- 1School of Rehabilitation Medicine, Gannan Medical University, Ganzhou, China
- 2School of Basic Medicine, Gannan Medical University, Ganzhou, China
- 3Key Laboratory of Prevention and Treatment of Cardiovascular and Cerebrovascular Diseases of Ministry of Education, Gannan Medical University, Ganzhou, China
Cardiovascular diseases, mainly characterized by atherosclerosis (AS), and depression have a high comorbidity rate. However, previous studies have been conducted under a single disease, and there is a lack of studies in comorbid states to explore the commonalities in the pathogenesis of both diseases. Modern high-throughput technologies have made it clear that the gut microbiome can affect the development of the host’s own disorders and have shown that their metabolites are crucial to the pathophysiology of AS and depression. The aim of this review is to summarize the current important findings on the role of gut microbiome metabolites such as pathogen-associated molecular patterns, bile acids, tryptophan metabolites, short-chain fatty acids, and trimethylamine N -oxide in depression and AS disease, with the aim of identifying potential biological targets for the early diagnosis and treatment of AS co-depression disorders.
Introduction
Cardiovascular disease, mainly characterized by atherosclerosis (AS), is the most common cause of death worldwide and is expected to be the top four causes of death worldwide by 2030 (WHO, 2008). There is growing evidence that depression, which is included among the top five causes of disability worldwide (Collaborators, G.D.a.I.I.a.P, 2017), is an independent risk factor for the occurrence of cardiac events (Zellweger et al., 2004; Hare et al., 2014; Vaccarino et al., 2020). Studies have shown that depression is associated with a higher risk ratio for cardiovascular mortality than hypercholesterolemia and obesity, and are intermediate between the “Big Five” classical cardiovascular risk factors (Ladwig et al., 2017) and is a common complication in patients with atherosclerosis cardiovascular disease (ASCVD; Levine et al., 2021). Individuals with depression are at substantially increased risk of cardiovascular disease and death (O'Connor, 2018; Levine et al., 2021), and in particular, are strongly associated with one of the most common cardiovascular diseases–ASCVD (Jee et al., 2019; Sun et al., 2019). In a multiracial study in the United States, depression was independently associated with atherosclerotic cardiovascular disease risk in all age groups (Dibato et al., 2021). In another large retrospective cohort analysis, it was found that patients with premature ASCVD had poor physical and mental health, with female patients more likely to report clinical depression and therefore require mental health interventions (Jain et al., 2022). Given that the two are so closely related clinically, we refer to this state as atherosclerosis co-depression disease.
Most of the current research is aimed at a single disease, and now is the dilemma facing the patient usually with a variety of diseases, because the interaction between the disease is complex, and the use of multiple drugs may lead to poor efficacy, in addition to the study of comorbidity is less, so we try to study the pathogenesis of common direction from both, identify common key targets for intervention. It is now believed that depression and atherosclerosis occur by similar mechanisms, including inflammation (Chrysohoou et al., 2018), hypothalamic–pituitary–adrenal axis dysregulation (Gu et al., 2012), endothelial dysfunction (van Dooren et al., 2016; Münzel and Daiber, 2020), and other major causes, while the development of modern high-throughput technologies has provided technical support for the study of the gut microbiome, and an increasing number of studies have revealed that gut microbiome is key factors mediating the development of the host’s diseases, including depression and AS (Jonsson and Bäckhed, 2017; Durack and Lynch, 2019). The gut microbiome can interact with the host by influencing metabolites, which are intermediate or final products of microbial metabolism, either directly from the bacteria themselves or the diet or the transformation of host-derived substrates. In this review, we will focus on the critical role played by gut microbiome-derived metabolites in the pathogenesis of depression and AS, which may provide valuable information for future diagnostic and therapeutic options for AS co-depression disorders.
The change in the gut microbiome is closely related to the occurrence of depression and AS
Early studies first noted that microorganisms located in the gut have some connection to the host’s central nervous system, which is referred to as the brain-gut axis (Rieder et al., 2017), which expanded the horizon for uncovering the potential pathogenesis of psychiatric disorders. Studies have shown that germ-free mice exhibit an overall defect in microglia, i.e., altered cell ratios and an immature phenotype that leads to impaired innate immune responses (Erny et al., 2015), as well as deficits in social cognition and social perception (Sherwin et al., 2019), and Gareau tested germ-free (GF) mice cognitively using a new object recognition experiment and a T-maze experiment, which showed low rates of exploration and spontaneous exploration, without show signs of non-spatial or working memory (Gareau et al., 2011), and these results fully confirm the findings of Cryan et al. that gut flora can modulate the developmental and functional status of the brain (Cryan and Dinan, 2012), and is one of the important influencing factors in the occurrence of depression. In addition to exploring the relationship between gut microbiome and CNS disorders such as depression using GF animals, the relationship between gut microbiome and AS was also confirmed through it. As found by Stepankova: compared to ApoE−/− mice raised under conventional conditions, GF ApoE−/− mice consuming the same low-cholesterol standard diet instead developed atherosclerotic plaques, suggesting that gut microbiome can protect mice from atherosclerosis (Stepankova et al., 2010). Interestingly, Kiouptsi in another study confirmed by GF mice that gut microbiome lowered plasma cholesterol levels in Ldlr−/− mice fed a normal diet, but not in Ldlr−/− mice fed a high-fat diet. The reason for this was that the high-fat diet led to cholesterol spillage, which masked the bacterial effect. In addition, he found that gut microbiome increase low-grade inflammation in the vessel wall and can promote the development of atherosclerosis (Kiouptsi et al., 2019). This does not contradict the results of the previous ApoE−/− model article, as the gut microbiome consist of pathogenic and protective bacteria and which specific members of the microbiota are not well studied in terms of promoting cholesterol excretion or plaque formation, which is an interesting direction of research.
Relationship between the gut microbiome and depression
Several subsequent studies have found that the gut microbiome is closely associated with the development of depression, one of the common psychiatric disorders (Simpson et al., 2021). Cheng et al. used microbiome-associated gene set enrichment analysis to identify gut microbiome associated with psychiatric disorders and showed that major depressive disorder (MDD) was associated with genus Desulfovibrio (p = 0.003), order Clostridiales (p = 0.004), family Lachnospiraceae (p = 0.007) and genus Bacteroides (p = 0.007; Cheng et al., 2020). By analyzing stool samples from 46 depressed patients and 30 healthy controls, Jiang et al. showed that MDD patients had increased levels of Enterobacteriaceae and Alistipes and decreased levels of Faecalibacterium, where Faecalibacterium was negatively correlated with the severity of depressive symptoms (Jiang et al., 2015). On the other hand, Lai et al. used a more advanced shotgun metagenomic sequencing on stool specimens from 26 MDD patients and 29 healthy controls and showed a significant decrease in the abundance of Bacteroidetes and a significant increase in the abundance of Actinobacteria in MDD patients, where it is noteworthy that Bifidobacterium levels were increased in MDD patients (Lai et al., 2021). While Bifidobacterium is a commonly used probiotic, this certainly suggests that we should try to control for extraneous factors affecting heterogeneity (e.g., a sample size of participants, dietary habits, clinical medication, and their condition, sequencing methods, statistical methods, etc.) in conducting microbiome studies. In another study, by testing the feces of depressed patients, a decrease in the abundance and diversity of gut microbiome was found, followed by gavage of fecal flora from depressed patients to microbiota-deficient rats, which revealed that rats subjected to flora transplantation showed behaviors characteristic of depression as well as physiological features of altered tryptophan metabolism (Kelly et al., 2016). More cases are shown in Table 1. All of the above studies confirm that alterations in the gut microbiome are potentially important factors in the pathogenesis of depression.
Relationship between the gut microbiome and AS
The interaction between the gut microbiome and the central system has provided ideas for research in other disease areas and has attracted many researchers to work to uncover the link between the gut microbiome and atherosclerosis. Several studies have shown significant alterations in the structure and composition of the gut microbiome in patients with AS-related diseases. A study conducted in Sweden involving 12 patients and 13 controls, using intestinal macrogenomics, confirmed that the genus Collinsella was enriched in patients with atherosclerosis, while Roseburia and Eubacterium were enriched in healthy controls (Karlsson et al., 2012). In another large clinical study, Jie performed a genome-wide association study of feces from 218 patients with atherosclerotic cardiovascular disease and 187 healthy individuals and showed that Enterobacteriaceae and Streptococcus spp. were enriched in the feces of patients with atherosclerotic cardiovascular disease (Jie et al., 2017). In addition, a multi-omics analysis based on 161 patients with Coronary artery disease (CAD) and 40 healthy individuals (sequence of the V3-V4 region of the 16S rRNA gene and metabolomics) showed that the composition of both gut microbiome and metabolites changed significantly with the severity of CAD. The abundance of bacterial co-abundance group17 (e.g., several Gram-negative bacteria such as Veillonella, Haemophilus, and Klebsiella) increased with the increasing severity of CAD. Another study confirmed that the metabolic modules of taurine and hypotaurine were negatively correlated with CAD severity, which could suggest that certain bacteria may affect atherosclerosis by modulating host metabolic pathways (e.g., taurine, sphingolipids, and ceramides) as well as benzene metabolism (Liu et al., 2019). For example, Roseburia intestinalis, which stands out for its potential role in the treatment of numerous human diseases, including AS, through the production of SCFAs, has gained recognition (Nie et al., 2021). As Kasahara found in his experiments, the abundance of Roseburia intestinalis in genetically diverse mouse populations was negatively correlated with the development of atherosclerotic lesions, for which the atheroprotective effect was mediated, at least in part, by the production of butyrate (Kasahara et al., 2018). Studies on the role of the gut microbiome in regulating cholesterol metabolism, a risk factor closely associated with AS, have also received wide attention. The results of Le Roy’s experiments showed that the gut microbiome strongly regulates plasma cholesterol levels, hepatic cholesterol synthesis, and enterohepatic circulation, and screened bacterial species or taxa involved in regulating cholesterol homeostasis as Betaproteobacteria, Alistipes, Bacteroides, and Barnesiella (Le Roy et al., 2019). Interestingly, the mechanism behind the cholesterol-lowering properties of Pu-erh tea lies in the fact that the Theabrownin in Pu-erh tea can act by inhibiting microbes associated with bile-salt hydrolase activity (Huang et al., 2019). In another animal experiment, the use of peptides reduced plasma total cholesterol levels and atherosclerotic plaque formation in Western diet-fed LDLr−/− mice, and this therapeutic effect was eliminated when the gut microbiome was depleted by antibiotics (Chen et al., 2020a). More details can be found in the review published by Vourakis (Vourakis et al., 2021), which focuses on the current knowledge about the potential mechanisms by which microbial metabolites regulate cholesterol homeostasis, providing therapeutic strategies to reduce the risk of AS-related diseases. In addition, more cases can be found in Table 2. These results also confirm that certain alterations in the gut microbiome are potentially important factors driving the development of AS.
Study of the gut microbiome in depression co-cardiovascular disease
Recent articles have systematically elaborated on the common underlying pathogenesis of depression and AS-related diseases, including gut microbes (Wu et al., 2021). Similarly, the key role of gut microbes has also been demonstrated in several studies of depression in combination with cardiovascular disease. Kemp used an exposure to early life stress model to investigate the relationship between altered gut microbiome and depression and cardiovascular disease, showing that the model reduced microbial alpha diversity and altered microbial composition (Kemp et al., 2021). A recent study found that dysregulation of gut microbiome composition contributed to the development of depression-like behavior induced by chronic myocardial infarction, suggesting that exogenous modulation of gut microbiome composition may be a potentially important strategy for treating depression-like behavior caused by adverse cardiac events (Zhang et al., 2022). Our previous study similarly showed that mainly Desulfovibrio and Akkermansia were altered within the gut microbiome of the AS co-depression mouse model compared to the Control group of mice and confirmed that some lipid metabolites in the brain are strongly associated with some bacteria (Hu et al., 2022). In addition to testing whether gut microbiome are altered in the context of AS co-depression disease, sun et al. used Bifidobacterium lactis Probio-M8 adjuvant therapy improved the clinical efficacy of coronary artery disease treatment as well as alleviated depression and anxiety in patients through targeted modulation of Gut-Heart/-Brain Axes (Sun et al., 2022) and the systematic description of the neuro- and cardioprotective effects of probiotics in clinical trials by Ciernikova in a recently published review (Ciernikova et al., 2021) further confirm the scientific validity of this hypothesis.
These findings confirm that the gut microbiome plays a key regulatory role in the pathological development of depression and AS disease (Figure 1), both in pure disease and in comorbid states, and can provide new insights into the potential pathogenesis of AS co-depression and its therapeutic targets through regulation of gut microbiome.
The available literature suggests that the interactions between the gut flora and the host are complex and not fully elucidated, but mainly include neural, the hypothalamic–pituitary–adrenal axis, immune and metabolic pathways (Grenham et al., 2011; Fung et al., 2017; Cryan et al., 2019). Next, we mainly elaborate on the important research results of several common and very important gut microbiome metabolites in the pathogenesis of AS and depression (Figure 2).
