- 1Longhua Hospital, Shanghai University of Traditional Chinese Medicine, Shanghai, China
- 2Shuguang Hospital, Shanghai University of Traditional Chinese Medicine, Shanghai, China
The gut microbiota (GM) has become recognized as a crucial element in preserving human fitness and influencing disease consequences. Commensal and pathogenic gut microorganisms are correlated with pathological progress in atherosclerosis (AS). GM may thus be a promising therapeutic target for AS. Natural products with cardioprotective qualities might improve the inflammation of AS by modulating the GM ecosystem, opening new avenues for researches and therapies. However, it is unclear what components of natural products are useful and what the actual mechanisms are. In this review, we have summarized the natural products relieving inflammation of AS by regulating the GM balance and active metabolites produced by GM.
Introduction
More than 3,000 distinct bacterial species, or trillions of microbes, can be found in the human gut, with each bacterium housing 200–300 different species (Qin et al., 2010). This microbial ecosystem is also known as the gut microbiota (GM). However, the specific taxa and the functional composition of our microbiome cannot be detected by culture-dependent bacterial identification techniques. We now have innovative methods to investigate these variations, including biological and enzymatic properties of our microbiome by whole genome shotgun sequencing (Koedooder et al., 2019).
Some evidence suggests that GM play roles in inflammation, nutrient absorption, vitamin production and xenobiotic metabolism (Milani et al., 2017). Despite our long-standing relationship with microbes, we are now beginning to realize their symbiotic dependence, especially with diseases caused by the imbalance between harmful and beneficial bacteria (Zhang and Chu, 2021). The GM has now been well characterized in models of inflammatory and autoimmune disease (Clemente et al., 2018). Research suggests that GM are critical environmental factors in the regulation of atherosclerosis (AS) inflammation (Vourakis et al., 2021). When there is an incidence of disease or infection, the mucosal immune system must have the ability to selectively and actively tolerate the GM under steady-state conditions while being able to mount an appropriate inflammatory response.
Natural products are the foundation of modern medicine and have been used to develop numerous pharmaceutical medications. Morphine (Thompson et al., 2022), strychnine (Zlotos et al., 2022), ephedrine (Yu et al., 2018), paclitaxel (Zhu and Chen, 2019), artemisinin, which earned the 2015 Nobel Prize in Physiology or Medicine, are among the most well-known. Because of their benefits to human health, natural products have been proved to be important in current medical research and innovation. Only a few active components of natural products have been found by in vitro and in vivo pharmacological and physiological evaluation. Natural medicines have been the focus of much research over the last decade, and their usage as supplementary and alternative treatments is already on the sharp rise.
Natural products are well-known for containing a large number of different components and being extremely bioactive. These substances are used to create new pharmacological treatments to treat disorders, such as cardiovascular disease (CVD) and AS through regulating GM (Zhou and Wang, 2014; Zhao et al., 2021). More research and development are needed to completely comprehend the core mechanism of action. Insights about how natural products might alter local and systemic inflammation in the human GM-AS disease axis will be discussed in this review.
Burden of cardiovascular disease
CVD (which includes coronary heart disease, heart failure, stroke, and hypertension) is becoming more prevalent among both males and females under the age of 20 (126.9 million in 2018). It is the leading cause of mortality worldwide, accounting for 18.6 million deaths (95% confidence interval: 17.1–19.7 million) in 2019, an increase of 17.1% (95% confidence interval: 11.4–22.9%) from 2010. In addition to the increased burden of morbidity and mortality, CVD currently represents the biggest direct cost ($96.2 billion) in 2016–2017. As a result, CVD is significantly linked to a higher death rate, a greater use of healthcare resources, and a greater financial burden (Virani et al., 2021).
Role of inflammation in AS
AS is a chronic degenerative pathological change of large and medium-sized arteries defined by lipid buildup in the arterial wall, accompanied by local neovascularization and apoptosis from the onset to the manifestation of problems (Moroni et al., 2019). AS is not only a major cause of CVD, but also leads to various types of cerebrovascular illness with a high death rate (Herrington et al., 2016). It has surpassed communicable illnesses to become the world’s leading and fastest-growing cause of death and disability (Roth et al., 2020).
Basic researches
The major pathogenic component that contributes to AS is lipid deposition. Oxidized low-density lipoprotein (ox-LDL) may carry endogenous cholesterol and modulate cholesterol expression in surrounding tissues (Groenen et al., 2021). Macrophages, which are the body’s main phagocytes, may absorb and breakdown Low density lipoprotein (LDL) and ox-LDL (Khoury et al., 2021). When macrophages phagocytose too much lipid and reach their maximal dynamic equilibrium, a mass of macrophage-derived foam cells gather in the injured arteries (Feng et al., 2021). Although AS starts in cholesterol plaques in the artery’s intima, it causes both local and systemic inflammation of both the adaptive and innate immune systems (Montarello et al., 2022). Chronic inflammatory response is thought to occur in three stages of the AS pathological process: early (lipid streak stage), advanced (fibrous plaque, atheromatous plaque formation), and late (fibrous plaque, atheromatous plaque formation; unstable plaque, plaque rupture, and thrombosis) stage (Jing et al., 2022). Ox-LDL and cytokines act on endothelial cells in the early stages of AS, causing them to release vascular cell adhesion molecules (VCAMs), selectin, and other adhesion molecules. Monocytes-macrophages, vascular smooth muscle cells (VSMCs), neutrophil cells, T lymphocytes, and B lymphocytes are among the cytokines and immune cells involved. These cells and cytokines engage with receptors on the cell membrane’s surface, which subsequently activate a number of linked signaling pathways through transmembrane signaling, inducing the production of target genes (Moriya, 2019; Roy et al., 2022). As a result, it’s critical to investigate the signaling route linked to inflammation and develop an effective therapeutic plan based on this target (Figures 1, 2).
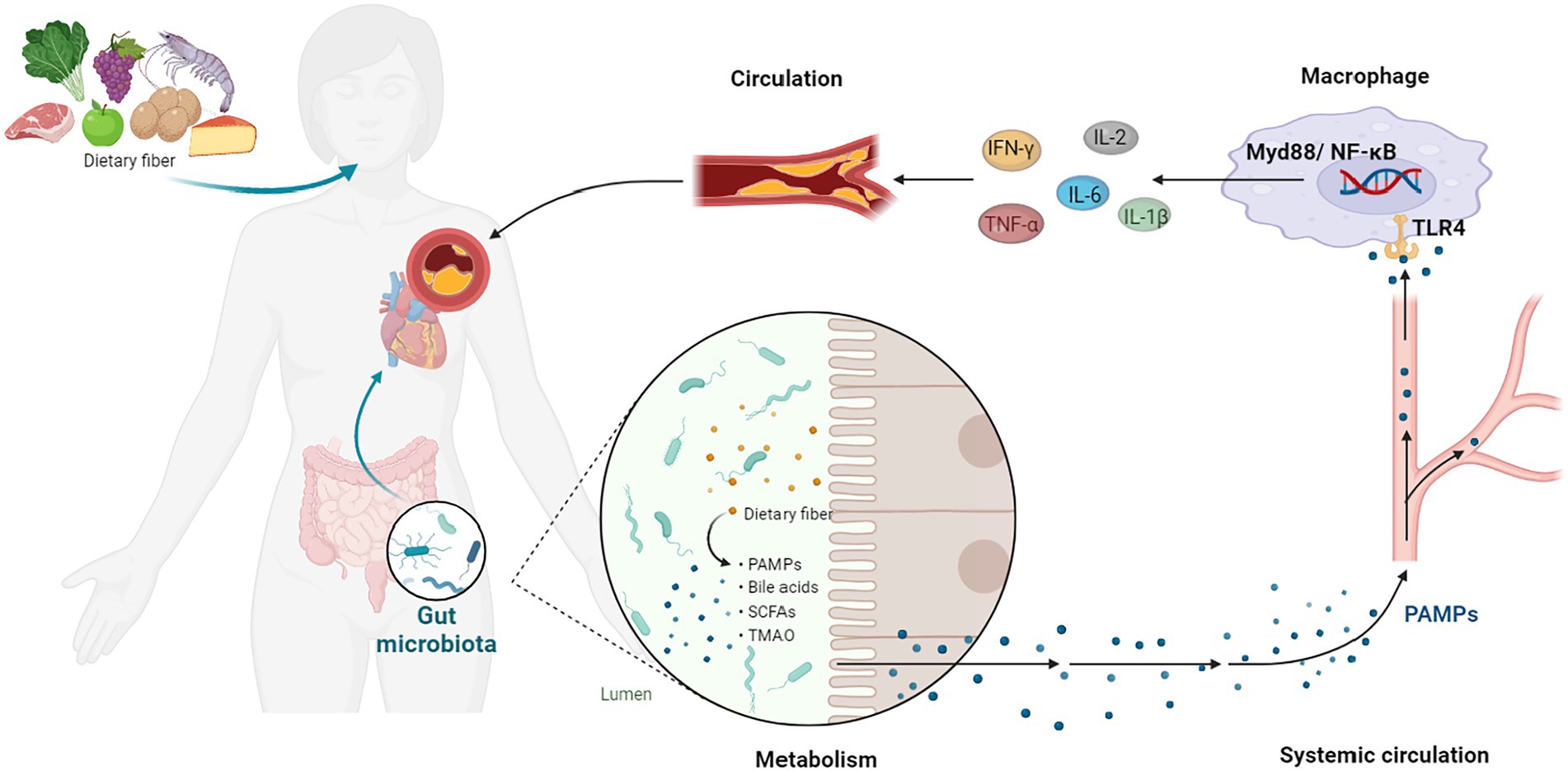
Figure 1. GM-Inflammation axis in AS. PAMPs, pathogen-associated patterns; TLR4, Toll-like receptor 4; NF-κB, NF-kappa B; TNF-α, tumor necrosis factor-α; IL, interleukin; IFN-γ, interferon-γ.
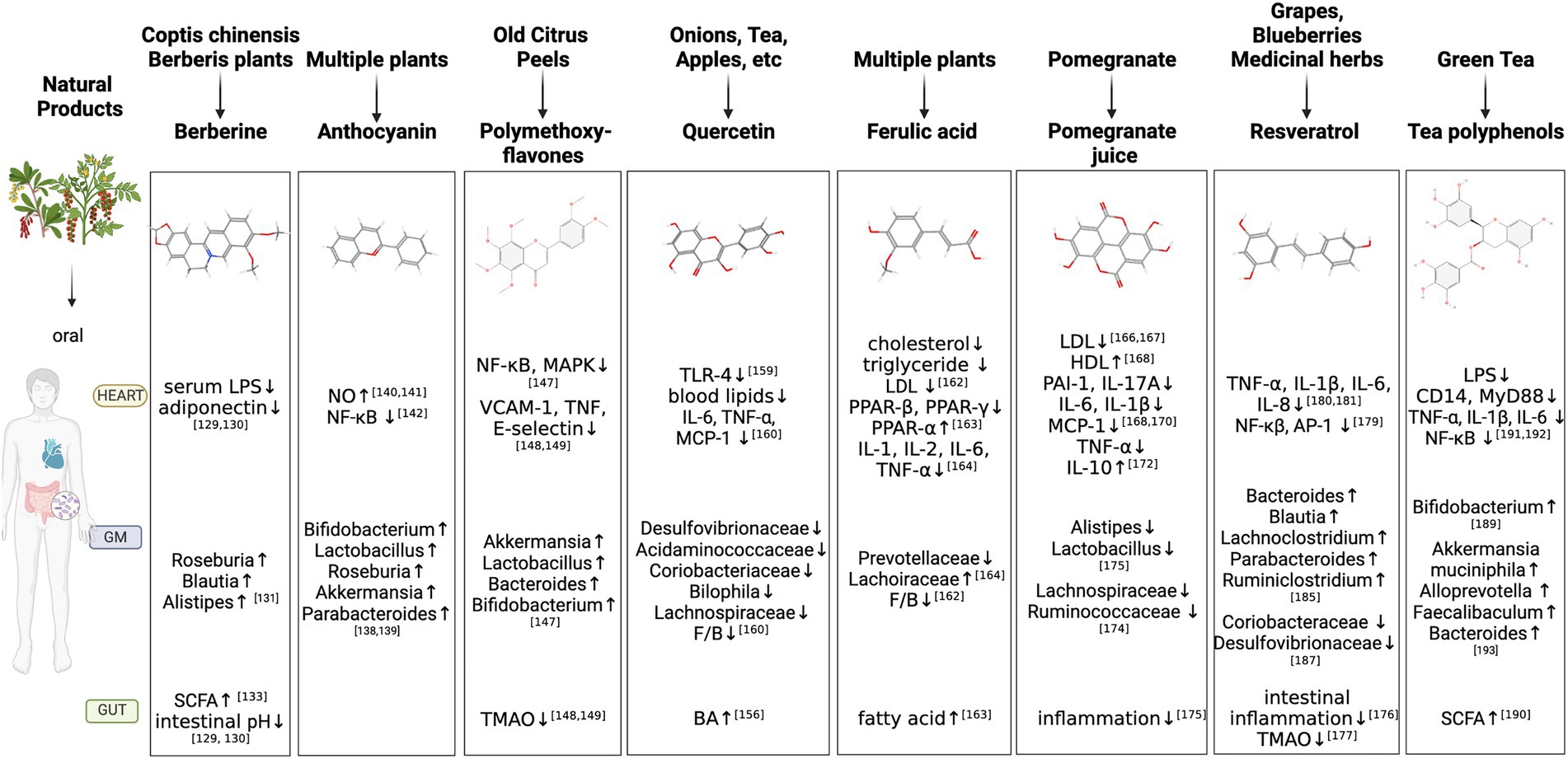
Figure 2. Natural Products regulate GM-inflammation axis in AS. GM, gut microbiota; LPS, Lipopolysaccharide; SCFA, Short-Chain Fatty Acids; NO, nitric oxide; VCAMs, vascular cell adhesion molecules; TNF, tumor necrosis factor; NF-κB, NF-kappa B; MAPK, mitogen-activated protein kinase; TMAO, Trimethylamine N-oxide; TLRs, Toll-like receptors; IL, interleukin; MCP, monocyte chemotactic protein; F/B, Firmicutes/Bacteroidetes; BA, Bile acids; LDL, Low density lipoprotein; PPAR, peroxisome proliferators-activated receptor; IL, interleukin; HDL, high density lipoprotein; PAI-1, plasminogen activator inhibitor-1; AP-1, activator protein-1; ↑, increase; ↓, decrease.
