- 1School of Medicine, Lishui University, Lishui, China
- 2Key Laboratory of Medical Genetics of Zhejiang Province, Key Laboratory of Laboratory Medicine, Ministry of Education of China, School of Laboratory Medicine and Life Sciences, Wenzhou Medical University, Wenzhou, China
- 3Medical Molecular Biology Laboratory, School of Medicine, Jinhua Polytechnic, Jinhua, China
- 4The People’s Hospital of Yuhuan, Yuhuan, China
- 5Department of Clinical Laboratory, Hospital of Ningbo University School of Medicine, Ningbo, Zhejiang, China
Introduction: Lelliottia amnigena, a bacterium usually isolated from natural environments, may cause human infections and has been suggested to be naturally resistant to second- and third-generation cephalosporins.
Methods: In this study, we determined the whole-genome sequence of an isolate, L. Amnigena P13, isolated from animal farm sewage. On the basis of genome sequence analysis, susceptibility testing, molecular cloning, and enzyme kinetic parameter analysis, we identified a novel chromosome-encoded AmpC β-lactamase, LAQ-1.
Results and Discussion: blaLAQ-1 is resistant to penicillin G, ampicillin, and several first- to fourth-generation cephalosporins, such as cefazolin, cefoxitin and cefepime. The MIC levels of some β-lactams, such as cefoxitin, cefepime, aztreonam and cefazolin, for the recombinant clone (pUCP24-blaLAQ-1/DH5α) increased by approximately 4- to 64-fold compared with those of the control strain (pUCP24/DH5α). The kinetic properties of LAQ-1, with the highest catalytic activity observed toward piperacillin, were basically the same as those of typical class C β-lactamases, and avibactam had a strong inhibitory effect on its hydrolytic activity. The genetic background of blaLAQ-1 was relatively conserved, and no mobile genetic element (MGE) was found around it. The plasmid pP13-67 of L. amnigena P13 harbored 12 resistance genes [qnrS1, aph(6)-Id, aadA2, sul1, sul2,blaTEM-1, qacEΔ1, dfrA12, tetA and floR] related to different mobile genetic elements within an ~22 kb multidrug resistance region. The multidrug resistance region shared the highest nucleotide sequence similarities with those of the chromosomes or plasmids of different bacterial species, indicating the possibility of horizontal transfer of these resistance genes among different bacterial species.
Introduction
In 1981, Enterobacter H3 was renamed Enterobacter amnigenus based on molecular hybridization (Izard et al., 1981). It was then proposed that the bacterium could be divided into two groups based on the molecular hybridization data (Izard et al., 1981). Subsequently, according to biochemical experiments, E. amnigenus was classified into two biogroups (Casin et al., 1986): Strains in Biogroup 1 ferment sucrose and raffinose but not D-sorbitol. In contrast, those in Biogroup 2 ferment D-sorbitol but not sucrose or raffinose (Rd et al., 1985). In 2013, E. amnigenus and E. nimipressuralis were reclassified as belonging to a new genus of the family Enterobacteriaceae designated Lelliottia based on multilocus sequence typing (MLST), DNA molecular hybridization, phenotypic sugar fermentation characteristics and cell wall fatty acid spectrum analysis (Brady et al., 2013). E. amnigenus was renamed Lelliottia amnigena and E. nimipressuralis was reclassified as Lelliottia nimipressuralis. Members of the genus Lelliottia are rod-shaped, motile and facultative anaerobic Gram-negative bacilli (Liu and Tang, 2016). L. amnigena was suspected to be pathogenic in humans since it was first isolated in nature, which was confirmed when it was isolated in samples from at least four patients whose clinical laboratory results indicated (Martin Guerra et al., 2018). Lelliottia amnigena can be isolated from a variety of environmental sources (e.g., soil, river/lake; Rd et al., 1985), food (e.g., milk, mushrooms, artisanal raw cheeses) (Yuk et al., 2018) and human clinical samples (e.g., respiratory tract, wound, blood, and stool samples). Moreover, it is an uncommon/unusual pathogen capable of causing sporadic reports of pyonephrosis, urinary tract infection, sepsis and endophthalmitis (Bollet et al., 1991; Westerfeld et al., 2009; Leal-Negredo et al., 2017). The natural resistance of L. amnigena to second- and third-generation cephalosporins such as cefoxitin, cefotaxime and cefaclor was described in the most extensive study of susceptibility (Murugaiyan et al., 2015). The research also showed that some strains of L. amnigena were resistant to gentamicin, doxycycline, nitrofurantoin and β-lactam/β-lactamase inhibitor combinations (such as amoxicillin/clavulanic; Fadare and Okoh, 2021). However, contradictory result had been reported that no natural resistance to cephalosporins, including cefoxitin, yet a decreased susceptibility to cefixime, cefpodoxime and ceftibutene was found (Stock and Wiedemann, 2002). To date, several studies have reported that L. amnigena possesses the ampC gene. However, no detailed studies on the L. amnigena AmpC β-lactamase have been documented (Literak et al., 2014).
β-lactamase is an enzyme that can hydrolyze the β-lactam ring and inactivate antibiotics before they bind to penicillin-binding proteins (Knotthunziker et al., 1982). AmpC β-lactamase (AmpC) is a serine-active protease and one of the important β-lactamases. In the Ambler molecular structure classification, it belongs to class C (Bush et al., 1995), with an apparent molecular weight of 38 ~ 42 kDa, and is distributed mainly in periplasm (Medeiros, 1989). Overexpression of the chromosomally encoded AmpC enzyme is the main mechanism underlying the resistance of Gram-negative bacilli to penicillin, cephalosporin, cephamycin and monocyclic β-lactam antibiotics (Jacoby, 2009). At the same time, the AmpC enzyme encoded by chromosomal and plasmid genes is also evolving (Hanson, 2003). Overexpression of this enzyme confers resistance to a wide range of cephalosporins, including cefotaxime, ceftazidime and ceftriaxone. Some organisms even show mutations that reduce influx (loss of outer membrane porin) or enhance efflux (activation of efflux pump; Hanson, 2002). Common β-lactamase inhibitors such as clavulanic acid, sulbactam and tazobactam had no obvious inhibitory effect on the AmpC enzyme, but avibactam had an inhibitory effect on the AmpC enzyme (Bush et al., 1993). Although the AmpC enzyme causes less resistance than extended-spectrum β-lactamases (ESBLs) in most parts of the world, it is more difficult to detect and has a wider range. Therefore, more in-depth research on AmpC will be of great significance for guiding clinical testing and follow-up medication.
In this study, we determined the whole-genome sequence of an isolate, L. amnigena P13, isolated from animal farm sewage. On the basis of genome sequence analysis, susceptibility testing, molecular cloning, and enzyme kinetic parameter analysis, we identified a novel chromosome-encoded AmpC β-lactamase, LAQ-1.
Materials and methods
Bacterial strains
Lelliottia amnigena P13 was isolated from sewage discharged from an animal farm in Wenzhou, Zhejiang Province, China. The strain was identified by a bioMérieux VITEK 2 compact instrument (bioMérieux, Marcy l’Etoile, France) and then verified by 16S rRNA gene homology and average nucleotide identity (ANI) analyses. The bacteria used in this work are listed in Table 1.
Antimicrobial susceptibility testing
According to the guidelines of the Clinical and Laboratory Standards Institute (CLSI), the minimum inhibitory concentrations (MICs) were determined by the agar dilution method. Drug susceptibility was determined according to the CLSI drug susceptibility test standard (CLSI, 2021) and the guidelines of the European Enterobacteriaceae Antimicrobial Susceptibility Test Committee (EUCAST, 2019). E. coli ATCC 25922 was used as the reference strain for quality control.
