- 1School of Biological and Behavioural Sciences, Queen Mary University of London, London, United Kingdom
- 2Department of Pathobiology and Population Sciences, Royal Veterinary College, Hatfield, United Kingdom
- 3Ecology and Genetics Research Unit, University of Oulu, Oulu, Finland
- 4Tvärminne Zoological Station, University of Helsinki, Hanko, Finland
- 5Department of Ecology and Evolution, University of Lausanne, Lausanne, Switzerland
Introduction: Insects share intimate relationships with microbes that play important roles in their biology. Yet our understanding of how host-bound microbial communities assemble and perpetuate over evolutionary time is limited. Ants host a wide range of microbes with diverse functions and are an emerging model for studying the evolution of insect microbiomes. Here, we ask whether phylogenetically related ant species have formed distinct and stable microbiomes.
Methods: To answer this question, we investigated the microbial communities associated with queens of 14 Formica species from five clades, using deep coverage 16S rRNA amplicon sequencing.
Results: We reveal that Formica species and clades harbor highly defined microbial communities that are dominated by four bacteria genera: Wolbachia, Lactobacillus, Liliensternia, and Spiroplasma. Our analysis reveals that the composition of Formica microbiomes mirrors the phylogeny of the host, i.e., phylosymbiosis, in that related hosts harbor more similar microbial communities. In addition, we find there are significant correlations between microbe co-occurrences.
Discussion: Our results demonstrate Formica ants carry microbial communities that recapitulate the phylogeny of their hosts. Our data suggests that the co-occurrence of different bacteria genera may at least in part be due to synergistic and antagonistic interactions between microbes. Additional factors potentially contributing to the phylosymbiotic signal are discussed, including host phylogenetic relatedness, host-microbe genetic compatibility, modes of transmission, and similarities in host ecologies (e.g., diets). Overall, our results support the growing body of evidence that microbial community composition closely depends on the phylogeny of their hosts, despite bacteria having diverse modes of transmission and localization within the host.
Introduction
All multicellular organisms interact with microbes that influence their biology, and this is particularly true for insects. Microbial associates of insects are integral to many aspects of their hosts’ biology, including nutrition, immunity, reproduction, growth, and metabolism (Feldhaar et al., 2007; Horak et al., 2020; Singh and Linksvayer, 2020). Both parasitic and beneficial relationships between insects and their microbes can therefore drive important population processes such as speciation, by inducing pre or postzygotic isolation (Miller et al., 2010), and ecological expansion, by unlocking previously inaccessible niches (Russell et al., 2009; Bennett and Moran, 2015; Sanders et al., 2017). Yet, we are only beginning to understand how the communities of microbes associated with insects – their microbiomes – assemble and perpetuate over evolutionary time.
Ants are an excellent system for understanding how and why microbiomes evolve, as they have formed a wide range of relationships with microbes, from stable associations with a single microbe to relatively complex gut communities. Stable associations include heritable endosymbionts, such as Wolbachia, Arsenophonus, and Spiroplasma (Russell et al., 2012), as well as Blochmannia and Westeberhardia, the ancient bacteriocyte-associated symbionts found in Camponotus and Cardiocondyla ants, respectively (Degnan et al., 2002; Klein et al., 2016). Several ant genera have also evolved stable communities of microbes housed in their guts, such as Cephalotes turtle ants, whereas other ant lineages show little to no consistent associations with any gut-associated microbes (Sanders et al., 2017; Hu et al., 2018). While there are a few detailed investigations of unique, vertically transmitted symbionts (e.g., Klein et al., 2016; Jackson et al., 2022), and broad comparative analyses across phylogenetically diverse host species (e.g., Russell et al., 2009; Sanders et al., 2017), ants, in general, have been understudied for microbes. More recently, studies on phylogenetically related species have shown ant microbiomes can be shaped by a number of factors including host geography, food sources, host species, and for some bacteria, co-cladogenesis with the host phylogeny has been observed (Martins and Moreau, 2020; Graber et al., 2022; Ramalho and Moreau, 2023). Although these studies have made progress on this topic, the relative importance of host genetic background and ecology in shaping the microbial communities associated with most ant genera remains unclear.
Studying microbiome structure across related hosts helps us disentangle the factors driving patterns observed in individual species and answer key questions, such as: whether phylogenetically related hosts evolved similar microbiomes, a signature of phylosymbiosis (Lim and Bordenstein, 2020); whether the presence of host-bound microbes is influenced by antagonistic or synergistic interactions with other microbes; or whether shared host ecologies shape the communities of microbes insects carry (Brooks et al., 2017). Formica ants are an ideal model to address these questions, as they have formed persistent associations with at least two heritable symbionts, the bacteriocyte-associated mutualist Liliensternia, and the reproductive parasite Wolbachia, as well as with gut-associated microbes such as Lactobacillus (Russell, 2012; Zheng et al., 2021; Jackson et al., 2022).
Here, we ask whether Formica ants harbor stable communities of microbes. To answer this question, we assess the composition and consistency of microbiomes in queens from 14 Formica species belonging to five clades, using deep coverage 16S rRNA amplicon sequencing. We focus the analysis on queens, because ant workers have been found to lose ovarially transmitted microbes as they age (Wenseleers et al., 2002; Wolschin et al., 2004). We first identify the genera of microbes associated with Formica ants. We then test whether there are significant differences in microbiome composition, and what microbes make up these differences, across Formica species and clades. Based on these results, we then test whether the microbiome composition of Formica species has a phylogenetic signal by examining if phylogenetically related hosts are more likely to carry similar microbial communities, and test for potential antagonistic or synergistic interaction between microbes.
Materials and methods
Sample collection procedure and Formica species identification
Samples were collected by opening or digging up ant colonies, sorting workers and queens by hand, and preserving them in ethanol. Species were identified using morphological features at established field sites within the species known distributions by Formica experts H. Helantera and M. Chapuisat. The location of each sample collection and identity of the collector are listed in Supplementary Table 1.
16S rRNA sequencing procedure
Genomic DNA was extracted from whole bodies of single adult queens using the Qiagen DNeasy Blood and Tissue Kit (Qiagen, Venlo, Netherlands) according to the manufacturers’ protocol or using a standard phenol chloroform extraction method. Sample extraction method is listed in Supplementary Table 1. We screened 130 adult queens from 89 colonies across 14 species, using two separate runs of 16S rRNA sequencing. See Supplementary Table 1 for samples identified by run. Samples were acquired at different times and were therefore sequenced on two separate Illumina runs. We included negative controls on each run which consisted of DNA extractions on purified water.
In run 1, we used the 515F/806R primer pair (Caporaso et al., 2011) to amplify the V4 region of the 16S rRNA gene (Supplementary Table 6). All PCR reactions were performed using Q5 High-Fidelity master mix (New England Biolabs, Ipswich, MA, USA). For the first stage PCR, amplification conditions were as follows: initial denaturation at 98°C for 30 s followed by 25 cycles of 98°C for 10 s, 50°C for 15 s, 72°C for 20 s, and a final extension of 72°C for 5 min. PCR clean-ups were performed using AMPureXP beads (Beckman Coulter Life Sciences, IN, United States) and then a second stage PCR was carried out to attach dual indices and Illumina sequencing adapters. Second stage PCR conditions were as follows: 95°C for 3 min followed by 8 cycles of 98°C for 20 s, 55°C for 15 s, 72°C for 15 s, and a final extension of 72°C for 5 min. A second PCR clean-up using AMPure XP beads was performed to clean up the libraries before quantification. Individual PCR products were quantified using the Qubit dsDNA HS Assay Kit (Thermo Fisher Scientific, MA, United States) and the libraries were then normalized and pooled. The pool was sequenced at Edinburgh Genomics (University of Edinburgh) on an Illumina MiSeq (paired-end, 2 × 250 bp reads).
For run 2, we used the same primer pair, PCR mix and PCR conditions as in run 1, however, the PCR products were submitted to the Centre for Genomic Research (University of Liverpool) for addition of indices and adapters, and pooling of libraries. Sequencing was then carried out on a MiSeq (paired-end, 2 × 250 bp reads).
Creation of amplicon sequence variants and operational taxonomic units
Adaptor sequences were removed using the function “ILLUMINACLIP” of Trimmomatic V.0.38 (Bolger et al., 2014), under default parameters. Each sequencing run was then imported into the Qiime2 bioinformatics platform (Bolyen et al., 2019) and denoised into amplicon sequence variants (ASVs) using DADA2 (Callahan et al., 2016). We then filtered the resulting ASVs for potential contamination by discarding those at <1% relative abundance within a sample and removed ASV’s belonging to mitochondria, chloroplasts, and non-bacterial taxa. Trace ASVs unique to controls were removed as potential contaminants, as were ASVs in negative controls that were also ubiquitously found in all other samples within a sequencing run. ASVs at trace levels in controls that were also present in other samples at higher abundances, but not ubiquitous across samples, were retained. After filtering, all samples with a summed count of <3,000 ASVs were removed which accounted for all controls and two experimental samples. This filter was based on examining the total count of ASVs per sample and identifying a cut-off point based on a natural separation of the data (Supplementary Image 1). We then ensured there was no significant relationship between total number of ASVs observed and number of unique ASVs observed (Supplementary Image 2). For each sequencing run, filtered ASVs were then combined into a single table (Supplementary Table 6). We then clustered ASVs into 97% operational taxonomic units (OTUs) using Vsearch (Rognes et al., 2016). ASV and OTU sequences were assigned to taxons using Qiime2’s classification algorithm with the silva 138 99% classifier database (Quast et al., 2013). ASV and OTU tables, alongside taxonomic assignments, were then exported for additional analysis in R Statistical Software v4.1.2 (R Core Team, 2019). All statistical analyses were conducted on ASVs. OTU data was used for visualization purposes only and was not included in statistical analyzed.
