- 1Department of Marine Biology and Oceanography, Institut de Ciències del Mar (ICM-CSIC), Barcelona, Catalunya, Spain
- 2Centre for Marine Ecosystems Research, School of Science, Edith Cowan University, Joondalup, WA, Australia
- 3International Atomic Energy Agency, City of Monaco, Monaco
Antarctic polynyas are highly productive open water areas surrounded by ice where extensive phytoplankton blooms occur, but little is known about how these surface blooms influence carbon fluxes and prokaryotic communities from deeper waters. By sequencing the 16S rRNA gene, we explored the vertical connectivity of the prokaryotic assemblages associated with particles of three different sizes in two polynyas with different surface productivity, and we linked it to the magnitude of the particle export fluxes measured using thorium-234 (234Th) as particle tracer. Between the sunlit and the mesopelagic layers (700 m depth), we observed compositional changes in the prokaryotic communities associated with the three size-fractions, which were mostly dominated by Flavobacteriia, Alphaproteobacteria, and Gammaproteobacteria. Interestingly, the vertical differences between bacterial communities attached to the largest particles decreased with increasing 234Th export fluxes, indicating a more intense downward transport of surface prokaryotes in the most productive polynya. This was accompanied by a higher proportion of surface prokaryotic taxa detected in deep particle-attached microbial communities in the station with the highest 234Th export flux. Our results support recent studies evidencing links between surface productivity and deep prokaryotic communities and provide the first evidence of sinking particles acting as vectors of microbial diversity to depth in Antarctic polynyas, highlighting the direct influence of particle export in shaping the prokaryotic communities of mesopelagic waters.
1. Introduction
The Southern Ocean (<35°S) is a key player in the global carbon cycle and the regulation of the Earth’s climate, accounting for ~40% of the anthropogenic CO2 oceanic uptake (Gruber et al., 2009 and references therein). Although the Southern Ocean is the largest high-nutrient low-chlorophyll region, highly biologically productive areas are found south of the circumpolar current (del Castillo et al., 2019), particularly on the continental shelf, in coastal zones including polynyas (Sedwick and DiTullio, 1997; Montes-Hugo and Yuan, 2012; Arrigo et al., 2015). Polynyas are ice-free areas created and maintained by katabatic winds and surrounded by consolidated sea ice. In the polynyas, solar radiation reaches the water column and, when it becomes stratified, light together with nutrient inputs from different sources (e.g., Lannuzel et al., 2010; de Jong et al., 2012; Shadwick et al., 2013) triggers significant phytoplankton blooms (Kang et al., 2001; Arrigo et al., 2015; Jena and Pillai, 2020). This enhanced primary productivity can lead to high local carbon export rates mediated by the sinking of organic particles, thus potentially contributing to the ocean uptake of atmospheric CO2 (Hoppema and Anderson, 2007; Miller and DiTullio, 2007; Arrigo et al., 2008). However, the timing, extent, and intensity of phytoplankton blooms and primary production can vary significantly even amongst closely located Antarctic polynyas (Arrigo et al., 2015; Moreau et al., 2019), with potential but poorly known implications for the efficiency of the export flux and for the underlying microbial communities that depend on surface-derived carbon inputs.
The structure of surface phytoplankton communities determines the amount, quality, and sinking rates of particles leaving the surface ocean. Besides, physical processes and complex food web interactions, including remineralization processes driven by bacteria, modulate the export and transfer efficiency of the biological carbon pump (Henson et al., 2019; Wiedmann et al., 2020; Nguyen et al., 2022). In turn, sinking particles have been shown to act as microbial diversity vectors between the surface waters and the deep ocean, delivering particle-attached microorganisms that seem able to colonize deeper waters (Mestre et al., 2018), and explaining why meso- and bathypelagic bacterial communities associated with different size-fractions reflect surface gradients of surface phytoplankton productivity (Ruiz-González et al., 2020). In coastal polar ecosystems, pelagic bacteria have been shown to respond quickly to spring and summertime phytoplankton blooms, although the fraction of primary production consumed by heterotrophic bacteria seems highly variable (Ducklow and Yager, 2007; Kirchman et al., 2009; Luria et al., 2016), which could be one of the reasons behind the inverse relationship between primary production and export efficiency often observed in the Southern Ocean (Maiti et al., 2013; le Moigne et al., 2016; Henson et al., 2019). This suggests that particle export fluxes and the interacting prokaryote assemblages are tightly dependent on each other. However, very few studies have coupled measurements of particle export with particle-attached prokaryotic community composition, especially in Antarctic polynyas.
Sinking particle fluxes in the ocean can be directly measured by using sediment traps, which have some advantages (e.g., temporal coverage in the case of moored sediment traps) but have a limited spatial coverage and are time consuming to deploy/recover. Therefore, indirect methods are essential to increase the number of observations across the ocean. One of the most common indirect methods for quantifying the magnitude of sinking particle fluxes in the ocean is the use of parent-daughter radionuclide pairs. Among them, the 234Th/238U pair is the most extensively used (Ceballos-Romero et al., 2022). 234Th is continuously produced by the decay of 238U, which has a conservative behavior in oxygenated ocean waters, and is highly particle reactive, so it can be used as a particle tracer. Moreover, the relatively short half-life of 234Th (T1/2 = 24.1 d) suits the biologically mediated temporal changes in particle production and export. 238U, on the other hand, has a very long half-life (T1/2 = 4.5 109 y). The difference in half-lives between 234Th and 238U implies that both radioisotopes should be in secular equilibrium (i.e., 234Th/238U activity ratio of 1) in the environment. However, in the presence of marine particles, 234Th sorbs onto them and can be scavenged when the particles sink, thus breaking the secular equilibrium in the water column (i.e., 234Th/238U activity ratio <1). The magnitude of the disequilibrium between both radioisotopes allows estimating the magnitude of the particle export flux, which can be converted to fluxes of particulate organic carbon, trace metals, or pollutants (Gustafsson et al., 1997; Black et al., 2019; Puigcorbé et al., 2020; Tesán Onrubia et al., 2020). Thus, combining 234Th fluxes with vertical variations in the composition of the communities attached to sinking particles can provide a direct way to link surface productivity, export fluxes, and impacts on microbial communities.
Here, we explore whether differences in surface productivity among Antarctic polynyas impact differentially the particle export fluxes and the microbial communities from the underlying mesopelagic waters. To do so, prokaryotic communities associated with particles of three different sizes were sampled in three stations located in two East Antarctic polynyas differing largely in their surface productivity and dominant phytoplankton groups. We examined the variability in the prokaryotic communities associated with free-living (0.2–0.8 μm), small (0.8–53 μm), and large (>53 μm) particles collected along the water column and compared them to the magnitude of the particle export fluxes estimated using thorium-234 (234Th) as particle tracer. We hypothesize that mesopelagic prokaryotic communities will be more similar to those in the surface in the stations with the highest surface productivity and higher particle export fluxes.
2. Materials and methods
2.1. Sampling and analyses of physicochemical and biological parameters of the study area
Sampling was performed on board of the RSV Aurora Australis (AU1602, AA-V02 2016/17) between 8 December 2016 and 21 January 2017, in one station belonging to the Dalton polynya (D02) and two stations (M36 and M48) located within the Mertz polynya (East Antarctica; 67.2–66.8 °S and 119.5–145.8°E; Supplementary Figure S1A). These stations were chosen due to their contrasting biological, chemical, and physical characteristics, extensively described and discussed by Moreau et al. (2019) and Ratnarajah et al. (2022). For comparison with Ratnarajah et al. (2022), D02 refers to St.2; M36 is EM03 and M48 is MG08. Briefly, both polynyas had similar sea surface temperatures ranging majorly between 0.0 and 1.5°C, but the Dalton polynya showed higher sea surface salinity than the Mertz polynya (34.0–34.3 g/kg vs. 32.5–33.5 g/kg, respectively), suggesting that the later could have experienced more sea ice melting than the Dalton polynya (Moreau et al., 2019). The Dalton polynya presented deeper euphotic depths (95 ± 56 m) and mixed layer depths (25 ± 12 m, excluding two stations where the mixed layer was down to 100 m and 154 m) than the Mertz polynya (40 ± 9 m and 13 ± 1 m, respectively) (Ratnarajah et al., 2022). In general, chlorophyll-a (Chl-a) concentrations in the surface were higher in the Dalton polynya compared to the Mertz polynya (max of 15 μg L−1 vs. 8 μg L−1), but Mertz presented a subsurface Chl-a maximum of ~10 μg L−1 located between 20 and 70 m depth that was consistent along the whole polynya, whereas in the Dalton that layer was more variable (Moreau et al., 2019). Both polynyas also presented global differences in nutrient ratios (i.e., Si:N or N:P) suggesting different nutrient sources (i.e., water masses) and different phytoplanktonic communities, something which was also corroborated by microscope analyses (Moreau et al., 2019). During the time of sampling, the dominant phytoplankton groups differed between both polynyas, with Phaeocystis antarctica dominating in the Dalton station and diatoms dominating in the much more productive Mertz stations (Moreau et al., 2019).
The sampling and analyses of physicochemical and environmental parameters were performed as described in Moreau et al. (2019) and Ratnarajah et al. (2022). Temperature and salinity were obtained from the CTD (Rosenberg and Rintoul, 2017) and fluorescence values were obtained with a fluorometer (ECO-AFL/FL 756, Wetlabs, United States) that was installed also on the CTD rosette. Of particular interest for this study is the concentration of Chl-a, particulate organic carbon (POC) and inorganic nutrients. Chl-a concentrations were obtained from ~500 ml of filtered seawater, extracted with acetone and stored at −20°C for 24–48 h in the dark prior to analysis with a Turner Trilogy fluorometer. POC was determined following Knap et al. (1996) and analyzed using a Thermo Finnigan EA 1112 Series Flash Elemental Analyzer. Concentrations of inorganic macronutrients (nitrate, nitrite and silicic acid) were analyzed after the voyage at the CSIRO laboratory (Hobart, Australia) following the methods described in Murphy and Riley (1962), Armstrong et al. (1967), Wood et al. (1967) and Kérouel and Aminot (1997).
2.2. Sampling of free-living and particle-attached prokaryotic communities
Seawater samples were collected using 12 L Niskin bottles attached to a CTD rosette. At each station, 6 depths were sampled covering the entire water column (down to ~50 m above the bottom, see Table 1). After collection, the samples (5–11 L) were filtered onboard at 4°C using a Masterflex peristaltic pump. The filtration was done sequentially through a 53 μm pore-size Nitex screen mesh, followed by a 0.8 μm pore-size polycarbonate membrane filter (Millipore) and finally through a 0.2 μm Sterivex filter, thus obtaining three size-fractions from each sample: >53 μm, 0.8–53 μm and 0.2–0.8 μm (for simplification, hereafter 53 μm, 0.8 μm, and 0.2 μm). After filtration, the filters were stored at −80°C for further analyses at the home laboratory.
