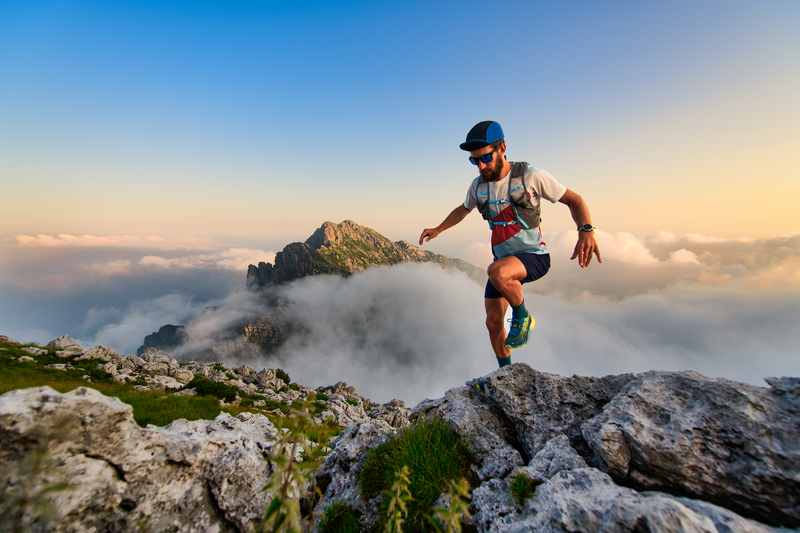
95% of researchers rate our articles as excellent or good
Learn more about the work of our research integrity team to safeguard the quality of each article we publish.
Find out more
REVIEW article
Front. Microbiol. , 19 October 2022
Sec. Terrestrial Microbiology
Volume 13 - 2022 | https://doi.org/10.3389/fmicb.2022.982611
This article is part of the Research Topic Recent advancements in microbe-pesticide interaction: A smart-soil bioremediation approach View all 18 articles
In the present scenario, the uncontrolled and irrational use of pesticides is affecting the environment, agriculture and livelihood worldwide. The excessive application of pesticides for better production of crops and to maintain sufficient food production is leading to cause many serious environmental issues such as soil pollution, water pollution and also affecting the food chain. The efficient management of pesticide use and remediation of pesticide-contaminated soil is one of the most significant challenges to overcome. The efficiency of the current methods of biodegradation of pesticides using different microbes and enzymes depends on the various physical and chemical conditions of the soil and they have certain limitations. Hence, a novel strategy is the need of the hour to safeguard the ecosystem from the serious environmental hazard. In recent years, the application of nanomaterials has drawn attention in many areas due to their unique properties of small size and increased surface area. Nanotechnology is considered to be a promising and effective technology in various bioremediation processes and provides many significant benefits for improving the environmental technologies using nanomaterials with efficient performance. The present article focuses on and discusses the role, application and importance of nano-bioremediation of pesticides and toxic pollutants to explore the potential of nanomaterials in the bioremediation of hazardous compounds from the environment.
The use of pesticides increased in Indian agriculture with the green revolution between years 1967 and 1972. The use of pesticides played an important role in increasing crop production, but on the other hand, it has raised several serious issues related to human and animal health. Pesticides have been used for protection from pests but the fact is that only 1% of pesticides used could target the pests and the remaining cause contamination of soil, water and air (Sun et al., 2018; Figure 1).
Pesticides used for crop protection not only integrate into the food chain but also affect the soil health by affecting the soil microbiome and its enzyme activity (Degeronimo, 2015). Moreover, ~40% of applied pesticides are converted into transformed products, which may remain in the soil for a longer period, even for a decade (Erinle et al., 2016). These transformed products contaminated groundwater via leaching (Robinson and Piatt, 2019). Pesticide residues entered into the food chain affect human health by targeting several organs for example they affect the endocrine system like the thyroid gland (Huang H.S. et al., 2017), cause neurological disorders (Schmidt et al., 2017), have direct cytotoxic effects (Erdoğmuş et al., 2015) and even may increase the rate of mutations in human beings (Teodoro et al., 2019).
Chlorpyrifos (Pesticide of the organophosphate group) has been reported to cause a reduction in children’s intelligence quotients (Sun et al., 2018). Pesticide residues also affect animal health and productivity. In human beings, pesticides cause health problems while in animals also, various disease conditions like cancer, immunosuppression (Nicolopoulou-Stamati et al., 2016), birth defects, hepatic and nephrotoxicity (Choudhary et al., 2018) have been reported in the farm as well as in wild animals. As pesticides target the endocrine system, several reproductive and fertility problems have been encountered in farm animals (Marlatt et al., 2022). Pesticides not only affect the female reproductive system (Ramakrishna et al., 2022) but also are detrimental to the male reproductive system, causing toxicity to the sperm plasma membrane (Torres-Badia et al., 2022). Pesticides have deleterious effects on the biodiversity of invertebrates, mainly insects have been observed in many countries in the last few decades (Vogel, 2017). Due to the indiscriminate use of pesticides, agricultural land used by winter migrating birds is reduced and resulting in a significant reduction in the bird population. Some pesticides like imidacloprid reduced the reproductive capacity and the survival rate of birds like the white crowned sparrow (Zonotrichia leucophrys; Eng et al., 2017). Adverse effects of pesticides like neonicotinoids were observed in bees, bumble bees and other useful insects (Phelps et al., 2020). Even the bats were affected due to an omnivorous diet and exposed to pesticide residual contamination (Oliveira et al., 2020). Due to a reduction in the population of bees, birds and beneficial insects, pesticides have made a tremendous economic impact on the environment (Ali et al., 2021) and according to a few estimates, this loss may be 100 times more than the money spent on the conservation of the global environment and biodiversity (Organization for Economic Co-operation and Development (OECD), 2019). Around 8,000 species of insects are at the risk of extinction and in some countries like Germany, the insect population has been reduced by 75% in the last three decades (Hallmann et al., 2017). Pesticides not only affected the natural habitats and biodiversity of places where they were used, but as an effect of wind and evaporation, they could reach the atmosphere and contaminate the sites which were located far away (De Neri, 2020). Various studies have concluded that the use of pesticides for a longer period may affect soil health by interacting with the microflora, microfauna, and macrofauna of the soil (Dahiya et al., 2022).
Keeping these facts in mind, essential measures should be taken for the remediation of pesticides from soil and the environment. In this article, we have focused and discussed the significant.
The objective of this review article is to highlight the importance, impact and significant applications of the nanomaterials in the bioremediation process, for the effective remediation of toxic pollutants such as pesticides and heavy metals from the environment.
The soil has its capacity for degradation of compounds used in the pesticides up to a certain extent but a high concentration of these compounds is toxic for the soil microflora involved in the bioremediation and therefore needs necessary interventions in this area (Cheng et al., 2016a). Several techniques based on physical, chemical and physicochemical principles have been used for the bioremediation of the soil (Baldissarelli et al., 2019).
Technique of advanced oxidative process has been used as a pretreatment or treatment technique. This technique works on the principle of oxidation of polluting compounds into water, carbon dioxide and inert compounds. Ambient temperature and pressure are the pre-requisites for this technique (Cheng et al., 2016b). Though this technique has shown promising potential, most of the studies have been conducted in laboratory conditions and need further modification for scale-up in field conditions. Economic aspects of the application of this technique are also yet to be studied (Morillo and Villaverde, 2017). There are several variations of an advanced oxidative process like Fenton’s reaction, photocatalysis, plasma oxidation and ozonization, which have been tested for soil bioremediation.
This process is based on the oxidation of iron ions (Fe2+) in a medium containing hydrogen peroxide to produce a reactive hydroxyl radical. Hydroxyl radicals further oxidize the organic pollutants into less harmful compounds. Apart from hydrogen peroxide, some other reagents like permanganate (MnO4−), persulfate (S2O8−) and ozone have also been used but each reagent exhibited its own merits and demerits (Cao et al., 2013; Cheng et al., 2016a). This technique has a few advantages as it may be used in situ (onsite) or ex situ (offsite) as well as it is environment friendly and easy to operate (Cao et al., 2013) but the application of this technique reduces the pH of the soil (pH < 4), which may affect the soil microbiome. Fenton’s method has mainly used an aqueous media for the treatment of groundwater or wastewater (Rosas et al., 2014). For the degradation of chlorinated pesticides like DDT (Dichlorodiphenyltrichloroethane), Fenton’s method was used in combination with other oxidative systems like zero valence ion, Ethylene diamine tetraacetic acid, air (ZVI/EDTA/Air; Cao et al., 2013) with the amino ZVI/Air system (Zhou et al., 2014) and with trisodium citrate (Vicente et al., 2012). Though the variety and efficiency of Fenton’s reaction to degrade contaminants and pollutant have shown promising potential but the studies using this technique for mass soil contaminants treatment is still the same (Baldissarelli et al., 2019).
In this technique, metal oxides like titanate and zinc oxide are based as photosensitizers in the photo induce process. As they have semi filled valence band structure, they cause displacement of electrons from hydroxyl radicals of pollutant compounds (Santos et al., 2015). The efficacy of this technique depends on several factors like soil morphology, surface, pH, particle size, soil depth and light intensity (Castro et al., 2016). Though the reuse of these metal oxides is laborious yet this technique has been demonstrated by some workers in their studies (Sharma et al., 2015).
Plasma oxidation is a technique of production of electrons by providing energy and space for reactive molecules. This technique may be used for the oxidation of various compounds like hydrocarbons and pesticides (Cheng et al., 2016a) but the requirement of a high energy source is a limitation of this technology. Therefore, some modifications were made to this technique and with low energy consumption; technique was used for remediation of non-miscible liquid pesticides from the soil in a short reaction time (Aggelopoulos et al., 2015). This technique has been used for the remediation of various pollutants like Pentachlorophenol (Barjasteh et al., 2021), pentanitrophenol and glyphosate (Wang et al., 2014).
The technique of soil washing was used as a physical method, chemical method, or a combination of the physical and chemical methods for the treatment of organic and inorganic contaminants (Usman et al., 2022). This technique was found more effective in soil having high permeability means containing a good ratio of sand and gravel (>50%; Morillo and Villaverde, 2017). Though the soil washing technique was used effectively, its application resulted in the production of wash solution containing a high concentration of diverse pollutants and xenobiotics. Soil washing was tested in a combination with a few other techniques like Fenton oxidation to increase the efficacy of soil washing. A combination of soil washing (Sodium dodecyl sulfate as surfactant) and electrolysis was tested to remove residual pesticides (Atrazine; dos Santos et al., 2015), β methyl cyclodextrin (MCD) in combination with sunflower oil was used to remove the organochlorine pesticides from the soil (Ye et al., 2014).
The technique is based on the extraction of soil contaminants by using supercritical fluid. Extraction using a solvent system like methanol has high solvency and recovery. In this method, carbon dioxide is passed through contaminated soil and assist solubilization of toxic compounds in a solvent system like methanol (Bielská et al., 2013). The solvent system is having high solvency, and may recover a wide variety of pollutants. Though the efficacy of removal of pollutants depends on several factors like the type of extraction, and soil properties (pH, organic content, etc.). In a selective extraction system, several organic compounds were extracted using carbon dioxide as an extraction fluid (Bielská et al., 2013). In an alternative method, Forero-Mendieta et al. (2012) used a combination of carbon dioxide and methanol as a solvent and co-solvent system and remove 31 pesticides like Iprodione, tetradifon, and acephate with an efficacy of more than 70%. Supercritical fluid extraction has been used in combination with dispersive liquid–liquid micro extraction (DLLM) to detect organophosphorus pesticides like Thionazin, Sulfotep, and disulfoton in the soil. The pesticide removal was reached up to 95% with supercritical CO2 under the conditions of 150 bar, 60°C, 10 min of static extraction and 30 min of dynamic extraction (Naeeni et al., 2011). Veterinary pharmaceutical products and fungicides were also detected and recovered from the soil by using the solvent extraction technique (Chitescu et al., 2012). A combination of soil washing techniques with solvent extraction methods could remove DDT from the soil up to 94% (Mao et al., 2013).