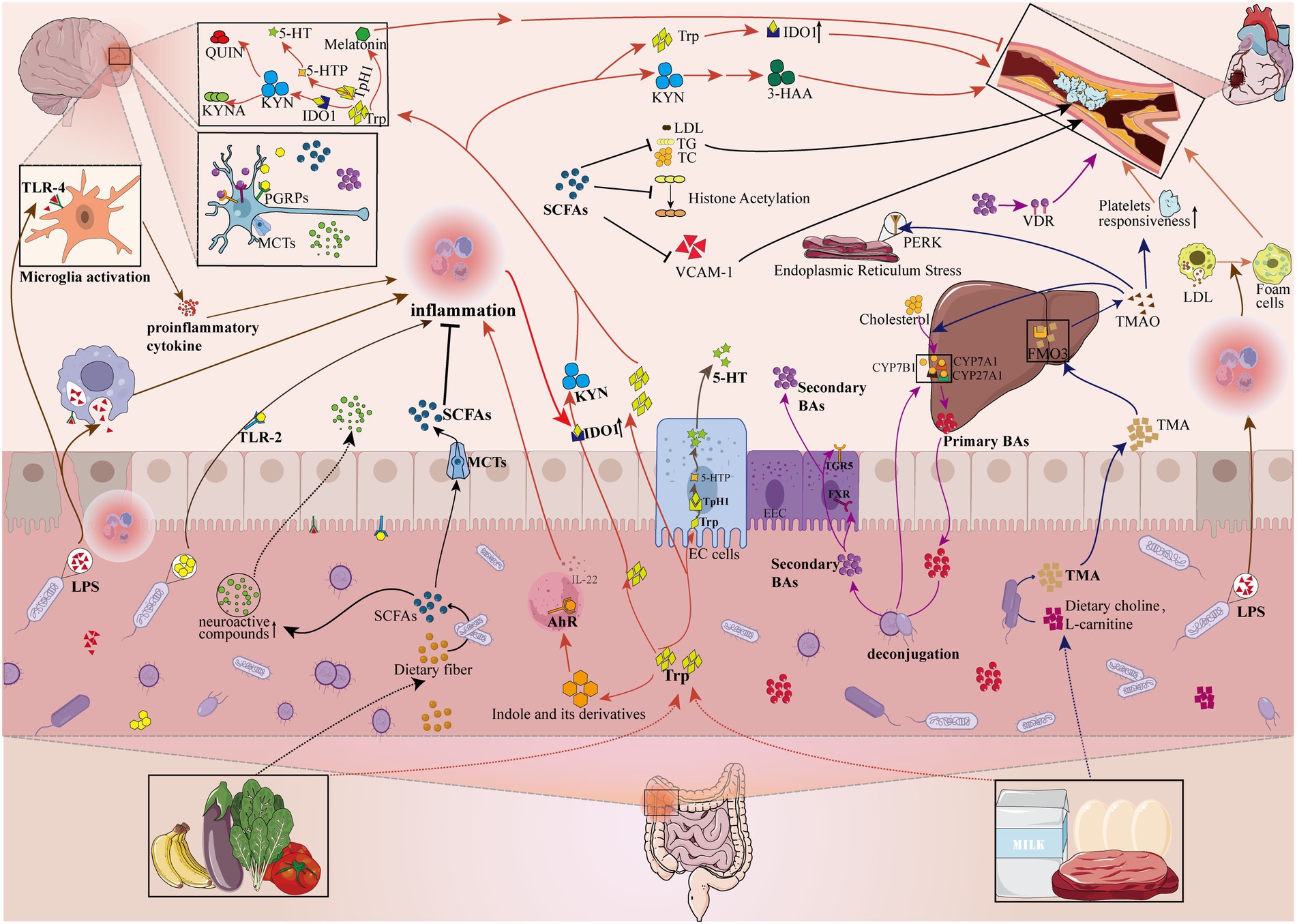
Figure 2. Gut microbiome disorders contribute to the pathologic development of depression and atherosclerosis by influencing metabolites. The gut microbiome can affect the pathological development of depression and atherosclerosis by participating in or mediating the production of metabolites (e.g., Pathogen-associated molecular patterns, Bile acids, Tryptophan metabolites, Short-chain fatty acids, Trimethylamine N-oxide). LPS, lipopolysaccharide; PGN, peptidoglycan; TLR-4, Toll-like receptors 4; TLR-2, Toll-like receptors 2; PGRPs, peptidoglycan recognition proteins; SCFAs, short-chain fatty acids; MCTs, monocarboxylic acid transport proteins; LDL, low-density lipoprotein cholesterol; TG, triglyceride; TC, total cholesterol; VCAM-1, vascular cell adhesion molecule-1; Trp, tryptophan; 5-HT, 5-hydroxytryptamine; KYN, kynurenine; AhR, aromatic hydrocarbon receptor; ECs, enterochromaffin cells; IDO1, indoleamine-2,3-dioxygenase 1; Tph1, tryptophan hydroxylase 1; 5-HTP, 5-hydroxytryptophan; KYNA, kynurenic acid; QUIN, quinolinic acid; 3-HAA, 3-hydroxyanthranilic acid; EEC, enteroendocrine cell; FXR, farnesoid X receptor; TGR5, Takeda G protein-coupled receptor 5; CYP7A1, cholesterol 7a-hydroxylase; CYP27A1, sterol-27-hydroxylase; CYP7B1, oxysterol 7α-hydroxylase; VDR, vitamin D receptor; TMA, trimethylamine; FMO3, flavin-containing monooxygenase isoform 3; TMAO, trimethylamine N-oxide; PERK, RNA-dependent protein kinase (PKR)-like ER kinase;
Pathogen-associated molecular patterns
Pathogen-associated molecular patterns (PAMP) mainly refer to certain highly conserved molecular structures (e.g., lipopolysaccharide (LPS) and peptidoglycan (PGN)) on the surface of pathogenic microorganisms that induce host immune activity by binding to pattern recognition receptors (PRR) (Fitzgerald and Kagan, 2020). External causes such as a high-fat diet (Paone and Cani, 2020) and aging (Tran and Greenwood-Van Meerveld, 2013) can affect intestinal permeability directly or indirectly (causing gut microbiome disorders) and are important influences that contribute to PAMP leakage. Among them, LPS can bind to TLR4 in enterocytes to trigger the inflammatory process, further downregulating the level of tight junction proteins, resulting in more LPS translocation into the systemic circulation (Violi et al., 2022). LPS and PGN that enter the circulation can trigger a series of pro-inflammatory responses and are potential risk factors for inducing or/and promoting chronic inflammatory diseases such as depression and AS.
The gut microbiome promotes depression through PAMP
Current studies have shown that LPS is one of the classical modeling modalities for conducting depressive disease studies due to its pro-inflammatory effects by participating in numerous signaling pathways closely related to depression, such as the autophagic pathway (Ali et al., 2020) or the activation of inflammatory vesicles (Arioz et al., 2019) or tryptophan metabolism (Walker et al., 2019) or Trkb/BDNF signaling (Li et al., 2022b). For example, systemic lipopolysaccharide administration induces the expression of IL-1β and other pro-inflammatory cytokine mRNAs and proteins in the brain, while IL-1β and TNF-α achieve 5-hydroxytryptamine uptake by stimulating synaptosomes in the midbrain and striatum of mice (Dantzer et al., 2008). Acute activation of TLR-4 (Toll-like receptors 4) by lipopolysaccharide or TLR-2 (Toll-like receptors 2) by peptidoglycan increases circulating levels of IFN-γ in mice, which can effectively activate indoleamine 2,3 dioxygenase (IDo) in the periphery and brain, resulting in a decrease in tryptophan levels (Lestage et al., 2002). And tryptophan levels are an important substrate for the synthesis of peripheral and central serotonin, which is one of the important guarantees for the normal functioning of the central nervous system and peripheral blood circulation system. On the other hand, peptidoglycan recognition proteins (PGRPs) are key sensing molecules in the innate immune system for the specific detection of bacterial peptidoglycan (PGN) and its derivatives and are considered potential key regulators of normal brain development and behavior. Bacterial peptidoglycans expressed on the cell walls of Gram-negative and Gram-positive bacteria can influence the development of social behavior by activating specific pathogen recognition receptors, such as PGLYRP2 expressed in the brain (Sherwin et al., 2019). Arentsen first discovered that peptidoglycan can affect the function of neutrophils derived from bone marrow, for which he experimentally found significantly lower levels of PGN in the cerebellum of GF male pups than in the SPF group, and detected the presence of PGN sensing molecules and PGN transporters in the brain, concluding that PGN can cross the blood–brain barrier under normal conditions (Arentsen et al., 2017). He next tested peptidoglycan recognition protein 2 (Pglyrp2) knockout (KO) mice to test the hypothesis that PGRPs play a role in motor control and anxiety-like behavior, and showed that both Pglyrp2 KO male and female mice exhibited anxiety-like behavior and that prefrontal cortex exhibited altered expression of genes related to synaptic plasticities, such as a significant increase in the expression of α-synaptic nuclear protein levels were significantly increased (Arentsen et al., 2018). It has been documented that it can inhibit tyrosine hydroxylase activity, which affects dopamine release (Somayaji et al., 2020), thus impeding normal brain functioning.
The gut microbiome promotes as through PAMP
It has become a consensus that atherosclerosis is a chronic inflammatory disease and that LPS with pro-inflammatory effects enters the bloodstream through the compromised intestinal barrier, thus influencing known risk factors for atherosclerosis, such as platelet invasiveness, thrombosis, foam cell formation, inflammatory response, and oxidative stress (Chen et al., 2020b). A 10-year follow-up study of 2,452 patients found that high levels of lipopolysaccharide were significantly associated with coronary events with a risk ratio of 1.88 (1.13–3.12, p = 0.013, Q2-4 vs. Q1; Kallio et al., 2015). Low-grade endotoxemia is defined when the concentration of circulating levels of LPS is greater than 20 ng/ml. Endotoxin is involved in the thrombogenic process through several mechanisms, including upregulation of macrophage tissue factor expression (Violi et al., 2016) and amplification of platelet responses to common agonists in interaction with Toll-like receptors, stimulation of platelet secretion, and enhancement of platelet aggregation (Zhang et al., 2009; Tunjungputri et al., 2015), representing a novel pathway to amplify thrombus growth at the site of arterial lesions (Carnevale et al., 2020). For example, Jäckel et al. first experimentally demonstrated that the gut microbiome regulates hepatic von Willebrand factor (VWF) expression and plasma VWF levels through the PAMP-triggered TLR2 signaling pathway, thereby promoting VWF-integrin interactions on platelets and inducing arterial thrombus formation (Jäckel et al., 2017). In a subsequent in-depth study, they demonstrated for the first time that ADP-triggered activation of integrin αIIbβ3 is regulated by commensal microbiota, and that integrin αIIbβ3 synergizes with other platelet adhesion receptors and contributes to the deposition of type I collagen matrix under various conditions, playing an important role in thrombus formation (Kiouptsi et al., 2020). On the other hand, Zhou et al. conducted a clinical trial in 100 patients with ST-segment elevation myocardial infarction and confirmed that gut microbiome translocation leads to the accumulation of LPS in the circulatory system, and hypothesized that elevated LPS triggers monocyte recruitment, which activates systemic inflammation and ultimately leads to cardiac injury (Zhou et al., 2018). Similarly, Ramana also suggested that LPS-induced endotoxemia can produce myocardial depression (Ramana et al., 2006). In addition to confirming the association of LPS from gut microbiome with the development of AS, its pro-AS effect was similarly confirmed by direct injection of external LPS. In animal experiments, direct injection of LPS into rabbits accelerated the pathological process of cholesterol-induced atherosclerosis (Lehr et al., 2001), and by subcutaneously injecting mice with lipopolysaccharide for 1 month, it was shown that their fasting blood glucose, as well as the increase in systemic adipose tissue, were similar to those of high-fat-fed mice (Cani et al., 2007). Furthermore, as another common microbiome cell wall component peptidoglycan, its recognition is mediated by several families of pattern recognition molecules, including toll-like receptors, nucleotide-binding oligomerization domain-containing proteins, and PGRPs (Guan and Mariuzza, 2007). Peptidoglycan recognition protein-1 (PGLYRP-1) is part of the innate immune system that binds peptidoglycan and has attracted the attention of a wide range of researchers. Rohatgi measured PGLYRP-1 in 3222 subjects and reported for the first time that its circulating levels were associated with widespread subclinical atherosclerosis in humans; Among 2,443 patients without cardiovascular disease at baseline, elevated levels of circulating PGLYRP-1 at baseline were independently associated with an increased risk of a first ASCVD event (Rohatgi et al., 2009; Brownell et al., 2016), suggesting that the biological processes reflected by elevated PGLYRP-1 may be strongly associated with the development of clinical ASCVD.
Bile acids
Bile acids, an important component of bile, are produced by the metabolism of host cholesterol in the liver and play an important role in fat digestion and energy metabolism. Circulating bile acids consist of primary bile acids produced by hepatic cholesterol and secondary bile acids formed by specific intestinal bacteria, which regulate their own and other substances’ metabolism by activating specific nuclear receptors (NRs) and G protein-coupled receptors (GPCRs), both as detergent molecules that facilitate nutrient absorption and as hormones that regulate nutrient metabolism (Ridlon et al., 2016). The biosynthesis of bile acids involves modification of the ring structure of cholesterol, oxidation and shortening of the side chain, and finally the coupling of bile acids to amino acids (Russell, 2003). The synthesis of bile acids relies on two main pathways: one is initiated by the cholesterol 7α-hydroxylation reaction catalyzed by the rate-limiting enzyme cholesterol 7a-hydroxylase (CYP7A1), which accounts for 75% of the total synthesis (Thomas et al., 2008); and the other is initiated by the catalysis of sterol-27-hydroxylase (CYP27A1; Russell, 2003), the formed 27-hydroxycholesterol is further hydroxylated by 7α-hydroxylase (CYP7B1; Thomas et al., 2008). Sayin et al. demonstrated that gut microbiome can regulate the expression of the enzymes cholesterol 7α-hydroxylase (CYP7A1), oxysterol 7α-hydroxylase (CYP7B1) and sterol-27-hydroxylase (CYP27A1; Sayin et al., 2013),which subsequently mediate the synthesis of bile acids. Interestingly, gut microbiome are also extensively involved in bile acid conversion and metabolic pathways. One of the most studied microbially driven biotransformations is the bile salt hydrolase (BSH) activity of bacteria that undergo bile acid deconjugation. BSH is widely distributed in the major bacterial divisions and archaea species in the human gut, including Clostridium, bifidobacterium, enterococcus, Lactobacillus, Bacteroides, Methanobacterium Smith, Methanococcus and many other species, and is more abundant in the gut microbiome than in other microbial ecosystems. Besides deconjugation, intestinal microorganisms are the only source of 7α and 7β dehydroxylase activity, which produces “secondary” bile acids such as deoxycholic acid (DCA), lithic cholic acid (LCA), Hyodeoxycholic acid (HDCA) and ursodeoxycholic acid (UDCA; Wahlström et al., 2016). Overall, gut microbiome chemically diversify the bile acid pool through deconjugation, oxidation, exo-isomerization, 7α/7β dehydroxylation, esterification and desulfation, thereby allowing secondary bile acids to enter the portal circulation and function as endocrine-like signaling molecules with effective effects on host physiology and disease (Brown and Hazen, 2018).
Bile acids are closely associated with depression
Conjugated and non-conjugated bile acids, as well as taurine or glycine, are potential neuroactive ligands (MahmoudianDehkordi et al., 2019; Spichak et al., 2021), such as ursodeoxycholic acid that can exert beneficial effects (MahmoudianDehkordi et al., 2019). The signaling of bile acids to the central nervous system includes direct and indirect pathways. The direct way is demonstrated by the fact that unconjugated and conjugated bile acids can cross the blood–brain barrier to reach the brain (Keene et al., 2001), where there are bile acid signaling mechanisms, i.e., receptors and transporter proteins capable of binding bile acids to transport them to neurons (Mertens et al., 2017). The indirect pathway is mainly triggered by the activation of the nuclear hormone receptor farnesoid X receptor (FXR) and Takeda G protein-coupled receptor 5 (TGR5). In line with this, both receptors, FXR (Huang et al., 2016; McMillin et al., 2016) and TGR5 (Keitel et al., 2010; Yanguas-Casás et al., 2017), in addition to being abundantly expressed in the enterohepatic circulation, have been detected in the brain. Interestingly, FXR knockout mice exhibited less depression-like and anxiety-related behaviors and altered neurotransmitter concentrations in different brain regions, such as an increased ratio of γ-aminobutyric acid to glutamate concentrations in the hippocampus, in addition to similar changes in serum and brain levels of various bile acids (Huang et al., 2015). Chen et al. similarly found that chronic unpredictable mild stress (CUMS) completely enhanced the expression of FXR protein and mRNA in the hippocampus, and overexpression of FXR in the hippocampus caused significant depression-like behavior and decreased expression of brain-derived neurotrophic factor (BDNF), while knockdown of FXR in the hippocampus completely inhibited the effects of CUMS on rat behavior and hippocampal BDNF expression (Chen et al., 2018). In addition, altered bile acid levels are closely associated with the development of central nervous system disorders. A study on Autism spectrum disorder found that reduced relative abundance of specific bacterial taxa (e.g., bile-metabolizing Bifidobacterium and Blautia species) was associated with deficient intestinal bile acid and tryptophan metabolism, significant gastrointestinal dysfunction, and social interaction impairment (Golubeva et al., 2017). By metabolomic analysis of the liver of depression model mice, Jia et al. found that initial bile acids play a key role in CUMS-induced depression in mice (Jia et al., 2016). In another study it was confirmed that abnormal activation of the secondary bile acid biosynthetic pathway thereby increasing the hydrophobicity of the bile acid pool, which in turn may contribute to the progression of metabolic disorders and depression-like behavior in CUMS mice (Qu et al., 2022). A recent review outlined the therapeutic potential of ursodeoxycholic acid and its conjugated species in neurological, neurodegenerative and neuropsychiatric disorders, affirming their positive anti-apoptotic, antioxidant and anti-inflammatory effects (Huang et al., 2022). These aforementioned findings provide new insights into the therapeutic options for depression.
