Corrigendum: In silico genomic analysis of the potential probiotic Lactiplantibacillus pentosus CF2-10N reveals promising beneficial effects with health promoting properties
- Área de Microbiología, Departamento de Ciencias de la Salud, Facultad de Ciencias Experimentales, Universidad de Jaén, Jaén, Spain
Lactiplantibacillus pentosus CF2-10 N, isolated from brines of naturally fermented Aloreña green table olives, exhibited high probiotic potential. High throughput sequencing and annotation of genome sequences underline the potential of L. pentosus CF2-10 N as excellent probiotic candidate of vegetable origin. In a previous study we could show the probiotic potential of CF2-10 N in vitro, while in this study in silico analysis of its genome revealed new insights into its safety and functionality. Our findings highlight the microorganism’s ecological flexibility and adaptability to a broad range of environmental niches, food matrices and the gastrointestinal tract. These features are shared by both phylogenetically very close L. pentosus strains (CF2-10 N and MP-10) isolated from the same ecological niche with respect to their genome size (≅ 3.6 Mbp), the presence of plasmids (4–5) and several other properties. Nonetheless, additional and unique features are reported in the present study for L. pentosus CF2-10 N. Notably, the safety of L. pentosus CF2-10 N was shown by the absence of virulence determinants and the determination of acquired antibiotic resistance genes, i.e., resistome, which is mostly represented by efflux-pump resistance genes responsible for the intrinsic resistance. On the other hand, defense mechanisms of L. pentosus CF2-10 N include eight prophage regions and a CRISPR/cas system (CRISPR-I and CRISPR-II) as acquired immune system against mobile elements. Finally, the probiotic potential of this strain was further demonstrated by the presence of genes coding for proteins involved in adhesion, exopolysaccharide biosynthesis, tolerance to low pH and bile salts, immunomodulation, and vitamin and enzyme production. Taken together these results, we propose the use of L. pentosus CF2-10 N as a potential and promising probiotic candidate able to colonize several niches and adapt to different lifestyles. The strain can provide attractive functional and probiotic features necessary for its application as starter culture and probiotic.
Introduction
Probiotics are defined by the Food and Agriculture Organization of the United Nations/World Health Organization (FAO/WHO) “as live microorganisms that, when administered in adequate amounts, confer a health benefit to the host” (Hill et al., 2014). In this regard, probiotic microorganisms are characterized by their diverse origin, taxonomy, fitness, effective dose, host and health benefits depending specifically on the strain employed. Thus, preliminary screening criteria for potential probiotic microorganisms include their capacity to withstand several barriers and challenges (1) in vitro, such as stressful environmental conditions; and (2) in vivo-notably during their passage through the gastrointestinal tract (acids and bile salts), their capacity to adhere and colonize human epithelial cells and their ability to produce beneficial effects in the host (antimicrobial activity, modulation of the immune system, degradation of toxic components, etc.).
In this sense, the key element for the differentiation of probiotic strains from each other is their specific functionality. Naturally, this has led to a considerable amount of research efforts put into determining the specific probiotic effect(s) of each potential probiotic strain and highlighting their potential targets over recent years (Allain et al., 2018; van de Wijgert and Verwijs, 2019; Yan et al., 2019; Yoha et al., 2022). In other words, a search for unique and attractive functional characteristics is crucial to provide new and helpful information on microorganisms with probiotic potential. This is especially important for those microorganisms that are naturally present in fermented foods, such as for example Lactiplantibacillus species.
On the other hand, probiotics as indicated by their name, act as a ‘promoter of life’ supporting in a natural way the improvement of the overall health status of the host organism (Amara and Shibl, 2015). It has further been shown that it is possible to combine several of these strains into multi-strain probiotics (Nayak, 2010), where the strains of this ‘probiotic cocktail’ can work synergistically, thus greatly increasing the overall benefit spectrum for the host (Puvanasundram et al., 2021).
The recently reclassified Lactiplantibacillus pentosus, formerly known as Lactobacillus pentosus (Zheng et al., 2020), colonizes a large set of environmental niches and therefore exhibits a huge ecological and metabolic adaptability (Anukam et al., 2013; Abriouel et al., 2017; Pérez-Díaz et al., 2021). Due to its genomic diversity and functionality, this species is found in several fermented foods (vegetables, meat, and dairy), plants, animals, vaginal, urogenital and gastrointestinal tract, while also having a large set of biotechnological and probiotic applications (Tofalo et al., 2014; Vaccalluzzo et al., 2020).
Lactiplantibacillus pentosus together with L. plantarum is an important member of the bacterial community found on the surface of olive fruits and thus represent the predominating bacteria in olive fermentation. Notably, they promote the fermentation process, conservation and extension of shelf life of the product, in addition to their role in organoleptic properties and the production of health promoting molecules such as amino acids, short chain fatty acids (SCFA), antioxidants, exopolysaccharides and vitamins (Caggianiello et al., 2016; Carrasco et al., 2018; Benítez-Cabello et al., 2019; Perpetuini et al., 2020). Furthermore, besides the production of the abovementioned molecules in foods such as olives, these bacteria are also able to produce these substances in vivo, i.e., in the gastrointestinal tract thus providing an important probiotic effect (Oguntoyinbo and Narbad, 2015; Saxami et al., 2017; Guantario et al., 2018). Consequently, several fermented foods have been classified as functional foods, as they are carriers of probiotic organisms and/or their molecules. In this regard, the health benefit and functionality of table olives goes beyond just “fermented food” due to their ability to deliver beneficial microbes adhering to the drupe epidermis into the human gastrointestinal tract where they may influence the microbial diversity and functionality (Lavermicocca et al., 2005; Rodríguez-Gómez et al., 2014, 2017).
Among olives, naturally fermented Aloreña green table olives are a promising carrier of probiotics since they are characterized by their diverse microbial community. This is mostly due to the richness of the ecosystem (soil, plant, and brine) and the progressive changes inherent to the production process (Abriouel et al., 2011).
The microbial diversity of Aloreña table olives includes lactic acid bacteria (LAB), mainly L. pentosus-yeasts and other contaminant microorganism, with microbial profiles greatly depending on the fermentation conditions (e.g., vat, fermenter or in cold). In this regard, however under vat and fermenter conditions, LAB and yeasts have been determined as the main actors (Abriouel et al., 2011). Among LAB, L. pentosus are considered potential probiotics due to their good growth capacity and survival rate under simulated gastro-intestinal conditions (acidic pH of 1.5, up to 4% of bile salts and 5 mM of nitrate), auto-aggregation, co-aggregation with pathogenic bacteria, adhesion to intestinal and vaginal cell lines, biofilm formation, fermentation of several prebiotics and their capacity to ferment lactose among others (Pérez Montoro et al., 2016). In addition, omics approaches were used by our group; including genomics, proteomics and transcriptomics, to determine and confirm the safety and functionality of the probiotic L. pentosus isolated from Aloreña table olives (Casado Muñoz et al., 2016; Pérez Montoro et al., 2018a, b; Alonso García et al., 2021, 2023).
Hence, in the present study, we extend the characterization of L. pentosus using in silico genomic analysis to unveil the genetic basis of the safety and probiotic ability of L. pentosus CF2-10 N – one of the most promising potential probiotic strains isolated from Aloreña table olives (Abriouel et al., 2012).
Materials and methods
Bacterial strain and growth conditions
Lactiplantibacillus pentosus CF2-10 N, originally isolated from naturally fermented Aloreña green table olives (Abriouel et al., 2012), was selected based on its probiotic profile as reported by Pérez Montoro et al. (2016). Lactiplantibacillus pentosus CF2-10 N was routinely cultured at 37°C in de Man, Rogosa and Sharpe (MRS) broth or agar (Fluka, Madrid, Spain) under aerobic (atmospheric) conditions for 24–48 h. The strain was kept in 20% glycerol at −80°C for long-term storage.
DNA extraction, library preparation and genome sequencing
Bacterial cells of L. pentosus CF2-10 N were harvested by centrifugation after 18 h incubation at 37°C under aerobic conditions in liquid medium. Total genomic DNA was obtained using the PureGene core kit B, according to the manufacturer’s instructions (Qiagen, Spain). DNA quantification and quality assessment were carried out using a NanoDrop 2000 spectrophotometer (Thermo Scientific), the PicoGreen ds DNA Reagent (Invitrogen) and/or agarose gel electrophoresis (0.8% agarose gel in Tris-borate-EDTA buffer, 90V, 45 min). Bacterial DNA was stored at −20°C until required.
Purified genomic DNA was sheared into 10- to 20-kb fragments using the protocol designed for DNA library preparation using the PacBio RS II System (Pacific Biosciences, Menlo Park, CA, United States). Resulting libraries (22–24 kb) were purified and sequenced using a P6-C4 DNA polymerase (Pacific Biosciences) and single-molecule real-time (SMRT) cells with a 240-min sequence capture protocol and Stage Start to maximize the subread length on the PacBio RS II.
Genome assembly and annotation
Raw sequence data were filtered (Q20) and a total of 150,292 reads were obtained with a median length of 14,991 bp. The resulting reads were assembled de novo following the Hierarchical Genome Assembly Process (HGAP3.0) approach (SMRT analysis version: 2.3.0, patch #4) for Pacific Bioscience using the WGS-Celera Assembler 7.0 (Myers et al., 2000) and Quiver algorithm (Chen-Shan Chin et al., 2013). Once assembled, the prediction of Coding DNA Sequences (CDS) was done with the help of the GenMark program (Besemer et al., 2001). Furthermore, prediction of tRNA, rRNA, and mRNA genes and signal peptides in the sequences was achieved using tRNAscan (version 2.0), RNAmmer (Version 1.2), HMMer [HMMER 3.1 (July 2017)]1 programs, respectively (Lowe and Eddy, 1997; Lagesen et al., 2007). The assembled genome sequences were annotated using the BLAST2go program version 4.1.9 (Conesa et al., 2005) followed by a complementary annotation specific for protein domains using the HMMer program [HMMER 3.1 (July 2017)] see footnote 2 and Pfam database. Furthermore, the annotation process also included blasting genes against Clusters of Orthologous Groups (COGs) of proteins using the WebMGA server (Wu et al., 2010). The circular maps of chromosome and plasmids were performed by Artemis and DNAPlotter software (Carver et al., 2005, 2009).
Genome sequencing, assembly, and annotation were done at Biopolis (Valencia, Spain). The complete genome sequence of L. pentosus CF2-10 N was deposited at the EMBL Nucleotide Sequence Database (accession number of ERR11550479).
Comparative genomic analysis of Lactiplantibacillus pentosus CF2-10 N and other Lactiplantibacillus pentosus strains
Genome sequences of L. pentosus CF2-10 N and other L. pentosus strains (MP-10, IG1 and KCA1) were aligned using MAUVE (Darling et al., 2004) available in DNASTAR Lasergene (version 17.3). Trees were then generated using RAxML with default parameters (Stamatakis, 2014). Further genome alignment and comparison of L. pentosus CF2-10 N and other L. pentosus strains (IG1 and KCA1) isolated from different ecological niches or L. plantarum WCFS1 (as reference strain) was done using the MUMmer program (version 3.0), considering alignment > 500 bp. The genome accession numbers of strains used in this study are as follows: L. pentosus IG1 (PRJEA67801), L. pentosus KCA1 (PRJNA81575, GenBank assembly accession GCA_000271445.1) and L. plantarum WCFS1 (PRJNA356, GenBank assembly accession GCA_000203855.3). Functional annotation of CDS (COG) for the three strains (L. pentosus IG1, L. pentosus KCA1 and L. plantarum WCFS1) was completed following the same strategy as for L. pentosus CF2-10 N by using reciprocal blast (BLAST2go) program version 4.1.9 (Conesa et al., 2005) and the available genome sequences in NCBI.
Genomic analysis of safety aspects and defense mechanisms of Lactiplantibacillus pentosus CF2-10 N
For specific annotation of antibiotic resistance genes (ARGs), the Resistance Gene Identifier (RGI) software (as part of the CARD “The Comprehensive Antibiotic Resistance Database” tools; Alcock et al., 2020) was used for the prediction of the L. pentosus CF2-10 N resistome from protein or nucleotide data based on homology and SNP (Single Nucleotide Polymorphism) models, employing the CARD’s curated AMR (antimicrobial resistance) detection models (last accessed in March 2022). In addition, the genome of L. pentosus CF2-10 N was investigated for acquired antibiotic resistance genes/chromosomal mutations mediating antimicrobial resistance through the ResFinder3 software version 4.1 (Zankari et al., 2012; Bortolaia et al., 2020) with selected %ID threshold of 90.00% and selected minimum length of 60% (last accessed in March 2022).