Electrokinetics, electrokinetic soil processing, or electromigration involves the application of a continuous and low-intensity current between the electrodes in the soil. Electric current causes electrolysis of soil, water and makes acidic solutions close to the anode. One of the acid fronts moves from the anode to the cathode, which results in the desorption of soil contaminants (Gomes et al., 2014). This technique results in the movement of soil pollutants and their concentration in a small area (Bocos et al., 2015). This method causes minimum disturbance in the soil environment and is economically feasible. This technique involves two processes; electromigration causes the removal of polar contaminants (ions) while electroosmosis removes non-polar contaminants (dos Santos et al., 2016). This technique is mainly used in fine granulometry soils, which have low hydraulic conductivity and large specific surface area (Morillo and Villaverde, 2017). The pesticides like Molinate and Bentazone were removed from the soil by the process of Electrokinetics, molinate was removed as catholyte while bentazone was found to be present on both of the electrodes (Ribeiro et al., 2011) but for the removal of the compounds like Pentachlorophenol (PCP), electrokinetics was not found to be very efficient technique and required techniques like permeable reactive barriers for efficient removal (Li et al., 2011). Electrokinetics could efficiently remove the commonly used pesticides 2, 4 D (2,4-dichlorophenoxyacetic acid) from the soil and reduced the concentration of 2,4 D in soil up to 80% within 60 days of treatment (Risco et al., 2015). Different configurations of electrodes have been tested to increase the efficiency of electrokinetics among which the formation of one anode and six cathodes was found more efficient than one cathode and six anodes (Risco et al., 2016). Solar power energies and wind energies were used as an alternative to conventional electric energy sources for the electrode to reduce the cost of the operation (Souza et al., 2016).
Among all the physiochemical processes described advanced oxidation process especially the Fenton technique may be considered to be the best method as it may be used for the bioremediation of a wide range of contaminants including many organic contaminants, it can be used in the area of contaminants or out of it. It is environmentally friendly and requires a shorter treatment time. It can be operated easily with low operation costs (Baldissarelli et al., 2019). But even Fenton oxidation suffers from a few limitations as it causes a reduction in soil pH, oxidizes harmless organic material of the soil and immobilization Fenton technique of inorganic reactive species on the treatment wall (Cheng et al., 2016a). Alternative techniques such as biodegradation, and using microorganisms have been used for effective decontamination of soil and water.
Pesticides can be classified by several means depending on the origin of the Pesticide, chemical properties, and pest controlling capacities. Based on their origin, pesticides may be classified into two groups; biopesticides and chemical pesticides. Biopesticides may further be divided into three subgroups, i.e., microbial, biochemical and plant-incorporated protectants. Chemical pesticides can be classified based on their nature (organic and inorganic) and based on ionization properties (ionic and non-ionic). In organic and inorganic based classification, inorganic pesticides are mainly mineral derivatives while organic pesticides may be divided further into four groups; synthetic, plant based, animal based and microorganism based. Synthetic organic pesticides are of three types; organophosphates (like chlorpyrifos), organochlorine (like Lindane) and carbamates (like carbaryl). Plant-based organic pesticides can be divided into two subgroups; synthetic (like Allethrins) and natural (like Nicotine). Animal-based organic pesticides can be divided into two groups; synthetic (Fish oil) and natural (dried blood). Microorganism-based organic pesticides are of three types; bacterial (Bacillus thuringiensis), fungal (Pseudozyma flocculus) and viral (Baculovirus; Giri et al., 2021). Based on the classification of their ionization properties, pesticides can be divided into two groups; ionic and non-ionic pesticides. Ionic pesticides are divided into four groups, cationic (like Chlormequat, Diquat), basic (like Atrazine, Cyanazine), acidic (like Laxynil, Fenae) and miscellaneous (like Cacodylic acid, Terbacil). Non-ionic pesticides can be classified into several subgroups, chlorinated HCs (like DDT, Lindane), organophosphates (Ethion, Methyl Parathion), Dinitroanilines (like Oryzalin, Nitralin), Carbanilate (like Chlorpropham, Swep, Barban), Benzonitrile (like Dichlobenil), Ester (eg methyl ester of Chloramine), Acetamides (like CCDA), Carbothioate (like Molinate), Thiocarbamates (Metam and Ferbam), Anilides (Alachlor, Propanil), Urea (cycluron) and Methyl carbamates (carbaryl dichromate, Terbutol; Giri et al., 2021).
The term bioremediation deals with the methods of degradation of pesticides by using the metabolic capacities of microbes. In this process, natural or genetically modified microbes utilize pesticides for their metabolic activities and convert them into environmentally benign metabolites (Karimi et al., 2022). The process of bioremediation can be classified into two groups, i.e., Bio-stimulation and Bio-augmentation.
In the process of biostimulation, microbial activity is enhanced by the addition of vitamins, substrate, oxygen and other nutrients. The addition of stimulatory nutrients results in swift depletion of the available stock of inorganic nutrient and result in pesticide degradation (Giri et al., 2021). To stimulate the process of biostimulation by microbes, water soluble nutrients like sodium nitrate, potassium nitrate, and potassium hydrogen phosphate have been added to the fertilizer (Adams et al., 2015). The ratio of Nitrogen: Phosphorous is maintained between 1:5 and 1:10 for 1%–5% N by weight of pesticide for the degradation of pesticides but if the site has been contaminated with the different types of pesticides. This ratio may not be sufficient enough for the biostimulation process. Fernandes et al. (2018) used Pseudomonas species for the degradation of atrazine in the soil at very high concentrations. For biostimulation, citrate at 4.8 mg/g of soil was used. The addition of citrate stimulated the bioremediation process and the efficiency of removal of atrazine was found to be 79.00 to 87%.
Bio-augmentation involves the addition of an exogenous micro population with the specific remediation efficacy into a polluted site. The native microbes are added to the contaminated site may be on site or off site to eliminate hazardous compounds. The process of bio-augmentation has been used for the degradation of a wide range of pollutants like NH3, H2S, organic compounds etc. from the soil and water (Hassan et al., 2022). The pre-grown microbial culture enhances the microbial population at the contaminated site and reduces the clean-up time and cost of the operation (Giri et al., 2021). Wang et al. (2013) removed atrazine from highly contaminated soil (atrazine concentration 400 mg/kg soil) by using Arthrobacter sp-based bio-augmentation. The process displayed up to 90% removal of atrazine from the soil. Bacillus cereus was used for the removal of chlorpyrifos contamination in the soil and the average degradation was found to be 88% within 8 days of the treatment (Farhan et al., 2021). The strains of Pseudomonas bacillus subtilis were used in the bio-augmentation process and removed 95% of Chlorpyrifos from the soil within 15 days of treatment (Gangola et al., 2018).
Dichloro-diphenyl-trichloroethane (DDT) has been banned in many countries for its use as a pesticide in agriculture but still, in most parts of the world, the residues of DDT are present as contaminants in the soil and its detoxification is a challenging task. Some fungus like Gloeophyllum trabeum and Daedalea dickinson has been used in the degradation of pesticides from the soil (Mathur and Gehlot, 2021). A wide range of pesticides has been degraded by the process of bio-augmentation using microorganisms. Carbofuran was degraded using Syncephalastrum racemosum and the rate of degradation was around 75% after inoculation of culture in the soil (de Sousa Lira and Orlanda, 2020). Cypermethrin was degraded using several bacterial strains from Bacillus, Pseudomonas, Streptomyces, etc. and by different fungi such as Aspergillus niger, Aspergillus terricola, Trichoderma viride (Maqbool et al., 2016; Bhatt et al., 2019a). Lindane and Parathion were degraded by a bio-augmentation process using Paenibacillus dendritiformis and Serratia marcescens, respectively, (Jaiswal et al., 2022). Various microorganisms, which degrade different pesticides, are enlisted (Table 1).
Bioremediation of pesticides in the soil is a complex process, which involves several interdependent interactions within the soil, soil to air, soil to water, and characteristics of the pesticides. The rate of bioremediation depends upon interdependent physiochemical and biological processes, which are regulated by several factors.
The chemical structure of pesticides plays an important role in regulating the rate of bioremediation of pesticides. The pesticides having polar groups like C-OH, -COOH, etc. on the phenyl ring are more susceptible to microbial biodegradation in comparison to halogen or alkyl substituents. Even a minor alteration in a structural substituent may cause drastic changes in microbial susceptibility (Geed et al., 2016). In the process of bioremediation, oxidation and reduction of active functional groups result in their conversion to simple molecules like CO2, H2O, Nitrate, Phosphate and NH3 (Sharma, 2020). Chlorinated hydrocarbons like DDT are more resistant to bioremediation as they have low solubility in water and high absorption affinity in soil. On the other hand, compounds like 2-4D and Carbofuran can be degraded from the soil by microorganisms in a few days within the same class of pesticides; a minor group substitution may change the susceptibility of pesticides to microbial degradation (Geed et al., 2017).
Pesticide concentration in soil is another important factor in deciding the rate of degradation of pesticides in the soil. The biodegradation rate depends upon the residual concentration of pesticide in the soil and it follows pseudo first order kinetics (Zaranyika et al., 2020). The rate of biodegradation decreases proportionally with residual concentration of pesticides
where, d[P]/dt = pesticide concentration gradient with respect to time; K = biodegradation rate constant.
The half life of pesticides may vary from 10 days to 200 days. Pesticides like Inceptisol and Ultimo have half life ranging from 10.1 to 29.2 days while several pesticides like DDT, endosulfan, and atrazine have half life varying from 100 to 200 days. The residues of less biodegradable pesticides remain adsorbed on soil particles so these are not available for microbial degradation (Giri et al., 2021).
Soil organic content, pH, the concentration of clay material and moisture contents are the important factors, which contribute to deciding the rate of degradation of pesticides in the soil (Rasool et al., 2022). Adsorption of pesticide residues with soil particles reduces the bioavailability of pesticides for the microbes and increases the half life of the residues (Giri et al., 2021). Water is one of the most important factors, which decides the motion and diffusion of pesticide molecules for microbial-assisted biodegradation. The rate of biodegradation of pesticides is directly proportional to soil moisture content and extremely low in dry soil (Chowdhury et al., 2008). Soil aeration and oxygen level also affect the rate of pesticide degradation as few pesticides like DDT, which is fairly stable in aerobic soil but degrades slowly in submerged soils (Raffa and Chiampo, 2021). Soil temperature produces a great impact on the stability of molecular conformation of the pesticides. It affects the solubility and rate of hydrolysis of pesticides in soil samples. The optimum soil temperature of microbial degradation ranges from 20°C to 40°C as in this range of temperature microbes has maximum activity (Singh et al., 2022).
The pH of the soil also affects the rate of bioremediation of pesticides. The degradation of the pesticides depends upon the activity of the enzymes produced by the microorganism. The enzymes have a very narrow range of pH for these activities. Most of the bacterial enzymes work at soil pH between 6.5 and 7.5. Apart from the activity of microbial enzymes produced by a microorganism, pH also influences the pesticide adsorption, biotic and abiotic degradation processes (Rasool et al., 2022). The degradation of pesticides also depends upon the chemical susceptibility toward hydrolysis by acidic or basic pH of the soil (Liu et al., 2015). Soil organic content also affects the rate of microbial degradation of pesticides. Organic contents increase the rate through co-metabolism of pesticides. The organic content of the soil also acts as a source of nutrients for the soil microbes. Therefore, increases the microbial population rapidly and results in an increased rate of microbial degradation of the pesticides. The rate of bacterial-mediated biodegradation of organochloride was increased with the addition of organic carbon sources in the soil (Krohn et al., 2021).
It has been deduced that a minimum of 1% of organic content should be present for effective microbial biodegradation of pesticides (Huang et al., 2018). In the case of pesticides, which are present in low concentrations in the soil, the co-metabolism of microbes has proven an effective measure for bioremediation. The organic content of the soil contains co-substrate, which facilitates co-metabolism of the microbes (Banwart et al., 2014).
