- 1Department of Pediatric Critical Care Medicine, Xinhua Hospital, Affiliated to the Medical School of Shanghai Jiao Tong University, Shanghai, China
- 2Department of Pediatric Respiratory, Xinhua Hospital, Affiliated to the Medical School of Shanghai Jiao Tong University, Shanghai, China
Sepsis most often involves the kidney and is one of the most common causes of acute kidney injury. The prevalence of septic acute kidney injury has increased significantly in recent years. The gut microbiota plays an important role in sepsis. It interacts with the kidney in a complex and multifactorial process, which is not fully understood. Sepsis may lead to gut microbiota alteration, orchestrate gut mucosal injury, and cause gut barrier failure, which further alters the host immunological and metabolic homeostasis. The pattern of gut microbiota alteration also varies with sepsis progression. Changes in intestinal microecology have double-edged effects on renal function, which also affects intestinal homeostasis. This review aimed to clarify the interaction between gut microbiota and renal function during the onset and progression of sepsis. The mechanism of gut–kidney crosstalk may provide potential insights for the development of novel therapeutic strategies for sepsis.
Highlights
- Sepsis-associated acute kidney injury is a growing global health challenge, affected by changes in the composition and function of the gut microbiota.
- The presence of a “gut–kidney axis” facilitates bidirectional communication between gut microbiota and renal function and is involved in the development of sepsis.
- Loss of endothelial barrier integrity and dysbiosis of the gut microbiota are the primary pathophysiological alterations in sepsis, which play a critical role in sepsis-associated acute kidney injury in response to various pathological insults, with both positive and negative effects.
- The gut–kidney axis has the potential to be an important target in the treatment of sepsis-associated acute kidney injury.
Introduction
Intestinal barrier dysfunction and gut microbiota disorders are important determinants of the occurrence and development of sepsis (Meng et al., 2017). As a vital component of the intestinal tract, the gut microbiota critically influences important physiological processes, such as biosynthesis, and metabolism (Yeh et al., 2016; Meng et al., 2017; Antushevich, 2020). The kidney is the organ that is most involved in sepsis. Studies have shown that patients with sepsis-associated acute kidney injury (SA-AKI) have a higher prevalence and more severe renal damage than that observed in those without SA-AKI (Zeng et al., 2014). Peng et al. (2014) reported that the incidence of SA-AKI was 11–31% and was as high as 41–78% in patients with septic shock. Paradoxically, patients with a normal serum creatinine (SCr) concentration may display augmented renal clearance (ARC) during the early stages of sepsis [i.e., the ability of kidneys to increase the glomerular filtration rate (GFR) in response to certain physiological or pathological stimuli] (Sharma et al., 2014). This counterintuitive and accelerated drug elimination was present in 39.5–56% of sepsis patients (Baptista et al., 2012; Carrié et al., 2018). Abundant evidence has indicated that the gut microbiota is involved in the regulation of renal function in sepsis patients (Miller et al., 2021). The core theoretical view of the “gut–kidney axis” is that during the progression of kidney damage, there are intestinal microecosystem disorders, including a decrease in beneficial bacteria and an increase in pathogenic bacteria that can produce urotoxin, leading to the accumulation of intestinal urotoxin in the blood. These toxins cannot be cleared by the damaged kidneys in time, thus exacerbating kidney damage and eventually forming a vicious circle between the intestines and kidneys (Nakade et al., 2018; Wu et al., 2020). The intestinal epithelial barrier in sepsis patients is compromised, such that intestinal urotoxin and conditional pathogenic bacteria are displaced and enter the blood circulation, activating the intestinal mucosal immune system and inducing systemic inflammatory response syndrome (SIRS) (Gao et al., 2015; Tan R. Z. et al., 2020). Some studies have suggested that a diverse gut microbiota can delay kidney aging and improve renal function (Nie et al., 2022). Deciphering the interaction between gut microbiota and renal function in sepsis patients, as well as its underlying mechanisms, is a major public health challenge in the development of new prevention or treatment strategies. In this review, we focused on studies exploring the effects of altered gut microbiota on renal function in sepsis patients.
Gut microbiota in sepsis
Most gut microbial species belong to four major phyla: Firmicutes, Actinobacteria, Proteobacteria, and Bacteroidetes. Gut microbial species in the phyla Bacteroidetes and Firmicutes account for more than 90% of all bacteria (Ramakrishna, 2013; Chen, 2016; Lankelma et al., 2017b; Akash et al., 2019; Firmino et al., 2020).
Dysbiosis of gut microbiota in sepsis patients
Compared with the healthy control group, there were differences in the number and distribution of bacteria as well as in the microbiota structure of the sepsis group (Yang et al., 2021). Several studies have shown that the ecological and functional microenvironment of the gut changes when sepsis occurs (Figure 1; Bassetti et al., 2020; Kullberg et al., 2021; Sun et al., 2022). First, gut microbiota diversity and richness are substantially decreased in sepsis. Using lipopolysaccharide (LPS)-induced septic shock and cecal ligation and puncture (CLP)-induced polymicrobial septic models, a study found that non-sepsis samples had a distinct bacterial diversity that was significantly enriched (i.e., Chao1 index, 2151.26 ± 230.87 vs. 1800.81 ± 325.82) and more diverse (i.e., Shannon index, 6.27 ± 0.39 vs. 5.29 ± 0.72) compared to that of sepsis (Chen et al., 2019). In another study, the gut microbiota of the healthy controls had a significantly higher Shannon index than that of the sepsis group (3.49 ± 0.45 vs. 2.98 ± 0.68) (Zhu et al., 2018). Second, there are relative changes in the community structure and composition of the gut microbiota during sepsis (Dickson, 2016). The most significant change in the gut microbiota composition in sepsis patients is the decrease in protective symbiotic flora, particularly obligate anaerobic bacteria and lactobacilli, and the abundance of pathogenic bacteria such as Enterococcus and Pseudomonas increases and can dominate the gut flora (Dickson, 2016; Lankelma et al., 2017b; Wan et al., 2018). In a recent prospective study involving intensive care unit (ICU) sepsis patients at admission, a relative high abundance of Proteobacteria was observed in approximately one-third of patients (healthy controls vs. ICU patients, P = 0.02), and the proportion of Gram-positive and Gram-negative bacteria also increased (Lankelma et al., 2017b). Another study also confirmed a higher abundance of Proteobacteria in sepsis patients (mean relative proportion, 23.71 vs. 3.53% in septic shock patients vs. healthy controls) (Wan et al., 2018). Shimizu et al. reported changes over time in the most abundant phyla, Bacteroidetes, and Firmicutes, in patients with SIRS (Ojima et al., 2016). At the same time, patients who presented with extremes have poor outcomes, including increased in-hospital mortality (Ojima et al., 2016). Furthermore, changes in the gut microbiota appear to result in chain reactions. An increase in pathogenic bacteria can degrade and penetrate the protective mucus layer, followed by adhesion and colonization of intestinal epithelial cells, disruption of the intestinal mucosal barrier, and exacerbation of infection (Paone and Cani, 2020). Animal experiments have shown that a decrease in beneficial intestinal bacteria in mouse models of sepsis could exacerbate the intestinal colonization and virulence of Pseudomonas aeruginosa (P. aeruginosa) (Christofi et al., 2019). Notably, antibiotic therapy, the main therapeutic measure for sepsis, can also lead to changes in the gut microbiota. Christofi et al. administered a regime containing three broad-spectrum antibiotics in mice and found that Escherichia coli (E. coli) was detected in a mouse model of sepsis without antibiotic treatment, but not in antibiotic-treated mice, which become susceptible to opportunistic infection. They also point out that antibiotic treatment induced dysbiosis, which is exemplified by eradication of E. coli as well as reduction of all commonly prevalent phyla in the gut to undetectable levels (Christofi et al., 2019). This observation is similar to the results of other human studies (Lankelma et al., 2017a; Du et al., 2021). Many broad-spectrum antibiotics inhibit or kill dominant flora, leading to increased colonization of conditioned pathogenic bacteria and fungi, and ultimately resulting in opportunistic or secondary infections and exacerbation of intestinal microbiome imbalances (Song and Kim, 2019). Several other studies have suggested that the use of broad-spectrum antibiotics increases the risk of subsequent Clostridium difficile (C. difficile) infections (Deshpande et al., 2015).
Alterations in the gut ecological and functional microenvironment in different stages of sepsis
Hayakawa et al. (2011) found that the gut microbiota changed significantly within the first 6 h of critical illness, mainly manifested by a significant decrease in the protective flora, especially obligate anaerobes, whereas harmful bacteria, such as Enterococcus faecalis and P. aeruginosa, increased, accompanied by a decrease in the three main short-chain fatty acids (SCFAs), including butyrate, propionate, and acetate. Zhu et al. (2018) indicated that the gut microbiota composition changed gradually over time. We summarized the most relevant clinical studies published to date on sepsis patients that correlated with the gut microbiota (Supplementary Table 1). A flowchart of the literature search and screening process was shown in Supplementary Figure 1.