Regarding virulence factors (VFs), the predicted CDSs were annotated using reciprocal BLAST against the Virulence Factors of Bacterial Pathogens (VFDB) database. Hits were considered positive when the results of reciprocal BLAST were similar, employing a 80% sequence similarity cut-off (Liu et al., 2019).
Concerning mobile genetic elements, the annotated genome sequence of L. pentosus CF2-10 N was screened for the presence of conjugative plasmid, transposase, transposon, IS elements and prophage coding genes. The genome was searched for Insertion Sequences (IS) using the ISfinder search tool (Zhang et al., 2000). Furthermore, complementary information on prophage DNA within the L. pentosus CF2-10 N genome was obtained by using bioinformatic tools such as PHASTER’s version (PHAge Search Tool Enhanced Release, last updated March 2016; corresponding to the updated prophage/virus database PHAST “PHAge Search Tool”) for the rapid identification and annotation of prophage sequences within bacterial genomes and plasmids (Zhou et al., 2011; Arndt et al., 2016).
Finally, the annotated genome sequence of L. pentosus CF2-10 N was screened for the presence of CRISPR (Clustered Regularly Interspaced Short Palindromic Repeats) coding genes and the localization of CRISPR RNAs targets was identified using the CRISPRDetect program version 2.4 (Biswas et al., 2016).4
Genomic analysis of probiotic properties of Lactiplantibacillus pentosus CF2-10 N
To identify the putative genes associated with probiotic characteristics in L. pentosus CF2-10 N, the annotated genome sequence was screened for the presence of genes coding for proteins involved in cell adhesion (mucus binding proteins, cell surface proteins and moonlighting proteins among others), exopolysaccharide (EPS) biosynthesis, tolerance to low pH and bile salts, enzyme production, vitamin biosynthesis and host immunomodulation.
Results
General genomic features of a probiotic Lactiplantibacilluis pentosus CF2-10 N
The analysis revealed that the Lactiplantibacillus pentosus CF2-10 N genome consisted of a single circular chromosome of 3,645,747 bp, with an estimated mol% G + C content of 46.42% and 4 plasmids ranging 58–120 kb (Figure 1). The annotated genome sequence (Figure 1) revealed 3,713 open reading frames (ORFs), of which 75.4% (2,801) were attributed to a COG (Cluster of Orthologous Groups) family and/or were given a functional description (Supplementary Table S1). Furthermore, 16 rRNA genes were predicted in L. pentosus CF2-10 N genome using RNAmmer (version 1.2), while 67 tRNA encoding sequences were identified corresponding to all 20 amino acids and three undermined amino acids (Supplementary Table S2).
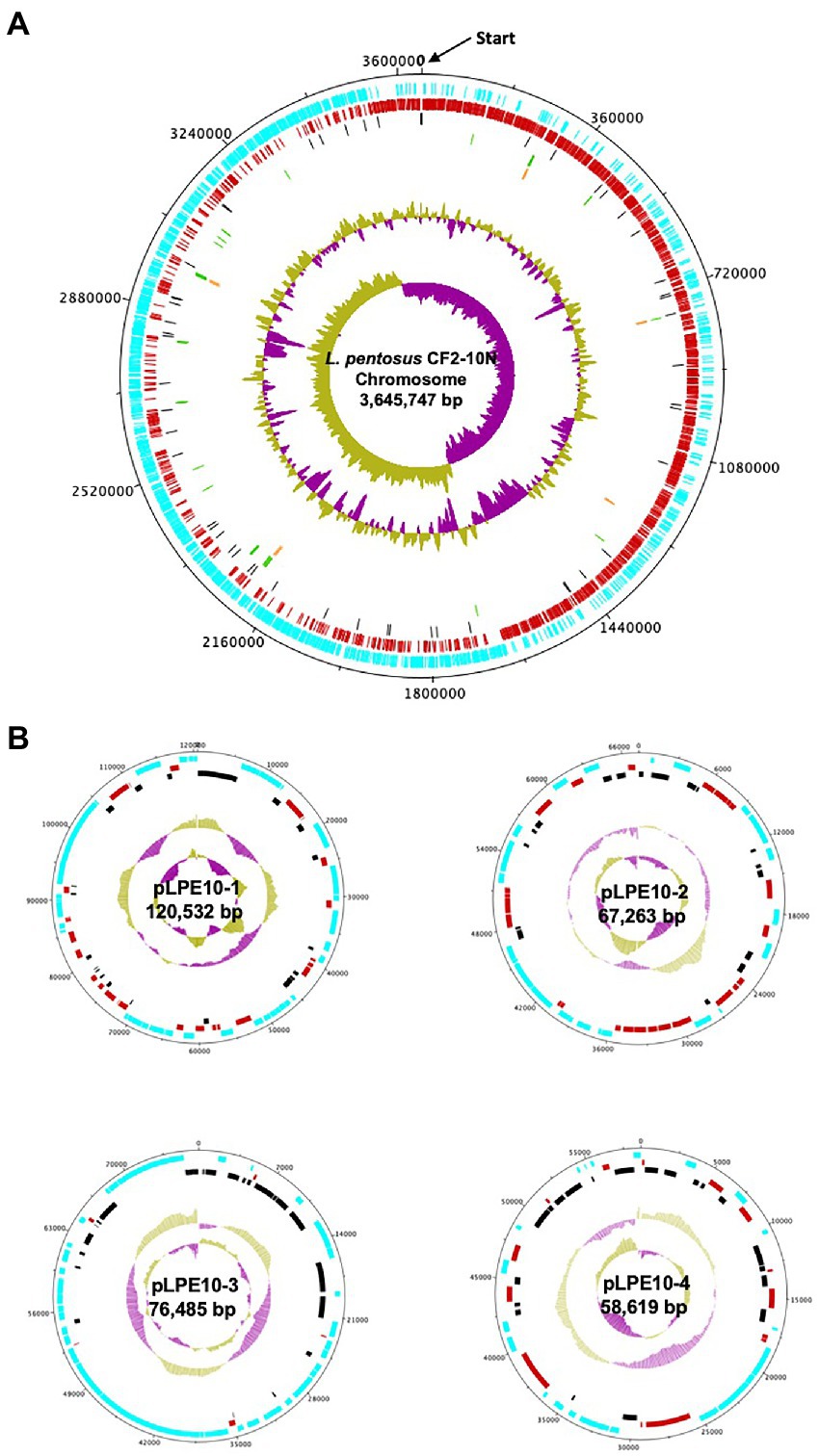
Figure 1. Circular map of the Lactiplantibacillus pentosus CF2-10N chromosome (A) and four plasmids (B). The circles from outside to inside are the annotated Coding DNA Sequences (CDS) elements in forward orientation (blue); the annotated CDS elements in the reverse orientation (red); the Pseudogenes (black); the tRNA (green); the rRNA (orange); the %GC plot and the GC skew.
Supplementary Figure S1 shows the biological processes, the cellular components and the molecular function frequencies predicted in L. pentosus CF2-10 N. Among the Gene Ontology (GO) terms, those related to biological processes such as oxidation–reduction process, regulation of transcription, DNA-templated transcription and DNA-templated transmembrane transport were the most identified. Regarding molecular function, ATP-binding and DNA binding were the most prevalent. However, in both biological process and molecular function about 1,250–1,550 genes have no known biological process/function (Supplementary Figure S1).
The most abundant COG category of L. pentosus CF2-10 N genome, except for “[S] Function unknown” (273 CDSs, 9.7%), was “[R] General function prediction only” (336 CDSs, 12%), followed by “[G] Carbohydrate transport and metabolism” (307 CDSs, 11%), “[K] Transcription” (235 CDSs, 8.4%), “[L] Replication, recombination and repair” (213 CDSs, 7.6%) and “[E] Amino acid transport and metabolism” (192 CDSs, 6.9%), accounting for 45.9% of the overall CDS (1,283/2,801 CDSs; Supplementary Table S3).
Comparative genome analysis of Lactiplantibacillus pentosus CF2-10 N
Comparative genomic analysis of L. pentosus CF2-10 N and L. pentosus MP-10 isolated from the same ecological niche (Aloreña table olives) showed that both L. pentosus strains shared 99.87% identity as revealed by sequence alignment using the MAUVE algorithm. This high similarity was further highlighted by large blocks of colinearization in the MAUVE alignment, being the synteny of genes similar, although inversion, insertion and rearrangement occurred (Figure 2). Besides L. pentosus MP-10 (isolated from Aloreña table olives), comparison with other L. pentosus strains by genome sequence alignment (using MAUVE), notably IG1 (isolated from olives) and KCA1 (isolated from the vaginal tract), revealed genetic differences among the studied strains (Supplementary Figure S2A). To illustrate this relationship, a maximum-likelihood core genome tree was constructed using RaxML which showed higher phylogenetic similarity in the case of L. pentosus CF2-10 N and MP-10 strains (evolutionary distance “ED,” ED = 0), followed by L. pentosus IG1 (ED = 0.02) and then L. pentosus KCA1 (ED = 0.08; Supplementary Figure S2B).
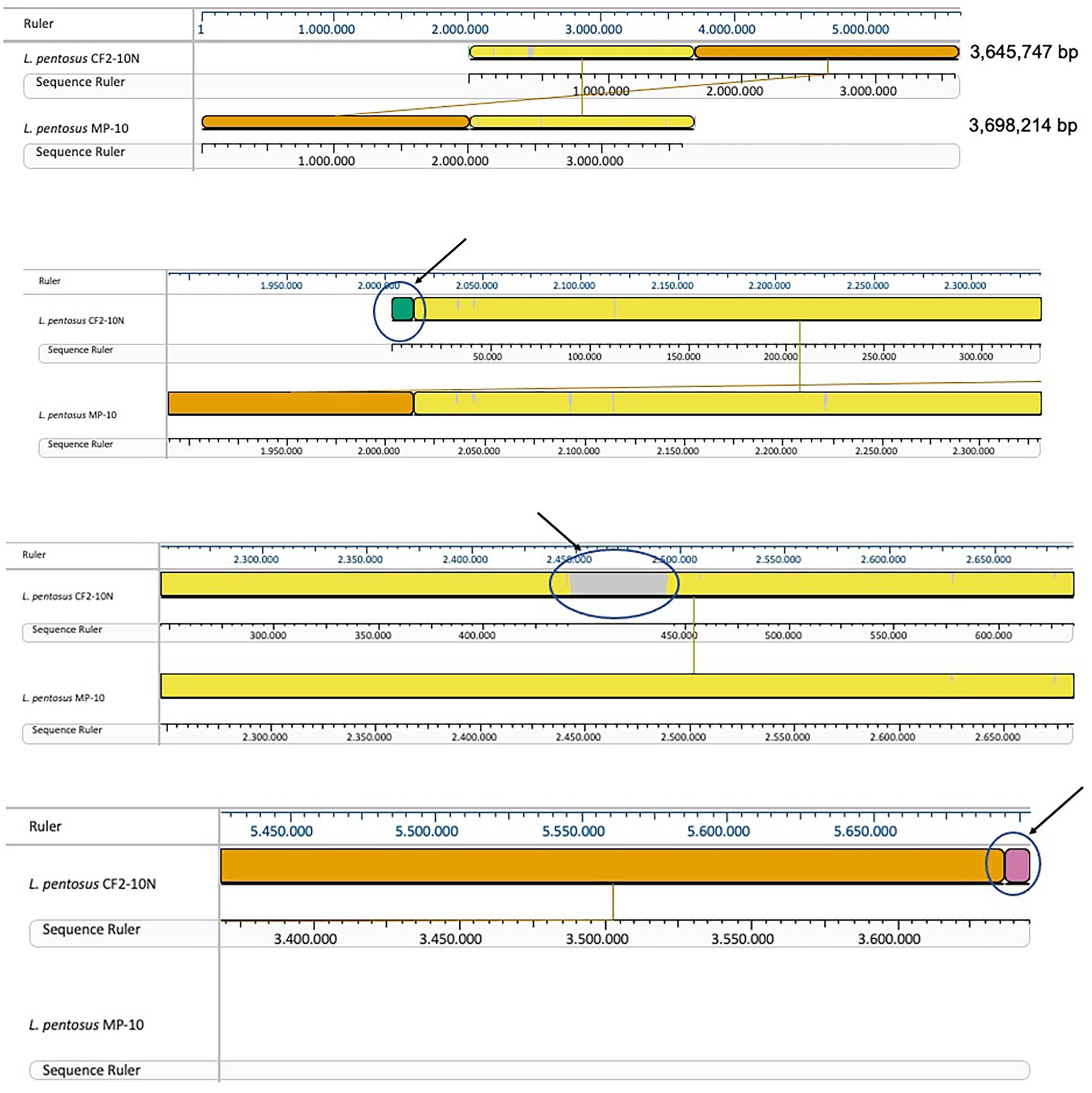
Figure 2. Mauve visualization of whole genome alignment of L. pentosus CF2-10N with L. pentosus MP-10.