To increase the efficacy and rate of bioremediation methods of immobilization were introduced. These methods limited the mobility of microbes and their enzymes and immobilization also enhanced the viability of microbes and the catalytic functions of their enzymes. In the process of immobilization, the natural activity of microorganisms to form biofilm on the surface of various materials was explored. Immobilization not only increased the efficacy of the bioremediation but also reduced the cost of operation as it made multiple uses of biocatalysts possible. It provided a stable environment for microbes and reduced the genetic mutations in a microorganism (Mehrotra et al., 2021). Mainly five techniques have been used in the process of immobilization; these were adsorption, binding on the surface, flocculation, entrapment and encapsulation.
In this process, microorganisms are adsorbed on the surface of water insoluble carriers by weak bonds. It is a simple and economic method but as there is a high probability of cells leaking from the carriers to the environment, this method is not recommended for the use in case of genetically modified microorganisms (Dzionek et al., 2016).
In this process, the surface of the carrier is washed with buffer, which makes the surface hydrophilic. The microbes and enzymes having a negative charge bind with the surface of the carrier. In another method of binding; covalent binding, a binding agent is required and carriers are chemically activated. This method is mainly used for the binding of enzymes because binding agents may affect the viability of microbes. As the covalent binding is very strong, the leaking of molecules (enzymes) is efficiently prevented by covalent bonding (Jiang et al., 2022).
This method has been mainly used in microbial bioremediation. As a result of entrapment, microbial cells can move within the carrier and prevent the leaking of the cells in the environment but allow the exchange of nutrients and metabolites. In a heterogeneous carrier system, the population of microorganisms is physiologically diverse as the cells located near the surface have high metabolic activity. The entrapment method has several advantages as it is a non-toxic, economical and highly versatile method. It efficiently prevents microorganisms from environmental factors. The efficiency of the entrapment method depends on the selection of a suitable ratio of the size of pores and cell size (Mehrotra et al., 2021).
In this method, immobilized particles are separated from the environment using a semi-permeable membrane. This method provides significant protection to microbes against external environmental conditions but the limited permeability of the membrane may affect the viability of the cells (Priyanka et al., 2022).
The method to be used for the immobilization process should sustain a few properties. It should be non-toxic, economic, stable, insoluble and regenerative. Carriers to be used for adsorption and binding on the surface should have high porosity (Dzionek et al., 2016). Carriers can be classified into two groups; natural carriers and synthetic carriers. Each group can be divided into two subgroups; organic and inorganic. Natural organic carriers include alginates, chitosan, sawdust, charcoal, plant fibers, bagasse, rice husk, etc. These carriers contain many functional groups which stabilize biocatalysts (Cubitto and Gentili, 2015). Most of these materials are waste of the food industries so these are economic and biocompatible but they have a low resistance to the biodegradation and sensitivity to organic solvent (Paliwal et al., 2015). They have a very narrow pH range for their stability. Synthetic organic carriers like polypropylene, polystyrene, polyacrylonitrile, polyvinyl alcohol and polyvinyl chloride have several functional groups with diversified properties. The macromolecular structure of synthetic organic carriers may be regulated as per the desired order of functional groups in the chain. Moreover, their porosity, polarity and hydrophobic nature can also be modified. These are commercially available at economical prices. Inorganic carriers like magnetite, silica-based material, ceramics, porous glass and nanoparticles have high chemical, physical and biological resistance. The number of functional groups present on these carriers is very less and it prevents their sufficient bonding with microorganisms and catalysts. Inorganic carriers can be used in the formation of hybrid carriers by combining them with natural polymers or synthetic nanoparticles (Yunoki et al., 2014).
Nanotechnology is a branch of science, which deals with synthesized particles, which are very small in size (<100 nm). In the last few years, nanotechnology has been used in various fields like medicine, textiles, optics, etc. The use of nanoparticles and application of nanotechnology in agriculture was started at the beginning of the 21st century (Fraceto et al., 2016) and more than 230 nano-products have been used in various agricultural operations (Rajput et al., 2022). Nanotechnology has been integrated with the bioremediation process and termed Nano-bioremediation. Nano-bioremediation targeted cleaning of the environment by accelerating bioremediation using nanoparticles (Bhatt et al., 2021). Nano-bioremediation is further subdivided into two subgroups, i.e., nano-phytoremediation of nanoparticles with phytoremediation and microbial nano-remediation (Singh et al., 2020; Kumari et al., 2022; Figure 2).
The basic principle of nano-bioremediation is the degradation of contaminants using a catalyst as nanoparticles. As the nanoparticles are very small in size, it allows them to interact more deeply and have a large surface area as per unit mass, more numbers of nanoparticles can come into contact with the environment. This enhances the efficacy and the rate of bioremediation (Cecchin et al., 2017).
The process of bioremediation involves the use of living organisms for the remediation of pollutants. Once nanotechnology is integrated with the process of bioremediation, the interaction of nanoparticles with living organisms becomes the key factor in deciding the efficacy of nano-bioremediation. In a few cases, the interaction of nanoparticles and biotic components resulted in biocidal and proven harmful to the organisms involved in the bioremediation (Juárez-Maldonado et al., 2019). Therefore, evaluation of the interaction of nanoparticles and biotic components is the prerequisite of the nano-bioremediation process. The efficacy of nano-bioremediation can be influenced by several factors like size, shape, chemical nature of the nanoparticles, the physiological properties of the organism, pH and temperature of the soil, nature of the contaminant, etc. (Tan et al., 2018). These factors work directly or indirectly. Temperature, pH and media are crucial for the optimal development of biological organisms while the direct interaction of nanoparticles and organisms regulates various actions like dissolution, absorption and biotransformation (Kranjc and Drobne, 2019). Nano-bioremediation is a two-step process. In the first contaminants are broken down by nanoparticles to a conducive level for bioremediation and in the second step; pollutants are biodegraded (Cecchin et al., 2017).
Nano-phytoremediation is a method for remediation of pollutants, and contaminants by using synthesized nanoparticles from the plants. Plants are the natural detoxifiers for the soil as they absorb diverse types of compounds and detoxify them. Phytoremediation is a Greek word, which means restore/remedy through plants (Pandya et al., 2022) but phytoremediation has a few limitations as slow remediation time and plant waste. Nanotechnology has increased the efficacy of remediation of contaminated soil and water. Organic contaminants like atrazine, molinate and chlorpyrifos have been degraded with nano-sized zerovalent irons. Enzymes encapsulated in nanoparticles increased the efficiency of bioremediation significantly (Yadav et al., 2017).
There are several factors, which affect the efficacy of nano-bioremediation. These are the physical and chemical properties of compounds, their molecular weight, solubility in the water, soil environment (pH, temperature and percentage of organic matter) and characteristics of plants (Gulzar and Mazumder, 2022). Integration of nanoparticles and phytoremediation is the most important step in nano-phytoremediation. The studies indicate that the application of nanoparticles detoxified various organic, inorganic and metal pollutants from the soil. The use of nano-zerovalent iron, and magnetite nanoparticles rapidly degraded organic pollutants from the soil (Madhura et al., 2019). Nanoparticles of TiO2 (n TiO2) and PEI-copper nanoparticles reduced the half lives of Phenanthrene and atrazine, respectively (Li et al., 2016; Kalidhasan et al., 2017). The technique of nano-phytoremediation worked for a wide range of soil pollutants ranging from heavy metals to organic compounds. The application of nanoparticles enhanced the uptake of pollutants by plants and also improved the stress tolerance capacity of the plants (Pillai and Kottekottil, 2016; Souri et al., 2017).
Though there are several factors, which affect the uptake of nanoparticles by plants like the type and chemical composition of nanomaterial, the size of nanoparticles plays the most crucial role in the uptake of the nanoparticles (Schwab et al., 2016). The nanoparticles can be transported into the plants in two ways; Apoplastic transport (transport through xylem vessels), Symplastic transport (transport between the cytoplasm and sieve plates; Horejs, 2022).
Apart from the size of nanoparticles, the soil temperature is also an important factor as it affects the growth substances and root lengths (Ahmadpour et al., 2012). The properties of the plants also affect the efficacy of nano-bioremediation. For achieving high efficiency, a plant should have fast growth, large biomass, a well-developed root system, high toxicity tolerance limit, high accumulation capacity, a non-consumable for animals and easy for genetic manipulation. Nanoparticles should be non-toxic for plants and should have the properties to enhance germination, root-shoot elongation, enhanced phoenzyme production, increased plant growth hormone and capabilities to bind with contaminants of the soil (Sajid et al., 2015). Nano-phytoremediation technology has been used with natural as well as genetically engineered plants. Nanoparticles enhanced plant growth and their efficacy in remediation of the soil contaminants. These particles increased the production of plant growth hormone and enhanced the uptake of soil pollutants by plants (Dimkpa et al., 2012; Liu et al., 2016) Nano-zerovalent iron nanoparticles were used with plants like Alpinia calcarata Roscoe, Ocimum sanctum, Cymbopogon citratus and with all three plants, these particles enhanced the remediation efficacy against Endosulfan (Pillai and Kottekottil, 2016). Similarly, sialic acid nanoparticles increased the phytoremediation efficacy of Isatis cappadocica Desv for Arsenic (Souri et al., 2017). Nanoparticles cause many physiological changes in the plants, which results in increased efficacy of phytoremediation but the effectiveness and safety of the nanoparticles are decided by several factors like chemical composition, size, shape, stability, concentration, and surface coating and reactivity of the nanoparticles. The efficacy of nanoparticles may vary from plant to plant also (Varma and Khanuja, 2017; Yang et al., 2017). Nano-phytoremediation technology has few limitations such as most of the experiments have been conducted at microcosm levels so extensive studies are required. Formation of aggregation is a common phenomenon with nanoparticles so studies to modify their surfaces to enhance the sustainability of nanoparticles are essentially required and in last the toxicity of various nanoparticles for the soil and the environment needs to be evaluated.
It is the process in which nanoparticles are used with soil microbes to enhance biodegradation processes. Microorganisms can uptake the metal ions and reduce them. In this process, the metal ions are converted into nanoparticles. Microbial enzymes along with the metals form useful nanoparticles for nano-bioremediation (Pandey, 2018). Microbial nano-bioremediation is a two-phase process, which involves abiotic and biotic processes (Usman et al., 2020). In the first phase, nanoparticles enter the system and particles of pollutants undergo varieties of the processes like adsorption, absorption, dissolution and photocatalysis (Abebe et al., 2018). In the second phase, several biotic processes like biostimulation and biotransformation remove these particles from the system (Desiante et al., 2021). The second phase (biotic phase) plays a very important role in the bioremediation of pollutants. Microbial nano-bioremediation has been used for a variety of pollutants like inorganic and organic (Figure 3). Nanoparticles involved in the microbial degradation and enlisted in Table 2.
Heavy metals are increasing in the environment and soil due to anthropogenic activities and disturbed biogeochemical cycles. Heavy metals like Pb, As, Cd, etc. not have any well-defined function in the biological system but they have a toxic effect on the biotic component of the environment even in the low concentration (Bist and Choudhary, 2022). In acidic soil with low nutrient levels, the toxicity of heavy metals increases (Liu et al., 2018). Heavy metals generate reactive oxygen, which damages the macromolecules (proteins and nucleic acids) of microorganisms and plants (Bist and Choudhary, 2022). Immobilization of heavy metal molecules is the most common technique used for their bioremediation (Suman et al., 2018). Nanoparticles including bio-organic nanoparticles (synthesized using biological organisms) have been used in the removal of heavy metals from the soil Bio-organic nanoparticles such as silver nanoparticles produced in Morganella psychrotolerans have been used for the remove of heavy metals (Arif et al., 2016; Enez et al., 2018). Iron oxide nanoparticles coated with polyvinyl pyrrolidone (PVP) are used with Halomonas sp. (gram-negative bacteria) for the bioremediation of lead and cadmium (Alabresm et al., 2018). Magnetic nanoparticles of Fe3O4 coated with phthalic acid treated with S. aureus were used for bioremediation of Cu, Ni and Pb. The efficiency of these particles was 83%–89% for Cu2+, 99.4%–100% for Pb2+ and 92.6%–97.5% for Ni2+. It was observed that the functional group present on the microbial surface and core of nanoparticles played an important role in the removal of heavy metals (Mahmoud et al., 2016). Heavy metals resistant bacteria B. cereus and L. macroides in combination with zinc oxide were used for the remediation of Cu, Cd, Cr and Pb. It was deduced that zinc oxide nanoparticles along with B. cereus could remove these metals efficiently (Baragaño et al., 2020). The strain of B. cereus (XMCr−6) reduced Cr+6 to Cr+3. The reduced Cr+3 exhibited affinity to the bacterial cell surface and by reacting with oxygen formed Chromium oxide nanoparticles as a byproduct (Laslo et al., 2022). Selenium nanoparticles in combination with probiotic bacteria (L. casei) were used for cadmium-contaminated land and water treatment. The efficiency of cadmium absorption was found 65% with L. casei, which was significantly higher than L. casei alone (43%–78%; Dong et al., 2013).