Statistical analyses and data visualization
In R, data were normalized by changing ASV counts per sample into a percentage of total ASVs for each sample (relative abundance). To create a visualization of the ASV data, ASVs were collapsed to the genus level. To visualize the data, any genus present in more than one sample, or >10% relative abundance in a single sample, was given a distinct color. All other genera were represented in gray as “Other”. Bar graphs depicting the relative abundance of the dominant bacterial genera within each sample were then produced using ggplot2 (Wickham, 2016).
For analyzing differences in bacterial community composition, all ASV data was converted into a Bray–Curtis distance matrix using the vegdist function from the vegan package in R (Oksanen et al., 2013). A permanova analysis of the distance matrix was performed using adonis. The first comparison made was DistanceMatrix ∼ Sequencing Run, to investigate the impact of Sequencing Run on the centroids and dispersion of samples. We then investigated the effect of clade and species with run as both an interactive effect (DistanceMatrix∼ Run × Clade/Species) and as a blocked effect (DistanceMatrix∼Clade/Species, strata = Run). Clade was defined in a manner identical to Romiguier et al. (2018). All analyses were run for 1,000 permutations. We analyzed the beta-dispersion using ANOVA and betadisper for both species and clade level differences. SIMPER tests were also run for species and clade, using 100 permutations. Finally, a metaNMDS visualization was created using the metaMDS function in combination with ggplot2.
Co-occurrence analysis
To test patterns of co-occurrence between genera of ASVs, we used a Fisher’s exact test on the count of samples with genus A only, genus B only, genus A and B, and neither genus. Fisher exact tests were performed on ASVs collapsed by bacterial genus under the following conditions: (i) only bacterial genera found in the same Formica species and run were compared; (ii) only samples from these shared run/species combination were counted in statistical comparisons, we excluded samples from run/species combinations where only one bacteria occurred (iii) only bacterial genera found in >2 samples were considered in the analysis. A significance level of P < 0.05 was considered significant for the Fisher’s exact test. All data for each significant analysis is available in Supplementary Table 4.
Ribotype diversity visualization
To visualize the distribution of bacterial ribotypes across host species, we used 97% OTUs identified as being either Lactobacillus, Wolbachia, Spiroplasma, or Liliensternia to reconstruct a tree using the Silva ACT (Alignment, Classification and Tree) service (Pruesse et al., 2012; Quast et al., 2012; Supplementary Figure 1). The workflow used was “add to neighbours tree” with RaxML, GTR, and GAMMA as additional parameters. Minimal identity with query sequence was set to 97% and maximum matches to query sequence were 2. The complete tree is available in Supplementary Figure 1, while Figure 4 shows a pruned version of the tree. ITOL (Letunic and Bork, 2021) was used to visualize this pruned tree and the relative abundance data from the relevant OTUs.
Phylobiome creation procedure
To generate phylobiome trees (microbiome phylogenies), we followed the procedure outlined by Brooks et al. (2017) and Lim and Bordenstein (2020). Individuals belonging to the same species were collapsed into single columns in the ASV table. ASV tables containing a single symbiont of interest (e.g., Lactobacillus and Wolbachia) were also created. We then created distance-matrices using the QIIME function “diversity beta-rarefaction” for the full collapsed ASV table and the ASV table of each symbiont of interest. We used the Bray–Curtis distance metric and the upgma clustering method. Sampling depth was 3,000 and the process was repeated for 100 iterations. Phylogenies were visualized using ITOL (Letunic and Bork, 2021).
We used TreeCmp (Goluch et al., 2020) to test for congruence between the phylobiome tree and host phylogeny. The host phylogeny is from Jackson et al. (2022), which combined a previous Formica phylogeny (Romiguier et al., 2018) with new sequencing information. TreeCmp generated scores normalized (to UniFrac) Robinson-Folds Cluster and Matching Cluster scores for each tree comparison. To determine P-values for these scores we preformed the same congruence testing for 1,000 randomly generated trees. Random phylogenies were generated using T-Rex (Alix et al., 2012).
Results
Four common bacterial genera are associated with Formica ants
We used 16S rRNA ASVs, taxonomically classified at genus, to quantify the composition of the microbiome of queens from 14 Formica species from five clades (Figure 1 and Supplementary Table 1). We used this information to evaluate the presence and abundance of the known symbionts of Formica ants, Liliensternia, Wolbachia, and Lactobacillus, and to identify other commonly occurring bacterial genera.
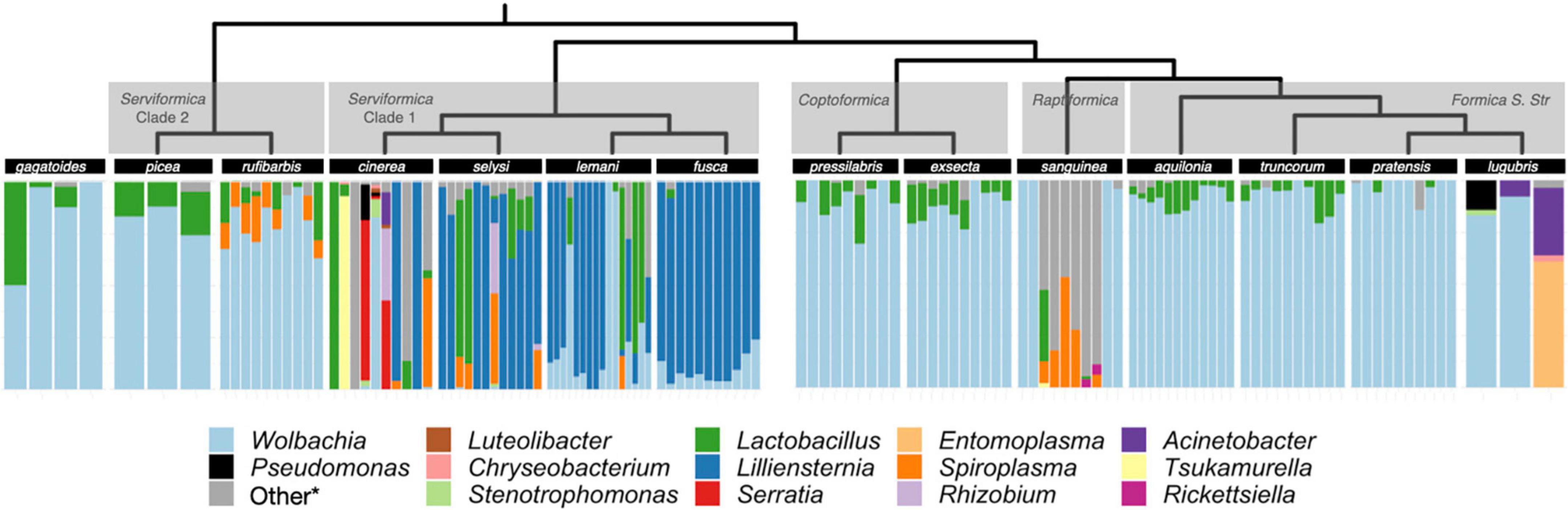
Figure 1. Microbiome composition in Formica. Each bar depicts the relative abundance of ASVs in the microbiome of a single queen. ASVs have been grouped and colored by genus. ASV’s that were found in only one sample or occurred at <10% relative abundance were collapsed into the *other category. The full ASV table containing all bacterial genera is available in Supplementary material. The Formica host phylogeny is from Jackson et al. (2022), which combined a previous Formica phylogeny (Romiguier et al., 2018) with new sequencing information from Jackson et al. (2022).
As previously reported by Jackson et al. (2022), we found that Liliensternia is restricted to a single clade of Serviformica ants, while Wolbachia is present in every species screened and the majority of queens screened overall (112/131). Wolbachia is not only widely distributed among Formica species but also highly abundant, accounting for 54% of all sequences across all queens analyzed. However, while most of the sequences came from Wolbachia in many queens, this was not the case in queens that were also infected with Liliensternia. When queens were co-infected with Liliensternia and Wolbachia, only a minority of the sequences typically came from Wolbachia.
Aside from Wolbachia, we found that two other bacterial genera commonly occur in Formica ants: Lactobacillus and Spiroplasma. Lactobacillus was taxonomically widespread, appearing in every clade of Formica. Lactobacillus occurred usually at low (<25%) relative abundance, but in several Serviformica queens, it was the dominant bacterium. In contrast, Spiroplasma was relatively uncommon, being found in only five species (Formica rufibarbis, Formica cinerea, Formica selysi, Formica lemani, and Formica sanguinea), where it typically occurred at <25% relative abundance.
Some species harbored distinct microbial associates or microbiomes (Figure 1). F. cinerea was the only species recorded to carry Serratia. F. rufibarbis was nearly always infected with Spiroplasma, although this latter host species was only collected at two sites. In F. sanguinea, there were two distinct microbiomes, as individuals had either Wolbachia-dominated microbiomes or microbiomes with Spiroplasma. The two distinct microbiomes of F. sanguinea correlated with two colonies in which this species was sampled in nature.