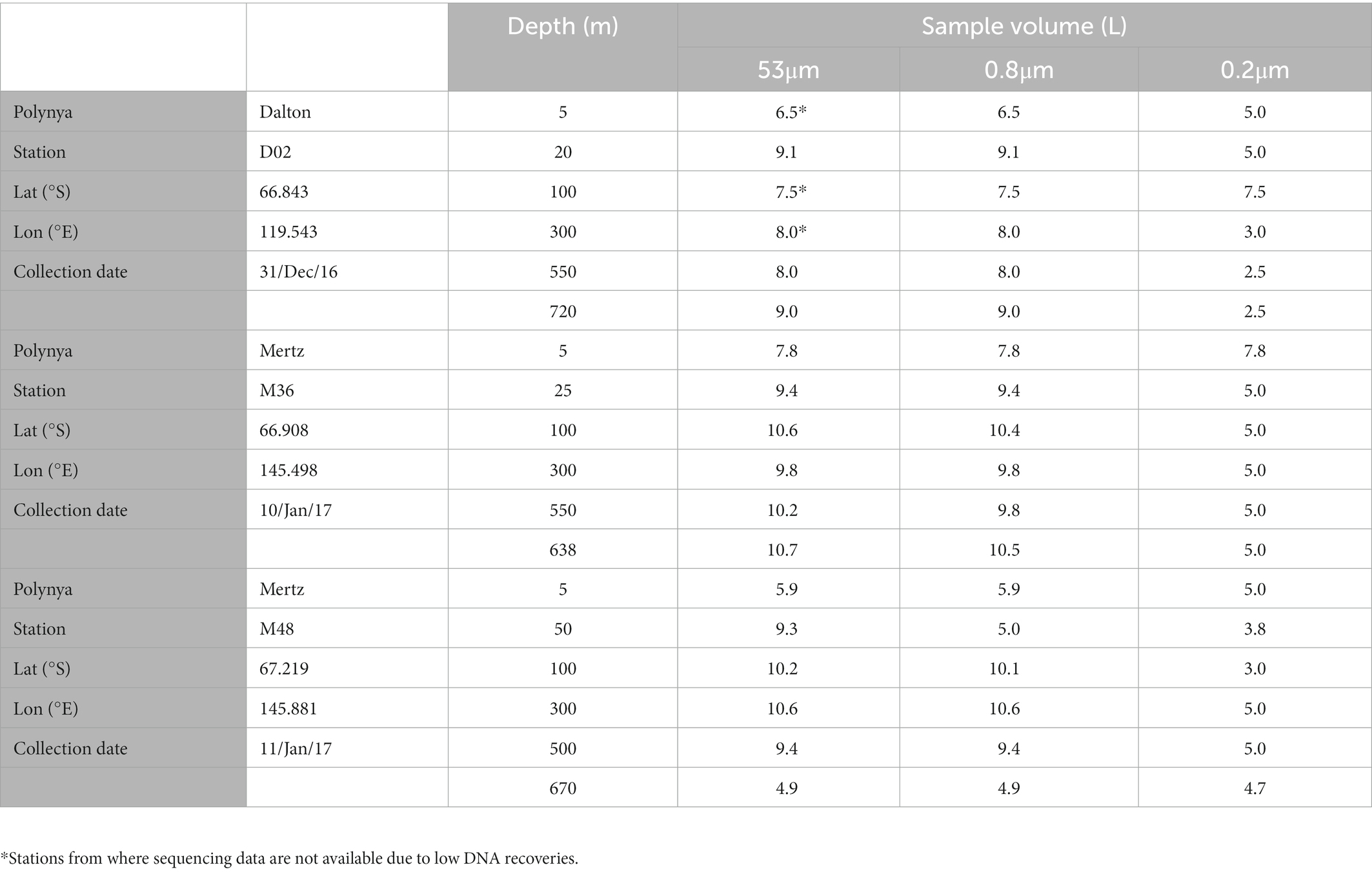
Table 1. Characteristics of the sampled sites—location, date, depth, and filtered volume for DNA samples per each size-fraction.
2.3. Particle export fluxes derived from the 238U-234Th method
234Th analyses are described in Ratnarajah et al. (2022). Briefly, 4 L seawater samples were collected at 12–14 depths along the water column at each station and processed using the manganese oxide co-precipitation technique (Clevenger et al., 2021), while 238U activity concentrations were derived from salinity data (Owens et al., 2011). Th-234 samples were counted onboard using a gas flow proportional low-level RISO beta counter (counting statistics <5%) and recounted >6 months later to account for background activities. Chemical recoveries were obtained following Puigcorbé et al. (2017a) and measured by inductively coupled plasma mass spectrometry at the Alfred Wegener Institute.
234Th export fluxes (proxies of particle fluxes) were estimated by integrating the 234Th deficit in the upper water column relative to 238U, using a 1D scavenging model assuming steady state conditions and no significant advection nor diffusion transport. The integration depth used here is the depth where 234Th and 238U reached secular equilibrium (i.e., 234Th/238U activity ratio = 1) (see Ratnarajah et al. (2022) for further details).
2.4. Characterization of prokaryotic communities
Prokaryotic community structure was determined by high-throughput Illumina sequencing of the 16S rRNA genes. A total of 54 samples were obtained, which were stored at −80°C until analyses were conducted. At the home laboratory, DNA was extracted using the PowerWater DNA Isolation kit following manufacturer’s instructions (MOBIO). The samples were sequenced using Illumina MiSeq 2 × 300 bp flow cells at RTL Genomics (Texas, United States) using primers 515F-Y and 926R (Parada et al., 2015) to amplify the V4-V5 region of the 16S rRNA gene. Three of the 53 μm samples from the Dalton polynya did not have enough DNA material and could not be sequenced (see Table 1). The sequences were processed according to Logares (2017). In brief, primers were removed with Cutadapt (Martin, 2011). The paired-end reads were merged with PEAR (Zhang et al., 2014). Quality filtering, chimera checking and operational taxonomic unit (OTU) clustering (99% similarity) were done with the UPARSE pipeline (Edgar, 2013). Singletons and chimeric OTUs were removed, and the remaining OTUs were taxonomically annotated using the SILVA v123 database. OTUs assigned to chloroplasts were removed, resulting in a total of 8,219 OTUs and 402,440 sequences. To enable comparisons between samples, the OTU table was randomly subsampled to ensure an equal number of sequences per sample (5,000 sequences) using rrarefy (Vegan package, R Core Team, 2017), retaining 253,545 sequences clustered into 6,546 OTUs. The raw sequence data have been deposited in the Figshare data repository, doi: 10.6084/m9.figshare.21385215.v1.
2.5. Statistical analyses
The spatial differences between prokaryotic communities were visualized using nonmetric multidimensional scaling (NMDS, Vegan metaMDS function) based on Bray–Curtis distances. Significant differences in taxonomic composition between depths, stations, or size-fraction were tested using ANOSIM (Vegan anosim function). Vertical differences between the prokaryotic communities within each size-fraction were estimated for each individual station as the Bray–Curtis dissimilarity between the surface (5 m) and each of the deeper prokaryotic communities, and the proportion of “surface-derived” OTUs in mesopelagic communities was estimated considering those mesopelagic OTUs that showed presence in surface (<100 m) waters. OTUs unique to a given sampling station (i.e., OTUs present exclusively in one of the three sampled stations) were identified considering all depths together within each station. Statistical analyses and data handling were done in R (R Core Team, 2017).
3. Results
3.1. Overview of the physicochemical and biological conditions
The two polynyas differed largely in their physicochemical conditions and surface productivity, which have been described in more detail in Moreau et al. (2019) and Ratnarajah et al. (2022) and are summarized in section 2.1. In the three sampled stations, we observed a much larger fluorescence peak (proxy of phytoplankton concentrations) at the two Mertz stations (M36 and M48), compared to the Dalton station (D02), coincident with peaks in ammonia (usually produced in the euphotic zone by heterotrophic bacteria and zooplankton grazing; Smith et al., 2022, and references therein) which were also clearly higher in the Mertz stations than in the Dalton one. Surface concentrations of H4SiO4, PO4−3, and NO3 were much lower in the two Mertz stations than in Dalton surface waters, which was suggested to be due to the higher consumption by phytoplankton, particularly diatoms (Moreau et al., 2019; Ratnarajah et al., 2022) (Supplementary Figure S1).
The Chl-a stocks (in the upper 20 m) were much lower in Dalton (51 mg Chl-a m−2) than in the M36 and M48 Mertz stations (243 mg Chl-a m−2 and 300 mg Chl-a m−2, respectively, Figure 1). Accordingly, POC stocks were also lowest at D02 (3.6 mg C m−2) compared to M36 (8.0 mg C m−2) and M48 (10.2 mg C m−2). 234Th export fluxes (indicative of sinking particle fluxes), as expected, followed the same trend of Chl-a and POC stocks and ranged from 167 dpm m−2 d−1 in D02, to 1,122 dpm m−2 d−1 and 1977 dpm m−2 d−1 at M36 and M48, respectively (Figure 1).
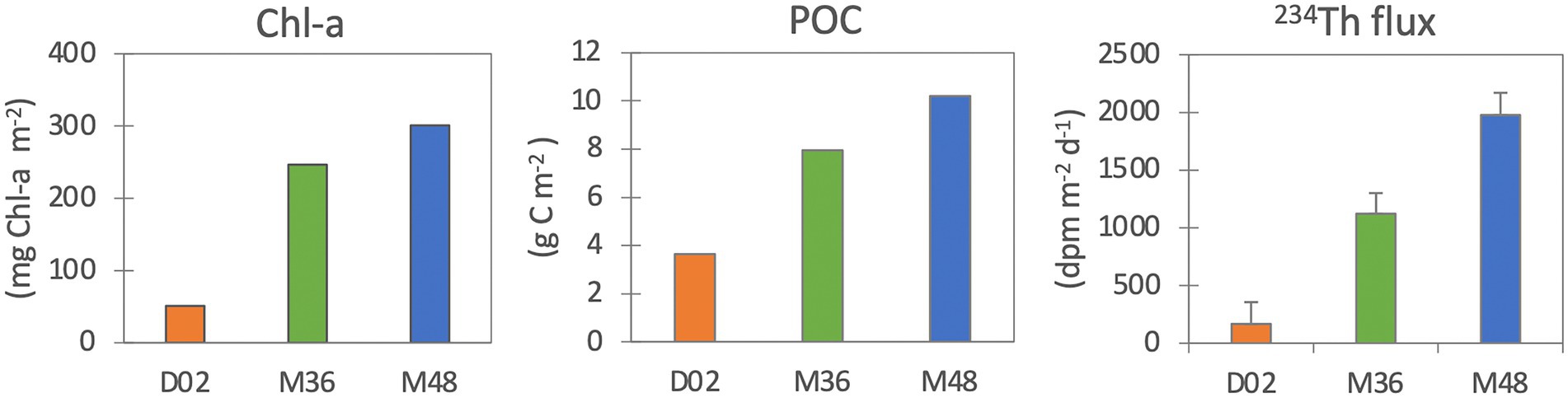
Figure 1. Chlorophyll-a (mg Chl-a m−2), particulate organic carbon (mg C m−2) stocks in the upper water column (upper 20 m) and 234Th export fluxes (dpm m−2 d−1).
3.2. Vertical variations in taxonomic richness and composition of prokaryotic communities
The number of observed OTUs ranged between 130 and 600 across the sampled sites and depths, being lower in communities from the largest particles than in the two other size-fractions (Figure 2). In all studied communities, there was an increase in richness from the most superficial sample to the depth of maximum Chl-a, which was most pronounced in the two smallest size-fractions from M36. In stations D02 and M48, the taxonomic richness decreased from subsurface to deeper waters in the three size-fractions, although this reduction in the number of OTUs was more pronounced in station M48. The richness of the communities from station M36 remained relatively constant throughout the water column below 100 m depth, except for an increase in richness at the deepest site in the two smallest size-fractions (Figure 2). It is also worth noticing that at station M48, which was the station with the highest export flux, the richness of communities from the large particles was much more similar to the richness of the smaller size-fractions, whereas at stations D02 and M36, the large fractions showed markedly lower number of OTUs along the entire water column.