In the approach of bio-organic nanoparticle synthesis, heavy metals pollutants can be used by selective microbes followed by their removal from the environment and yielding value for the waste. In this approach, Enterococcus faecelis was used for the removal and recovery of lead. The lead nanoparticles were synthesized by bacteria in extracellular and intracellular modes. The size of these nanoparticles was ~10 nm. These particles exhibited high catalytic efficiency and reduced 5.0 μmol Cr+6 in 12 h (Cao et al., 2020).
In anaerobic sludge, tellurium nanoparticles were synthesized by supplementation with riboflavin in the presence of Rhodobacter capsulates using polluted tellurite Te4+ oxy anions present in the wastewater (Ramos-Ruiz et al., 2016). These findings indicate that nano-bioremediation may be effectively used for the remediation of heavy metals toxicity.
Organic pollutants mainly persistent organic pollutants have negative impacts on human and environmental health. Therefore, the remediation of these compounds from the soil is essential. Magnetic nanoparticles in combination with Rhodococcus engthropolis caused the desulfurization of dibenzothiophene (DBT; Ansari et al., 2009). Bimetallic (Pd/nFe) nanoparticles were used in combination with Sphingomonas wittichii for bioremediation of NBR.2, 3, 7, 8-tetrachlorodibenzo-p-dioxin hydrocarbons (Bokare et al., 2012). The silica nanoparticles biofunctionalized with the lipid bilayer of Pseudomonas aeruginosa were used to clean up PAH(benzo[a] Pyrene). The membrane lipids of Pseudomonas played a role to enhance the sequestration of PAH (Wang et al., 2015). The grapheme oxide nanoparticles along with the laccase enzyme of Trametes versicolor were studied for biodegradation of PAH and were found to be effective in remediation of PAH (Patil et al., 2016). Alcaligenes faecelis in combination with iron oxide nanoparticles improved the degradation of hydrocarbon compounds of crude oil contamination (Oyewole et al., 2019). Sphingomonas strain NM05 has been used for the degradation of hexachlorocyclohexane (HCH). Once the strain was treated with Pd/FeO bimetallic nanoparticles, the degradation efficacy of the strain was enhanced ~2 folds (Singh R. et al., 2013). The peroskite (LaFeO3) nanoparticles with proteobacteria were used for the degradation of organic contaminants in marine sediments (Hung et al., 2021). Nanoparticles not only enhanced the remediation efficacy of microbes but are also used for the improvement of soil health. Silicon nanoparticles have been reported to improve the soil microflora and biomass. These particles enhanced the growth of rhizospheric microbes (Rajput et al., 2022).
Phyto-nanotechnology is an efficient, cost-effective and eco-friendly strategy, which is extensively used for the remediation of toxic compounds from the ecosystem (Gole et al., 2022). This technology involves the plant based synthesis of nanoparticles with almost no risk to the ecosystem and humans. Various types of metal nanoparticles like silver, palladium and gold have been synthesized with algae belonging to different groups such as Chlorophyceae, Cyanophyceae, Phaeophyceae and Rhodophyceae. Algae are the largest photoautotrophic group of microbes, having the potential to act as nano-machineries for the metallic nanoparticles. The fabrication of algae based nanoparticles is less time consuming process (Khanna et al., 2019). Algae have several properties like high potential of metal uptake, easy to handle and harvest, low cost, low toxicity, which make them suitable to serve as nano-factories (Sharma et al., 2015). Silver nanoparticles were produced using number of brown algae species such as Cystophora moniliformis, Gelidiella acerosa and Padina pavonica (Azizi et al., 2014) while other species like Cystoseira baccata, Dictyota bartayresianna, Ecklonia cava, and Sargassum wightii have been used in the fabrication of gold nanoparticles (AuNPs). Similarly Phormidium valderianum and S. platensis are also responsible for the AuNPs biosynthesis (Iravani et al., 2017). Algal species such as Cylindrospermum stagnale, Spirulina platensis, Plectonema boryanum, and Microchaete diplosiphon have been reported for the synthesis of AgNPs having varied morphologies (Husain et al., 2015). Various algae have been recognized for the remediation of toxic compounds and heavy metals from the wastewater (Goswami et al., 2021). Studies showed that the microalgae from Chlorellaceae family removed heavy metals such as lead, copper, and selenium from the wastewater (Oyebamiji et al., 2019). Microalgae have the ability to remove the toxic heavy metals from the acid mine drainage, which facilitate the inhibition of direct discharge of acid mines into the water bodies that may lead to the damage to aquatic habitat as well as create serious environmental pollution (Samal et al., 2020). The synthesis of nanoparticles and the algae mediated bioremediation belong to same process, which occur simultaneously (Dahoumane et al., 2016). Recently Chlorella vulgaris, a green microalgae was reported for the efficient removal of Au(I) and Au(III) complexes (He and Chen, 2014). The metal uptake potential of Nannochloropsis oculata was evaluated from the acid mine drainage. The result revealed that 99% of copper content was removed by N. oculata (Martínez-Macias et al., 2019). Furthermore, a microalga such as Chlorella kessleri was used for the removal of heavy metals from wastewater (Sultana et al., 2020). Studies have showed that microalgae have potential to remove heavy metals from the wastewater. However extensive research is required in this aspect to enhance the remediation efficiency and complete utilization of the biomass. Various studies have reported the wastewater treatment by the immobilization of microalgae biomass, which is considered as an effective technique for the remediation of the heavy metal (Cheng et al., 2019). The consortium of microalgae has attracted interest of the researchers to remove heavy metals for the wastewater treatment. The heavy metals such as nickel, cadmium and lead have been removed from the wastewater using the consortia of microalgae (Abdel-Razek et al., 2019).
Fungi are the eukaryotic microorganisms, which include molds, yeasts, mildews and mushrooms (Duhan et al., 2017). Fungi act as biocatalysts and are used in bioremediation as they can survive in intense conditions as well as elevated concentration of heavy metals (Dixit et al., 2015). In green nanotechnology, nanoparticles are synthesized using fungi, which play a pivotal function in the removal of toxic compounds and organic pollutants (Singh et al., 2018). In recent times, the synthesis of metal nanoparticles from fungi has gained a big interest of researchers around the world (Sunny et al., 2022). There are several advantages of metal nanoparticles synthesized using fungi such as higher capacity of metal uptake, simple and low cost fabrication, tolerant against metals, high scalability, highly stable (Yadav et al., 2015).
Various fungus like Fusarium, Verticillium, Penicillium, and Aspergillus have been used as potential candidates for the metallic nanoparticles synthesis (Ovais et al., 2018). Studies have reported that metals like gold, silver, titanium, platinum, selenium, palladium and silica can be utilized for the fabrication of nanoparticles (Gholami-Shabani et al., 2016). Silver nanoparticles (AgNPs) synthesized from F. oxysporum are different in features from those synthesized with Aspergillus fumigates (Alves and Murray, 2022). Studies have reported the synthesis of AgNPs using Coriolus versicolor and Trichoderma reesei (Vahabi et al., 2011; Deniz et al., 2019). The gold nanoparticles (AuNPs) have been synthesized using Cylindrocladium floridanum fungus (Narayanan and Sakthivel, 2011). Platinum nanoparticles were synthesized using the N. crassa fungus and Fusarium oxysporum was used for fabrication of silica nanoparticles (Castro-Longoria et al., 2012). The selenium nanoparticles have been synthesized from Mariannaea sp. (Zhang et al., 2019). The green synthesis of the nanoparticles from different fungus has led to a significant application in the remediation of hazardous organic pollutants through the adsorption of heavy metals (Gaur et al., 2014). The kind of metal, environmental factors and fungal biomass affect the capacity of biosorption (Dhankhar and Hooda, 2011). Studies have showed the proficient adsorption, immobilization capacity and chelation activity of heavy metal ions by arbuscular mycorrhizal fungi (Upadhyaya et al., 2010). Various fungal species like Allescheriella sp., Botryosphaeria rhodina sp., Stachybotrys sp. exhibited the metal-binding capability (Benjamin et al., 2019). The synthesis of AgNPs from Rhizopus oryzae have several ecological uses like wastewater treatment (Zhang et al., 2014) and adsorption of pesticides (Das et al., 2012). Fungi like Fusarium solani have higher tolerance against few heavy metals like cadmium, nickel and lead, also have better capacity of nanoparticles synthesis (Rasha, 2017). The extremophilic fungi have significant ability and application in nano-bioremediation of heavy metals due to ability to survive in severe conditions, which makes them significant for the purpose of nano-bioremediation (Bahrulolum et al., 2021). The marine fungi were assessed for their potential of bioremediation as well as the ecological importance (Thatoi et al., 2013). The degradation of pentachlorophenol was observed by Trichoderma harzianum (Vacondio et al., 2015). The heavy metals like CdCl2, CuSO4, Pb and ZnSO4 could not affect the growth of Cryptococcus sp., a psychrophilic fungus (Singh M.P. et al., 2013).
Nanoparticles in combination with white-rot fungi (WRF) have immense potential of bioremediation (He et al., 2017). Studies have reported the remediation of toxic contaminants from wastewater and good stability of WRF-magnetic nanoparticles due to proficient immobilization. Antibiotics like sulfonamide have been reported to be degraded by Echinodontium taxodii-Fe3O4 nanoparticles (Shi et al., 2015). The remediation of cadmium and 2,4-dichlorophenol was achieved through immobilization of Phanerochaete chrysosporium along with titanium oxide nanoparticles (Chen et al., 2013) P. chrysosporium along with Fe2O3 nanoparticles revealed degradation of phenols (Huang Z. et al., 2017). Likewise, selenium nanoparticles in combination with Phanerochaete chrysosporium reported effective remediation of zinc (Espinosa-Ortiz et al., 2016). Similarly P. chrysosporium, with silver nanoparticles showed enhanced removal of Cd2+ and 2,4-dichlorophenol (Zuo et al., 2015; Huang Z. et al., 2017). Therefore, it can be concluded that nanoparticles in combination with fungi resulted in an increased rate of remediation. Hence more studies and research should be conducted for development of such effective strategies using ecological microbiology and nanotechnology.
The various reports available on pesticide contamination of soil indicate that the level of pesticides in the soil is increasing day by day, which is affecting human, soil and environmental health. Hence pesticides should be used rationally, especially in the underdeveloped and developing countries where an efficient monitoring system is lacking. In case of real need, the preference should be given to the application of pesticides, which have short half life and high biodegradability. To improve soil health, crop rotation programs and the use of organic manure should be implemented more effectively. Though there are various techniques used for the remediation of soil pesticide contamination, most of these techniques have their limitations. The various experiments conducted with the integration of nanoparticles with the bioremediation process have shown promising potential but more extensive research and experimentation are required in this area. Various nanoparticles studied have enhanced the efficacy of the bioremediation but the safety of the nanoparticles for the environment and the food chain is still a matter of concern. Therefore, extensive research is required in the area of the safety analysis of nanoparticles.
YS wrote the manuscript. MK conceptualized and reviewed manuscript. All authors contributed to the article and approved the submitted version.