Whole-genome sequencing and functional annotation of the genome sequence
The whole-genome DNA of L. amnigena P13 was extracted with the AxyPrep Bacterial Genomic DNA Miniprep Kit (Axygen Science, Union City, CA, United States) and sequenced with the Illumina HiSeq 2,500 (Shanghai, China) and PacBio RS II (Pacific Biosciences) platforms. The PacBio long reads were initially assembled by Canu (Koren et al., 2017), and then two FASTQ sequence files generated by the Illumina sequencer were mapped onto the primary assembly to control assembly quality and to correct possibly misidentified bases using Pilon (Walker et al., 2014). Prokka was used to predict the potential open reading frames (ORFs; Seemann, 2014) of the assembled genome, and the functional annotation of these proteins was performed by DIAMOND (Buchfink et al., 2021) with an e-value threshold of 1e-5 against the nonredundant protein sequence (NR) database of the National Center for Biotechnology Information (NCBI). The drug resistance genes were annotated by drug resistance gene recognizer (RGI) software against the comprehensive antibiotic resistance database (Mcarthur et al., 2013). The mobile genetic elements (MGEs) were annotated using ISfinder (Siguier et al., 2006) and INTEGRALL (Siguier et al., 2006). ANI was calculated using FastANI (Jain et al., 2018). The basic characteristics of the plasmid and the comparison with its close relatives were visualized by the CGView Comparison Tool (Petkau et al., 2010). The molecular weight and the pI value of LAQ-1 were predicted using ProtParam1. The putative signal peptide cleavage site of LAQ-1 was identified by SignalP 5.0 (Almagro Armenteros et al., 2019). Multiple sequence alignment of the amino acid sequences, neighbor-joining phylogenetic tree construction and visualization of LAQ-1 with other AmpC β-lactamases were performed using MAFFT (Kazutaka and Standley, 2013), MEGA (Kumar and Tamura, 2016) and ggtree (Yu, 2020), respectively. The structures of the approximately 20-kb flanking regions of the blaLAQ-1 gene and its relatives were compared using the GenePlotR package (Guy et al., 2010). GenePlotR was also used to generate graphs showing the structural comparison and nucleotide identity of different MDR regions (Sullivan et al., 2011). GNU Parallel was used to access various data in the NCBI database in parallel.
Cloning of the blaLAQ-1 gene and expression and purification of LAQ-1
The primers to amplify the blaLAQ-1 gene together with its promoter region (pro-blaLAQ-1) were designed using SnapGene Viewer (Qiao et al., 2019), with a pair of restriction endonuclease digestion sites (BamHI and HindIII) included at the 5′-end of both primers. The forward primer sequence was 5’-CGCGGATCCGCGTGCAAAATCCGGTGGTGGTGATC-3′, and the reverse primer sequence was 5’-CCCAAGCTTGGGATCGGTTATTTCAACGTGTCCAGGA-3′ (Supplementary Table S1). Then, the PCR product was eluted from an agarose gel and digested with BamHI and HindIII, and the digested product was ligated into the pUCP24 vector, which was also digested with BamHI and HindIII, by a T4 DNA Ligase Cloning Kit (Takara Bio, Inc., Dalian, China). The recombinant plasmid (pUCP24-pro-blaLAQ-1) was transformed into competent E. coli DH5α cells by the calcium chloride method (Cohen et al., 1972), and the transformants (pUCP24-pro-blaLAQ-1/DH5α) were cultured on Luria-Bertani (LB) agar plates containing 40 μg/mL gentamicin. The cloned resistance gene in the recombinant plasmid was verified by Sanger sequencing (Shanghai Sunshine Biotechnology Co., Ltd., Shanghai, China). The resistance activity of the cloned gene blaLAQ-1 was further determined. To obtain the β-lactamase LAQ-1, the ORF of blaLAQ-1 without the signal sequence was cloned by the above mentioned procedure, using pET-28a as the cloning vector and E. coli BL21 as the recipient. The recombinant clone (pET-28a-blaLAQ-1/BL21) was selected on LB agar plates containing 50 μg/mL kanamycin. The recombinant clone was cultured overnight in LB medium containing 50 μg/mL kanamycin. The overnight culture was diluted 100-fold in 100 mL of LB medium and shaken at 37°C for 2–3 h until the OD600 reached 0.6–0.8. Isopropyl-β-d-thiogalactopyranoside (IPTG; Sigma Chemicals Co., St. Louis, MO, United States) was added at a final concentration of 1 mM, and incubation was continued for an additional 4 h. According to the instructions for BeyoGold His tag Purification Resin (Beyotime, Shanghai, China), the protein was purified by affinity chromatography, followed by incubation with Enterokinase (GenScript, Nanjing, China) at 16°C for 36 h to remove the histidine tag (Zhou et al., 2020).
Determination of kinetic parameters
Kinetic parameters for hydrolysis of β-lactams by the purified LAQ-1 β-lactamase were examined using a UV–VIS spectrophotometer (U-3900, HITACHI, Japan) at 37°C in 10 mM phosphate buffer (pH 7.0) in a final reaction volume of 200 μl. The steady-state kinetic parameters (kcat and Km) were determined by nonlinear regression of the initial reaction rates with the Michaelis–Menten equation in GraphPad Prism 8 (GraphPad Software, CA, United States). The inhibitory effect of β-lactamase was studied using 100 μM nitrocephalosporin as a substrate (Chen et al., 2019). The β-lactamase inhibitor avibactam and GMP (disodium 50-guanosine monophosphate) were preincubated with purified LAQ-1 at different concentrations for 5 min at 37°C. The inhibitor concentration required to reduce the hydrolysis of 100 μM nitrocefin by 50% was determined by nonlinear regression with the log (inhibitor) versus response-(three parameters) in GraphPad Prism 8 (Chen et al., 2019).
Nucleotide sequence accession numbers
The chromosome, the plasmid pP13-67 and the blaLAQ-1 gene sequences of L. amnigena P13 have been submitted to GenBank with accession numbers CP099511.1, CP099512.1 and MZ497396.1, respectively.
Results and discussion
Genome characteristics and resistance profile of Lelliottia amnigena P13
Lelliottia amnigena P13 was isolated from sewage discharged from an animal farm in Wenzhou, southern China, in 2017. The 16S rRNA gene sequence homology analysis showed that P13 was most closely related to L. amnigena NCTC12124 (with a coverage of 98% and an identity of 99.93%). The genome-wide ANI analysis between the genomes of P13 and other bacteria in the NCBI assembly database revealed that the 20 bacterial genomes showed ≥98.00% ANI with that of P13 were all from L. amnigena, of which the genome of the type strain L. amnigena NCTC 12124 (GenBank: LR134135.1) showed 98.84% ANI with the P13 genome. It confirmed that the isolate belonged to the species L. amnigena and thus designated L. amnigena P13.
The P13 genome consists of a circular chromosome and a plasmid designated pP13-67. The chromosome size is 4,555,627 bp with a GC content of 52.85%, and it encodes 4,349 ORFs (Table 2). pP13-67 was 66,758 bp in length and encoded 79 ORFs including 11 resistance genes related to eight classes of antimicrobials (Figure 1). Similar to most Enterobacteriaceae, the in vitro drug susceptibility test showed that L. amnigena P13 was resistant to ampicillin, penicillin G, cefoxitin, cefazolin, tetracycline and ticarcillin/clavulanate (Table 3).