Bile acids play an important role in the pathogenesis of AS
In addition to acting on the central nervous system, there is evidence that bile acids act by binding to different receptors, including but not limited to facilitating lipid digestion, maintaining glucose, lipid and energy homeostasis, and inflammation (Witkowski et al., 2020; Brown et al., 2021). One host bile acid receptor that has received attention in recent years is the G protein-coupled receptor TGR5 (Watanabe et al., 2006; Pols et al., 2011), and TGR5 knockout mice are protected from the effects of atherosclerosis (Watanabe et al., 2006). Interestingly, certain bacterially modified bile acids (3-oxo-staphylococcal acid and staphylococcal acid) can also activate the vitamin D receptor (VDR), and genetic studies in both humans and mice have shown that VDR activation is associated with cardiovascular disease (Makishima et al., 2002). Notably, the hormone FGF19, which is secreted due to the activation of FXR by bile acid-binding, and its overexpression in the mouse brain leads to increased energy expenditure, and animals on a high-fat diet do not become diabetic or obese (Tomlinson et al., 2002), whereas the beneficial effect of systemic FGF19 on glucose metabolism is reduced by 50% when FGFR antagonists are injected into the brain (Morton et al., 2013), and the disruption of glucose metabolism is one of the factors contributing to the development of AS. In addition to binding to receptors, alterations in specific bile acid levels in cardiometabolic phenotypes and disease susceptibly have received a lot of attention. For example, alterations in plasma bile acid levels are associated with insulin resistance in type 2 diabetes, specifically referring to higher 12α-hydroxy/non-12α-hydroxy BA ratios were associated with lower insulin sensitivity and higher plasma triglyceride (Haeusler et al., 2013), in line with this, Gu et al. found that the treatment of diabetes was achieved by altering the relative abundance of microorganisms involved in bile acid metabolism, which in turn increased the ratio between primary and secondary bile acids and the level of unconjugated bile acids in plasma (Gu et al., 2017). Given the important regulatory role of bile acids in the development of AS, Xu et al. used activated transcription factor 3 to prevent atherosclerosis by inducing intrahepatic scavenger receptor group B type 1 and repressing cholesterol 12α-hydroxylase to interact with p53 and hepatocyte nuclear factor 4α, respectively, to regulate HDL and bile acid metabolism (Xu et al., 2021). Similarly, another study confirmed that by administering Resveratrol, the levels of genera Lactobacillus and Bifidobacterium could be increased, thus increasing the activity of bile salt hydrolases, which enhanced bile acid deconjugation and excretion to attenuate the formation of AS in mice (Chen et al., 2016). Overall, the above findings suggest that the gut microbiome can influence the development of AS co-depression pathology by affecting the synthesis and metabolism of bile acids.
Tryptophan and derivatives
Tryptophan (Trp) is mainly ingested through food, and modern studies have found that the gut microbiome plays an important regulatory role in all three major pathways of Trp metabolism, including the 5-hydroxytryptamine (5-HT) pathway, the kynurenine (KYN) pathway, and the production of ligand-indole derivatives of the aromatic hydrocarbon receptor (AhR; Agus et al., 2018). However, it is not clear how the gut microbiome mediates the tryptophan metabolic pathway, but there is experimental evidence that microbiome metabolites such as secondary bile acids (Peregrin et al., 1999; Bunnett, 2014; Yano et al., 2015) and short-chain fatty acids (Fukumoto et al., 2003; Atarashi et al., 2013; Reigstad et al., 2015; Barbara et al., 2016) can act on Enterochromaffin cells (ECs) to promote the synthesis and release of serotonin. On the other hand, many microorganisms can synthesize serotonin directly from tryptophan (O'Mahony et al., 2015), which may be related to their ability to express tryptophan synthase. That gut microbiome is an important factor in 5-HT production is well documented in GF animal models. In one experiment, Sjogren found lower 5-HT concentrations in the blood of GF mice than in the normal group of mice (Sjögren et al., 2012), and another experiment similarly confirmed reduced 5-HT levels in the hippocampus of GF rats (Crumeyrolle-Arias et al., 2014). It is estimated that about 90% of Trp is used to produce KYN, which is mainly regulated by indoleamine-2,3-dioxygenase (IDO) or tryptophan 2,3-dioxygenase (TDO) in the KYN pathway (Gao et al., 2020). Furthermore, KYN and its metabolites, mainly kynurenic acid (KYNA) and quinolinic acid (QUIN), of which KYNA is considered to be a neuroprotective N-methyl-D-aspartic acid receptor (NMDA) receptor antagonist and QUIN is considered to be a neurotoxic NMDA receptor agonist (Pierozan et al., 2016), are closely associated with mental health (Cervenka et al., 2017). Some metabolites produced by intestinal microorganisms, such as SCFAs, particularly butyrate, are also known to regulate the KYN pathway (Kennedy et al., 2017), and Martin-Gallausiaux found that butyrate downregulates IDO-1 expression through a dual mechanism of reduced STAT1 levels and histone deacetylases (HDACs) inhibitor properties of SCFAs (Martin-Gallausiaux et al., 2018). In addition, Trp can be converted into several indole derivatives such as IAld and ILA by some Lactobacilli (Cervantes-Barragan et al., 2017). Many Gram-positive and Gram-negative bacteria encode a copy of the tryptophanase gene in their chromosomes and produce indoles, 85 species according to the count at that time (Lee and Lee, 2010).
Studies of tryptophan and metabolites for depression
It has been shown that Trp can cross the blood–brain barrier and that circulating Trp from the periphery can affect Trp levels in the brain (Schwarcz et al., 2012). Messaoud found through clinical studies that low plasma Trp levels may be a biomarker of suicide in MDD and MDD patients and he suggested that reduced effectiveness of Trp for 5-HT synthesis and increased activation of the KYN pathway for associated with depression and suicide (Messaoud et al., 2019). As one of the tryptophan metabolites, 5-HT, is an important neurotransmitter involved in the control of adaptive responses in the central nervous system and associated with changes in mood, anxiety, or cognition (Canli and Lesch, 2007; Gonçalves et al., 2022), and plays an important role in neuronal differentiation and migration, as well as in axon growth, myelin and synapse formation (Gao et al., 2020), and the reduced availability of 5-HT in the brain is a depression a key feature of the pathogenesis of depression (Agus et al., 2018), consistent with the classic monoamine hypothesis in the etiological content of depression. One study found that male GF mice exhibited anxious behavior and significantly higher concentrations of 5-HT and its major metabolite 5-hydroxyindoleacetic acid in the hippocampus compared to conventional mice, suggesting that microbes can affect 5-HT neurotransmission in the central nervous system through humoral pathways (Clarke et al., 2013).
Many current studies confirm that depression is likewise a chronic inflammatory state. The Th1-type cytokine interferon-γ can lead to increased Trp catabolism and increased KYN/TRP ratio through activation of IDO activity (Yoshida et al., 1981; Taylor and Feng, 1991; Hwu et al., 2000). Increased levels of QUIN, one of the products of the KYN metabolic pathway, are closely associated with several distinguishing features of depression: reduced reaction time, cognitive deficits, and learning ability (Müller and Schwarz, 2007). QUIN can activate the NMDA receptor signaling pathway, leading to excitotoxicity and amplifying the inflammatory response, while KYNA is an antagonist of all ionotropic glutamate receptors and therefore could potentially block some of the effects of QUIN and other excitotoxins (Lim et al., 2017). In addition, KYNA is also a noncompetitive antagonist of low concentrations of alpha-7 nicotinic acetylcholine receptor (α7 nAChR), which is associated with learning and memory (Banerjee et al., 2012), and reduced KYNA levels may be involved in the pathophysiological mechanisms of depression by inhibiting α7 nAChR. When there is a relative imbalance between KYNA, which has neuroprotective effects, and QUIN, which has neurotoxic effects, it could explain the result that Meier et al. observed a reduction in medial prefrontal cortex thickness in MDD (Meier et al., 2016). Notably, Ogyu et al. showed reduced levels of KYNA and KYN in depressed patients by performing a meta-analysis of KYN pathway metabolite levels in depressed patients versus controls (Ogyu et al., 2018). The limitation of this study is that most of the reports included examined peripheral blood, but it is unclear to what extent peripheral KYN metabolites reflect the amount contained in the brain; after all, it is controversial whether each metabolite can cross the blood–brain barrier, or the amount that can enter the brain is inconsistent.
Studies of tryptophan and metabolites in the development of AS pathology
5-HT was first identified from serum, also called serotonin. Very early studies have confirmed that platelets secrete serotonin, which promotes thrombogenesis, mitotic and proliferative processes in smooth muscle cells and is closely associated with AS development (Vikenes et al., 1999). Interestingly, Rami et al. found a pro-AS effect of SSRIs, confirming experimentally that SSRIs deplete major peripheral 5-HT stores mainly by inhibiting 5-HT reuptake transporter-mediated uptake in platelets (Rami et al., 2018), suggesting that peripheral serotonin levels are not the only key factor contributing to AS, which led us to focus more attention on related receptors. It has been noted that all 5-HT receptors, except the 5-HT6 type, are involved in cardiovascular regulation (Ramage and Villalón, 2008). Furthermore, it has been demonstrated that upregulation and/or increased sensitivity of peripheral 5-HT2A/1B receptors and downregulation of 5-HT transporter receptors may contribute to an increased risk of thromboembolic events in patients with depression and cardiovascular disease (Schins et al., 2003). Activation of the KYN pathway also plays an important role in atherogenesis. Song noted in his article that IDO activity in the blood positively correlates significantly with the progression of atherosclerosis (Song et al., 2017), and inhibiting IDO1 leads to more significant atherosclerotic lesions in ApoE−/− mice fed a high-fat diet (Polyzos et al., 2015), while treatment of Ldlr−/− mice with the tryptophan metabolite 3-hydroxycyanuric acid inhibits atherosclerosis by modulating lipid metabolism and inflammation (Zhang et al., 2012) is more evidence that activation of the KYN pathway can influence the pathological development of AS. On the other hand, a large fraction of Trp enters the indole pathway and is metabolized to Tryptamine and indole metabolites with signaling activity, which are subsequently involved in the pathogenesis of AS. For example, one of the products, indoxyl sulfate, is harmful to various cell types, including vascular endothelial cells (Hung et al., 2016), and has been shown to promote a procoagulant state in vitro and endothelial dysfunction in vivo (Dou et al., 2004) as well as aortic calcification (Adijiang et al., 2008), while indole-3-propionic acid and indole-3-aldehyde have anti-inflammatory protective effects (Paeslack et al., 2022). Direct injection of indole-3-propionic acid elevates blood pressure and increases cardiac contractility and cardiomyocyte metabolic activity (Konopelski et al., 2021). Indole derivatives act mainly through activation of AHR (Zelante et al., 2013), and AHR signaling is recognized to contribute to the development of AS-related diseases through inducing of IL-1β, IL-8 expression (Dahlem et al., 2020) and effects on different cell types closely related to atherogenesis (Wang et al., 2020), while treatment with AhR antagonists reduces the progression of atherosclerotic lesions (Wu et al., 2011) further confirms this important conclusion.
On the other hand, melatonin, located in the pineal gland, is similarly derived from the synthesis of L-Trp. There is growing evidence that melatonin has anti-inflammatory, antioxidant, hypotensive, and possibly anti-lipidemic properties (Dominguez-Rodriguez et al., 2010). It has been found that disorders of Trp metabolism resulting in melatonin deficiency may lead to abnormal hormone levels (e.g., aldosterone retention of water leading to increased blood pressure), which can lead to cardiovascular disease (Doi et al., 2010). In addition, melatonin has been shown to significantly improve antioxidant defense (increased catalase activity and reduced levels of thiobarbituric acid reactive substrates) and lipid-lowering (reduced LDL-C) and to lower blood pressure and inhibit plasma cholesterol levels in hypercholesterolemic rats (Koziróg et al., 2011). In line with this, Dominguez-Rodriguez et al. reported elevated levels of oxidized LDL and impaired nocturnal melatonin synthesis in patients with myocardial infarction, and this study was the first to confirm an independent correlation between oxidized LDL levels and melatonin levels at night in patients with myocardial infarction (Dominguez-Rodriguez et al., 2005). Overall, melatonin effectively interacts with various reactive oxygen species and reactive nitrogen species, and it also upregulates antioxidant enzymes and downregulates pro-oxidant enzymes, attenuating the molecular and cellular damage caused by free radicals involved in cardiac ischemia/reperfusion. These anti-inflammatory and antioxidant properties contribute to the prevention of atherosclerosis (Tengattini et al., 2008).
Short-chain fatty acids
Short-chain fatty acids (SCFAs) are saturated fatty acids with carbon atoms ranging from one to six in length and are the main products of dietary fiber fermentation in the colon (Dalile et al., 2019). In the intestine, the conversion of dietary fiber to SCFAs involves a series of major reactions that are mediated by enzymes of specific members of the gut microbiome, and the end products are mainly acetic, propionic, and butyric acids (Koh et al., 2016). SCFAs are rapidly absorbed by colon cells mainly through active transport mediated by monocarboxylic acid transport proteins (MCTs), and the absorbed SCFAs pass through the blood circulation, reaching all parts of the body, including the brain (Dalile et al., 2019), which may be related to the high expression of MCTs on endothelial cells (Vijay and Morris, 2014). In addition, it has been found that propionic acid has a beneficial protective effect on the blood–brain barrier by inhibiting pathways associated with nonspecific microbial infections through a CD14-dependent mechanism and inhibiting LRP-1 expression to reducing harmful inflammation and oxidative stimulation (Hoyles et al., 2018), in line with this, treatment with SCFAs reversed the pathology of increased permeability of the blood–brain barrier in GF mice (Braniste et al., 2014).