The work was accomplished working as Young Scientist, Department of Science and Technology (SYST) (Grant Number: SP/YO/2019/1385(G)). DST is highly acknowledged for providing the research grant.
The authors declare that the research was conducted in the absence of any commercial or financial relationships that could be construed as a potential conflict of interest.
All claims expressed in this article are solely those of the authors and do not necessarily represent those of their affiliated organizations, or those of the publisher, the editors and the reviewers. Any product that may be evaluated in this article, or claim that may be made by its manufacturer, is not guaranteed or endorsed by the publisher.
Abdel-Razek, M. A., Abozeid, A. M., Eltholth, M. M., Abouelenien, F. A., El-Midany, S. A., Moustafa, N. Y., et al. (2019). Bioremediation of a pesticide and selected heavy metals in wastewater from various sources using a consortium of microalgae and cyanobacteria. Slov Vet. 56, 61–73. doi: 10.26873/SVR-744-2019
Abebe, B., Murthy, H. A., and Amare, E. (2018). Summary on adsorption and photocatalysis for pollutant remediation: mini review. J. Encapsulation Adsorpt. Sci. 8, 225–255. doi: 10.4236/jeas.2018.84012
Adams, G. O., Fufeyin, P. T., Okoro, S. E., and Ehinomen, I. (2015). Bioremediation, biostimulation and bioaugmention: a review. Int. J Environ Bioremediat Biodegrad 3, 28–39. doi: 10.12691/ijebb-3-1-5
Aggelopoulos, C. A., Svarnas, P., Klapa, M. I., and Tsakiroglou, C. D. (2015). Dielectric barrier discharge plasma used as a means for the remediation of soils contaminated by non-aqueous phase liquids. Chem. Eng. J. 270, 428–436. doi: 10.1016/j.cej.2015.02.056
Ahmadpour, P., Ahmadpour, F., Mahmud, T. M. M., Abdu, A., Soleimani, M., and Tayefeh, F. H. (2012). Phytoremediation of heavy metals: a green technology. African J. Biotechnol. 11, 14036–14043. doi: 10.5897/AJB12.459
Alabresm, A., Chen, Y. P., Decho, A. W., and Lead, J. (2018). A novel method for the synergistic remediation of oil-water mixtures using nanoparticles and oil-degrading bacteria. Sci. Total Environ. 630, 1292–1297. doi: 10.1016/j.scitotenv.2018.02.277
Ali, S., Ullah, M. I., Sajjad, A., Shakeel, Q., and Hussain, A. (2021). “Environmental and health effects of pesticide residues,” in Sustainable Agriculture Reviews, Vol. 48. eds. M. I. Inamuddin and A. E. Lichtfouse (Cham: Springer), 311–336.
Alves, M. F., and Murray, P. G. (2022). Biological synthesis of Monodisperse uniform-size silver nanoparticles (AgNPs) by fungal cell-free extracts at elevated temperature and pH. J. Fungi 8:439. doi: 10.3390/jof8050439
Amani, F., Safari Sinegani, A. A., Ebrahimi, F., and Nazarian, S. (2018). Biodegradation of chlorpyrifos and diazinon organophosphates by two bacteria isolated from contaminated agricultural soils. Biological J. Microorganism 7, 27–39.
Ansari, F., Grigoriev, P., Libor, S., Tothill, I. E., and Ramsden, J. J. (2009). DBT degradation enhancement by decorating Rhodococcus erythropolis IGST8 with magnetic Fe3O4 nanoparticles. Biotechnol. Bioeng. 102, 1505–1512. doi: 10.1002/bit.22161
Anwar, S., Liaquat, F., Khan, Q. M., Khalid, Z. M., and Iqbal, S. (2009). Biodegradation of chlorpyrifos and its hydrolysis product 3, 5, 6-trichloro-2-pyridinol by Bacillus pumilus strain C2A1. J. Hazard. Mater. 168, 400–405. doi: 10.1016/j.jhazmat.2009.02.059
Arif, N., Yadav, V., Singh, S., Singh, S., Ahmad, P., Mishra, R. K., et al. (2016). Influence of high and low levels of plant-beneficial heavy metal ions on plant growth and development. Front. Environ. Sci. 4:69. doi: 10.3389/fenv.201600069
Azizi, S., Ahmad, M. B., Namvar, F., and Mohamad, R. (2014). Green biosynthesis and characterization of zinc oxide nanoparticles using brown marine microalga Sargassum muticum aqueous extract. Mater. Lett. 116, 275–277. doi: 10.1016/j.matlet.2013.11.038
Bahrulolum, H., Nooraei, S., Javanshir, N., Tarrahimofrad, H., Mirbagheri, V. S., Easton, A. J., et al. (2021). Green synthesis of metal nanoparticles using microorganisms and their application in the agrifood sector. J.Nanobiotechnol. 19, 1–26. doi: 10.1186/s12951-021-00834-3
Baldissarelli, D. P., Vargas, G. D. L. P., Korf, E. P., Galon, L., Kaufmann, C., and Santos, J. B. (2019). Remediation of soils contaminated by pesticides using physicochemical processes: a brief review. Planta Daninha 37:e019184975. doi: 10.1590/s0100-83582019370100054
Banwart, S. A., Noellemeyer, E., and Milne, E. (Eds.) (2014). Soil Carbon: Science, Management and Policy for Multiple Benefits, vol. 71. Boston, MA, USA: CABI.
Baragaño, D., Forján, R., Welte, L., and Gallego, J. L. R. (2020). Nanoremediation of as and metals polluted soils by means of graphene oxide nanoparticles. Sci. Rep. 10, 1–10. doi: 10.1038/s41598-020-58852-4
Barjasteh, A., Dehghani, Z., Lamichhane, P., Kaushik, N., Choi, E. H., and Kaushik, N. K. (2021). Recent progress in applications of non-thermal plasma for water purification, bio-sterilization, and decontamination. Appl. Sci. 11:3372. doi: 10.3390/app11083372
Benjamin, S. R., Lima, F. D., Florean, E. O. P. T., and Guedes, M. I. F. (2019). Current trends in nanotechnology for bioremediation. Int. J. Environ. Pollut. 66, 19–40. doi: 10.1504/IJEP.2019.104526
Bhatt, P., Huang, Y., Rene, E. R., Kumar, A. J., and Chen, S. (2020). Mechanism of allethrin biodegradation by a newly isolated Sphingomonas trueperi strain CW3 from wastewater sludge. Bioresour. Technol. 305:123074. doi: 10.1016/j.biortech.2020.123074
Bhatt, P., Huang, Y., Zhan, H., and Chen, S. (2019a). Insight into microbial applications for the biodegradation of Pyrethroid insecticides. Front. Microbiol. 10:1778. doi: 10.3389/fmicb.2019.01778
Bhatt, P., Pandey, S. C., Joshi, S., Chaudhary, P., Pathak, V. M., Huang, Y., et al. (2021). Nanobioremediation: a sustainable approach for the removal of toxic pollutants from the environment. J. Hazard. Mater. 427, 1–18. doi: 10.1016/j.jhazmat.2021.128033
Bielská, L., Šmídová, K., and Hofman, J. (2013). Supercritical fluid extraction of persistent organic pollutants from natural and artificial soils and comparison with bioaccumulation in earthworms. Environ. Pollut. 176, 48–54. doi: 10.1016/j.envpol.2013.01.005
Bist, P., and Choudhary, S. (2022). Impact of heavy metal toxicity on the gut microbiota and its relationship with metabolites and future probiotics strategy: a review. Biol. Trace Elem. Res., 1–23. doi: 10.1007/s12011-021-03092-4
Bocos, E., Fernández-Costas, C., Pazos, M., and Sanromán, M. Á. (2015). Removal of PAHs and pesticides from polluted soils by enhanced electrokinetic-Fenton treatment. Chemosphere 125, 168–174. doi: 10.1016/j.chemosphere.2014.12.049
Bokare, V., Murugesan, K., Kim, J. H., Kim, E. J., and Chang, Y. S. (2012). Integrated hybrid treatment for the remediation of 2, 3, 7, 8-tetrachlorodibenzo-p-dioxin. Sci. Total Environ. 435–436, 563–566. doi: 10.1016/j.scitotenv.2012.07.079
Cabrera-Penna, M., and Rodríguez-P’aez, J. E. (2021). Calcium oxyhydroxide (CaO/Ca(OH)2) nanoparticles: synthesis, characterization and evaluation of their capacity to degrade glyphosate-based herbicides (GBH). Adv. Powder Technol. 32, 237–253. doi: 10.1016/j.apt.2020.12.007
Cao, X., Alabresm, A., Chen, Y. P., Decho, A. W., and Lead, J. (2020). Improved metal remediation using a combined bacterial and nanoscience approach. Sci. Total Environ. 704:135378. doi: 10.1016/j.scitotenv.2019.135378
Cao, M., Wang, L., Wang, L., Chen, J., and Lu, X. (2013). Remediation of DDTs contaminated soil in a novel Fenton-like system with zero-valent iron. Chemosphere 90, 2303–2308. doi: 10.1016/j.chemosphere.2012.09.098
Castro, D. C., Cavalcante, R. P., Jorge, J., Martines, M. A., Oliveira, L., Casagrande, G. A., et al. (2016). Synthesis and characterization of mesoporous Nb2 O5 and its application for photocatalytic degradation of the herbicide methylviologen. J. Braz. Chem. Soc. 27, 303–313. doi: 10.5935/0103-5053.20150244
Castro-Longoria, E., Moreno-Velasquez, S. D., Vilchis-Nestor, A. R., Arenas-Berumen, E., and Avalos-Borja, M. (2012). Production of platinum nanoparticles and nanoaggregates using Neurospora crassa. J. Microbiol. Biotechnol. 22, 1000–1004. doi: 10.4014/jmb.1110.10085
Cecchin, I., Reddy, K. R., Thomé, A., Tessaro, E. F., and Schnaid, F. (2017). Nanobioremediation: integration of nanoparticles and bioremediation for sustainable remediation of chlorinated organic contaminants in soils. Int. Biodeterior. Biodegradation 119, 419–428. doi: 10.1016/j.ibiod.2016.09.027
Chen, G., Guan, S., Zeng, G., Li, X., Chen, A., Shang, C., et al. (2013). Cadmium removal and 2,4-dichlorophenol degradation by immobilized Phanerochaete chrysosporium loaded with nitrogen-doped TiO2 nanoparticles. Appl. Microbiol. Biotechnol. 97, 3149–3157. doi: 10.1007/s00253-012-4121-1
Cheng, S. Y., Show, P. L., Lau, B. F., Chang, J. S., and Ling, T. C. (2019). New prospects for modified algae in heavy metal adsorption. Trends Biotechnol. 37, 1255–1268. doi: 10.1016/j.tibtech.2019.04.007
Cheng, M., Zeng, G., Huang, D., Lai, C., Xu, P., Zhang, C., et al. (2016b). Hydroxyl radicals based advanced oxidation processes (AOPs) for remediation of soils contaminated with organic compounds: a review. Chem. Eng. J. 284, 582–598. doi: 10.1016/j.cej.2015.09.001
Cheng, M., Zeng, G., Huang, D., Lai, C., Xu, P., Zhang, C., et al. (2016a). Degradation of atrazine by a novel Fenton-like process and assessment the influence on the treated soil. J. Hazard. Mater. 312, 184–191. doi: 10.1016/j.jhazmat.2016.03.033
Chitescu, C. L., Oosterink, E., de Jong, J., and Stolker, A. A. M. L. (2012). Ultrasonic or accelerated solvent extraction followed by U-HPLC-high mass accuracy MS for screening of pharmaceuticals and fungicides in soil and plant samples. Talanta 88, 653–662. doi: 10.1016/j.talanta.2011.11.054
Choudhary, S., Yamini, N. R., Yadav, S. K., Kamboj, M., and Sharma, A. (2018). A review: pesticide residue: cause of many animal health problems. J. Entomol. Zool. Stud. 6, 330–333.
Chowdhury, A., Pradhan, S., Saha, M., and Sanyal, N. (2008). Impact of pesticides on soil microbiological parameters and possible bioremediation strategies. Indian J Microbiol 48, 114–127.