Most of the commonly occurring microbes in Formica, e.g., Wolbachia, Liliensternia, and Spiroplasma, typically have only a single copy of the 16S rRNA gene (Stoddard et al., 2015), so the abundance of these genera is unlikely to be a by-product of high gene copy number. In contrast, Lactobacillus can vary in copy number, ranging from 3 to 12 (Stoddard et al., 2015), so its abundance may be overestimated.
Microbiome composition across Formica species
To test whether the microbiomes of Formica had distinguishable structure across species and clades, we analyzed the composition of individual ASVs with permanova and beta-dispersion analyses. Serviformica clades 1 and 2 were analyzed separately, as they are paraphyletic (Romiguier et al., 2018). We complemented the statistical analysis with a metaNMDS visualization, to aid in interpretation of the data (Figure 2).
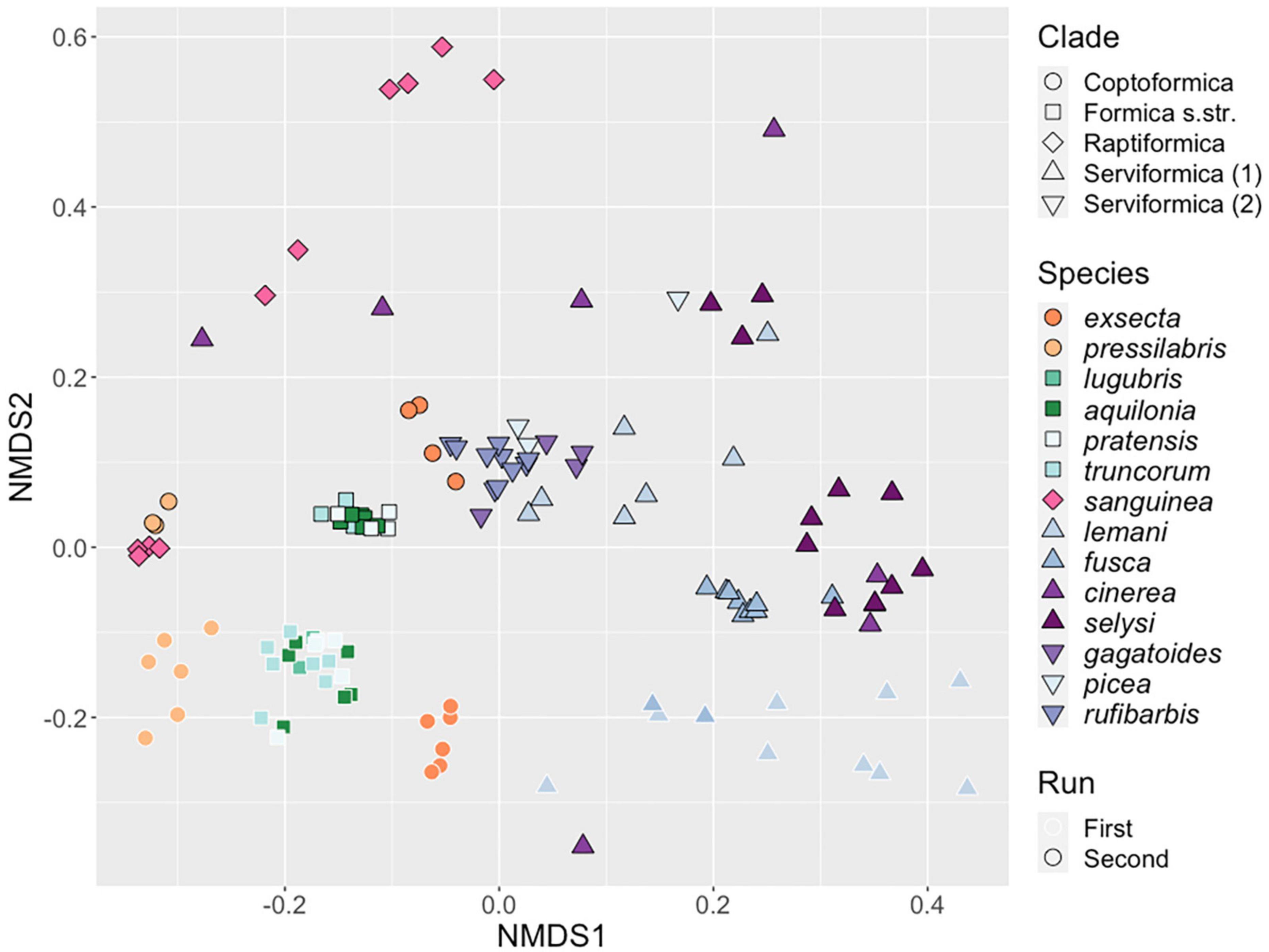
Figure 2. Similarity of microbial communities hosted by Formica queens. NMDS clustering of bacterial ASVs associated with individual Formica queens. Points clustering together represent samples with more similar microbial communities. Point shape designates clades, and colors represent different species. Points with a black outline are from the first run of 16S rRNA sequencing, points with a white outline are from the second run.
Our analysis revealed an effect of the sequencing run (Permanova: F1,128 = 17.889, P < 0.001). However, we still found highly significant differences across host clades and species when we analyzed the runs separately (by blocking), or together with run as an interactive effect (all P < 0.001, Supplementary Table 2). We also found that there were significant differences in dispersion between taxonomic groups (Clade: F4,125 = 6.15, P < 0.001, Species: F13,116 = 4.89, P < 0.001). Individuals from some species and clade, such as Formica exsecta and Formica pressilabris, showed high degrees of similarity in their microbiome profile, resulting in low group-level dispersion. In contrast, other taxonomic groups, like F. cinerea, showed much higher levels of dissimilarity in microbiome structure and dispersion (Figure 2).
We used a SIMPER test to investigate which ASVs were driving these differences between species and clades (Supplementary Table 3). Ten bacterial genera accounted for more than 1% of the variation between Formica species: Acinetobacter, Rhizobium, Lactobacillus, Pseudomonas, Serratia, Liliensternia, Spiroplasma, Stenotrophomonas, Tsukamurella, and Wolbachia. Differences between Formica clades were explained by only four of these genera: Lactobacillus, Liliensternia, Spiroplasma, and Wolbachia.
In general, Wolbachia and Liliensternia, along with the two previously identified bacterial genera of interest, Spiroplasma and Lactobacillus, accounted for most of the variation between Formica species and clades. Wolbachia accounted for an average of 47 ± 2 and 55 ± 2% of the variation in microbial genera across host species and clades, respectively (Supplementary Table 3). The other ASV genus that accounted for a large amount of variation across species, but not clades, was Liliensternia (range of 10–67% across species, mean 33%). Despite being present in the majority of species and clades, Lactobacillus accounted for a small amount of the variation (range of 1–13% across species, mean 6%). Finally, Spiroplasma accounted for >1% of the bacterial variation across species within the clades Serviformica and Raptiformica. Other bacterial genera accounted for differences between a small number of ant species.
Interactions between symbiotic bacterial genera
We hypothesized that interactions between genera of bacteria may be one of the factors contributing to the overall structure observed in Formica microbiomes. Using ASV data from each amplicon sequencing run, we evaluated whether the presence of any genus of ASV was significantly associated with the presence or absence of another genus of ASV (Figure 3, full statistics for all comparisons are available in Supplementary Table 4). This analysis was intended to reveal co-occurrences between different bacterial genera. Further investigations will be required to determine the cause of positive or negative relationships.