Studies related to SCFAs in depression
It has been found that butyric acid (Braniste et al., 2014) and acetate (Soliman and Rosenberger, 2011), inhibit the activity of HDACs and promote the hyperacetylation of histones, which are associated with neuropsychiatric disorders such as depression (Covington 3rd et al., 2009; Sarkar et al., 2014). Li et al. demonstrated by static and dynamic metabolomic analysis that propionic acid is a differential metabolite in CUMS rats, based on these findings, subsequent intrarectal administration of sodium propionate (the salt form of propionic acid) was used to confirm that propionic acid improved depression-like behavior in CUMS rats, which was linked to reduced catabolism of norepinephrine, tryptophan, and dopamine in the prefrontal cortex (Li et al., 2018b). In line with this, Marcel van de Wouw et al. found that short-chain fatty acids counteracted the lasting effects of chronic psychosocial stress and acted as antidepressants and anxiolytics by orally administering a mixture of three major short-chain fatty acids (acetic acid, propionic acid, and butyric acid) to mice (van de Wouw et al., 2018). The aforementioned studies have explored the direct therapeutic effects of SCFAs, and exploring the indirect therapeutic effects of SCFAs by focusing on the gut microbiome has also attracted widespread interest. For example, prebiotic administration increased cecum acetate and propionate concentrations and decreased isobutyrate concentrations, and these changes were significantly correlated with improvements in depressive behavior (Burokas et al., 2017). Another study found that treatment with electroacupuncture increased the relative abundance of SCFAs-producing bacteria, including Ruminococcaceae, Phascolarctobacterium, Akkermansiaceae, Romboutsia, and Blautia, which may be a result of electroacupuncture mediating SCFAs through the microbiota-gut-brain to improve evidence of anxiety and depression-like behaviors (Zhou et al., 2022). In addition to validation in animal experiments, the correlation between the concentration of SCFAs and the severity of depressive symptoms was even more revealed in a clinical trial, which found that the vast majority of SCFAs concentrations were higher in non-depressed women, with significantly lower levels of acetic acid, decreasing levels of propionic acid, and significantly higher concentrations of isocaproic acid compared to non-depressed women; Spearman correlation analysis showed that the concentrations of acetic acid and propionic acid were negatively correlated with BDI score (Skonieczna-Żydecka et al., 2018). As described by Oleskin, short-chain fatty acids act within intestinal endocrine cells, thereby stimulating the production of histamine, serotonin, 5-aminovaleric acid, and γ-aminobutyric acid, all of which are neuroactive compounds that are strongly associated with depressive-like behavior (Oleskin and Shenderov, 2016). Based on the analysis of the above findings, not all SCFAs have antidepressant effects, and there are contradictory results in some of the animal and human experiments; excluding the reasons for the differences between species, more experiments are still needed to investigate the specific substances that exert therapeutic effects.
SCFAs as a potential strategy for the treatment of AS
Available evidence suggests that reliance on short-chain fatty acids produced by fermentation of dietary fiber by the gut microbiome may likewise be an effective preventive strategy for ameliorating atherosclerosis. Bartolomaeus et al. found a significant reduction in aortic atherosclerotic lesion area in propionic acid-treated ApoE−/− mice (Bartolomaeus et al., 2019), and administration of Lactobacillus fermentum caused cecum microbiota alterations, increased colonic short-chain fatty acid levels, and reduced AS-related risk factors such as serum LDL, total cholesterol, and triglyceride levels (Yang et al., 2021). In addition to the hypolipidemic effect, Li et al. found that short-chain fatty acids could inhibit LPS or TNFα-induced endothelial inflammatory response and excessive vascular cell adhesion molecule-1 (VCAM-1) expression, which are two important steps in the development of atherosclerosis (Li et al., 2018d), and further studies found that SCFA could activate G-protein coupled receptor 41/43 and inhibit HDACs, playing a beneficial role in the treatment of AS-related diseases (Li et al., 2018c). Similarly, Shi et al. experimentally confirmed that the mechanism of Pae anti-AS is related to the improvement of Treg/Th17 balance in the spleen by increasing the production of microbiome-derived short-chain fatty acids (Shi et al., 2021).
Trimethylamine N-oxide
In rodents and humans, gut microbiome enzymes convert choline and L-carnitine from food into a volatile gas called trimethylamine (TMA; Baker and Chaykin, 1962). TMA enters the liver through the portal circulation where it is converted to trimethylamine N-oxide (TMAO; Komaroff, 2018).
TMAO is a strong predictor of AS-related diseases
TMAO production from phosphatidylcholine in feed has long been found to be associated with an increased risk of major adverse cardiovascular events, which is dependent on the metabolism of the gut microbiome (Wang et al., 2011; Koeth et al., 2013; Tang et al., 2013). Using non-targeted metabolomics as a platform for discovery, we further believe that TMAO is a strong predictor of AS-related disease by showing a causal relationship between TMAO and atherosclerosis (Wang et al., 2011). Interestingly, Lindskog Jonsson et al. later found experimentally that TMAO concentrations were not associated with atherosclerotic lesion size, and he attributed the contradictory findings of his experiments with those of previous investigators to a different experimental setup probably: previous studies used antibiotics to deplete the gut microbiome and started choline supplementation at weaning, whereas his experiments supplemented choline at 8 weeks of age when the atherosclerotic disease had already begun to develop. In addition, he suggested the interesting conjecture that dietary choline may be an important factor influencing the development of early atherosclerosis (Lindskog Jonsson et al., 2018). The mechanisms by which TMAO is thought to increase the risk of cardiovascular disease are diverse and include altered tissue sterol metabolism, enhanced endothelial cell activation and vascular inflammation, and pro-fibrotic signaling pathway stimulation (Roberts et al., 2018). Notably, the gut microbiome can directly promote platelet hyperresponsiveness and enhance thrombogenic potential through the production of TMAO (Zhu et al., 2016), and in subsequent human feeding studies, TMAO levels were significantly increased by 10-fold in healthy volunteers following oral choline administration, platelet reactivity and aggregation were enhanced, and a significant dose-dependent association was observed between plasma TMAO levels and platelet function (Zhu et al., 2017). These results suggest the possibility of using the TMAO pathway of the gut microbiome as a therapeutic strategy, such as inhibiting atherosclerotic plaque formation through dietary control, improving the microbial community with probiotics, or inhibiting key enzymes that produce TMAO and-moderating the platelet hyperreactivity associated with elevated TMAO, is worthy of further investigation.
Advances in the study of TMAO in depression
TMAO promotes brain aging and cognitive impairment in addition to being an influential factor in cardiovascular disease (Li et al., 2018a). One study found that participants with PTSD symptoms had significantly higher TMAO levels immediately after acute myocardial infarction than patients without acute myocardial infarction symptoms and that TMAO could be a significant predictor of PTSD symptoms (Baranyi et al., 2021). In addition, a clinical trial based on 251 individuals found a positive correlation between serum TMAO levels and the severity of depressive symptoms (Meinitzer et al., 2020). Consistent with this, TMAO levels were significantly higher in depressed patients than in healthy controls (Liu et al., 2015). Although the above study only found peripheral TMAO levels to be associated with depressive symptoms, this suggests to us that TMAO is capable of contributing in some way to the development of central disorders. Coincidentally, Chen et al. experimentally identified the endoplasmic reticulum stress kinase PERK [RNA-dependent protein kinase (PKR)-like ER kinase] as a receptor for TMAO, which induces the endoplasmic reticulum stress signaling pathway by binding to the endoplasmic reticulum stress protein PERK (Chen et al., 2019), More importantly, endoplasmic reticulum stress has been shown in many studies to mediate one of the pathogenic mechanisms of psychiatric disorders such as depression (Gold et al., 2013; Xiang et al., 2017). In addition to the detection of TMAO levels alone, Zheng et al. similarly demonstrated that depression patients had altered concentrations of gut microbiome metabolites, including TMAO (Zheng et al., 2013). Overall, these studies support the association of TMAO with the development of depression and provide new treatment options for the treatment of depression.
Conclusion
The time has come to study microbiome metabolites, mainly PAMP, bile acid, tryptophan and derivatives, SCFAs, and TMAO, which play a central role in the pathophysiology of depression and AS. There is reason to believe that this is potential pathogenesis common to both diseases, depression and AS, and that some of these factors may serve as potential biological targets for early diagnosis and treatment of AS co-depression disorders. We can expect that soon, patients with AS co-depression can get rid of the physical and mental burden caused by multiple drug use, which is an important and valuable research direction that needs to be explored deeply.
Limitations
In this review, due to the limited existing research results related to AS co-depression, we only elaborated on the important research results of each microbiome metabolite in depression and AS disease, respectively. Due to the interaction between two kinds of the disease being complex and has not yet been studied clearly, the studies in a separate state of disease are flawed, and more researchers are required to conduct more in-depth follow-up experiments in the state of AS co-depression. In addition, the gut microbiome can communicate with its hosts in both directions and can influence each other, and the ways of communication include neurological, immunological, and metabolic pathways. This review simply describes how gut microbes affect the host’s disease by mediating metabolites in a unidirectional manner, and only a few common metabolites are described to provide the reader with a preliminary overview of the critical role of gut microbiome metabolites in AS co-depression diseases and to provide ideas for researchers interested in this field. Finally, it is still unclear which specific bacteria and the metabolites mediated by them are most conducive to improving the state of AS co-depression, which needs to be further verified in larger preclinical and clinical studies.
Author contributions
X-XL: writing and drawing—original draft preparation. X-YW, Y-LZ, and J-JL: writing—review and editing. Y-LW and J-JZ: supervision. Y-LW: project administration. X-YW: funding acquisition. All authors contributed to the article and approved the submitted version.
Funding
This work was supported by The Talent Starting Fund Project of Gannan Medical University [grant numbers QD202208 and QD202012] and The Open Project of Key Laboratory of Prevention and Treatment of Cardiovascular and Cerebrovascular Diseases, Ministry of Education [grant number XN202015].
Conflict of interest
The authors declare that the research was conducted in the absence of any commercial or financial relationships that could be construed as a potential conflict of interest.
Publisher’s note
All claims expressed in this article are solely those of the authors and do not necessarily represent those of their affiliated organizations, or those of the publisher, the editors and the reviewers. Any product that may be evaluated in this article, or claim that may be made by its manufacturer, is not guaranteed or endorsed by the publisher.
References
Adijiang, A., Goto, S., Uramoto, S., Nishijima, F., and Niwa, T. (2008). Indoxyl sulphate promotes aortic calcification with expression of osteoblast-specific proteins in hypertensive rats. Nephrol. Dial. Transplant. 23, 1892–1901. doi: 10.1093/ndt/gfm861
Agus, A., Planchais, J., and Sokol, H. (2018). Gut microbiota regulation of tryptophan metabolism in health and disease. Cell Host Microbe 23, 716–724. doi: 10.1016/j.chom.2018.05.003
Ali, T., Rahman, S. U., Hao, Q., Li, W., Liu, Z., Ali Shah, F., et al. (2020). Melatonin prevents neuroinflammation and relieves depression by attenuating autophagy impairment through FOXO3a regulation. J. Pineal Res. 69:e12667. doi: 10.1111/jpi.12667
Arentsen, T., Khalid, R., Qian, Y., and Diaz Heijtz, R. (2018). Sex-dependent alterations in motor and anxiety-like behavior of aged bacterial peptidoglycan sensing molecule 2 knockout mice. Brain Behav. Immun. 67, 345–354. doi: 10.1016/j.bbi.2017.09.014
Arentsen, T., Qian, Y., Gkotzis, S., Femenia, T., Wang, T., Udekwu, K., et al. (2017). The bacterial peptidoglycan-sensing molecule Pglyrp2 modulates brain development and behavior. Mol. Psychiatry 22, 257–266. doi: 10.1038/mp.2016.182
Arioz, B. I., Tastan, B., Tarakcioglu, E., Tufekci, K. U., Olcum, M., Ersoy, N., et al. (2019). Melatonin attenuates LPS-induced acute depressive-like behaviors and microglial NLRP3 Inflammasome activation through the SIRT1/Nrf2 pathway. Front. Immunol. 10:1511. doi: 10.3389/fimmu.2019.01511
Atarashi, K., Tanoue, T., Oshima, K., Suda, W., Nagano, Y., Nishikawa, H., et al. (2013). Treg induction by a rationally selected mixture of clostridia strains from the human microbiota. Nature 500, 232–236. doi: 10.1038/nature12331
Baker, J. R., and Chaykin, S. (1962). The biosynthesis of trimethylamine-N-oxide. J. Biol. Chem. 237, 1309–1313. doi: 10.1016/S0021-9258(18)60325-4
Banerjee, J., Alkondon, M., and Albuquerque, E. X. (2012). Kynurenic acid inhibits glutamatergic transmission to CA1 pyramidal neurons via α7 nAChR-dependent and -independent mechanisms. Biochem. Pharmacol. 84, 1078–1087. doi: 10.1016/j.bcp.2012.07.030
Baranyi, A., Enko, D., von Lewinski, D., Rothenhäusler, H. B., Amouzadeh-Ghadikolai, O., Harpf, H., et al. (2021). Assessment of trimethylamine N-oxide (TMAO) as a potential biomarker of severe stress in patients vulnerable to posttraumatic stress disorder (PTSD) after acute myocardial infarction. Eur. J. Psychotraumatol. 12:1920201. doi: 10.1080/20008198.2021.1920201
Barbara, G., Feinle-Bisset, C., Ghoshal, U. C., Quigley, E. M., Santos, J., Vanner, S., et al. (2016). The intestinal microenvironment and functional gastrointestinal disorders. Gastroenterology 150, 1305–1318. doi: 10.1053/j.gastro.2016.02.028
Bartolomaeus, H., Balogh, A., Yakoub, M., Homann, S., Markó, L., Höges, S., et al. (2019). Short-chain fatty acid propionate protects from hypertensive cardiovascular damage. Circulation 139, 1407–1421. doi: 10.1161/circulationaha.118.036652
Brandsma, E., Kloosterhuis, N. J., Koster, M., Dekker, D. C., Gijbels, M. J. J., van der Velden, S., et al. (2019). A Proinflammatory gut microbiota increases systemic inflammation and accelerates atherosclerosis. Circ. Res. 124, 94–100. doi: 10.1161/circresaha.118.313234
Braniste, V., Al-Asmakh, M., Kowal, C., Anuar, F., Abbaspour, A., Tóth, M., et al. (2014). The gut microbiota influences blood-brain barrier permeability in mice. Sci. Transl. Med. 6:263ra158. doi: 10.1126/scitranslmed.3009759
Brown, R. M., Guerrero-Hreins, E., Brown, W. A., le Roux, C. W., and Sumithran, P. (2021). Potential gut-brain mechanisms behind adverse mental health outcomes of bariatric surgery. Nat. Rev. Endocrinol. 17, 549–559. doi: 10.1038/s41574-021-00520-2
Brown, J. M., and Hazen, S. L. (2018). Microbial modulation of cardiovascular disease. Nat. Rev. Microbiol. 16, 171–181. doi: 10.1038/nrmicro.2017.149
Brownell, N. K., Khera, A., de Lemos, J. A., Ayers, C. R., and Rohatgi, A. (2016). Association between peptidoglycan recognition Protein-1 and incident atherosclerotic cardiovascular disease events: the Dallas heart study. J. Am. Coll. Cardiol. 67, 2310–2312. doi: 10.1016/j.jacc.2016.02.063
Bunnett, N. W. (2014). Neuro-humoral signalling by bile acids and the TGR5 receptor in the gastrointestinal tract. J. Physiol. 592, 2943–2950. doi: 10.1113/jphysiol.2014.271155
Burokas, A., Arboleya, S., Moloney, R. D., Peterson, V. L., Murphy, K., Clarke, G., et al. (2017). Targeting the microbiota-gut-brain Axis: prebiotics have anxiolytic and antidepressant-like effects and reverse the impact of chronic stress in mice. Biol. Psychiatry 82, 472–487. doi: 10.1016/j.biopsych.2016.12.031
Cai, Y. Y., Huang, F. Q., Lao, X., Lu, Y., Gao, X., Alolga, R. N., et al. (2022). Integrated metagenomics identifies a crucial role for trimethylamine-producing Lachnoclostridium in promoting atherosclerosis. NPJ Biofilms Microbiomes 8:11. doi: 10.1038/s41522-022-00273-4
Cani, P. D., Amar, J., Iglesias, M. A., Poggi, M., Knauf, C., Bastelica, D., et al. (2007). Metabolic endotoxemia initiates obesity and insulin resistance. Diabetes 56, 1761–1772. doi: 10.2337/db06-1491
Canli, T., and Lesch, K.-P. (2007). Long story short: the serotonin transporter in emotion regulation and social cognition. Nat. Neurosci. 10, 1103–1109.