Cubitto, M. A., and Gentili, A. R. (2015). Bioremediation of crude oil–contaminated soil by immobilized bacteria on an agroindustrial waste—sunflower seed husks. Biorem. J. 19, 277–286. doi: 10.1080/10889868.2014.995376
Dahiya, U. R., Das, J., and Bano, S. (2022). “Biological indicators of soil health and biomonitoring” in Advances in Bioremediation and Phytoremediation for Sustainable Soil Management. ed. J. A. Malik (Cham: Springer), 327–347.
Dahoumane, S. A., Wujcik, E. K., and Jeffryes, C. (2016). Noble metal, oxide and chalcogenide-based nanomaterials from scalable phototrophic culture systems. Enzym. Microb. Technol. 95, 13–27. doi: 10.1016/j.enzmictec.2016.06.008
Das, S. K., Dickinson, C., Lafir, F., Brougham, D. F., and Marsili, E. (2012). Synthesis, characterization and catalytic activity of gold nanoparticles biosynthesized with Rhizopus oryzae protein extract. Green Chem. 14, 1322–1334. doi: 10.1039/c2gc16676c
De Neri, A. (2020). Neppure l’Evian è stata risparmiata dai pesticidi (Not Even Evian Is Spared from Pesticides). Available at: https://m.tio.ch/svizzera/attualita/1448903/acqua-evian-pesticidi-ricercatori-residui
de Sousa Lira, R. K., and Orlanda, J. F. F. (2020). Biodegradation of the carbofuran insecticide by Syncephalastrum racemosum. Res Soc. Dev. 9, –e824974932. doi: 10.33448/rsd-v9i7.4932
Degeronimo, K. (2015). Fragile ecosystems: pesticide use in conventional agriculture. New York, USA: Fordham University.
Deniz, F., Adigüzel, A. O., and Mazmanci, M. A. (2019). The biosynthesis of silver nanoparticles by cytoplasmic fluid of coriolus versicolor. Turkish J. Eng. 3, 1–10. doi: 10.31127/tuje.429072
Desiante, W. L., Minas, N. S., and Fenner, K. (2021). Micropollutant biotransformation and bioaccumulation in natural stream biofilms. Water Res. 193:116846. doi: 10.1016/j.watres.2021.116846
Dhankhar, R., and Hooda, A. (2011). Fungal biosorption–an alternative to meet the challenges of heavy metal pollution in aqueous solutions. Environ. Technol. 32, 467–491. doi: 10.1080/09593330.2011.572922
Dimkpa, C. O., McLean, J. E., Latta, D. E., Manangón, E., Britt, D. W., Johnson, W. P., et al. (2012). CuO and ZnO nanoparticles: phytotoxicity, metal speciation, and induction of oxidative stress in sand-grown wheat. J. Nanopart. Res. 14, 1–15. doi: 10.1007/s11051-012-1125-9
Dixit, R., Malaviya, D., Pandiyan, K., Singh, U. B., Sahu, A., Shukla, R., et al. (2015). Bioremediation of heavy metals from soil and aquatic environment: an overview of principles and criteria of fundamental processes. Sustainability 7, 2189–2212. doi: 10.3390/su7022189
Dong, H., Rowland, I., Thomas, L. V., and Yaqoob, P. (2013). Immunomodulatory effects of a probiotic drink containing lactobacillus casei Shirota in healthy older volunteers. Eur. J. Nutr. 52, 1853–1863. doi: 10.1007/s00394-012-0487-1
dos Santos, E. V., Saez, C., Martínez-Huitle, C. A., Canizares, P., and Rodrigo, M. A. (2015). The role of particle size on the conductive diamond electrochemical oxidation of soil-washing effluent polluted with atrazine. Electrochem. Commun. 55, 26–29. doi: 10.1016/j.elecom.2015.03.003
dos Santos, E. V., Souza, F., Saez, C., Cañizares, P., Lanza, M. R., Martinez-Huitle, C. A., et al. (2016). Application of electrokinetic soil flushing to four herbicides: a comparison. Chemosphere 153, 205–211. doi: 10.1016/j.chemosphere.2016.03.047
Duhan, J. S., Kumar, R., Kumar, N., Kaur, P., Nehra, K., and Duhan, S. (2017). Nanotechnology: the new perspective in precision agriculture. Biotechnol. Rep. 15, 11–23. doi: 10.1016/j.btre.2017.03.002
Dzionek, A., Wojcieszyńska, D., and Guzik, U. (2016). Natural carriers in bioremediation: a review. Electron. J. Biotechnol. 23, 28–36. doi: 10.1016/j.ejbt.2016.07.003
Enez, A., Hudek, L., and Bräu, L. (2018). Reduction in trace element mediated oxidative stress towards cropped plants via beneficial microbes in irrigated cropping systems: a review. Appl. Sci. 8:1953. doi: 10.3390/app8101953
Eng, M. L., Stutchbury, B. J., and Morrissey, C. A. (2017). Imidacloprid and chlorpyrifos insecticides impair migratory ability in a seed-eating songbird. Sci. Rep. 7, 1–9. doi: 10.1038/s41598-017-15446-x
Erdoğmuş, S. F., Eren, Y., Akyıl, D., Özkara, A., Konuk, M., and Sağlam, E. (2015). Evaluation of in vitro genotoxic effects of benfuracarb in human peripheral blood lymphocytes. Fresenius Environ. Bull. 24, 796–799.
Erinle, K. O., Jiang, Z., Ma, B., Li, J., Chen, Y., Ur-Rehman, K., et al. (2016). Exogenous calcium induces tolerance to atrazine stress in Pennisetum seedlings and promotes photosynthetic activity, antioxidant enzymes and psbA gene transcripts. Ecotoxicol. Environ. Saf. 132, 403–412. doi: 10.1016/j.ecoenv.2016.06.035
Espinosa-Ortiz, E. J., Shakya, M., Jain, R., Rene, E. R., van Hullebusch, E. D., and Lens, P. N. (2016). Sorption of zinc onto elemental selenium nanoparticles immobilized in Phanerochaete chrysosporium pellets. Environ. Sci. Pollut. Res. 23, 21619–21630. doi: 10.1007/s11356-016-7333-6
Farhan, M., Ahmad, M., Kanwal, A., Butt, Z. A., Khan, Q. F., Raza, S. A., et al. (2021). Biodegradation of chlorpyrifos using isolates from contaminated agricultural soil, its kinetic studies. Sci. Rep. 11, 1–14. doi: 10.1038/s41598-021-88264-x
Fernandes, A. F. T., Braz, V. S., Bauermeister, A., Paschoal, J. A. R., Lopes, N. P., and Stehling, E. G. (2018). Degradation of atrazine by Pseudomonas sp. and Achromobacter sp. isolated from Brazilian agricultural soil. Int. Biodeterior. Biodegradation 130, 17–22. doi: 10.1016/j.ibiod.2018.03.011
Forero-Mendieta, J. R., Castro-Vargas, H. I., Parada-Alfonso, F., and Guerrero-Dallos, J. A. (2012). Extraction of pesticides from soil using supercritical carbon dioxide added with methanol as co-solvent. J. Supercrit. Fluids 68, 64–70. doi: 10.1016/j.supflu.2012.03.017
Fouda, A., Hassan, S. E. D., Abdel-Rahman, M. A., Farag, M. M., Shehal-Deen, A., Mohamed, A. A., et al. (2021). Catalytic degradation of wastewater from the textile and tannery industries by green synthesized hematite (α-Fe2O3) and magnesium oxide (MgO) nanoparticles. Curr. Res. Biotechnol. 3, 29–41. doi: 10.1016/j.crbiot.2021.01.004
Fraceto, L. F., Grillo, R., de Medeiros, G. A., Scognamiglio, V., Rea, G., and Bartolucci, C. (2016). Nanotechnology in agriculture: which innovation potential does it have? Front. Environ. Sci. 4, 20–21. doi: 10.3389/fenvs.2016.00020
Gangola, S., Sharma, A., Bhatt, P., Khati, P., and Chaudhary, P. (2018). Presence of esterase and laccase in Bacillus subtilis facilitates biodegradation and detoxification of cypermethrin. Sci. Rep. 8, 1–11. doi: 10.1038/s41598-018-31082-5
Gaur, N., Flora, G., Yadav, M., and Tiwari, A. (2014). A review with recent advancements on bioremediation-based abolition of heavy metals. Environ. Sci. Process Impacts 16, 180–193. doi: 10.1039/C3EM00491K
Geed, S. R., Kureel, M. K., Giri, B. S., Singh, R. S., and Rai, B. N. (2017). Performance evaluation of Malathion biodegradation in batch and continuous packed bed bioreactor (PBBR). Bioresour. Technol. 227, 56–65. doi: 10.1016/j.biortech.2016.12.020
Geed, S. R., Kureel, M. K., Shukla, A. K., Singh, R. S., and Rai, B. N. (2016). Biodegradation of malathion and evaluation of kinetic parameters using three bacterial species. Resour.-Effic. Technol. 2, S3–S11. doi: 10.1016/j.reffit.2016.09.005
Gholami-Shabani, M., Shams-Ghahfarokhi, M., Gholami-Shabani, Z., and Razzaghi-Abyaneh, M. (2016). “Microbial enzymes: current features and potential applications in nanobiotechnology” in Advances and Applications Through Fungal Nanobiotechnology. ed. R. Prasad (Cham: Springer), 91–127.
Gholizadeh, A. M., Zarei, M., Ebratkhahan, M., and Hasanzadeh, A. (2021). Phenazopyridine degradation by electro-Fenton process with magnetite nanoparticles-activated carbon cathode, artificial neural networks modeling. J. Environ. Chem. Eng. 9:104999. doi: 10.1016/j.jece.2020.104999
Giri, B. S., Geed, S., Vikrant, K., Lee, S. S., Kim, K. H., Kailasa, S. K., et al. (2021). Progress in bioremediation of pesticide residues in the environment. Environ. Eng. Res. 26:20046. doi: 10.4491/eer.2020.446
Gole, A., John, D., Krishnamoorthy, K., Wagh, N. S., Lakkakula, J., Khan, M. S., et al. (2022). Role of Phytonanotechnology in the removal of water contamination. J. Nanomater. 2022, 1–19. doi: 10.1155/2022/7957007
Gomes, H. I., Fan, G., Mateus, E. P., Dias-Ferreira, C., and Ribeiro, A. B. (2014). Assessment of combined electro–nanoremediation of molinate contaminated soil. Sci. Total Environ. 493, 178–184. doi: 10.1016/j.scitotenv.2014.05.112
Goswami, R. K., Mehariya, S., Verma, P., Lavecchia, R., and Zuorro, A. (2021). Microalgae-based biorefineries for sustainable resource recovery from wastewater. J. Water Process Eng 40:101747. doi: 10.1016/j.jwpe.2020.101747
Gulzar, A. B. M., and Mazumder, P. B. (2022). Helping plants to deal with heavy metal stress: the role of nanotechnology and plant growth promoting rhizobacteria in the process of phytoremediation. Environ. Sci. Pollut. Res. 29, 40319–40341. doi: 10.1007/s11356-022-19756-0
Hallmann, C. A., Sorg, M., Jongejans, E., Siepel, H., Hofland, N., Schwan, H., et al. (2017). More than 75 percent decline over 27 years in total flying insect biomass in protected areas. PLoS One 12:e0185809. doi: 10.1371/journal.pone.0185809
Hassan, A., Pariatamby, A., Ossai, I. C., Ahmed, A., Muda, M. A., Wen, T. Z., et al. (2022). Bioaugmentation-assisted bioremediation and kinetics modelling of heavy metal-polluted landfill soil. Int. J. Environ. Sci. Technol. 19, 6729–6754. doi: 10.1007/s13762-021-03626-2
He, J., and Chen, J. P. (2014). A comprehensive review on biosorption of heavy metals by algal biomass: materials, performances, chemistry, and modeling simulation tools. Bioresour. Technol. 160, 67–78. doi: 10.1016/j.biortech.2014.01.068
He, K., Chen, G., Zeng, G., Huang, Z., Guo, Z., Huang, T., et al. (2017). Applications of white rot fungi in bioremediation with nanoparticles and biosynthesis of metallic nanoparticles. Appl. Microbiol. Biotechnol. 101, 4853–4862. doi: 10.1007/s00253-017-8328-z
Hong, Q., Zhang, Z., Hong, Y., and Li, S. (2007). A microcosm study on bioremediation of fenitrothion-contaminated soil using Burkholderia sp. FDS-1. Int. Biodeterior. Biodegradation 59, 55–61. doi: 10.1016/j.ibiod.2006.07.013
Horejs, C. (2022). Nanoparticle transport in plants. Nat. Rev. Mater 7:5. doi: 10.1038/s41578-021-00403-y
Huang, Z., Chen, G., Zeng, G., Guo, Z., He, K., Hu, L., et al. (2017). Toxicity mechanisms and synergies of silver nanoparticles in 2,4-dichlorophenol degradation by Phanerochaete chrysosporium. J. Hazard. Mater. 321, 37–46. doi: 10.1016/j.jhazmat.2016.08.075
Huang, H. S., Lee, K. W., Ho, C. H., Hsu, C. C., Su, S. B., Wang, J. J., et al. (2017). Increased risk for hypothyroidism after anticholinesterase pesticide poisoning: a nationwide population-based study. Endocrine 57, 436–444. doi: 10.1007/s12020-017-1373-7
Huang, Y., Xiao, L., Li, F., Xiao, M., Lin, D., Long, X., et al. (2018). Microbial degradation of pesticide residues and an emphasis on the degradation of cypermethrin and 3-phenoxy benzoic acid: a review. Molecules 23:2313. doi: 10.3390/molecules23092313
Hung, C. M., Huang, C. P., Chen, C. W., and Dong, C. D. (2021). Degradation of organic contaminants in marine sediments by peroxymonosulfate over LaFeO3 nanoparticles supported on water caltrop shell-derived biochar and the associated microbial community responses. J. Hazard. Mater. 420:126553. doi: 10.1016/j.jhazmat.2021.126553
Husain, S., Sardar, M., and Fatma, T. (2015). Screening of cyanobacterial extracts for synthesis of silver nanoparticles. World J. Microbiol. Biotechnol. 31, 1279–1283. doi: 10.1007/s11274-015-1869-3
Iravani, S., Thota, S., and Crans, D. (2017). Methods for Preparation of Metal Nanoparticles. 63, 15–31. Weinheim: Wiley.