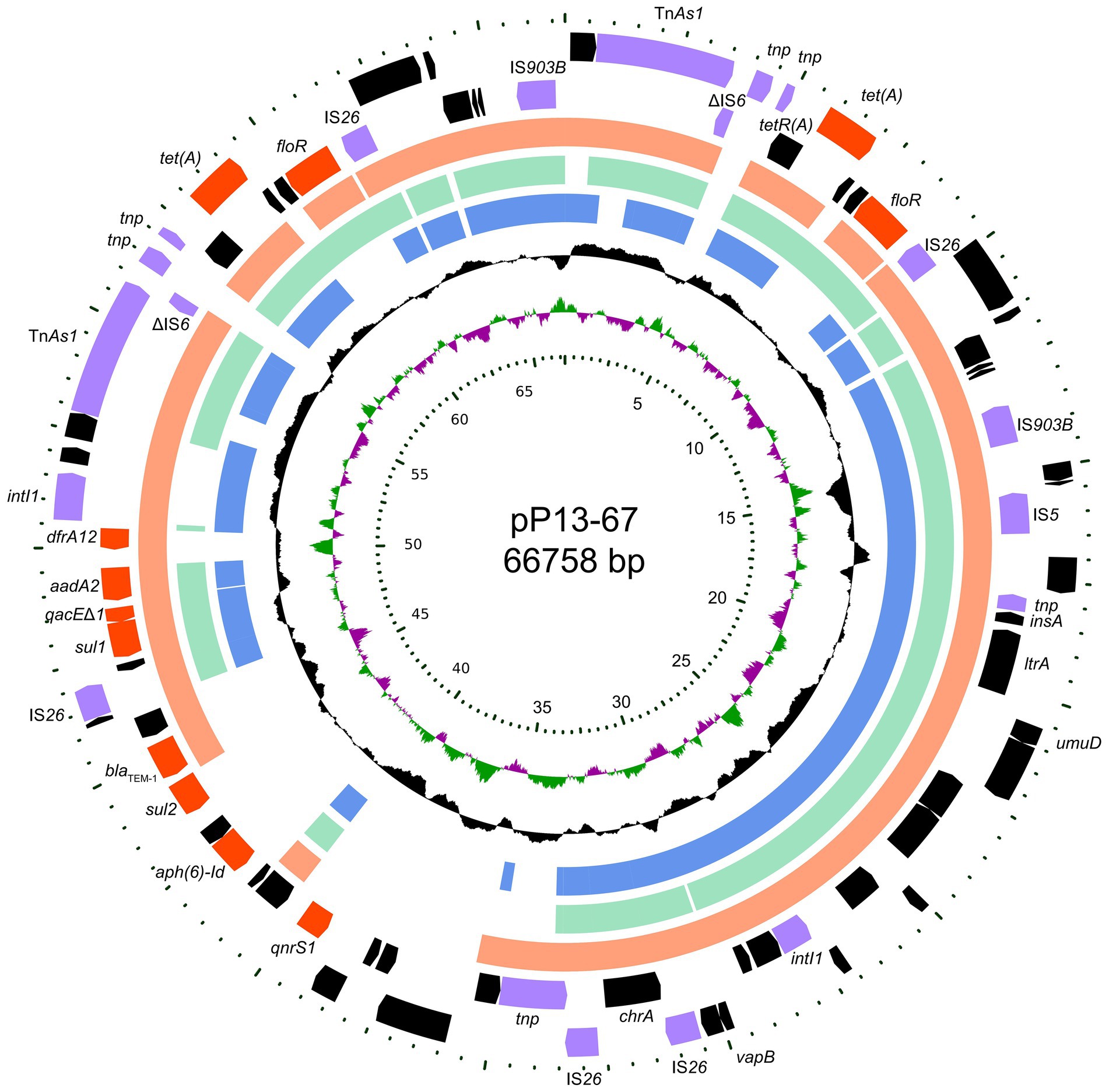
Figure 1. Circular map of pP13-67 and comparative genomic analysis with other closely related plasmids. From inside to outside: Circle 1 shows the scale in kb. Circles 2 and 3 display the GC skew and GC content, respectively. Circles 4–6 show the homologous sequences of pEcl5-2 of Enterobacter hormaechei Eho-5 (CP047738.1), pN56639 of Escherichia coli CVM N56639 (CP043753.1) and p1 of Klebsiella pneumoniae JM45 (CP006657.1), respectively, while areas displaying different features have been left blank. Circles 7 and 8 present genes encoded in the reverse and forward strands of pP13-67, respectively.
Identification of the novel β-lactamase gene blaLAQ-1
When analyzing the resistance mechanism, we found that only one of the function- characterized resistance gene for β-lactam antibiotics (a blaTEM-1 encoded on the plasmid pP13-67) was annotated in the P13 genome, even though this strain shows resistance to many kinds of β-lactam antibiotics tested. To investigate whether there was a putative β-lactamase gene encoded in the L. amnigena P13 genome, we checked the annotation result of the genome and found that one of the predicted genes contained the conserved motif of an Ambler class C β-lactamase, which showed the highest amino acid homology (with an identity of 78.95%) with the function-characterized AmpC β-lactamase ACT-22 (Porres-Osante et al., 2015). Other β-lactamases with higher amino acid identities to LAQ-1 were ACT-6 (ACJ05686.1, 78.42%, 298/380), CMY-20 (AAX58682.2, 78.42%, 298/380) and so on.
To determine the potential resistance function of the gene, we cloned the ORF of the predicted gene (finally designated blaLAQ-1) with its promoter region into the pUCP24 vector. The blaLAQ-1 gene was functional, and the recombinant clone (pUCP24-blaLAQ-1/DH5α) showed increased MIC levels (by approximately 4- to 64-fold) for some β-lactams, including several first- to fourth-generation cephalosporins, such as cefazolin, cefoxitin and cefepime, compared with the control strains (DH5α or DH5α carrying pUCP24; Table 3). The resistance profile of blaLAQ-1 is consistent with that of most Enterobacter species with inducible ampC genes (Jacoby, 2009). However, the recombinant clone harboring blaLAQ-1 did not show any change in MIC levels to carbapenems. blaLAQ-1 conferred resistance to fourth-generation cephalosporins, unlike ACT-6 (Zhu et al., 2011) and CMY-2 (Bauernfeind et al., 1996). Classic class A β-lactam inhibitors, such as clavulanic acid and sulbactam, had poor inhibitory effects on the activity of LAQ-1 (Table 3).
Molecular characterization of the novel AmpC β-lactamase LAQ-1
The novel β-lactamase gene blaLAQ-1, which is 1,143 bp in length, encodes a 380 amino acid preprotein of approximately 39.6 kDa. The cleavage site of the signal peptide is predicted to be located between amino acid residues 19 (alanine) and 20 (alanine). It is further predicted that the isoelectric point of LAQ-1 is 8.58. Although a putative class C β-lactamase (LR134135.1) showing the highest nucleotide homology with blaLAQ-1 (99.39%, 1,136/1,143) was found to be encoded in the L. amnigena NCTC12124 chromosome (NZ_CP023529.1), the two function-characterized β-lactamases with the highest amino acid identities to LAQ-1 were ACT-6 (ACJ05686.1, 78.42%, 298/380) and CMY-20 (AAX58682.2, 78.42%, 298/380).
Five genes with the highest nucleotide similarity to blaLAQ-1 (>93.79%) were retrieved from the NCBI nucleotide database, all of which originated from L. amnigena (one was isolated from soil, while the sources of the remaining four are unknown). The rooted phylogenetic tree analysis demonstrated that LAQ-1 formed a new branch on the phylogenetic tree of AmpC β-lactamases (Figure 2). Comparison of the deduced amino acid sequence of LAQ-1 with the function-characterized β-lactamases revealed that LAQ-1 showed the highest identities of 78.42, 78.42, 77.89, 77.89, 77.63, 77.49 and 77.11% with ACT-6, CMY-20, CMY-2, ACT-10, CMY-4, CMY-18 and ACT-10, respectively (Figure 3). Notably, all of these deduced amino acid sequences had the obligatory serine-active site of the β-lactamase catalytic motif S-X-S-K (serine-valine-serine-lysine) at positions 83–86, the typical class C β-lactamase motif Y-A-N (tryptophan-alanine-asparagine) at positions 169–171, D/E (a peptide segment containing two dicarboxylic amino acids) at positions 236–238 and the conserved triad K-T-G (lysine-threonine-glycine) at positions 334–336 (Ghuysen, 1991; Figure 3).