Clinical studies
Inflammation is largely to blame for the higher risk of CVD patients, according to decades of preclinical research. The CANTOS (Canakinumab Anti-inflammatory Thrombosis Outcome Study), which is reported in 2017, is the most important of the IL (interleukin)-1β trials. IL-1β has been linked to atherogenesis in experimental studies for decades. CANTOS find that inhibiting IL-1β decrease recurrent significant adverse cardiovascular events in CAD patients with well-controlled LDL levels, irrespective of cholesterol reduction (Ridker et al., 2017). This groundbreaking study shows that inhibiting the IL-1β/ IL-6 signaling cascade can reduce cardiovascular risk without reducing cholesterol levels, but at the cost of an increased risk of severe illness. CANTOS took inflammatory therapy in AS from a scientific hypothesis to a practical reality.
Following the success of CANTOS, many techniques for interfering with IL-1β or its production have evolved, including inflammasome inhibition. Further to that, the Colchicine Cardiovascular Outcomes Trial (COLCOT) and Low-Dose Colchicine 2 trial (LoDoCo2) demonstrate that treatment with colchicine, an anti-inflammation medication that targets multiple inflammatory pathways, reduces cardiac events in patients with atherosclerotic CVD (Tardif et al., 2019; Nidorf et al., 2020). In patients with recent or momentarily remote acute coronary syndromes (ACS), all of these investigations have shown therapeutic benefit.
Meanwhile, some potential research areas are now under active development but have not yet entered the clinical trial phase. Tocilizumab, a humanized monoclonal antibody targeting the IL-6 receptor, has already been evaluated in patients with ACS. The tocilizumab group has a higher myocardial salvage index than the placebo group, while the placebo group has a lower C-reactive protein (CRP; Kleveland et al., 2016; Broch et al., 2021). In the Cardiovascular Inflammation Reduction trial (CIRT), 4,786 individuals with past ACS and either type 2 diabetes mellitus or metabolic syndrome are randomized to low-dose methotrexate 15 to 20 mg weekly or placebo. Participants in the methotrexate group have lower high sensitivity CRP levels to begin with, and methotrexate have no effect on decreasing IL-1β, IL-6, or high sensitivity CRP levels (Ridker et al., 2019).
As a result, targeting inflammatory pathways might be a potential new method to prevent and cure AS (Kong et al., 2022). Inhibition of the immune response weakens the host’s defenses, making patients more vulnerable to infection. Hence, reducing chronic inflammation in atherosclerotic CVD is a goal. Finding a strategy to do so without affecting the immune system is critical.
Interplay of GM and AS
Several studies in recent years have verified the presence of bacterial deoxyribonucleic acid (DNA) in atherosclerotic plaques, and the amount of DNA linked with the presence of many leukocytes in the plaque, which may contribute to the CVD development (Koren et al., 2011; Torres et al., 2015). Furthermore, researchers discover that AS patients have abnormalities in their GM when compared to persons without AS (Koren et al., 2011; Baragetti et al., 2021; Ji et al., 2021; Szabo et al., 2021). The impact of GM on the development of atherosclerotic lesions is investigated in a few experiments. Endothelium plaque lesions are reduced in specific-pathogen-free (SPF) model animals compared with germ-free (GF) model animals at various time intervals. At 52 weeks, the SPF group has mild aortic atherosclerotic lesions compared to the GF group, whereas the GF group had significant coronary AS (Liu et al., 2018; Chuang et al., 2022). GM dose not indicate a major role to the late aortic AS of LDL receptor-deficient (LDLR−/−) mice in GF environment (Kiouptsi et al., 2020). Chen et al. demonstrate the possibility of altering the GM to prevent the AS onset and progression in LDLR−/− mice, indicating that the GM plays a role in AS and may also give a novel treatment option (Chen et al., 2020).
A higher Firmicutes/Bacteroidetes (F/B) ratio, which is frequently observed in obese persons and can validate the AS preventive effect, is detected in CAD patients according to two clinical investigations (Cui et al., 2017; Szabo et al., 2021). Phylum Proteobacteria, genus Escherichia is abundant in individuals with subclinical carotid AS and CAD, providing a novel predictor in the AS progression (Zhu et al., 2018; Baragetti et al., 2021). Phylum Bacillota, genus Acidaminococcus is formerly often found in people with inflammatory disorders and a pro-inflammatory diet, also more prevalent in the AS patients (Ji et al., 2021). Mitra et al. (2015) observed that the GM in patients with unstable plaque have more abundant Helicobacteraceae and Neisseriaceae than individuals with stable plaques. However, Lindskog Jonsson et al. (2017) point that there are no significant variations in bacterial DNA quantity or microbial composition between asymptomatic and symptomatic individuals’ plaques, or between various plaque areas. It indicates that other variables are more crucial in deciding plaque susceptibility.
Finally, there is no complete consensus on whether the GM plays a role in AS development. The relationship between the GM and AS has to be investigated further.
Altered composition and development of GM
The GM is a collection of microorganisms such as viruses, archaea, fungus, and bacteria that make up the gut’s most prevalent components. The microbiota is most commonly found in the gastrointestinal tract, particularly in the ascending colon, which is largely anaerobic and has a nutrient-rich environment that is conducive to microbe proliferation (Shanahan et al., 2021). The infant’s GM appears to be unstable and lacking in variety, as indicated by the technique of delivery. According to Watson et al., a cesarean section is used to deliver 64–82% of babies infected with methicillin-resistant Phylum Staphylococcus, genus Staphylococcus aureus (James et al., 2008). Babies born through cesarean section may be more vulnerable to pathogen infections. In addition, analogous to human skin, the GM of a baby delivered via cesarean section is changed and dominated by Phylum Streptococcus, Phylum Corynebacterium, and Phylum Propionibacterium (Rautava, 2017). The intestinal microbiota evolves until around the age of three, when it becomes a varied, complicated, and steady collection with 60–70% resemblance to the adult GM, influenced by the environment surrounding the baby (Kashtanova et al., 2016). Some elements can impact the microbiota throughout development, such as food (i.e., breast milk or formula feeding) and antibiotic usage, which is frequently linked to disturbance of the newborn GM. Several studies have discovered that the GM of infants can promote long-term health and that human GM at an early age is linked to certain adult health issues (Milani et al., 2017; Butel et al., 2018; Kim et al., 2019).
Nine phyla that make up the intestinal flora of adults, which are Actinobacteria, Bacteroidetes, Firmicutes, Fusobacteria, Proteobacteria, Cerrucomicrobia, Lentisphaerae, Verrucomicrobia, Euryarchaeota, and Spirochaetes (Li et al., 2014). Bacteroidetes and Firmicutes make up over 90% of the total bacterial species in a healthy bacterial ecosystem (Qin et al., 2010). Simultaneously, the F/B ratio varies greatly, which is used as a health indicator of the GM in all persons. The F/B ratio rises in obese persons (the phylum Firmicutes rises, while the phylum Bacteroidetes falls) and is linked to several CVD (Crovesy et al., 2020; Magne et al., 2020). It has been demonstrated that the microbial makeup of CAD patients’ changes, with a large rise in Firmicutes abundance and a reduction in Bacteroidetes abundance (Emoto et al., 2016; Sanchez-Rodriguez et al., 2020). The GM evolves not just in the early stages of life, but even in the elderly, with changes in bacterial diversity and shifting of dominating species (e.g., decreasing abundance of beneficial microorganisms and increasing abundance of facultative anaerobic bacteria). These alterations might be linked to the onset of AS in the elderly (Shen et al., 2021; Vourakis et al., 2021). Details are shown in Table 1.
Trimethylamine N-oxide
GM, especially those belonging to the Phylum Fusobacterium, genus Clostridia and Phylum Escherichia, genus Escherichia coli, process dietary phosphatidylcholine and l-carnitine into Trimethylamine (TMA; Brown and Hazen, 2015). Through the portal circulation, TMA is transported to the liver, where it is converted to TMAO by hepatic flavin monooxygenase 3 (FMO3). The suppression of the FMO3 gene has been shown in certain studies to greatly lower the formation of TMAO (Rath et al., 2017; Luo et al., 2022). Plasma levels of TMAO are very variable both within and across individuals, making it difficult to compare research (Papandreou et al., 2020).
TMAO is different from traditional CVD risk factors in that it is produced by gut microbes. More experiments in recent years have discovered that TMAO may become a whole new marker for predicting future CVD risk, with an emphasis on the proper serum levels to diagnose CVDs (Zheng et al., 2019; Tang et al., 2021). Several innovative techniques to inhibit TMAO production and prevent AS have been discovered in recent years (Iglesias-Carres et al., 2021; Li et al., 2021). Researchers reveal in one experiment that a high-choline diet offered to mice increases intraplaque bleeding rather than changing atherosclerotic load or plaque composition, which is a very new result (Lindskog Jonsson et al., 2018). As part of immune defense, TMAO activates the heat shock protein 60, which has been demonstrated to initiate AS and to participate in foam cell formation via Toll like receptors, which are also activated by SR-A1 and CD36 in macrophages after TMAO stimulation (Febbraio et al., 2000; Wick et al., 2004, 2014; Collot-Teixeira et al., 2007). Apart from that, TMAO can activate NLRP3, which can lead to IL-1β, IL-6 and tumor necrosis factor (TNF)-α production, triggering inflammation and endothelial injury that eventually leads to AS (Wu et al., 2020). TMAO also promotes the AS development by enhancing the production of IL-1β, IL-6, TNF-α and CRP through the activation of mitogen-activated protein kinase (MAPK) and NF-kappa B (NF-κB) signaling (Seldin et al., 2016; Chen et al., 2017; Chou et al., 2019). Additionally, TMAO could reduce reverse cholesterol transport by activating the nuclear receptor Farnesoid X receptor (FXR), which in turn might reduce bile acid synthesis in the liver with the effect of accelerating AS development (Ding et al., 2018; Tan et al., 2019).
In such cases, lowering TMAO to lower the AS incidence using certain effective, safe techniques might have a big impact on public health. However, the majority of in vivo investigations are undertaken in mouse models, with only a handful of people. According to Senthong et al. (2016) and Tan et al. (2019) plasma TMAO levels are closely related to coronary atherosclerotic burden in patients with ST-segment elevation myocardial infarction (STEMI) and stable coronary artery disease (CAD). Liu et al. (2018) previously demonstrate that non-culprit plaques in CAD patients with greater TMAO levels have more susceptible features, including a thinner fibrous cap, a higher presence of thin-cap fibroatheroma and microvessels. However, A comparison of plasma TMAO levels in CAD patients and control subjects reveal no significant differences in another trial (Bordoni et al., 2020).
TMAO plays a role in the complex pathological processes of atherosclerotic lesion formation. The preliminary findings suggest that TMAO might serve as a potential target for the prevention and treatment of AS, which is conceptually novel, compared with existing treatments. The regulating of TMAO production and associated GM may be an effective strategy against AS (Zhu et al., 2020).
Short-chain fatty acids
Certain gut microbes produce SCFAs (such as acetate, proprionate, and butyrate) by fermenting otherwise indigestible dietary fibers. It has been shown that fecal and plasma levels of SCFA are related to the presence of SCFA-producing microbiota in the gut and the consumption of dietary fibers (Calderon-Perez et al., 2020; Deehan et al., 2020; Li et al., 2020; Verhaar et al., 2020). SCFAs are absorbed and delivered through the portal circulatory system to control immunological responses and a variety of metabolic activities in the host (Wenzel et al., 2020; Yang et al., 2020). SCFAs have emerged as beneficial biological microbial products with well-known anti-atherogenic properties, such as lowering systemic inflammation, increasing endothelial cell function, and maintaining intestinal barrier integrity (Lin and Zhang, 2017).
According to a study, aortic atherosclerotic plagues are decreased by 50% when apolipoprotein E (ApoE)−/− mice had a diet containing 1% butyrate for 10 weeks, with lower macrophage infiltration and greater collagen deposition, implying a more durable fibrous cap. These events are largely linked to a decrease in CD36 in macrophages and endothelial cells, as well as a decrease in pro-inflammatory cytokines and NF-κB activation (Aguilar et al., 2016). Butyrate also can prevent inflammation development by effecting proliferation of VSMCs (Ranganna et al., 2003; Mathew et al., 2014). Atherosclerotic GF ApoE−/− mice were inoculated with a specified population of eight bacteria. The size of atherosclerotic plaques is decreased by 30% using that axis without changing cholesterol or TMAO levels, possibly due to SCFAs’ capacity to keep the gut healthy (Bultman, 2018; Kasahara et al., 2018). Furthermore, Bartolomaeus et al. (2019) discover that improving vascular inflammation and atherosclerotic lesion load in ApoE−/−mice, as well as lowering blood pressure, all of which are classic plaque rupture risk factors. In addition to mitigating CVD risks, they have also been useful in mitigating diabetes, obesity, hypertension (Koh et al., 2016), etc. SCFAs improves gut barrier integrity, vascular dysfunction, hypertrophy fibrosis, and cardiac ventricular arrhythmias in ApoE−/− mice (Du et al., 2020).