The synteny linkage of L. pentosus CF2-10 N against L. pentosus IG1 and KCA1 strains or L. plantarum WCFS1 was further analyzed using the MUMmer program and represented using Circos (Figure 3; Supplementary Tables S4–S6). Here, the genome comparison revealed the presence of highly conserved syntenic blocks between L. pentosus strains (IG1 and KCA1; Figures 3A,B), and to a lesser extent with L. plantarum WCFS1 (Figure 3C). On the other hand, comparison of the number of unique and shared annotated genes of L. pentosus CF2-10 N and other strains (L. pentosus IG1, L. pentosus KCA1 or L. plantarum WCFS1) using reciprocal blast revealed unique genomic features in L. pentosus CF2-10 N (Figure 4).
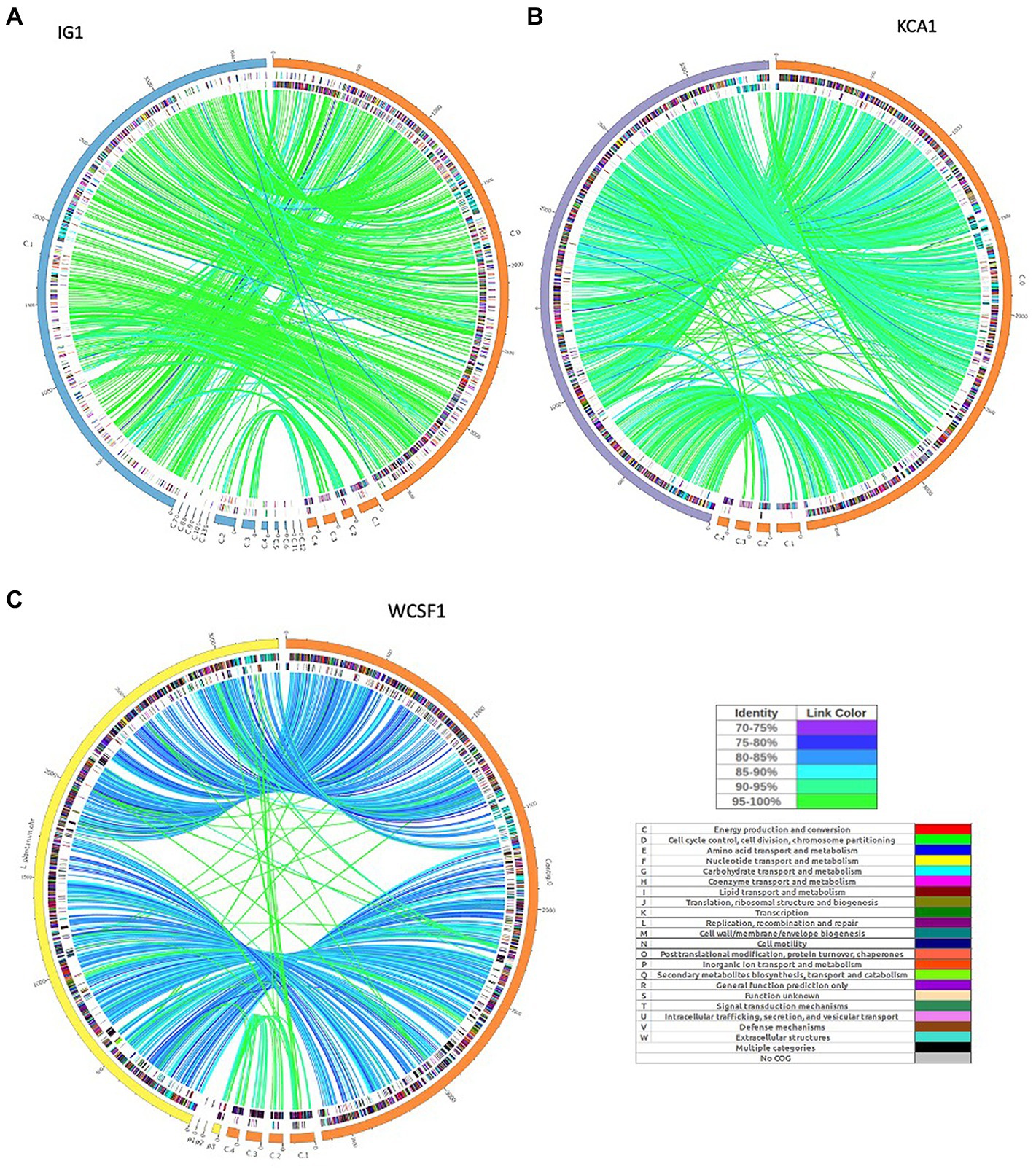
Figure 3. Circos map showing genome synteny between the genetic linkage map of L. pentosus CF2-10N and the reference genome sequences: L. pentosus IG1 (A), L. pentosus KCA1 (B) or L. plantarum WCFS1 (C). Color synteny linkages were generated using Circos. Rings from outside to inside are genomes of L. pentosus CF2-10 N (orange) and L. pentosus IG1 (blue), L. pentosus KCA1 (purple) or L. plantarum WCFS1 (yellow); shared Cluster of Orthologous Groups of proteins (COG) annotated coding sequences between L. pentosus CF2-10 N and the reference strain as analyzed by reciprocal blast (BLAST2go) colored by their COG annotation; and the unique Cluster of Orthologous Groups of proteins (COG) annotated coding sequences of each genome (L. pentosus CF2-10 N and the reference strain).
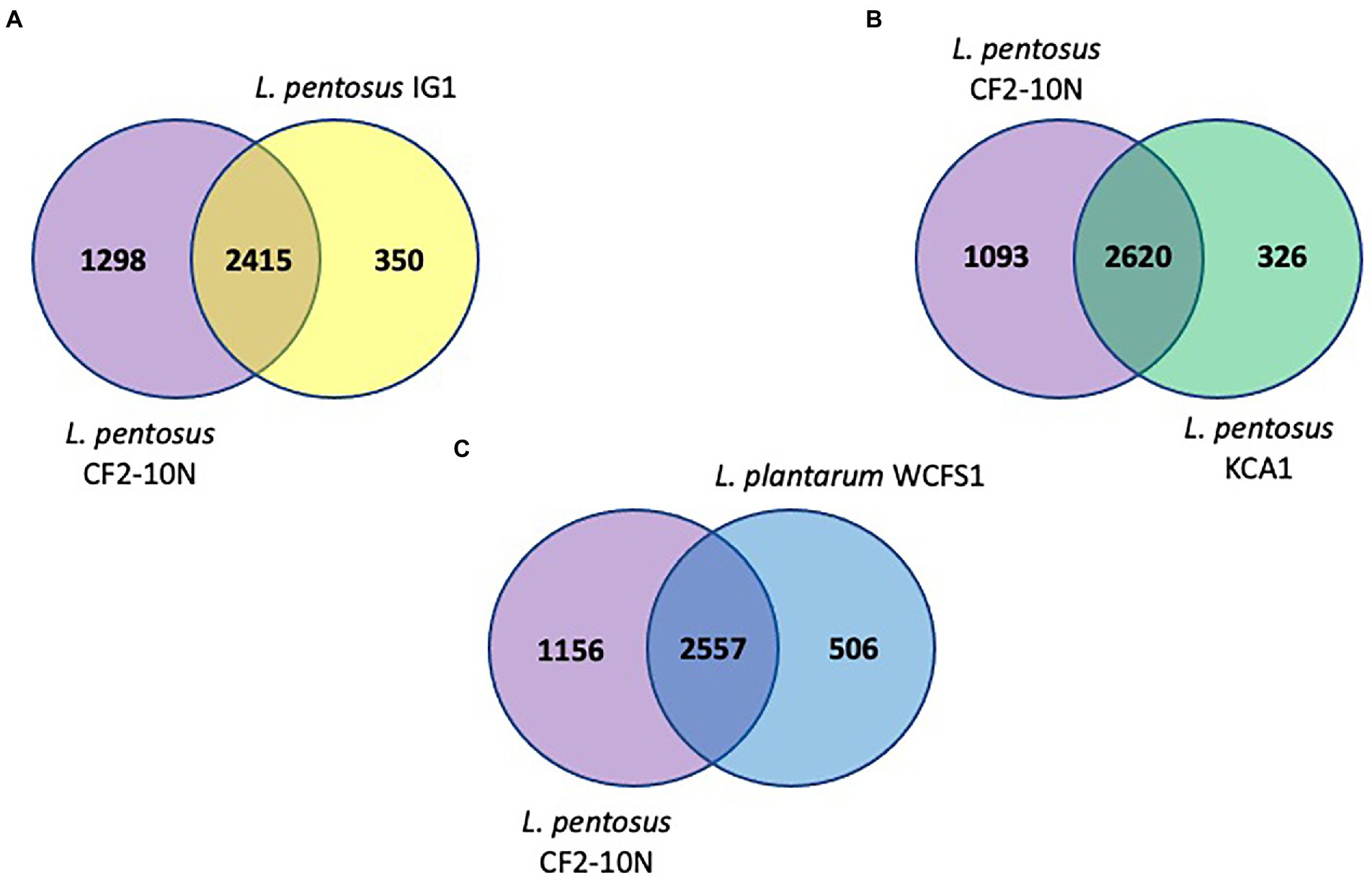
Figure 4. Venn diagrams showing number of reciprocal best hits among L. pentosus CF2-10 N and other lactobacilli subset of core genomes. (A) Number of shared and unique genes of core genomes of L. pentosus CF2-10N and L. pentosus IG1. (B) Number of shared and unique genes of core genomes of L. pentosus CF2-10N and L. pentosus KCA1. (C) Number of shared and unique genes of core genomes of L. pentosus CF2-10N and L. plantarum WCFS1.
Finally, L. pentosus CF2-10 N appears to share both core and accessory annotated genes with L. pentosus KCA1 (88.93% hits, Figure 4B) and L. pentosus IG1 (87.34% hits; Figure 4A) and to a slightly lesser extent with L. plantarum WCFS1 (83.48%, Figure 4C).
In silico analysis of safety determinants and defense mechanisms of Lactiplantibacillus pentosus CF2-10 N
Safety properties are a crucial feature of potential probiotic strains and their determination is considered a priority when characterizing a new potential probiotic. Hence, in a first step, antibiotic resistance and virulence determinants were screened in the L. pentosus CF2-10 N genome sequence. To do so, in silico prediction of antibiotic resistance genes (ARG) was done against the Comprehensive Antibiotic Resistance Database (CARD) using the RGI tool v3.2.1 available in the CARD database5 which used archive’s curated AMR (antimicrobial resistance) detection models. Results indicated no ARG in the L. pentosus CF2-10 N genome sequence. Thus, neither resistance genes nor mutations conferring antibiotic resistance was predicted in the complete resistome of L. pentosus CF2-10 N. However BLAST2go annotation revealed the presence of non-specific antimicrobial resistance mechanisms relying on efflux transporters or transmembrane proteins involved in response to antibiotics such as ABC transporter ATP-binding protein (encoded by LPE_03051, LPE_00789, FD24_GL000501 genes), TIGR00374 family protein (encoded by mprF gene), undecaprenyl-diphosphatase (encoded by uppP gene), QacE family quaternary ammonium compound efflux SMR transporter (encoded by FD24_GL003284 gene), MATE family efflux transporter (encoded by LPE_00986 gene) and cation efflux pump (encoded by FD24_GL002035 gene).
With regard to acquired resistance by horizontal gene transfer, ResFinder did not detect any acquired antibiotic resistance genes for aminoglycoside, beta-lactam, colistin, disinfectant, fluoroquinolone, fosfomycin, fusidic acid, glycopeptide, MLS-series (Macrolide, lincosamide and streptogramin B), nitroimidazole, oxazolidinone, phenicol, pseudomonic acid, rifampicin, sulphonamide, tetracycline and trimethoprim (data not shown).
Regarding virulence, the predicted CDSs annotated using reciprocal BLAST against VFDB (database including only experimentally validated virulence factors) did not identify any known virulence factors including toxins.