Carnevale, R., Sciarretta, S., Valenti, V., di Nonno, F., Calvieri, C., Nocella, C., et al. (2020). Low-grade endotoxaemia enhances artery thrombus growth via toll-like receptor 4: implication for myocardial infarction. Eur. Heart J. 41, 3156–3165. doi: 10.1093/eurheartj/ehz893
Cervantes-Barragan, L., Chai, J. N., Tianero, M. D., Di Luccia, B., Ahern, P. P., Merriman, J., et al. (2017). Induces gut intraepithelial CD4CD8αα T cells. Science (New York, N.Y.) 357, 806–810. doi: 10.1126/science.aah5825
Cervenka, I., Agudelo, L. Z., and Ruas, J. L. (2017). Kynurenines: Tryptophan's metabolites in exercise, inflammation, and mental health. Science (New York, N.Y.) 357:9794. doi: 10.1126/science.aaf9794
Chen, P. B., Black, A. S., Sobel, A. L., Zhao, Y., Mukherjee, P., Molparia, B., et al. (2020a). Directed remodeling of the mouse gut microbiome inhibits the development of atherosclerosis. Nat. Biotechnol. 38, 1288–1297. doi: 10.1038/s41587-020-0549-5
Chen, S., Henderson, A., Petriello, M. C., Romano, K. A., Gearing, M., Miao, J., et al. (2019). Trimethylamine N-oxide binds and activates PERK to promote metabolic dysfunction. Cell Metab. 30, 1141–1151. doi: 10.1016/j.cmet.2019.08.021
Chen, J., Qin, Q., Yan, S., Yang, Y., Yan, H., Li, T., et al. (2021). Gut microbiome alterations in patients with carotid atherosclerosis. Front Cardiovasc Med 8:739093. doi: 10.3389/fcvm.2021.739093
Chen, M. L., Yi, L., Zhang, Y., Zhou, X., Ran, L., Yang, J., et al. (2016). Resveratrol attenuates trimethylamine-N-oxide (TMAO)-induced atherosclerosis by regulating TMAO synthesis and bile acid metabolism via remodeling of the gut microbiota. mBio 7, e02210–e02215. doi: 10.1128/mBio.02210-15
Chen, W., Zhang, S., Wu, J., Ye, T., Wang, S., Wang, P., et al. (2020b). Butyrate-producing bacteria and the gut-heart axis in atherosclerosis. Clin. Chim. Acta 507, 236–241. doi: 10.1016/j.cca.2020.04.037
Chen, W. G., Zheng, J. X., Xu, X., Hu, Y. M., and Ma, Y. M. (2018). Hippocampal FXR plays a role in the pathogenesis of depression: a preliminary study based on lentiviral gene modulation. Psychiatry Res. 264, 374–379. doi: 10.1016/j.psychres.2018.04.025
Cheng, S., Han, B., Ding, M., Wen, Y., Ma, M., Zhang, L., et al. (2020). Identifying psychiatric disorder-associated gut microbiota using microbiota-related gene set enrichment analysis. Brief. Bioinform. 21, 1016–1022. doi: 10.1093/bib/bbz034
Choroszy, M., Sobieszczańska, B., Litwinowicz, K., Łaczmański, Ł., Chmielarz, M., Walczuk, U., et al. (2022). Co-toxicity of endotoxin and Indoxyl sulfate, gut-derived bacterial metabolites, to vascular endothelial cells in coronary arterial disease accompanied by gut Dysbiosis. Nutrients 14:424. doi: 10.3390/nu14030424
Chrysohoou, C., Kollia, N., and Tousoulis, D. (2018). The link between depression and atherosclerosis through the pathways of inflammation and endothelium dysfunction. Maturitas 109, 1–5. doi: 10.1016/j.maturitas.2017.12.001
Ciernikova, S., Mego, M., and Chovanec, M. (2021). Exploring the potential role of the gut microbiome in chemotherapy-induced neurocognitive disorders and cardiovascular toxicity. Cancers (Basel) 13:782. doi: 10.3390/cancers13040782
Clarke, G., Grenham, S., Scully, P., Fitzgerald, P., Moloney, R. D., Shanahan, F., et al. (2013). The microbiome-gut-brain axis during early life regulates the hippocampal serotonergic system in a sex-dependent manner. Mol. Psychiatry 18, 666–673. doi: 10.1038/mp.2012.77
Collaborators, G.D.a.I.I.a.P (2017). Global, regional, and national incidence, prevalence, and years lived with disability for 328 diseases and injuries for 195 countries, 1990-2016: a systematic analysis for the global burden of disease study 2016. Lancet 390, 1211–1259. doi: 10.1016/s0140-6736(17)32154-2
Covington, H. E. 3rd, Maze, I., LaPlant, Q. C., Vialou, V. F., Ohnishi, Y. N., Berton, O., et al. (2009). Antidepressant actions of histone deacetylase inhibitors. J. Neurosci. 29, 11451–11460. doi: 10.1523/jneurosci.1758-09.2009
Crumeyrolle-Arias, M., Jaglin, M., Bruneau, A., Vancassel, S., Cardona, A., Daugé, V., et al. (2014). Absence of the gut microbiota enhances anxiety-like behavior and neuroendocrine response to acute stress in rats. Psychoneuroendocrinology 42, 207–217. doi: 10.1016/j.psyneuen.2014.01.014
Cryan, J. F., and Dinan, T. G. (2012). Mind-altering microorganisms: the impact of the gut microbiota on brain and behaviour. Nat. Rev. Neurosci. 13, 701–712. doi: 10.1038/nrn3346
Cryan, J. F., O'Riordan, K. J., Cowan, C. S. M., Sandhu, K. V., Bastiaanssen, T. F. S., Boehme, M., et al. (2019). The microbiota-gut-brain Axis. Physiol. Rev. 99, 1877–2013. doi: 10.1152/physrev.00018.2018
Dahlem, C., Kado, S. Y., He, Y., Bein, K., Wu, D., Haarmann-Stemmann, T., et al. (2020). AHR signaling interacting with nutritional factors regulating the expression of markers in vascular inflammation and Atherogenesis. Int. J. Mol. Sci. 21:287. doi: 10.3390/ijms21218287
Dalile, B., Van Oudenhove, L., Vervliet, B., and Verbeke, K. (2019). The role of short-chain fatty acids in microbiota-gut-brain communication. Nat. Rev. Gastroenterol. Hepatol. 16, 461–478. doi: 10.1038/s41575-019-0157-3
Dantzer, R., O'Connor, J. C., Freund, G. G., Johnson, R. W., and Kelley, K. W. (2008). From inflammation to sickness and depression: when the immune system subjugates the brain. Nat. Rev. Neurosci. 9, 46–56. doi: 10.1038/nrn2297
Deng, Y., Zhou, M., Wang, J., Yao, J., Yu, J., Liu, W., et al. (2021). Involvement of the microbiota-gut-brain axis in chronic restraint stress: disturbances of the kynurenine metabolic pathway in both the gut and brain. Gut Microbes 13, 1–16. doi: 10.1080/19490976.2020.1869501
Dibato, J. E., Montvida, O., Zaccardi, F., Sargeant, J. A., Davies, M. J., Khunti, K., et al. (2021). Association of Cardiometabolic Multimorbidity and Depression with Cardiovascular Events in early-onset adult type 2 diabetes: a multiethnic study in the U.S. Diabetes Care 44, 231–239. doi: 10.2337/dc20-2045
Doi, M., Takahashi, Y., Komatsu, R., Yamazaki, F., Yamada, H., Haraguchi, S., et al. (2010). Salt-sensitive hypertension in circadian clock-deficient cry-null mice involves dysregulated adrenal Hsd3b6. Nat. Med. 16, 67–74. doi: 10.1038/nm.2061
Dominguez-Rodriguez, A., Abreu-Gonzalez, P., Garcia-Gonzalez, M., Ferrer-Hita, J., Vargas, M., and Reiter, R. J. (2005). Elevated levels of oxidized low-density lipoprotein and impaired nocturnal synthesis of melatonin in patients with myocardial infarction. Atherosclerosis 180, 101–105. doi: 10.1016/j.atherosclerosis.2004.11.003
Dominguez-Rodriguez, A., Abreu-Gonzalez, P., Sanchez-Sanchez, J. J., Kaski, J. C., and Reiter, R. J. (2010). Melatonin and circadian biology in human cardiovascular disease. J. Pineal Res. 49, 14–22. doi: 10.1111/j.1600-079X.2010.00773.x
Dou, L., Bertrand, E., Cerini, C., Faure, V., Sampol, J., Vanholder, R., et al. (2004). The uremic solutes p-cresol and indoxyl sulfate inhibit endothelial proliferation and wound repair. Kidney Int. 65, 442–451. doi: 10.1111/j.1523-1755.2004.00399.x
Durack, J., and Lynch, S. V. (2019). The gut microbiome: relationships with disease and opportunities for therapy. J. Exp. Med. 216, 20–40. doi: 10.1084/jem.20180448
Erny, D., Hrabě de Angelis, A. L., Jaitin, D., Wieghofer, P., Staszewski, O., David, E., et al. (2015). Host microbiota constantly control maturation and function of microglia in the CNS. Nat. Neurosci. 18, 965–977. doi: 10.1038/nn.4030
Fitzgerald, K. A., and Kagan, J. C. (2020). Toll-like receptors and the control of immunity. Cells 180, 1044–1066. doi: 10.1016/j.cell.2020.02.041
Fukumoto, S., Tatewaki, M., Yamada, T., Fujimiya, M., Mantyh, C., Voss, M., et al. (2003). Short-chain fatty acids stimulate colonic transit via intraluminal 5-HT release in rats. Am. J. Physiol. Regul. Integr. Comp. Physiol. 284, R1269–R1276. doi: 10.1152/ajpregu.00442.2002
Fung, T. C., Olson, C. A., and Hsiao, E. Y. (2017). Interactions between the microbiota, immune and nervous systems in health and disease. Nat. Neurosci. 20, 145–155. doi: 10.1038/nn.4476
Gao, K., Mu, C.-L., Farzi, A., and Zhu, W.-Y. (2020). Tryptophan metabolism: a link between the gut microbiota and brain. Advances in nutrition (Bethesda, Md.) 11, 709–723. doi: 10.1093/advances/nmz127
Gareau, M. G., Wine, E., Rodrigues, D. M., Cho, J. H., Whary, M. T., Philpott, D. J., et al. (2011). Bacterial infection causes stress-induced memory dysfunction in mice. Gut 60, 307–317. doi: 10.1136/gut.2009.202515
Gold, P. W., Licinio, J., and Pavlatou, M. G. (2013). Pathological parainflammation and endoplasmic reticulum stress in depression: potential translational targets through the CNS insulin, klotho and PPAR-γ systems. Mol. Psychiatry 18, 154–165. doi: 10.1038/mp.2012.167
Golubeva, A. V., Joyce, S. A., Moloney, G., Burokas, A., Sherwin, E., Arboleya, S., et al. (2017). Microbiota-related changes in Bile Acid & Tryptophan Metabolism are associated with gastrointestinal dysfunction in a mouse model of autism. EBioMedicine 24, 166–178. doi: 10.1016/j.ebiom.2017.09.020
Gonçalves, S., Nunes-Costa, D., Cardoso, S. M., Empadinhas, N., and Marugg, J. D. (2022). Enzyme promiscuity in serotonin biosynthesis, from bacteria to plants and humans. Front. Microbiol. 13:873555. doi: 10.3389/fmicb.2022.873555
Grenham, S., Clarke, G., Cryan, J. F., and Dinan, T. G. (2011). Brain-gut-microbe communication in health and disease. Front. Physiol. 2:94. doi: 10.3389/fphys.2011.00094
Gu, H. F., Tang, C. K., and Yang, Y. Z. (2012). Psychological stress, immune response, and atherosclerosis. Atherosclerosis 223, 69–77. doi: 10.1016/j.atherosclerosis.2012.01.021
Gu, Y., Wang, X., Li, J., Zhang, Y., Zhong, H., Liu, R., et al. (2017). Analyses of gut microbiota and plasma bile acids enable stratification of patients for antidiabetic treatment. Nat. Commun. 8:1785. doi: 10.1038/s41467-017-01682-2
Guan, R., and Mariuzza, R. A. (2007). Peptidoglycan recognition proteins of the innate immune system. Trends Microbiol. 15, 127–134. doi: 10.1016/j.tim.2007.01.006
Haeusler, R. A., Astiarraga, B., Camastra, S., Accili, D., and Ferrannini, E. (2013). Human insulin resistance is associated with increased plasma levels of 12α-hydroxylated bile acids. Diabetes 62, 4184–4191. doi: 10.2337/db13-0639
Hare, D. L., Toukhsati, S. R., Johansson, P., and Jaarsma, T. (2014). Depression and cardiovascular disease: a clinical review. Eur. Heart J. 35, 1365–1372. doi: 10.1093/eurheartj/eht462
Hoyles, L., Snelling, T., Umlai, U. K., Nicholson, J. K., Carding, S. R., Glen, R. C., et al. (2018). Microbiome-host systems interactions: protective effects of propionate upon the blood-brain barrier. Microbiome 6:55. doi: 10.1186/s40168-018-0439-y
Hu, K., Liao, X. X., Wu, X. Y., Wang, R., Hu, Z. W., Liu, S. Y., et al. (2022). Effects of the lipid metabolites and the gut microbiota in ApoE(−/−) mice on atherosclerosis co-depression from the microbiota-gut-brain Axis. Front. Mol. Biosci. 9:786492. doi: 10.3389/fmolb.2022.786492
Huang, F., Pariante, C. M., and Borsini, A. (2022). From dried bear bile to molecular investigation: a systematic review of the effect of bile acids on cell apoptosis, oxidative stress and inflammation in the brain, across pre-clinical models of neurological, neurodegenerative and neuropsychiatric disorders. Brain Behav. Immun. 99, 132–146. doi: 10.1016/j.bbi.2021.09.021
Huang, C., Wang, J., Hu, W., Wang, C., Lu, X., Tong, L., et al. (2016). Identification of functional farnesoid X receptors in brain neurons. FEBS Lett. 590, 3233–3242. doi: 10.1002/1873-3468.12373
Huang, F., Wang, T., Lan, Y., Yang, L., Pan, W., Zhu, Y., et al. (2015). Deletion of mouse FXR gene disturbs multiple neurotransmitter systems and alters neurobehavior. Front. Behav. Neurosci. 9:70. doi: 10.3389/fnbeh.2015.00070
Huang, F., Zheng, X., Ma, X., Jiang, R., Zhou, W., Zhou, S., et al. (2019). Theabrownin from Pu-erh tea attenuates hypercholesterolemia via modulation of gut microbiota and bile acid metabolism. Nat. Commun. 10:4971. doi: 10.1038/s41467-019-12896-x
Hung, S. C., Kuo, K. L., Huang, H. L., Lin, C. C., Tsai, T. H., Wang, C. H., et al. (2016). Indoxyl sulfate suppresses endothelial progenitor cell-mediated neovascularization. Kidney Int. 89, 574–585. doi: 10.1016/j.kint.2015.11.020
Hwu, P., Du, M. X., Lapointe, R., Do, M., Taylor, M. W., and Young, H. A. (2000). Indoleamine 2,3-dioxygenase production by human dendritic cells results in the inhibition of T cell proliferation. Journal of immunology 164, 3596–3599.