In GM-AS disease axis research, SCFA butyrate is therefore believed to act antagonistically to its counterpart secondary metabolite-TMAO. It might be a novel strategy—supplementing SCFAs to prevent AS and stabilize plaque in the event of a cardiovascular event. However, because few human studies have been completed, additional research into the role of SCFAs is still needed.
Bile acids
Another group of GM-produced metabolites involved in various metabolic disorders is BAs, which are stored in the gallbladder and released into the intestine to aid dietary lipid absorption and dietary fat-soluble vitamin absorption (Kuipers et al., 2014). The GM-derived enzymes usually transform primary BAs (such as deoxycholic acid, lithocholic acid hyodeoxycholic acid, and ursodeoxycholic acid) into secondary BAs. The enterohepatic system of GF mice exhibit higher levels of primary BAs, but no secondary BAs (Chiang, 2013). High fat diet (HFD)-induced gut microbiome changes are inhibited when liver BA biosynthesis is suppressed. This illustrates a metabolic link between the liver, the microbiome of the gut, and the BAs (Zheng et al., 2017). Consequently, there exists a bidirectional relationship between GM and BAs metabolism (Yan et al., 2021).
Through the BAs receptor (also known as FXR), the GM can affect lipid and cholesterol metabolism. It is vital for the absorption of dietary lipids and fat-soluble vitamins that bile acids are present (Parseus et al., 2017). Upon feeding mice a chow diet, FXR-deficient mice develop hypercholesterolemia, related to impaired high density lipoprotein (HDL) metabolism and reverse cholesterol transport (Lambert et al., 2003). Studies using FXR agonists in mice prone to AS have also demonstrated that this receptor may contribute to AS prevention, but this effect may be weight-dependent (Miyazaki-Anzai et al., 2014). Moreover, double-knockout mice for ApoE and FXR have a deteriorated plasma lipid profile and more atherosclerotic lesions compared to ApoE−/− mice (Hanniman et al., 2005). Since FXR is expressed by aortic smooth muscle cells and endothelial cells, this leads to aortic smooth muscle cell injury (He et al., 2006; Zhang et al., 2008). Several FXR-expressing tissues may be involved in atherogenesis, and the role of microbial regulation of BAs is not fully understood. Animal studies account for the majority of current data, while human data is sparse.
GM and inflammation in AS
Several diseases are characterized by inflammation, such as AS, cancer, asthma, autoimmune disorders and neurodegenerative disorders (Leuti et al., 2020). Pathogens are prevented by the epithelium of the gut, the first barrier of the host. In the presence of an impaired intestinal epithelial barrier, invasion of pathogen-associated molecular patterns (PAMPs) elicits an immune response, leading to systemic and tissue-specific inflammation (Desai et al., 2016). Dysbiosis of the gut has been identified as a risk factor for chronic inflammation, consequently reducing gut barrier integrity (Barcik et al., 2020).
Lipopolysaccharide (LPS) and peptidoglycan are microbial components known to contribute to CVD (Roberts and Buford, 2021). In 1999, plasma endotoxins are measured in the clinic to establish the link between LPS and CVD (Wiedermann et al., 1999). Gram-negative bacteria produce LPS as a component of their cell walls, which has been extensively studied in relation to CVD risk. Numbers of subsequent studies conducted by different groups of researchers have gradually confirmed the relationship (Battson et al., 2018; Asada et al., 2019; Yoshida et al., 2020). A significant increase in CVD is associated with the transportation of LPS derived from microbiomes. The LPS derived from gut dysbiosis may modulate Toll-like receptors (TLRs) and their downstream targets, such as myeloid differentiation factor88 (Myd88) and NF-κB, resulting in increased expression of pro-inflammatory cytokines and an increased risk of CVD (Guzzo et al., 2012; Zhao et al., 2021; Lai et al., 2022). Low-grade chronic inflammation triggered by LPS may also lead metabolic endotoxemia (ME), which is commonly observed in CVD patients. Increased intestinal permeability is associated with ME (Moludi et al., 2020).
Furthermore, another bacterial PAMPs—peptidoglycan (PG) has been linked to an increased risk of CVD via compromising the intestinal epithelial barrier (Han et al., 2021). PG is a small component from the cell wall of Gram-negative bacteria, whereas it is a large component of Gram-positive bacteria. Scientists discover that patients with AS have an amplification of genes that encoded PG production through metagenomic sequencing (Karlsson et al., 2012; Jin et al., 2021). Undoubtedly, atherosclerotic arteries are shown to include pro-inflammatory bacterial PG, which is linked to susceptible plaques (Laman et al., 2002).
Other PAMPs, such as CpG-oligodeoxynucleotides, flagellin, lipopeptides and others, can stimulate inflammatory processes by interacting with host pattern recognition receptors (Frostegard, 2022). All of the data points to functional alterations in the GM as a possible contributor to the development of AS risk. As shown in the Figure 1.
Natural products regulate GM and inflammation in AS
Until recently, non-invasive therapeutic interventions for AS mostly concentrate on pharmaceutically reducing risk variables, such like arterial hypertension and hypercholesterolemia following healthy lifestyle choices. Inflammation, on the other hand, provides a series of pathways that connect established risk variables to altered arterial wall cell behavior and leukocyte recruitment. These pathways contribute to the progression of disease and its effects. Natural medicines have been the subjects of much research, and their clinical application as an appropriate and supplementary treatment is growing at an increasing pace across countries. Natural products formed from natural remedies are substances with a well-known chemical diversity and bioavailability. These items are still being used as a model for creating new pharmacological medications to prevent ailments like CVD (Zhou et al., 2020). Natural products have been proved to improve inflammation, foam cell production, lipid oxidation and abnormal platelet function (Hong et al., 2019).
The GM’s composition and activity can affect intestinal permeability, digestion, metabolism and immunological responses. Alterations in GM equilibrium generate a pro-inflammatory status, which contributes to the beginning of a variety of illnesses varying from gastrointestinal and metabolic disorders to immunological disorders (Gomaa, 2020). In recent years, the GM sector has shifted its attention to natural products and medical research, as well as attempts to recognize natural products’ pharmacological activities. As shown in the Figure 2.
Berberine
BBR is a major bioactive component of Coptis chinensis and other Berberis plants, which compound with numerous pharmacological properties. In addition to improving cardiovascular hemodynamics, BBR inhibits ischemic arrhythmia, slows down AS development, and reduces hypertension (Cicero and Baggioni, 2016; Song et al., 2020). The mechanisms of BBR include platelet activation inhibited, endothelial function normalized, macrophage-derived foam cell generation suppressed, inflammation and inflammasome activation inhibited and so on (Feng et al., 2019). It also has antibacterial properties, preventing bacterial reproduction (Zhang et al., 2021).
BBR increases gut microbial community, decreases intestinal pH and exogenous antigen deposition, raises LPS-binding protein and leptin levels, decreases serum adiponectin, has lipid-lowering and hypoglycemic benefits, boosts antibiotic culture and SCFAs synthesis (Han et al., 2011; Zhang et al., 2012). BBR boosts the synthesis of SCFAs in these phyla: Roseburia, Blautia and Alistipes (Wu et al., 2020). Cohousing BBR-treated mice with HFD-fed animals inhibits atherosclerotic progression in a comparable way to non-cohoused BBR-treated mice (Shi et al., 2018). In addition to this, Wu et al. further argue that different doses of BBR demonstrate different sensitivity towards GM composition. High doses of BBR display profound GM metabolic activity through the production of SCFAs. This activity is linked to BBR-induced GM compositional shift (Zhu et al., 2018).
By restoring intestinal SCFAs levels, reducing serum LPS levels, reducing intestinal inflammation and repairing intestinal barrier integrity, BBR treatments proved effective (Zhang et al., 2019). The BBR also reverses structural and compositional changes in the GM and influences gut microbe-dependent metabolic pathways (Yang et al., 2021). These results suggest that BBR interacts with GMs in order to play a regulatory role in dysmetabolism and AS.
Anthocyanin
Anthocyanin is flavonoid polyphenols with antioxidant properties that have been associated with reduced CVD risk (Aboonabi and Singh, 2015; Garcia and Blesso, 2021). Isolated anthocyanin successfully modifies the “pro-atherogenic” GM communities by raising percentages of five genera (Bifidobacterium, Lactobacillus, Roseburia, Akkermansia and Parabacteroides; Lavefve et al., 2020; Si et al., 2021).
In addition to controlling GM, Anthocyanin enhances vascular function by increasing nitric oxide (NO) production, lowering oxidative stress, and decreasing aortic inflammation. The aortas from these animals have much less leukocyte infiltration, less plaque development and lower quantities of blood inflammatory cytokines than those in the control mice (Joo et al., 2018; Festa et al., 2021). The NF-κB inflammatory pathway, hepatic lipid metabolism and cell redox state are all under the influence of Anthocyanin at the same time (Mena et al., 2014).
In cultured macrophages and endothelial cells, Anthocyanins may also change the mRNA levels of genes associated with AS. According to a nutrigenomic research, Anthocyanins alters the expressions of 1,261 genes in the aorta, which are linked to the development and prevention of AS (Mauray et al., 2012). Protocatechuic acid is a kind of Anthocyanin metabolites that partially inhibits AS by activating the miRNA-10b-ABCA1/ABCG1-cholesterol efflux signaling pathway (Wang et al., 2012).
As a result, Anthocyanin-mediated activities significantly alleviates AS, however it is unclear how these effects would translate in “real world” with GM confounders.
Polymethoxyflavones
PMFs, which are abundant in old citrus peels, have a wide range of biochemical functions, including antioxidant and anti-inflammatory properties (Lai et al., 2015). Aside from a well broad spectrum of their anti-AS properties, the current wave of microbial investigation has motivated scientists to explore their influence on GM balance (Yen et al., 2011).
PMFs change the GM structure to a healthy phenotype by boosting Akkermansia, Lactobacillus, Bacteroides, and Bifidobacterium genera. They increase butyrate-producing bacteria and reduces TMA-producing microorganisms, respectively. PMFs also suppress hepatic FMO3, which reduce the conversion of L-carnitine to TMAO. Apart from inhibiting the TMAO synthesis route, PMFs can also decrease the NF-κB and MAPK signaling pathways, preventing vascular inflammation (Yang et al., 2019). By lowering markers of inflammation (VCAM-1, TNF and E-selectin) and TMAO, PMFs reliably reduce L-carnitine-induced vascular inflammation. Therapy with PMFs further reduce the development of macrophage foam cells (Whitman et al., 2005; Chen et al., 2019). By using high performance liquid chromatography electrospray ionization tandem mass spectrometry, a total of 21 PMF metabolites that are discovered (Chen et al., 2020).
Quercetin
Quercetin is a flavonoid that may be found in a variety of foods (onions, tea, apples, etc.) and reduces platelet aggregation and thrombus development in human participants (Hubbard et al., 2004). Similarly, it benefits cholesterol metabolism, intestinal inflammation, atherosclerotic lesions and sizes of plaques, and promote gut health (Nie et al., 2019; Uyanga et al., 2021).
Quercetin has been found to have concentrated action in GM regulation, which is useful in helping mice recover their GM after receiving antibiotics and may serve as a prebiotic in the fight against gut dysbiosis (Shi et al., 2020; Zhao et al., 2021). It’s worth noting that 32 metabolic markers are discovered to drive Quercetin’s effects. The major BA production route, specifically 3, 7, 12, and 26-tetrahydroxy-5-cholestane, is one of them (C27H48O4). This suggests Quercetin may increase the GM metabolite BA, correlated with circulating BAs and liver lipid and BA metabolism genes (Juarez-Fernandez et al., 2021). BAs, as aforementioned, play an important role in maintaining human metabolic processes such adipose tissue browning, insulin sensitivity and intestinal barrier integrity (Chiang and Ferrell, 2020). Additionally, intestinal Cu/Zn-superoxide dismutase, glutathione peroxidase and nutritional transporters such glucose transporter 2, peptide transporter 1 and fatty acid synthase genes have significantly higher levels of mRNA expression in the groups who received quercetin supplements (Abdel-Latif et al., 2021). With the consequent suppression of the inflammasome sensitivity and stimulation of the reticulum stress pathway, quercetin restores GM imbalance and associates with endotoxemia-mediated TLR-4 pathway stimulation (Porras et al., 2017). Quercetin and Resveratrol have positive impacts in improving the dysbiosis of the GM and obesity brought on by the HFD. They attenuate blood lipids, serum IL-6 and TNF-α and monocyte chemotactic protein (MCP)-1. Significantly limit the relative abundance of Desulfovibrionaceae, Acidaminococcaceae, Coriobacteriaceae, Bilophila, Lachnospiraceae family and result in a decrease in the F/B ratio (Zhao et al., 2017).