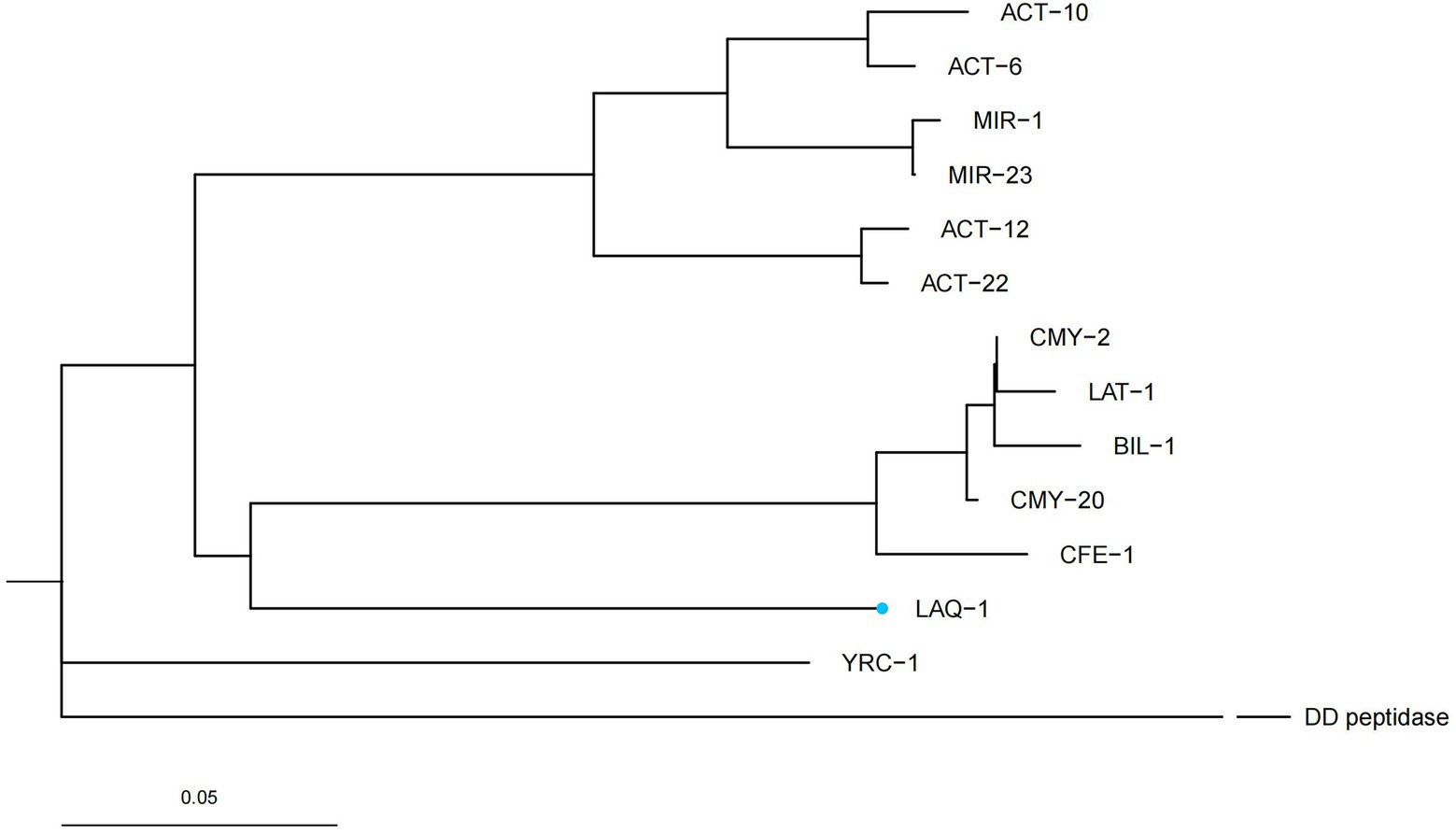
Figure 2. A rooted phylogenetic tree of LAQ-1 with its function-characterized relatives. DD peptidase of Streptomyces R61 (D-alanyl-d-alanine-Carboxypeptidase, 1CEF_A) was used as an out group. LAQ-1 from our study is shown with a blue dot. The other sequences and their accession numbers are ACT-12 (AFU25650.1), ACT-22 (AHM76774.1), BIL-1 (CAA52618.1), CMY-2 (CAA62957.1), CMY-20 (AAX58682.2), LAT-1 (CAA55007.1), CFE-1 (BAC76072.1), YRC-1 (ABA70720.1), MIR-1 (AAD22636.1), MIR-23 (AIG20028.1), ACT-6 (ACJ05686.1), ACT-10 (AEV91214.1).
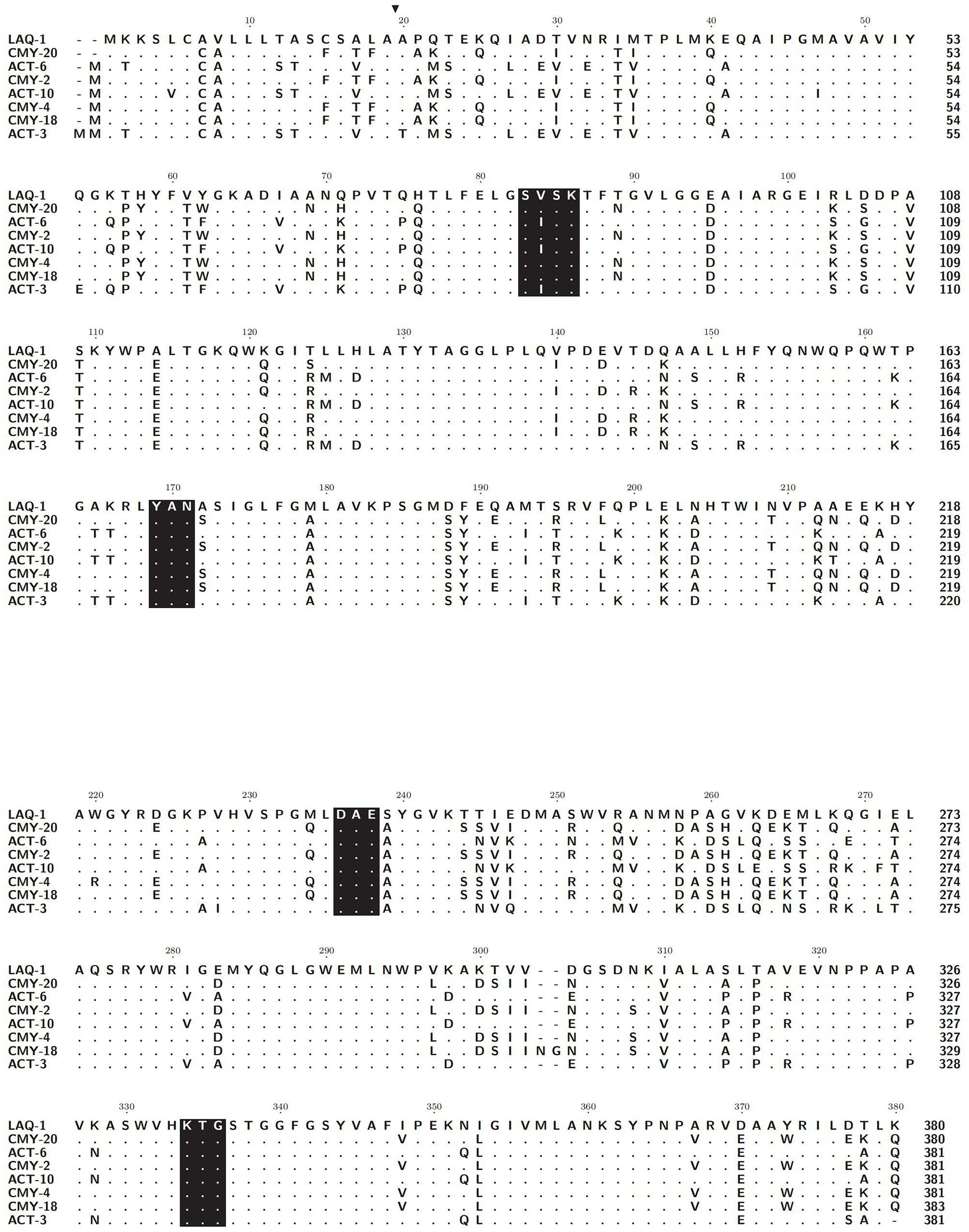
Figure 3. Alignment of the amino acid sequences of LAQ-1 and its homologous function-characterized class C β-lactamases. Identical amino acids are indicated by dots. Signal sequences are highlighted in black, and four conserved motifs are shaded in black. LAQ-1 numbering is based on the standard numbering scheme for class C beta-lactamases. The sequences and their accession numbers are CMY-20 (AAX58682.2), ACT-6 (ACJ05686.1), CMY-2 (CAA62957.1), ACT-10 (AEV91214.1), CMY-4 (CAA07695.1), CMY-18 (AAU95778.1) and ACT-10 (ABL67017.1).