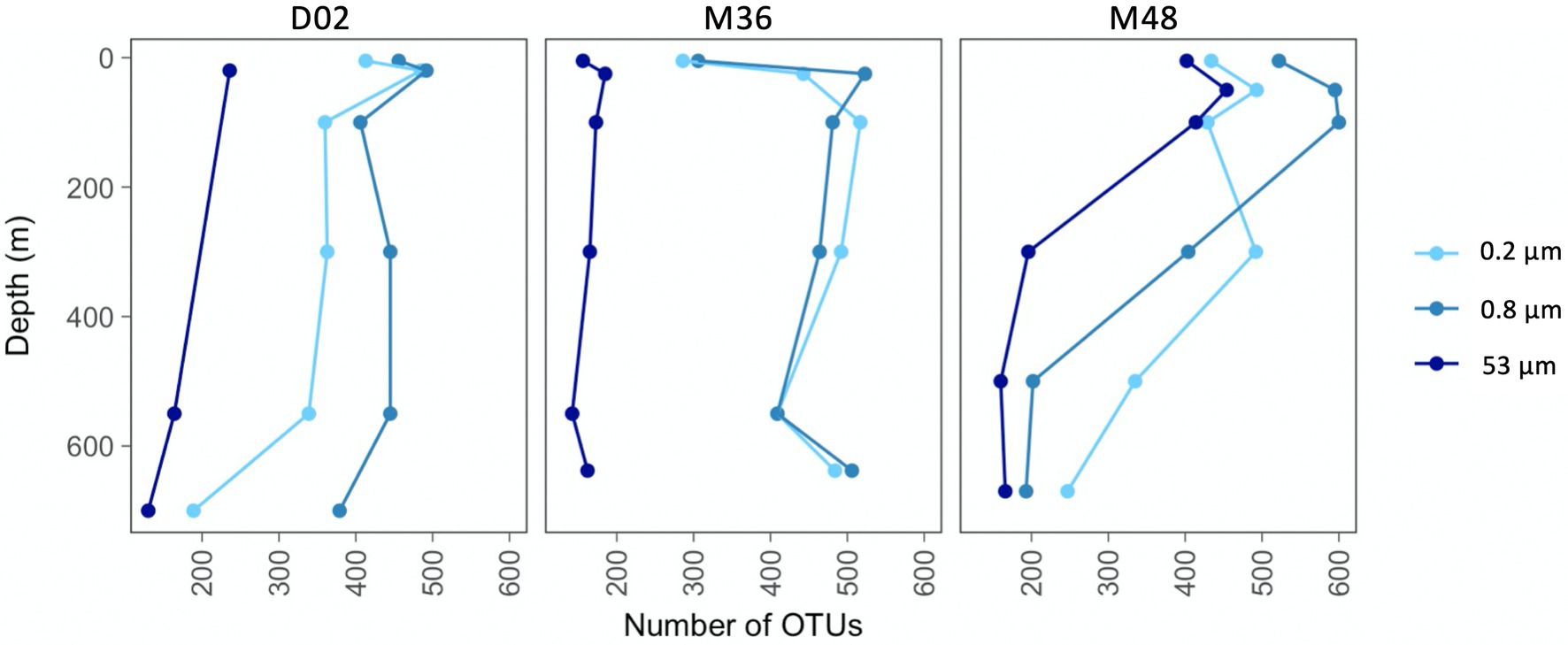
Figure 2. Vertical variations in taxonomic richness (number of OTUs per community) across stations and size-fractions.
Overall, the communities were dominated by class Gammaproteobacteria (53% of total reads), followed by Alphaproteobacteria (26%), Flavobacteriia (17%), and the Thaumarchaeota Marine Group I (1.5%). Less than 2% of the sequences were classified as Archaea and < 0.05% as Eukaryotes. Communities from the two smallest size-fractions were generally dominated by Gammaproteobacteria and/or Flavobacteriia across the three stations, whereas Alphaproteobacteria accounted for the majority of the sequences in most assemblages associated with the largest particles (Figure 3).
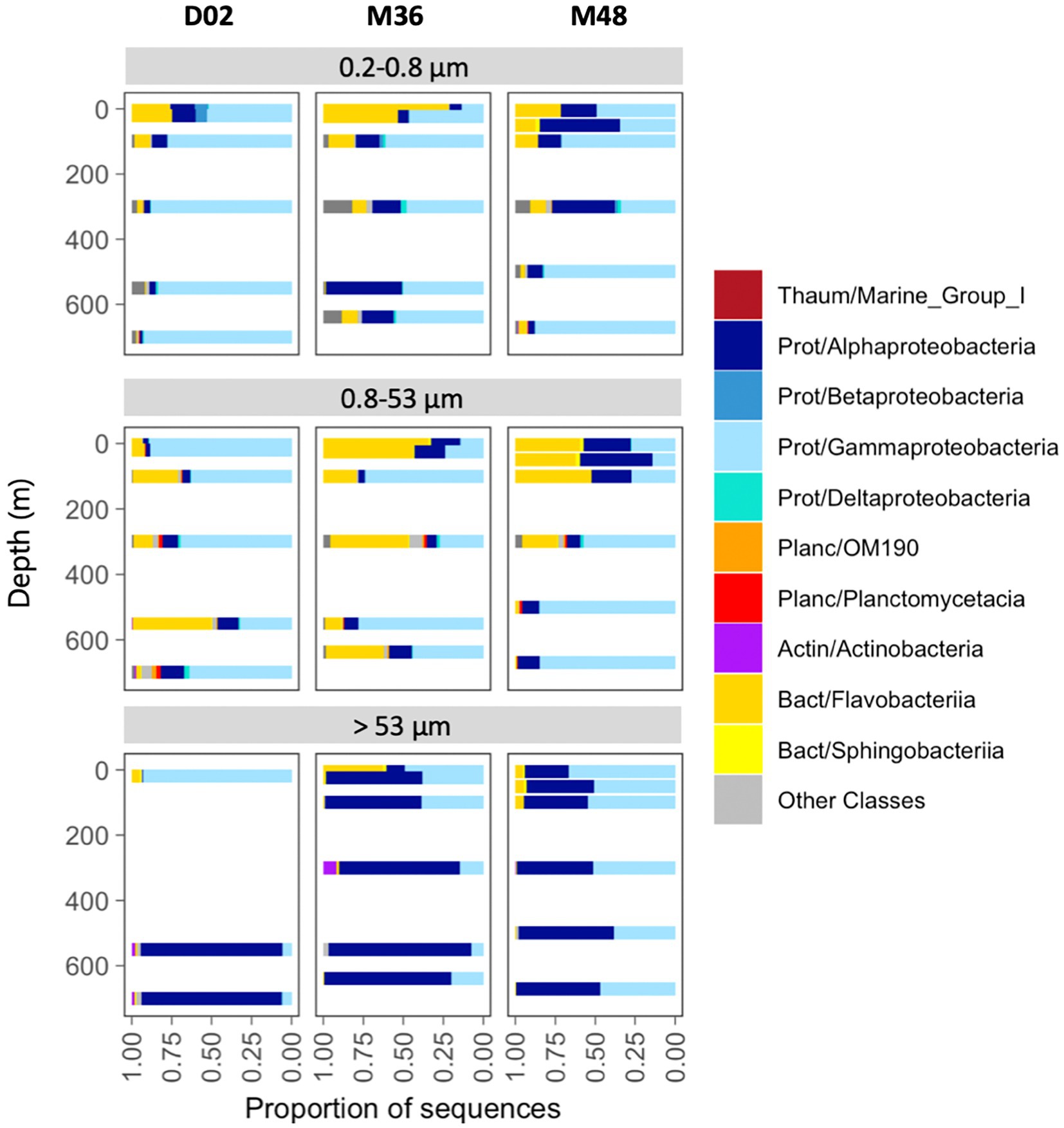
Figure 3. Taxonomic composition across size-fractions and depth in the three stations. The classification was performed at the class level, indicating the phylum: Thaum, thaumarchaeota; Actin, actinobacteria; Bact, bacteroidetes; Planc, planctomycetes; Prot, proteobacteria.
The relative contribution of these groups also changed vertically. In the free-living size-fraction (0.2 μm−0.8 μm), Flavobacteriia decreased their abundances from the surface to mesopelagic waters, where communities comprised mostly Gammaproteobacteria. Alphaproteobacteria decreased with depth in stations D02 and M48 but increased in M36. Particles of intermediate size were dominated mostly by Gammaproteobacteria and Flavobacteriia, but their vertical patterns differed across stations. Finally, communities associated with the largest particles showed much higher proportions of Alphaproteobacteria, which increased their abundances toward mesopelagic waters in the three stations. The largest vertical variations were observed in D02, where communities changed from a dominance of Gammaproteobacteria (95% of the sequences) in the surface toward mesopelagic assemblages comprising mostly Alphaproteobacteria (~94% of community sequences, Figure 3). Classes like Deltaproteobacteria, OM190, Planctomycetacia, Actinobacteria, and Sphingobacteriia were also detected locally but at much lower abundances (Figure 3).
At the genera level, the communities were more variable across stations and size-fractions (Supplementary Figure S2). Some groups like Colwellia, Pseudoalteromonas, Balneatrix (Gammaproteobacteria), and Polaribacter (Flavobacteriia) were relatively common and present in many of the studied communities, although at varying proportions with depth and size-fraction. Other groups, such as the Alphaproteobacteria, Brevundimonas or Sphingorhabdus, were present only in specific samples, such as in the mesopelagic large particles of D02, where together they accounted for most of the community sequences. Conversely, Pseudophaeobacter (Alphaproteobacteria) and Alcanivorax (Gammaproteobacteria) were mostly found associated with the large particles in the two Mertz stations along most of the sampled depths (Supplementary Figure S2).
All studied communities harbored a relatively large fraction of OTUs that were exclusive from a given sampling station (i.e., unique OTUs, range 14–69% of community OTUs, Figure 4A), although these accounted for a smaller fraction of local community sequences (range 1–13%) except for two communities from M48 where unique OTUs comprised 39 and 76% of local sequences (Figure 4B). In stations D02 and M36, the highest percentage of unique OTUs was found in some of the communities associated with the largest particles, whereas in M48, a similar contribution of unique OTUs was found across size-fractions (Figure 4A). These unique OTUs belonged to different orders within Gammaproteobacteria (mostly Alteromonadales, Cellvibrionales, and Oceanospirillales), Alphaproteobacteria (Sphingomonadales and Rhodobacterales) and Flavobacteriia across most size-fractions, except for a large contribution of Actinobacteria unique OTUs in the large particles from station M36 (Figure 4C).
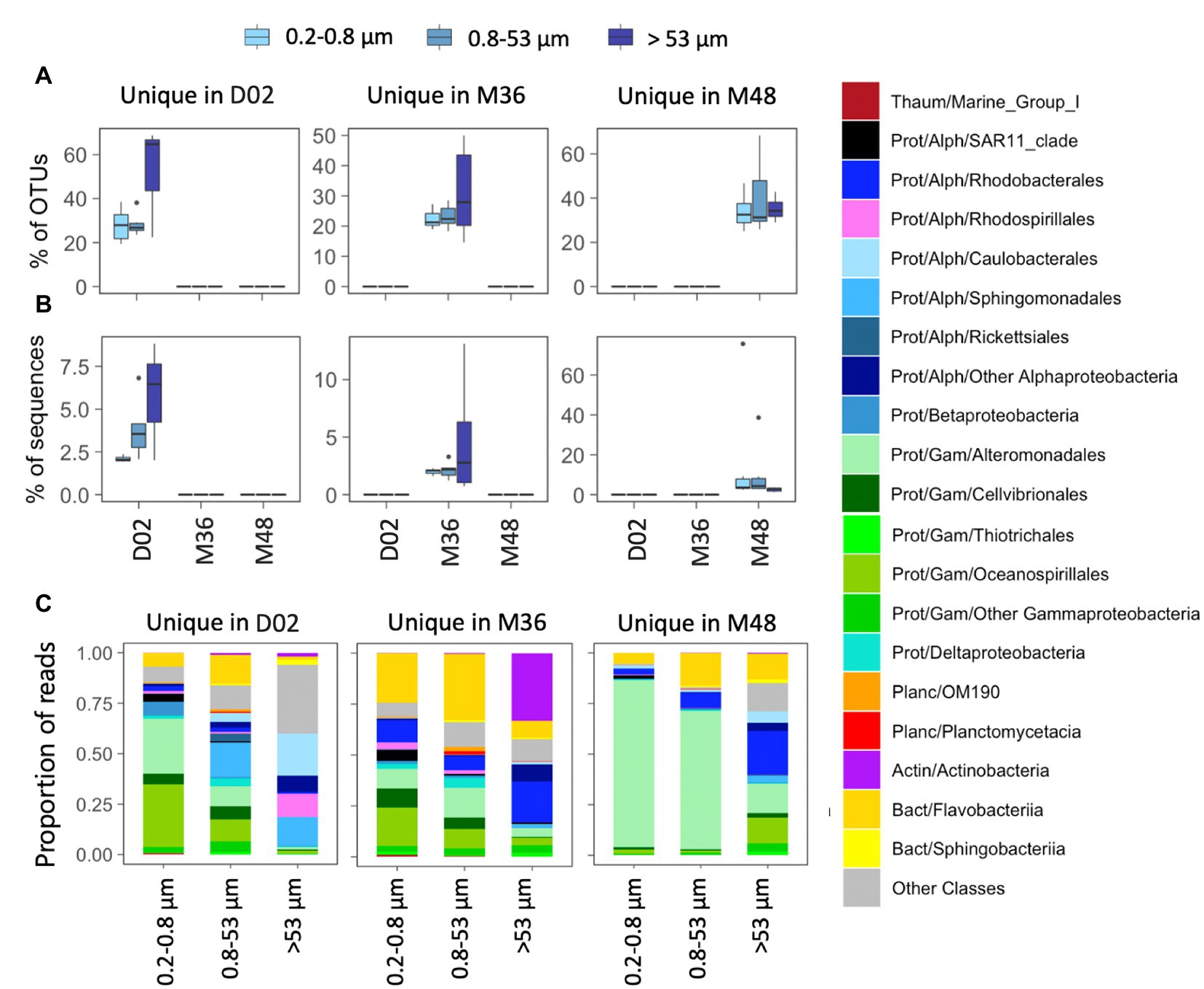
Figure 4. (A,B) Contribution in terms of % OTUs (A) or sequences (B) of those OTUs detected exclusively in one station (“unique” OTUs). (C) Taxonomic composition of unique OTUs in the three size-fractions. The classification was performed at the class level although in some cases the main orders are also indicated. The corresponding phyla and classes are indicated in each case: Thaum, thaumarchaeota; Actin, actinobacteria; Bact, bacteroidetes; Planc, planctomycetes; Prot, proteobacteria; Alph-, Alphaproteobacteria and Gam-, Gammaproteobacteria. Note the different scales of the Y axes.