Within 48 h following the confirmation of sepsis, the abundance of the gut microbiota and the bacterial diversity in sepsis patients were reduced, as compared with those in healthy controls, and this phenomenon could persist for approximately 1°week. Moreover, treatment with antibiotics or probiotics could affect the outcomes. In seven clinical studies, the dominant gut microbiota was different in sepsis patients, mainly Firmicutes and Proteobacteria (Shimizu et al., 2018; Liu et al., 2019, 2020, 2021; Stadlbauer et al., 2019; Du et al., 2021; Reyman et al., 2022). Notably, Niu and Chen, 2021 found that the composition and functional components of the gut microbiota in sepsis patients exhibited a robust circadian rhythm. Current evidence suggested an increase in the abundance of Firmicutes during the eating phase and an increase in Actinobacteria and Proteobacteria during the fasting phase (Parkar et al., 2019). Based on the circadian interactions between the host and microbiome, strict regulation between the gut microbiota and immune system will aid in the development of more precise therapies for treating sepsis. Additionally, significant individual differences have been noted among these studies (Shimizu et al., 2018; Stadlbauer et al., 2019; Liu et al., 2020, 2021; Du et al., 2021; Reyman et al., 2022). Therefore, changes in the gut microbiota during sepsis cannot be generalized. The results of some studies require further verification by clinical randomized controlled trials; nevertheless, a few trials investigating the gut microbiota in sepsis patients (e.g., NCT02469571 and TCTR20191017004) are currently underway.
Gut–kidney crosstalk in sepsis
Changes in the gut microbiota in sepsis not only are diverse but also have various effects on the body.
Gut microbiota has deleterious effects on the kidneys
Meijers and Evenepoel (2011) proposed the concept of “gut–kidney axis” in 2011. Subsequently, Pahl and Vaziri, 2015 refined this theory. The foundation of this theory is that kidney injury can lead to gut microbiota disorders and damage the intestinal epithelial barrier function. Intestinal microecological imbalance can also produce metabolic toxins, aggravating kidney injury. With the increasing availability of genomics, the “microbiota-toxin-barrier-inflammation” event chain of the gut–kidney axis has been elucidated (Figure 2).
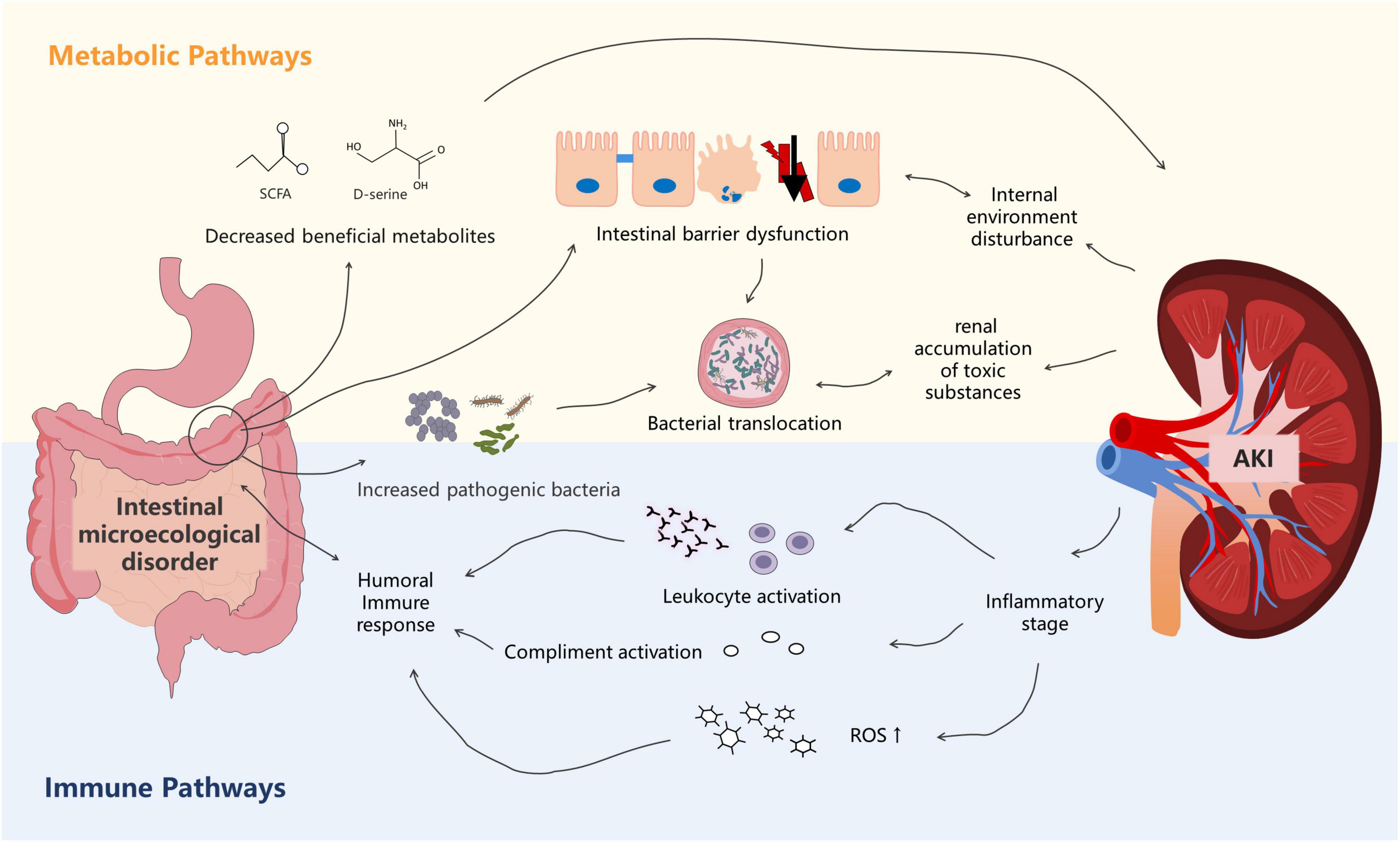
Figure 2. The metabolism-dependent and immune pathways of the gut–kidney axis in sepsis. Dysbiosis of the gut microbiota is associated with altered metabolic pathways that cause accumulation of uremic toxins, leading to kidney injury. Kidney injury, in turn, affects the gut microecology. In the immune pathway, products derived from bacterial members of the gut microbiota evoke immune signaling pathways in the host, leading to systemic inflammatory syndrome. Kidney injury also affects immunity.
First, sepsis itself can affect the abundance and composition of the gut microbiota, which can subsequently promote the occurrence and development of acute kidney injury (AKI) (Emal et al., 2017; Yuan et al., 2020). Nakade et al. (2018) found that fecal transplantation from normal mice attenuated renal pathology in germ-free C57BL/6 mice, suggesting that the gut microbiota in normal mice may produce molecules that protect the kidneys. Recent studies have indicated that, among the bacterial metabolites, SCFAs play a protective role in AKI (Andrade-Oliveira et al., 2015). In addition to SCFAs, gut microbiota–derived D-serine has also been suggested to improve kidney injury (Sasabe et al., 2016; Nakade et al., 2018). Paradoxically, Emal et al. (2017) found that gut microbiota depletion with broad-spectrum antibiotics protected against renal ischemia–reperfusion (I/R) injury. Mechanistically, Emal et al. (2017) reported that microbiota depletion significantly attenuated renal damage by reducing the maturation status of F4/80 renal-resident macrophages and bone marrow monocytes. These two different mechanisms suggest that the gut microbiota exerts multiple effects on the kidneys. Changes in the gut microbiota composition also play an important role in renal function. Gut microbiota alteration leads to the release of inflammatory mediators that pass into the circulation, thereby affecting the renal function. Druml (2014) suggested that the release of cytokines and inflammatory mediators increased oxidative stress, activation of various immune cells, neutrophil extravasation, and generalized kidney injury. Using a model of endotoxin-induced sepsis, Yu et al. (2018) showed that IL-1β, TNF-α, MCP-1, and NOS-2 levels were significantly increased in kidney tissues. Li et al. (2019) also showed that gut-derived endotoxin, resulting from increased intestinal permeability after severe renal I/R, subsequently amplified the intrarenal inflammatory response by activating renal TLR4 signaling. Furthermore, gut microbiota alteration leads to an increase in the oxidative stress level in AKI and exacerbates the ischemic injury. Xie et al. (2020) described that a significant increase in Helicobacter in the gut microbiota of mice might directly or indirectly affect the oxidative stress level through serum biochemical indicators. Moreover, Clostridium was negatively correlated with superoxide dismutase and glutathione peroxidase but was positively correlated with malondialdehyde.
Second, sepsis can disrupt the intestinal barrier, exacerbating the impact of the gut microbiota on the kidneys. Many studies have shown that sepsis causes changes in the integral and peripheral membrane proteins (i.e., myosin) that make up tight junctions, leading to significant increases in intestinal permeability (Meng et al., 2017; Paone and Cani, 2020). Lorentz et al. (2017) found that myosin light chain kinase (MLCK) in mouse models of sepsis phosphorylated myosin regulatory light chains, impairing the intestinal barrier function and increasing permeability. The survival rate of MLCK-knockout mice was significantly higher than that of the control group (95 vs. 24%, respectively, P < 0.0001), and the rates of kidney injury were also lower. The intestinal chemical barrier is also disrupted in sepsis patients owing to changes in the gut microbiota composition (Paone and Cani, 2020). Although the precise mechanism remains unknown, Johansson et al. (2015) suggested that it may be related to changes in glycosyltransferase expression levels and that O-glycan patterns dynamically change with changes in the gut microbiota. Using a model of Goodpasture disease that resulted in nephrotoxic nephritis, Pagan et al. (2018) found that extracellular glycosyltransferases likely influence IgG Fc glycoforms and could attenuate inflammation. Several studies have shown that damage to the intestinal mucosal barrier may stimulate pro-inflammatory events, exacerbating the displacement of the microflora, which can cause kidney damage (Druml, 2014; Capaldo et al., 2017; Li et al., 2019).