Kinetic parameters of LAQ-1
The purified β-lactamase LAQ-1 showed the highest catalytic efficiency for piperacillin (kcat/Km of 107.68) and showed moderate catalytic efficiency against first- (cefazolin, kcat/Km of 39.88) and second-generation cephalosporins (cefoxitin, kcat/Km of 16.92), and penicillins (ampicillin, kcat/Km of 20.96). It demonstrated low catalytic efficiencies against the third-generation cephalosporin (such as ceftazidime with a kcat/Km of 4.58) and the extended-spectrum cephalosporins (such as cefepime, with a kcat/Km of 8.18; Table 4), which is similar to the values for other chromosome- or plasmid-encoded class C β-lactamases, such as ACT-6 (Zhu et al., 2011) and CMY-2 (Bauernfeind et al., 1996). LAQ-1 was more active against cefazolin and cefoxitin than ACT-6 (Zhu et al., 2011) because of its much higher kcat, which was partially compensated by its higher Km values (Table 4). However, the enzyme kinetic hydrolytic activities were not completely consistent with the changes in the MIC levels of the recombinant clone (pUCP24-blaLAQ-1/DH5α). For example, LAQ-1 had obvious hydrolytic activity against cefazolin (kcat/Km of 39.88) and piperacillin (kcat/Km of 191.5), but the recombinant with blaLAQ-1 showed no significant change in the MIC level (increased merely 4-fold) compared to the control strains, while the MIC of cefoxitin (kcat/Km of 16.92) changed greatly (increased 64-fold). This phenomenon may be attributed to its low activity in vitro (Toth et al., 2016). In contrast, no obvious hydrolytic activity of LAQ-1 against aztreonam was detected, which contradicts the increase in the MIC value of it (increased 4-fold). It has been reported that the hydrolysis rate of aztreonam is slower than that of other β-lactams, which may make its hydrolysis difficult to detect (David et al., 2012). A similar phenomenon was observed for CMY-2, the recombinant form of which had an increased MIC value against aztreonam, but the hydrolysis of aztreonam was not detectable (Kotsakis et al., 2015). The half inhibitory concentrations (IC50) of β-lactamase inhibitors showed that avibactam (IC50: 0.001451 μM) had a strong inhibitory effect on LAQ-1 and a weaker inhibitory effect on GMP (IC50: 2.626 μM). This result is consistent with the nature of AmpC β-lactamase inhibition (David et al., 2012).
Genetic context of the blaLAQ-1 gene
To analyze the genetic environment of blaLAQ-1 of L. amnigena P13 and its relatives, a fragment that was approximately 20 kb in length with the blaLAQ-1 gene at the center was queried against the NCBI nucleotide database. Five fragments of the highest similarities were retrieved, which were all from L. amnigena chromosomes (>99% coverage and >95.00% identity). Comparative genomic analysis of the six sequences (including the one from this work) showed that they shared conserved structures in both the gene context and the gene order, except that the gene context of L. amnigena NCTC12124 was slightly different from the other five (Figure 4). The blaLAQ-1 homologous genes identified in L. amnigena P13 and its relatives were located in the chromosomes, and no MGE was detected around them. This finding suggests that these genes may be intrinsic in the bacteria of this speice (Figure 4). On the other hand, although no MGE was predicted in the region surrounding blaLAQ-1, there is a pair of perfect 8 bp inverted repeats (IRs) on both sides of the fragment encoding fraA-D-blaLAQ-1−sugE, suggesting that they might play a role in mobilization and horizontal gene transfer (HGT) of this gene array.
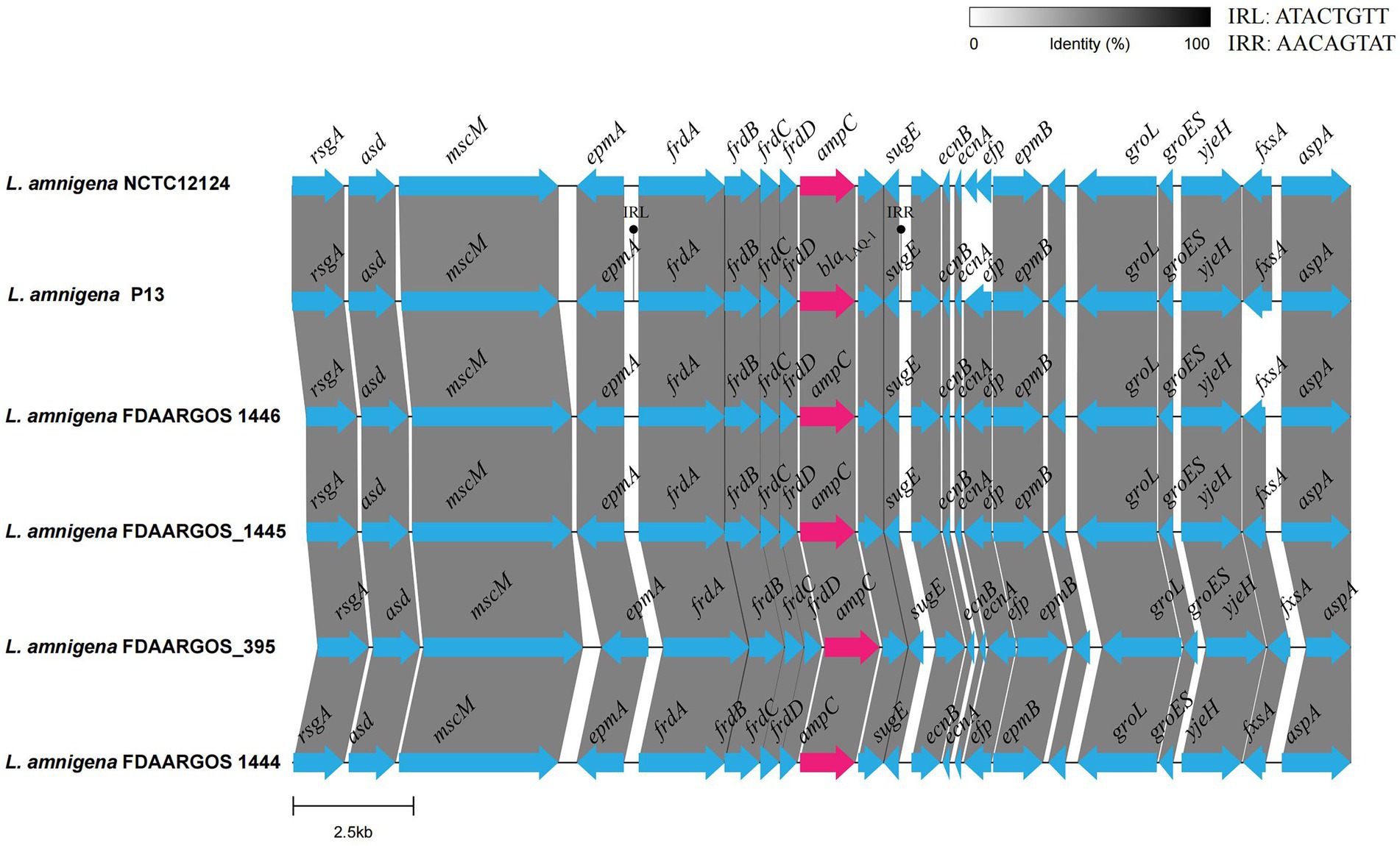
Figure 4. Comparative genomic analysis of the genetic context of blaLAQ-1 with the sequences carrying their homologous genes. The direction of genes is shown via an arrow. The blaLAQ-1 gene and putative ampC (blaLAQ-1-like) genes are colored red, and the other genes are colored blue. The predicted hypothetical genes are in gray. The sequences and their accession numbers are as follows: L. amnigena NCTC12124 (LR134135.1), L. amnigena FDAARGOS 1446 (CP077331.1), L. amnigena FDAARGOS_1445 (CP079896.1), L. amnigena FDAARGOS 1444 (CP077236.1) and L. amnigena FDAARGOS 395 (CP023529.1).