A non-metric multidimensional scaling (NMDS) analysis showed that, when pooling all samples together, communities from the largest particles differed from assemblages associated with the other two size-fractions, which were more similar to each other. These differences between size-fractions (Figures 5A,B, ANOSIMbysize R = 0.46, p < 0.001) were more important than the spatial differences among stations (Figure 5A, ANOSIMbystation R = 0.14, p < 0.001) and the vertical differences throughout the water column (Figure 5B, ANOSIMbydepth R = 0.18, p < 0.005).
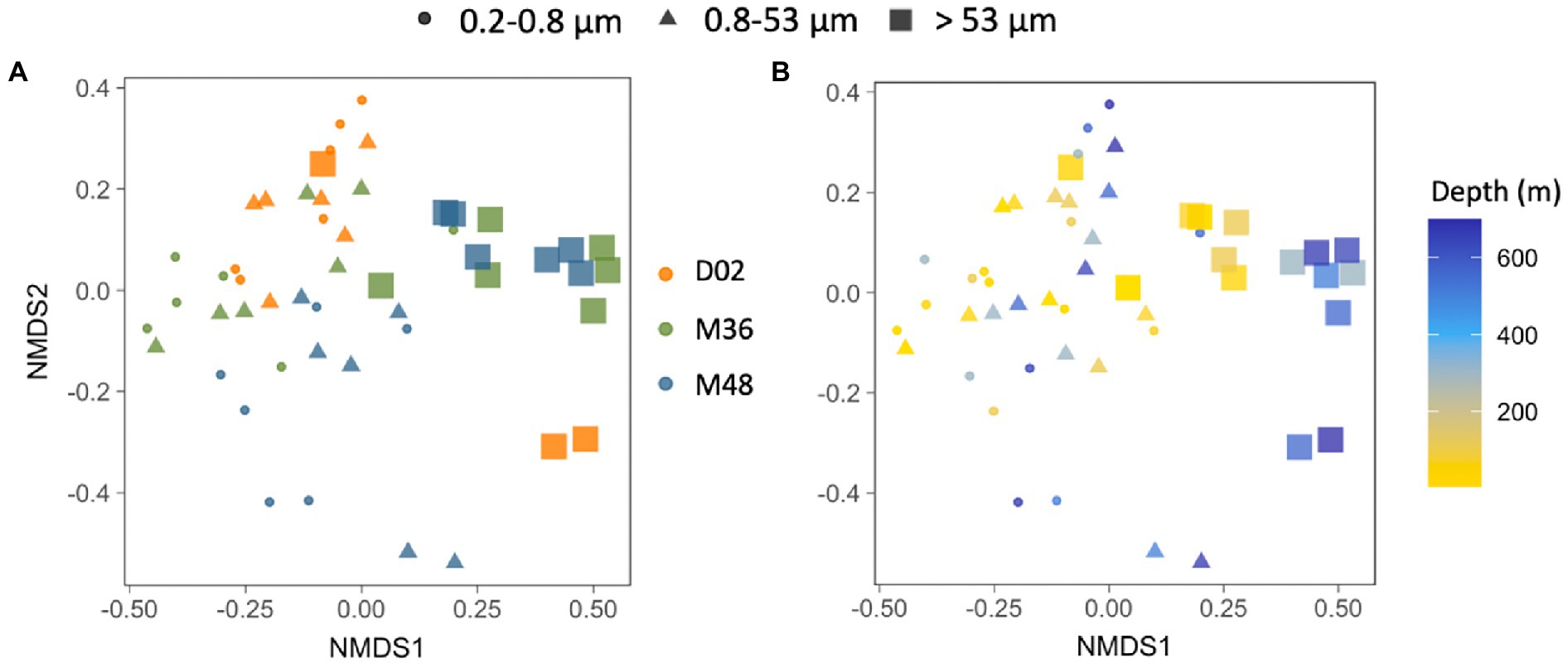
Figure 5. (A,B) Non-multidimensional scaling analysis (NMDS) based on the Bray–Curtis dissimilarity between all the studied prokaryotic communities, color-coded by sampling station (A) or by sampling depth (B) and indicating the different size-fractions. Stress value = 0.18.
To determine whether the particle flux explained the vertical differences within each size-fraction, we estimated the Bray–Curtis dissimilarity between the surface (5 m) and each of the deeper prokaryotic communities and explored how these vertical differences varied along environmental gradients related to surface productivity (using Chl-a and POC as proxies) and particle flux (Figure 6). For the two smaller size-fractions, we found that, as expected, the vertical dissimilarity with the surface communities increased with depth, but no clear patterns were found along the productivity gradients (Figure 6). However, we found that the vertical differences between surface and all deeper prokaryotic communities associated with the largest particles decreased along a gradient of Chl-a and POC stocks, and with increasing 234Th export fluxes, and this happened between all depths, suggesting that communities from the large size-fraction were more similar along the water column in situations of higher surface productivity and 234Th export fluxes.
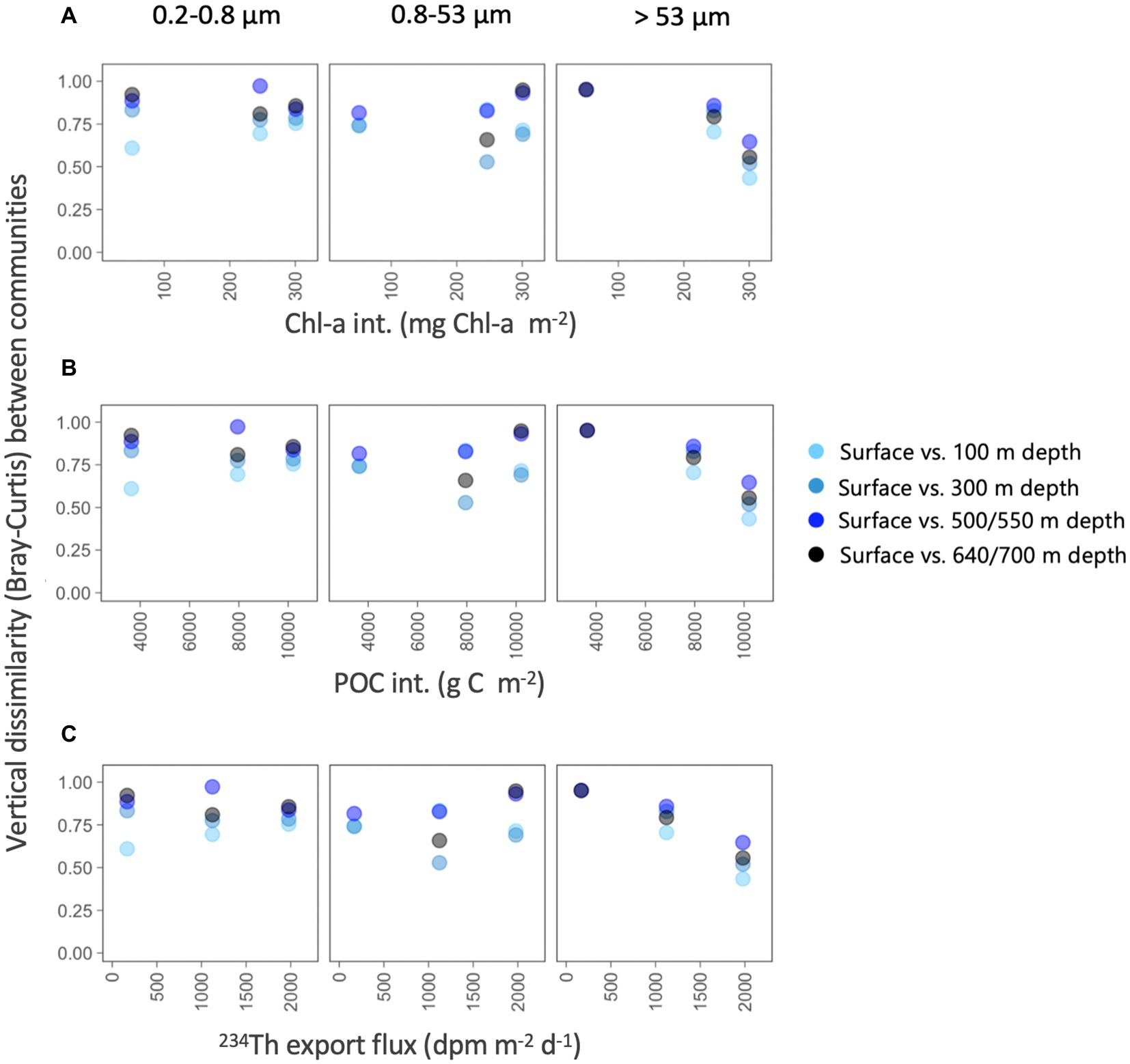
Figure 6. Variation in vertical taxonomic differences (Bray–Curtis dissimilarity) between surface and each of the deeper prokaryotic communities (100–700 m depth), along gradients in depth-integrated chlorophyll-a concentration, depth-integrated POC concentration and estimated 234Th export fluxes for each of the three size-fractions.
Finally, we explored whether the contribution of surface-derived OTUs (i.e., OTUs with presence in any of the surface waters studied, i.e., ≤100 m) to the communities of the mesopelagic samples changed along these surface gradients related to productivity and particle fluxes (Figure 7). We found that mesopelagic communities in the >53 μm particles had a larger proportion of surface-derived OTUs along the increasing 234Th flux gradient, ranging from 13 to 63% of the mesopelagic OTUs in this size-fraction (Figure 7A), accounting for 83 to 99% of the local sequences (Figure 7B). In other words, the higher the particle export flux, the higher the proportion of surface-derived taxa present in the large mesopelagic particles. This tendency was not observed for the other two size-fractions, although in general, we found that all mesopelagic communities harbored a large fraction of OTUs (range 28–52%) and of sequences (range 88–97%) which had first been detected in the overlying surface waters (Figure 7).