Acute kidney injury (AKI) also affects the gut microbiota. A recent study using a model of AKI reported a decreased abundance of Bifidobacterium and an increased abundance of Lactobacillus, Clostridium, and Ruminococcus after I/R injury (Nakade et al., 2018). The authors suggested that this finding might result from the protective effect of the gut microbiota against tubular injury in this mouse model of AKI. Further studies suggested that the protective effect may be related to T-helper 17 (Th17) cells (Krebs et al., 2016). Krebs et al. (2016) found that Th17 cells egressed from the gut in an S1P-receptor-1-dependent fashion and subsequently migrated to the kidney via the CCL20/CCR6 axis, and depletion of intestinal Th17 cells in germ-free and antibiotic-treated mice ameliorated renal disease. However, this self-regulatory mechanism has a limited effect. The inflammatory response triggered by AKI and the high volume load altered vascular permeability, leading to vascular leakage and angioedema, eventually disrupting intestinal epithelial tight junctions, increasing intestinal epithelial cell apoptosis, and suppressing epithelial turnover (Gong et al., 2019). Takeda et al. (2021) found that ileal villous epithelial cells in a rat model of AKI were shed, and the integrity of the intrinsic layer was impaired. Persistent impairment of the permeability and integrity of the intestinal epithelial barrier led to spillage of bacteria, endotoxins, and/or macromolecular substances, exacerbating sepsis (Hudson et al., 2017; Nakade et al., 2018). Moreover, Ranganathan et al. (2010) indicated that the metabolites produced by this altered gut microbiota resulted in the accumulation of uremic toxins, such as indoxyl sulfate (IS) and p-cresyl sulfate, causing kidney injury by contributing to the inflammatory infiltrate and tubular atrophy.
From an immunological perspective, the cytokines released by the kidneys can have an important effect on the intestines through the circulation (Hato and Dagher, 2015). Using a mouse model of LPS-induced endotoxemia, Quan et al. (2020) showed that B cell-activating factor levels were significantly upregulated in the kidneys, whereas serum IL-1β, IL-6, and TNF-α levels were significantly increased. Furthermore, they revealed that inflammatory mediator in the blood circulation influenced the gut microbiome by increasing intestinal hyperpermeability (Quan et al., 2020). Further studies have indicated that TLR4 activation in SA-AKI could result in the release of cytokines and chemokines as well as leukocyte infiltration, which lead to endothelial and renal tubular dysfunction and further contribute to this inflammatory state, causing multiple organ dysfunctions syndrome (Anderberg et al., 2017). Wang L. et al. (2016) found that the activation of the TLR4 receptor increased the release of endotoxins from the gut microbiota, leading to SIRS and aggravated AKI.
Unraveling the contribution of the gut microbiota to the development of augmented renal clearance
In 2010, Udy et al. (2010) found that patients with severe disease often experienced hyperfunction in the early stages of the disease, and some began to develop kidney damage as the disease progressed. Grootaert et al. (2012) observed that 30% of severely ill patients had at least one episode of ARC, lasting for 5–7 days on average. Most studies defined ARC as a creatinine clearance rate of ≥ 130 mL/min/1.73 m2 (De Waele et al., 2015; Mahmoud and Shen, 2017; Bilbao-Meseguer et al., 2018). However, the understanding of the fundamental mechanisms of ARC is incomplete. Currently, three main mechanisms of ARC are widely accepted—namely, SIRS, renal function reserve (RFR), and brain–kidney crosstalk (Cook and Hatton-Kolpek, 2019; Luo et al., 2021). Interestingly, all of them appear to be linked to the gut microbiota (Figure 3).
The theory of systemic inflammatory response syndrome
In the early stages of sepsis, the organism is in a state of hypermetabolism, accompanied by the release of pro-inflammatory mediators, which may reduce vascular resistance and increase cardiac output, leading to increased renal blood flow and changes in glomerular filtration (Langenberg et al., 2005; Trof et al., 2010). Gut microbiota plays an important role in this process. First of all, in sepsis, intestinal permeability increases, bacteria and toxins originally present in the intestinal lumen pass through the intestinal wall, direct venous invasion to distant organs with involvement of lymph nodes and proliferate in large quantities, subsequently causing constant generation of inflammatory cytokines and leading to the “waterfall” cascading effects (Vaishnavi, 2013; Paone and Cani, 2020). Second, gut microbiota affects vascular resistance. Traykova et al. (2017) reported that when bacterial translocation occurred, the number of bacterial species and the total bacterial DNA were positively correlated with the serum nitric oxide level [odds ratio (OR): 0.64, 95% confidence interval (CI): 0.247–0.852, P = 0.0041 and OR: 0.62, 95% CI: 0.216–0.842, P = 0.0062, respectively] and were inversely correlated with systemic vascular resistance (OR: −0.593, 95% CI: −0.83 to −0.175, P = 0.0095 and OR: −0.621, 95% CI: −0.843 to −0.218, P = 0.0060, respectively). Huang et al. (2021) also found that fecal microbiota transplantation (FMT) restored the normal gut microbiota composition and downregulated phosphorylated endothelial nitric oxide synthase, leading to improved vasodilation. Therefore, we speculated that the gut microbiota in sepsis patients may promote increased renal blood flow by participating in the SIRS response, leading to ARC. However, relevant research has not directly reached this conclusion. Although studies have shown that the gut microbiota affected nitric oxide expression, these reports experimented with cirrhosis, and it is unclear whether the underlying disease affected this process (Traykova et al., 2017; Huang et al., 2021).
The theory of renal function reserve
In the resting state, the kidneys work with basic abilities, and can make corresponding adjustments according to physiological stimuli, increasing to a certain high-level state (Thomas et al., 1994). The earliest RFR elaboration can be traced back to 1983. Bosch et al. (1983) found that normal subjects had a significant increase in GFR after acute protein loading (1.0–1.2 g/kg). RFR is also present in sepsis patients, and Belcher et al. (2011) found that in the early stage of sepsis, the increase in SCr in patients was not obvious due to the strong reserve function of the kidneys. Langenberg reviewed experimental animal and human studies of SA-AKI and found that 62% of animals showed a decrease in renal blood flow (RBF), while the remaining 38% exhibited no change or increase in RBF (Langenberg et al., 2005). Maiden et al. (2016) monitored sheep models of sepsis and found that renal oxygen consumption did not change with disease severity. However, the stimulation conditions for RFR in sepsis patients remain unclear, and multiple studies have suggested that protein intake may be involved, and may increase GFR through vasodilation and increased RBF (Bosch et al., 1983; Sharma et al., 2014; Ronco et al., 2017). Interestingly, this phenomenon also seems inextricably linked to gut microbiota. First, dysbiosis of the gut microbiota after a high-protein diet triggers an inflammatory response. Rong et al. found that bacteria that contribute to protein fermentation in a high-protein diet were significantly higher than those in the control group (Tan R. et al., 2020). The proliferation of pathogenic microbiota leads to increased intestinal permeability, and a study also found that the levels of serum inflammatory markers in mice on high-protein diets and the water level of serum diamine oxidase, a classical permeability marker, demonstrated that high-protein diets could cause increased intestinal permeability and the occurrence of intestinal inflammation (Tan R. et al., 2020). Therefore, we speculate that a high-protein diet may result in increased RBF through the inflammatory response pathway, which in turn causes an increase in the GFR. Second, the amino acid metabolites of the intestinal flora increased in the high-protein diet group, and the intestinal mucosa was damaged. SCFAs, hydrogen sulfide, indole compounds, histamine, and serotonin are amino acid-derived bacterial metabolites that play an important role in stimulating the immune response and maintaining the integrity of the intestinal epithelial barrier. Andriamihaja et al. (2010) showed that a high-protein diet significantly reduced the height of the absorptive colonic epithelial cell brush border in mice while reducing cellular energy production. Toden et al. (2005) showed that a high-protein diet could cause an increase in cresol levels in bacterial-derived genotoxic metabolites, which in turn induced DNA damage in colon cells. Wu et al. (2001) found that plasma amino acid levels gradually increased at 6 h after sepsis in a rat model intraperitoneally injected with endotoxins and reached a peak after 24 h. Additional studies have found that elevation of plasma amino acid levels increased proximal tubular reabsorption and endothelium-derived relaxing factors, leading to blood vessel dilation and RBF increase (Sharma et al., 2014). RFR reserves are limited and largely depend on complete renal function. When more than 50% of the renal units are destroyed, renal functional reserves are lost and SCr values tend to increase (Ronco et al., 2017).