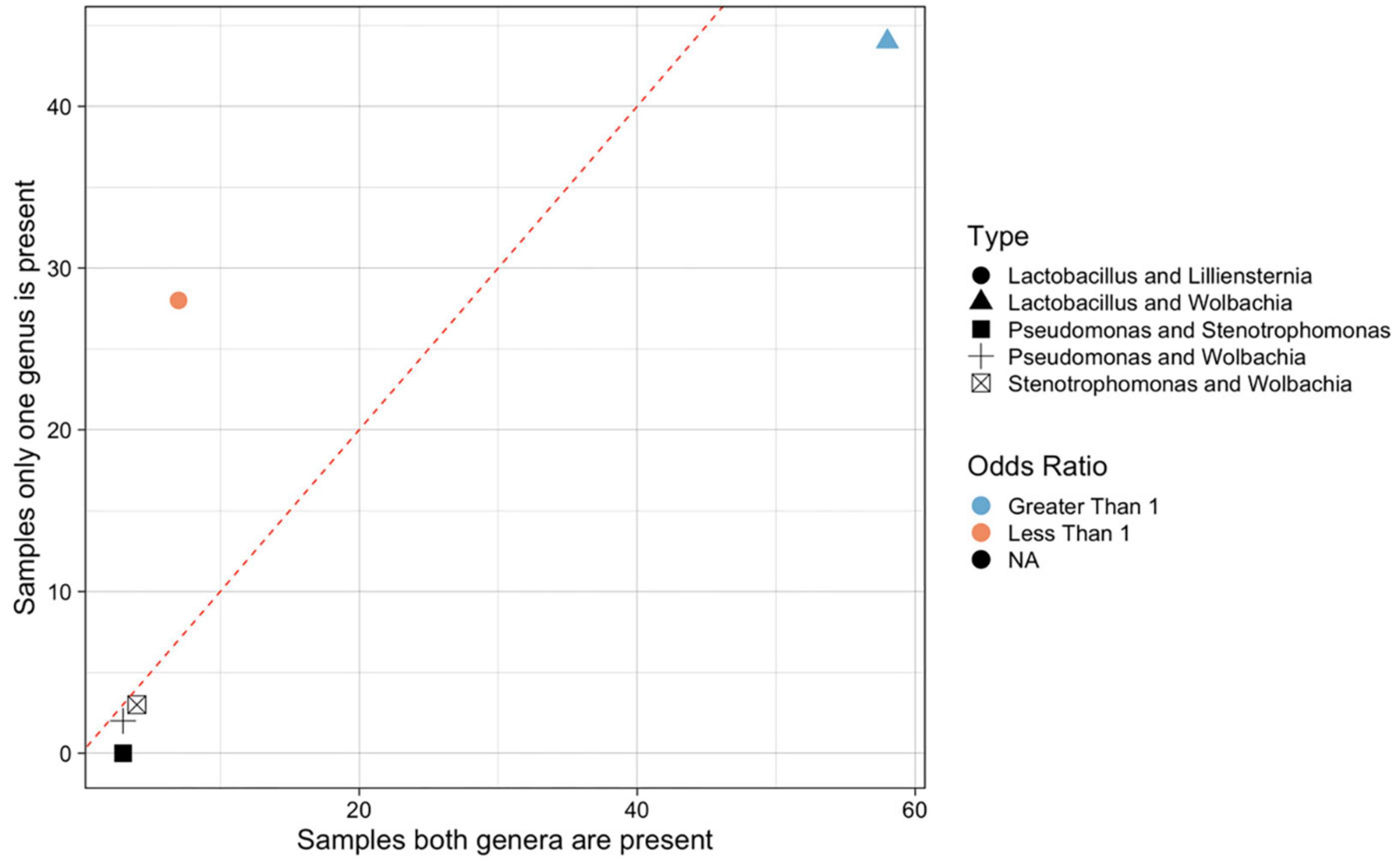
Figure 3. Significant positive and negative co-occurrences between microbes in Formica. Significantly co-occurring bacterial genera (under a Fisher’s exact test), by number of samples where both genera are present versus number of samples where only one of the two genera are present. Each microbe is represented by ASVs collapsed into taxonomic genus. Points are colored by the odds ratio calculated by the Fisher test. Odds ratio’s greater than 1 (blue) indicate significant co-occurrence, where as ratios less than 1 (red) indicate a significant lack of co-occurrence. Odds ratios are not meaningful when one of the values is zero, these points are colored black. Point shape denote different genera that shown significant correlation. Dotted line divide genera that tend to co-occur (below the line) from those that do not tend to co-occur (above the line). All data available in Supplementary Table 4.
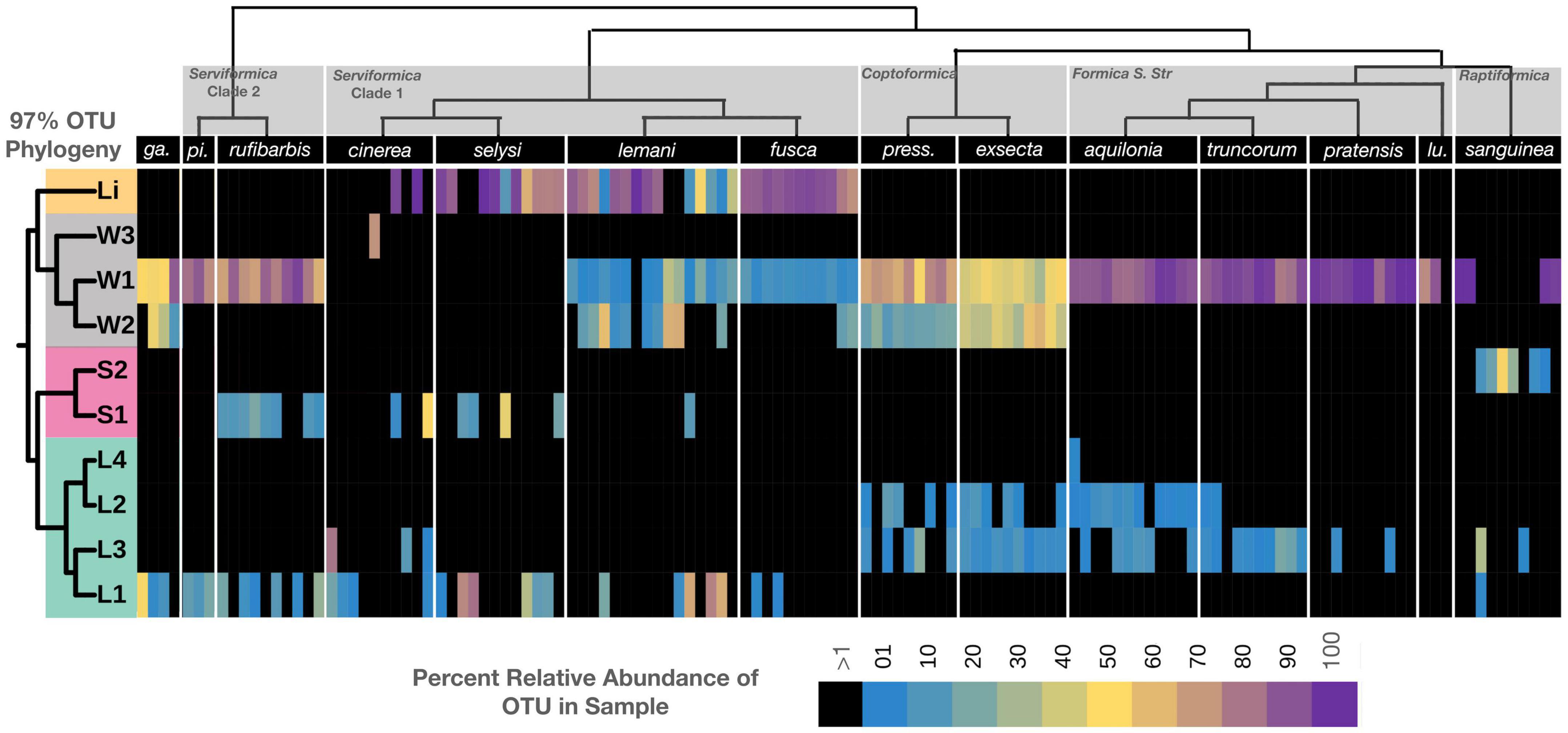
Figure 4. Bacterial ribotypes mapped on the phylogeny of Formica ants. The relative abundance of distinct lineage groups (based on 97% OTUs of the 16S rRNA gene) mapped on to the Formica phylogeny. Heatmap reflects the relative abundance of the four dominant bacterial genera found in each individual. Host and bacterial phylogeny are displayed above and to the left of the heatmap, respectively. Prefix refer to: Li, Liliensternia; W, Wolbachia; S, Spiroplasma; and L, Lactobacillus.
We found five instances of significant co-occurrences between bacterial genera. Lactobacillus and Liliensternia were negatively correlated, i.e., they were less likely to co-occur than expected by chance. In contrast, Lactobacillus and Wolbachia tended to be positively associated, and commonly co-occurred. There were also significant co-occurrences of Pseudomonas and Stenotrophomonas, Pseudomonas, and Wolbachia, and Wolbachia and Stenotrophomonas. However, these last three co-occurrences should be viewed with caution as Pseudomonas and Stenotrophomonas only occurred in a small number of samples (<5 each), so there is limited power to resolve their associations with other microbes.
Bacterial ribotypes are non-randomly distributed across Formica species
After identifying Liliensternia, Wolbachia, Lactobacillus, and Spiroplasma as being the dominant bacterial genera composing Formica microbiomes, we plotted the distribution of bacterial ribotypes across host species. The clustering of bacterial ASVs into 97% OTUs resulted in one Liliensternia, three Wolbachia, two Spiroplasma, and four Lactobacillus lineages (Figure 4). Further metagenomic analysis using additional genetic markers, or those with longer read lengths, would help clarify strain-level details and test for more fine-scale patterns of phylosymbiosis.
The most dominant and widespread lineage was Wolbachia ribotype W1, which was found in every Formica species except F. cinerea and F. selysi, often at over 50% relative abundance. In contrast, Wolbachia ribotype W2 was found only in Coptoformica and Serviformica species. Wolbachia ribotype W2 was dominant in F. exsecta, whereas ribotype W3 appeared only in one F. cinerea individual.
The most widespread bacterial genus after Wolbachia was Lactobacillus. Lactobacillus Ribotype L2 was found exclusively in Coptoformica and Formica S. Str. species, while L3 was predominantly found in the same species as L2, plus F. sanguinea. Lactobacillus L1 was identified in species belonging to the Serviformica clades 1 and 2, as well as in one F. sanguinea individual, whereas L4 was only found in a single Formica aquilonia individual.
There were two unique Spiroplasma ribotypes, one found in some Serviformica species and the other in F. sanguinea. A single ribotype associated with Liliensternia was found in one clade of Serviformica, as previously reported (Jackson et al., 2022), and in a single Formica pratensis individual.
Within each bacterial genus, each ribotype tended to be associated with a restricted number of Formica clades, and in several cases more than one ribotype was present in the same individual. Wolbachia lineage W1 stood out from this pattern in that it appeared across all host clades, although it still often co-occurred with other ribotypes.
Phylosymbiosis in Formica ants
Given the strong structure of microbial ASVs and ribotypes associated with different Formica species and clades, we tested whether microbiome composition mirrors the phylogeny of Formica species, i.e., phylosymbiosis. We also tested whether individual bacterial genera showed signs of phylogenetic structure across hosts. Using the protocol established by Brooks et al. (2017), we generated trees based on the differences in abundance of each unique ASV across the microbiome of each Formica species (Figure 5) and compared these to the host phylogeny using Robinson–Folds clustering, as well as unadjusted and normalized Matching Cluster metrics.