Ferulic acid
FA is a well-known phenolic compound that has anti-inflammatory and antioxidant properties. It helps the metabolism of lipids, diabetes and thrombosis in addition to having cardioprotective and antihypertensive effects (Neto-Neves et al., 2021). A study shows that FA can lower blood cholesterol, triglyceride and LDL levels as well as the ratio of F/B in GM composition (Ma et al., 2019). Additionally, FA lowers the expression of peroxisome proliferator-activated receptor (PPAR)-β and PPAR-γ and increases the expression of PPAR-α, a gene linked to fat metabolism. The fatty acid production and metabolism benefits from these alterations in gene expression, which also reduces fat accumulation (Yin et al., 2022).
In mice, FA can modulate the GM and inflammation and drastically reduce AS. The GM and fecal metabolites undergo noticeable structural alterations due to FA. In contrast to enhancing the Lachoiraceaea family, FA can reduce the levels of IL-1β, IL-2, IL-6, TNF-α and relative proportions of the Prevotellaceae family (Hu et al., 2022).
Pomegranate juice
PJ is high in polyphenols and is renowned for its anti-AS and anti-inflammation characteristics (Danesi and Ferguson, 2017). PJ results in a significant reduction of the atherosclerotic lesion size and intima media thickness, a 20% reduction in the absorption of ox-LDL and native LDL by peritoneal macrophages (Aviram et al., 2000; Basu and Penugonda, 2009), a 27% rise in HDL content and a 12 to 18% reduction in the risk of CVD (Michicotl-Meneses et al., 2021).
In RAW264.7 macrophages, PJ stimulates cholesterol efflux from foam cells and inhibits the pathophysiology of initial atherogenesis (Zhao et al., 2016). The expression of vascular inflammation indicators, arterial endothelial nitric oxide synthase (eNOS), and levels of inflammatory cytokines [TNF-α, plasminogen activator inhibitor-1 (PAI-1), IL-17A, IL-6, IL-1β, and MCP-1] all show a considerable decline (de Nigris et al., 2007; Michicotl-Meneses et al., 2021). A PJ supplementation reduces oxidative stress in macrophages and cholesterol flux in macrophages, even also attenuates AS in mice (Kaplan et al., 2001). A dose-dependent impact of PJ and its primary polyphenols decreases TNF-α and IL-6 secretion significantly. A considerable increase in the spontaneous release of IL-10 revealed that PJ dose-dependently influences macrophages toward an anti-inflammatory M2 phenotype (Aharoni et al., 2015).
After using PJ supplements, the levels of the phenolic metabolites 3-phenylpropionic acid, catechol, hydroxytyrosol and urolithin A in the feces significantly increase (Mosele et al., 2015). PJ is reported to reverse acrolein-induced GM dysbiosis by rebalancing the F/B ratio and lowering the prevalence of Ruminococcaceae and Lachnospiraceae at the family level. In vivo and in vitro atherosclerotic models, it also preventes acrolein-induced lipid buildup, peroxidation and oxidative stress by decreasing critical lipid biosynthesis pathways (Rom et al., 2017). Furthermore, PJ with chitinglucan reduces the relative abundance of phylum Bacteroidetes, genus Alistipes and Phylum Firmicutes, genus Lactobacillus through altering their genes, also reduces hepatic lipids, inflammation and endothelial dysfunction through activating eNOS (Neyrinck et al., 2019).
Resveratrol
RSV is a stilbenoid phenol that may be discovered in grapes, blueberries and medicinal herbs, which can reduce intestinal permeability and inflammation and enhance gut barrier (Cai et al., 2020). It is also effective anti TMAO-induced AS in a GM-dependent way and increase the abundance of genera Lactobacillus and Bifidobacterium (Chen et al., 2016).
RSV has been found by researchers to have anti-inflammatory effects and to have the ability to prevent the generation of inflammatory cytokines. This anti-inflammatory impact may be linked to the modulatory effect of microRNAs transcription with either an anti-inflammatory or a pro-inflammatory role (Dvorakova and Landa, 2017), which is predominantly mediated by the capacity to regulate NF-κβ and activator protein-1 (AP-1; Latruffe et al., 2015). In this setting, RSV has been shown to be able to reduce chronic low-grade inflammation, which is defined by the buildup of macrophages in the adipose tissue and excessive cytokine production. For instance, it reduces the release of TNF-α, IL-1β, IL-6 and IL-8 in murine adipocytes and human adipose tissue explants (Olholm et al., 2010; Yen et al., 2011). RSV may reduce inflammation linked to obesity in genetically (Gomez-Zorita et al., 2013). Even more, it can have an immediate anti-inflammatory impact in healthy persons (Ghanim et al., 2011).
RSV reduces the GM structural alterations and inflammatory markers by HFD (Man et al., 2020). Genera Bacteroides, Blautia, Lachnoclostridium, Parabacteroides and Ruminiclostridium 9 are enriched in the gut of mice treated with RSV, which is a striking modification in the makeup of the microbiota (Wang et al., 2020). The anti-inflammation benefits of RSV are substantially dependent on GM, as demonstrated by a fecal microbiota transplantation experiment (Wang et al., 2020, 2022). RSV also successfully stops the growth of Coriobacteraceae and Desulfovibrionaceae, two family that have a strong relationship with body weight that are caused by the HFD (Campbell et al., 2019).
Tea polyphenols
Given atherosclerotic circumstances, TPs are reported to exhibit a wide range of biological actions, which have antioxidants and anti-inflammatory effects (Bornhoeft et al., 2012).
TPs decrease plaque/lumen area and increase Bifidobacterium colony abundances in the intestines of HFD-fed ApoE−/− mice. Furthermore, the rise in Bifidobacterium count is approximately equal to the plaque/lumen size. This means that TPs target Bifidobacterium specifically to reduce atherosclerotic plaque (Liao et al., 2016). TPs increase SCFAs synthesis in C57BL/6 J mice with human flora (Wang et al., 2018). TPs may decrease systemic LPS levels and inflammation brought on by obesity. Toll-like receptor 4 (TLR4), as well as CD14, MyD88 and other co-receptors, are downregulated in hepatic and adipose tissues in response to the drop in the level of LPS in the blood. This further prevents NF-κB to be activated. In mice fed with HFD, TPs dramatically reduce the blood levels of TNF-α, IL-1β and IL-6. By boosting intestinal tight junction proteins, TPs also preserve the integrity of the intestinal barrier and repair gut dysbiosis in obese mice (Li et al., 2020; Ye et al., 2022).
The performance of the barrier function, particularly intestinal inflammation and the integrity of the intestinal barrier, are improved by TPs. They also reduce the phylogenetic variation and F/B ratio associated with GM dysbiosis brought on by HFD. Other fundamental bacteria are discovered to benefit from TPs include genera Akkermansia muciniphila, Alloprevotella, Bacteroides and Faecalibaculum (Zhou et al., 2021). Another study finds that TPs increase the relative abundance of phylum Firmicutes while decreasing the relative number of genera Bacteroidetes and genera Fusobacteria by 16S rRNA gene sequencing. In addition, significant correlations exist between the weight loss caused by TPs and the relative amount of the genera Acidaminococcus, Anaerobiospirillum, Anaerovibrio, Bacteroides, Blautia, Catenibactetium, Citrobacter, Clostridium, Collinsella and Escherichia (Li et al., 2020).
Conclusion
Natural products can reduce the pro-inflammation cytokines and inhibit inflammation-related signaling pathways in AS-related CVD by regulating the GM composition. The interplay of natural products with GM and inflammation gives unique insights into the gut-heart axis, expanding routes and concepts for using medicinal plants and naturally derived medications. Further research encompassing pharmacodynamics, pharmacokinetics, microbiomics, and metabolomics is urgently required to have a thorough understanding of this developing field in terms of creating innovative therapeutic methods for the prospective therapy of atherosclerotic CVD.
Author contributions
LT wrote the first draft of the review. BD compiled the tables and figures, and all the revisions according to the reviewers. YW accepts overall responsibility for the final submitted version of this review. All authors contributed to the article and approved the submitted version.
Funding
This study was supported by the National Natural Science Foundation to YW (grant number 82204849), Traditional Chinese Medicine Research Project of Shanghai Municipal Health Commission to YW (grant number 2022QN056), Clinical Technology Innovation Cultivation Program of Longhua Hospital Affiliated to Shanghai University of Traditional Chinese Medicine to YW (grant number PY2022008), and Regional medical Centre of Longhua Hospital Affiliated to Shanghai University of Traditional Chinese Medicine to YW (grant number ZYZK001-029).
Conflict of interest
The authors declare that the research was conducted in the absence of any commercial or financial relationships that could be construed as a potential conflict of interest.
Publisher’s note
All claims expressed in this article are solely those of the authors and do not necessarily represent those of their affiliated organizations, or those of the publisher, the editors and the reviewers. Any product that may be evaluated in this article, or claim that may be made by its manufacturer, is not guaranteed or endorsed by the publisher.
References
Abdel-Latif, M. A., Elbestawy, A. R., El-Far, A. H., Noreldin, A. E., Emam, M., Baty, R. S., et al. (2021). Quercetin dietary supplementation advances growth performance, gut microbiota, and intestinal mRNA expression genes in broiler chickens. Animals (Basel). 11:2302. doi: 10.3390/ani11082302
Aboonabi, A., and Singh, I. (2015). Chemopreventive role of anthocyanins in atherosclerosis via activation of Nrf2-are as an indicator and modulator of redox. Biomed. Pharmacother. 72, 30–36. doi: 10.1016/j.biopha.2015.03.008
Aguilar, E. C., Santos, L. C., Leonel, A. J., de Oliveira, J. S., Santos, E. A., Navia-Pelaez, J. M., et al. (2016). Oral butyrate reduces oxidative stress in atherosclerotic lesion sites by a mechanism involving NADPH oxidase down-regulation in endothelial cells. J. Nutr. Biochem. 34, 99–105. doi: 10.1016/j.jnutbio.2016.05.002
Aharoni, S., Lati, Y., Aviram, M., and Fuhrman, B. (2015). Pomegranate juice polyphenols induce a phenotypic switch in macrophage polarization favoring a M2 anti-inflammatory state. Biofactors 41, 44–51. doi: 10.1002/biof.1199
Asada, M., Oishi, E., Sakata, S., Hata, J., Yoshida, D., Honda, T., et al. (2019). Serum lipopolysaccharide-binding protein levels and the incidence of cardiovascular disease in a general Japanese population: the Hisayama study. J. Am. Heart Assoc. 8:e013628. doi: 10.1161/JAHA.119.013628
Aviram, M., Dornfeld, L., Rosenblat, M., Volkova, N., Kaplan, M., Coleman, R., et al. (2000). Pomegranate juice consumption reduces oxidative stress, atherogenic modifications to LDL, and platelet aggregation: studies in humans and in atherosclerotic apolipoprotein E-deficient mice. Am. J. Clin. Nutr. 71, 1062–1076. doi: 10.1093/ajcn/71.5.1062
Baragetti, A., Severgnini, M., Olmastroni, E., Dioguardi, C. C., Mattavelli, E., Angius, A., et al. (2021). Gut microbiota functional dysbiosis relates to individual diet in subclinical carotid atherosclerosis. Nutrients 13:304. doi: 10.3390/nu13020304