The theory of brain–kidney crosstalk
Dias et al. (2015) found that intracranial pressure, cerebral perfusion pressure, and cerebrovascular reactivity pressure index were significantly associated with ARC in patients with traumatic brain injury, suggesting the presence of a “brain–kidney crosstalk.” Yang et al. (2018) further proposed the concept of the brain–gut–kidney axis. The gut microbiota communicates with the brain through three parallel and interacting pathways, including the neural, immune, and metabolite pathways (Yu and Zhao, 2021). Evidence suggests that the vagus nerve can sense microbial signals in forms of bacterial metabolites or be affected by the microbiota-mediated regulation of enterocrine and intestinal epithelial cells (Margolis et al., 2021). Nerve fluid axis activation further affects renal function by regulating RBF (Bouglé and Duranteau, 2011). Notably, changes in the limbic system of sepsis patients regulated intestinal immunity through the autonomic nervous system, acetylcholine anti-inflammatory pathways, hypothalamic–pituitary–adrenal axis, and neuropeptides (Ye et al., 2019). Ben-Shaanan et al. (2016) used direct neuronal manipulation to establish a causal relationship between the brain’s reward system and immunity and found that activation of the reward system increased the primary antibacterial immune response and that after pathogen re-exposure. Increased paracellular permeability during brain injury may contribute to gut microbiota translocation and promote systemic inflammatory responses (Li X. J. et al., 2020). Inflammation induced by gut pathogens in turn increased the risk of brain damage (Franceschi et al., 2019).
Metabolites produced by the gut microbiota can act as chemical signals that directly or indirectly regulate homeostasis of the central nervous system. Studies have shown that D-serine can be synthesized and secreted by glial cells and act as a co-agonist of the extrasynaptic N-methyl-D-aspartate subtype of glutamate receptors, which are involved in neurodegenerative disorders and cell death (Holeček, 2022). Nakade et al. (2018) showed that increased D-serine secretion improved renal function.
Gut–kidney axis-based treatment for sepsis
Based on the aforementioned mechanisms of enterorenal interaction in sepsis, some studies have suggested that the gut microbiota is the target of treatment for SA-AKI, which can be divided into the following three categories according to its mechanism: (i) probiotic supplementation or gut microbiota metabolite supplementation/inhibition, (ii) selective digestive tract decontamination (SDD), and (iii) microbial replacement therapies (Yuan et al., 2020; Kullberg et al., 2021; Zhu et al., 2021).
Supplementation or inhibition of gut microbiota metabolites
Fecal microbiota transplantation (FMT) carries certain risks, and direct supplementation with gut microbiota-related metabolites to improve renal function is a hot topic. Multiple studies have reported that treatment with SCFAs reduced kidney injury in various animal models (Machado et al., 2012; Sun et al., 2013; Andrade-Oliveira et al., 2015). SCFAs protect the kidneys through mechanisms, including anti-inflammatory effects, immune regulation, and intestinal barrier reparation (Hayakawa et al., 2011; Andrade-Oliveira et al., 2015). Therefore, SCFAs are expected to become a new type of drug to improve renal function. However, the amount and timing of SCFAs supplementation are still matters of debate. The effective concentration of SCFAs for renoprotective effects in the aforementioned studies is also markedly higher than their physiological concentration, and the safety of their clinical application remains to be demonstrated. We believe that giving full consideration to the positive effects of gut microbiota on renal function can also improve kidney damage. Si et al. (2013) found that adrenocorticosteroid gel could prevent tumor necrosis factor-induced damage to the renal tubular cells in rats and confirmed that the use of the brain–gut–kidney axis could also exert a protective effect against kidney damage. In addition, the suppression of harmful metabolites is the focus of improving renal function. Protein can be transformed by gut microbiota into a variety of metabolites including p-cresol sulfate and IS, which, as uremic toxins, can cause renal damage by promoting mononuclear/macrophage infiltration (Ranganathan et al., 2010; Wu et al., 2020). Currently, the best treatment strategy against toxins produced by gut microbiota metabolism is to change the gut microenvironment, including dietary adjustment, the use of probiotics, and targeted intervention in the gut (Skye and Hazen, 2016). Animal studies have shown that administration of AST-120 resulted in the reduction of plasma endotoxin and inflammatory cytokines, chemokines, and adhesion molecules, thereby attenuating systemic inflammation (Vaziri et al., 2013). Hatakeyama et al. (2012) found that the use of AST-120 in patients with chronic kidney disease (CKD) could reduce IS levels in blood and urine and delay the time for CKD patients to enter dialysis. Of interest, in 2016, Devlin et al. (2016) knocked out tryptophanases in mice to control IS production, a true case of targeting gut microbiota for disease treatment. This finding suggested that the host IS concentration can be controlled by targeting the gut microbiota, which may be a possible strategy to treat kidney injury.
Probiotic supplementation
A prospective study conducted on patients receiving oral probiotics [90 billion colony forming units (CFUs)/day] reported decreases in urea nitrogen (n = 29, 63%), SCr (n = 20, 43%), and uric acid levels (n = 15, 33%) (Ranganathan et al., 2010). Zhu et al. (2021) showed that oral administration of Lactobacillus casei Zhang could alleviate kidney injury and that this probiotic as a preventive intervention could improve gut microbiota disorder, intestinal inflammation, and intestinal mucosal barrier injury caused by renal I/R injury. Mechanistically, a number of studies showed that probiotics could improve the immune function of sepsis patients by effectively reducing the inflammatory response (Wu et al., 2017; Bassetti et al., 2020; Zhu et al., 2021). However, some researchers have questioned the benefits of probiotics in SA-AKI treatment. Two reviews in 2019 noted that current clinical trials of probiotics have not been highly consistent, and the quality of evidence is often low (Abbasi, 2019; The Lancet Gastroenterology Hepatology, 2019). Zmora et al. (2018) studied 25 healthy volunteers who took probiotics for 2°months and found that whether probiotics could take root in the gut was entirely different in each participant. More targeted use of probiotics in the future, particularly based on an individual’s specific microbiome composition, may benefit SA-AKI patients.
Antibiotics-based selective digestive tract decontamination
While the early use of antibiotics for sepsis patients has been a diagnosis and treatment consensus, the impact of antibiotics on gut microbiota cannot be ignored. Emal et al. used broad-spectrum antibiotics (1 g/L ampicillin, 1 g/L metronidazole, 1 g/L neomycin, and 0.5 g/L vancomycin) for SDD. Reduced gut microbiota can affect the maturation status of macrophages/monocytes and the release of chemokines, thus ameliorating renal I/R injury (Emal et al., 2017). In contrast, Lee et al. (2021) found that some antibiotics, such as ciprofloxacin, increased the secretion of Shiga toxin 2 (Stx2), by enhancing the expression of Stx2, thereby increasing the risk of hemolytic uremic syndrome. However, these two studies examined different subjects, antibiotics, and time frames. In addition, Lee et al. found that gentamicin is more efficient in reducing O157 infection and STX2-induced renal cell damage compared to ciprofloxacin, indicating that the effects of different antibiotic classes on gut microbiota are largely unknown. It is important to note that, according to the SIRS theory, the effects of early inflammatory responses on the kidneys are not all unfavorable, and it is debatable whether active use of broad-spectrum antibiotics to deplete the intestinal flora increases the risk of kidney damage (Langenberg et al., 2005; Trof et al., 2010). Ng et al. tracked microbiota dynamics with high temporal and taxonomic resolution during antibiotic treatment in a controlled murine system, isolating for variables such as diet, treatment history, and housing co-inhabitants, and found transient dominance of resistant Bacteroides and sustained loss in α-diversity during antibiotic treatment. Initial antibiotic treatment can condition the Bacteroides response of a human microbiota for subsequent treatments, suggesting the importance of FMT and targeted microbial treatments in restoring gut microbiota (Ng et al., 2019).
Microbial replacement therapies: Benefits and risks
Microbial replacement therapies, particularly FMT, refer to the process of transplanting the gut microbiota from healthy donors to patients in order to reconstruct the normal intestinal microecology. FMT plays an important protective role through anti-inflammatory and antioxidant mechanisms, as well as the supplementation of intestinal probiotic metabolite production (Xiao et al., 2020). Nakade et al. (2018) showed that FMT from normal mice attenuated renal I/R injury by increasing the D-serine levels. Assimakopoulos et al. reported that FMT had a positive effect on intestinal barrier integrity by inhibiting the nuclear factor kappa-light-chain enhancer of activated B cells and increasing the expression of occludin (56 ± 15%) and claudin-1 (84 ± 7%). Moreover, FMT could restore immune ecological regulation by increasing the number of Paneth cells and inhibiting the release of inflammatory factors (Assimakopoulos et al., 2021). Li B. et al. (2020) showed that FMT could restore the gut microbiota, increasing the production of beneficial bacteria and SCFAs, restraining harmful bacteria, and inhibiting the activation of the TGF-β1/Smad/ERK signaling pathway. Nonetheless, thus far, no clinical studies have shown the effects of FMT on renal function. As such, this research direction may be further explored in the future. Moreover, long-term safety trials on FMT are lacking, with some studies suggesting that FMT is not always beneficial (Wang S. et al., 2016). Emal et al. (2017) reported that FMT exacerbated kidney damage in mice treated with antibiotics, suggesting that this result might be related to the inflammatory response produced by the gut microbiota. Wang et al. found that, among 7,562 studies in the literature, 78 reported FMT-related adverse events, of which serious adverse events included death (3.5%), inflammatory bowel disease recurrence (0.6%), and C. difficile infection (0.9%). The occurrence of FMT-related adverse events has been shown to be directly related to donor selection and closely related to transplantation methods (Li, 2020). Notably, the timing and composition of FMT are also topics of debate. Li et al. suggested that the future development direction should focus on personalized FMT based on donor–recipient precision microbiota analysis so that the current practice of whole fecal transplantation progresses to precision transplantation treatment using specific gut microbiota (Li, 2020).