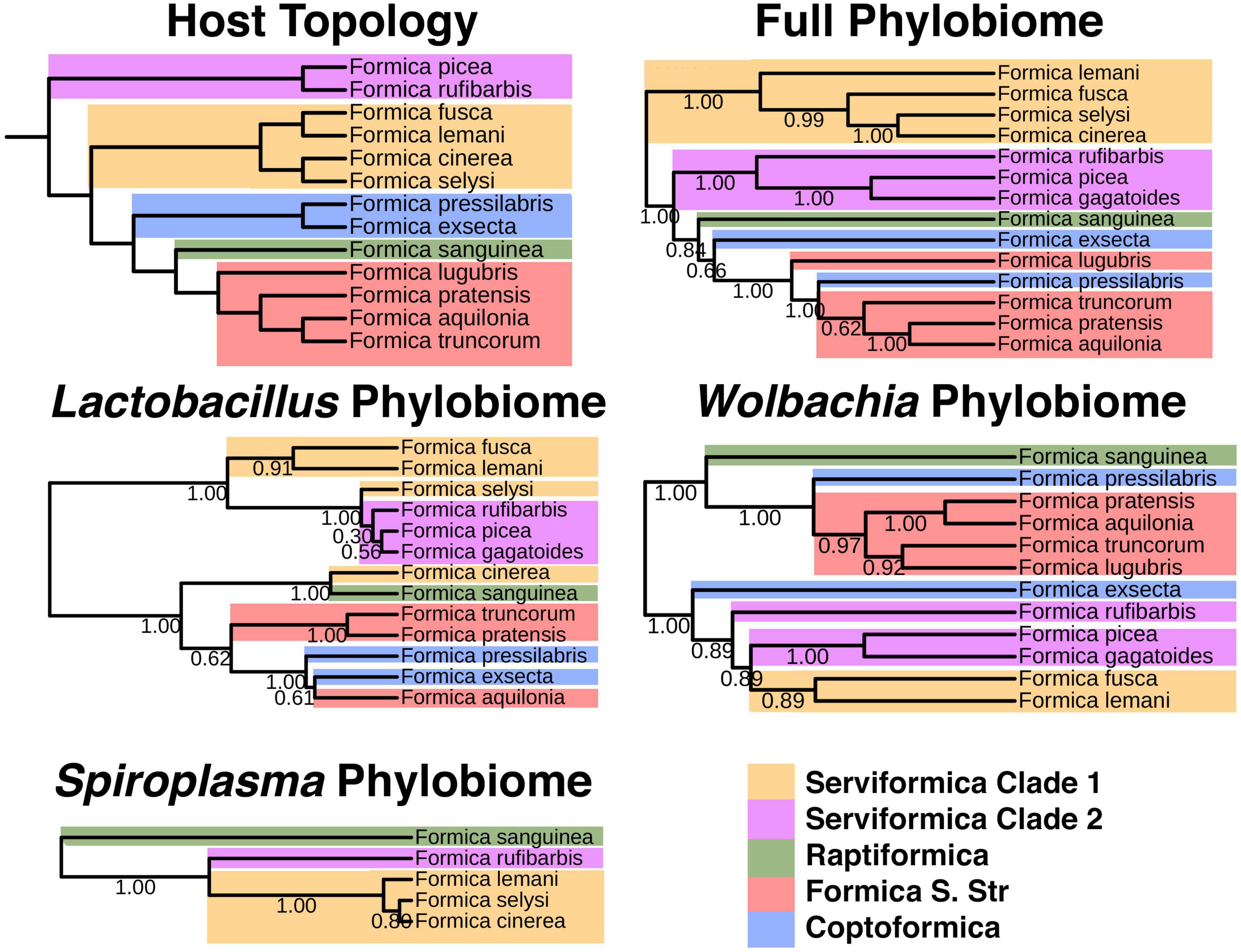
Figure 5. Host phylogeny versus Phylobiomic signatures of Formica species. Bootstrap values on bacterial phylogenies indicate proportion out of 100 iterations of subsampling within microbiome data resulted in the given placement of branches. The bacterial phylogeny was generated using bacterial ASVs and the protocol established by Brooks et al. (2017).
All phylobiome trees were highly congruent with the host tree (P < 0.01; Supplementary Table 5). In addition to significantly corresponding to the host tree, we documented two interesting patterns when comparing phylobiome trees (Figure 5). First, the paraphyletic nature of Serviformica species was mirrored in all phylobiome trees. We expected this pattern in the full phylobiome tree, because one clade of Serviformica carries Liliensternia, a strictly vertically transmitted symbiont whose phylogeny mirrors that of its host (Jackson et al., 2022). However, even when we considered trees of the single bacterial genera Spiroplasma, Wolbachia, and Lactobacillus, the paraphyletic pattern was still apparent (Figure 5). Second, the position of Coptoformica species (F. exsecta and F. pressilabris) was not consistent across phylobiome trees (Figure 5).
Discussion
Components of a highly structured microbiome in Formica ants
Our investigation has revealed that Formica ants contain highly structured stable microbiomes that recapitulate the phylogeny of their host. The structure is principally due to the prevalence and distribution of four genera of bacteria: the maternally transmitted microbes Wolbachia, Liliensternia, and Spiroplasma, as well as the gut bacteria Lactobacillus. Of the four, Wolbachia, Liliensternia, and Lactobacillus have been previously identified as symbionts of Formica (Russell, 2012; Zheng et al., 2021; Jackson et al., 2022), whereas we have newly identified Spiroplasma as a common microbe associated with several Formica species, suggesting it may be symbiotic in nature. The most dominant microbes in terms of relative abundance were Wolbachia and Liliensternia, a pattern likely explained by the fact that we analyzed adult queens. Queens have large reproductive tissues, in which Wolbachia and Liliensternia are maintained (Moran et al., 2008; Frost et al., 2014; Jackson et al., 2022).
Wolbachia, the most abundant bacterium across Formica queens, is a common heritable reproductive manipulator found in a wide range of insects (Zug and Hammerstein, 2012). It is also found in many ant species, although it is unclear to what extent it behaves as a reproductive manipulator in ants (Keller et al., 2001; Russell, 2012). The frequency of Wolbachia infection within Formica species is particularly high compared to other ants and insects (Russell, 2012). Our results are consistent with previous measurements of Wolbachia infection in Formica species, which indicated that over 80% of individuals were infected (Russell, 2012; Russell et al., 2012). In Formica truncorum, Wolbachia is close to fixation, and heavily infected colonies produced significantly fewer sexual castes, suggesting the microbe has a deleterious effect in this species, despite its prevalence (Wenseleers et al., 2002). One factor that has been proposed to contribute to the varying success of Wolbachia in ants is variation in effective population size, where species with small effective population sizes resulting from limited queen dispersal or dependent colony founding have higher rates of Wolbachia infection (Wenseleers et al., 1998; Treanor and Hughes, 2019). However, we observe high rates of Wolbachia infection across all Formica species, which included those with different colony founding strategies, queen dispersal strategies, and lifestyles.
Lactobacillus has been described in multiple ant species (Anderson et al., 2012), including F. exsecta (Johansson et al., 2013; Zheng et al., 2021). The contribution of Lactobacillus to the structured microbiomes of Formica ants pairs well with recent findings that ants that frequently feed on aphid honeydew, such as Formica, commonly carry lactic acid bacteria that help catabolize sugars known to be found in the sugary excretion (Engel et al., 2012; Zheng et al., 2021). However, it was surprising that it appeared to contribute to inter-clade and inter-species differentiation of Formica microbiomes. Lactobacillus is typically a gut-associated microbe (Zheng et al., 2021) and is not known to be ovarially transmitted. As a result, phylogenetic signal deriving from Lactobacillus strains is not likely to be driven by inheritance, unless long term vertical transmission within or between colonies has been sustained through trophallaxis, such as in Cephalotus ants (Ramalho and Moreau, 2023). Additionally, it is not expected for all ants to possess stable gut microbiomes, as many species appear to exist devoid of an appreciable gut bacterial community (Sanders et al., 2017). Studies have shown that Lactobacillus can serve as a defensive or nutritional symbiont in Drosophila and in honeybees (Forsgren et al., 2010; Storelli et al., 2011; Vásquez et al., 2012). If Lactobacillus served a similar role in Formica, it might explain why it has established a stable presence across species, as previously proposed by Zheng et al. (2021).
Spiroplasma is a common heritable insect endosymbiont that is often pathogenic and known for its ability to manipulate the reproduction of its host (Majerus et al., 1999; Jiggins et al., 2000). However, studies have also shown that Spiroplasma can benefit insects, by defending against pathogens such as fungi (Łukasik et al., 2013). Spiroplasma has been detected in several ant species (Ishak et al., 2011; Ballinger et al., 2018). In Solenopsis and Myrmica ants, Spiroplasma is found in nearly all individuals, suggesting it may play a beneficial role for its host (Ishak et al., 2011; Ballinger et al., 2018). The role of Spiroplasma in Formica ants is currently unclear. As Spiroplasma was very common in F. rufibarbis and F. sanguinea – found at high frequencies in 10 colonies from 5 sites across Finland – it would be interesting to know whether a parasitic, or potentially a beneficial relationship, explains its prevalence in these two host species.