Jäckel, S., Kiouptsi, K., Lillich, M., Hendrikx, T., Khandagale, A., Kollar, B., et al. (2017). Gut microbiota regulate hepatic von Willebrand factor synthesis and arterial thrombus formation via toll-like receptor-2. Blood 130, 542–553. doi: 10.1182/blood-2016-11-754416
Jain, V., Al Rifai, M., Turpin, R., Eken, H. N., Agrawal, A., Mahtta, D., et al. (2022). Evaluation of factors underlying sex-based disparities in cardiovascular Care in Adults with Self-reported Premature Atherosclerotic Cardiovascular Disease. JAMA Cardiol. 7, 341–345. doi: 10.1001/jamacardio.2021.5430
Jee, Y. H., Chang, H., Jung, K. J., and Jee, S. H. (2019). Cohort study on the effects of depression on atherosclerotic cardiovascular disease risk in Korea. BMJ Open 9:e026913. doi: 10.1136/bmjopen-2018-026913
Jia, H. M., Li, Q., Zhou, C., Yu, M., Yang, Y., Zhang, H. W., et al. (2016). Chronic unpredictive mild stress leads to altered hepatic metabolic profile and gene expression. Sci. Rep. 6:23441. doi: 10.1038/srep23441
Jiang, H., Ling, Z., Zhang, Y., Mao, H., Ma, Z., Yin, Y., et al. (2015). Altered fecal microbiota composition in patients with major depressive disorder. Brain Behav. Immun. 48, 186–194. doi: 10.1016/j.bbi.2015.03.016
Jie, Z., Xia, H., Zhong, S.-L., Feng, Q., Li, S., Liang, S., et al. (2017). The gut microbiome in atherosclerotic cardiovascular disease. Nat. Commun. 8:845. doi: 10.1038/s41467-017-00900-1
Jonsson, A. L., and Bäckhed, F. (2017). Role of gut microbiota in atherosclerosis. Nat. Rev. Cardiol. 14, 79–87. doi: 10.1038/nrcardio.2016.183
Kallio, K. A., Hätönen, K. A., Lehto, M., Salomaa, V., Männistö, S., and Pussinen, P. J. (2015). Endotoxemia, nutrition, and cardiometabolic disorders. Acta Diabetol. 52, 395–404. doi: 10.1007/s00592-014-0662-3
Kappel, B. A., De Angelis, L., Heiser, M., Ballanti, M., Stoehr, R., Goettsch, C., et al. (2020). Cross-omics analysis revealed gut microbiome-related metabolic pathways underlying atherosclerosis development after antibiotics treatment. Mol Metab 36:100976. doi: 10.1016/j.molmet.2020.100976
Karlsson, F. H., Fåk, F., Nookaew, I., Tremaroli, V., Fagerberg, B., Petranovic, D., et al. (2012). Symptomatic atherosclerosis is associated with an altered gut metagenome. Nat. Commun. 3:1245. doi: 10.1038/ncomms2266
Kasahara, K., Krautkramer, K. A., Org, E., Romano, K. A., Kerby, R. L., Vivas, E. I., et al. (2018). Interactions between Roseburia intestinalis and diet modulate atherogenesis in a murine model. Nat. Microbiol. 3, 1461–1471. doi: 10.1038/s41564-018-0272-x
Keene, C. D., Rodrigues, C. M., Eich, T., Linehan-Stieers, C., Abt, A., Kren, B. T., et al. (2001). A bile acid protects against motor and cognitive deficits and reduces striatal degeneration in the 3-nitropropionic acid model of Huntington's disease. Exp. Neurol. 171, 351–360. doi: 10.1006/exnr.2001.7755
Keitel, V., Görg, B., Bidmon, H. J., Zemtsova, I., Spomer, L., Zilles, K., et al. (2010). The bile acid receptor TGR5 (Gpbar-1) acts as a neurosteroid receptor in brain. Glia 58, 1794–1805. doi: 10.1002/glia.21049
Kelly, J. R., Borre, Y., C, O. B., Patterson, E., El Aidy, S., Deane, J., et al. (2016). Transferring the blues: depression-associated gut microbiota induces neurobehavioural changes in the rat. J. Psychiatr. Res. 82, 109–118. doi: 10.1016/j.jpsychires.2016.07.019
Kemp, K. M., Colson, J., Lorenz, R. G., Maynard, C. L., and Pollock, J. S. (2021). Early life stress in mice alters gut microbiota independent of maternal microbiota inheritance. Am. J. Physiol. Regul. Integr. Comp. Physiol. 320, R663–r674. doi: 10.1152/ajpregu.00072.2020
Kennedy, P. J., Cryan, J. F., Dinan, T. G., and Clarke, G. (2017). Kynurenine pathway metabolism and the microbiota-gut-brain axis. Neuropharmacology 112, 399–412. doi: 10.1016/j.neuropharm.2016.07.002
Kiouptsi, K., Jäckel, S., Pontarollo, G., Grill, A., Kuijpers, M. J. E., Wilms, E., et al. (2019). The microbiota promotes arterial thrombosis in low-density lipoprotein receptor-deficient mice. mBio 10:298. doi: 10.1128/mBio.02298-19
Kiouptsi, K., Jäckel, S., Wilms, E., Pontarollo, G., Winterstein, J., Karwot, C., et al. (2020). The commensal microbiota enhances ADP-triggered integrin α(IIb)β(3) activation and von Willebrand factor-mediated platelet deposition to type I collagen. Int. J. Mol. Sci. 21:7171. doi: 10.3390/ijms21197171
Koeth, R. A., Wang, Z., Levison, B. S., Buffa, J. A., Org, E., Sheehy, B. T., et al. (2013). Intestinal microbiota metabolism of L-carnitine, a nutrient in red meat, promotes atherosclerosis. Nat. Med. 19, 576–585. doi: 10.1038/nm.3145
Koh, A., De Vadder, F., Kovatcheva-Datchary, P., and Bäckhed, F. (2016). From dietary fiber to host physiology: short-chain fatty acids as key bacterial metabolites. Cells 165, 1332–1345. doi: 10.1016/j.cell.2016.05.041
Komaroff, A. L. (2018). The microbiome and risk for atherosclerosis. JAMA 319, 2381–2382. doi: 10.1001/jama.2018.5240
Konopelski, P., Chabowski, D., Aleksandrowicz, M., Kozniewska, E., Podsadni, P., Szczepanska, A., et al. (2021). Indole-3-propionic acid, a tryptophan-derived bacterial metabolite, increases blood pressure via cardiac and vascular mechanisms in rats. Am. J. Physiol. Regul. Integr. Comp. Physiol. 321, R969–r981. doi: 10.1152/ajpregu.00142.2021
Koziróg, M., Poliwczak, A. R., Duchnowicz, P., Koter-Michalak, M., Sikora, J., and Broncel, M. (2011). Melatonin treatment improves blood pressure, lipid profile, and parameters of oxidative stress in patients with metabolic syndrome. J. Pineal Res. 50, 261–266. doi: 10.1111/j.1600-079X.2010.00835.x
Ladwig, K. H., Baumert, J., Marten-Mittag, B., Lukaschek, K., Johar, H., Fang, X., et al. (2017). Room for depressed and exhausted mood as a risk predictor for all-cause and cardiovascular mortality beyond the contribution of the classical somatic risk factors in men. Atherosclerosis 257, 224–231. doi: 10.1016/j.atherosclerosis.2016.12.003
Lai, W. T., Deng, W. F., Xu, S. X., Zhao, J., Xu, D., Liu, Y. H., et al. (2021). Shotgun metagenomics reveals both taxonomic and tryptophan pathway differences of gut microbiota in major depressive disorder patients. Psychol. Med. 51, 90–101. doi: 10.1017/s0033291719003027
Le Roy, T., Lécuyer, E., Chassaing, B., Rhimi, M., Lhomme, M., Boudebbouze, S., et al. (2019). The intestinal microbiota regulates host cholesterol homeostasis. BMC Biol. 17:94. doi: 10.1186/s12915-019-0715-8
Lee, J.-H., and Lee, J. (2010). Indole as an intercellular signal in microbial communities. FEMS Microbiol. Rev. 34, 426–444. doi: 10.1111/j.1574-6976.2009.00204.x
Lehr, H. A., Sagban, T. A., Ihling, C., Zähringer, U., Hungerer, K. D., Blumrich, M., et al. (2001). Immunopathogenesis of atherosclerosis: endotoxin accelerates atherosclerosis in rabbits on hypercholesterolemic diet. Circulation 104, 914–920. doi: 10.1161/hc3401.093153
Lestage, J., Verrier, D., Palin, K., and Dantzer, R. (2002). The enzyme indoleamine 2,3-dioxygenase is induced in the mouse brain in response to peripheral administration of lipopolysaccharide and superantigen. Brain Behav. Immun. 16, 596–601. doi: 10.1016/S0889-1591(02)00014-4
Levine, G. N., Cohen, B. E., Commodore-Mensah, Y., Fleury, J., Huffman, J. C., Khalid, U., et al. (2021). Psychological health, well-being, and the mind-heart-body connection: a scientific statement from the American Heart Association. Circulation 143, e763–e783. doi: 10.1161/CIR.0000000000000947
Li, W., Ali, T., Zheng, C., He, K., Liu, Z., Shah, F. A., et al. (2022b). Anti-depressive-like behaviors of APN KO mice involve Trkb/BDNF signaling related neuroinflammatory changes. Mol. Psychiatry 27, 1047–1058. doi: 10.1038/s41380-021-01327-3
Li, J., Hou, L., Wang, C., Jia, X., Qin, X., and Wu, C. (2018b). Short term Intrarectal Administration of Sodium Propionate Induces Antidepressant-like Effects in rats exposed to chronic unpredictable mild stress. Front. Psych. 9:454. doi: 10.3389/fpsyt.2018.00454
Li, D., Ke, Y., Zhan, R., Liu, C., Zhao, M., Zeng, A., et al. (2018a). Trimethylamine-N-oxide promotes brain aging and cognitive impairment in mice. Aging Cell 17:e12768. doi: 10.1111/acel.12768
Li, D., Liu, R., Wang, M., Peng, R., Fu, S., Fu, A., et al. (2022a). 3β-Hydroxysteroid dehydrogenase expressed by gut microbes degrades testosterone and is linked to depression in males. Cell Host Microbe 30, 329–339.e5. doi: 10.1016/j.chom.2022.01.001
Li, X., Su, C., Jiang, Z., Yang, Y., Zhang, Y., Yang, M., et al. (2021). Berberine attenuates choline-induced atherosclerosis by inhibiting trimethylamine and trimethylamine-N-oxide production via manipulating the gut microbiome. NPJ Biofilms Microbiomes 7:36. doi: 10.1038/s41522-021-00205-8
Li, M., van Esch, B., Henricks, P. A. J., Folkerts, G., and Garssen, J. (2018c). The anti-inflammatory effects of short chain fatty acids on lipopolysaccharide- or tumor necrosis factor α-stimulated endothelial cells via activation of GPR41/43 and inhibition of HDACs. Front. Pharmacol. 9:533. doi: 10.3389/fphar.2018.00533
Li, M., van Esch, B., Henricks, P. A. J., Garssen, J., and Folkerts, G. (2018d). Time and concentration dependent effects of short chain fatty acids on lipopolysaccharide- or tumor necrosis factor α-induced endothelial activation. Front. Pharmacol. 9:233. doi: 10.3389/fphar.2018.00233
Li, N., Wang, Q., Wang, Y., Sun, A., Lin, Y., Jin, Y., et al. (2019). Fecal microbiota transplantation from chronic unpredictable mild stress mice donors affects anxiety-like and depression-like behavior in recipient mice via the gut microbiota-inflammation-brain axis. Stress 22, 592–602. doi: 10.1080/10253890.2019.1617267
Lim, C. K., Fernández-Gomez, F. J., Braidy, N., Estrada, C., Costa, C., Costa, S., et al. (2017). Involvement of the kynurenine pathway in the pathogenesis of Parkinson's disease. Prog. Neurobiol. 155, 76–95. doi: 10.1016/j.pneurobio.2015.12.009
Lindskog Jonsson, A., Caesar, R., Akrami, R., Reinhardt, C., Fåk Hållenius, F., Borén, J., et al. (2018). Impact of gut microbiota and diet on the development of atherosclerosis in Apoe(−/−) mice. Arterioscler. Thromb. Vasc. Biol. 38, 2318–2326. doi: 10.1161/atvbaha.118.311233
Liu, H., Chen, X., Hu, X., Niu, H., Tian, R., Wang, H., et al. (2019). Alterations in the gut microbiome and metabolism with coronary artery disease severity. Microbiome 7:68. doi: 10.1186/s40168-019-0683-9
Liu, C. C., Wu, Y. F., Feng, G. M., Gao, X. X., Zhou, Y. Z., Hou, W. J., et al. (2015). Plasma-metabolite-biomarkers for the therapeutic response in depressed patients by the traditional Chinese medicine formula Xiaoyaosan: a (1)H NMR-based metabolomics approach. J. Affect. Disord. 185, 156–163. doi: 10.1016/j.jad.2015.05.005
Liu, S., Zhao, W., Liu, X., and Cheng, L. (2020). Metagenomic analysis of the gut microbiome in atherosclerosis patients identify cross-cohort microbial signatures and potential therapeutic target. FASEB J. 34, 14166–14181. doi: 10.1096/fj.202000622R
MahmoudianDehkordi, S., Arnold, M., Nho, K., Ahmad, S., Jia, W., Xie, G., et al. (2019). Altered bile acid profile associates with cognitive impairment in Alzheimer's disease-an emerging role for gut microbiome. Alzheimers Dement. 15, 76–92. doi: 10.1016/j.jalz.2018.07.217
Makishima, M., Lu, T. T., Xie, W., Whitfield, G. K., Domoto, H., Evans, R. M., et al. (2002). Vitamin D receptor as an intestinal bile acid sensor. Science (New York, N.Y.) 296, 1313–1316. doi: 10.1126/science.1070477
Martin-Gallausiaux, C., Larraufie, P., Jarry, A., Béguet-Crespel, F., Marinelli, L., Ledue, F., et al. (2018). Butyrate produced by commensal bacteria Down-regulates () expression a dual mechanism in human intestinal epithelial cells. Front. Immunol. 9:2838. doi: 10.3389/fimmu.2018.02838