Comparative analysis of the plasmids carrying multiple resistance genes
In this study, 12 resistance genes related to different MGEs were identified in the plasmid pP13-67, including one quinolone resistance gene (qnrS1), two aminoglycoside resistance genes [aph(6)-Id and aadA2], two sulfonamide resistance genes (sul1 and sul2), one β-lactam resistance gene (blaTEM-1), one truncated quaternary ammonium compound resistance gene (qacEΔ1), one diaminopyrimidine resistance gene (dfrA12), two copies of tetracycline resistance gene tetA and two copies of chloramphenicol/florfenicol resistance gene floR.
Comparative genomic analysis revealed that pP13-67 shared the highest identity with three plasmids, including p1 of Klebsiella pneumoniae JM45 (CP006657.1, 85.0% coverage and 99.94% identity), pEcl5-2 of Enterobacter hormaechei Eho-5 (CP047738.1, 70.0% coverage and 99.97% identity) and pN56639 of Escherichia coli CVM N56639 (CP043753.1, 73.0% coverage and 99.81% identity). The differences in the sequences of the four plasmids were mainly located in a ~22 kb multidrug resistance (MDR) region encoding a typical class 1 integron of pP13-67 (Figure 1).
Interestingly, this plasmid contained two identical fragments in a tandem repeat structure at positions 0–14 kb and 53–67 kb (from TnAs1 to IS903B). The repeat sequence was composed of a number of MGEs (such as insertion sequences, ISs) and two resistance genes [floR and tet(A)], approximately 28 kb in length, which contributed to the high MIC levels for tetracycline (64 μg/mL) and florfenicol (128 μg/mL). Similar sequences were also found in the three plasmids mentioned above (with p1 of Klebsiella pneumoniae JM45 lacking the floR gene).
To better characterize the distribution of the MDR-related sequences, the MDR region of pP13-67 was comparatively analyzed with other relative sequences. The results showed that the MDR region of pP13-67 shared the highest nucleotide sequence similarities with those of chromosomes or plasmids of bacteria of different species, including the chromosome of Escherichia albertii strain Sample 166 (CP070292.1, 88.0% coverage and 99.92% identity), the chromosome of Escherichia coli strain LHM10-1 (CP070292.1, 76.0% coverage and 99.97% identity), p2018N17–066-1 of Klebsiella pneumoniae strain 2018 N17-066 (CP044390.1, 86.0% coverage and 99.84% identity), pSAL4578-1 of Salmonella enterica subsp. enterica serovar 4,[5],12:i:-L-4578 (AP023310.1, 82.0% coverage and 99.97% identity), and pRW8-1_122k_tetX of Escherichia coli RW8-1 (MT219826.1, 79.0% coverage and 99.84% identity). Notably, there was a typical class 1 integron (organized with a 5-conserved segment [5-CS: intI1], a variable region [VR: aad A2 and dfrA12]) and a 3-CS [3-CS: qacE11-sul1] on pP13-67 (Figure 5). The typical class 1 integron was also found in the remaining five sequences. The integron in pP13-67 was connected with transposon TnAs1, enabling its transfer between different positions in chromosomes and/or plasmids.
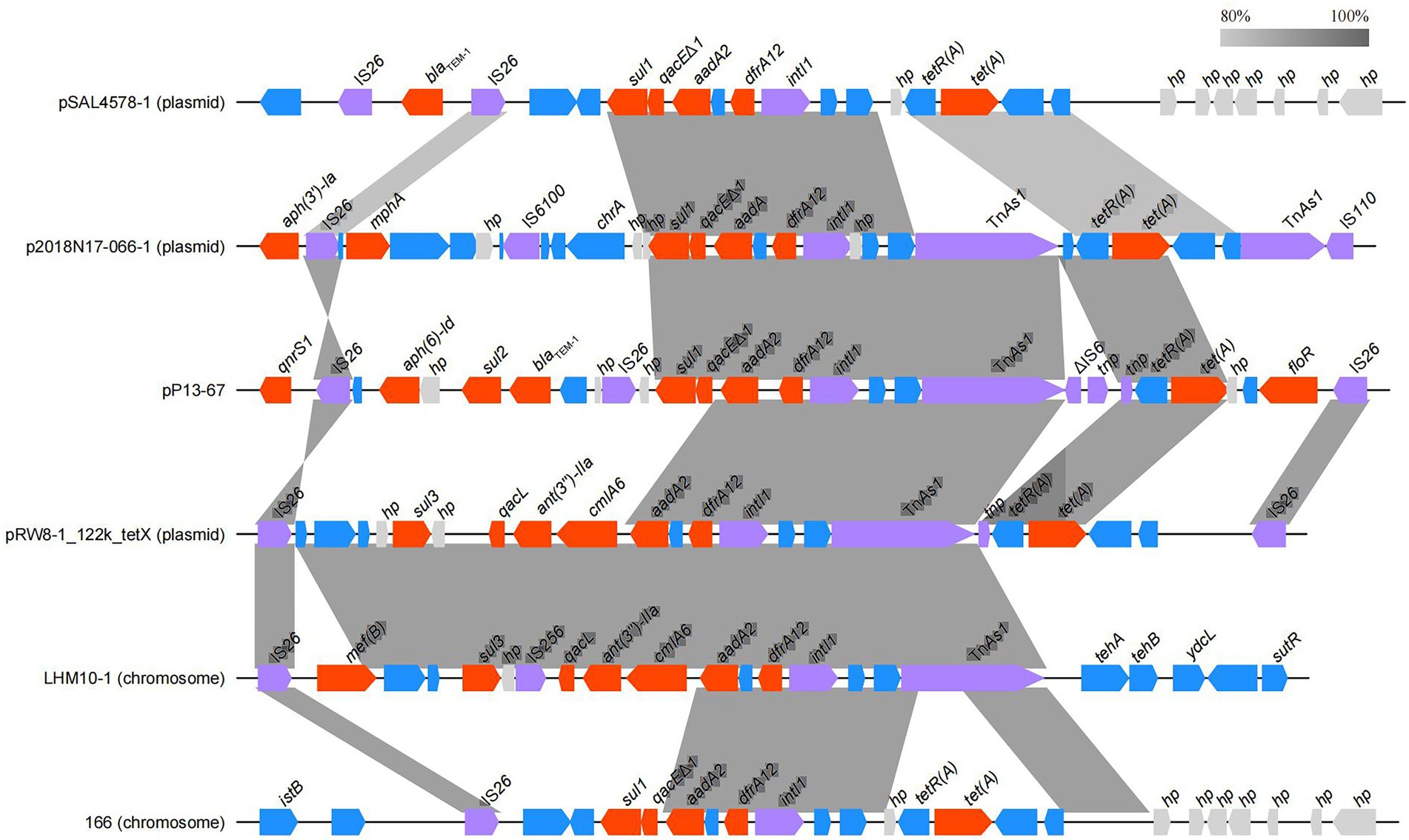
Figure 5. Comparison of the MDR region of pP13-67 with the related sequences on the other plasmids and chromosomes. Genes are denoted by arrows and colored according to gene function classification. Shading indicates the homologous regions.
Conclusion
In this study, on the basis of whole-genome sequencing, we identified a novel chromosome-encoded AmpC β-lactamase gene designated blaLAQ-1 in an environmental bacterium L. amnigena P13. Among all the reported functional β-lactamases, LAQ-1 shares the highest amino acid identity of 78.95% with ACT-22. blaLAQ-1 confers resistance to a number of β-lactam antimicrobials, including several first- to fourth-generation cephalosporins. In addition to the chromosomal blaLAQ-1, a number of resistance genes conferring resistance to tetracyclines, chloramphenicol, florfenicol and streptomycin were identified in the plasmid (designated pP13-67) of L. amnigena P13. All these findings will help in the elucidation of the resistance mechanisms of this unusual opportunistic pathogen and in the development of treatment methods for human (or animal) infections caused by L. amnigena.