Analysis of the L. pentosus CF2-10 N mobilome showed that the bacterial genome included 66 transposases: 19 transposases, 1 transposase family protein A and 46 transposases belonging to nine IS transposase families (4 IS3, 6 IS5, 5 IS21, 17 IS30, 4 IS66, 3 IS1380, 2 ISL3, 2 DDE, 2 IS6501, 1 IS200/IS605), mainly located on plasmids (pLPE10-1, pLPE10-2 and pLPE10-4) rather than on the chromosome (50 on plasmids/16 on chromosome) and appearing in multiple copies ranging from two to five (Table 1). IS30 family transposases were abundant (17 of 66 transposases) and were represented by seven different genes (Table 1). Furthermore, Blastp alignment of transposase protein sequences detected in L. pentosus CF2-10 N genome showed high similarity with L. pentosus (29 of 66 transposases, 98.9–100%), L. plantarum (11 of 66 transposases, 95.2–100%) and other lactobacilli. It is noteworthy to indicate the presence of 34 paired (adjacent to each other in the genome) transposase genes (2 or 3 genes) being different genes or belonging to different families and located on both chromosome and plasmids (Table 1). Regarding IS elements, 45 CDS were predicted distributed into 16 different families and in various bacteria (Table 2). Here, IS30 and IS3 were the most detected elements followed by IS5 (Table 2).
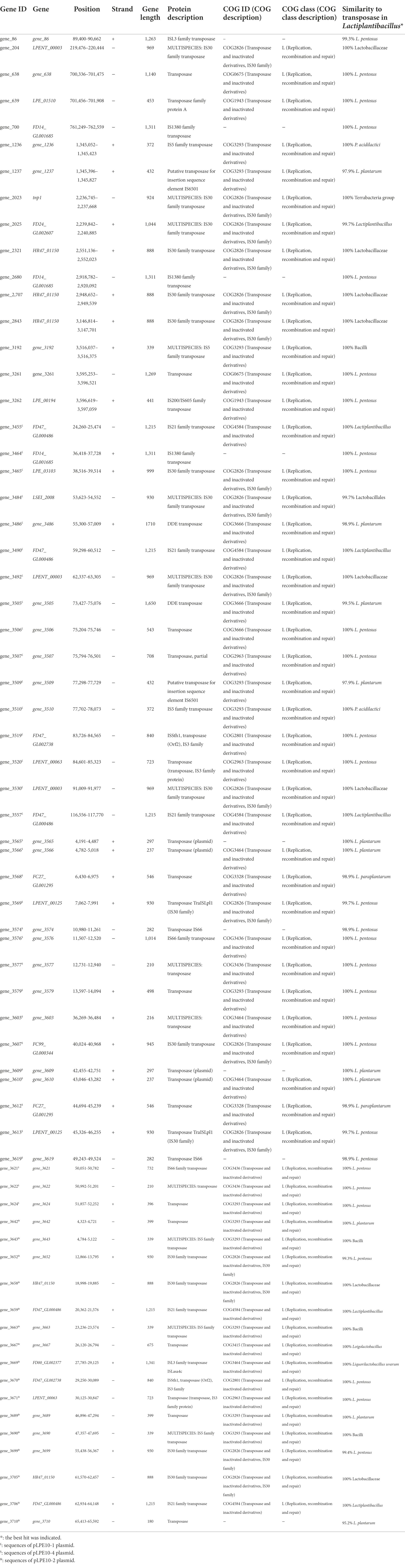
Table 1. Characterization of transposases predicted in the Lactiplantibacillus pentosus CF2-10N genome.
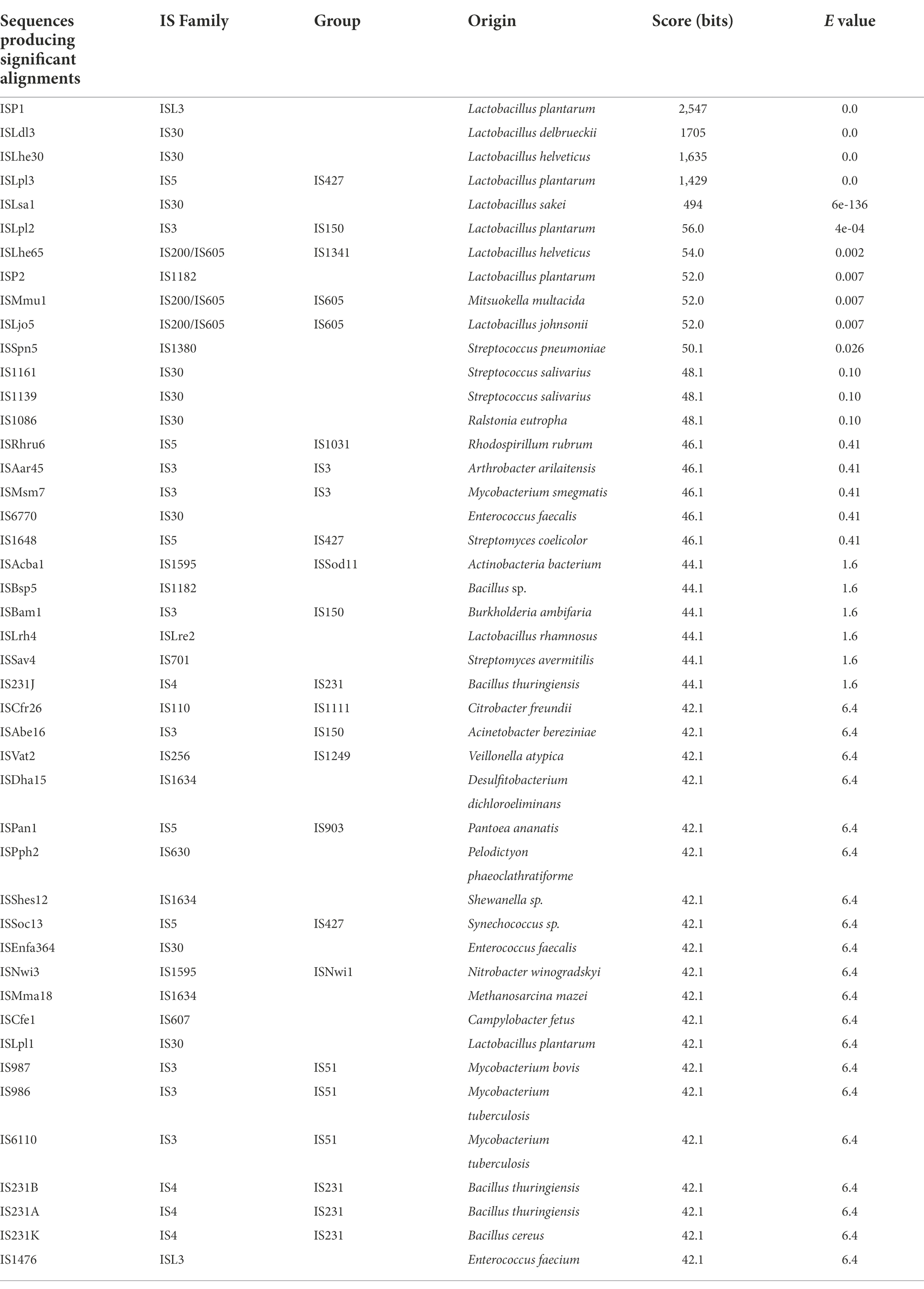
Table 2. Characterization of IS elements found within the genome of Lactiplantibacillus pentosus CF2-10 N using the ISfinder search tool.
On the other hand, screening for prophage DNA within the L. pentosus CF2-10 N genome, using bioinformatic tools such as PHASTER, determined the presence of eight temperate phage regions (Table 3). Two regions were intact (Regions 2 and 3, score > 90), the other three were questionable (Regions 5, 6 and 7, score 70 ± 90), and the last three regions were incomplete (Regions 1, 4 and 8, score < 70). The complete prophage regions of the L. pentosus CF2-10 N chromosome were identified as Lactobacillus phage Sha1 (Regions 2 and 3; GC content, 41.55–41.88%; region length, 39.9–47.7 kb). Regarding the questionable prophage regions, they corresponded to Staphylococcus phage SP beta-like (Regions 5 and 6; GC content, 34.83–40.70%; region length, 13.7–19.4 kb) and Escherichia phage 500,465–1 (Region 7; GC content, 41.54%; region length, 18.8 kb). With respect to the incomplete prophage region, we identified three regions corresponding to Lactobacillus phage PLE3 (Region 1; GC content, 41.26%; region length, 15 kb), Enterobacteria phage fiAA91-ss (Region 4; GC content, 38.27%; region length, 23.4 kb) and Escherichia phage 500,465–1 (Region 8; GC content, 31.68%; region length, 6.7 kb; Table 3).
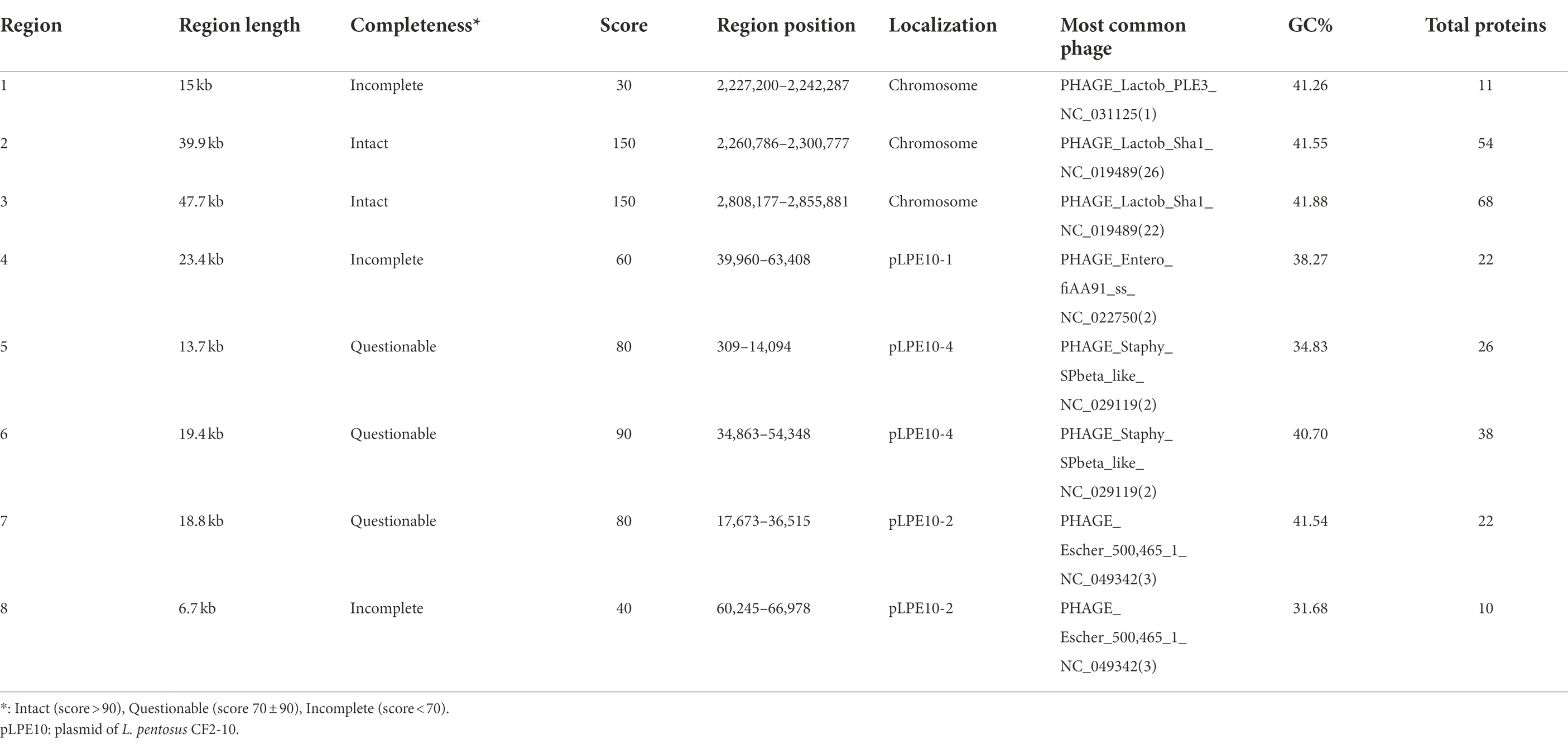
Table 3. Description of prophage regions detected in the Lactiplantibacillus pentosus CF2-10N genome by using the PHASTER bioinformatic tool.
Among the defense mechanisms revealed by in silico analysis of the L. pentosus CF2-10 N genome sequence, CRISPR I and II systems (both signature genes for the Type I “cas3” and Type II “cas9” systems) were detected as defense response to mobile genetic elements (i.e., viruses, transposable elements and conjugative plasmids; Table 4). In this sense, 13 genes were identified as CRISPR associated protein responsible genes (cas genes) organized in two operons (Supplementary Figure S3), and six of them were new genes found in the L. pentosus CF2-10 N genome (Table 4). Regarding CRISPR arrays (CR), five CRISPR unquestionable arrays were identified by using the CRISPRDetect program and they are distributed throughout the genome sequence between 1,791,840 and 3,235,959 bp (Table 5).