Previous studies have shown that Liliensternia is housed in bacteriocytes that surround the midgut (Lilienstern, 1932; Jackson et al., 2022), is strictly vertically inherited from the common ancestor in Serviformica clade 1, and has retained the pathways to synthesize certain amino acids and vitamins, suggesting it is a beneficial symbiosis (Lilienstern, 1932; Jackson et al., 2020, 2022). This explains the overwhelming presence of Liliensternia in this clade of ants. Normally, a bacteriocyte-associated symbiont would be present in all reproductive females. However, the curious feature that Liliensternia is being lost in some queens, especially in species such as F. cinerea (Jackson et al., 2022), explains why it is not universally found in queens within this clade of hosts.
Synergy and antagonism in the microbiome
Our analysis of pairs of bacterial genera across Formica microbiomes revealed both positive and negative associations between microbes. Some of these correlations indicated that there may be some underlying synergism or antagonism between microbes. For example, we found a positive relationship between Wolbachia and Lactobacillus. This was unexpected, as these two symbionts are likely localized in different parts of the insect and are presumed to have different routes of transmission – ovarial versus environmental (Ramalho et al., 2018; Zheng et al., 2021), so there would be fewer opportunities for the microbes to interact. It may be that an indirect interaction mediated through the host leads to the positive association. However, at present it is unclear how this type of interaction would occur. We also found that Liliensternia does not tend to co-occur with Lactobacillus. This was intriguing, as Lactobacillus is known to behave as a nutritional mutualist in some other insect species (Storelli et al., 2011). It may be that Lactobacillus does not tend to co-occur with Liliensternia because the host only needs one symbiont to take on the nutrient provisioning role. It may also be that hosting a bacteriocyte-associated symbiont strengthens the insects immune response making it less hospitable to other microbes as observed in Blochmannia containing Camponotus ants (De Souza et al., 2009).
Phylosymbiosis in Formica ants
Our analyses revealed that Formica microbiomes show evidence of phylosymbiosis, in that the bacterial community composition mirrors the phylogeny of the host (Lim and Bordenstein, 2020). A wide range of mechanisms can lead to phylosymbiosis, including vertical transmission of microbes, interactions between microbes, host-microbe genetic compatibility, or the similarities in host ecologies that impact the microbes they carry (e.g., diets). In Formica ants, several life history traits correlate with host phylogeny (Borowiec et al., 2020), including colony founding method and mound building style. As a result, it is not possible to fully disentangle to which extent similarities in the ecologies, or the phylogenetic proximity of Formica species, contribute to the microbes they carry. However, our results suggest that host phylogenetic relatedness, in combination with vertical transmission, may play important roles.
We have shown that Formica microbiomes are largely dominated by vertically transmitted microbes. This includes Liliensternia, which is believed to be an ancient strictly vertically transmitted symbiont that has co-speciated with its host (Jackson et al., 2022), and undoubtedly contributes to the phylosymbiosis signal. However, even when Liliensternia was not considered in the analysis – as is the case in the Lactobacillus, Wolbachia, and Spiroplasma phylobiome trees – Formica still show signals of phylosymbiosis, demonstrating that the pattern is not solely driven by the presence of this nutritional mutualist. Phylogenetic studies of Wolbachia and Spiroplasma have shown that although both microbes are maternally transmitted, they are often horizontally transferred between host lineages on evolutionary time scales, and therefore typically do not co-speciate with their hosts (e.g., Viljakainen et al., 2008; Ahmed et al., 2016). There are a few instances where co-diversification has been observed, for example between Spiroplasma and Myrmica ants, and between Wolbachia in both filarial nematodes and bedbugs (Fenn and Blaxter, 2004; Ballinger et al., 2018; Balvín et al., 2018). The widespread occurrence of the same Wolbachia and Spiroplasma ribotypes (e.g., W1 and S1) in distantly related Formica species found in our data, and in a separate study on Wolbachia in Formica (Viljakainen et al., 2008), indicates that horizontal transfer between species is relatively common on evolutionary time scales. Therefore, vertical inheritance from a common ancestor is unlikely to have led to the observed pattern of phylosymbiosis. The high frequency of specific Wolbachia strains in certain Formica species is therefore more likely the product of host specific factors, e.g., genetic compatibilities of Wolbachia strains with related hosts increasing the likelihood that the symbiont is horizontally transferred between related species, with vertical transmission helping to maintain the relationship within populations and species.
The life-history traits of Formica also provide some capacity to tease apart the importance of host ecology and phylogeny in structuring their microbiomes. All of the Formica ant species studied, except those in the Serviformica clades, have lost the ability to found their colonies independently, and rely partly or completely on socially parasitic strategies for colony founding (Romiguier et al., 2018; Borowiec et al., 2021). This means they take over colonies of other ants, specifically those in the Serviformica clades, to found new colonies (temporary social parasitism). Additionally, F. sanguinea facultatively raids workers from Serviformica species to complement their own worker force. As a result, different clades of Formica ants come into close association with each other regularly. However, despite coming into close physical proximity with other species – living within the same colony and feeding other species via trophallaxis – we still find that socially parasitic species and the species they parasitize have distinct microbial profiles. This indicates that environmental acquisition or transfer between unrelated species in close physical proximity does not play a significant role in structuring the microbiomes of Formica ants. Given this, we hypothesize that phylogenetic relatedness of Formica species plays a more substantive role in the retention of microbial species, and the overall similarity of microbiomes between related host species found in our study. Studies have shown that the horizontal transfer of heritable intracellular symbionts is more likely to occur between closely related species (Łukasik et al., 2015; McLean et al., 2019). Furthermore, recent studies on aphids have shown that co-adaptation of facultative symbionts to different hosts, and their specific ecologies, is largely responsible for the distribution of symbiont genotypes across host species (Henry et al., 2022; Wu et al., 2022). However, we cannot exclude that similarities in host ecology may also play a role in the phylosymbiotic signal we find in Formica. Recent studies on Formica and Lasius ants demonstrated that Lactobacillus was more common in ants that feed on aphid honeydew (Zheng et al., 2021). Formica ants commonly tend aphids for honeydew and species may differ in their reliance on aphids for this resource (Fiedler et al., 2007). It is possible that the prevalence of Lactobacillus, and its contribution to the phylosymbiotic signal may be partially explained by shared dietary ecologies, if for example related Formica species have similar tendencies to feed on honeydew as a food source.
Conclusion
We have shown that Formica ants have formed stable enduring relationships with a core set of microbes that are dominated by four genera of bacteria. When placed in a phylogenetic context, we reveal that the composition of microbes carried by different Formica species recapitulates the phylogeny of the host. For the bacteriocyte-associated Liliensternia, it is believed that the association between host and microbe is perpetuated by a mutualistic benefit, with vertical transmission explaining its occurrence in related species. For the other dominant microbes, we hypothesize that the genetic proximity among microbes and among hosts, respectively, and possibly host feeding ecology, may explain the communities of microbes associated with Formica ants. The relationship with Lactobacillus and Formica may also be mutualistic in nature, by helping their host digest honeydew (Engel et al., 2012; Zheng et al., 2021), which is an important food source for many Formica species. It is less clear, however, why many Formica species share a strong, near ubiquitous, association with Wolbachia. Future studies should investigate whether Wolbachia’s high prevalence in Formica reflects a very successful pathogen, or whether the ants have evolved some form of dependence on the microbe (Comandatore et al., 2015), which may help explain its prevalence across host species.
Data availability statement
The datasets presented in this study can be found in online repositories. The names of the repository/repositories and accession number(s) can be found below: NCBI – PRJNA639935 (SRR14246076 - SRR14246279).
Ethics statement
None of the species included in our study are considered threatened or near-threatened. Collection permits are not required for collecting Formica ants in Finland, and the Swiss ants were collected under authorization from the Service des forêts, des cours d’eau et du paysage du Canton du Valais.
Author contributions
RJ contributed to the conception and design of the study, analyzed the data, and contributed to writing the manuscript. PP, GG, and CE conducted the lab work and initially analyzed the data. HH and MC contributed to the samples. LH contributed to the conception and design of the study, helped write the manuscript, supervised, and funded the study. All authors contributed to manuscript revision, read, and approved the submitted version.
Funding
This work was funded by LMH’s NERC IRF (NE/M018016/1).
Acknowledgments
We thank Dominic Burns for providing ant samples.
Conflict of interest
The authors declare that the research was conducted in the absence of any commercial or financial relationships that could be construed as a potential conflict of interest.
Publisher’s note
All claims expressed in this article are solely those of the authors and do not necessarily represent those of their affiliated organizations, or those of the publisher, the editors and the reviewers. Any product that may be evaluated in this article, or claim that may be made by its manufacturer, is not guaranteed or endorsed by the publisher.