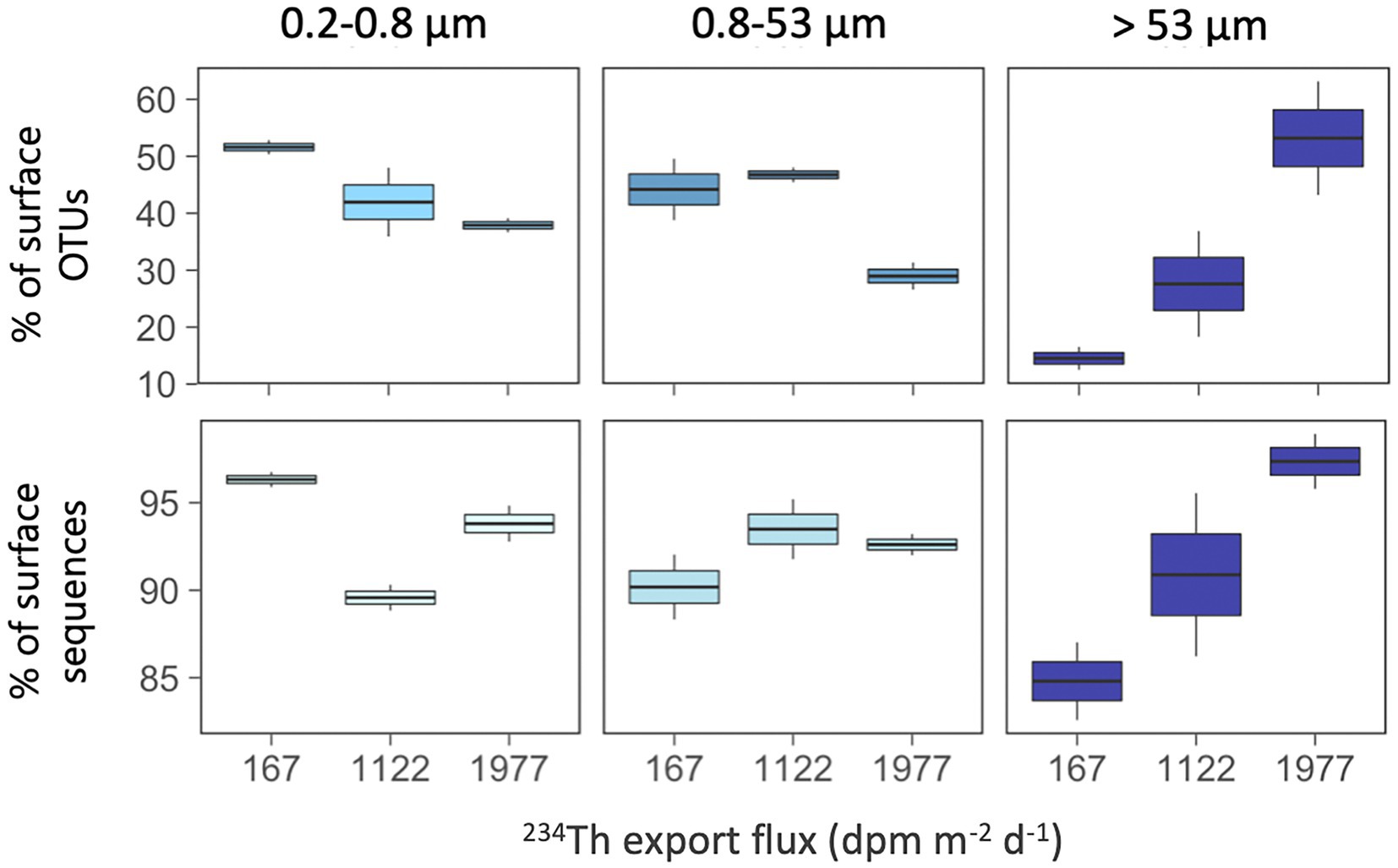
Figure 7. Contribution of surface-derived OTUs (top panels) and their sequences (bottom panels) in mesopelagic communities (≥500 m) along the gradient of 234Th export fluxes. Surface-derived OTUs are those mesopelagic OTUs that were also present in surface waters (≤100 m). Note the different scales of the Y axes.
4. Discussion
The seasonally ice-covered coastal regions of Antarctica host a disproportionately large fraction of its primary production relative to their surface area and support complex food webs with a diversity of upper trophic levels (Arrigo and van Dijken, 2003; Karnovsky et al., 2007), yet these marginal seas and polynyas are heavily understudied. Modeling efforts suggest that about 30% of the Southern Ocean primary production is exported below the euphotic zone (Henson et al., 2012), but high variability has been reported at a smaller spatial scale. For example, in the Amundsen Sea Polynya, the reported most productive coastal Antarctic polynya, bacterial respiration remineralized >95% of the surface-derived particulate organic carbon within the upper 400 m, leading to very low export efficiencies and minimal carbon sequestration (Ducklow et al., 2015; Lee et al., 2017). Particles sinking from the surface ocean undergo remineralization by microbial communities colonizing them, leading to an attenuation of the flux of organic matter toward the deep ocean (Buesseler and Boyd, 2009). This suggests that different particle-attached microbial communities may sway the efficiency of the biological carbon pump in these productive systems, but very few studies have characterized prokaryotic communities associated with particles from Antarctic polynyas. Here, by coupling particle export fluxes with vertical variations in prokaryotic communities associated with different particle size-fractions, we show a large influence of surface productivity on the structure of mesopelagic particle-attached communities, suggesting that expected changes in Antarctic productivity due to global change may have large impacts on carbon sequestration and deep-sea microbiota.
Polynyas, despite sharing similarities in size or location, can be biogeochemically very different. These differences result from a myriad of physicochemical processes related to, among others, mixing, ice melting, ocean currents, interaction with the continental shelf, etc., and can lead to local differences in the timing, magnitude, and extension of phytoplankton blooms in these ecosystems even in closely located sites. Actually, despite their relative spatial proximity, the two polynyas sampled for this study were markedly different in terms of physicochemical and biological characteristics. As discussed in Moreau et al. (2019), the lower phytoplankton biomass and net community production in the Dalton Polynya was a consequence of the different water masses present in both polynyas, with warm modified Circumpolar Deep Water (rich in iron, an essential micronutrient that limits the growth of phytoplankton in the Southern Ocean) being widespread down to the ocean floor at Dalton, whereas it was found at shallower depths in the Mertz. Nutrients measured in both polynyas also indicated differences in the phytoplanktonic community between them, as the depletion of silica in surface waters of the Mertz Polynya coincided with the dominance of the diatoms Fragilariopsis curta and F. cylindrus, whereas small flagellates (mainly Phaeocystis antarctica) dominated the community in the Dalton Polynya (Moreau et al., 2019). Within the Mertz Polynya, station M36 presented clearly warmer and saltier surface waters compared to M48 and had a slightly smaller fluorescence peak that was concentrated in the upper 20 m vs. a fluorescence peak that extended down to 100 m at station M48 (Supplementary Figure S1B). M36 also had lower Chl-a and POC concentrations and stocks as well as lower 234Th export flux, thus creating a gradient of surface “productivity” and particle export across the three stations (D02 < M36 < M48).
4.1. Prokaryotic communities in the Dalton and Mertz polynyas
We characterized the prokaryotic assemblages associated with three size-fractions from surface to mesopelagic waters in three stations to explore whether the different surface conditions explained variations in the prokaryotic communities. The largest size-fractions harbored the prokaryotic communities with the lowest number of OTUs at the three stations. This pattern is contrary to that found in previous size-fractionation studies in the Mediterranean where prokaryotic richness was shown to increase with particle size and was attributed to higher niche availability (Mestre et al., 2017, 2020) but agrees with the decrease in richness from free-living to particle-attached found during the global Malaspina ocean expedition (Salazar et al., 2015; Mestre et al., 2018).
The communities from the three size-fractions showed pronounced vertical variations in OTU number, but these did not seem related to variations in surface productivity. For example, whereas at stations D02 (the less productive) and M48 (the most productive), richness decreased from the subsurface to mesopelagic waters, at station M36, smaller vertical changes were observed, except for a subsurface (~20–50 m) peak in richness which was observed across the three stations. This contrasts with studies in the Amundsen Sea polynya showing depth-driven increases in richness in free-living prokaryotic communities (Kim et al., 2014; Richert et al., 2019), and highlights a complexity in the nature of the particles and the associated microbial communities depending on the location and likely the surface conditions.
We found a dominance of groups like Alphaproteobacteria, Gammaproteobacteria, and Flavobacteriia which are the most common ones in the polar oceans (Ghiglione et al., 2012), although their abundances varied depending on the size-fraction and depth. Within them, groups like Colwellia, Pseudoalteromonas, Alcanivorax (Gammaproteobacteria), Polaribacter (Flavobacteriia), and Pseudophaeobacter (Alphaproteobacteria) were the most abundant. Previous reports in the Amundsen Sea polynya have shown a surface dominance of fast-growing copiotrophs including members of Flavobacteriia, Polaribacter, Gammaproteobacteria SAR92, and Oceanospirillaceae and/or of different members within Flavobacteriia and Alpha- and Gammaproteobacteria in mesopelagic waters (Delmont et al., 2014; Kim et al., 2014; Richert et al., 2015, Choi et al., 2016). Marine Flavobacteria have been described as major components of marine aggregates (e.g., Zhang et al., 2007), with abundances of particle-associated Flavobacteria suggested to be related to enhanced primary production (Abell and Bowman, 2005). However, none of these studies conducted particle size-fractionation analyses, so very little is known about the particle-attached microbiome of Antarctic polynyas. In our study site, we found that their abundance drastically decreased in the largest size-fraction, yet there was a higher abundance of Flavobacteriia in the Mertz stations (more productive) compared to the Dalton station. We found a remarkable dominance of Alphaproteobacteria in the largest size-fractions across most our samples, which comprised mostly the genera Brevundimonas and Sphingorhabdus at the Dalton station, and Pseudophaeobacter in the case of the two Mertz stations, pointing to large differences in the particle-associated communities between both polynyas. Although Alphaproteobacteria are usually found as free-living (Salazar et al., 2016; Mestre et al., 2020), they have been shown to dominate large particles in some areas of the ocean (Mestre et al., 2018) and genera such as Brevundimonas have been detected in late stages of particle colonization (Pelve et al., 2017). The differences in the community composition of the large particles from deep waters between the two polynyas could be due to differences in the origin and composition of particles, as well as to differences in the prokaryotic surface inocula (Mestre et al., 2018; Ruiz-González et al., 2020). Actually, we found that all studied communities harbored a relatively high fraction of OTUs that were exclusive from each station (unique OTUs), and this proportion was higher in some of the largest size-fractions from the Dalton D02 and the Mertz M36 stations. This supports that the local physicochemical or biotic conditions established within each polynya may select for specific taxa or that there is dispersal limitation of species across the sampled sites. However, in general, these unique OTUs represented a small fraction of communities in most cases and communities were dominated by taxa that showed presence in both polynyas.
Surface water properties are known to mold marine microbial communities in Antarctic waters (Piquet et al., 2011; Ghiglione et al., 2012; del Negro et al., 2018). For example, Wilkins et al. (2013) conducted a metagenomic survey from Hobart to the Mertz Glacier and found different taxonomic and functional microbial assemblages north and south of the Polar Front. The differences observed concurred with the more oligotrophic characteristics found north of the Polar Front compared to the usually enhanced primary production observed in summer in the Antarctic coastal areas. However, and despite the relevance of Antarctic polynyas in carbon cycling, few studies have characterized their pelagic prokaryotic communities, and most have focused on polynyas from the Amundsen Sea and on the free-living fraction of prokaryotic communities (e.g., Delmont et al., 2014; Kim et al., 2014; Richert et al., 2015, 2019; Choi et al., 2016). These studies have reported vertical differences in the abundances of several prokaryotic groups at different areas within the polynya (Kim et al., 2014) as well as clear linkages between phytoplankton communities and prokaryotic assemblages (Delmont et al., 2014; Kim et al., 2014; Richert et al., 2019). For example, Kim et al. (2014) found that free-living bacterioplankton abundance was strongly correlated with the abundance of Phaeocystis spp. and diatoms, and Richert et al. (2019) reported increases in surface bacterioplankton abundance as chlorophyll increased in surface waters during a phytoplankton bloom. Delmont et al. (2014) found that groups such as the SAR92 clade and Colwellia, which dominated different size-fractions and depths in our study, were prevalent particle-attached prokaryotic communities at the surface and at 250 m depth, respectively, in the Amundsen Polynya during a Phaeocystis bloom, suggesting that they may play important roles at different stages of the bloom. Our results also show highly different communities between surface and deep microbial communities from the two Antarctic polynyas, but the magnitude of these vertical changes differed largely between the studied sites and size-fractions.