Barcik, W., Boutin, R. C. T., Sokolowska, M., and Finlay, B. B. (2020). The role of lung and gut microbiota in the pathology of asthma. Immunity 52, 241–255. doi: 10.1016/j.immuni.2020.01.007
Bartolomaeus, H., Balogh, A., Yakoub, M., Homann, S., Marko, L., Hoges, S., et al. (2019). Short-chain fatty acid propionate protects from hypertensive cardiovascular damage. Circulation 139, 1407–1421. doi: 10.1161/CIRCULATIONAHA.118.036652
Basu, A., and Penugonda, K. (2009). Pomegranate juice: a heart-healthy fruit juice. Nutr. Rev. 67, 49–56. doi: 10.1111/j.1753-4887.2008.00133.x
Battson, M. L., Lee, D. M., Weir, T. L., and Gentile, C. L. (2018). The gut microbiota as a novel regulator of cardiovascular function and disease. J. Nutr. Biochem. 56, 1–15. doi: 10.1016/j.jnutbio.2017.12.010
Bordoni, L., Samulak, J. J., Sawicka, A. K., Pelikant-Malecka, I., Radulska, A., Lewicki, L., et al. (2020). Trimethylamine N-oxide and the reverse cholesterol transport in cardiovascular disease: a cross-sectional study. Sci. Rep. 10:18675. doi: 10.1038/s41598-020-75633-1
Bornhoeft, J., Castaneda, D., Nemoseck, T., Wang, P., Henning, S. M., and Hong, M. Y. (2012). The protective effects of green tea polyphenols: lipid profile, inflammation, and antioxidant capacity in rats fed an atherogenic diet and dextran sodium sulfate. J. Med. Food 15, 726–732. doi: 10.1089/jmf.2011.0258
Broch, K., Anstensrud, A. K., Woxholt, S., Sharma, K., Tollefsen, I. M., Bendz, B., et al. (2021). Randomized trial of Interleukin-6 receptor inhibition in patients with acute st-segment elevation myocardial infarction. J. Am. Coll. Cardiol. 77, 1845–1855. doi: 10.1016/j.jacc.2021.02.049
Brown, J. M., and Hazen, S. L. (2015). The gut microbial endocrine organ: bacterially derived signals driving cardiometabolic diseases. Annu. Rev. Med. 66, 343–359. doi: 10.1146/annurev-med-060513-093205
Bultman, S. J. (2018). Bacterial butyrate prevents atherosclerosis. Nat. Microbiol. 3, 1332–1333. doi: 10.1038/s41564-018-0299-z
Butel, M. J., Waligora-Dupriet, A. J., and Wydau-Dematteis, S. (2018). The developing gut microbiota and its consequences for health. J. Dev. Orig. Health Dis. 9, 590–597. doi: 10.1017/S2040174418000119
Cai, T. T., Ye, X. L., Li, R. R., Chen, H., Wang, Y. Y., Yong, H. J., et al. (2020). Resveratrol modulates the gut microbiota and inflammation to protect against diabetic nephropathy in mice. Front. Pharmacol. 11:1249. doi: 10.3389/fphar.2020.01249
Calderon-Perez, L., Gosalbes, M. J., Yuste, S., Valls, R. M., Pedret, A., Llaurado, E., et al. (2020). Gut metagenomic and short chain fatty acids signature in hypertension: a cross-sectional study. Sci. Rep. 10:6436. doi: 10.1038/s41598-020-63475-w
Campbell, C. L., Yu, R., Li, F., Zhou, Q., Chen, D., Qi, C., et al. (2019). Modulation of fat metabolism and gut microbiota by resveratrol on high-fat diet-induced obese mice. Diabetes Metab. Syndr. Obes. 12, 97–107. doi: 10.2147/DMSO.S192228
Chen, P. B., Black, A. S., Sobel, A. L., Zhao, Y., Mukherjee, P., Molparia, B., et al. (2020). Directed remodeling of the mouse gut microbiome inhibits the development of atherosclerosis. Nat. Biotechnol. 38, 1288–1297. doi: 10.1038/s41587-020-0549-5
Chen, P. Y., Li, S., Koh, Y. C., Wu, J. C., Yang, M. J., Ho, C. T., et al. (2019). Oolong tea extract and citrus peel polymethoxyflavones reduce transformation of L-carnitine to trimethylamine-N-oxide and decrease vascular inflammation in L-carnitine feeding mice. J. Agric. Food Chem. 67, 7869–7879. doi: 10.1021/acs.jafc.9b03092
Chen, J., Wang, Y., Zhu, T., Yang, S., Cao, J., Li, X., et al. (2020). Beneficial regulatory effects of polymethoxyflavone-rich fraction from Ougan (Citrus Reticulata cv. Suavissima) fruit on gut microbiota and identification of its intestinal metabolites in mice. Antioxidants (Basel). 9:831. doi: 10.3390/antiox9090831
Chen, M. L., Yi, L., Zhang, Y., Zhou, X., Ran, L., Yang, J., et al. (2016). Resveratrol attenuates trimethylamine-N-oxide (TMAO)-induced atherosclerosis by regulating TMAO synthesis and bile acid metabolism via remodeling of the gut microbiota. mBio 7, e02210–e02215. doi: 10.1128/mBio.02210-15
Chen, M. L., Zhu, X. H., Ran, L., Lang, H. D., Yi, L., and Mi, M. T. (2017). Trimethylamine-N-oxide induces vascular inflammation by activating the NLRP3 inflammasome through the Sirt3-Sod2-mtROS signaling pathway. J. Am. Heart Assoc. 6. doi: 10.1161/JAHA.117.006347
Chiang, J. Y. (2013). Bile acid metabolism and signaling. Compr. Physiol. 3, 1191–1212. doi: 10.1002/cphy.c120023
Chiang, J. Y. L., and Ferrell, J. M. (2020). Targeting the gut microbiota for treating colitis: is FGF19 a magic bullet? EBioMedicine 55:102754. doi: 10.1016/j.ebiom.2020.102754
Chou, R. H., Chen, C. Y., Chen, I. C., Huang, H. L., Lu, Y. W., Kuo, C. S., et al. (2019). Trimethylamine N-oxide, circulating endothelial progenitor cells, and endothelial function in patients with stable angina. Sci. Rep. 9:4249. doi: 10.1038/s41598-019-40638-y
Chuang, H. L., Chiu, C. C., Lo, C., Hsu, C. C., Liu, J. Y., Hung, S. W., et al. (2022). Circulating gut microbiota-related metabolites influence endothelium plaque lesion formation in ApoE knockout rats. PLoS One 17:e0264934. doi: 10.1371/journal.pone.0264934
Cicero, A. F., and Baggioni, A. (2016). Berberine and its role in chronic disease. Adv. Exp. Med. Biol. 928, 27–45. doi: 10.1007/978-3-319-41334-1_2
Clemente, J. C., Manasson, J., and Scher, J. U. (2018). The role of the gut microbiome in systemic inflammatory disease. BMJ. 360:j5145. doi: 10.1136/bmj.j5145
Collot-Teixeira, S., Martin, J., McDermott-Roe, C., Poston, R., and McGregor, J. L. (2007). Cd36 and macrophages in atherosclerosis. Cardiovasc. Res. 75, 468–477. doi: 10.1016/j.cardiores.2007.03.010
Crovesy, L., Masterson, D., and Rosado, E. L. (2020). Profile of the gut microbiota of adults with obesity: a systematic review. Eur. J. Clin. Nutr. 74, 1251–1262. doi: 10.1038/s41430-020-0607-6
Cui, L., Zhao, T., Hu, H., Zhang, W., and Hua, X. (2017). Association study of gut flora in coronary heart disease through high-throughput sequencing. Biomed. Res. Int. 2017:3796359. doi: 10.1155/2017/3796359
Danesi, F., and Ferguson, L. R. (2017). Could pomegranate juice help in the control of inflammatory diseases? Nutrients 9:958. doi: 10.3390/nu9090958
de Nigris, F., Balestrieri, M. L., Williams-Ignarro, S., D'Armiento, F. P., Fiorito, C., Ignarro, L. J., et al. (2007). The influence of pomegranate fruit extract in comparison to regular pomegranate juice and seed oil on nitric oxide and arterial function in obese Zucker rats. Nitric Oxide 17, 50–54. doi: 10.1016/j.niox.2007.04.005
Deehan, E. C., Yang, C., Perez-Munoz, M. E., Nguyen, N. K., Cheng, C. C., Triador, L., et al. (2020). Precision microbiome modulation with discrete dietary fiber structures directs short-chain fatty acid production. Cell Host Microbe 27, 389–404.e6 e6. doi: 10.1016/j.chom.2020.01.006
Desai, M. S., Seekatz, A. M., Koropatkin, N. M., Kamada, N., Hickey, C. A., Wolter, M., et al. (2016). A dietary fiber-deprived gut microbiota degrades the colonic mucus barrier and enhances pathogen susceptibility. Cells 167, 1339–1353.e21 e21. doi: 10.1016/j.cell.2016.10.043
Ding, L., Chang, M., Guo, Y., Zhang, L., Xue, C., Yanagita, T., et al. (2018). Trimethylamine-N-oxide (TMAO)-induced atherosclerosis is associated with bile acid metabolism. Lipids Health Dis. 17:286. doi: 10.1186/s12944-018-0939-6
Du, Y., Li, X., Su, C., Xi, M., Zhang, X., Jiang, Z., et al. (2020). Butyrate protects against high-fat diet-induced atherosclerosis via up-regulating ABCA1 expression in apolipoprotein E-deficiency mice. Br. J. Pharmacol. 177, 1754–1772. doi: 10.1111/bph.14933
Dvorakova, M., and Landa, P. (2017). Anti-inflammatory activity of natural stilbenoids: a review. Pharmacol. Res. 124, 126–145. doi: 10.1016/j.phrs.2017.08.002
Emoto, T., Yamashita, T., Sasaki, N., Hirota, Y., Hayashi, T., So, A., et al. (2016). Analysis of gut microbiota in coronary artery disease patients: a possible link between gut microbiota and coronary artery disease. J. Atheroscler. Thromb. 23, 908–921. doi: 10.5551/jat.32672
Febbraio, M., Podrez, E. A., Smith, J. D., Hajjar, D. P., Hazen, S. L., Hoff, H. F., et al. (2000). Targeted disruption of the class B scavenger receptor Cd36 protects against atherosclerotic lesion development in mice. J. Clin. Invest. 105, 1049–1056. doi: 10.1172/JCI9259
Feng, X., Chen, W., Ni, X., Little, P. J., Xu, S., Tang, L., et al. (2021). Metformin, macrophage dysfunction and atherosclerosis. Front. Immunol. 12:682853. doi: 10.3389/fimmu.2021.682853
Feng, X., Sureda, A., Jafari, S., Memariani, Z., Tewari, D., Annunziata, G., et al. (2019). Berberine in cardiovascular and metabolic diseases: from mechanisms to therapeutics. Theranostics 9, 1923–1951. doi: 10.7150/thno.30787
Festa, J., Da Boit, M., Hussain, A., and Singh, H. (2021). Potential benefits of berry anthocyanins on vascular function. Mol. Nutr. Food Res. 65:e2100170. doi: 10.1002/mnfr.202100170
Frostegard, J. (2022). Antibodies against phosphorylcholine and protection against atherosclerosis, cardiovascular disease and chronic inflammation. Expert. Rev. Clin. Immunol. 18, 525–532. doi: 10.1080/1744666X.2022.2070475
Garcia, C., and Blesso, C. N. (2021). Antioxidant properties of anthocyanins and their mechanism of action in atherosclerosis. Free Radic. Biol. Med. 172, 152–166. doi: 10.1016/j.freeradbiomed.2021.05.040
Ghanim, H., Sia, C. L., Korzeniewski, K., Lohano, T., Abuaysheh, S., Marumganti, A., et al. (2011). A resveratrol and polyphenol preparation suppresses oxidative and inflammatory stress response to a high-fat, high-carbohydrate meal. J. Clin. Endocrinol. Metab. 96, 1409–1414. doi: 10.1210/jc.2010-1812
Gomaa, E. Z. (2020). Human gut microbiota/microbiome in health and diseases: a review. Antonie Van Leeuwenhoek 113, 2019–2040. doi: 10.1007/s10482-020-01474-7
Gomez-Zorita, S., Fernandez-Quintela, A., Lasa, A., Hijona, E., Bujanda, L., and Portillo, M. P. (2013). Effects of resveratrol on obesity-related inflammation markers in adipose tissue of genetically obese rats. Nutrition 29, 1374–1380. doi: 10.1016/j.nut.2013.04.014
Groenen, A. G., Halmos, B., Tall, A. R., and Westerterp, M. (2021). Cholesterol efflux pathways, inflammation, and atherosclerosis. Crit. Rev. Biochem. Mol. Biol. 56, 426–439. doi: 10.1080/10409238.2021.1925217
Guzzo, C., Ayer, A., Basta, S., Banfield, B. W., and Gee, K. (2012). Il-27 enhances LPS-induced proinflammatory cytokine production via upregulation of TLR4 expression and signaling in human monocytes. J. Immunol. 188, 864–873. doi: 10.4049/jimmunol.1101912
Han, Y., Hua, S., Chen, Y., Yang, W., Zhao, W., Huang, F., et al. (2021). Circulating PGLYRP1 levels as a potential biomarker for coronary artery disease and heart failure. J. Cardiovasc. Pharmacol. 77, 578–585. doi: 10.1097/FJC.0000000000000996
Han, J., Lin, H., and Huang, W. (2011). Modulating gut microbiota as an anti-diabetic mechanism of berberine. Med. Sci. Monit. 17:RA164-7. doi: 10.12659/msm.881842
Hanniman, E. A., Lambert, G., McCarthy, T. C., and Sinal, C. J. (2005). Loss of functional farnesoid X receptor increases atherosclerotic lesions in apolipoprotein E-deficient mice. J. Lipid Res. 46, 2595–2604. doi: 10.1194/jlr.M500390-JLR200
He, F., Li, J., Mu, Y., Kuruba, R., Ma, Z., Wilson, A., et al. (2006). Downregulation of endothelin-1 by farnesoid X receptor in vascular endothelial cells. Circ. Res. 98, 192–199. doi: 10.1161/01.RES.0000200400.55539.85