Data availability statement
The datasets presented in this study can be found in online repositories. The names of the repository/repositories and accession number(s) can be found at: https://www.ncbi.nlm.nih.gov/, CP099511.1, CP099512.1, MZ497396.1.
Author contributions
QB, CC, XS, and DH: conceived and designed the experiments. AL, CY, LZ, SL, CF, LHZ, FD, and LW: performed the experiments. AL, YZ, JL JX, LZ, and DH: data analysis and interpretation. AL, CF, QB, CC, and DH: drafting of the manuscript. All authors contributed to the article and approved the submitted version.
Funding
This study was supported by Zhejiang Provincial Natural Science Foundation of China (LGF19H200003); the Science & Technology Project of Taizhou City, China (21ywb126); the Science & Technology Project of Wenzhou City, China (N20210001); Technology Planning Project of Zhejiang Province (LGN19C180002) and the Science & Technology Project of Jinhua City, China (2022-4-017).
Acknowledgments
The authors would like to acknowledge all study participants and individuals who contributed to this study.
Conflict of interest
The authors declare that the research was conducted in the absence of any commercial or financial relationships that could be construed as a potential conflict of interest.
Publisher’s note
All claims expressed in this article are solely those of the authors and do not necessarily represent those of their affiliated organizations, or those of the publisher, the editors and the reviewers. Any product that may be evaluated in this article, or claim that may be made by its manufacturer, is not guaranteed or endorsed by the publisher.
Supplementary material
The Supplementary material for this article can be found online at: https://www.frontiersin.org/articles/10.3389/fmicb.2022.990736/full#supplementary-material
Footnotes
References
Almagro Armenteros, J. J., Tsirigos, K. D., Sønderby, C., Petersen, T. N., Winther, O., Brunak, S., et al. (2019). SignalP 5.0 improves signal peptide predictions using deep neural networks. Nat. Biotechnol. 37, 420–423. doi: 10.1038/s41587-019-0036-z
Bauernfeind, A., Stemplinger, I., Jungwirth, R., and Giamarellou, H. (1996). Characterization of the plasmidic beta-lactamase CMY-2, which is responsible for cephamycin resistance. Antimicrob. Agents Chemother. 40:221. doi: 10.1128/AAC.40.1.221
Bollet, C., Elkouby, A., Pietri, P., and Micco, P. D. (1991). Isolation of Enterobacter amnigenus from a heart transplant recipient. Eur. J. Clin. Microbiol. Infect. Dis. 10, 1071–1073. doi: 10.1007/BF01984933
Brady, C., Cleenwerck, I., Venter, S., Coutinho, T., and De Vos, P. (2013). Taxonomic evaluation of the genus Enterobacter based on multilocus sequence analysis (MLSA): proposal to reclassify E. nimipressuralis and E. amnigenus into Lelliottia gen. Nov. as Lelliottia nimipressuralis comb. nov. and Lelliottia amnigena comb. nov., respectively, E. gergoviae and E. pyrinus into Pluralibacter gen. Nov. as Pluralibacter gergoviae comb. nov. and Pluralibacter pyrinus comb. nov., respectively, E. cowanii, E. radicincitans, E. oryzae and E. arachidis into Kosakonia gen. Nov. as Kosakonia cowanii comb. nov., Kosakonia radicincitans comb. nov., Kosakonia oryzae comb. nov. and Kosakonia arachidis comb. nov., respectively, and E. turicensis, E. helveticus and E. pulveris into Cronobacter as Cronobacter zurichensis nom. Nov., Cronobacter helveticus comb. nov. and Cronobacter pulveris comb. nov., respectively, and emended description of the genera Enterobacter and Cronobacter. Syst. Appl. Microbiol. 36, 309–319. doi: 10.1016/j.syapm.2013.03.005
Buchfink, B., Reuter, K., and Drost, H. G. (2021). Sensitive protein alignments at tree-of-life scale using DIAMOND. Nat. Methods 18, 366–368. doi: 10.1038/s41592-021-01101-x
Bush, K., Jacoby, G. A., and Medeiros, A. A. (1995). A functional classification scheme for beta-lactamases and its correlation with molecular structure. Antimicrob. Agents Chemother. 39, 1211–1233.
Bush, M., Rasmussen, B. A., and Lee, V. J. (1993). Kinetic interactions of tazobactam with beta-lactamases from all major structural classes. Antimicrob. Agents Chemother. 37, 851–858. doi: 10.1128/AAC.37.4.851
Casin, I., Grimont, F., and Grimont, P. A. D. (1986). Deoxyribonucleic acid relatedness between Haemophilus aegyptius and Haemophilus influenzae. Anna. Inst. Pasteur Microbiol. 137, 155–163.
Chen, Q., Zhou, W., Qian, C., Shen, K., and Lu, J. (2019). OXA-830, a novel chromosomally encoded extended-Spectrum class D β-lactamase in Aeromonas simiae. Front. Microbiol. 10:2732. doi: 10.3389/fmicb.2019.02732
CLSI (2021). Performance standards for antimicrobial susceptibility testing: CLSI Supplement M100. 31th edn. Wayne, PA: Clinical and Laboratory Standards Institute.
Cohen, S. N., Chang, A. C., and Hsu, L. (1972). Nonchromosomal antibiotic resistance in bacteria: genetic transformation of Escherichia coli by R-factor DNA. Proc. Natl. Acad. Sci. U. S. A. 69, 2110–2114.
David, E., Ehmann, H. J., Ross, P. L., Gu, R.-F., Hu, J., Kern, G., et al. (2012). Avibactam is a covalent, reversible, non-β-lactam β-lactamase inhibitor. Proc. Natl. Acad. Sci. U. S. A. 109, 11663–11668. doi: 10.1073/pnas.1205073109
EUCAST (2019). Breakpoint Tables for Interpretation of MICs and Zone Diameters, Version 9.0. European Committee on Antimicrobial Susceptibility Testing (EUCAST). Available at: http://www.eucast.org.
Fadare, F. T., and Okoh, A. I. (2021). Distribution and molecular characterization of ESBL, pAmpC beta-lactamases, and non-beta-lactam encoding genes in Enterobacteriaceae isolated from hospital wastewater in eastern Cape Province, South Africa. PLoS One 16:e0254753. doi: 10.1371/journal.pone.0254753
Ghuysen, J. M. (1991). Serine Beta-lactamases and penicillin-binding proteins. Annu. Rev. Microbiol. 45, 37–67.
Guy, L., Kultima, J. R., and Andersson, S. G. E. (2010). genoPlotR: comparative gene and genome visualization in R. Bioinformatics 26, 2334–2335. doi: 10.1093/bioinformatics/btq413
Hanson, N. D. (2002). Unusual salmonella enterica serotype typhimurium isolate producing CMY-7, SHV-9 and OXA-30 β-lactamases. J. Antimicrob. Chemother. 49, 1011–1014. doi: 10.1093/jac/dkf052
Hanson, N. D. (2003). AmpC beta-lactamases: what do we need to know for the future? J. Antimicrob. Chemother. 52, 2–4. doi: 10.1093/jac/dkg284
Izard, D., Gavini, F., Trinel, P. A., and Leclerc, H. (1981). Deoxyribonucleic acid relatedness between Enterobacter cloacae and Enterobacter amnigenus sp. nov. Int. J. Syst. Evol. Microbiol. 31, 382–383.