McMillin, M., Frampton, G., Quinn, M., Ashfaq, S., de los Santos, M. 3rd, Grant, S., et al. (2016). Bile acid signaling is involved in the neurological decline in a murine model of acute liver failure. Am. J. Pathol. 186, 312–323. doi: 10.1016/j.ajpath.2015.10.005
Meier, T. B., Drevets, W. C., Wurfel, B. E., Ford, B. N., Morris, H. M., Victor, T. A., et al. (2016). Relationship between neurotoxic kynurenine metabolites and reductions in right medial prefrontal cortical thickness in major depressive disorder. Brain Behav. Immun. 53, 39–48. doi: 10.1016/j.bbi.2015.11.003
Meinitzer, S., Baranyi, A., Holasek, S., Schnedl, W. J., Zelzer, S., Mangge, H., et al. (2020). Sex-specific associations of trimethylamine-N-oxide and Zonulin with signs of depression in carbohydrate Malabsorbers and Nonmalabsorbers. Dis. Markers 2020:7897240. doi: 10.1155/2020/7897240
Mertens, K. L., Kalsbeek, A., Soeters, M. R., and Eggink, H. M. (2017). Bile acid signaling pathways from the enterohepatic circulation to the central nervous system. Front. Neurosci. 11:617. doi: 10.3389/fnins.2017.00617
Messaoud, A., Mensi, R., Douki, W., Neffati, F., Najjar, M. F., Gobbi, G., et al. (2019). Reduced peripheral availability of tryptophan and increased activation of the kynurenine pathway and cortisol correlate with major depression and suicide. The world journal of biological psychiatry: the official journal of the World Federation of Societies of Biological Psychiatry 20, 703–711. doi: 10.1080/15622975.2018.1468031
Morton, G. J., Matsen, M. E., Bracy, D. P., Meek, T. H., Nguyen, H. T., Stefanovski, D., et al. (2013). FGF19 action in the brain induces insulin-independent glucose lowering. J. Clin. Invest. 123, 4799–4808. doi: 10.1172/jci70710
Müller, N., and Schwarz, M. J. (2007). The immune-mediated alteration of serotonin and glutamate: towards an integrated view of depression. Mol. Psychiatry 12, 988–1000. doi: 10.1038/sj.mp.4002006
Münzel, T., and Daiber, A. (2020). Novel concept for the regulation of eNOS (endothelial nitric oxide synthase) activity: inhibitory effects of the enigma homolog protein and the PHLPP (Pleckstrin homology domain and leucine-rich repeat protein phosphatase)-2 on Akt (protein kinase B)-dependent nitric oxide synthase activation. Arterioscler. Thromb. Vasc. Biol. 40, 1608–1610. doi: 10.1161/atvbaha.120.314474
Nie, K., Ma, K., Luo, W., Shen, Z., Yang, Z., Xiao, M., et al. (2021). Roseburia intestinalis: a beneficial gut organism from the discoveries in genus and species. Front. Cell. Infect. Microbiol. 11:757718. doi: 10.3389/fcimb.2021.757718
O'Connor, C. M. (2018). Depression in heart failure. Beyond a SADHART 6, 885–886. doi: 10.1016/j.jchf.2018.08.004
Ogyu, K., Kubo, K., Noda, Y., Iwata, Y., Tsugawa, S., Omura, Y., et al. (2018). Kynurenine pathway in depression: a systematic review and meta-analysis. Neurosci. Biobehav. Rev. 90, 16–25. doi: 10.1016/j.neubiorev.2018.03.023
Oleskin, A. V., and Shenderov, B. A. (2016). Neuromodulatory effects and targets of the SCFAs and gasotransmitters produced by the human symbiotic microbiota. Microb. Ecol. Health Dis. 27:30971. doi: 10.3402/mehd.v27.30971
O'Mahony, S. M., Clarke, G., Borre, Y. E., Dinan, T. G., and Cryan, J. F. (2015). Serotonin, tryptophan metabolism and the brain-gut-microbiome axis. Behav. Brain Res. 277, 32–48. doi: 10.1016/j.bbr.2014.07.027
Paeslack, N., Mimmler, M., Becker, S., Gao, Z., Khuu, M. P., Mann, A., et al. (2022). Microbiota-derived tryptophan metabolites in vascular inflammation and cardiovascular disease. Amino Acids. doi: 10.1007/s00726-022-03161-5 [Epub ahead of print]
Paone, P., and Cani, P. D. (2020). Mucus barrier, mucins and gut microbiota: the expected slimy partners? Gut 69, 2232–2243. doi: 10.1136/gutjnl-2020-322260
Peregrin, A. T., Ahlman, H., Jodal, M., and Lundgren, O. (1999). Involvement of serotonin and calcium channels in the intestinal fluid secretion evoked by bile salt and cholera toxin. Br. J. Pharmacol. 127, 887–894. doi: 10.1038/sj.bjp.0702615
Pierozan, P., Biasibetti, H., Schmitz, F., Ávila, H., Parisi, M. M., Barbe-Tuana, F., et al. (2016). Quinolinic acid neurotoxicity: differential roles of astrocytes and microglia via FGF-2-mediated signaling in redox-linked cytoskeletal changes. Biochim. Biophys. Acta 1863, 3001–3014. doi: 10.1016/j.bbamcr.2016.09.014
Pols, T. W. H., Nomura, M., Harach, T., Lo Sasso, G., Oosterveer, M. H., Thomas, C., et al. (2011). TGR5 activation inhibits atherosclerosis by reducing macrophage inflammation and lipid loading. Cell Metab. 14, 747–757. doi: 10.1016/j.cmet.2011.11.006
Polyzos, K. A., Ovchinnikova, O., Berg, M., Baumgartner, R., Agardh, H., Pirault, J., et al. (2015). Inhibition of indoleamine 2,3-dioxygenase promotes vascular inflammation and increases atherosclerosis in Apoe−/− mice. Cardiovasc. Res. 106, 295–302. doi: 10.1093/cvr/cvv100
Qin, Y., Havulinna, A. S., Liu, Y., Jousilahti, P., Ritchie, S. C., Tokolyi, A., et al. (2022). Combined effects of host genetics and diet on human gut microbiota and incident disease in a single population cohort. Nat. Genet. 54, 134–142. doi: 10.1038/s41588-021-00991-z
Qu, Y., Su, C., Zhao, Q., Shi, A., Zhao, F., Tang, L., et al. (2022). Gut microbiota-mediated elevated production of secondary bile acids in chronic unpredictable mild stress. Front. Pharmacol. 13:837543. doi: 10.3389/fphar.2022.837543
Ramage, A. G., and Villalón, C. M. (2008). 5-hydroxytryptamine and cardiovascular regulation. Trends Pharmacol. Sci. 29, 472–481. doi: 10.1016/j.tips.2008.06.009
Ramana, K. V., Willis, M. S., White, M. D., Horton, J. W., DiMaio, J. M., Srivastava, D., et al. (2006). Endotoxin-induced cardiomyopathy and systemic inflammation in mice is prevented by aldose reductase inhibition. Circulation 114, 1838–1846. doi: 10.1161/circulationaha.106.630830
Rami, M., Guillamat-Prats, R., Rinne, P., Salvermoser, M., Ring, L., Bianchini, M., et al. (2018). Chronic intake of the selective serotonin reuptake inhibitor fluoxetine enhances atherosclerosis. Arterioscler. Thromb. Vasc. Biol. 38, 1007–1019. doi: 10.1161/atvbaha.117.310536
Reigstad, C. S., Salmonson, C. E., Rainey, J. F., Szurszewski, J. H., Linden, D. R., Sonnenburg, J. L., et al. (2015). Gut microbes promote colonic serotonin production through an effect of short-chain fatty acids on enterochromaffin cells. FASEB J. 29, 1395–1403. doi: 10.1096/fj.14-259598
Reininghaus, E. Z., Platzer, M., Kohlhammer-Dohr, A., Hamm, C., Mörkl, S., Bengesser, S. A., et al. (2020). PROVIT: supplementary probiotic treatment and vitamin B7 in depression-a randomized controlled trial. Nutrients 12:422. doi: 10.3390/nu12113422
Ridlon, J. M., Harris, S. C., Bhowmik, S., Kang, D.-J., and Hylemon, P. B. (2016). Consequences of bile salt biotransformations by intestinal bacteria. Gut Microbes 7, 22–39. doi: 10.1080/19490976.2015.1127483
Rieder, R., Wisniewski, P. J., Alderman, B. L., and Campbell, S. C. (2017). Microbes and mental health: a review. Brain Behav. Immun. 66, 9–17. doi: 10.1016/j.bbi.2017.01.016
Roberts, A. B., Gu, X., Buffa, J. A., Hurd, A. G., Wang, Z., Zhu, W., et al. (2018). Development of a gut microbe-targeted nonlethal therapeutic to inhibit thrombosis potential. Nat. Med. 24, 1407–1417. doi: 10.1038/s41591-018-0128-1
Rohatgi, A., Ayers, C. R., Khera, A., McGuire, D. K., Das, S. R., Matulevicius, S., et al. (2009). The association between peptidoglycan recognition protein-1 and coronary and peripheral atherosclerosis: observations from the Dallas heart study. Atherosclerosis 203, 569–575. doi: 10.1016/j.atherosclerosis.2008.07.015
Russell, D. W. (2003). The enzymes, regulation, and genetics of bile acid synthesis. Annu. Rev. Biochem. 72, 137–174. doi: 10.1146/annurev.biochem.72.121801.161712
Sarkar, A., Chachra, P., Kennedy, P., Pena, C. J., Desouza, L. A., Nestler, E. J., et al. (2014). Hippocampal HDAC4 contributes to postnatal fluoxetine-evoked depression-like behavior. Neuropsychopharmacology 39, 2221–2232. doi: 10.1038/npp.2014.73
Sayin, S. I., Wahlström, A., Felin, J., Jäntti, S., Marschall, H. U., Bamberg, K., et al. (2013). Gut microbiota regulates bile acid metabolism by reducing the levels of tauro-beta-muricholic acid, a naturally occurring FXR antagonist. Cell Metab. 17, 225–235. doi: 10.1016/j.cmet.2013.01.003
Schins, A., Honig, A., Crijns, H., Baur, L., and Hamulyák, K. (2003). Increased coronary events in depressed cardiovascular patients: 5-HT2A receptor as missing link? Psychosom. Med. 65, 729–737. doi: 10.1097/01.PSY.0000088596.42029.10
Schwarcz, R., Bruno, J. P., Muchowski, P. J., and Wu, H.-Q. (2012). Kynurenines in the mammalian brain: when physiology meets pathology. Nat. Rev. Neurosci. 13, 465–477. doi: 10.1038/nrn3257
Sherwin, E., Bordenstein, S. R., Quinn, J. L., Dinan, T. G., and Cryan, J. F. (2019). Microbiota and the social brain. Science (New York, N.Y.) 366:2016. doi: 10.1126/science.aar2016
Shi, X., Huang, H., Zhou, M., Liu, Y., Wu, H., and Dai, M. (2021). Paeonol attenuated vascular fibrosis through regulating Treg/Th17 balance in a gut microbiota-dependent manner. Front. Pharmacol. 12:765482. doi: 10.3389/fphar.2021.765482
Simpson, C. A., Diaz-Arteche, C., Eliby, D., Schwartz, O. S., Simmons, J. G., and Cowan, C. S. M. (2021). The gut microbiota in anxiety and depression - a systematic review. Clin. Psychol. Rev. 83:101943. doi: 10.1016/j.cpr.2020.101943
Sjögren, K., Engdahl, C., Henning, P., Lerner, U. H., Tremaroli, V., Lagerquist, M. K., et al. (2012). The gut microbiota regulates bone mass in mice. J. Bone Miner. Res. 27, 1357–1367. doi: 10.1002/jbmr.1588
Skonieczna-Żydecka, K., Grochans, E., Maciejewska, D., Szkup, M., Schneider-Matyka, D., Jurczak, A., et al. (2018). Faecal short chain fatty acids profile is changed in polish depressive women. Nutrients 10:939. doi: 10.3390/nu10121939
Soliman, M. L., and Rosenberger, T. A. (2011). Acetate supplementation increases brain histone acetylation and inhibits histone deacetylase activity and expression. Mol. Cell. Biochem. 352, 173–180. doi: 10.1007/s11010-011-0751-3
Somayaji, M., Cataldi, S., Choi, S. J., Edwards, R. H., Mosharov, E. V., and Sulzer, D. (2020). A dual role for α-synuclein in facilitation and depression of dopamine release from substantia nigra neurons in vivo. Proc. Natl. Acad. Sci. U. S. A. 117, 32701–32710. doi: 10.1073/pnas.2013652117
Song, P., Ramprasath, T., Wang, H., and Zou, M. H. (2017). Abnormal kynurenine pathway of tryptophan catabolism in cardiovascular diseases. Cell. Mol. Life Sci. 74, 2899–2916. doi: 10.1007/s00018-017-2504-2
Spichak, S., Bastiaanssen, T. F. S., Berding, K., Vlckova, K., Clarke, G., Dinan, T. G., et al. (2021). Mining microbes for mental health: determining the role of microbial metabolic pathways in human brain health and disease. Neurosci. Biobehav. Rev. 125, 698–761. doi: 10.1016/j.neubiorev.2021.02.044
Stepankova, R., Tonar, Z., Bartova, J., Nedorost, L., Rossman, P., Poledne, R., et al. (2010). Absence of microbiota (germ-free conditions) accelerates the atherosclerosis in ApoE-deficient mice fed standard low cholesterol diet. J. Atheroscler. Thromb. 17, 796–804. doi: 10.5551/jat.3285
Strandwitz, P., Kim, K. H., Terekhova, D., Liu, J. K., Sharma, A., Levering, J., et al. (2019). GABA-modulating bacteria of the human gut microbiota. Nat. Microbiol. 4, 396–403. doi: 10.1038/s41564-018-0307-3
Sun, B., Ma, T., Li, Y., Yang, N., Li, B., Zhou, X., et al. (2022). Bifidobacterium lactis Probio-M8 adjuvant treatment confers added benefits to patients with coronary artery disease via target modulation of the gut-heart/-brain axes. mSystems 7:e0010022. doi: 10.1128/msystems.00100-22