Herrington, W., Lacey, B., Sherliker, P., Armitage, J., and Lewington, S. (2016). Epidemiology of atherosclerosis and the potential to reduce the global burden of atherothrombotic disease. Circ. Res. 118, 535–546. doi: 10.1161/CIRCRESAHA.115.307611
Hong, E. Y., Kim, T. Y., Hong, G. U., Kang, H., Lee, J. Y., Park, J. Y., et al. (2019). Inhibitory effects of roseoside and icariside E4 isolated from a natural product mixture (No-ap) on the expression of angiotensin II receptor 1 and oxidative stress in angiotensin II-stimulated H9c2 cells. Molecules 24:414. doi: 10.3390/molecules24030414
Hu, R., Wu, S., Li, B., Tan, J., Yan, J., Wang, Y., et al. (2022). Dietary ferulic acid and vanillic acid on inflammation, gut barrier function and growth performance in lipopolysaccharide-challenged piglets. Anim. Nutr. 8, 144–152. doi: 10.1016/j.aninu.2021.06.009
Hubbard, G. P., Wolffram, S., Lovegrove, J. A., and Gibbins, J. M. (2004). Ingestion of quercetin inhibits platelet aggregation and essential components of the collagen-stimulated platelet activation pathway in humans. J. Thromb. Haemost. 2, 2138–2145. doi: 10.1111/j.1538-7836.2004.01067.x
Iglesias-Carres, L., Hughes, M. D., Steele, C. N., Ponder, M. A., Davy, K. P., and Neilson, A. P. (2021). Use of dietary phytochemicals for inhibition of trimethylamine N-oxide formation. J. Nutr. Biochem. 91:108600. doi: 10.1016/j.jnutbio.2021.108600
James, L., Gorwitz, R. J., Jones, R. C., Watson, J. T., Hageman, J. C., Jernigan, D. B., et al. (2008). Methicillin-resistant Staphylococcus aureus infections among healthy full-term newborns. Arch. Dis. Child. Fetal Neonatal Ed. 93, F40–F44. doi: 10.1136/adc.2006.104026
Ji, L., Chen, S., Gu, G., Zhou, J., Wang, W., Ren, J., et al. (2021). Exploration of crucial mediators for carotid atherosclerosis pathogenesis through integration of microbiome, metabolome, and transcriptome. Front. Physiol. 12:645212. doi: 10.3389/fphys.2021.645212
Jin, Y., Huang, H., Shu, X., Liu, Z., Lu, L., Dai, Y., et al. (2021). Peptidoglycan recognition protein 1 attenuates atherosclerosis by suppressing endothelial cell adhesion. J. Cardiovasc. Pharmacol. 78, 615–621. doi: 10.1097/FJC.0000000000001100
Jing, L., Shu-Xu, D., and Yong-Xin, R. (2022). A review: pathological and molecular biological study on atherosclerosis. Clin. Chim. Acta 531, 217–222. doi: 10.1016/j.cca.2022.04.012
Joo, H. K., Choi, S., Lee, Y. R., Lee, E. O., Park, M. S., Park, K. B., et al. (2018). Anthocyanin-rich extract from Red Chinese cabbage alleviates vascular inflammation in endothelial cells and Apo E(−/−) mice. Int. J. Mol. Sci. 19:816. doi: 10.3390/ijms19030816
Juarez-Fernandez, M., Porras, D., Petrov, P., Roman-Saguillo, S., Garcia-Mediavilla, M. V., Soluyanova, P., et al. (2021). The synbiotic combination of akkermansia muciniphila and quercetin ameliorates early obesity and NAFLD through gut microbiota reshaping and bile acid metabolism modulation. Antioxidants (Basel). 10:2001. doi: 10.3390/antiox10122001
Kaplan, M., Hayek, T., Raz, A., Coleman, R., Dornfeld, L., Vaya, J., et al. (2001). Pomegranate juice supplementation to atherosclerotic mice reduces macrophage lipid peroxidation, cellular cholesterol accumulation and development of atherosclerosis. J. Nutr. 131, 2082–2089. doi: 10.1093/jn/131.8.2082
Karlsson, F. H., Fak, F., Nookaew, I., Tremaroli, V., Fagerberg, B., Petranovic, D., et al. (2012). Symptomatic atherosclerosis is associated with an altered gut metagenome. Nat. Commun. 3:1245. doi: 10.1038/ncomms2266
Kasahara, K., Krautkramer, K. A., Org, E., Romano, K. A., Kerby, R. L., Vivas, E. I., et al. (2018). Interactions between Roseburia intestinalis and diet modulate atherogenesis in a murine model. Nat. Microbiol. 3, 1461–1471. doi: 10.1038/s41564-018-0272-x
Kashtanova, D. A., Popenko, A. S., Tkacheva, O. N., Tyakht, A. B., Alexeev, D. G., and Boytsov, S. A. (2016). Association between the gut microbiota and diet: fetal life, early childhood, and further life. Nutrition 32, 620–627. doi: 10.1016/j.nut.2015.12.037
Khoury, M. K., Yang, H., and Liu, B. (2021). Macrophage biology in cardiovascular diseases. Arterioscler. Thromb. Vasc. Biol. 41, e77–e81. doi: 10.1161/ATVBAHA.120.313584
Kim, H., Sitarik, A. R., Woodcroft, K., Johnson, C. C., and Zoratti, E. (2019). Birth mode, breastfeeding, pet exposure, and antibiotic use: associations with the gut microbiome and sensitization in children. Curr. Allergy Asthma Rep. 19:22. doi: 10.1007/s11882-019-0851-9
Kiouptsi, K., Pontarollo, G., Todorov, H., Braun, J., Jackel, S., Koeck, T., et al. (2020). Germ-free housing conditions do not affect aortic root and aortic arch lesion size of late atherosclerotic low-density lipoprotein receptor-deficient mice. Gut Microbes 11, 1809–1823. doi: 10.1080/19490976.2020.1767463
Kleveland, O., Kunszt, G., Bratlie, M., Ueland, T., Broch, K., Holte, E., et al. (2016). Effect of a single dose of the Interleukin-6 receptor antagonist tocilizumab on inflammation and troponin T release in patients with non-St-elevation myocardial infarction: a double-blind, randomized, placebo-controlled phase 2 trial. Eur. Heart J. 37, 2406–2413. doi: 10.1093/eurheartj/ehw171
Koedooder, R., Mackens, S., Budding, A., Fares, D., Blockeel, C., Laven, J., et al. (2019). Identification and evaluation of the microbiome in the female and male reproductive tracts. Hum. Reprod. Update 25, 298–325. doi: 10.1093/humupd/dmy048
Koh, A., De Vadder, F., Kovatcheva-Datchary, P., and Backhed, F. (2016). From dietary fiber to host physiology: short-chain fatty acids as key bacterial metabolites. Cells 165, 1332–1345. doi: 10.1016/j.cell.2016.05.041
Kong, P., Cui, Z. Y., Huang, X. F., Zhang, D. D., Guo, R. J., and Han, M. (2022). Inflammation and atherosclerosis: signaling pathways and therapeutic intervention. Signal Transduct. Target. Ther. 7:131. doi: 10.1038/s41392-022-00955-7
Koren, O., Spor, A., Felin, J., Fak, F., Stombaugh, J., Tremaroli, V., et al. (2011). Human oral, gut, and plaque microbiota in patients with atherosclerosis. Proc. Natl. Acad. Sci. U. S. A. 108, 4592–4598. doi: 10.1073/pnas.1011383107
Kuipers, F., Bloks, V. W., and Groen, A. K. (2014). Beyond intestinal soap–bile acids in metabolic control. Nat. Rev. Endocrinol. 10, 488–498. doi: 10.1038/nrendo.2014.60
Lai, H. C., Lin, T. L., Chen, T. W., Kuo, Y. L., Chang, C. J., Wu, T. R., et al. (2022). Gut microbiota modulates COPD pathogenesis: role of anti-inflammatory Parabacteroides Goldsteinii lipopolysaccharide. Gut 71, 309–321. doi: 10.1136/gutjnl-2020-322599
Lai, C. S., Wu, J. C., Ho, C. T., and Pan, M. H. (2015). Disease chemopreventive effects and molecular mechanisms of hydroxylated polymethoxyflavones. Biofactors 41, 301–313. doi: 10.1002/biof.1236
Laman, J. D., Schoneveld, A. H., Moll, F. L., van Meurs, M., and Pasterkamp, G. (2002). Significance of peptidoglycan, a proinflammatory bacterial antigen in atherosclerotic arteries and its association with vulnerable plaques. Am. J. Cardiol. 90, 119–123. doi: 10.1016/s0002-9149(02)02432-3
Lambert, G., Amar, M. J., Guo, G., Brewer, H. B. Jr., Gonzalez, F. J., and Sinal, C. J. (2003). The farnesoid X-receptor is an essential regulator of cholesterol homeostasis. J. Biol. Chem. 278, 2563–2570. doi: 10.1074/jbc.M209525200
Latruffe, N., Lancon, A., Frazzi, R., Aires, V., Delmas, D., Michaille, J. J., et al. (2015). Exploring new ways of regulation by resveratrol involving miRNAs, with emphasis on inflammation. Ann. N. Y. Acad. Sci. 1348, 97–106. doi: 10.1111/nyas.12819
Lavefve, L., Howard, L. R., and Carbonero, F. (2020). Berry polyphenols metabolism and impact on human gut microbiota and health. Food Funct. 11, 45–65. doi: 10.1039/c9fo01634a
Leuti, A., Fazio, D., Fava, M., Piccoli, A., Oddi, S., and Maccarrone, M. (2020). Bioactive lipids, inflammation and chronic diseases. Adv. Drug Deliv. Rev. 159, 133–169. doi: 10.1016/j.addr.2020.06.028
Li, Y. J., Chen, X., Kwan, T. K., Loh, Y. W., Singer, J., Liu, Y., et al. (2020). Dietary fiber protects against diabetic nephropathy through short-chain fatty acid-mediated activation of G protein-coupled receptors Gpr43 and Gpr109a. J. Am. Soc. Nephrol. 31, 1267–1281. doi: 10.1681/ASN.2019101029
Li, J., Jia, H., Cai, X., Zhong, H., Feng, Q., Sunagawa, S., et al. (2014). An integrated catalog of reference genes in the human gut microbiome. Nat. Biotechnol. 32, 834–841. doi: 10.1038/nbt.2942
Li, Y., Rahman, S. U., Huang, Y., Zhang, Y., Ming, P., Zhu, L., et al. (2020). Green tea polyphenols decrease weight gain, ameliorate alteration of gut microbiota, and mitigate intestinal inflammation in canines with high-fat-diet-induced obesity. J. Nutr. Biochem. 78:108324. doi: 10.1016/j.jnutbio.2019.108324
Li, X., Su, C., Jiang, Z., Yang, Y., Zhang, Y., Yang, M., et al. (2021). Berberine attenuates choline-induced atherosclerosis by inhibiting trimethylamine and trimethylamine-N-oxide production via manipulating the gut microbiome. NPJ Biofilms Microb. 7:36. doi: 10.1038/s41522-021-00205-8
Liao, Z. L., Zeng, B. H., Wang, W., Li, G. H., Wu, F., Wang, L., et al. (2016). Impact of the consumption of tea polyphenols on early atherosclerotic lesion formation and intestinal bifidobacteria in high-fat-fed Apoe(−/−) mice. Front. Nutr. 3:42. doi: 10.3389/fnut.2016.00042
Lin, L., and Zhang, J. (2017). Role of intestinal microbiota and metabolites on gut homeostasis and human diseases. BMC Immunol. 18:2. doi: 10.1186/s12865-016-0187-3
Lindskog Jonsson, A., Caesar, R., Akrami, R., Reinhardt, C., Fak Hallenius, F., Boren, J., et al. (2018). Impact of gut microbiota and diet on the development of atherosclerosis in Apoe(−/−) mice. Arterioscler. Thromb. Vasc. Biol. 38, 2318–2326. doi: 10.1161/ATVBAHA.118.311233
Lindskog Jonsson, A., Hallenius, F. F., Akrami, R., Johansson, E., Wester, P., Arnerlov, C., et al. (2017). Bacterial profile in human atherosclerotic plaques. Atherosclerosis 263, 177–183. doi: 10.1016/j.atherosclerosis.2017.06.016
Liu, X., Xie, Z., Sun, M., Wang, X., Li, J., Cui, J., et al. (2018). Plasma trimethylamine N-oxide is associated with vulnerable plaque characteristics in cad patients as assessed by optical coherence tomography. Int. J. Cardiol. 265, 18–23. doi: 10.1016/j.ijcard.2018.04.126
Liu, B., Zhang, Y., Wang, R., An, Y., Gao, W., Bai, L., et al. (2018). Western diet feeding influences gut microbiota profiles in ApoE knockout mice. Lipids Health Dis. 17:159. doi: 10.1186/s12944-018-0811-8
Luo, T., Guo, Z., Liu, D., Guo, Z., Wu, Q., Li, Q., et al. (2022). Deficiency of PSRC1 accelerates atherosclerosis by increasing TMAO production via manipulating gut microbiota and flavin monooxygenase 3. Gut Microbes 14:2077602. doi: 10.1080/19490976.2022.2077602
Ma, Y., Chen, K., Lv, L., Wu, S., and Guo, Z. (2019). Ferulic acid ameliorates nonalcoholic fatty liver disease and modulates the gut microbiota composition in high-fat diet fed Apoe(−/−) mice. Biomed. Pharmacother. 113:108753. doi: 10.1016/j.biopha.2019.108753
Magne, F., Gotteland, M., Gauthier, L., Zazueta, A., Pesoa, S., Navarrete, P., et al. (2020). The firmicutes/bacteroidetes ratio: a relevant marker of gut dysbiosis in obese patients? Nutrients 12:1474. doi: 10.3390/nu12051474
Man, A. W. C., Li, H., and Xia, N. (2020). Resveratrol and the interaction between gut microbiota and arterial remodelling. Nutrients 12:119. doi: 10.3390/nu12010119
Mathew, O. P., Ranganna, K., and Milton, S. G. (2014). Involvement of the antioxidant effect and anti-inflammatory response in butyrate-inhibited vascular smooth muscle cell proliferation. Pharmaceuticals 7, 1008–1027. doi: 10.3390/ph7111008