Supplementary material
The Supplementary Material for this article can be found online at: https://www.frontiersin.org/articles/10.3389/fmicb.2023.1044286/full#supplementary-material
References
Ahmed, M. Z., Breinholt, J. W., and Kawahara, A. Y. (2016). Evidence for common horizontal transmission of Wolbachia among butterflies and moths. BMC Evol. Biol. 16:118. doi: 10.1186/s12862-016-0660-x
Alix, B., Boubacar, D. A., and Vladimir, M. (2012). T-REX: A web server for inferring, validating and visualizing phylogenetic trees and networks. Nucleic Acids Res. 40, 573–579. doi: 10.1093/nar/gks485
Anderson, K. E., Russell, J. A., Moreau, C. S., Kautz, S., Sullam, K. E., Hu, Y., et al. (2012). Highly similar microbial communities are shared among related and trophically similar ant species. Mol. Ecol. 21, 2282–2296. doi: 10.1111/j.1365-294X.2011.05464.x
Ballinger, M. J., Moore, L. D., and Perlman, S. J. (2018). Evolution and diversity of inherited Spiroplasma symbionts in Myrmica ants. Appl. Environ. Microbiol. 84, e2299–e2217. doi: 10.1128/AEM.02299-17
Balvín, O., Roth, S., Talbot, B., and Reinhardt, K. (2018). Co-speciation in bedbug Wolbachia parallel the pattern in nematode hosts. Sci. Rep. 8:8797. doi: 10.1038/s41598-018-25545-y
Bennett, G. M., and Moran, N. A. (2015). Heritable symbiosis: The advantages and perils of an evolutionary rabbit hole. Proc. Natl. Acad. Sci. U.S.A. 112, 10169–10176. doi: 10.1073/pnas.1421388112
Bolger, A. M., Lohse, M., and Usadel, B. (2014). Trimmomatic: A flexible trimmer for illumina sequence data. Bioinformatics 30, 2114–2120. doi: 10.1093/bioinformatics/btu170
Bolyen, E., Rideout, J. R., Dillon, M. R., Bokulich, N. A., Abnet, C. C., Al-Ghalith, G. A., et al. (2019). Reproducible, interactive, scalable and extensible microbiome data science using QIIME 2. Nat. Biotechnol. 37, 852–857. doi: 10.1038/s41587-019-0209-9
Borowiec, M. L., Cover, S. P., and Rabeling, C. (2020). The evolution of social parasitism in Formica ants revealed by a global phylogeny. bioRxiv [Preprint]. doi: 10.1101/2020.12.17.423324
Borowiec, M. L., Cover, S. P., and Rabeling, C. (2021). The evolution of social parasitism in Formica ants revealed by a global phylogeny. Proc. Natl. Acad. Sci. U.S.A. 118:e2026029118. doi: 10.1073/pnas.2026029118
Brooks, A. W., Kohl, K. D., Brucker, R. M., van Opstal, E. J., and Bordenstein, S. R. (2017). Correction: Phylosymbiosis: Relationships and functional effects of microbial communities across host evolutionary history. PLoS Biol. 15:e1002587. doi: 10.1371/journal.pbio.1002587
Callahan, B. J., McMurdie, P. J., Rosen, M. J., Han, A. W., Johnson, A. J. A., and Holmes, S. P. (2016). DADA2: High-resolution sample inference from illumina amplicon data. Nat Methods 13, 581–583. doi: 10.1038/nmeth.3869
Caporaso, J. G., Lauber, C. L., Walters, W. A., Berg-Lyons, D., Lozupone, C. A., Turnbaugh, P. J., et al. (2011). Global patterns of 16S rRNA diversity at a depth of millions of sequences per sample. Proc. Natl. Acad. Sci. U.S.A. 108, 4516–4522. doi: 10.1073/pnas.1000080107
Comandatore, F., Cordaux, R., Bandi, C., Blaxter, M., Darby, A., Makepeace, B. L., et al. (2015). Supergroup C Wolbachia, mutualist symbionts of filarial nematodes, have a distinct genome structure. Open Biol. 5:150099. doi: 10.1098/rsob.150099
De Souza, D. J., Bezier, A., Depoix, D., Drezen, J., and Lenoir, A. (2009). Blochmannia endosymbiont improves colony growth and immune defense in the ant Camponotus fellah. BMC Microbiol. 9:29. doi: 10.1186/1471-2180-9-29
Degnan, P. H., Lazarus, A. B., and Wernegreen, J. J. (2002). Small genome of Candidatus Blochmannia, the bacterial endosymbiont of Camponotus, implies irreversible specialization to an intracellular lifestyle. Microbiology 148, 2551–2556. doi: 10.1099/00221287-148-8-2551
Engel, P., Martinson, V. G., and Moran, N. A. (2012). Functional diversity within the simple gut microbiota of the honey bee. Proc. Natl. Acad. Sci. U.S.A. 109, 11002–11007. doi: 10.1073/pnas.1202970109
Feldhaar, H., Straka, J., Krischke, M., Berthold, K., Stoll, S., Mueller, M. J., et al. (2007). Nutritional upgrading for omnivorous carpenter ants by the endosymbiont Blochmannia. BMC Biol. 5:48. doi: 10.1186/1741-7007-5-48
Fenn, K., and Blaxter, M. (2004). Are filarial nematode Wolbachia obligate mutualist symbionts? Trends Ecol. Evol. 19, 163–166. doi: 10.1016/j.tree.2004.01.002
Fiedler, K., Kuhlmann, F., Schlick-Steiner, B. C., Steiner, F. M., and Gebauer, G. (2007). Stable N-isotope signatures of central European ants - Assessing positions in a trophic gradient. Insectes Soc. 54, 393–402. doi: 10.1007/s00040-007-0959-0
Forsgren, E., Olofsson, T. C., Vásquez, A., and Fries, I. (2010). Novel lactic acid bacteria inhibiting Paenibacillus larvae in honey bee larvae. Apidologie 41, 99–108. doi: 10.1051/apido/2009065
Frost, C. L., Pollock, S. W., Smith, J. E., and Hughes, W. O. H. (2014). Wolbachia in the flesh: Symbiont intensities in germ-line and somatic tissues challenge the conventional view of Wolbachia transmission routes. PLoS One 9:e95122. doi: 10.1371/journal.pone.0095122
Goluch, T., Bogdanowicz, D., and Giaro, K. (2020). Visual TreeCmp: Comprehensive comparison of phylogenetic trees on the web. Methods Ecol Evol 11, 494–499. doi: 10.1111/2041-210X.13358
Graber, L. C., Ramalho, M. O., Powell, S., and Moreau, C. S. (2022). Identifying the role of elevation, geography, and species identity in structuring turtle ant (Cephalotes Latreille, 1802) bacterial communities. Microb. Ecol. 1–14. doi: 10.1007/s00248-022-02128-z
Henry, Y., Brechbühler, E., and Vorburger, C. (2022). Gated communities: Inter- and intraspecific diversity of endosymbionts across four sympatric aphid species. Front. Ecol. Evol. 10, 1–7. doi: 10.3389/fevo.2022.816184
Horak, R. D., Leonard, S. P., and Moran, N. A. (2020). Symbionts shape host innate immunity in honeybees: Symbionts shape honey bee immunity. Proc. Biol. Sci. 287:20201184. doi: 10.1098/rspb.2020.1184
Hu, Y., Sanders, J. G., Łukasik, P., D’Amelio, C. L., Millar, J. S., Vann, D. R., et al. (2018). Herbivorous turtle ants obtain essential nutrients from a conserved nitrogen-recycling gut microbiome. Nat. Commun. 9:964. doi: 10.1038/s41467-018-03357-y
Ishak, H. D., Plowes, R., Sen, R., Kellner, K., Meyer, E., Estrada, D. A., et al. (2011). Bacterial diversity in Solenopsis invicta and Solenopsis geminata ant colonies characterized by 16s amplicon 454 pyrosequencing. Microb. Ecol. 61, 821–831. doi: 10.1007/s00248-010-9793-4
Jackson, R., Henry, L. M., and Wurm, Y. (2020). Evolution: The legacy of endosymbiosis in ants. Curr. Biol. 30, R1385-R1387. doi: 10.1016/j.cub.2020.09.023
Jackson, R., Monnin, D., Patapiou, P. A., Golding, G., Helanterä, H., Oettler, J., et al. (2022). Convergent evolution of a labile nutritional symbiosis in ants. ISME J. 16, 2114–2122. doi: 10.1038/s41396-022-01256-1
Jiggins, F. M., Hurst, G. D., Jiggins, C. D., v d Schulenburg, J. H., and Majerus, M. E. (2000). The butterfly Danaus chrysippus is infected by a male-killing Spiroplasma bacterium. Parasitology 120, 439–446. doi: 10.1017/s0031182099005867
Johansson, H., Dhaygude, K., Lindström, S., Helanterä, H., Sundström, L., and Trontti, K. (2013). A metatranscriptomic approach to the identification of microbiota associated with the ant Formica exsecta. PLoS One 8:e79777. doi: 10.1371/journal.pone.0079777
Keller, L., Liautard, C., Reuter, M., Brown, W. D., Sundström, L., and Chapuisat, M. (2001). Sex ratio and Wolbachia infection in the ant formica exsecta. Heredity 87, 227–233. doi: 10.1046/j.1365-2540.2001.00918.x
Klein, A., Schrader, L., Gil, R., Manzano-Marín, A., Flórez, L., Wheeler, D., et al. (2016). A novel intracellular mutualistic bacterium in the invasive ant cardiocondyla obscurior. ISME J. 10, 376–388. doi: 10.1038/ismej.2015.119
Letunic, I., and Bork, P. (2021). Interactive tree of life (iTOL) v5: An online tool for phylogenetic tree display and annotation. Nucleic Acids Res. 49, W293–W296. doi: 10.1093/nar/gkab301
Lim, S. J., and Bordenstein, S. R. (2020). An introduction to phylosymbiosis. Proc. Soc. Biol. Sci. 287:20192900. doi: 10.1098/rspb.2019.2900
Łukasik, P., Guo, H., van Asch, M., Henry, L. M., Godfray, H. C. J., and Ferrari, J. (2015). Horizontal transfer of facultative endosymbionts is limited by host relatedness. Evolution 69, 2757–2766. doi: 10.1111/evo.12767
Łukasik, P., van Asch, M., Guo, H., Ferrari, J., Charles, J., and Godfray, H. (2013). Unrelated facultative endosymbionts protect aphids against a fungal pathogen. Ecol. Lett. 16, 214–218. doi: 10.1111/ele.12031
Majerus, T. M. O., von der Schulenburg, J. H. G., Majerus, M. E. N., and Hurst, G. D. D. (1999). Molecular identification of a male-killing agent in the ladybird Harmonia axyridis (Pallas) (Coleoptera: Coccinellidae). Insect Mol. Biol. 8, 551–555. doi: 10.1046/j.1365-2583.1999.00151.x
Martins, C., and Moreau, C. S. (2020). Influence of host phylogeny, geographical location and seed harvesting diet on the bacterial community of globally distributed Pheidole ants. Peer J 8:e8492. doi: 10.7717/peerj.8492
McLean, A. H. C., Godfray, H. C. J., Ellers, J., and Henry, L. M. (2019). Host relatedness influences the composition of aphid microbiomes. Environ. Microbiol. Rep. 11, 808–816. doi: 10.1111/1758-2229.12795
Miller, W. J., Ehrman, L., and Schneider, D. (2010). Infectious speciation revisited: Impact of symbiont-depletion on female fitness and mating behavior of Drosophila paulistorum. PLoS Pathog. 6:e1001214. doi: 10.1371/journal.ppat.1001214
Moran, N. A., McCutcheon, J. P., and Nakabachi, A. (2008). Genomics and evolution of heritable bacterial symbionts. Annu. Rev. Genet. 42, 165–190. doi: 10.1146/annurev.genet.41.110306.130119
Oksanen, A. J., Blanchet, F. G., Friendly, M., Kindt, R., Legendre, P., Mcglinn, D., et al. (2013). Package ‘ Vegan. Vegan: Community Ecology Package, 0–291.