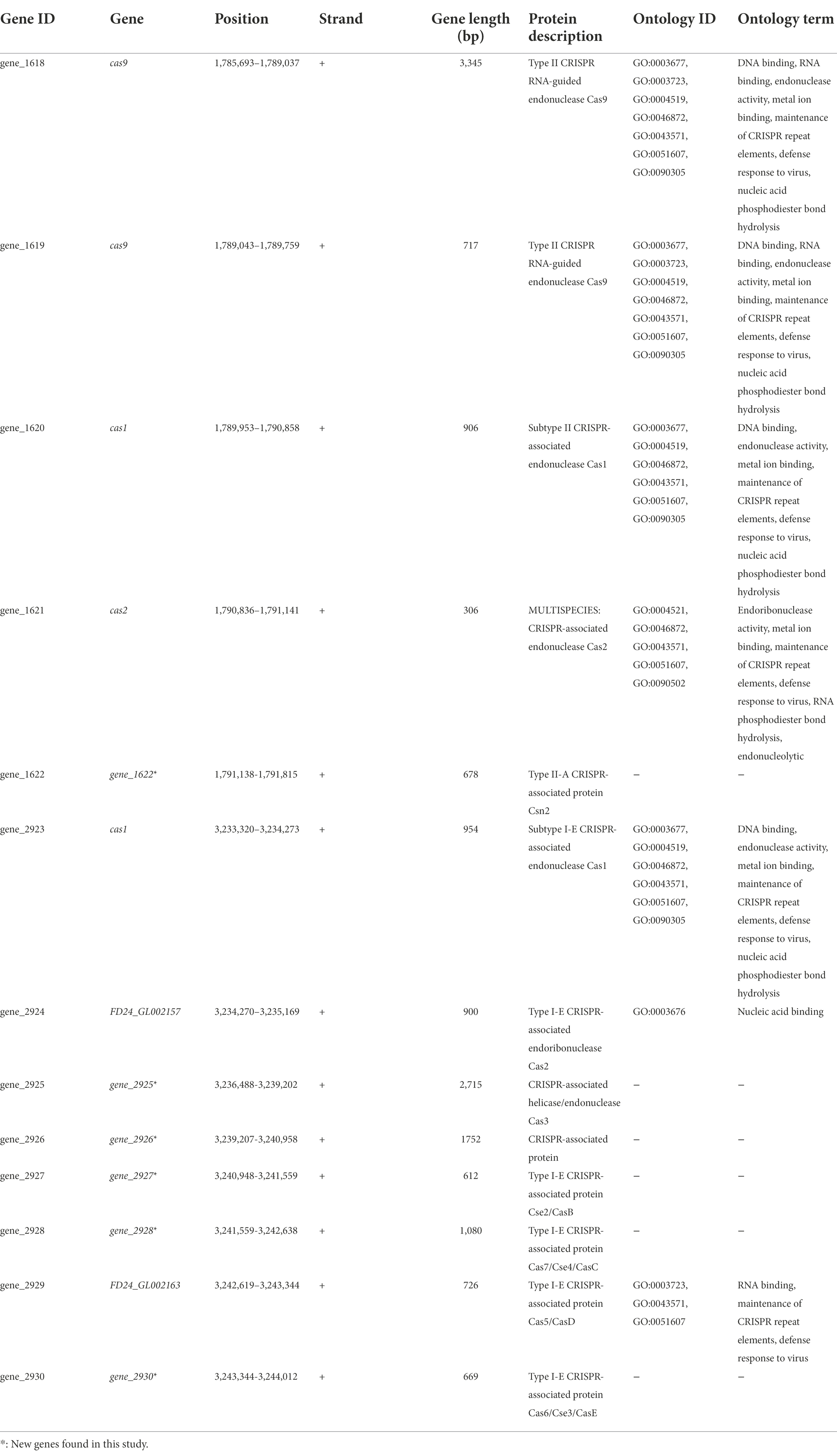
Table 4. Characterization CRISPR associated proteins predicted in the Lactiplantibacillus pentosus CF2-10N genome.
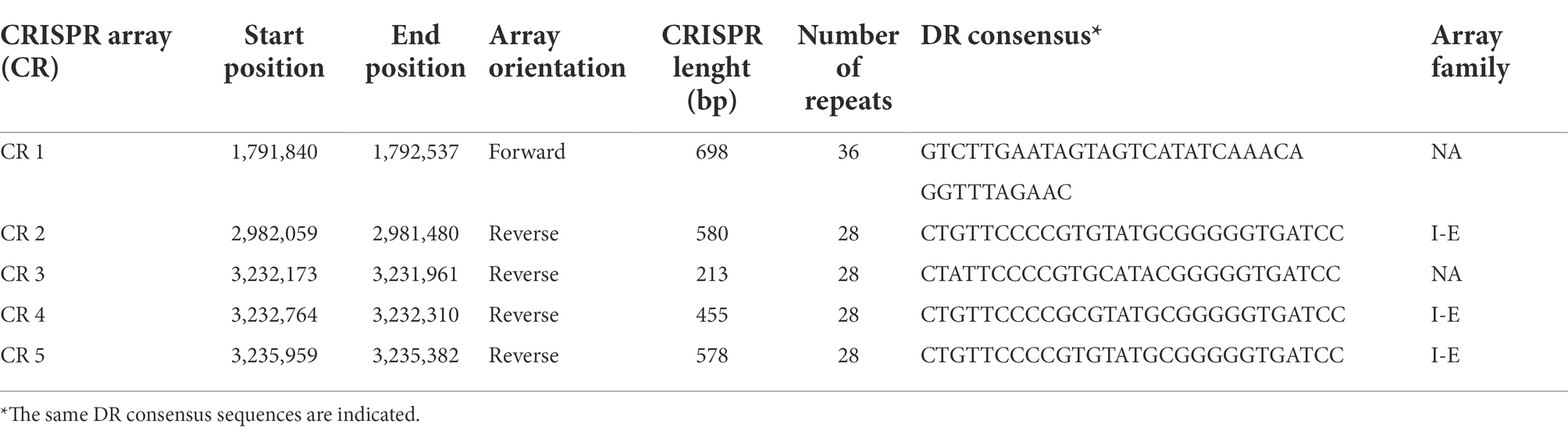
Table 5. Characterization of CRISPR arrays predicted in the Lactiplantibacillus pentosus CF2-10N genome.
Identification of genes associated with probiotic characteristics in Lactiplantibacilluis pentosus CF2-10 N
In silico genome analysis of probiotic characteristics of L. pentosus CF2-10 N revealed the presence of genes coding for adhesion, exopolysaccharide biosynthesis, tolerance to low pH and bile salts, vitamin and enzyme production and immunomodulation among others (Table 6). With respect to adhesion, several genes were identified such as 3 mucus-binding proteins, 1 fibronectin/fibrinogen-binding protein, 1 Chitin-binding protein (located on pLPE10-1 plasmid), 1 ABC superfamily ATP binding cassette transporter, binding protein, 2 cell surface proteins, 1 manganese ABC transporter substrate-binding protein, 1 elongation factor Tu, 1 Molecular chaperone DnaK, 1 molecular chaperone GroEL, 1 co-chaperone GroES, 1 class A sortase and 1 type I glyceraldehyde-3-phosphate dehydrogenase (Table 6). Regarding exopolysaccharides, four genes coding for exopolysaccharide biosynthesis protein were identified (Table 6). For adaptation to different lifestyles, L. pentosus CF2-10 N harbored in its genome several genes involved in stress response such as acids and bile. These included three GNAT family acetyltransferases, two Na+/H+ antiporter NhaC, 1 phosphoglycerate mutase, nine elongation factors (factor G, factor GreA, factor 4, factor P, factor Ts and factor Tu) and 1 phosphoglycerate kinase (Table 6).
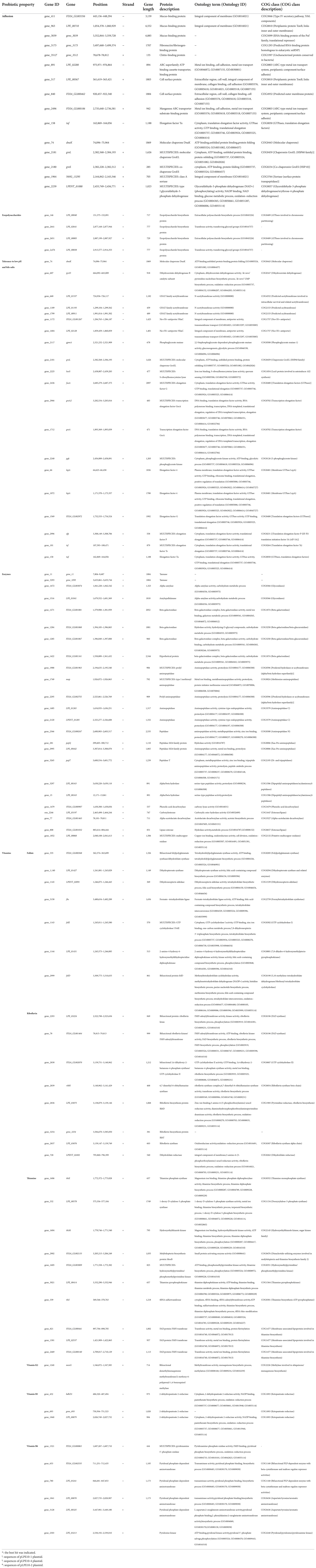
Table 6. Characterization of genes associated with probiotic properties predicted in the Lactiplantibacillus pentosus CF2-10N genome.
On the other hand, several genes were identified coding for enzymes involved in probiotic functions such as two genes coding for tannase (exclusive to this strain), 1 alpha-amylase, 1 amylopullulanase, 3 beta-galactosidases, 5 aminopeptidases, 1 lipase esterase, 4 peptidases, 2 alpha/beta hydrolases, 1 phenolic acid decarboxylase, 1 carboxylesterase, 1 alpha-acetolactate decarboxylase, and 1 multicopper oxidase (Table 6).
With respect to vitamin biosynthesis, we detected genes coding for proteins involved in vitamins B1 or thiamine (10 genes), B2 or riboflavin (8 genes), B5 (3 genes) and B6 (6 genes), folate (7 genes) and vitamin K2 or menaquinone (1 gene) production (Table 6). In this regard, vitamin production ability of L. pentosus CF2-10 N was validated in vitro.
Discussion
Aloreña table olives, naturally fermented traditional green olives with a denomination of protection (DOP), are considered as potential source of probiotic L. pentosus strains with high genetic diversity (Abriouel et al., 2012). Several L. pentosus strains isolated from Aloreña table olives throughout the fermentation process were shown to be potential probiotics, with L. pentosus MP-10, L. pentosus CF1-6 and L. pentosus CF2-10 N as the best candidates (Pérez Montoro et al., 2016). Among these strains, L. pentosus CF2-10 N was selected for a more in-depth analysis in the current study on the basis of its excellent probiotic properties. These include notably good growth capacity and survival under simulated gastro-intestinal conditions (acidic pH of 1.5, up to 4% of bile salts and 5 mM of nitrate), good ability to auto-aggregate and co-aggregate with pathogenic bacteria, adherence to intestinal and vaginal cell lines, antimicrobial activity by means of plantaricins and fermentation of prebiotics and lactose (Pérez Montoro et al., 2016). It is also noteworthy that L. pentosus CF2-10 N was isolated from the same ecological niche as the potential previously described probiotic L. pentosus MP-10 (Abriouel et al., 2012), hence, they are exposed to the same ecological conditions and pressure (soil, plant and brine) as well as the same progressive changes throughout the production process. It is thus not surprising that their genetic relatedness is further highlighted by shared genetic, functional and probiotic properties although both strains showed different genomic profiles belonging to different clusters or genomic groups (G1 and G2) as reported by Abriouel et al. (2012). In this sense, both strains harbor a single circular chromosome of similar size of 3,698,214 bp (L. pentosus MP-10, GC content of 46.32%) and 3,645,747 bp (L. pentosus CF2-10 N, GC content of 46.42%) and 4 (L. pentosus CF2-10 N, 58–120 kb) to 5 (L. pentosus MP-10, 29–46.5 kb) plasmids (Abriouel et al., 2016). This similarity highlights the effect of the ecosystem (soil, plant and brine) on the genetic diversity of microbial communities present in Aloreña table olives.