Mauray, A., Felgines, C., Morand, C., Mazur, A., Scalbert, A., and Milenkovic, D. (2012). Bilberry anthocyanin-rich extract alters expression of genes related to atherosclerosis development in aorta of Apo E-deficient mice. Nutr. Metab. Cardiovasc. Dis. 22, 72–80. doi: 10.1016/j.numecd.2010.04.011
Mena, P., Dominguez-Perles, R., Girones-Vilaplana, A., Baenas, N., Garcia-Viguera, C., and Villano, D. (2014). Flavan-3-Ols, anthocyanins, and inflammation. IUBMB Life 66, 745–758. doi: 10.1002/iub.1332
Michicotl-Meneses, M. M., Thompson-Bonilla, M. D. R., Reyes-Lopez, C. A., Garcia-Perez, B. E., Lopez, T. II, Ordaz-Pichardo, C., et al. (2021). Inflammation markers in adipose tissue and cardiovascular risk reduction by pomegranate juice in obesity induced by a hypercaloric diet in Wistar rats. Nutrients 13:2577. doi: 10.3390/nu13082577
Milani, C., Duranti, S., Bottacini, F., Casey, E., Turroni, F., Mahony, J., et al. (2017). The first microbial colonizers of the human gut: composition, activities, and health implications of the infant gut microbiota. Microbiol. Mol. Biol. Rev. 81:e00036-17. doi: 10.1128/MMBR.00036-17
Mitra, S., Drautz-Moses, D. I., Alhede, M., Maw, M. T., Liu, Y., Purbojati, R. W., et al. (2015). In silico analyses of metagenomes from human atherosclerotic plaque samples. Microbiome 3:38. doi: 10.1186/s40168-015-0100-y
Miyazaki-Anzai, S., Masuda, M., Levi, M., Keenan, A. L., and Miyazaki, M. (2014). Dual activation of the bile acid nuclear receptor FXR and G-protein-coupled receptor TGR5 protects mice against atherosclerosis. PLoS One 9:e108270. doi: 10.1371/journal.pone.0108270
Moludi, J., Maleki, V., Jafari-Vayghyan, H., Vaghef-Mehrabany, E., and Alizadeh, M. (2020). Metabolic endotoxemia and cardiovascular disease: a systematic review about potential roles of prebiotics and probiotics. Clin. Exp. Pharmacol. Physiol. 47, 927–939. doi: 10.1111/1440-1681.13250
Montarello, N. J., Nguyen, M. T., Wong, D. T. L., Nicholls, S. J., and Psaltis, P. J. (2022). Inflammation in coronary atherosclerosis and its therapeutic implications. Cardiovasc. Drugs Ther. 36, 347–362. doi: 10.1007/s10557-020-07106-6
Moriya, J. (2019). Critical roles of inflammation in atherosclerosis. J. Cardiol. 73, 22–27. doi: 10.1016/j.jjcc.2018.05.010
Moroni, F., Ammirati, E., Norata, G. D., Magnoni, M., and Camici, P. G. (2019). The role of monocytes and macrophages in human atherosclerosis, plaque neoangiogenesis, and atherothrombosis. Mediat. Inflamm. 2019:7434376. doi: 10.1155/2019/7434376
Mosele, J. I., Gosalbes, M. J., Macia, A., Rubio, L., Vazquez-Castellanos, J. F., Jimenez Hernandez, N., et al. (2015). Effect of daily intake of pomegranate juice on fecal microbiota and feces metabolites from healthy volunteers. Mol. Nutr. Food Res. 59, 1942–1953. doi: 10.1002/mnfr.201500227
Neto-Neves, E. M., da Silva Maia Bezerra Filho, C., Dejani, N. N., and de Sousa, D. P. (2021). Ferulic acid and cardiovascular health: therapeutic and preventive potential. Mini Rev. Med. Chem. 21, 1625–1637. doi: 10.2174/1389557521666210105122841
Neyrinck, A. M., Catry, E., Taminiau, B., Cani, P. D., Bindels, L. B., Daube, G., et al. (2019). Chitin-glucan and pomegranate polyphenols improve endothelial dysfunction. Sci. Rep. 9:14150. doi: 10.1038/s41598-019-50700-4
Nidorf, S. M., Fiolet, A. T. L., Mosterd, A., Eikelboom, J. W., Schut, A., Opstal, T. S. J., et al. (2020). Colchicine in patients with chronic coronary disease. N. Engl. J. Med. 383, 1838–1847. doi: 10.1056/NEJMoa2021372
Nie, J., Zhang, L., Zhao, G., and Du, X. (2019). Quercetin reduces atherosclerotic lesions by altering the gut microbiota and reducing atherogenic lipid metabolites. J. Appl. Microbiol. 127, 1824–1834. doi: 10.1111/jam.14441
Olholm, J., Paulsen, S. K., Cullberg, K. B., Richelsen, B., and Pedersen, S. B. (2010). Anti-inflammatory effect of resveratrol on adipokine expression and secretion in human adipose tissue explants. Int. J. Obes. 34, 1546–1553. doi: 10.1038/ijo.2010.98
Papandreou, C., More, M., and Bellamine, A. (2020). Trimethylamine N-oxide in relation to cardiometabolic health-cause or effect? Nutrients 12:1330. doi: 10.3390/nu12051330
Parseus, A., Sommer, N., Sommer, F., Caesar, R., Molinaro, A., Stahlman, M., et al. (2017). Microbiota-induced obesity requires farnesoid X receptor. Gut 66, 429–437. doi: 10.1136/gutjnl-2015-310283
Porras, D., Nistal, E., Martinez-Florez, S., Pisonero-Vaquero, S., Olcoz, J. L., Jover, R., et al. (2017). Protective effect of quercetin on high-fat diet-induced non-alcoholic fatty liver disease in mice is mediated by modulating intestinal microbiota imbalance and related gut-liver axis activation. Free Radic. Biol. Med. 102, 188–202. doi: 10.1016/j.freeradbiomed.2016.11.037
Qin, J., Li, R., Raes, J., Arumugam, M., Burgdorf, K. S., Manichanh, C., et al. (2010). A human gut microbial gene catalogue established by metagenomic sequencing. Nature 464, 59–65. doi: 10.1038/nature08821
Ranganna, K., Yousefipour, Z., Yatsu, F. M., Milton, S. G., and Hayes, B. E. (2003). Gene expression profile of butyrate-inhibited vascular smooth muscle cell proliferation. Mol. Cell. Biochem. 254, 21–36. doi: 10.1023/a:1027383710582
Rath, S., Heidrich, B., Pieper, D. H., and Vital, M. (2017). Uncovering the trimethylamine-producing bacteria of the human gut microbiota. Microbiome 5:54. doi: 10.1186/s40168-017-0271-9
Rautava, S. (2017). Microbial composition of the initial colonization of newborns. Nestle Nutr. Inst. Workshop Ser. 88, 11–21. doi: 10.1159/000455209
Ridker, P. M., Everett, B. M., Pradhan, A., Mac Fadyen, J. G., Solomon, D. H., Zaharris, E., et al. (2019). Low-dose methotrexate for the prevention of atherosclerotic events. N. Engl. J. Med. 380, 752–762. doi: 10.1056/NEJMoa1809798
Ridker, P. M., Everett, B. M., Thuren, T., Mac Fadyen, J. G., Chang, W. H., Ballantyne, C., et al. (2017). Antiinflammatory therapy with canakinumab for atherosclerotic disease. N. Engl. J. Med. 377, 1119–1131. doi: 10.1056/NEJMoa1707914
Roberts, L. M., and Buford, T. W. (2021). Lipopolysaccharide binding protein is associated with CVD risk in older adults. Aging Clin. Exp. Res. 33, 1651–1658. doi: 10.1007/s40520-020-01684-z
Rom, O., Korach-Rechtman, H., Hayek, T., Danin-Poleg, Y., Bar, H., Kashi, Y., et al. (2017). Acrolein increases macrophage atherogenicity in association with gut microbiota remodeling in atherosclerotic mice: protective role for the polyphenol-rich pomegranate juice. Arch. Toxicol. 91, 1709–1725. doi: 10.1007/s00204-016-1859-8
Roth, G. A., Mensah, G. A., Johnson, C. O., Addolorato, G., Ammirati, E., Baddour, L. M., et al. (2020). Global burden of cardiovascular diseases and risk factors, 1990–2019: update from the Gbd 2019 study. J. Am. Coll. Cardiol. 76, 2982–3021. doi: 10.1016/j.jacc.2020.11.010
Roy, P., Orecchioni, M., and Ley, K. (2022). How the immune system shapes atherosclerosis: roles of innate and adaptive immunity. Nat. Rev. Immunol. 22, 251–265. doi: 10.1038/s41577-021-00584-1
Sanchez-Rodriguez, E., Egea-Zorrilla, A., Plaza-Díaz, J., Aragón-Vela, J., Muñoz-Quezada, S., Tercedor-Sánchez, L., et al. (2020). The gut microbiota and its implication in the development of atherosclerosis and related cardiovascular diseases. Nutrients 12:605. doi: 10.3390/nu12030605
Seldin, M. M., Meng, Y., Qi, H., Zhu, W., Wang, Z., Hazen, S. L., et al. (2016). Trimethylamine N-oxide promotes vascular inflammation through signaling of mitogen-activated protein kinase and nuclear factor-kappab. J. Am. Heart Assoc. 5:e002767. doi: 10.1161/JAHA.115.002767
Senthong, V., Wang, Z., Li, X. S., Fan, Y., Wu, Y., Tang, W. H., et al. (2016). Intestinal microbiota-generated metabolite trimethylamine-N-oxide and 5-year mortality risk in stable coronary artery disease: the contributory role of intestinal microbiota in a courage-like patient cohort. J. Am. Heart Associat. 5:e002816. doi: 10.1161/JAHA.115.002816
Shanahan, F., Ghosh, T. S., and O'Toole, P. W. (2021). The healthy microbiome-what is the definition of a healthy gut microbiome? Gastroenterology 160, 483–494. doi: 10.1053/j.gastro.2020.09.057
Shen, X., Li, L., Sun, Z., Zang, G., Zhang, L., Shao, C., et al. (2021). Gut microbiota and atherosclerosis-focusing on the plaque stability. Front. Cardiovasc. Med. 8:668532. doi: 10.3389/fcvm.2021.668532
Shi, T., Bian, X., Yao, Z., Wang, Y., Gao, W., and Guo, C. (2020). Quercetin improves gut dysbiosis in antibiotic-treated mice. Food Funct. 11, 8003–8013. doi: 10.1039/d0fo01439g
Shi, Y., Hu, J., Geng, J., Hu, T., Wang, B., Yan, W., et al. (2018). Berberine treatment reduces atherosclerosis by mediating gut microbiota in Apoe−/− mice. Biomed. Pharmacother. 107, 1556–1563. doi: 10.1016/j.biopha.2018.08.148
Si, X., Bi, J., Chen, Q., Cui, H., Bao, Y., Tian, J., et al. (2021). Effect of blueberry anthocyanin-rich extracts on peripheral and hippocampal antioxidant defensiveness: the analysis of the serum fatty acid species and gut microbiota profile. J. Agric. Food Chem. 69, 3658–3666. doi: 10.1021/acs.jafc.0c07637
Song, D., Hao, J., and Fan, D. (2020). Biological properties and clinical applications of berberine. Front. Med. 14, 564–582. doi: 10.1007/s11684-019-0724-6
Szabo, H., Hernyes, A., Piroska, M., Ligeti, B., Fussy, P., Zoldi, L., et al. (2021, 3). Association between gut microbial diversity and carotid intima-media thickness. Medicina 57:195. doi: 10.3390/medicina57030195
Tan, X., Liu, Y., Long, J., Chen, S., Liao, G., Wu, S., et al. (2019). Trimethylamine N-oxide aggravates liver steatosis through modulation of bile acid metabolism and inhibition of farnesoid X receptor signaling in nonalcoholic fatty liver disease. Mol. Nutr. Food Res. 63:e1900257. doi: 10.1002/mnfr.201900257
Tan, Y., Sheng, Z., Zhou, P., Liu, C., Zhao, H., Song, L., et al. (2019). Plasma trimethylamine N-oxide as a novel biomarker for plaque rupture in patients with St-segment-elevation myocardial infarction. Circ. Cardiovasc. Interv. 12:e007281. doi: 10.1161/CIRCINTERVENTIONS.118.007281
Tang, W. H. W., Li, X. S., Wu, Y., Wang, Z., Khaw, K. T., Wareham, N. J., et al. (2021). Plasma trimethylamine N-oxide (TMAO) levels predict future risk of coronary artery disease in apparently healthy individuals in the epic-norfolk prospective population study. Am. Heart J. 236, 80–86. doi: 10.1016/j.ahj.2021.01.020
Tardif, J. C., Kouz, S., Waters, D. D., Bertrand, O. F., Diaz, R., Maggioni, A. P., et al. (2019). Efficacy and safety of low-dose colchicine after myocardial infarction. N. Engl. J. Med. 381, 2497–2505. doi: 10.1056/NEJMoa1912388
Thompson, R. A., Morello, D. R., Panicker, S., Toske, S. G., and Li, L. (2022). Carbon and nitrogen isotopic analysis of morphine from opium and heroin samples originating in the four major heroin producing regions. Drug Test. Anal. 14, 505–513. doi: 10.1002/dta.3194
Torres, N., Guevara-Cruz, M., Velazquez-Villegas, L. A., and Tovar, A. R. (2015). Nutrition and atherosclerosis. Arch. Med. Res. 46, 408–426. doi: 10.1016/j.arcmed.2015.05.010
Uyanga, V. A., Amevor, F. K., Liu, M., Cui, Z., Zhao, X., and Lin, H. (2021). Potential implications of citrulline and quercetin on gut functioning of monogastric animals and humans: a comprehensive review. Nutrients 13:3782. doi: 10.3390/nu13113782
Verhaar, B. J. H., Collard, D., Prodan, A., Levels, J. H. M., Zwinderman, A. H., Backhed, F., et al. (2020). Associations between gut microbiota, faecal short-chain fatty acids, and blood pressure across ethnic groups: the helius study. Eur. Heart J. 41, 4259–4267. doi: 10.1093/eurheartj/ehaa704