Jaiswal, S., Singh, D. K., and Shukla, P. (2022). Lindane bioremediation by Paenibacillus dendritiformis SJPS-4, its metabolic pathway analysis and functional gene annotation. Environ. Technol. Innov. 27:102433. doi: 10.1016/j.eti.2022.102433
Jiang, Y., Yang, F., Dai, M., Ali, I., Shen, X., Hou, X., et al. (2022). Application of microbial immobilization technology for remediation of Cr (VI) contamination: a review. Chemosphere 286:131721. doi: 10.1016/j.chemosphere.2021.131721
Juárez-Maldonado, A., Ortega-Ortíz, H., Morales-Díaz, A. B., González-Morales, S., Morelos-Moreno, Á., Cabrera-De la Fuente, M., et al. (2019). Nanoparticles and nanomaterials as plant biostimulants. Int. J. Mol. Sci. 20:162. doi: 10.3390/ijms20010162
Kalidhasan, S., Dror, I., and Berkowitz, B. (2017). Atrazine degradation through PEI-copper nanoparticles deposited onto montmorillonite and sand. Sci. Rep. 7, 1–13. doi: 10.1038/s41598-017-01429-5
Karafas, E. S., Romanias, M. N., Stefanopoulos, V., Binas, V., Zachopoulos, A., Kiriakidis, G., et al. (2019). Effect of metal doped and co-doped TiO2 photocatalysts oriented to degrade indoor/outdoor pollutants for air quality improvement. A kinetic and product study using acetaldehyde as probe molecule. J. Photochem. Photobiol. A Chem. 371, 255–263. doi: 10.1016/j.jphotochem.2018.11.023
Karimi, H., Mahdavi, S., Asgari Lajayer, B., Moghiseh, E., Rajput, V. D., Minkina, T., et al. (2022). Insights on the bioremediation technologies for pesticide-contaminated soils. Environ. Geochem. Health 44, 1329–1354. doi: 10.1007/s10653-021-01081-z
Keum, Y. S., Lee, Y. J., and Kim, J. H. (2008). Metabolism of nitrodiphenyl ether herbicides by dioxin-degrading bacterium Sphingomonas wittichii RW1. J Agri Food Chem 56, 9146–9151. doi: 10.1021/jf801362k
Khanna, P., Kaur, A., and Goyal, D. (2019). Algae-based metallic nanoparticles: synthesis, characterization and applications. J. Microbiol. Methods 163:105656. doi: 10.1016/j.mimet.2019.105656
Kranjc, E., and Drobne, D. (2019). Nanomaterials in plants: a review of hazard and applications in the Agri-food sector. Nano 9:1094. doi: 10.3390/nano9081094
Krohn, C., Jin, J., Wood, J. L., Hayden, H. L., Kitching, M., Ryan, J., et al. (2021). Highly decomposed organic carbon mediates the assembly of soil communities with traits for the biodegradation of chlorinated pollutants. J. Hazard. Mater. 404:124077. doi: 10.1016/j.jhazmat.2020.124077
Kumari, A., Kumari, P., Rajput, V. D., Sushkova, S. N., and Minkina, T. (2022). Metal (loid) nanosorbents in restoration of polluted soils: geochemical, ecotoxicological, and remediation perspectives. Environ. Geochem. Health 44, 235–246. doi: 10.1007/s10653-021-00996-x
Laslo, V., Pinzaru, S. C., Zaguła, G., Kluz, M., Vicas, S. I., and Cavalu, S. (2022). Synergic effect of selenium nanoparticles and lactic acid bacteria in reduction cadmium toxicity. J. Mol. Struct. 1247:131325. doi: 10.1016/j.molstruc.2021.131325
Li, Q., Chen, X., Zhuang, J., and Chen, X. (2016). Decontaminating soil organic pollutants with manufactured nanoparticles. Environ. Sci. Pollut. Res. 23, 11533–11548. doi: 10.1007/s11356-016-6255-7
Li, Z., Yuan, S., Wan, J., Long, H., and Tong, M. (2011). A combination of electrokinetics and Pd/Fe PRB for the remediation of pentachlorophenol-contaminated soil. J. Contam. Hydrol. 124, 99–107. doi: 10.1016/j.jconhyd.2011.03.002
Lin, W., Huang, Z., Li, X., Liu, M., and Cheng, Y. (2016). Bio-remediation of acephate–Pb (II) compound contaminants by Bacillus subtilis FZUL-33. J. Environ. Sci. 45, 94–99. doi: 10.1016/j.jes.2015.12.010
Liu, L., Li, W., Song, W., and Guo, M. (2018). Remediation techniques for heavy metal-contaminated soils: principles and applicability. Sci. Total Environ. 633, 206–219. doi: 10.1016/j.scitotenv.2018.03.161
Liu, C., Xu, X., and Fan, J. (2015). Accelerated anaerobic dechlorination of DDT in slurry with Hydragric Acrisols using citric acid and anthraquinone-2, 6-disulfonate (AQDS). J. Environ. Sci. 38, 87–94. doi: 10.1016/j.jes.2015.05.005
Liu, R., Zhang, H., and Lal, R. (2016). Effects of stabilized nanoparticles of copper, zinc, manganese, and iron oxides in low concentrations on lettuce (Lactuca sativa) seed germination: nanotoxicants or nanonutrients? Water Air Soil Pollut. 227, 1–14. doi: 10.1007/s11270-015-2738-2
Madhura, L., Singh, S., Kanchi, S., Sabela, M., and Bisetty, K. (2019). Nanotechnology-based water quality management for wastewater treatment. Environ. Chem. Lett. 17, 65–121. doi: 10.1007/s10311-018-0778-8
Mahanty, S., Chatterjee, S., Ghosh, S., Tudu, P., Gaine, T., and Bakshi, M. (2020). Synergistic approach towards the sustainable management of heavy metals in wastewater using mycosynthesized iron oxide nanoparticles: biofabrication, adsorptive dynamics and chemometric modeling study. J. Water Process. Eng. 37:101426. doi: 10.1016/j.jwpe.2020.101426
Mahmoud, M. E., Abdou, A. E., Mohamed, S. M., and Osman, M. M. (2016). Engineered Staphylococcus aureus via immobilization on magnetic Fe3O4-phthalate nanoparticles for biosorption of divalent ions from aqueous solutions. J. Environ. Chem. Eng. 4, 3810–3824. doi: 10.1016/j.jece.2016.08.022
Malghani, S., Chatterjee, N., Yu, H. X., and Luo, Z. (2009). Isolation and identification of profenofos degrading bacteria. Braz. J. Microbiol. 40, 893–900. doi: 10.1590/S1517-83822009000400021
Mao, Y. E., Xing-Lun, Y. A. N. G., Ming-Ming, S. U. N., Yong-Rong, B. I. A. N., Fang, W. A. N. G., Cheng-Gang, G. U., et al. (2013). Use of organic solvents to extract organochlorine pesticides (OCPs) from aged contaminated soils. Pedosphere 23, 10–19. doi: 10.1016/S1002-0160(12)60075-7
Maqbool, Z., Hussain, S., Imran, M., Mahmood, F., Shahzad, T., Ahmed, Z., et al. (2016). Perspectives of using fungi as bioresource for bioremediation of pesticides in the environment: a critical review. Environ. Sci. Pollut. Res. 23, 16904–16925. doi: 10.1007/s11356-016-7003-8
Marlatt, V. L., Bayen, S., Castaneda-Cortès, D., Delbès, G., Grigorova, P., Langlois, V. S., et al. (2022). Impacts of endocrine disrupting chemicals on reproduction in wildlife and humans. Environ. Res. 208:112584. doi: 10.1016/j.envres.2021.112584
Martínez-Macias, M. D. R., Correa-Murrieta, M. A., Villegas-Peralta, Y., Dévora-Isiordia, G. E., Álvarez-Sánchez, J., Saldivar-Cabrales, J., et al. (2019). Uptake of copper from acid mine drainage by the microalgae Nannochloropsis oculata. Environ. Sci. Pollut. Res. 26, 6311–6318. doi: 10.1007/s11356-018-3963-1
Mathur, M., and Gehlot, P. (2021). “Mechanistic evaluation of bioremediation properties of fungi” in New and Future Developments in Microbial Biotechnology and Bioengineering. eds. J. Singh and P. Gehlot (Amsterdam, The Netherlands: Elsevier), 267–286.