Concluding remarks
The interaction between the gut and kidney remains an interesting and unsolved mystery. During sepsis, the gut microbiota and intestinal mucosal barrier are destroyed, and the effects of alteration on renal function are double-edged. Renal function, in turn, affects the gut microbiota. Currently, the role of the gut–kidney axis in the occurrence and development of sepsis is poorly understood. Therefore, large-scale, well-controlled translation and preclinical studies are needed to evaluate the changes in the gut–kidney axis during sepsis at different time windows. SA-AKI treatment strategies based on the gut microbiota have been studied. In this regard, rational therapies, which theoretically give full consideration to the positive effects of the gut microbiota on the kidney while restoring intestinal integrity and maintaining the homeostasis of the gut microbiota, represent an exciting way to fight critically ill diseases.
Author contributions
YX, XK, and YZ designed the systematic review. JX, HM, and JL conducted the literature search and assessed data quality. YX and XK drafted the manuscript and figures. JZ and XZ critically revised the review. All authors approved the final submitted version for publication.
Funding
This work was supported by generous funding from the Xinhua Hospital Development Fund.
Conflict of interest
The authors declare that the research was conducted in the absence of any commercial or financial relationships that could be construed as a potential conflict of interest.
Publisher’s note
All claims expressed in this article are solely those of the authors and do not necessarily represent those of their affiliated organizations, or those of the publisher, the editors and the reviewers. Any product that may be evaluated in this article, or claim that may be made by its manufacturer, is not guaranteed or endorsed by the publisher.
Supplementary material
The Supplementary Material for this article can be found online at: https://www.frontiersin.org/articles/10.3389/fmicb.2022.985283/full#supplementary-material
Supplementary Figure 1 | Flow chart of literature selection process. Studies were included if they met the following criteria: (i) it was a randomized controlled trial targeting the gut microbiota of sepsis patients; (ii) all subjects were free from the original heart, brain, endocrine, and other basic diseases; (iii) species identification was performed by DNA sequencing of 16S rDNA; (iv) reported more than one of the following primary outcome parameters: richness, abundance, evenness, α-diversity, or compositional dissimilarity (β-diversity). We did not include studies that were non-clinical (experimental and basic studies), observational, or retrospective. Study protocols, review articles, abstracts, editorials, and animal studies were excluded.
Abbreviations
SA-AKI, sepsis-associated acute kidney injury; SCr, serum creatinine; ARC, augmented renal clearance; GFR, glomerular filtration rate; SIRS, systemic inflammatory response syndrome; LPS, lipopolysaccharide; CLP, cecal ligation and puncture; ICU, intensive care unit; SCFAs, short-chain fatty acids; I/R, renal ischemia–reperfusion; MLCK, myosin light chain kinase; Th17, T-helper 17; IS, indoxyl sulfate; RFR, renal function reserve; OR, odds ratio; CI, confidence interval; FMT, fecal microbiota transplantation; RBF, renal blood flow; SDD, selective digestive tract decontamination; CKD, chronic kidney disease; Stx2, Shiga toxin 2.
References
Abbasi, J. (2019). Are probiotics money down the toilet? Or worse? JAMA 321, 633–635. doi: 10.1001/jama.2018.20798
Akash, M. S. H., Fiayyaz, F., Rehman, K., Sabir, S., and Rasool, M. H. (2019). Gut microbiota and metabolic disorders: Advances in therapeutic interventions. Crit. Rev. Immunol. 39, 223–237. doi: 10.1615/CritRevImmunol.2019030614
Anderberg, S. B., Luther, T., and Frithiof, R. (2017). Physiological aspects of Toll-like receptor 4 activation in sepsis-induced acute kidney injury. Acta Physiol. (Oxf). 219, 573–588. doi: 10.1111/apha.12798
Andrade-Oliveira, V., Amano, M. T., Correa-Costa, M., Castoldi, A., Felizardo, R. J., de Almeida, D. C., et al. (2015). Gut bacteria products prevent AKI induced by ischemia-reperfusion. J. Am. Soc. Nephrol. 26, 1877–1888. doi: 10.1681/ASN.2014030288
Andriamihaja, M., Davila, A. M., Eklou-Lawson, M., Petit, N., Delpal, S., Allek, F., et al. (2010). Colon luminal content and epithelial cell morphology are markedly modified in rats fed with a high-protein diet. Am. J. Physiol. Gastrointest. Liver Physiol. 299, G1030–G1037. doi: 10.1152/ajpgi.00149.2010
Antushevich, H. (2020). Fecal microbiota transplantation in disease therapy. Clin. Chim. Acta 503, 90–98. doi: 10.1016/j.cca.2019.12.010
Assimakopoulos, S. F., Papadopoulou, I., Bantouna, D., de Lastic, A. L., Rodi, M., Mouzaki, A., et al. (2021). Fecal Microbiota transplantation and hydrocortisone ameliorate intestinal barrier dysfunction and improve survival in a rat model of cecal ligation and puncture-induced sepsis. Shock 55, 666–675. doi: 10.1097/SHK.0000000000001566
Baptista, J. P., Sousa, E., Martins, P. J., and Pimentel, J. M. (2012). Augmented renal clearance in septic patients and implications for vancomycin optimisation. Int. J. Antimicrob. Agents 39, 420–423. doi: 10.1016/j.ijantimicag.2011.12.011
Bassetti, M., Bandera, A., and Gori, A. (2020). Therapeutic potential of the gut microbiota in the management of sepsis. Crit. Care 24:105. doi: 10.1186/s13054-020-2780-3
Belcher, J. M., Edelstein, C. L., and Parikh, C. R. (2011). Clinical applications of biomarkers for acute kidney injury. Am. J. Kidney Dis. 57, 930–940. doi: 10.1053/j.ajkd.2010.11.032
Ben-Shaanan, T. L., Azulay-Debby, H., Dubovik, T., Starosvetsky, E., Korin, B., Schiller, M., et al. (2016). Activation of the reward system boosts innate and adaptive immunity. Nat. Med. 22, 940–944. doi: 10.1038/nm.4133
Bilbao-Meseguer, I., Rodríguez-Gascón, A., Barrasa, H., Isla, A., and Solinís, M. Á (2018). Augmented renal clearance in critically Ill patients: A systematic review. Clin. Pharmacokinet. 57, 1107–1121. doi: 10.1007/s40262-018-0636-7
Bosch, J. P., Saccaggi, A., Lauer, A., Ronco, C., Belledonne, M., and Glabman, S. (1983). Renal functional reserve in humans. Effect of protein intake on glomerular filtration rate. Am. J. Med. 75, 943–950. doi: 10.1016/0002-9343(83)90873-2
Bouglé, A., and Duranteau, J. (2011). Pathophysiology of sepsis-induced acute kidney injury: the role of global renal blood flow and renal vascular resistance. Contrib. Nephrol. 174, 89–97. doi: 10.1159/000329243
Capaldo, C. T., Powell, D. N., and Kalman, D. (2017). Layered defense: how mucus and tight junctions seal the intestinal barrier. J. Mol. Med. (Berl). 95, 927–934. doi: 10.1007/s00109-017-1557-x
Carrié, C., Petit, L., d’Houdain, N., Sauvage, N., Cottenceau, V., Lafitte, M., et al. (2018). Association between augmented renal clearance, antibiotic exposure and clinical outcome in critically ill septic patients receiving high doses of β-lactams administered by continuous infusion: a prospective observational study. Int. J. Antimicrob. Agents 51, 443–449. doi: 10.1016/j.ijantimicag.2017.11.013
Chen, D. C. (2016). Gut microbiota and intestinal decolonization of pathogenic microorganisms. Chin. Med. J. (Engl). 129, 1639–1642. doi: 10.4103/0366-6999.185872
Chen, L., Li, H., Li, J., Chen, Y., and Yang, Y. (2019). Lactobacillus rhamnosus GG treatment improves intestinal permeability and modulates microbiota dysbiosis in an experimental model of sepsis. Int. J. Mol. Med. 43, 1139–1148. doi: 10.3892/ijmm.2019.4050
Christofi, T., Panayidou, S., Dieronitou, I., Michael, C., and Apidianakis, Y. (2019). Metabolic output defines Escherichia coli as a health-promoting microbe against intestinal Pseudomonas aeruginosa. Sci. Rep. 9:14463. doi: 10.1038/s41598-019-51058-3
Cook, A. M., and Hatton-Kolpek, J. (2019). Augmented renal clearance. Pharmacotherapy 39, 346–354. doi: 10.1002/phar.2231
De Waele, J. J., Dumoulin, A., Janssen, A., and Hoste, E. A. (2015). Epidemiology of augmented renal clearance in mixed ICU patients. Minerva Anestesiol. 81, 1079–1085.