Pruesse, E., Peplies, J., and Glöckner, F. O. (2012). SINA: Accurate high-throughput multiple sequence alignment of ribosomal RNA genes. Bioinformatics 28, 1823–1829. doi: 10.1093/bioinformatics/bts252
Quast, C., Pruesse, E., Yilmaz, P., Gerken, J., Schweer, T., Yarza, P., et al. (2012). The SILVA ribosomal RNA gene database project: Improved data processing and web-based tools. Nucleic Acids Res. 41, D590–D596.
Quast, C., Pruesse, E., Yilmaz, P., Gerken, J., Schweer, T., Yarza, P., et al. (2013). The SILVA ribosomal RNA gene database project: Improved data processing and web-based tools. Nucleic Acids Res. 41, D590–D596.
Ramalho, M. O., Vieira, A. S., Pereira, M. C., Moreau, C. S., and Bueno, O. C. (2018). Transovarian transmission of Blochmannia and Wolbachia endosymbionts in the neotropical weaver ant camponotus textor (Hymenoptera, Formicidae). Curr. Microbiol. 75, 866–873. doi: 10.1007/s00284-018-1459-3
Ramalho, M. O., and Moreau, S. C. (2023). Untangling the complex interactions between turtle ants and their microbial partners. Anim. Microbiome. 5:1. doi: 10.1186/s42523-022-00223-7
Rognes, T., Flouri, T., Nichols, B., Quince, C., and Mahé, F. (2016). VSEARCH: A versatile open source tool for metagenomics. PeerJ 2016, 1–22. doi: 10.7717/peerj.2584
Romiguier, J., Rolland, J., Morandin, C., and Keller, L. (2018). Phylogenomics of palearctic Formica species suggests a single origin of temporary parasitism and gives insights to the evolutionary pathway toward slave-making behaviour. BMC Evol. Biol. 18:40. doi: 10.1186/s12862-018-1159-4
Russell, J. A. (2012). The ants (Hymenoptera: Formicidae) are unique and enigmatic hosts of prevalent Wolbachia (Alphaproteobacteria) symbionts. Myrmecol News 16, 7–23.
Russell, J. A., Funaro, C. F., Giraldo, Y. M., Goldman-Huertas, B., Suh, D., Kronauer, D. J. C., et al. (2012). A veritable menagerie of heritable bacteria from ants, butterflies, and beyond: Broad molecular surveys and a systematic review. PLoS One 7:e51027. doi: 10.1371/journal.pone.0051027
Russell, J. A., Moreau, C. S., Goldman-Huertas, B., Fujiwara, M., Lohman, D. J., and Pierce, N. E. (2009). Bacterial gut symbionts are tightly linked with the evolution of herbivory in ants. Proc. Natl. Acad. Sci. U.S.A. 106, 21236–21241. doi: 10.1073/pnas.0907926106
Sanders, J. G., Lukasik, P., Frederickson, M. E., Russell, J. A., Koga, R., Knight, R., et al. (2017). Dramatic differences in gut bacterial densities correlate with diet and habitat in rainforest ants. Integr. Comp. Biol. 57, 705–722. doi: 10.1093/icb/icx088
Singh, R., and Linksvayer, T. A. (2020). Wolbachia-infected ant colonies have increased reproductive investment and an accelerated life cycle. J. Exp. Biol. 223:jeb220079. doi: 10.1242/jeb.220079
Stoddard, S. F., Smith, B. J., Hein, R., Roller, B. R. K., and Schmidt, T. M. (2015). rrnDB: Improved tools for interpreting rRNA gene abundance in bacteria and archaea and a new foundation for future development. Nucleic Acids Res. 43, D593–D598. doi: 10.1093/nar/gku1201
Storelli, G., Defaye, A., Erkosar, B., Hols, P., Royet, J., and Leulier, F. (2011). Lactobacillus plantarum promotes Drosophila systemic growth by modulating hormonal signals through TOR-dependent nutrient sensing. Cell Metab. 14, 403–414. doi: 10.1016/j.cmet.2011.07.012
Treanor, D., and Hughes, W. O. H. (2019). Limited female dispersal predicts the incidence of Wolbachia across ants (Hymenoptera: Formicidae). J. Evol. Biol. 32, 1163–1170. doi: 10.1111/jeb.13510
Vásquez, A., Forsgren, E., Fries, I., Paxton, R. J., Flaberg, E., Szekely, L., et al. (2012). Symbionts as major modulators of insect health: Lactic acid bacteria and honeybees. PLoS One 7:e33188. doi: 10.1371/journal.pone.0033188
Viljakainen, L., Reuter, M., and Pamilo, P. (2008). Wolbachia transmission dynamics in Formica wood ants. BMC Evol. Biol. 8:55. doi: 10.1186/1471-2148-8-55
Wenseleers, T., Ito, F., Van Borm, S., Huybrechts, R., Volckaert, F., and Billen, J. (1998). Widespread occurrence of the micro-organism Wolbachia in ants. Proc. Biol. Sci. 265, 1447–1452. doi: 10.1098/rspb.1998.0456
Wenseleers, T., Sundström, L., and Billen, J. (2002). Deleterious Wolbachia in the ant Formica truncorum. Proc. Biol. Sci. 269, 623–629. doi: 10.1098/rspb.2001.1927
Wolschin, F., Hölldobler, B., Gross, R., and Zientz, E. (2004). Replication of the endosymbiotic bacterium Blochmannia floridanus is correlated with the developmental and reproductive stages of its ant host. Appl. Environ. Microbiol. 70, 4096–4102. doi: 10.1128/AEM.70.7.4096-4102.2004
Wu, T., Monnin, D., Lee, R. A. R., and Henry, L. M. (2022). Local adaptation to hosts and parasitoids shape Hamiltonella defensa genotypes across aphid species. Proc. Biol. Sci. 289:20221269. doi: 10.1098/rspb.2022.1269
Zheng, Z., Zhao, M., Zhang, Z., Hu, X., Xu, Y., Wei, C., et al. (2021). Lactic acid bacteria are prevalent in the infrabuccal pockets and crops of ants that prefer aphid honeydew. Front. Microbiol. 12:785016. doi: 10.3389/fmicb.2021.785016
Keywords: phylosymbiosis, endosymbiont, ant, microbiome, evolution
Citation: Jackson R, Patapiou PA, Golding G, Helanterä H, Economou CK, Chapuisat M and Henry LM (2023) Evidence of phylosymbiosis in Formica ants. Front. Microbiol. 14:1044286. doi: 10.3389/fmicb.2023.1044286
Received: 14 September 2022; Accepted: 31 March 2023;
Published: 05 May 2023.
Edited by:
Jose Luis Ramirez, United States Department of Agriculture (USDA), United StatesReviewed by:
Łukasz Kajtoch, Polish Academy of Sciences, PolandVeronica M. Sinotte, University of Copenhagen, Denmark
Copyright © 2023 Jackson, Patapiou, Golding, Helanterä, Economou, Chapuisat and Henry. This is an open-access article distributed under the terms of the Creative Commons Attribution License (CC BY). The use, distribution or reproduction in other forums is permitted, provided the original author(s) and the copyright owner(s) are credited and that the original publication in this journal is cited, in accordance with accepted academic practice. No use, distribution or reproduction is permitted which does not comply with these terms.
*Correspondence: Lee M. Henry, bC5oZW5yeUBxbXVsLmFjLnVr