A comparison with other bacterial strains from table olives showed similarities in genomic size and GC content. These strains included L. pentosus IG1 harboring a circular chromosome of 3,687,424 bp (GC content of 44.9%) and 7 plasmids (2.5–125.9 kb; Maldonado-Barragán et al., 2011), L. pentosus strains (IG8, IG9, IG10 and IG11) recovered from biofilms on the skin of green table olives with circular chromosome sizes in the range of 3,787,967 to 3,811,295 bp (GC content of 45.9–45.95%) and 6 to 7 plasmids (Calero-Delgado et al., 2019) and L. pentosus O17 isolated from brines of treated table olives (Cerignola cv.) with a circular chromosome of 3,850,701 bp (GC content of 45.9%; Zotta et al., 2022). This fact indicated their adaptation to a brine-specific lifestyle notably in relation to genes involved in carbohydrate transport and metabolism (307 CDSs and 279 in L. pentosus CF2-10 N and MP-10, respectively) and amino acid metabolism (192 CDSs and 173 in L. pentosus CF2-10 N and MP-10, respectively), among others. On the other hand, the presence of plasmids in L. pentosus isolated from table olives highlight their key role in the fermentation process. In this sense, Abriouel et al. (2019) reported that L. pentosus MP-10 plasmids play an important role as metal bioquencher reducing the amount of these potentially toxic elements in humans and animals, food matrices, and in environmental bioremediation.
Duar et al. (2017) reported a high level of niche conservatism within the well-supported phylogenetic groups of the genus Lactobacillus (including the recently reclassified genus Lactoplantibacillus), with lifestyles ranging from free-living with large genome size to strictly symbiotic or host adapted with small genome size. Considering that the metabolic and physiological properties of L. pentosus strains are reflective of their lifestyle, strains isolated from fermented table olives are characterized by their large genome size of 3.6–3.8 Mbp encoding a versatile repertoire of enzymes to utilize a wide spectrum of substrates available in brines. Comparative genomic analysis of both strains isolated from Aloreña table olives - L. pentosus MP-10 and L. pentosus CF2-10 N- demonstrated their close phylogenetic relation (ED = 0) and a high similarity although some event traits (inversion, insertion or gene rearrangement) occurred, conferring exclusive features to L. pentosus CF2-10 N. However, when genomic comparison was done with L. pentosus IG1 isolated from the Spanish-Style Green Olive fermentation (different ecological niche than Aloreña table olives), genetic differences (ED = 0.02) were detected which were further increased when compared with L. pentosus KCA1 isolated from vagina (ED = 0.08). The ecological adaptability of L. pentosus is thus highly dependent on the ecological niche, with the specific environmental and fermentation conditions and olive material being the key elements to determine the genetic diversity.
Concerning the safety properties of L. pentosus CF2-10 N, no ARGs were detected in the genome sequence, however non-specific antimicrobial mechanisms such as mutation in ddl gene coding for D-Ala-D-lactate in the peptidoglycan instead of the normal dipeptide D-Ala-D-Ala (position 260) and /or efflux transporters or transmembrane proteins were found responsible of the strain’s phenotypic resistance to streptomycin and vancomycin as detected by antibiotic susceptibility testing (Casado Muñoz et al., 2014). Furthermore, in silico analysis of antibiotic resistance in L. pentosus CF2-10 N showed the absence of acquired antibiotic resistance genes. Thus, we can conclude that the resistome is mostly represented by efflux-pump resistance genes or other alternative resistance mechanisms responsible for the intrinsic resistance exhibited by this strain as mentioned above. On the other hand, no virulence determinants were detected in the L. pentosus CF2-10 N genome. Taken together these results, we suggest for L. pentosus CF2-10 N to be considered as safe for food processing as well as probiotic.
Regarding the mobilome (corresponding to genetic elements able to move within a genome or between different genomes), this consists of 66 transposases, 45 IS elements and 8 temperate phage regions in the L. pentosus CF2-10 N genome. The high number and the great diversity of transposases and IS elements identified by in silico analysis of the L. pentosus CF2-10 N genome indicated a frequent genetic diversification within the L. pentosus CF2-10 N genome, which is notably higher than in other lactobacilli such as L. plantarum WCFS1 (36 genes), L. pentosus KCA1 (25 genes), L. pentosus DSM 20314 (14 genes) or L. pentosus IG1 (5 genes; Abriouel et al., 2017). Interestingly, L. pentosus CF2-10 N showed an even higher genetic diversification in comparison to L. pentosus MP-10 (29 genes), even though both strains are isolated from the same ecological niche (Abriouel et al., 2017). Furthermore, most of transposases belonged to IS30 families frequently located on plasmids, while the IS were mainly represented by IS30 and IS3 found in various bacteria and being responsible for information transfer and extreme adaptation. This fact suggests the high adaptability potential of L. pentosus CF2-10 N enabling the bacterium to withstand different environmental and gut stress conditions. Furthermore, the presence of eight prophage regions in the L. pentosus CF2-10 N genome highlights once more the genetic diversity and fitness of its genome, conferring a selective advantage for the survivability and resistance of this strain in view of the potential risk of losses associated with phage infection in different ecosystems. The presence of prophages in lactobacilli genomes is widely distributed (more than 92%, Sun et al., 2015) and is species-specific (Pei et al., 2021), while being highly dependent on the habitat. In this regard L. pentosus CF2-10 N contained intact lactobacilli prophage and incomplete or questionable prophage fragments similar to other bacteria (Staphylococcus, Escherichia and Enterobacteria phages) indicating its adaptability to harsh conditions (fermentation) which may confer flexibility against various stress triggers (phages from different sources such as air, water or soil). Other defense mechanisms were predicted in the L. pentosus CF2-10 N including a CRISPR system (CRISPR-I and CRISPR-II) represented by five CRISPR unquestionable arrays and 13 CRISPR associated proteins (six of them were exclusive of this strain) organized in two operons. This acquired immunity system, which provides protection against mobile genetic elements (conjugative plasmids, transposable elements, and phages) in L. pentosus CF2-10 N, was slightly different from L. pentosus MP-10 isolated from the same ecological niche. Notably, 11 CRISPR associated proteins and 9 CRISPR arrays (3 of them were questionable CRISPRs) were detected in L. pentosus MP-10, which indicated that the increased fitness greatly depends on the strain itself, under changing ecological lifestyles. Among the six newly detected genes, the CRISPR-I system was found to be coding for a Type II-A CRISPR-associated protein Csn2, involved in CRISPR adaptation for new spacer acquisition (Nam et al., 2011) and was associated with the cas9-cas1-cas2 cassette. Furthermore, the other genes (gene_2925 [cas 3] and a cascade of five genes coding for Type I-E CRISPR associated proteins) were found to be involved in interference and infection neutralization as reported by Xue and Sashital (2019).
Concerning functional properties, L. pentosus CF2-10 N genome analysis revealed the presence of genes coding for adhesion, exopolysaccharide biosynthesis, tolerance to low pH and bile salts, immunomodulation, as well as vitamin and enzyme production. In this context, the adhesion capacity exhibited by this strain in vitro to Enterocyte-like Caco-2 ECACC86010202 (from colon adenocarcinoma) and HeLa 229 ECACC86090201(from vaginal cervix carcinoma) cells (Pérez Montoro et al., 2016) was confirmed by the presence of genes coding for several adhesion/multifunctional proteins such as mucus-binding proteins, fibronectin/fibrinogen-binding protein, Chitin-binding protein, ABC superfamily ATP binding cassette transporter, binding protein, cell surface proteins, manganese ABC transporter substrate-binding protein, elongation factor Tu, Molecular chaperone DnaK, molecular chaperone GroEL, co-chaperone GroES, class A sortase and type I glyceraldehyde-3-phosphate dehydrogenase. These proteins were reported to be involved in the adhesion to intestinal epithelial cells (Granato et al., 2004; Vélez et al., 2007; Lebeer et al., 2008; Sánchez et al., 2011; Jensen et al., 2014; Hymes et al., 2016), however, some of these proteins can also be involved in other functions such as stress response, drug efflux, carbohydrate transport and metabolism and other probiotic actions (Lebeer et al., 2008; Lewis et al., 2012; Monteagudo-Mera et al., 2019). The specific functionality notably depends on the surrounding conditions which induce gene expression, with differences detected in both in vitro and in vivo scenarios. On the other hand, other genes coding for proteins involved in cell recognition and adhesion to intestinal mucosae such as the four genes coding for exopolysaccharide biosynthesis proteins were identified in the L. pentosus CF2-10 N genome. These were found to be identical to those detected in L. pentosus MP-10 isolated from Aloreña table olives (Abriouel et al., 2016). Besides their role in niche adaptation, promoting auto-aggregation and biofilm formation, these proteins were also attributed anti-inflammatory, antioxidant, antiviral and antiproliferative activity functions through their interaction with the immune system (Castro-Bravo et al., 2018; Nguyen et al., 2020; Riaz Rajoka et al., 2020).
To allow the adaptation to different lifestyles, L. pentosus CF2-10 N harbored in its genome several genes involved in stress response such as acids and bile. In this sense, Pérez Montoro et al. (2016) reported the strain’s excellent tolerance properties in vitro (acidic pH of 1.5, up to 4% of bile salts and 5 mM of nitrate), while in the present study we detected for the first time several genes coding for proteins involved in bile/acids resistance particularly including cell protection (dnaK and groL), modifications in cell membranes (genes coding for Na+/H+ antiporter NhaC, lepA, pyrD), general function (genes coding for GNAT family acetyltransferase), and key components of central metabolism (pgk, gpm, CysK, luxS, tuf, efp, tsf, FD24_GL002972, greA, greA2, fusA) as it was reported elsewhere for other bacteria (Wu et al., 2010; Liu et al., 2018; Bagon et al., 2021). Most of these proteins are considered moonlighting proteins involved in adhesion to the intestinal epithelium among other functions (Pagnini et al., 2018).
Concerning attractive and promising biotechnological features revealed by in silico analysis of the L. pentosus CF2-10 N genome, detected enzymes were involved in the degradation of toxic/complex substrates such as tannase, alpha-amylase, amylopullulanase, beta-galactosidase, aminopeptidase, lipase esterase, peptidases, alpha/beta hydrolase, phenolic acid decarboxylase, carboxylesterase, alpha-acetolactate decarboxylase and multicopper oxidase. These findings indicate the high adaptability of this strain to a broad range of environmental niches, food matrices and also the gastrointestinal tract, while being able to ferment lactose and starch. Findings further demonstrate the strain’s potential ability to synthesize and degrade a broad array of simple and complex carbohydrates, such as starch, pullulan, amylopectin, tannin, beta-galactosides, phenolic acids and other substrates. It is further noteworthy that L. pentosus CF2-10 N harbored genes coding for vitamin biosynthesis such as the vitamin B group (B1 or thiamine, B2 or riboflavin and B5), folate and vitamin K2 or menaquinone. In this regard, preliminary in vitro studies hinted towards a potential vitamin production ability of L. pentosus CF2-10 N. However, future studies are necessary and will be performed to investigate this potential in further detail.
Conclusion
The results obtained in the present study support the hypothesis that L. pentosus CF2-10 N is an excellent probiotic candidate of vegetable origin. Notably, besides fulfilling the main criteria for probiotic selection in vitro as shown by our previous studies, in silico genome analysis in this study revealed novel insights into its safety and functionality, greatly highlighting the microorganism’s ecological flexibility and adaptability to a broad range of environmental niches, food matrices and the gastrointestinal tract. The safety of L. pentosus CF2-10 N was further confirmed by the absence of virulence determinants and acquired antibiotic resistance genes, with the resistome mostly represented by efflux-pump resistance genes responsible for the intrinsic resistance exhibited by this strain. On the other hand, defense mechanisms of L. pentosus CF2-10 N consist of eight prophage regions as well as a CRISPR (clustered regularly interspaced short palindromic repeats)/cas (CRISPR-associated protein genes) system (CRISPR-I and CRISPR-II) as acquired immune system against mobile elements. The latter is notably represented by five CRISPR unquestionable arrays and 13 CRISPR associated proteins (six of them were exclusive of this strain). Furthermore, the functionality of this strain was supported by the presence of genes coding for proteins involved in adhesion, exopolysaccharide biosynthesis, tolerance to low pH and bile salts, immunomodulation as well as vitamin and enzyme production.
Taken together these results we suggest that L. pentosus CF2-10 N could be considered as potential and promising probiotic candidate able to colonize several niches and adapt to different lifestyles, while providing attractive probiotic features, which will be explored in vivo in future studies with the aim to be applied in vegetable fermentations (including olives) and/or other substrates.
Data availability statement
The datasets presented in this study can be found in online repositories. The names of the repository/repositories and accession number(s) can be found in the article/Supplementary material.