Deshpande, A., Pasupuleti, V., Thota, P., Pant, C., Rolston, D. D., Hernandez, A. V., et al. (2015). Risk factors for recurrent Clostridium difficile infection: a systematic review and meta-analysis. Infect. Control Hosp. Epidemiol. 36, 452–460. doi: 10.1017/ice.2014.88
Devlin, A. S., Marcobal, A., Dodd, D., Nayfach, S., Plummer, N., Meyer, T., et al. (2016). Modulation of a circulating uremic solute via rational genetic manipulation of the gut microbiota. Cell Host Microbe 20, 709–715. doi: 10.1016/j.chom.2016.10.021
Dias, C., Gaio, A. R., Monteiro, E., Barbosa, S., Cerejo, A., Donnelly, J., et al. (2015). Kidney-brain link in traumatic brain injury patients? A preliminary report. Neurocrit. Care 22, 192–201. doi: 10.1007/s12028-014-0045-1
Dickson, R. P. (2016). The microbiome and critical illness. Lancet Respir. Med. 4, 59–72. doi: 10.1016/S2213-2600(15)00427-0
Druml, W. (2014). Systemic consequences of acute kidney injury. Curr. Opin. Crit. Care 20, 613–619. doi: 10.1097/MCC.0000000000000150
Du, B., Shen, N., Tao, Y., Sun, S., Zhang, F., Ren, H., et al. (2021). Analysis of gut microbiota alteration and application as an auxiliary prognostic marker for sepsis in children: a pilot study. Transl. Pediatr. 10, 1647–1657. doi: 10.21037/tp-21-51
Emal, D., Rampanelli, E., Stroo, I., Butter, L. M., Teske, G. J., Claessen, N., et al. (2017). Depletion of gut microbiota protects against renal ischemia-reperfusion injury. J. Am. Soc. Nephrol. 28, 1450–1461. doi: 10.1681/ASN.2016030255
Firmino, F. C., Porcellato, D., Cox, M., Suen, G., Broadbent, J. R., and Steele, J. L. (2020). Characterization of microbial communities in ethanol biorefineries. J. Ind. Microbiol. Biotechnol. 47, 183–195. doi: 10.1007/s10295-019-02254-7
Franceschi, F., Ojetti, V., Candelli, M., Covino, M., Cardone, S., Potenza, A., et al. (2019). Microbes and Alzheimer’ disease: lessons from H. pylori and GUT microbiota. Eur. Rev. Med. Pharmacol. Sci. 23, 426–430. doi: 10.26355/eurrev_201901_16791
Gao, X., Hao, S., Yan, H., Ding, W., Li, K., and Li, J. (2015). Neutrophil extracellular traps contribute to the intestine damage in endotoxemic rats. J. Surg. Res. 195, 211–218. doi: 10.1016/j.jss.2014.12.019
Gong, J., Noel, S., Pluznick, J. L., Hamad, A. R. A., and Rabb, H. (2019). Gut microbiota-kidney cross-talk in acute kidney injury. Semin Nephrol. 39, 107–116. doi: 10.1016/j.semnephrol.2018.10.009
Grootaert, V., Willems, L., Debaveye, Y., Meyfroidt, G., and Spriet, I. (2012). Augmented renal clearance in the critically ill: how to assess kidney function. Ann. Pharmacother. 46, 952–959. doi: 10.1345/aph.1Q708
Hatakeyama, S., Yamamoto, H., Okamoto, A., Imanishi, K., Tokui, N., Okamoto, T., et al. (2012). Effect of an oral adsorbent, AST-120, on dialysis initiation and survival in patients with chronic kidney disease. Int. J. Nephrol. 2012:376128. doi: 10.1155/2012/376128
Hato, T., and Dagher, P. C. (2015). How the innate immune system senses trouble and causes trouble. Clin. J. Am. Soc. Nephrol. 10, 1459–1469. doi: 10.2215/CJN.04680514
Hayakawa, M., Asahara, T., Henzan, N., Murakami, H., Yamamoto, H., Mukai, N., et al. (2011). Dramatic changes of the gut flora immediately after severe and sudden insults. Dig. Dis. Sci. 56, 2361–2365. doi: 10.1007/s10620-011-1649-3
Holeček, M. (2022). Serine metabolism in health and disease and as a conditionally essential amino acid. Nutrients 14:1987. doi: 10.3390/nu14091987
Huang, H. C., Tsai, M. H., Chang, C. C., Pun, C. K., Huang, Y. H., Hou, M. C., et al. (2021). Microbiota transplants from feces or gut content attenuated portal hypertension and portosystemic collaterals in cirrhotic rats. Clin. Sci. (Lond). 135, 2709–2728. doi: 10.1042/CS20210602
Hudson, L. E., Anderson, S. E., Corbett, A. H., and Lamb, T. J. (2017). Gleaning insights from fecal microbiota transplantation and probiotic studies for the rational design of combination microbial therapies. Clin. Microbiol. Rev. 30, 191–231. doi: 10.1128/CMR.00049-16
Johansson, M. E., Jakobsson, H. E., Holmén-Larsson, J., Schütte, A., Ermund, A., Rodríguez-Piñeiro, A. M., et al. (2015). Normalization of host intestinal mucus layers requires long-term microbial colonization. Cell Host Microbe 18, 582–592. doi: 10.1016/j.chom.2015.10.007
Krebs, C. F., Paust, H. J., Krohn, S., Koyro, T., Brix, S. R., Riedel, J. H., et al. (2016). Autoimmune renal disease is exacerbated by S1P-receptor-1-dependent intestinal Th17 cell migration to the kidney. Immunity 45, 1078–1092. doi: 10.1016/j.immuni.2016.10.020
Kullberg, R. F. J., Wiersinga, W. J., and Haak, B. W. (2021). Gut microbiota and sepsis: from pathogenesis to novel treatments. Curr. Opin. Gastroenterol. 37, 578–585. doi: 10.1097/MOG.0000000000000781
Langenberg, C., Bellomo, R., May, C., Wan, L., Egi, M., and Morgera, S. (2005). Renal blood flow in sepsis. Crit. Care 9, R363–R374. doi: 10.1186/cc3540
Lankelma, J. M., Cranendonk, D. R., Belzer, C., de Vos, A. F., de Vos, W. M., van der Poll, T., et al. (2017a). Antibiotic-induced gut microbiota disruption during human endotoxemia: a randomised controlled study. Gut 66, 1623–1630. doi: 10.1136/gutjnl-2016-312132
Lankelma, J. M., van Vught, L. A., Belzer, C., Schultz, M. J., van der Poll, T., de Vos, W. M., et al. (2017b). Critically ill patients demonstrate large interpersonal variation in intestinal microbiota dysregulation: a pilot study. Intensive Care Med. 43, 59–68. doi: 10.1007/s00134-016-4613-z
Lee, Y., Kim, M. H., Alves, D. R., Kim, S., Lee, L. P., Sung, J. H., et al. (2021). Gut-kidney axis on chip for studying effects of antibiotics on risk of hemolytic uremic syndrome by Shiga Toxin-producing Escherichia coli. Toxins (Basel) 13:775. doi: 10.3390/toxins13110775
Li, B., Yin, G. F., Wang, Y. L., Tan, Y. M., Huang, C. L., and Fan, X. M. (2020). Impact of fecal microbiota transplantation on TGF-β1/Smads/ERK signaling pathway of endotoxic acute lung injury in rats. 3 Biotech. 10:52. doi: 10.1007/s13205-020-2062-4
Li, J., Moturi, K. R., Wang, L., Zhang, K., and Yu, C. (2019). Gut derived-endotoxin contributes to inflammation in severe ischemic acute kidney injury. BMC Nephrol. 20:16. doi: 10.1186/s12882-018-1199-4
Li, N. (2020). Practice and consideration of fecal microbiota transplantation. Chin. J. Gastrointest. Surg. Suppl. 23, 1–4. doi: 10.3760/cma.j.cn.441530-20200420-00230
Li, X. J., You, X. Y., Wang, C. Y., Li, X. L., Sheng, Y. Y., Zhuang, P. W., et al. (2020). Bidirectional brain-gut-microbiota axis in increased intestinal permeability induced by central nervous system injury. CNS Neurosci. Ther. 26, 783–790. doi: 10.1111/cns.13401
Liu, J., Wang, M., Chen, W., Ma, J., Peng, Y., Zhang, M., et al. (2021). Altered gut microbiota taxonomic compositions of patients with sepsis in a pediatric intensive care unit. Front. Pediatr. 9:645060. doi: 10.3389/fped.2021.645060
Liu, W., Cheng, M., Li, J., Zhang, P., Fan, H., Hu, Q., et al. (2020). Classification of the gut microbiota of patients in intensive care units during development of sepsis and septic shock. Genomics Proteomics Bioinformatics 18, 696–707. doi: 10.1016/j.gpb.2020.06.011
Liu, Z., Li, N., Fang, H., Chen, X., Guo, Y., Gong, S., et al. (2019). Enteric dysbiosis is associated with sepsis in patients. FASEB J. 33, 12299–12310. doi: 10.1096/fj.201900398RR
Lorentz, C. A., Liang, Z., Meng, M., Chen, C. W., Yoseph, B. P., Breed, E. R., et al. (2017). Myosin light chain kinase knockout improves gut barrier function and confers a survival advantage in polymicrobial sepsis. Mol. Med. 23, 155–165. doi: 10.2119/molmed.2016.00256