Sun, G. Z., Ye, N., Wu, S. J., Zhou, Y., and Sun, Y. X. (2019). 10-year ASCVD risk is positively correlated with depressive symptoms in a large general population. BMC Psychiatry 19:125. doi: 10.1186/s12888-019-2114-7
Tang, W. H., Wang, Z., Levison, B. S., Koeth, R. A., Britt, E. B., Fu, X., et al. (2013). Intestinal microbial metabolism of phosphatidylcholine and cardiovascular risk. N. Engl. J. Med. 368, 1575–1584. doi: 10.1056/NEJMoa1109400
Taylor, M. W., and Feng, G. S. (1991). Relationship between interferon-gamma, indoleamine 2,3-dioxygenase, and tryptophan catabolism. FASEB J. 5, 2516–2522. doi: 10.1096/fasebj.5.11.1907934
Tengattini, S., Reiter, R. J., Tan, D.-X., Terron, M. P., Rodella, L. F., and Rezzani, R. (2008). Cardiovascular diseases: protective effects of melatonin. J. Pineal Res. 44, 16–25. doi: 10.1111/j.1600-079X.2007.00518.x
Thomas, C., Pellicciari, R., Pruzanski, M., Auwerx, J., and Schoonjans, K. (2008). Targeting bile-acid signalling for metabolic diseases. Nat. Rev. Drug Discov. 7, 678–693. doi: 10.1038/nrd2619
Tomlinson, E., Fu, L., John, L., Hultgren, B., Huang, X., Renz, M., et al. (2002). Transgenic mice expressing human fibroblast growth factor-19 display increased metabolic rate and decreased adiposity. Endocrinology 143, 1741–1747. doi: 10.1210/endo.143.5.8850
Tran, L., and Greenwood-Van Meerveld, B. (2013). Age-associated remodeling of the intestinal epithelial barrier. J. Gerontol. A Biol. Sci. Med. Sci. 68, 1045–1056. doi: 10.1093/gerona/glt106
Tunjungputri, R. N., van der Ven, A. J., Riksen, N., Rongen, G., Tacke, S., van den Berg, T. N., et al. (2015). Differential effects of platelets and platelet inhibition by ticagrelor on TLR2- and TLR4-mediated inflammatory responses. Thromb. Haemost. 113, 1035–1045. doi: 10.1160/th14-07-0579
Vaccarino, V., Badimon, L., Bremner, J. D., Cenko, E., Cubedo, J., Dorobantu, M., et al. (2020). Depression and coronary heart disease: 2018 position paper of the ESC working group on coronary pathophysiology and microcirculation. Eur. Heart J. 41, 1687–1696. doi: 10.1093/eurheartj/ehy913
van de Wouw, M., Boehme, M., Lyte, J. M., Wiley, N., Strain, C., O'Sullivan, O., et al. (2018). Short-chain fatty acids: microbial metabolites that alleviate stress-induced brain-gut axis alterations. J. Physiol. 596, 4923–4944. doi: 10.1113/jp276431
van Dooren, F. E., Schram, M. T., Schalkwijk, C. G., Stehouwer, C. D., Henry, R. M., Dagnelie, P. C., et al. (2016). Associations of low grade inflammation and endothelial dysfunction with depression - the Maastricht study. Brain Behav. Immun. 56, 390–396. doi: 10.1016/j.bbi.2016.03.004
Vijay, N., and Morris, M. E. (2014). Role of monocarboxylate transporters in drug delivery to the brain. Curr. Pharm. Des. 20, 1487–1498. doi: 10.2174/13816128113199990462
Vikenes, K., Farstad, M., and Nordrehaug, J. E. (1999). Serotonin is associated with coronary artery disease and cardiac events. Circulation 100, 483–489. doi: 10.1161/01.cir.100.5.483
Violi, F., Cammisotto, V., Bartimoccia, S., Pignatelli, P., Carnevale, R., and Nocella, C. (2022). Gut-derived low-grade endotoxaemia, atherothrombosis and cardiovascular disease. Nat. Rev. Cardiol. 1-14:737. doi: 10.1038/s41569-022-00737-2
Violi, F., Lip, G. Y., and Cangemi, R. (2016). Endotoxemia as a trigger of thrombosis in cirrhosis. Haematologica 101, e162–e163. doi: 10.3324/haematol.2015.139972
Vourakis, M., Mayer, G., and Rousseau, G. (2021). The role of gut microbiota on cholesterol metabolism in atherosclerosis. Int. J. Mol. Sci. 22:8074. doi: 10.3390/ijms22158074
Wahlström, A., Sayin, S. I., Marschall, H.-U., and Bäckhed, F. (2016). Intestinal crosstalk between bile acids and microbiota and its impact on host metabolism. Cell Metab. 24, 41–50. doi: 10.1016/j.cmet.2016.05.005
Walker, A. K., Wing, E. E., Banks, W. A., and Dantzer, R. (2019). Leucine competes with kynurenine for blood-to-brain transport and prevents lipopolysaccharide-induced depression-like behavior in mice. Mol. Psychiatry 24, 1523–1532. doi: 10.1038/s41380-018-0076-7
Wang, Z., Klipfell, E., Bennett, B. J., Koeth, R., Levison, B. S., Dugar, B., et al. (2011). Gut flora metabolism of phosphatidylcholine promotes cardiovascular disease. Nature 472, 57–63. doi: 10.1038/nature09922
Wang, F., Liang, S., Hu, J., and Xu, Y. (2020). Aryl hydrocarbon receptor connects dysregulated immune cells to atherosclerosis. Immunol. Lett. 228, 55–63. doi: 10.1016/j.imlet.2020.10.003
Watanabe, M., Houten, S. M., Mataki, C., Christoffolete, M. A., Kim, B. W., Sato, H., et al. (2006). Bile acids induce energy expenditure by promoting intracellular thyroid hormone activation. Nature 439, 484–489. doi: 10.1038/nature04330
WHO. (2008). The global burden of disease: 2004 update. Available at https://apps.who.int/iris/handle/10665/43942?locale-attribute=en&
Witkowski, M., Weeks, T. L., and Hazen, S. L. (2020). Gut microbiota and cardiovascular disease. Circ. Res. 127, 553–570. doi: 10.1161/circresaha.120.316242
Wu, D., Nishimura, N., Kuo, V., Fiehn, O., Shahbaz, S., Van Winkle, L., et al. (2011). Activation of aryl hydrocarbon receptor induces vascular inflammation and promotes atherosclerosis in apolipoprotein E−/− mice. Arterioscler. Thromb. Vasc. Biol. 31, 1260–1267. doi: 10.1161/atvbaha.110.220202
Wu, Y., Zhu, B., Chen, Z., Duan, J., Luo, A., Yang, L., et al. (2021). New insights into the comorbidity of coronary heart disease and depression. Curr. Probl. Cardiol. 46:100413. doi: 10.1016/j.cpcardiol.2019.03.002
Xiang, C., Wang, Y., Zhang, H., and Han, F. (2017). The role of endoplasmic reticulum stress in neurodegenerative disease. Apoptosis 22, 1–26. doi: 10.1007/s10495-016-1296-4
Xu, Y., Li, Y., Jadhav, K., Pan, X., Zhu, Y., Hu, S., et al. (2021). Hepatocyte ATF3 protects against atherosclerosis by regulating HDL and bile acid metabolism. Nat. Metab. 3, 59–74. doi: 10.1038/s42255-020-00331-1
Yang, D., Lyu, W., Hu, Z., Gao, J., Zheng, Z., Wang, W., et al. (2021). Probiotic effects of lactobacillus fermentum ZJUIDS06 and lactobacillus plantarum ZY08 on hypercholesteremic Golden hamsters. Front. Nutr. 8:705763. doi: 10.3389/fnut.2021.705763
Yang, J., Zheng, P., Li, Y., Wu, J., Tan, X., Zhou, J., et al. (2020). Landscapes of bacterial and metabolic signatures and their interaction in major depressive disorders. Sci. Adv. 6:555. doi: 10.1126/sciadv.aba8555
Yanguas-Casás, N., Barreda-Manso, M. A., Nieto-Sampedro, M., and Romero-Ramírez, L. (2017). TUDCA: an agonist of the bile acid receptor GPBAR1/TGR5 with anti-inflammatory effects in microglial cells. J. Cell. Physiol. 232, 2231–2245. doi: 10.1002/jcp.25742
Yano, J. M., Yu, K., Donaldson, G. P., Shastri, G. G., Ann, P., Ma, L., et al. (2015). Indigenous bacteria from the gut microbiota regulate host serotonin biosynthesis. Cells 161, 264–276. doi: 10.1016/j.cell.2015.02.047
Yoshida, N., Emoto, T., Yamashita, T., Watanabe, H., Hayashi, T., Tabata, T., et al. (2018). Bacteroides vulgatus and Bacteroides dorei reduce gut microbial lipopolysaccharide production and inhibit atherosclerosis. Circulation 138, 2486–2498. doi: 10.1161/circulationaha.118.033714
Yoshida, R., Imanishi, J., Oku, T., Kishida, T., and Hayaishi, O. (1981). Induction of pulmonary indoleamine 2,3-dioxygenase by interferon. Proc. Natl. Acad. Sci. U. S. A. 78, 129–132.
Zelante, T., Iannitti, R. G., Cunha, C., De Luca, A., Giovannini, G., Pieraccini, G., et al. (2013). Tryptophan catabolites from microbiota engage aryl hydrocarbon receptor and balance mucosal reactivity via interleukin-22. Immunity 39, 372–385. doi: 10.1016/j.immuni.2013.08.003
Zellweger, M. J., Osterwalder, R. H., Langewitz, W., and Pfisterer, M. E. (2004). Coronary artery disease and depression. Eur. Heart J. 25, 3–9. doi: 10.1016/j.ehj.2003.09.009
Zhang, K. K., Chen, L. J., Li, J. H., Liu, J. L., Wang, L. B., Xu, L. L., et al. (2022). Methamphetamine disturbs gut homeostasis and reshapes serum metabolome, inducing neurotoxicity and abnormal behaviors in mice. Front. Microbiol. 13:755189. doi: 10.3389/fmicb.2022.755189
Zhang, G., Han, J., Welch, E. J., Ye, R. D., Voyno-Yasenetskaya, T. A., Malik, A. B., et al. (2009). Lipopolysaccharide stimulates platelet secretion and potentiates platelet aggregation via TLR4/MyD88 and the cGMP-dependent protein kinase pathway. J. Immunol. 182, 7997–8004. doi: 10.4049/jimmunol.0802884
Zhang, Y., Huang, R., Cheng, M., Wang, L., Chao, J., Li, J., et al. (2019). Gut microbiota from NLRP3-deficient mice ameliorates depressive-like behaviors by regulating astrocyte dysfunction via circHIPK2. Microbiome 7:116. doi: 10.1186/s40168-019-0733-3
Zhang, L., Ovchinnikova, O., Jönsson, A., Lundberg, A. M., Berg, M., Hansson, G. K., et al. (2012). The tryptophan metabolite 3-hydroxyanthranilic acid lowers plasma lipids and decreases atherosclerosis in hypercholesterolaemic mice. Eur. Heart J. 33, 2025–2034. doi: 10.1093/eurheartj/ehs175
Zheng, P., Wang, Y., Chen, L., Yang, D., Meng, H., Zhou, D., et al. (2013). Identification and validation of urinary metabolite biomarkers for major depressive disorder. Mol. Cell. Proteomics 12, 207–214. doi: 10.1074/mcp.M112.021816
Zheng, P., Wu, J., Zhang, H., Perry, S. W., Yin, B., Tan, X., et al. (2021). The gut microbiome modulates gut-brain axis glycerophospholipid metabolism in a region-specific manner in a nonhuman primate model of depression. Mol. Psychiatry 26, 2380–2392. doi: 10.1038/s41380-020-0744-2
Zheng, P., Zeng, B., Zhou, C., Liu, M., Fang, Z., Xu, X., et al. (2016). Gut microbiome remodeling induces depressive-like behaviors through a pathway mediated by the host's metabolism. Mol. Psychiatry 21, 786–796. doi: 10.1038/mp.2016.44
Zhou, F., Jiang, H., Kong, N., Lin, J., Zhang, F., Mai, T., et al. (2022). Electroacupuncture attenuated anxiety and depression-like behavior via inhibition of hippocampal inflammatory response and metabolic disorders in TNBS-induced IBD rats. Oxidative Med. Cell. Longev. 2022:8295580. doi: 10.1155/2022/8295580
Zhou, X., Li, J., Guo, J., Geng, B., Ji, W., Zhao, Q., et al. (2018). Gut-dependent microbial translocation induces inflammation and cardiovascular events after ST-elevation myocardial infarction. Microbiome 6:66. doi: 10.1186/s40168-018-0441-4
Zhu, W., Gregory, J. C., Org, E., Buffa, J. A., Gupta, N., Wang, Z., et al. (2016). Gut microbial metabolite TMAO enhances platelet Hyperreactivity and thrombosis risk. Cells 165, 111–124. doi: 10.1016/j.cell.2016.02.011
Keywords: microbiome metabolites, atherosclerosis, depression, gut microbiome, comorbid
Citation: Liao X-X, Wu X-Y, Zhou Y-L, Li J-J, Wen Y-L and Zhou J-J (2022) Gut microbiome metabolites as key actors in atherosclerosis co-depression disease. Front. Microbiol. 13:988643. doi: 10.3389/fmicb.2022.988643
Edited by:
Giovanni Tarantino, University of Naples Federico II, ItalyReviewed by:
Christoph Reinhardt, Johannes Gutenberg University Mainz, GermanyLei Ji, Zhejiang University, China
Copyright © 2022 Liao, Wu, Zhou, Li, Wen and Zhou. This is an open-access article distributed under the terms of the Creative Commons Attribution License (CC BY). The use, distribution or reproduction in other forums is permitted, provided the original author(s) and the copyright owner(s) are credited and that the original publication in this journal is cited, in accordance with accepted academic practice. No use, distribution or reproduction is permitted which does not comply with these terms.
*Correspondence: You-Liang Wen, MTAzNzExNDg0NUBxcS5jb20=; Jun-Jie Zhou, emhvdWp1bmppZUBnbXUuZWR1LmNu