Jain, C., Rodriguez, R. L., Phillippy, A. M., Konstantinidis, K. T., and Aluru, S. (2018). High throughput ANI analysis of 90K prokaryotic genomes reveals clear species boundaries. Nat. Commun. 9:5114. doi: 10.1038/s41467-018-07641-9
Kazutaka, K., and Standley, D. M. (2013). MAFFT multiple sequence alignment software version 7: improvements in performance and usability. Mol. Biol. Evol. 30, 772–780. doi: 10.1093/molbev/mst010
Knotthunziker, V., Petursson, S., Jayatilake, G. S., Waley, S. G., Jaurin, B., and Grundström, T. (1982). Active sites of beta-lactamases. The chromosomal beta-lactamases of Pseudomonas aeruginosa and Escherichia coli. Biochem. J. 201, 621–627. doi: 10.1042/bj2010621
Koren, S., Walenz, B., Berlin, K., Miller, J., Bergman, N., and Phillippy, A. (2017). Canu: scalable and accurate long-read assembly via adaptive κ-mer weighting and repeat separation. Genome Res. 27, 215087–215116. doi: 10.1101/gr.215087.116
Kotsakis, S. D., Miriagou, V., Vetouli, E. E., Bozavoutoglou, E., Lebessi, E., Tzelepi, E., et al. (2015). Increased hydrolysis of Oximino-β-lactams by CMY-107, a Tyr199Cys mutant form of CMY-2 produced by Escherichia coli. Antimicrob. Agents Chemother. 59, 7894–7898. doi: 10.1128/AAC.01793-15
Kumar, S. S. G., and Tamura, K. (2016). MEGA7: molecular evolutionary genetics analysis version 7.0 for bigger datasets. Mol. Biol. Evol. 33, 1870–1874. doi: 10.1093/molbev/msw054
Leal-Negredo, Á., Castelló-Abietar, C., Leiva, P. S., and Fernández, J. (2017). Infección urinaria por Lelliottia amnigena (Enterobacter amnigenus): Un patógeno infrecuente. Rev. Esp. Quimioter. 30:483.
Literak, I., Manga, I., Wojczulanis-Jakubas, K., Chroma, M., Jamborova, I., Dobiasova, H., et al. (2014). Enterobacter cloacae with a novel variant of ACT AmpC beta-lactamase originating from glaucous gull (Larus hyperboreus) in Svalbard. Vet. Microbiol. 171, 432–435. doi: 10.1016/j.vetmic.2014.02.015
Liu, S., and Tang, Y. (2016). Identification and characterization of a new Enterobacter onion bulb decay caused by Lelliottia amnigena in China. Appl. Microbiol. 2:114. doi: 10.4172/2471-9315.1000114
Martin Guerra, J. M., Martin Asenjo, M., and Duenas Gutierrez, C. J. (2018). Pyonephrosis by Lelliottia amnigena. Med. Clin. (Barc) 151, 419–420. doi: 10.1016/j.medcli.2018.01.011
Mcarthur, A. G., Waglechner, N., Nizam, F., Yan, A., Azad, M. A., Baylay, A. J., et al. (2013). The comprehensive antibiotic resistance database. Antimicrob. Agents Chemother. 57, 3348–3357. doi: 10.1128/AAC.00419-13
Murugaiyan, J., Krueger, K., Roesler, U., Weinreich, J., and Schierack, P. (2015). Assessment of species and antimicrobial resistance among Enterobacteriaceae isolated from mallard duck faeces. Environ. Monit. Assess. 187:127. doi: 10.1007/s10661-015-4346-4
Petkau, A., Stuart-Edwards, M., Stothard, P., and Van Domselaar, G. (2010). Interactive microbial genome visualization with GView. Bioinformatics 26, 3125–3126. doi: 10.1093/bioinformatics/btq588
Porres-Osante, N., Saenz, Y., Somalo, S., and Torres, C. (2015). Characterization of Beta-lactamases in faecal Enterobacteriaceae recovered from healthy humans in Spain: focusing on AmpC polymorphisms. Microb. Ecol. 70, 132–140. doi: 10.1007/s00248-014-0544-9
Qiao, S., Huang, S., Deng, Y., Deng, H., Luo, C., Shi, D., et al. (2019). Construction of eukaryotic expression vector of Buffalo miR-302s and its bioinformatics analysis. China Animal Husbandry Vet. Med. 46, 2497–2506. doi: 10.16431/j.cnki.1671-7236.2019.09.001
Rd, J. J. F., Davis, B. R., Hickmanbrenner, F. W., Mcwhorter, A., Huntleycarter, G. P., Asbury, M. A., et al. (1985). Biochemical identification of new species and biogroups of Enterobacteriaceae isolated from clinical specimens. J. Clin. Microbiol. 21, 46–76. doi: 10.1128/jcm.21.1.46-76.1985
Seemann, T. (2014). Prokka: rapid prokaryotic genome annotation. Bioinformatics 30, 2068–2069. doi: 10.1093/bioinformatics/btu153
Siguier, P., Perochon, J., Lestrade, L., Mahillon, J., and Chandler, M. (2006). ISfinder: the reference Centre for bacterial insertion sequences. Nucleic Acids Res. 34, 32–36. doi: 10.1093/nar/gkj014
Stock, I., and Wiedemann, B. (2002). Natural antibiotic susceptibility of Enterobacter amnigenus, Enterobacter cancerogenus, Enterobacter gergoviae and Enterobacter sakazakii strains. Clin. Microbiol. Infect. 8, 564–578. doi: 10.1046/j.1469-0691.2002.00413.x
Sullivan, M. J., Petty, N. K., and Beatson, S. A. (2011). Easyfig: a genome comparison visualizer. Bioinformatics 27, 1009–1010. doi: 10.1093/bioinformatics/btr039
Toth, M., Antunes, N. T., Stewart, N. K., Frase, H., Bhattacharya, M., Smith, C. A., et al. (2016). Class D β-lactamases do exist in gram-positive bacteria. Nat. Chem. Biol. 12, 9–14. doi: 10.1038/nchembio.1950
Walker, B. J., Abeel, T., Shea, T., Priest, M., and Earl, A. M. (2014). Pilon: an integrated tool for comprehensive microbial variant detection and genome assembly improvement. PLoS One 9:112963. doi: 10.1371/journal.pone.0112963
Westerfeld, C., Papaliodis, G. N., Behlau, I., Durand, M. L., and Sobrin, L. (2009). Enterobacter amnigenus endophthalmitis. Retinal Cases Brief Rep. 3, 409–411. doi: 10.1097/ICB.0b013e31818a46c0
Yu, G. (2020). Using ggtree to visualize data on tree-like structures. Curr. Protoc. Bioinformatics 69:e96. doi: 10.1002/cpbi.96
Yuk, K. J., Kim, Y. T., Huh, C. S., and Lee, J. H. (2018). Lelliottia jeotgali sp. nov., isolated from a traditional Korean fermented clam. Int. J. Syst. Evol. Microbiol. 68, 1725–1731. doi: 10.1099/ijsem.0.002737
Zhou, D., Sun, Z., Lu, J., Liu, H., and Bao, Q. (2020). Characterization of a novel chromosomal class C β-lactamase, YOC-1, and comparative genomics analysis of a multidrug resistance plasmid in Yokenella regensburgei W13. Front. Microbiol. 11:2021. doi: 10.3389/fmicb.2020.02021
Keywords: Lelliottia amnigena P13, novel resistance gene, β-lactamase, blaLAQ-1, kinetic parameter
Citation: Li A, Yan C, Zhang L, Liu S, Feng C, Zhang L, Dong F, Sheng X, Wang L, Zhang Y, Lu J, Xu J, Zheng L, Bao Q, Cheng C and Huang D (2022) Characterization and identification of a novel chromosomal class C β-lactamase, LAQ-1, and comparative genomic analysis of a multidrug resistance plasmid in Lelliottia amnigena P13. Front. Microbiol. 13:990736. doi: 10.3389/fmicb.2022.990736
Edited by:
Amar A. Telke, Norwegian Veterinary Institute (NVI), NorwayReviewed by:
Teresa G. Ribeiro, University of Porto, PortugalYonghong Xiao, Zhejiang University, China
Copyright © 2022 Li, Yan, Zhang, Liu, Feng, Zhang, Dong, Sheng, Wang, Zhang, Lu, Xu, Zheng, Bao, Cheng and Huang. This is an open-access article distributed under the terms of the Creative Commons Attribution License (CC BY). The use, distribution or reproduction in other forums is permitted, provided the original author(s) and the copyright owner(s) are credited and that the original publication in this journal is cited, in accordance with accepted academic practice. No use, distribution or reproduction is permitted which does not comply with these terms.
*Correspondence: Cong Cheng, MTEzMjQ2NTcwQHFxLmNvbQ==; Dawei Huang, d3V5ZW1lbmdoYW5AMTYzLmNvbQ==
†These authors have contributed equally to this work