Luo, Y., Wang, Y., Ma, Y., Wang, P., Zhong, J., and Chu, Y. (2021). Augmented renal clearance: What have we known and what will we do? Front. Pharmacol. 12:723731. doi: 10.3389/fphar.2021.723731
Machado, R. A., Constantino Lde, S., Tomasi, C. D., Rojas, H. A., Vuolo, F. S., Vitto, M. F., et al. (2012). Sodium butyrate decreases the activation of NF-κB reducing inflammation and oxidative damage in the kidney of rats subjected to contrast-induced nephropathy. Nephrol. Dial. Transplant. 27, 3136–3140. doi: 10.1093/ndt/gfr807
Mahmoud, S. H., and Shen, C. (2017). Augmented renal clearance in critical illness: An important consideration in drug dosing. Pharmaceutics 9:36. doi: 10.3390/pharmaceutics9030036
Maiden, M. J., Otto, S., Brealey, J. K., Finnis, M. E., Chapman, M. J., Kuchel, T. R., et al. (2016). Structure and function of the kidney in septic shock. A prospective controlled experimental study. Am. J. Respir. Crit. Care Med. 194, 692–700. doi: 10.1164/rccm.201511-2285OC
Margolis, K. G., Cryan, J. F., and Mayer, E. A. (2021). The microbiota-gut-brain axis: From motility to mood. Gastroenterology 160, 1486–1501. doi: 10.1053/j.gastro.2020.10.066
Meijers, B. K., and Evenepoel, P. (2011). The gut-kidney axis: indoxyl sulfate, p-cresyl sulfate and CKD progression. Nephrol. Dial. Transplant. 26, 759–761. doi: 10.1093/ndt/gfq818
Meng, M., Klingensmith, N. J., and Coopersmith, C. M. (2017). New insights into the gut as the driver of critical illness and organ failure. Curr. Opin. Crit. Care 23, 143–148. doi: 10.1097/MCC.0000000000000386
Miller, W. D., Keskey, R., and Alverdy, J. C. (2021). Sepsis and the microbiome: A vicious cycle. J. Infect. Dis. 223(12 Suppl. 2), S264–S269. doi: 10.1093/infdis/jiaa682
Nakade, Y., Iwata, Y., Furuichi, K., Mita, M., Hamase, K., Konno, R., et al. (2018). Gut microbiota-derived D-serine protects against acute kidney injury. JCI Insight 3:e97957. doi: 10.1172/jci.insight.97957
Ng, K. M., Aranda-Díaz, A., Tropini, C., Frankel, M. R., Van Treuren, W., O’Loughlin, C. T., et al. (2019). Recovery of the gut microbiota after antibiotics depends on host diet, community context, and environmental reservoirs. Cell Host Microbe 26, 650–665.e4. doi: 10.1016/j.chom.2019.10.011
Nie, C., Li, Y., Li, R., Yan, Y., Zhang, D., Li, T., et al. (2022). Distinct biological ages of organs and systems identified from a multi-omics study. Cell Rep. 38:110459. doi: 10.1016/j.celrep.2022.110459
Niu, M., and Chen, P. (2021). Crosstalk between gut microbiota and sepsis. Burns Trauma 9:tkab036. doi: 10.1093/burnst/tkab036
Ojima, M., Motooka, D., Shimizu, K., Gotoh, K., Shintani, A., Yoshiya, K., et al. (2016). Metagenomic analysis reveals dynamic changes of whole gut microbiota in the acute phase of intensive care unit patients. Dig. Dis. Sci. 61, 1628–1634. doi: 10.1007/s10620-015-4011-3
Pagan, J. D., Kitaoka, M., and Anthony, R. M. (2018). Engineered sialylation of pathogenic antibodies in vivo attenuates autoimmune disease. Cell 172, 564–577.e13. doi: 10.1016/j.cell.2017.11.041
Pahl, M. V., and Vaziri, N. D. (2015). The chronic kidney disease – colonic axis. Semin Dial. 28, 459–463. doi: 10.1111/sdi.12381
Paone, P., and Cani, P. D. (2020). Mucus barrier, mucins and gut microbiota: the expected slimy partners? Gut 69, 2232–2243. doi: 10.1136/gutjnl-2020-322260
Parkar, S. G., Kalsbeek, A., and Cheeseman, J. F. (2019). Potential role for the gut microbiota in modulating host circadian rhythms and metabolic health. Microorganisms 7:41. doi: 10.3390/microorganisms7020041
Peng, Q., Zhang, L., Ai, Y., and Zhang, L. (2014). Epidemiology of acute kidney injury in intensive care septic patients based on the KDIGO guidelines. Chin. Med. J. (Engl). 127, 1820–1826.
Quan, R., Chen, C., Yan, W., Zhang, Y., Zhao, X., and Fu, Y. B. A. F. F. (2020). Blockade attenuates inflammatory responses and intestinal barrier dysfunction in a murine endotoxemia model. Front. Immunol. 11:570920. doi: 10.3389/fimmu.2020.570920
Ramakrishna, B. S. (2013). Role of the gut microbiota in human nutrition and metabolism. J. Gastroenterol. Hepatol. 28(Suppl. 4), 9–17. doi: 10.1111/jgh.12294
Ranganathan, N., Ranganathan, P., Friedman, E. A., Joseph, A., Delano, B., Goldfarb, D. S., et al. (2010). Pilot study of probiotic dietary supplementation for promoting healthy kidney function in patients with chronic kidney disease. Adv. Ther. 27, 634–647. doi: 10.1007/s12325-010-0059-9
Reyman, M., van Houten, M. A., Watson, R. L., Chu, M. L. J. N., Arp, K., de Waal, W. J., et al. (2022). Effects of early-life antibiotics on the developing infant gut microbiome and resistome: a randomized trial. Nat. Commun. 13:893. doi: 10.1038/s41467-022-28525-z
Ronco, C., Bellomo, R., and Kellum, J. (2017). Understanding renal functional reserve. Intensive Care Med. 43, 917–920. doi: 10.1007/s00134-017-4691-6
Sasabe, J., Miyoshi, Y., Rakoff-Nahoum, S., Zhang, T., Mita, M., Davis, B. M., et al. (2016). Interplay between microbial d-amino acids and host d-amino acid oxidase modifies murine mucosal defence and gut microbiota. Nat. Microbiol. 1:16125. doi: 10.1038/nmicrobiol.2016.125
Sharma, A., Mucino, M. J., and Ronco, C. (2014). Renal functional reserve and renal recovery after acute kidney injury. Nephron Clin. Pract. 127, 94–100. doi: 10.1159/000363721
Shimizu, K., Yamada, T., Ogura, H., Mohri, T., Kiguchi, T., Fujimi, S., et al. (2018). Synbiotics modulate gut microbiota and reduce enteritis and ventilator-associated pneumonia in patients with sepsis: a randomized controlled trial. Crit. Care 22:239. doi: 10.1186/s13054-018-2167-x
Si, J., Ge, Y., Zhuang, S., Wang, L. J., Chen, S., and Gong, R. (2013). Adrenocorticotropic hormone ameliorates acute kidney injury by steroidogenic-dependent and –independent mechanisms. Kidney Int. 83, 635–646. doi: 10.1038/ki.2012.447
Skye, S. M., and Hazen, S. L. (2016). Microbial modulation of a uremic toxin. Cell Host Microbe 20, 691–692. doi: 10.1016/j.chom.2016.11.005
Song, J. H., and Kim, Y. S. (2019). Recurrent Clostridium difficile infection: Risk factors, treatment, and prevention. Gut Liver 13, 16–24. doi: 10.5009/gnl18071
Stadlbauer, V., Horvath, A., Komarova, I., Schmerboeck, B., Feldbacher, N., Klymiuk, I., et al. (2019). Dysbiosis in early sepsis can be modulated by a multispecies probiotic: A randomised controlled pilot trial. Benef Microbes 10, 265–278. doi: 10.3920/BM2018.0067
Sun, T., Wang, L., and Zhang, H. (2022). Intestinal microbiota in sepsis. Intensive Care Res. 2, 1–7. doi: 10.1007/s44231-022-00001-8
Sun, X., Zhang, B., Hong, X., Zhang, X., and Kong, X. (2013). Histone deacetylase inhibitor, sodium butyrate, attenuates gentamicin-induced nephrotoxicity by increasing prohibitin protein expression in rats. Eur. J. Pharmacol. 707, 147–154. doi: 10.1016/j.ejphar.2013.03.018
Takeda, F., Oda, M., Terasaki, M., Kubota, A., Asada, K., Ichimura, Y., et al. (2021). Downregulated expression of organic anion transporting polypeptide (Oatp) 2b1 in the small intestine of rats with acute kidney injury. Drug Metab. Pharmacokinet. 40:100411. doi: 10.1016/j.dmpk.2021.100411
Tan, R., Chen, Z., Yang, D., Jin, M., Yin, J., Shi, D., et al. (2020). Study on intestinal inflammation induced by high protein diet mediated by intestinal flora in mice. J. Prev. Med. Chin. PLA. 38, 81–83. doi: 10.13704/j.cnki.jyyx.2020.10.028