4.2. Links between surface and mesopelagic communities
The differences in surface conditions and phytoplankton communities (Phaeocystis vs. diatoms) between the two studied polynyas translated into different particle fluxes (estimated through 234Th export fluxes) and carbon export efficiency, which were significantly lower in the Dalton Polynya compared with the Mertz Polynya (5% vs. 15%, respectively; see Ratnarajah et al., 2022). Estimates of particle export using 234Th proxy have not been previously obtained in Antarctic polynyas, but based on basin-wide and global compilations (e.g., Le Moigne et al., 2013; Owens et al., 2015; Puigcorbé et al., 2017b) the 234Th export flux estimated in D02 is very low, characteristic of oligotrophic and low productive ocean areas, whereas the flux at M36 is within the range of values found in temperate areas and the flux observed at M48 resembles fluxes observed under bloom conditions. POC export efficiencies (i.e., fraction of net primary production, NPP, that is exported to certain depth: POC flux/NPP*100) were found to be 3 times lower in the Dalton Polynya (5%) compared to the Mertz Polynya (15%), where transfer efficiencies down to 300 m were >80% (Ratnarajah et al., 2022).
The structure of phytoplankton communities impacts the particles produced in surface waters, consequently affecting carbon export rates (Siegel et al., 2014 and references therein). These sinking particles are known to influence the ecology and assembly of deep-sea microbial communities, not only by delivering surface-derived organic carbon (Arístegui et al., 2009; Herndl and Reinthaler, 2013), but also by directly transporting surface prokaryotes, some of which may colonize deeper waters (Mestre et al., 2018; Wenley et al., 2021). Despite the gradient in surface conditions and particle export, no clear clustering of the deep prokaryotic communities was observed by station (Figure 5), suggesting that the environmental characteristics were not different enough to lead to strong taxonomic variation between the three stations, not even between the highly contrasting Dalton and Mertz polynyas, when all samples were considered together. However, we found that the vertical dissimilarity between surface and deep communities was reduced (i.e., similarity was higher) when there was an increase in the Chl-a and POC inventories or when the flux of particles increased; in other words, the higher the production and export of particles (using Chl-a, POC and 234Th deficit as proxies), the more taxonomically similar were the surface and mesopelagic prokaryotic communities associated with the largest particles. It is worth mentioning that these correlations were observed for the >53 μm size-fraction only. This agrees with the observation that particles of large sizes are more efficient vectors of diversity from the surface to the deep ocean (Mestre et al., 2018), and also with the increases in vertical similarity between surface and meso- or bathypelagic particle-attached communities with increasing surface productivity observed across the global ocean (Ruiz-González et al., 2020).
The estimated variations in particle export fluxes also coincided with a higher contribution of surface-derived OTUs to mesopelagic communities in the largest size-fractions, with the station with the highest particle export fluxes having higher contributions of surface OTUs, supporting the hypothesis of a direct transport of surface bacteria down to mesopelagic waters in highly productive Antarctic polynyas. Similarly, a recent study by Valencia et al. (2022) conducted in the Eastern North Pacific, showed that the taxa found in sinking particles were more similar to the taxa found in the upper water column in highly productive areas than in oligotrophic waters. Also, a global expedition showed that the deep-sea prokaryotic taxa with presence in the overlying surface waters were found to be mainly typical copiotrophs or eukaryote-associated groups (Ruiz-González et al., 2020). All these support that particles reaching deeper waters may comprise larger, fast-sinking material of recent phytoplankton origin that may avoid remineralization processes occurring in shallow layers (Agusti et al., 2015; Grabowski et al., 2019), representing a direct inoculation of surface particle-attached prokaryotic taxa into deep waters, and explaining the increase in the contribution of surface OTUs to large mesopelagic particles in station M48, the one with the highest estimated particle export flux.
Several previous studies have also evidenced tight linkages between the surface and deep-ocean microbial communities. For example, changes in bathypelagic prokaryotic abundance or activity have been related to high carbon fluxes or surface primary production in different oceanic sites (Nagata et al., 2000; Hansell and Ducklow, 2003; Tamburini and Garcin, 2003; Yokokawa et al., 2013) and taxonomic shifts in deep-sea communities have been linked to spatial or temporal variations in surface conditions related to particle formation and sinking (Cram et al., 2015; Parada and Fuhrman, 2017; Santoro et al., 2017; Ruiz-González et al., 2020; Wenley et al., 2021). To our knowledge, however, very few studies have compared the vertical changes in prokaryotic community composition with particle export fluxes, except for the study by Poff et al. (2021), who found that in situations of elevated carbon flux events in the North Pacific Subtropical Gyre, particle-attached bacteria reaching abyssal depths had surface water origins, and Fadeev et al. (2021), who observed that ice-covered areas in the Arctic had higher carbon export and were also associated with lower dissimilarity between surface and deep sea microbial clades. Our study is in line with these recent findings and provides the first direct attempt to link simultaneous analyses of particle flux with the microbiome of particles in Antarctic polynyas. Our results suggest that changes in surface phytoplankton assemblages and/or productivity may strongly affect the deep-ocean microbial communities associated with the largest sinking particles.
5. Conclusion
The opening timings and size of Antarctic polynyas are likely to increase in coming years due to climate change, which will lead to changes in phytoplankton blooms (Montes-Hugo and Yuan, 2012; Deppeler and Davidson, 2017), as well as changes in the structure and function of microbial communities in these highly productive ecosystems (Piquet et al., 2011; Hörstmann et al., 2022) with yet unknown consequences for ecosystem functioning and carbon export production (Fan et al., 2020). Here, we evidenced that the vertical structuring of particle-attached microbial communities from two contrasting polynyas differed markedly depending on surface conditions. In general terms, there was a segregation of communities between surface and mesopelagic waters, yet differences were less strong at certain stations (M48) and particularly for communities associated with the largest size-fraction.
Alphaproteobacteria, Gammaproteobacteria, and Flavobacteriia dominated all communities, but different genera were found at different depths and size-fractions. The observed vertical, spatial, and size-fraction dependent variations in prokaryotic community composition support that there are different microbial niches within and across the studied polynyas. Estimates of particle export fluxes based on 234Th coincided with higher chlorophyll-a and particulate organic carbon concentrations and stocks. We found that when the particle flux was higher, mesopelagic prokaryotic communities from the largest size-fraction were more similar to those found in sunlit waters and contained higher proportions of surface-derived taxa, evidencing an intense downward transport of surface bacteria mediated by the largest particles. In consequence, surface conditions will influence differently the deep ocean prokaryotic assemblages depending on the size, origin, and composition of the sinking material. To our knowledge, this is the first direct evidence of compositional differences in particle-attached assemblages linked to the magnitude of the particle flux in Antarctic polynyas. Further research is needed to stablish a mechanistical model that could allow to predict bacterial community structures based on particle export and surface productivity.
Data availability statement
The raw sequence data have been deposited in the Figshare data repository, together with the non-rarefied OTU table, the taxonomy table and the environmental data used in this study, doi: 10.6084/m9.figshare.21385215.v1.
Author contributions
VP, JG, and PM participated in the design of the sampling scheme. VP performed the sampling and sample processing. VP and CR-G compiled the needed data, analyzed the data, and wrote this article. All authors contributed to the article and approved the submitted version.
Funding
The project that gave rise to these results received the support of a fellowship to VP from “la Caixa” Foundation (ID 100010434) and from the European Union’s Horizon 2020 research and innovation program under the Marie Skłodowska-Curie grant agreement no 847648 (fellowship code LCF/BQ/PI21/11830020). VP also received funding from Edith Cowan University (G1003456) and from the School of Science at Edith Cowan University (G1003362) to support this work. CR-G was supported by the grants RTI2018-101025-B-I00 and a Ramon y Cajal contract (RYC2019-026758-I) and JG by grants CTM2015-70340-R and PID2021-125469NB-C31 of the Spanish Ministry of Science and Innovation and by the Generalitat de Catalunya Consolidated Research Group 2017SGR/1568.
Acknowledgments
We would like to thank the RSV Aurora Australis crew and the Australian Antarctic Division technical support crew for their assistance and their great work during the 2016/2017 AA-v2 voyage (AU1602). Special thanks to the biogeochemistry group, Delphine Lannuzel, Sébastien Moreau, Matthew Corkill, Julie Janssens, Lavenia Ratnarajah, Mar Arroyo, and Cristina Genovese, also like to thank CSIRO hydrochemists for the analyses of nutrients. We warmly thank Montserrat Roca-Martí for her invaluable help with sample collection and preparation onboard of the RSV Aurora Australis, and Vanessa Balagué for DNA extraction and initial data processing. The IAEA is grateful for the support provided to its Environment Laboratories by the Government of the Principality of Monaco. This work acknowledges the “Severo Ochoa Centre of Excellence” accreditation (CEX2019-000928-S).
Conflict of interest
The authors declare that the research was conducted in the absence of any commercial or financial relationships that could be construed as a potential conflict of interest.
Publisher’s note
All claims expressed in this article are solely those of the authors and do not necessarily represent those of their affiliated organizations, or those of the publisher, the editors and the reviewers. Any product that may be evaluated in this article, or claim that may be made by its manufacturer, is not guaranteed or endorsed by the publisher.
