeon, B. G. (2012). ChLae1 and ChVel1 regulate T-toxin production, virulence, oxidative stress response, and development of the maize pathogen Cochliobolus heterostrophus. PLoS Pathog. 8:e1002542. doi: 10.1371/journal.ppat.1002542
Wu, Y., Xu, L., Yin, Z., Dai, Q., Gao, X., Feng, H., et al. (2018). Two members of the velvet family, VmVeA and VmVelB, affect conidiation, virulence and pectinase expression in Valsa mali. Mol. Plant Pathol. 19, 1639–1651. doi: 10.1111/mpp.12645
Xiong, D., Yu, L., Shan, H., and Tian, C. (2021). CcPmk1 is a regulator of pathogenicity in Cytospora chrysosperma and can be used as a potential target for disease control. Mol. Plant Pathol. 22, 710–726. doi: 10.1111/mpp.13059
Yang, G., Cao, X., Ma, G., Qin, L., Wu, Y., Lin, J., et al. (2020). MAPK pathway-related tyrosine phosphatases regulate development, secondary metabolism and pathogenicity in fungus Aspergillus flavus. Environ. Microbiol. 22, 5232–5247. doi: 10.1111/1462-2920.15202
Yao, C., Chou, J., Wang, T., Zhao, H., and Zhang, B. (2018). Pantothenic acid, Vitamin C, and biotin play important roles in the growth of Lactobacillus helveticus. Front. Microbiol. 9:1194. doi: 10.3389/fmicb.2018.01194
Yin, Z., Liu, H., Li, Z., Ke, X., Dou, D., Gao, X., et al. (2015). Genome sequence of Valsa canker pathogens uncovers a potential adaptation of colonization of woody bark. New Phytol. 208, 1202–1216. doi: 10.1111/nph.13544
Yu, L., Wen, D., Yang, Y., Qiu, X., Xiong, D., and Tian, C. (2022a). Comparative transcriptomic analysis of MAPK-mediated regulation of pathogenicity, stress responses and development in Cytospora chrysosperma. Phytopathology. doi: 10.1094/phyto-04-22-0126-r [Epub ahead of print].
Yu, L., Yang, Y., Xiong, D., and Tian, C. (2022b). Phosphoproteomic and metabolomic profiling uncovers the roles of CcPmk1 in the pathogenicity of Cytospora chrysosperma. Microbiol. Spectr. 10:e0017622. doi: 10.1128/spectrum.00176-22
Yu, L., Xiong, D., Han, Z., Liang, Y., and Tian, C. (2019). The mitogen-activated protein kinase gene CcPmk1 is required for fungal growth, cell wall integrity and pathogenicity in Cytospora chrysosperma. Fungal Genet. Biol. 128, 1–13. doi: 10.1016/j.fgb.2019.03.005
Keywords: Cytospora chrysosperma, secondary metabolites, terpene cyclase, pathogenicity, metabonomics
Citation: Yang Y, Yu L, Qiu X, Xiong D and Tian C (2023) A putative terpene cyclase gene (CcPtc1) is required for fungal development and virulence in Cytospora chrysosperma. Front. Microbiol. 14:1084828. doi: 10.3389/fmicb.2023.1084828
Received: 31 October 2022; Accepted: 06 February 2023;
Published: 20 February 2023.
Edited by:
Masamichi Nishiguchi, Ehime University, JapanReviewed by:
Fengyu Du, Qingdao Agricultural University, ChinaDandan Zhang, Chinese Academy of Agricultural Sciences (CAAS), China
Anwar Hussain, Abdul Wali Khan University Mardan, Pakistan
Copyright © 2023 Yang, Yu, Qiu, Xiong and Tian. This is an open-access article distributed under the terms of the Creative Commons Attribution License (CC BY). The use, distribution or reproduction in other forums is permitted, provided the original author(s) and the copyright owner(s) are credited and that the original publication in this journal is cited, in accordance with accepted academic practice. No use, distribution or reproduction is permitted which does not comply with these terms.
*Correspondence: Dianguang Xiong, eGlvbmdkaWFuZ3VhbmdAMTI2LmNvbQ==; Chengming Tian,
Y2hlbmdtdEBiamZ1LmVkdS5jbg==