Virani, S. S., Alonso, A., Aparicio, H. J., Benjamin, E. J., Bittencourt, M. S., Callaway, C. W., et al. (2021). Heart disease and stroke statistics-2021 update: a report from the American Heart Association. Circulation 143, e254–e743. doi: 10.1161/CIR.0000000000000950
Vourakis, M., Mayer, G., and Rousseau, G. (2021). The role of gut microbiota on cholesterol metabolism in atherosclerosis. Int. J. Mol. Sci. 22:8074. doi: 10.3390/ijms22158074
Wang, P., Li, D., Ke, W., Liang, D., Hu, X., and Chen, F. (2020). Resveratrol-induced gut microbiota reduces obesity in high-fat diet-fed mice. Int. J. Obes. 44, 213–225. doi: 10.1038/s41366-019-0332-1
Wang, P., Ma, Y., Wang, D., Zhao, W., Hu, X., Chen, F., et al. (2022). Protective effects of dietary resveratrol against chronic low-grade inflammation mediated through the gut microbiota in high-fat diet mice. Nutrients 14:1994. doi: 10.3390/nu14101994
Wang, D., Xia, M., Yan, X., Li, D., Wang, L., Xu, Y., et al. (2012). Gut microbiota metabolism of anthocyanin promotes reverse cholesterol transport in mice via repressing Mirna-10b. Circ. Res. 111, 967–981. doi: 10.1161/CIRCRESAHA.112.266502
Wang, L., Zeng, B., Liu, Z., Liao, Z., Zhong, Q., Gu, L., et al. (2018). Green tea polyphenols modulate colonic microbiota diversity and lipid metabolism in high-fat diet treated HFA mice. J. Food Sci. 83, 864–873. doi: 10.1111/1750-3841.14058
Wenzel, T. J., Gates, E. J., Ranger, A. L., and Klegeris, A. (2020). Short-chain fatty acids (SCFAs) alone or in combination regulate select immune functions of microglia-like cells. Mol. Cell. Neurosci. 105:103493. doi: 10.1016/j.mcn.2020.103493
Whitman, S. C., Kurowska, E. M., Manthey, J. A., and Daugherty, A. (2005). Nobiletin, a citrus flavonoid isolated from tangerines, selectively inhibits class A scavenger receptor-mediated metabolism of acetylated LDL by mouse macrophages. Atherosclerosis 178, 25–32. doi: 10.1016/j.atherosclerosis.2004.07.034
Wick, G., Jakic, B., Buszko, M., Wick, M. C., and Grundtman, C. (2014). The role of heat shock proteins in atherosclerosis. Nat. Rev. Cardiol. 11, 516–529. doi: 10.1038/nrcardio.2014.91
Wick, G., Knoflach, M., and Xu, Q. (2004). Autoimmune and inflammatory mechanisms in atherosclerosis. Annu. Rev. Immunol. 22, 361–403. doi: 10.1146/annurev.immunol.22.012703.104644
Wiedermann, C. J., Kiechl, S., Dunzendorfer, S., Schratzberger, P., Egger, G., Oberhollenzer, F., et al. (1999). Association of endotoxemia with carotid atherosclerosis and cardiovascular disease: prospective results from the Bruneck study. J. Am. Coll. Cardiol. 34, 1975–1981. doi: 10.1016/s0735-1097(99)00448-9
Wu, M., Yang, S., Wang, S., Cao, Y., Zhao, R., Li, X., et al. (2020). Effect of berberine on atherosclerosis and gut microbiota modulation and their correlation in high-fat diet-fed ApoE−/− mice. Front. Pharmacol. 11:223. doi: 10.3389/fphar.2020.00223
Wu, K., Yuan, Y., Yu, H., Dai, X., Wang, S., Sun, Z., et al. (2020). The gut microbial metabolite trimethylamine N-oxide aggravates GVHD by inducing M1 macrophage polarization in mice. Blood 136, 501–515. doi: 10.1182/blood.2019003990
Yan, N., Yan, T., Xia, Y., Hao, H., Wang, G., and Gonzalez, F. J. (2021). The pathophysiological function of non-gastrointestinal farnesoid X receptor. Pharmacol. Ther. 226:107867. doi: 10.1016/j.pharmthera.2021.107867
Yang, S., Li, D., Yu, Z., Li, Y., and Wu, M. (2021). Multi-pharmacology of berberine in atherosclerosis and metabolic diseases: potential contribution of gut microbiota. Front. Pharmacol. 12:709629. doi: 10.3389/fphar.2021.709629
Yang, G., Lin, C. C., Yang, Y., Yuan, L., Wang, P., Wen, X., et al. (2019). Nobiletin prevents trimethylamine oxide-induced vascular inflammation via inhibition of the NF-Kappab/Mapk pathways. J. Agric. Food Chem. 67, 6169–6176. doi: 10.1021/acs.jafc.9b01270
Yang, W., Yu, T., Huang, X., Bilotta, A. J., Xu, L., Lu, Y., et al. (2020). Intestinal microbiota-derived short-chain fatty acids regulation of immune cell Il-22 production and gut immunity. Nat. Commun. 11:4457. doi: 10.1038/s41467-020-18262-6
Ye, Y., Warusawitharana, H., Zhao, H., Liu, Z., Li, B., Wu, Y., et al. (2022). Tea polyphenols attenuates inflammation via reducing lipopolysaccharides level and inhibiting Tlr4/Nf-Kappab pathway in obese mice. Plant Foods Hum. Nutr. 77, 105–111. doi: 10.1007/s11130-021-00937-0
Yen, G. C., Chen, Y. C., Chang, W. T., and Hsu, C. L. (2011). Effects of polyphenolic compounds on tumor necrosis factor-alpha (TNF-alpha)-induced changes of adipokines and oxidative stress in 3t3-L1 adipocytes. J. Agric. Food Chem. 59, 546–551. doi: 10.1021/jf1036992
Yen, J. H., Weng, C. Y., Li, S., Lo, Y. H., Pan, M. H., Fu, S. H., et al. (2011). Citrus flavonoid 5-demethylnobiletin suppresses scavenger receptor expression in THP-1 cells and alters lipid homeostasis in HepG2 liver cells. Mol. Nutr. Food Res. 55, 733–748. doi: 10.1002/mnfr.201000226
Yin, X., Liu, W., Chen, H., Qi, C., Chen, H., Niu, H., et al. (2022). Effects of ferulic acid on muscle development and intestinal microbiota of zebrafish. J. Anim. Physiol. Anim. Nutr. 106, 429–440. doi: 10.1111/jpn.13631
Yoshida, N., Yamashita, T., Kishino, S., Watanabe, H., Sasaki, K., Sasaki, D., et al. (2020). A possible beneficial effect of Bacteroides on faecal lipopolysaccharide activity and cardiovascular diseases. Sci. Rep. 10:13009. doi: 10.1038/s41598-020-69983-z
Yu, W. N., Wang, L. H., and Cheng, H. W. (2018). Regulatory analysis on the medical use of ephedrine-related products in Taiwan. J. Food Drug Anal. 26, 481–486. doi: 10.1016/j.jfda.2017.11.002
Zhang, L., and Chu, C. Q. (2021). Gut microbiota-medication interaction in rheumatic diseases. Front. Immunol. 12:796865. doi: 10.3389/fimmu.2021.796865
Zhang, Q., He, F., Kuruba, R., Gao, X., Wilson, A., Li, J., et al. (2008). FXR-mediated regulation of angiotensin type 2 receptor expression in vascular smooth muscle cells. Cardiovasc. Res. 77, 560–569. doi: 10.1093/cvr/cvm068
Zhang, X., Miao, F., Niu, L., Wei, Y., Hu, Y., Lian, X., et al. (2021). Berberine carried gelatin/sodium alginate hydrogels with antibacterial and EDTA-induced detachment performances. Int. J. Biol. Macromol. 181, 1039–1046. doi: 10.1016/j.ijbiomac.2021.04.114
Zhang, W., Xu, J. H., Yu, T., and Chen, Q. K. (2019). Effects of berberine and metformin on intestinal inflammation and gut microbiome composition in Db/Db mice. Biomed. Pharmacother. 118:109131. doi: 10.1016/j.biopha.2019.109131
Zhang, X., Zhao, Y., Zhang, M., Pang, X., Xu, J., Kang, C., et al. (2012). Structural changes of gut microbiota during berberine-mediated prevention of obesity and insulin resistance in high-fat diet-fed rats. PLoS One 7:e42529. doi: 10.1371/journal.pone.0042529
Zhao, S., Li, J., Wang, L., and Wu, X. (2016). Pomegranate peel polyphenols inhibit lipid accumulation and enhance cholesterol efflux in raw264.7 macrophages. Food Funct. 7, 3201–3210. doi: 10.1039/c6fo00347h
Zhao, Z., Ning, J., Bao, X. Q., Shang, M., Ma, J., Li, G., et al. (2021). Fecal microbiota transplantation protects rotenone-induced Parkinson's disease mice via suppressing inflammation mediated by the lipopolysaccharide-TLR4 signaling pathway through the microbiota-gut-brain axis. Microbiome 9:226. doi: 10.1186/s40168-021-01107-9
Zhao, X., Oduro, P. K., Tong, W., Wang, Y., Gao, X., and Wang, Q. (2021). Therapeutic potential of natural products against atherosclerosis: targeting on gut microbiota. Pharmacol. Res. 163:105362. doi: 10.1016/j.phrs.2020.105362
Zhao, L., Zhang, Q., Ma, W., Tian, F., Shen, H., and Zhou, M. (2017). A combination of quercetin and resveratrol reduces obesity in high-fat diet-fed rats by modulation of gut microbiota. Food Funct. 8, 4644–4656. doi: 10.1039/c7fo01383c
Zhao, L., Zhu, X., Xia, M., Li, J., Guo, A. Y., Zhu, Y., et al. (2021). Quercetin ameliorates gut microbiota dysbiosis that drives hypothalamic damage and hepatic lipogenesis in monosodium glutamate-induced abdominal obesity. Front. Nutr. 8:671353. doi: 10.3389/fnut.2021.671353
Zheng, X., Huang, F., Zhao, A., Lei, S., Zhang, Y., Xie, G., et al. (2017). Bile acid is a significant host factor shaping the gut microbiome of diet-induced obese mice. BMC Biol. 15:120. doi: 10.1186/s12915-017-0462-7
Zheng, L., Zheng, J., Xie, Y., Li, Z., Guo, X., Sun, G., et al. (2019). Serum gut microbe-dependent trimethylamine N-oxide improves the prediction of future cardiovascular disease in a community-based general population. Atherosclerosis 280, 126–131. doi: 10.1016/j.atherosclerosis.2018.11.010
Zhou, R., Gao, J., Xiang, C., Liu, Z., Zhang, Y., Zhang, J., et al. (2020). Salvianolic acid a attenuated myocardial infarction-induced apoptosis and inflammation by activating Trx. Naunyn Schmiedeberg's Arch. Pharmacol. 393, 991–1002. doi: 10.1007/s00210-019-01766-4
Zhou, F., Li, Y. L., Zhang, X., Wang, K. B., Huang, J. A., Liu, Z. H., et al. (2021). Polyphenols from Fu Brick tea reduce obesity via modulation of gut microbiota and gut microbiota-related intestinal oxidative stress and barrier function. J. Agric. Food Chem. 69, 14530–14543. doi: 10.1021/acs.jafc.1c04553
Zhou, W., and Wang, Y. (2014). A network-based analysis of the types of coronary artery disease from traditional Chinese medicine perspective: potential for therapeutics and drug discovery. J. Ethnopharmacol. 151, 66–77. doi: 10.1016/j.jep.2013.11.007
Zhu, L., and Chen, L. (2019). Progress in research on paclitaxel and tumor immunotherapy. Cell. Mol. Biol. Lett. 24:40. doi: 10.1186/s11658-019-0164-y
Zhu, Q., Gao, R., Zhang, Y., Pan, D., Zhu, Y., Zhang, X., et al. (2018). Dysbiosis signatures of gut microbiota in coronary artery disease. Physiol. Genomics 50, 893–903. doi: 10.1152/physiolgenomics.00070.2018
Zhu, Y., Li, Q., and Jiang, H. (2020). Gut microbiota in atherosclerosis: focus on trimethylamine N-oxide. APMIS 128, 353–366. doi: 10.1111/apm.13038
Zhu, L., Zhang, D., Zhu, H., Zhu, J., Weng, S., Dong, L., et al. (2018). Berberine treatment increases Akkermansia in the gut and improves high-fat diet-induced atherosclerosis in Apoe(−/−) mice. Atherosclerosis 268, 117–126. doi: 10.1016/j.atherosclerosis.2017.11.023
Zlotos, D. P., Mandour, Y. M., and Jensen, A. A. (2022). Strychnine and its mono- and dimeric analogues: a pharmaco-chemical perspective. Nat. Prod. Rep. 39, 1910–1937. doi: 10.1039/d1np00079a
Glossary
Keywords: gut microbiota, atherosclerosis, inflammation, natural products, immune
Citation: Deng B, Tao L and Wang Y (2022) Natural products against inflammation and atherosclerosis: Targeting on gut microbiota. Front. Microbiol. 13:997056. doi: 10.3389/fmicb.2022.997056
Edited by:
Christoph Reinhardt, Johannes Gutenberg University Mainz, GermanyReviewed by:
Latifa Bousarghin, University of Rennes 1, FranceJia Qing Yun, Linyi People's Hospital, China
Copyright © 2022 Deng, Tao and Wang. This is an open-access article distributed under the terms of the Creative Commons Attribution License (CC BY). The use, distribution or reproduction in other forums is permitted, provided the original author(s) and the copyright owner(s) are credited and that the original publication in this journal is cited, in accordance with accepted academic practice. No use, distribution or reproduction is permitted which does not comply with these terms.
*Correspondence: Yiru Wang, wangyiruenen@shutcm.edu.cn; Liyu Tao, taoliyu37@163.com