Supplementary material
The Supplementary material for this article can be found online at: https://www.frontiersin.org/articles/10.3389/fmicb.2023.1078469/full#supplementary-material
References
Abell, G. C. J., and Bowman, J. P. (2005). Ecological and biogeographic relationships of class Flavobacteria in the Southern Ocean. FEMS Microbiol. Ecol. 51, 265–277. doi: 10.1016/j.femsec.2004.09.001
Agusti, S., González-Gordillo, J. I., Vaqué, D., Estrada, M., Cerezo, M. I., Salazar, G., et al. (2015). Ubiquitous healthy diatoms in the deep sea confirm deep carbon injection by the biological pump. Nat. Commun. 6:7608. doi: 10.1038/ncomms8608
Arístegui, J., Gasol, J. M., Duarte, C. M., and Herndld, G. J. (2009). Microbial oceanography of the dark ocean’s pelagic realm. Limnol. Oceanogr. 54, 1501–1529. doi: 10.4319/lo.2009.54.5.1501
Armstrong, F. A. J., Stearns, C. R., and Strickland, J. D. H. (1967). The measurement of upwelling and subsequent biological process by means of the Technicon autoanalyzer® and associated equipment. Deep-Sea Res. Oceanogr. Abstr. 14, 381–389. doi: 10.1016/0011-7471(67)90082-4
Arrigo, K. R., and van Dijken, G. L. (2003). Phytoplankton dynamics within 37 Antarctic coastal polynya systems. J. Geophys. Res. Oceans 108:108. doi: 10.1029/2002JC001739
Arrigo, K. R., Dijken, G. L.Van, and Bushinsky, S. (2008). Primary production in the Southern Ocean, 1997–2006. J. Geophys. Res. Oceans 113:113. doi: 10.1029/2007JC004551
Arrigo, K. R., Dijken, G. L.Van, and Strong, A. L. (2015). Environmental controls of marine productivity hot spots around Antarctica. J Geophys Res Oceans 120, 5545–5565. doi: 10.1002/2015JC010888
Black, E. E., Lam, P. J., Lee, J. -M., and Buesseler, K. O. (2019). Insights from the 238U-234Th method into the coupling of biological export and the cycling of cadmium, cobalt, and manganese in the Southeast Pacific Ocean. Global Biogeochem. Cycles 33, 15–36. doi: 10.1029/2018GB005985
Buesseler, K. O., and Boyd, P. W. (2009). Shedding light on processes that control particle export and flux attenuation in the twilight zone of the open ocean. Limnol. Oceanogr. 54, 1210–1232. doi: 10.4319/lo.2009.54.4.1210
Ceballos-Romero, E., Buesseler, K. O., and Villa-Alfageme, M. (2022). Revisiting five decades of 234Th data: a comprehensive global oceanic compilation. Earth Syst Sci Data 14, 2639–2679. doi: 10.5194/essd-14-2639-2022
Choi, S.-B., Kim, J.-G., Jung, M.-Y., Kim, S.-J., Min, U.-G., Si, O.-J., et al. (2016). Cultivation and biochemical characterization of heterotrophic bacteria associated with phytoplankton bloom in the Amundsen Sea polynya, Antarctica. Deep-Sea Res. II Top. Stud. Oceanogr. 123, 126–134. doi: 10.1016/j.dsr2.2015.04.027
Clevenger, S. J., Benitez-Nelson, C. R., Drysdale, J., Pike, S., Puigcorbé, V., and Buesseler, K. O. (2021). Review of the analysis of 234Th in small volume (2–4 L) seawater samples: improvements and recommendations. J. Radioanal. Nucl. Chem. 329, 1–13. doi: 10.1007/s10967-021-07772-2
Cram, J. A., Chow, C.-E. T., Sachdeva, R., Needham, D. M., Parada, A. E., Steele, J. A., et al. (2015). Seasonal and interannual variability of the marine bacterioplankton community throughout the water column over ten years. ISME J. 9, 563–580. doi: 10.1038/ismej.2014.153
de Jong, J., Schoemann, V., Lannuzel, D., Croot, P., de Baar, H., and Tison, J.-L. (2012). Natural iron fertilization of the Atlantic sector of the Southern Ocean by continental shelf sources of the Antarctic peninsula. J. Geophys. Res. 117:G01029. doi: 10.1029/2011JG001679
del Castillo, C. E., Signorini, S. R., Karaköylü, E. M., and Rivero-Calle, S. (2019). Is the Southern Ocean getting greener? Geophys. Res. Lett. 46, 6034–6040. doi: 10.1029/2019GL083163
del Negro, P., Celussi, M., de Vittor, C., and Fonda Umani, S. (2018). Rapid acclimation of microbes to changing substrate pools in epipelagic waters of an Antarctic polynya during austral summer 2003. Polar Biol. 41, 1–10. doi: 10.1007/s00300-017-2165-5
Delmont, T. O., Hammar, K. M., Ducklow, H. W., Yager, P. L., and Post, A. F. (2014). Phaeocystis Antarctica blooms strongly influence bacterial community structures in the Amundsen Sea polynya. Front. Microbiol. 5:646. doi: 10.3389/fmicb.2014.00646
Deppeler, S. L., and Davidson, A. T. (2017). Southern Ocean phytoplankton in a changing climate. Front. Mar. Sci. 4:40. doi: 10.3389/fmars.2017.00040
Ducklow, H. W., Wilson, S. E., Post, A. F., Stammerjohn, S. E., Erickson, M., Lee, S., et al. (2015). Particle flux on the continental shelf in the Amundsen Sea polynya and Western Antarctic peninsula. Elementa Sci. Anthropocene 3:46. doi: 10.12952/journal.elementa.000046
Ducklow, H. W., and Yager, P. L. (2007). “Chapter 10 pelagic bacterial processes in polynyas” in Polynyas: Windows to the World Elsevier Oceanography Series. eds. W. O. Smith and D. G. Barber (Elsevier), 323–361.
Edgar, R. C. (2013). UPARSE: highly accurate OTU sequences from microbial amplicon reads. Nat. Methods 10, 996–998. doi: 10.1038/nmeth.2604
Fadeev, E., Rogge, A., Ramondenc, S., Nöthig, E.-M., Wekerle, C., Bienhold, C., et al. (2021). Sea ice presence is linked to higher carbon export and vertical microbial connectivity in the Eurasian Arctic Ocean. Commun Biol 4:1255. doi: 10.1038/s42003-021-02776-w
Fan, G., Han, Z., Ma, W., Chen, S., Chai, F., Mazloff, M. R., et al. (2020). Southern Ocean carbon export efficiency in relation to temperature and primary productivity. Sci. Rep. 10:13494. doi: 10.1038/s41598-020-70417-z
Ghiglione, J.-F., Galand, P. E., Pommier, T., Pedrós-Alió, C., Maas, E. W., Bakker, K., et al. (2012). Pole-to-pole biogeography of surface and deep marine bacterial communities. Proc. Natl. Acad. Sci. 109, 17633–17638. doi: 10.1073/pnas.1208160109
Grabowski, E., Letelier, R. M., Laws, E. A., and Karl, D. M. (2019). Coupling carbon and energy fluxes in the North Pacific subtropical gyre. Nat. Commun. 10:1895. doi: 10.1038/s41467-019-09772-z
Gruber, N., Gloor, M., Fletcher, S. E. M., Doney, S. C., Dutkiewicz, S., Follows, M. J., et al. (2009). Oceanic sources, sinks, and transport of atmospheric CO2. Global Biogeochem. Cycles 23:GB1005. doi: 10.1029/2008GB003349
Gustafsson, Ö., Gschwend, P. M., and Buesseler, K. O. (1997). Using 234Th disequilibria to estimate the vertical removal rates of polycyclic aromatic hydrocarbons from the surface ocean. Mar. Chem. 57, 11–23. doi: 10.1016/S0304-4203(97)00011-X
Hansell, D. A., and Ducklow, H. W. (2003). Bacterioplankton distribution and production in the bathypelagic ocean: directly coupled to particulate organic carbon export? Limnol. Oceanogr. 48, 150–156. doi: 10.4319/lo.2003.48.1.0150
Henson, S., le Moigne, F., and Giering, S. (2019). Drivers of carbon export efficiency in the Global Ocean. Global Biogeochem. Cycles 33, 891–903. doi: 10.1029/2018GB006158
Henson, S. A., Sanders, R., and Madsen, E. (2012). Global patterns in efficiency of particulate organic carbon export and transfer to the deep ocean. Global Biogeochem. Cycles 26:4099. doi: 10.1029/2011GB004099
Herndl, G. J., and Reinthaler, T. (2013). Microbial control of the dark end of the biological pump. Nat. Geosci. 6, 718–724. doi: 10.1038/ngeo1921
Hoppema, M., and Anderson, L. G. (2007). “Chapter 6 biogeochemistry of polynyas and their role in sequestration of anthropogenic constituents” in Polynyas: Windows to the World Elsevier Oceanography Series. eds. W. O. Smith and D. G. Barber (Elsevier), 193–221.
Hörstmann, C., Buttigieg, P. L., John, U., Raes, E. J., Wolf-Gladrow, D., Bracher, A., et al. (2022). Microbial diversity through an oceanographic lens: refining the concept of ocean provinces through trophic-level analysis and productivity-specific length scales. Environ. Microbiol. 24, 404–419. doi: 10.1111/1462-2920.15832
Jena, B., and Pillai, A. N. (2020). Satellite observations of unprecedented phytoplankton blooms in the Maud rise polynya, Southern Ocean. Cryosphere 14, 1385–1398. doi: 10.5194/tc-14-1385-2020
Kang, S.-H., Kang, J.-S., Lee, S., Chung, K. H., Kim, D., and Park, M. G. (2001). Antarctic phytoplankton assemblages in the marginal ice zone of the northwestern Weddell Sea. J. Plankton Res. 23, 333–352. doi: 10.1093/plankt/23.4.333
Karnovsky, N., Ainley, D. G., and Lee, P. (2007). “Chapter 12 the impact and importance of production in polynyas to top-trophic predators: three case histories” in Polynyas: Windows to the World Elsevier Oceanography Series. eds. W. O. Smith and D. G. Barber (Elsevier), 391–410.
Kérouel, R., and Aminot, A. (1997). Fluorometric determination of ammonia in sea and estuarine waters by direct segmented flow analysis. Mar. Chem. 57, 265–275. doi: 10.1016/S0304-4203(97)00040-6
Kim, J.-G., Park, S.-J., Quan, Z.-X., Jung, M.-Y., Cha, I.-T., Kim, S.-J., et al. (2014). Unveiling abundance and distribution of planktonic bacteria and archaea in a polynya in Amundsen Sea, Antarctica. Environ. Microbiol. 16, 1566–1578. doi: 10.1111/1462-2920.12287
Kirchman, D. L., Morán, X. A. G., and Ducklow, H. (2009). Microbial growth in the polar oceans—role of temperature and potential impact of climate change. Nat. Rev. Microbiol. 7, 451–459. doi: 10.1038/nrmicro2115
Knap, A. H., Michaels, A., Close, A. R., Ducklow, H., and Dickson, A. G. (1996). Protocols for the joint global ocean flux study (JGOFS) core measurements. J. GOFS 19, 1–210.
Lannuzel, D., Schoemann, V., de Jong, J., Pasquer, B., van der Merwe, P., Masson, F., et al. (2010). Distribution of dissolved iron in Antarctic Sea ice: spatial, seasonal, and inter-annual variability. J. Geophys. Res. Biogeosci. 115:1031. doi: 10.1029/2009JG001031
le Moigne, F. A. C., Henson, S. A., Cavan, E., Georges, C., Pabortsava, K., Achterberg, E. P., et al. (2016). What causes the inverse relationship between primary production and export efficiency in the Southern Ocean? Geophys. Res. Lett. 43, 4457–4466. doi: 10.1002/2016GL068480
le Moigne, F. A. C., Henson, S. A., Sanders, R. J., and Madsen, E. (2013). Global database of surface ocean particulate organic carbon export fluxes diagnosed from the 234Th technique. Earth Syst Sci Data 5, 295–304. doi: 10.5194/essd-5-295-2013
Lee, S., Hwang, J., Ducklow, H. W., Hahm, D., Lee, S. H., Kim, D., et al. (2017). Evidence of minimal carbon sequestration in the productive Amundsen Sea polynya. Geophys. Res. Lett. 44, 7892–7899. doi: 10.1002/2017GL074646
Logares, R. (2017). Workflow for Analysing MiSeq Amplicons Based on Uparse v1.5. doi: 10.5281/zenodo.259579
Luria, C. M., Amaral-Zettler, L. A., Ducklow, H. W., and Rich, J. J. (2016). Seasonal succession of free-living bacterial communities in coastal waters of the Western Antarctic peninsula. Front. Microbiol. 7:1731. doi: 10.3389/fmicb.2016.01731
Maiti, K., Charette, M. A., Buesseler, K. O., and Kahru, M. (2013). An inverse relationship between production and export efficiency in the Southern Ocean. Geophys. Res. Lett. 40, 1557–1561. doi: 10.1002/grl.50219
Martin, M. (2011). Cutadapt removes adapter sequences from high-throughput sequencing reads. EMBnet J 17:10. doi: 10.14806/ej.17.1.200
Mestre, M., Borrull, E., Sala, M. M., and Gasol, J. M. (2017). Patterns of bacterial diversity in the marine planktonic particulate matter continuum. ISME J. 11, 999–1010. doi: 10.1038/ismej.2016.166
Mestre, M., Höfer, J., Sala, M. M., and Gasol, J. M. (2020). Seasonal variation of bacterial diversity along the marine particulate matter continuum. Front. Microbiol. 11:1590. doi: 10.3389/fmicb.2020.01590
Mestre, M., Ruiz-González, C., Logares, R., Duarte, C. M., Gasol, J. M., and Sala, M. M. (2018). Sinking particles promote vertical connectivity in the ocean microbiome. Proc. Natl. Acad. Sci. 115, E6799–E6807. doi: 10.1073/pnas.1802470115
Miller, L. A., and DiTullio, G. R. (2007). “Chapter 5 gas fluxes and dynamics in polynyas” in Polynyas: Windows to the World Elsevier Oceanography Series. eds. W. O. Smith and D. G. Barber (Elsevier), 163–191.