Tan, R. Z., Diao, H., Li, J. C., Xia, Z., Xiao-Jia, W., Dan, W., et al. (2020). Astragalus mongholicus Bunge and Panax Notoginseng formula (A&P) combined with Bifidobacterium contribute a renoprotective effect in chronic kidney disease through inhibiting macrophage inflammatory response in kidney and intestine. Front. Physiol. 11:583668. doi: 10.3389/fphys.2020.583668
The Lancet Gastroenterology Hepatology (2019). Probiotics: elixir or empty promise? Lancet Gastroenterol. Hepatol. 4:81. doi: 10.1016/S2468-1253(18)30415-1
Thomas, D. M., Coles, G. A., and Williams, J. D. (1994). What does the renal reserve mean? Kidney Int. 45, 411–416. doi: 10.1038/ki.1994.53
Toden, S., Bird, A. R., Topping, D. L., and Conlon, M. A. (2005). Resistant starch attenuates colonic DNA damage induced by higher dietary protein in rats. Nutr. Cancer 51, 45–51. doi: 10.1207/s15327914nc5101_7
Traykova, D., Schneider, B., Chojkier, M., and Buck, M. (2017). Blood microbiome quantity and the hyperdynamic circulation in decompensated cirrhotic patients. PLoS One 12:e0169310. doi: 10.1371/journal.pone.0169310
Trof, R. J., Sukul, S. P., Twisk, J. W., Girbes, A. R., and Groeneveld, A. B. (2010). Greater cardiac response of colloid than saline fluid loading in septic and non-septic critically ill patients with clinical hypovolaemia. Intensive Care Med. 36, 697–701. doi: 10.1007/s00134-010-1776-x
Udy, A. A., Roberts, J. A., Boots, R. J., Paterson, D. L., and Lipman, J. (2010). Augmented renal clearance: implications for antibacterial dosing in the critically ill. Clin. Pharmacokinet. 49, 1–16. doi: 10.2165/11318140-000000000-00000
Vaishnavi, C. (2013). Translocation of gut flora and its role in sepsis. Indian J. Med. Microbiol. 31, 334–342. doi: 10.4103/0255-0857.118870
Vaziri, N. D., Yuan, J., Khazaeli, M., Masuda, Y., Ichii, H., and Liu, S. (2013). Oral activated charcoal adsorbent (AST-120) ameliorates chronic kidney disease-induced intestinal epithelial barrier disruption. Am. J. Nephrol. 37, 518–525. doi: 10.1159/000351171
Wan, Y. D., Zhu, R. X., Wu, Z. Q., Lyu, S. Y., Zhao, L. X., Du, Z. J., et al. (2018). Gut microbiota disruption in septic shock patients: A pilot study. Med. Sci. Monit. 24, 8639–8646. doi: 10.12659/MSM.911768
Wang, L., Chen, Q., Qi, H., Wang, C., Wang, C., Zhang, J., et al. (2016). Doxorubicin-induced systemic inflammation is driven by upregulation of toll-like receptor TLR4 and endotoxin leakage. Cancer Res. 76, 6631–6642. doi: 10.1158/0008-5472.CAN-15-3034
Wang, S., Xu, M., Wang, W., Cao, X., Piao, M., Khan, S., et al. (2016). Systematic review: Adverse events of fecal microbiota transplantation. PLoS One 11:e0161174. doi: 10.1371/journal.pone.0161174
Wu, I. W., Lin, C. Y., Chang, L. C., Lee, C. C., Chiu, C. Y., Hsu, H. J., et al. (2020). Gut microbiota as diagnostic tools for mirroring disease progression and circulating nephrotoxin levels in chronic kidney disease: Discovery and validation study. Int. J. Biol. Sci. 16, 420–434. doi: 10.7150/ijbs.37421
Wu, R. Y., Määttänen, P., Napper, S., Scruten, E., Li, B., Koike, Y., et al. (2017). Non-digestible oligosaccharides directly regulate host kinome to modulate host inflammatory responses without alterations in the gut microbiota. Microbiome 5:135. doi: 10.1186/s40168-017-0357-4
Wu, Y. Q., Cai, J. K., and Li, J. Y. (2001). Changes in plasma free amino acid concentrations in rats during sepsis. Chin. J. Surg. 39, 638–642. doi: 10.3760/j:issn:0529-5815.2001.08.020
Xiao, Y., Angulo, M. T., Lao, S., Weiss, S. C., and Liu, Y. L. (2020). An ecological framework to understand the efficacy of fecal microbiota transplantation. Nat. Commun. 11:3329. doi: 10.1038/s41467-020-17180-x
Xie, D., Jiang, L., Lin, Y., and Liu, Z. (2020). Antioxidant activity of selenium-enriched Chrysomyia megacephala (Fabricius) larvae powder and its impact on intestinal microflora in D-galactose induced aging mice. BMC Complement Med. Ther. 20:264. doi: 10.1186/s12906-020-03058-4
Yang, T., Richards, E. M., Pepine, C. J., and Raizada, M. K. (2018). The gut microbiota and the brain-gut-kidney axis in hypertension and chronic kidney disease. Nat. Rev. Nephrol. 14, 442–456. doi: 10.1038/s41581-018-0018-2
Yang, X. J., Liu, D., Ren, H. Y., Zhang, X. Y., Zhang, J., and Yang, X. J. (2021). Effects of sepsis and its treatment measures on intestinal flora structure in critical care patients. World J. Gastroenterol. 27, 2376–2393. doi: 10.3748/wjg.v27.i19.2376
Ye, H., Zhai, Q., and Fang, X. M. (2019). The role of ‘brain gut axis’ in the development of sepsis. Int. J. Anesth. Resus. 40, 490–493. doi: 10.3760/cma.j.issn.1673-4378.2019.05.016
Yeh, A., Rogers, M. B., Firek, B., Neal, M. D., Zuckerbraun, B. S., and Morowitz, M. J. (2016). Dysbiosis across multiple body sites in critically Ill adult surgical patients. Shock 46, 649–654. doi: 10.1097/SHK.0000000000000691
Yu, G., Liu, Q., Dong, X., Tang, K., Li, B., Liu, C., et al. (2018). Inhibition of inflammation using diacerein markedly improved renal function in endotoxemic acute kidney injured mice. Cell. Mol. Biol. Lett. 23:38. doi: 10.1186/s11658-018-0107-z
Yu, Y., and Zhao, F. (2021). Microbiota-gut-brain axis in autism spectrum disorder. J. Genet. Genomics 48, 755–762. doi: 10.1016/j.jgg.2021.07.001
Yuan, J. J., Chang, X. N., Li, M., Yuan, Q. X., and Yang, X. L. (2020). Clinical utility of characterizing intestinal flora in septic kidney injury. Chin. Med. J. (Engl). 133, 842–846. doi: 10.1097/CM9.0000000000000724
Zeng, X., McMahon, G. M., Brunelli, S. M., Bates, D. W., and Waikar, S. S. (2014). Incidence, outcomes, and comparisons across definitions of AKI in hospitalized individuals. Clin. J. Am. Soc. Nephrol. 9, 12–20. doi: 10.2215/CJN.02730313
Zhu, H., Cao, C., Wu, Z., Zhang, H., Sun, Z., Wang, M., et al. (2021). The probiotic L. casei Zhang slows the progression of acute and chronic kidney disease. Cell Metab. 33, 1926–1942.e8. doi: 10.1016/j.cmet.2021.06.0144
Zhu, H., Liu, Y., Li, S., Jin, Y., Zhao, L., Zhao, F., et al. (2018). Altered gut microbiota after traumatic splenectomy is associated with endotoxemia. Emerg. Microbes Infect. 7:197. doi: 10.1038/s41426-018-0202-2
Keywords: intestinal microbiology, augmented renal clearance, gut–kidney crosstalk, sepsis, gut micro flora
Citation: Xu Y, Kong X, Zhu Y, Xu J, Mao H, Li J, Zhang J and Zhu X (2022) Contribution of gut microbiota toward renal function in sepsis. Front. Microbiol. 13:985283. doi: 10.3389/fmicb.2022.985283
Received: 03 July 2022; Accepted: 16 August 2022;
Published: 06 September 2022.
Edited by:
Khalid Mehmood, Islamia University of Bahawalpur, PakistanReviewed by:
Blanda Di Luccia, Stanford University, United StatesWei Yan, National Institutes of Health, United States
Selçuk Yüksel, Pamukkale University, Turkey
Copyright © 2022 Xu, Kong, Zhu, Xu, Mao, Li, Zhang and Zhu. This is an open-access article distributed under the terms of the Creative Commons Attribution License (CC BY). The use, distribution or reproduction in other forums is permitted, provided the original author(s) and the copyright owner(s) are credited and that the original publication in this journal is cited, in accordance with accepted academic practice. No use, distribution or reproduction is permitted which does not comply with these terms.
*Correspondence: Jianhua Zhang, empoMTIxOTVAMTI2LmNvbQ==; Xiaodong Zhu, eGluaHVheGlhb2RvbmdAMTI2LmNvbQ==
†These authors have contributed equally to this work and share first authorship
‡These authors have contributed equally to this work and share co-corresponding authors