Author contributions
HA and NB conceived, designed the experiments, and drafted the paper. HA, JM, NC, and NB performed the experiments and analyzed the data. HA contributed reagents, materials, and analysis tools. All authors contributed to the article and approved the submitted version.
Acknowledgments
We acknowledge the Research Team (University of Jaen, EI_BIO1_2021).
Conflict of interest
The authors declare that the research was conducted in the absence of any commercial or financial relationships that could be construed as a potential conflict of interest.
Publisher’s note
All claims expressed in this article are solely those of the authors and do not necessarily represent those of their affiliated organizations, or those of the publisher, the editors and the reviewers. Any product that may be evaluated in this article, or claim that may be made by its manufacturer, is not guaranteed or endorsed by the publisher.
Supplementary material
The Supplementary material for this article can be found online at: https://www.frontiersin.org/articles/10.3389/fmicb.2022.989824/full#supplementary-material
Footnotes
References
Abriouel, H., Benomar, N., Cobo, A., Caballero, N., Fernández Fuentes, M. A., Pérez-Pulido, R., et al. (2012). Characterization of lactic acid bacteria from naturally-fermented Manzanilla Aloreña green table olives. Food Microbiol. 32, 308–316. doi: 10.1016/j.fm.2012.07.006
Abriouel, H., Benomar, N., Lucas, R., and Gálvez, A. (2011). Culture-independent study of the diversity of microbial populations in brines during fermentation of naturally fermented Aloreña green table olives. Int. J. Food Microbiol. 144, 487–496. doi: 10.1016/j.ijfoodmicro.2010.11.006
Abriouel, H., Pérez Montoro, B., Casado Muñoz, M. D. C., Lavilla Lerma, L., Hidalgo Pestaña, M., Caballero Gómez, N., et al. (2016). Complete genome sequence of a potential probiotic, lactobacillus pentosus MP-10, isolated from fermented Aloreña table olives. Genome Announc. 4, e00854–e00816. doi: 10.1128/genomeA.00854-16
Abriouel, H., Pérez Montoro, B., Casimiro-Soriguer, C. S., Pérez Pulido, A. J., Knapp, C. W., Caballero Gómez, N., et al. (2017). Insight into potential probiotic markers predicted in lactobacillus pentosus MP-10 genome sequence. Front. Microbiol. 8:891. doi: 10.3389/fmicb.2017.00891
Abriouel, H., Pérez Montoro, B., de la Fuente Ordoñez, J. J., Lavilla Lerma, L., Knapp, C. W., and Benomar, N. (2019). New insights into the role of plasmids from probiotic lactobacillus pentosus MP-10 in Aloreña table olive brine fermentation. Sci. Reports 9:10938. doi: 10.1038/s41598-019-47384-1
Alcock, B. P., Raphenya, A. R., Lau, T. T. Y., Tsang, K. K., Bouchard, M., Edalatmand, A., et al. (2020). CARD 2020: antibiotic resistome surveillance with the comprehensive antibiotic resistance database. Nucleic Acids Res. 48, D517–D525. doi: 10.1093/nar/gkz935
Allain, T., Chaouch, S., Thomas, M., Vallée, I., Buret, A. G., Langella, P., et al. (2018). Bile-salt-hydrolases from the probiotic strain lactobacillus johnsonii La1 mediate anti-giardial activity in vitro and in vivo. Front. Microbiol. 8:2707. doi: 10.3389/fmicb.2017.02707
Alonso García, A., Benomar, B., Lavilla Lerma, F., de la Fuente Ordoñez, J. J., Knapp, C. W., and Abriouel, H. (2023). Changes in resistome profile of potential probiotic Lactiplantibacillus pentosus in response to edible oil adaptation. Food Microbiol. 104148. doi: 10.1016/j.fm.2022.104148
Alonso García, E., de la Fuente Ordoñez, J. J., Lavilla Lerma, L., Estudillo-Martínez, M. D., Castillo-Gutiérrez, S., Benomar, N., et al. (2021). Transcriptomic profile and probiotic properties of Lactiplantibacillus pentosus pre-adapted to edible oils. Front. Microbiol. 12:747043. doi: 10.3389/fmicb.2021.747043
Amara, A. A., and Shibl, A. (2015). Role of probiotics in health improvement, infection control and disease treatment and management. Saudi Pharm. J. 23, 107–114. doi: 10.1016/j.jsps.2013.07.001
Anukam, K. C., Macklaim, J. M., Gloor, G. B., Reid, G., Boekhorst, J., Renckens, B., et al. (2013). Genome sequence of lactobacillus pentosus KCA1: vaginal isolate from a healthy premenopausal woman. PLoS One 8:e59239. doi: 10.1371/journal.pone.0059239
Arndt, D., Grant, J., Marcu, A., Sajed, T., Pon, A., Liang, Y., et al. (2016). PHASTER: a better, faster version of the PHAST phage search tool. Nucleic Acids Res. 44, W16–W21. doi: 10.1093/nar/gkw387
Bagon, B. B., Valeriano, V. D. V., Oh, J. K., Pajarillo, E. A. B., Lee, J. Y., and Kang, D. K. (2021). Exoproteome perspective on the bile stress response of lactobacillus johnsonii. Proteomes 9:10. doi: 10.3390/proteomes9010010
Benítez-Cabello, A., Calero-Delgado, B., Rodríguez-Gómez, F., Garrido-Fernández, A., Jiménez-Díaz, R., and Arroyo-López, F. N. (2019). Biodiversity and multifunctional features of lactic acid bacteria isolated from table olive biofilms. Front. Microbiol. 10:836. doi: 10.3389/fmicb.2019.00836
Besemer, J., Lomsadze, A., and Borodovsky, M. (2001). GeneMarkS: a self-training method for prediction of gene starts in microbial genomes. Implications for finding sequence motifs in regulatory regions. Nucleic Acids Res. 29, 2607–2618. doi: 10.1093/nar/29.12.2607
Biswas, A., Staals, R. H. J., Morales, S. E., Fineran, P. C., and Brown, C. M. (2016). CRISPRDetect: a flexible algorithm to define CRISPR arrays. BMC Genom. 17:356. doi: 10.1186/s12864-016-2627-0
Bortolaia, V., Kaas, R. S., Ruppe, E., Roberts, M. C., Schwarz, S., Cattoir, V., et al. (2020). ResFinder 4.0 for predictions of phenotypes from genotypes. J. Antimicrob. Chemother. 75, 3491–3500. doi: 10.1093/jac/dkaa345
Caggianiello, G., Kleerebezem, M., and Spano, G. (2016). Exopolysaccharides produced by lactic acid bacteria: from health-promoting benefits to stress tolerance mechanisms. Appl. Microbiol. Biotechnol. 100, 3877–3886. doi: 10.1007/s00253-016-7471-2
Calero-Delgado, B., Pérez-Pulido, A. J., Benítez-Cabello, A., Martín-Platero, A. M., Casimiro- Soriguer, C. S., Martínez-Bueno, M., et al. (2019). Multiple genome sequences of lactobacillus pentosus strains isolated from biofilms on the skin of fermented green table olives. Microbiol. Resour. Announc. 8, e01546–e01518. doi: 10.1128/MRA.01546-18
Carrasco, J. A., Lucena-Padrós, H., Brenes, M., and Ruiz-Barba, J. L. (2018). Expression of genes involved in metabolism of phenolic compounds by lactobacillus pentosus and its relevance for table-olive fermentations. Food Microbiol. 76, 382–389. doi: 10.1016/j.fm.2018.06.020
Carver, T. J., Rutherford, K. M., Berriman, M., Rajandream, M. A., Barrell, B. G., and Parkhill, J. (2005). ACT: the Artemis comparison tool. Bioinform. 21, 3422–3423. doi: 10.1093/bioinformatics/bti553
Carver, T. J., Thomson, N., Bleasby, A., Berriman, M., and Parkhill, J. (2009). DNAPlotter: circular and linear interactive genome visualization. Bioinform. 25, 119–120. doi: 10.1093/bioinformatics/btn578
Casado Muñoz, M. C., Benomar, N., Ennahar, S., Horvatovich, P., Lavilla Lerma, L., Knapp, C. W., et al. (2016). Comparative proteomic analysis of a potentially probiotic lactobacillus pentosus MP-10 for the identification of key proteins involved in antibiotic resistance and biocide tolerance. Int. J. Food Microbiol. 222, 8–15. doi: 10.1016/j.ijfoodmicro.2016.01.012
Casado Muñoz, M. C., Benomar, N., Lerma, L. L., Gálvez, A., and Abriouel, H. (2014). Antibiotic resistance of lactobacillus pentosus and Leuconostoc pseudomesenteroides isolated from naturally-fermented Aloreña table olives throughout fermentation process. Int. J. Food Microbiol. 172, 110–118. doi: 10.1016/j.ijfoodmicro.2013.11.025
Castro-Bravo, N., Wells, J. M., Margolles, A., and Ruas-Madiedo, P. (2018). Interactions of surface exopolysaccharides from Bifidobacterium and lactobacillus within the intestinal environment. Front. Microbiol. 9:2426. doi: 10.3389/fmicb.2018.02426
Chin, C. S., Alexander, D. H., Marks, P., Klammer, A. A., Drake, J., Heiner, C., et al. (2013). Nonhybrid, finished microbial genome assemblies from long-read SMRT sequencing data. Nat. Methods 10, 563–569. doi: 10.1038/nmeth.2474
Conesa, A., Götz, S., García-Gómez, J. M., Terol, J., Talón, M., and Robles, M. (2005). Blast2GO: a universal tool for annotation, visualization and analysis in functional genomics research. Bioinform. 21, 3674–3676. doi: 10.1093/bioinformatics/bti610
Darling, A. C. E., Mau, B., Blattner, F. R., and Perna, N. T. (2004). Mauve: multiple alignment of conserved genomic sequence with rearrangements. Genome Res. 14, 1394–1403. doi: 10.1101/gr.2289704
Duar, R. M., Lin, X. B., Zheng, J. Z., Martino, M. E., Grenier, T., Pérez-Muñoz, M. E., et al. (2017). Lifestyles in transition: evolution and natural history of the genus lactobacillus. FEMS Microbiol. Rev. 41, S27–S48. doi: 10.1093/femsre/fux030
Granato, D., Bergonzelli, G. E., Pridmore, R. D., Marvin, L., Rouvet, M., and Corthésy-Theulaz, I. E. (2004). Cell surface-associated elongation factor Tu mediates the attachment of lactobacillus johnsonii NCC533 (La1) to human intestinal cells and mucins. Inf. Immun. 72, 2160–2169. doi: 10.1128/IAI.72.4.2160-2169.2004
Guantario, B., Zinno, P., Schifano, E., Roselli, M., Perozzi, G., Palleschi, C., et al. (2018). In vitro and in vivo selection of potentially probiotic lactobacilli from Nocellara del Belice table olives. Front. Microbiol. 9:595. doi: 10.3389/fmicb.2018.00595
Hill, C., Guarner, F., Reid, G., Gibson, G. R., Merenstein, D. J., Pot, B., et al. (2014). The international scientific Association for Probiotics and Prebiotics consensus statement on the scope and appropriate use of the term probiotic. Nat. Rev. Gastroenterol. Hepatol. 11, 506–514. doi: 10.1038/nrgastro.2014.66
Hymes, J. P., Johnson, B. R., Barrangou, R., and Klaenhammer, T. R. (2016). Functional analysis of an S-layer-associated fibronectin-binding protein in Lactobacillus acidophilus NCFM. Appl. Environ. Microbiol. 82, 2676–2685. doi: 10.1128/AEM.00024-16
Jensen, H., Roos, S., Jonsson, H., Rud, I., Grimmer, S., van Pijkeren, J. P., et al. (2014). Role of lactobacillus reuteri cell and mucus-binding protein a (CmbA) in adhesion to intestinal epithelial cells and mucus in vitro. Microbiol. 160, 671–681. doi: 10.1099/mic.0.073551-0
Lagesen, K., Hallin, P., Rødland, E. A., Stærfeldt, H. H., and Rognes, T. (2007). RNAmmer: consistent and rapid annotation of ribosomal RNA genes. Nucleic Acids Res. 35, 3100–3108. doi: 10.1093/nar/gkm160
Lavermicocca, P., Valerio, F., Lonigro, S. L., de Angelis, M., Morelli, L., and Callegari, M. L. (2005). Study of adhesion and survival of lactobacilli and bifidobacteria on table olives with the aim of formulating a new probiotic food. Appl. Environ. Microbiol. 71, 4233–4240. doi: 10.1128/AEM.71.8.4233-4240.2005
Lebeer, S., Vanderleyden, J., and De Keersmaecker, S. C. J. (2008). Genes and molecules of lactobacilli supporting probiotic action. Microbiol. Mol. Biol. Rev. 72:728. doi: 10.1128/MMBR.00017-08
Lewis, V. G., Ween, M. P., and McDevitt, C. A. (2012). The role of ATP-binding cassette transporters in bacterial pathogenicity. Protoplasma 249, 919–942. doi: 10.1007/s00709-011-0360-8
Liu, L., Wu, R., Zhang, J., and Li, P. (2018). Overexpression of luxS promotes stress resistance and biofilm formation of lactobacillus paraplantarum L-ZS9 by regulating the expression of multiple genes. Front. Microbiol. 9:2628. doi: 10.3389/fmicb.2018.02628
Liu, B., Zheng, D., Jin, Q., Chen, L., and Yang, J. (2019). VFDB 2019: a comparative pathogenomic platform with an interactive web interface. Nucleic Acids Res. 47, D687–D692. doi: 10.1093/nar/gky1080
Lowe, T. M., and Eddy, S. R. (1997). tRNAscan-SE: a program for improved detection of transfer RNA genes in genomic sequence. Nucl. Acids Res. 25, 955–964.