Montes-Hugo, M. A., and Yuan, X. (2012). Climate patterns and phytoplankton dynamics in Antarctic latent heat polynyas. J Geophys Res Oceans 117:6597. doi: 10.1029/2010JC006597
Moreau, S., Lannuzel, D., Janssens, J., Arroyo, M. C., Corkill, M., Cougnon, E., et al. (2019). Sea-ice meltwater and circumpolar deep water drive contrasting productivity in three Antarctic polynyas. J Geophys Res Oceans 124, 2943–2968. doi: 10.1029/2019JC015071
Murphy, J., and Riley, J. P. (1962). A modified single solution method for the determination of phosphate in natural waters. Anal. Chim. Acta 27, 31–36. doi: 10.1016/S0003-2670(00)88444-5
Nagata, T., Fukuda, H., Fukuda, R., and Koike, I. (2000). Bacterioplankton distribution and production in deep Pacific waters: large–scale geographic variations and possible coupling with sinking particle fluxes. Limnol. Oceanogr. 45, 426–435. doi: 10.4319/lo.2000.45.2.0426
Nguyen, T. T. H., Zakem, E. J., Ebrahimi, A., Schwartzman, J., Caglar, T., Amarnath, K., et al. (2022). Microbes contribute to setting the ocean carbon flux by altering the fate of sinking particulates. Nat. Commun. 13:1657. doi: 10.1038/s41467-022-29297-2
Owens, S. A., Buesseler, K. O., and Sims, K. W. W. (2011). Re-evaluating the 238U-salinity relationship in seawater: implications for the 238U-234Th disequilibrium method. Mar. Chem. 127, 31–39. doi: 10.1016/j.marchem.2011.07.005
Owens, S. A., Pike, S., and Buesseler, K. O. (2015). Thorium-234 as a tracer of particle dynamics and upper ocean export in the Atlantic Ocean. Deep-Sea Res. II Top. Stud. Oceanogr. 116, 42–59. doi: 10.1016/j.dsr2.2014.11.010
Parada, A. E., and Fuhrman, J. A. (2017). Marine archaeal dynamics and interactions with the microbial community over 5 years from surface to seafloor. ISME J. 11, 2510–2525. doi: 10.1038/ismej.2017.104
Parada, A. E., Needham, D. M., and Fuhrman, J. A. (2015). Every base matters: assessing small subunit rRNA primers for marine microbiomes with mock communities, time series and global field samples. Environ. Microbiol. 18, 1403–1414. doi: 10.1111/1462-2920.13023
Pelve, E. A., Fontanez, K. M., and DeLong, E. F. (2017). Bacterial succession on sinking particles in the Ocean’s interior. Front. Microbiol. 8:2269. doi: 10.3389/fmicb.2017.02269
Piquet, A. M.-T., Bolhuis, H., Meredith, M. P., and Buma, A. G. J. (2011). Shifts in coastal Antarctic marine microbial communities during and after melt water-related surface stratification. FEMS Microbiol. Ecol. 76, 413–427. doi: 10.1111/j.1574-6941.2011.01062.x
Poff, K. E., Leu, A. O., Eppley, J. M., Karl, D. M., and DeLong, E. F. (2021). Microbial dynamics of elevated carbon flux in the open ocean’s abyss. Proc. Natl. Acad. Sci. 118:e2018269118. doi: 10.1073/pnas.2018269118
Puigcorbé, V., Masqué, P., and le Moigne, F. A. C. (2020). Global database of ratios of particulate organic carbon to thorium-234 in the ocean: improving estimates of the biological carbon pump. Earth Syst Sci Data 12, 1267–1285. doi: 10.5194/essd-12-1267-2020
Puigcorbé, V., Roca-Martí, M., Masqué, P., Benitez-Nelson, C. R., Rutgers, V. D., Loeff, M., et al. (2017a). Particulate organic carbon export across the Antarctic circumpolar current at 10°E: differences between north and south of the Antarctic polar front. Deep-Sea Res. II Top. Stud. Oceanogr. 138, 86–101. doi: 10.1016/j.dsr2.2016.05.016
Puigcorbé, V., Roca-Martí, M., Masqué, P., Benitez-Nelson, C., Rutgers Van Der Loeff, M., Bracher, A., et al. (2017b). Latitudinal distributions of particulate carbon export across the North Western Atlantic Ocean. Deep Sea Res 1 Oceanogr Res Pap 129, 116–130. doi: 10.1016/j.dsr.2017.08.016
Ratnarajah, L., Puigcorbé, V., Moreau, S., Roca-Martí, M., Janssens, J., Corkill, M., et al. (2022). Distribution and export of particulate organic carbon in East Antarctic coastal polynyas. Deep Sea Research Part I: Oceanographic Research Papers. 103899. doi: 10.1016/j.dsr.2022.103899
R Core Team (2017). R: A Language and Environment for Statistical Computing. Available at: https://www.R-project.org/
Richert, I., Dinasquet, J., Logares, R., Riemann, L., Yager, P. L., Wendeberg, A., et al. (2015). The influence of light and water mass on bacterial population dynamics in the Amundsen Sea polynya. Elementa Sci. Anthropocene 3:44. doi: 10.12952/journal.elementa.000044
Richert, I., Yager, P. L., Dinasquet, J., Logares, R., Riemann, L., Wendeberg, A., et al. (2019). Summer comes to the Southern Ocean: how phytoplankton shape bacterioplankton communities far into the deep dark sea. Ecosphere 10:e02641. doi: 10.1002/ecs2.2641
Rosenberg, M., and Rintoul, S. (2017). Aurora Australis Marine Science Cruise AU1602, Dalton, Mertz and Ninnis CTD’s—Oceanographic Field Measurements and Analysis. Hobart: Australian Antarctic Data Centre.
Ruiz-González, C., Mestre, M., Estrada, M., Sebastián, M., Salazar, G., Agustí, S., et al. (2020). Major imprint of surface plankton on deep ocean prokaryotic structure and activity. Mol. Ecol. 29, 1820–1838. doi: 10.1111/mec.15454
Salazar, G., Cornejo-Castillo, F. M., Benitez-Barrios, V., Fraile-Nuez, E., Alvarez-Salgado, X. A., Duarte, C. M., et al. (2016). Global diversity and biogeography of deep-sea pelagic prokaryotes. ISME J. 10, 596–608. doi: 10.1038/ismej.2015.137
Salazar, G., Cornejo-Castillo, F. M., Borrull, E., Díez-Vives, C., Lara, E., Vaqué, D., et al. (2015). Particle-association lifestyle is a phylogenetically conserved trait in bathypelagic prokaryotes. Mol. Ecol. 24, 5692–5706. doi: 10.1111/mec.13419
Santoro, A. E., Saito, M. A., Goepfert, T. J., Lamborg, C. H., Dupont, C. L., and DiTullio, G. R. (2017). Thaumarchaeal ecotype distributions across the equatorial Pacific Ocean and their potential roles in nitrification and sinking flux attenuation. Limnol. Oceanogr. 62, 1984–2003. doi: 10.1002/lno.10547
Sedwick, P. N., and DiTullio, G. R. (1997). Regulation of algal blooms in Antarctic shelf waters by the release of iron from melting sea ice. Geophys. Res. Lett. 24, 2515–2518. doi: 10.1029/97GL02596
Shadwick, E. H., Rintoul, S. R., Tilbrook, B., Williams, G. D., Young, N., Fraser, A. D., et al. (2013). Glacier tongue calving reduced dense water formation and enhanced carbon uptake. Geophys. Res. Lett. 40, 904–909. doi: 10.1002/grl.50178
Siegel, D. A., Buesseler, K. O., Doney, S. C., Sailley, S. F., Behrenfeld, M. J., and Boyd, P. W. (2014). Global assessment of ocean carbon export by combining satellite observations and food-web models. Global Biogeochem. Cycles 28, 181–196. doi: 10.1002/2013GB004743
Smith, S., Altieri, K. E., Mdutyana, M., Walker, D. R., Parrott, R. G., Gallie, S., et al. (2022). Biogeochemical controls on ammonium accumulation in the surface layer of the Southern Ocean. Biogeosciences 19, 715–741. doi: 10.5194/bg-19-715-2022
Tamburini, C., and Garcin, J. (2003). Role of deep-sea bacteria in organic matter mineralization and adaptation to hydrostatic pressure conditions in the NW Mediterranean Sea. Aquat. Microb. Ecol. 32, 209–218. doi: 10.3354/ame032209
Tesán Onrubia, J. A., Petrova, M. V., Puigcorbé, V., Black, E. E., Valk, O., Dufour, A., et al. (2020). Mercury export flux in the Arctic Ocean estimated from 234Th/238U disequilibria. ACS Earth Space Chem 4, 795–801. doi: 10.1021/acsearthspacechem.0c00055
Valencia, B., Stukel, M. R., Allen, A. E., McCrow, J. P., Rabines, A., and Landry, M. R. (2022). Microbial communities associated with sinking particles across an environmental gradient from coastal upwelling to the oligotrophic ocean. Deep-Sea Res. I Oceanogr. Res. Pap. 179:103668. doi: 10.1016/j.dsr.2021.103668
Wenley, J., Currie, K., Lockwood, S., Thomson, B., Baltar, F., and Morales, S. E. (2021). Seasonal prokaryotic community linkages between surface and Deep Ocean water. Front. Mar. Sci. 8:659641. doi: 10.3389/fmars.2021.659641
Wiedmann, I., Ceballos-Romero, E., Villa-Alfageme, M., Renner, A. H. H., Dybwad, C., van der Jagt, H., et al. (2020). Arctic observations identify phytoplankton community composition as driver of carbon flux attenuation. Geophys. Res. Lett. 47:e2020GL087465. doi: 10.1029/2020GL087465
Wilkins, D., Lauro, F. M., Williams, T. J., Demaere, M. Z., Brown, M. V., Hoffman, J. M., et al. (2013). Biogeographic partitioning of Southern Ocean microorganisms revealed by metagenomics. Environ. Microbiol. 15, 1318–1333. doi: 10.1111/1462-2920.12035
Wood, E. D., Armstrong, F. A. J., and Richards, F. A. (1967). Determination of nitrate in sea water by cadmium-copper reduction to nitrite. J. Mar. Biol. Assoc. U. K. 47, 23–31. doi: 10.1017/S002531540003352X
Yokokawa, T., Yang, Y., Motegi, C., and Nagata, T. (2013). Large-scale geographical variation in prokaryotic abundance and production in meso- and bathypelagic zones of the Central Pacific and Southern Ocean. Limnol. Oceanogr. 58, 61–73. doi: 10.4319/lo.2013.58.1.0061
Zhang, J., Kobert, K., Flouri, T., and Stamatakis, A. (2014). PEAR: a fast and accurate Illumina paired-end reAd mergeR. Bioinformatics 30, 614–620. doi: 10.1093/bioinformatics/btt593
Keywords: prokaryotic communities, marine particles, 234Thorium, particle export, polynyas, Antarctica, particle size fractionation, particle-attached and free-living prokaryotes
Citation: Puigcorbé V, Ruiz-González C, Masqué P and Gasol JM (2023) Impact of particle flux on the vertical distribution and diversity of size-fractionated prokaryotic communities in two East Antarctic polynyas. Front. Microbiol. 14:1078469. doi: 10.3389/fmicb.2023.1078469
Edited by:
Hongbin Liu, Hong Kong University of Science and Technology, Hong Kong SAR, ChinaReviewed by:
Jiwen Liu, Ocean University of China, ChinaAngelina Lo Giudice, Department of Earth System Sciences and Technologies for the Environment, Institute of Polar Sciences (CNR), Italy
Copyright © 2023 Puigcorbé, Ruiz-González, Masqué and Gasol. This is an open-access article distributed under the terms of the Creative Commons Attribution License (CC BY). The use, distribution or reproduction in other forums is permitted, provided the original author(s) and the copyright owner(s) are credited and that the original publication in this journal is cited, in accordance with accepted academic practice. No use, distribution or reproduction is permitted which does not comply with these terms.
*Correspondence: Viena Puigcorbé, ✉ dmllbmEucHVpZ2NvcmJlQG91dGxvb2suY29t; ✉ dmllbmFwQGljbS5jc2ljLmVz; Clara Ruiz-González, ✉ Y2xhcmFyZ0BpY20uY3NpYy5lcw==
†These authors have contributed equally to this work and share first authorship