Maldonado-Barragán, A., Caballero-Guerrero, B., Lucena-Padrós, H., and Ruiz-Barba, J. L. (2011). Genome sequence of lactobacillus pentosus IG1, a strain isolated from Spanish-style green olive fermentations. J. Bacteriol. 193:5605. doi: 10.1128/JB.05736-11
Monteagudo-Mera, A., Rastall, R. A., Gibson, G. R., Charalampopoulos, D., and Chatzifragkou, A. (2019). Adhesion mechanisms mediated by probiotics and prebiotics and their potential impact on human health. Appl. Microbiol. Biotechnol. 103, 6463–6472. doi: 10.1007/s00253-019-09978-7
Myers, E. W., Sutton, G. G., Delcher, A. L., Dew, I. M., Fasulo, D. P., Flanigan, M. J., et al. (2000). A whole-genome assembly of drosophila. Sci 287, 2196–2204.
Nam, K. H., Kurinov, I., and Ke, A. (2011). Crystal structure of clustered regularly interspaced short palindromic repeats (CRISPR)-associated Csn2 protein revealed Ca2+-dependent double-stranded DNA binding activity. J. Biol. Chem. 286, 30759–30768. doi: 10.1074/jbc.M111.256263
Nayak, S. K. (2010). Probiotics and immunity: a fish perspective. Fish Shellfish Immunol. 29, 2–14. doi: 10.1016/j.fsi.2010.02.017
Nguyen, P. T., Nguyen, T. T., Bui, D. C., Hong, P. T., Hoang, Q. K., and Nguyen, H. T. (2020). Exopolysaccharide production by lactic acid bacteria: the manipulation of environmental stresses for industrial applications. AIMS Microbiol. 6, 451–469. doi: 10.3934/microbiol.2020027
Oguntoyinbo, F. A., and Narbad, A. (2015). Multifunctional properties of lactobacillus plantarum strains isolated from fermented cereal foods. J. Funct. Foods 17, 621–631. doi: 10.1016/j.jff.2015.06.022
Pagnini, C., Corleto, V. D., Martorelli, M., Lanini, C., D’Ambra, G., Di Giulio, E., et al. (2018). Mucosal adhesion and anti-inflammatory effects of lactobacillus rhamnosus GG in the human colonic mucosa: a proof-of-concept study. World J. Gastroenterol. 24, 4652–4662. doi: 10.3748/wjg.v24.i41.4652
Pei, Z., Sadiq, F. A., Han, X., Zhao, J., Zhang, H., Ross, R. P., et al. (2021). Comprehensive scanning of prophages in lactobacillus: distribution, diversity, antibiotic resistance genes, and linkages with CRISPR-Cas systems. mSystems 6:e0121120. doi: 10.1128/mSystems.01211-20
Pérez Montoro, B., Benomar, N., Caballero Gómez, N., Ennahar, S., Horvatovich, P., Knapp, C. W., et al. (2018b). Proteomic analysis of lactobacillus pentosus for the identification of potential markers of adhesion and other probiotic features. Food Res. Int. 111, 58–66. doi: 10.1016/j.foodres.2018.04.072
Pérez Montoro, B., Benomar, N., Caballero Gómez, N., Ennahar, S., Horvatovich, P., Knapp, C. W., et al. (2018a). Proteomic analysis of lactobacillus pentosus for the identification of potential markers involved in acid resistance and their influence on other probiotic features. Food Microbiol. 72, 31–38. doi: 10.1016/j.fm.2017.11.006
Pérez Montoro, B., Benomar, N., Lavilla Lerma, L., Castillo Gutiérrez, S., Gálvez, A., and Abriouel, H. (2016). Fermented Aloreña table olives as a source of potential probiotic lactobacillus pentosus strains. Front. Microbiol. 7:1583. doi: 10.3389/fmicb.2016.01583
Pérez-Díaz, I. M., Johanningsmeier, S. D., Anekella, K., Pagán-Medina, C. G., Méndez-Sandoval, L., Arellano, C., et al. (2021). Genotypic and phenotypic diversity among lactobacillus plantarum and lactobacillus pentosus isolated from industrial scale cucumber fermentations. Food Microbiol. 94:103652. doi: 10.1016/j.fm.2020.103652
Perpetuini, G., Prete, R., Garcia-Gonzalez, N., Khairul Alam, M., and Corsetti, A. (2020). Table olives more than a fermented food. Foods 9:178. doi: 10.3390/foods9020178
Puvanasundram, P., Chong, C. M., Sabri, S., Yusoff, M. S., and Karim, M. (2021). Multi-strain probiotics: functions, effectiveness and formulations for aquaculture applications. Aquac. Rep. 21:100905. doi: 10.1016/j.aqrep.2021.100905
Riaz Rajoka, M. S., Wu, Y., Mehwish, H. M., Bansal, M., and Zhao, L. (2020). Lactobacillus exopolysaccharides: new perspectives on engineering strategies, physiochemical functions, and immunomodulatory effects on host health, trends food Sci. Technol. 103, 36–48. doi: 10.1016/j.tifs.2020.06.003
Rodríguez-Gómez, F., Romero-Gil, V., Arroyo-López, F. N., Roldán-Reyes, J. C., Torres-Gallardo, R., Bautista-Gallego, J., et al. (2017). Assessing the challenges in the application of potential probiotic lactic acid bacteria in the large-scale fermentation of Spanish-style table olives. Front. Microbiol. 8:915. doi: 10.3389/fmicb.2017.00915
Rodríguez-Gómez, F., Romero-Gil, V., Bautista-Gallego, J., García-García, P., Garrido-Fernández, A., and Arroyo-López, F. N. (2014). Production of potential probiotic Spanish-style green table olives at pilot plant scale using multifunctional starters. Food Microbiol. 44, 278–287. doi: 10.1016/j.fm.2014.03.023
Sánchez, B., González-Tejedo, C., Ruas-Madiedo, P., Urdaci, M. C., and Margolles, A. (2011). Lactobacillus plantarum extracellular chitin-binding protein and its role in the interaction between chitin, Caco-2 cells, and mucin. Appl. Environ. Microbiol. 77, 1123–1126. doi: 10.1128/AEM.02080-10
Saxami, G., Karapetsas, A., Chondrou, P., Vasiliadis, S., Lamprianidou, E., Kotsianidis, I., et al. (2017). Potentially probiotic lactobacillus strains with anti-proliferative activity induce cytokine/chemokine production and neutrophil recruitment in mice. Benef. Microbes 8, 615–623. doi: 10.3920/BM2016.0202
Stamatakis, A. (2014). RAxML version 8: a tool for phylogenetic analysis and post-analysis of large phylogenies. Bioinform. 30, 1312–1313. doi: 10.1093/bioinformatics/btu033
Sun, Z., Harris, H. M., McCann, A., Guo, C., Argimon, S., Zhang, W., et al. (2015). Expanding the biotechnology potential of lactobacilli through comparative genomics of 213 strains and associated genera. Nat. Commun. 6:8322. doi: 10.1038/ncomms9322
Tofalo, R., Perpetuini, G., Schirone, M., Ciarrocchi, A., Fasoli, G., Suzzi, G., et al. (2014). Lactobacillus pentosus dominates spontaneous fermentation of Italian table olives. LWT-Food Sci. Technol. 57, 710–717. doi: 10.1016/J.LWT.2014.01.035
Vaccalluzzo, A., Pino, A., Russo, N., De Angelis, M., Caggia, C., and Randazzo, C. L. (2020). FoodOmics as a new frontier to reveal microbial community and metabolic processes occurring on table olives fermentation. Food Microbiol. 92:103606. doi: 10.1016/j.fm.2020.103606
van de Wijgert, J. H. H. M., and Verwijs, M. C. (2019). Lactobacilli-containing vaginal probiotics to cure or prevent bacterial or fungal vaginal dysbiosis: a systematic review and recommendations for future trial designs. BJOG 127, 287–299. doi: 10.1111/1471-0528.15870
Vélez, M. P., De Keersmaecker, S. C., and Vanderleyden, J. (2007). Adherence factors of lactobacillus in the human gastrointestinal tract. FEMS Microbiol. Lett. 276, 140–148. doi: 10.1111/j.1574-6968.2007.00908.x
Wu, R., Sun, Z., Wu, J., Meng, H., and Zhang, H. (2010). Effect of bile salts stress on protein synthesis of lactobacillus casei Zhang revealed by 2-dimensional gel electrophoresis. J. Dairy Sci. 93, 3858–3868. doi: 10.3168/jds.2009-2967
Xue, C., and Sashital, D. G. (2019). Mechanisms of type I-E and I-F CRISPR-Cas Systems in Enterobacteriaceae. EcoSal Plus 8:10.1128/ecosalplus.ESP-0008-2018. doi: 10.1128/ecosalplus.ESP-0008-2018
Yan, S., Tian, Z., Li, M., Li, B., and Cui, W. (2019). Effects of probiotic supplementation on the regulation of blood lipid levels in overweight or obese subjects: a meta-analysis. Food Funct. 10, 1747–1759. doi: 10.1039/c8fo02163e
Yoha, K. S., Nida, S., Dutta, S., Moses, J. A., and Anandharamakrishnan, C. (2022). Targeted delivery of probiotics: perspectives on research and commercialization. Probiotics Antimicro. Prot. 14, 15–48. doi: 10.1007/s12602-021-09791-7
Zankari, E., Hasman, H., Cosentino, S., Vestergaard, M., Rasmussen, S., Lund, O., et al. (2012). Identification of acquired antimicrobial resistance genes. J. Antimicrob. Chemother. 67, 2640–2644. doi: 10.1093/jac/dks261
Zhang, Z., Schwartz, S., Wagner, L., and Miller, W. (2000). A greedy algorithm for aligning DNA sequences. J. Comput. Biol. 7, 203–214.
Zheng, J., Wittouck, S., Salvetti, E., Franz, C. M. A. P., Harris, H. M. B., Mattarelli, P., et al. (2020). A taxonomic note on the genus lactobacillus: description of 23 novel genera, emended description of the genus lactobacillus Beijerinck 1901, and union of Lactobacillaceae and Leuconostocaceae. Int. J. Syst. Evol. Microbiol. 70, 2782–2858. doi: 10.1099/ijsem.0.004107
Zhou, Y., Liang, Y., Lynch, K., Dennis, J. J., and Wishart, D. S. (2011). PHAST: a fast phage search tool. Nucl. Acids Res. 39, W347–W352. doi: 10.1093/nar/gkr485
Keywords: Aloreña table olives, Lactiplantibacillus pentosus, probiotics, in silico analysis, safety, functional properties
Citation: Abriouel H, Manetsberger J, Caballero Gómez N and Benomar N (2022) In silico genomic analysis of the potential probiotic Lactiplantibacillus pentosus CF2-10N reveals promising beneficial effects with health promoting properties. Front. Microbiol. 13:989824. doi: 10.3389/fmicb.2022.989824
Edited by:
Joaquin Bautista-Gallego, University of Extremadura, SpainReviewed by:
Gloria Díaz-Ruiz, National Autonomous University of Mexico, MexicoZhihong Sun, Inner Mongolia Agricultural University, China
Alex Galanis, Democritus University of Thrace, Greece
Copyright © 2022 Abriouel, Manetsberger, Caballero Gómez and Benomar. This is an open-access article distributed under the terms of the Creative Commons Attribution License (CC BY). The use, distribution or reproduction in other forums is permitted, provided the original author(s) and the copyright owner(s) are credited and that the original publication in this journal is cited, in accordance with accepted academic practice. No use, distribution or reproduction is permitted which does not comply with these terms.
*Correspondence: Hikmate Abriouel, hikmate@ujaen.es