Mehrotra, T., Dev, S., Banerjee, A., Chatterjee, A., Singh, R., and Aggarwal, S. (2021). Use of immobilized bacteria for environmental bioremediation: a review. J. Environ. Chem. Eng. 9:105920. doi: 10.1016/j.jece.2021.105920
Morillo, E., and Villaverde, J. (2017). Advanced technologies for the remediation of pesticide-contaminated soils. Sci. Total Environ. 586, 576–597. doi: 10.1016/j.scitotenv.2017.02.020
Nadi, A., Boyer, D., Charbonnel, N., Boukhriss, A., Forestier, C., and Gmouh, S. (2019). Immobilisation of bacteria onto magnetic nanoparticles for the decolorisation and degradation of azo dyes. IET Nanobiotechnol. 13, 144–149. doi: 10.1049/iet-nbt.2018.5026
Naeeni, M. H., Yamini, Y., and Rezaee, M. (2011). Combination of supercritical fluid extraction with dispersive liquid–liquid microextraction for extraction of organophosphorus pesticides from soil and marine sediment samples. J. Supercrit. Fluids 57, 219–226. doi: 10.1016/j.supflu.2011.03.005
Narayanan, M., Kumarasamy, S., Ranganathan, M., Kandasamy, S., Kandasamy, G., and Gnanavel, K. (2020). Enzyme and metabolites attained in degradation of chemical pesticides ß Cypermethrin by Bacillus cereus. Mater. Today Proc. 33, 3640–3645. doi: 10.1016/j.jhazmat.2011.02.069
Narayanan, K. B., and Sakthivel, N. (2011). Synthesis and characterization of nano-gold composite using Cylindrocladium floridanum and its heterogeneous catalysis in the degradation of 4-nitrophenol. J. Hazard. Mater. 189, 519–525. doi: 10.1016/j.jhazmat.2011.02.069
Nicolopoulou-Stamati, P., Maipas, S., Kotampasi, C., Stamatis, P., and Hens, L. (2016). Chemical pesticides and human health: the urgent need for a new concept in agriculture. Front. Public Health 4, 148–155. doi: 10.3389/fpubh.2016.00148
Nwanya, A. C., Razanamahandry, L. C., Bashir, A. K. H., Ikpo, C. O., Nwanya, S. C., Botha, S., et al. (2019). Industrial textile effluent treatment and antibacterial effectiveness of Zea mays L. dry husk mediated bio-synthesized copper oxide nanoparticles. J. Hazard. Mater. 375, 281–289. doi: 10.1016/j.jhazmat.2019.05.004
Oliveira, J. M., Destro, A. L. F., Freitas, M. B., and Oliveira, L. L. (2020). How do pesticides affect bats?–A brief review of recent publications. Braz. J. Biol. 81, 499–507. doi: 10.1590/1519-6984.225330
Organization for Economic Co-operation and Development (OECD) (2019). Biodiversity: France and the Economics and Business Case for Action. Report Prepared for G7 Environment Ministers Meeting. Metz, 29-30.
Ovais, M., Khalil, A. T., Ayaz, M., Ahmad, I., Nethi, S. K., and Mukherjee, S. (2018). Biosynthesis of metal nanoparticles via microbial enzymes: a mechanistic approach. Int. J. Mol. Sci. 19:4100. doi: 10.3390/ijms19124100
Oyebamiji, O. O., Boeing, W. J., Holguin, F. O., Ilori, O., and Amund, O. (2019). Green microalgae cultured in textile wastewater for biomass generation and biodetoxification of heavy metals and chromogenic substances. Bioresour. Technol Rep 7:100247. doi: 10.1016/j.biteb.2019.100247
Oyewole, O. A., Raji, R. O., Musa, I. O., Enemanna, C. E., Abdulsalam, O. N., and Yakubu, J. G. (2019). Enhanced degradation of crude oil with Alcaligenes faecalis ADY25 and iron oxide nanoparticle. Int. J. Biol. Res. 10, 62–72.
Pailan, S., Gupta, D., Apte, S., Krishnamurthi, S., and Saha, P. (2015). Degradation of organophosphate insecticide by a novel bacillus aryabhattai strain SanPS1, isolated from soil of agricultural field in Burdwan, West Bengal, India. Int. Biodeterior. Biodegradation 103, 191–195. doi: 10.1016/j.ibiod.2015.05.006
Paliwal, R., Uniyal, S., and Rai, J. P. N. (2015). Evaluating the potential of immobilized bacterial consortium for black liquor biodegradation. Environ. Sci. Pollut. Res. 22, 6842–6853. doi: 10.1007/s11356-014-3872-x
Pandey, G. (2018). Prospects of nanobioremediation in environmental cleanup. Orient. J. Chem. 34, 2838–2850. doi: 10.13005/ojc/340622
Pandya, K., Rajhans, S., Pandya, H., and Mankad, A. (2022). Phytoremediation: an innovative perspective for reclaiming contaminated environment using ornamental plants. World J. Adv. Res. Rev. 13, 001–014. doi: 10.30574/wjarr.2022.13.2.0088
Patil, S. S., Shedbalkar, U. U., Truskewycz, A., Chopade, B. A., and Ball, A. S. (2016). Nanoparticles for environmental clean-up: a review of potential risks and emerging solutions. Environ. Technol. Innov. 5, 10–21. doi: 10.1016/j.eti.2015.11.001
Phelps, J. D., Strang, C. G., and Sherry, D. F. (2020). Imidacloprid impairs performance on a model flower handling task in bumblebees (Bombus impatiens). Ecotoxicology 29, 359–374. doi: 10.1007/s10646-020-02182-8
Pillai, H. P., and Kottekottil, J. (2016). Nano-phytotechnological remediation of endosulfan using zero valent iron nanoparticles. J. Environ. Prot. Sci. 7, 734–744. doi: 10.4236/jep.2016.75066
Priyanka, J. V., Rajalakshmi, S., Kumar, P. S., Krishnaswamy, V. G., Al Farraj, D. A., Elshikh, M. S., et al. (2022). Bioremediation of soil contaminated with toxic mixed reactive azo dyes by co-cultured cells of Enterobacter cloacae and Bacillus subtilis. Environ. Res. 204:112136. doi: 10.1016/j.envres.2021.112136
Qiu, X., Zhong, Q., Li, M., Bai, W., and Li, B. (2007). Biodegradation of p-nitrophenol by methyl parathion-degrading Ochrobactrum sp. B2. Int. Biodeterior. Biodegradation 59, 297–301. doi: 10.1016/j.ibiod.2006.09.005
Raffa, C. M., and Chiampo, F. (2021). Bioremediation of agricultural soils polluted with pesticides: a review. Bioengineering 8:92. doi: 10.3390/bioengineering8070092
Rajput, V. D., Minkina, T., Kumari, A., Shende, S. S., Ranjan, A., Faizan, M., et al. (2022). A review on nanobioremediation approaches for restoration of contaminated soil. Eurasian J. Soil Sci 11, 43–60. doi: 10.18393/ejss.990605
Ramakrishna, M. P., Prasad, R. M., Huchegowda, S., Ramanna, M., Sharma, M. K., and Huchegowda, R. (2022). Adverse effects of plasticizers and pesticides on female reproductive health. J. Psychosexual Health 4, 38–42. doi: 10.1177/26318318211060292
Rambabu, K., Bharath, G., Banat, F., and Show, P. L. (2021). Green synthesis of zinc oxide nanoparticles using Phoenix dactylifera waste as bioreductant for effective dye degradation and antibacterial performance in wastewater treatment. J. Hazard. Mater. 402:123560. doi: 10.1016/j.jhazmat.2020.123560
Ramos-Ruiz, A., Field, J. A., Wilkening, J. V., and Sierra-Alvarez, R. (2016). Recovery of elemental tellurium nanoparticles by the reduction of tellurium oxyanions in a methanogenic microbial consortium. Environ. Sci. Technol. 50, 1492–1500. doi: 10.1021/acs.est.5b04074
Rasha, F. M. (2017). Intracellular siderophore detection in an Egyptian, cobalt-treated F. solani isolate using SEM-EDX with reference to its tolerance. Pol. J. Microbiol. 66, 235–243. doi: 10.5604/01.3001.0010.7856
Rasool, S., Rasool, T., and Gani, K. M. (2022). A review of interactions of pesticides within various interfaces of intrinsic and organic residue amended soil environment. Chem. Eng. J. Adv. 11:100301. doi: 10.1016/j.ceja.2022.100301
Ribeiro, A. B., Mateus, E. P., and Rodríguez-Maroto, J. M. (2011). Removal of organic contaminants from soils by an electrokinetic process: the case of molinate and bentazone. Experimental and modeling. Sep. Purif. Technol. 79, 193–203. doi: 10.1016/j.seppur.2011.01.045
Risco, C., Rodrigo, S., López-Vizcaíno, R., Yustres, A., Sáez, C., Cañizares, P., et al. (2015). Electrochemically assisted fences for the electroremediation of soils polluted with 2, 4-D: a case study in a pilot plant. Sep. Purif. Technol. 156, 234–241. doi: 10.1016/j.seppur.2015.10.006
Risco, C., Rodrigo, S., Vizcaíno, R. L., Yustres, A., Saez, C., Cañizares, P., et al. (2016). Removal of oxyfluorfen from spiked soils using electrokinetic soil flushing with linear rows of electrodes. Chem. Eng. J. 294, 65–72. doi: 10.1016/j.cej.2016.02.112
Robinson, L. I., and Piatt, E. R. (2019). En Rx chemical Inc, soil and water contamination remediation injector and method of use. U.S. Patent 10252304.
Rosas, J. M., Vicente, F., Saguillo, E. G., Santos, A., and Romero, A. (2014). Remediation of soil polluted with herbicides by Fenton-like reaction: kinetic model of diuron degradation. Appl Catal B 144, 252–260. doi: 10.1016/j.apcatb.2013.07.011
Sajid, M., Ilyas, M., Basheer, C., Tariq, M., Daud, M., Baig, N., et al. (2015). Impact of nanoparticles on human and environment: review of toxicity factors, exposures, control strategies, and future prospects. Environ. Sci. Pollut. Res. 22, 4122–4143. doi: 10.1007/s11356-014-3994-1
Saljooqi, A., Shamspur, T., and Mostafavi, A. (2020). Synthesis and photocatalytic activity of porous ZnO stabilized by TiO2 and Fe3O4 nanoparticles: investigation of pesticide degradation reaction in water treatment. Environ. Sci. Pollut. Res. 28, 9146–9156. doi: 10.1007/s11356-020-11122-2
Samal, D. K., Sukla, L. B., Pattanaik, A., and Pradhan, D. (2020). Role of microalgae in treatment of acid mine drainage and recovery of valuable metals. Mater. Today Proc. 30, 346–350. doi: 10.1016/j.matpr.2020.02.165
Santos, L. M., Amorim, K. P. D., Andrade, L. S., Batista, P. S., Trovó, A. G., and Machado, A. E. (2015). Dye degradation enhanced by coupling electrochemical process and heterogeneous photocatalysis. J. Braz. Chem. Soc. 26, 1817–1823. doi: 10.5935/0103-5053.20150158
Schmidt, R. J., Kogan, V., Shelton, J. F., Delwiche, L., Hansen, R. L., Ozonoff, S., et al. (2017). Combined prenatal pesticide exposure and folic acid intake in relation to autism spectrum disorder. Environ. Health Perspect. 125:097007. doi: 10.1289/EHP604
Schwab, F., Zhai, G., Kern, M., Turner, A., Schnoor, J. L., and Wiesner, M. R. (2016). Barriers, pathways and processes for uptake, translocation and accumulation of nanomaterials in plants–critical review. Nanotoxicology 10, 257–278. doi: 10.3109/17435390.2015.1048326
Shah, A., Haq, S., Rehman, W., Waseem, M., Shoukat, S., and Rehman, M. (2019). Photocatalytic and antibacterial activities of paeonia emodi mediated silver oxide nanoparticles. Mater. Res. Express 6:45045. doi: 10.1088/2053-1591/aafd42
Sharma, I. (2020). “Bioremediation techniques for polluted environment: concept, advantages, limitations, and prospects,” in Trace Metals in the Environment-New Approaches and Recent Advances.eds. M. A. Murillo-Tovar, H. Saldarriaga-Noreña, and A. Saeid (London: IntechOpen)
Sharma, T., Toor, A. P., and Rajor, A. (2015). Photocatalytic degradation of imidacloprid in soil: application of response surface methodology for the optimization of parameters. RSC Adv. 5, 25059–25065. doi: 10.1039/C5RA02224J
Shi, C., Zhu, N., Cao, Y., and Wu, P. (2015). Biosynthesis of gold nanoparticles assisted by the intracellular protein extract of Pycnoporus sanguineus and its catalysis in degradation of 4-nitroaniline. Nanoscale Res. Lett. 10, 1–8. doi: 10.1186/s11671-015-0856-9
Singh, R., Behera, M., and Kumar, S. (2020). “Nano-bioremediation: an innovative remediation technology for treatment and management of contaminated sites,” in Bioremediation of Industrial Waste for Environmental Safety. eds. R. N. Bharagava and G. Saxena (Singapore: Springer), 165–182.
Singh, J., Dutta, T., Kim, K. H., Rawat, M., Samddar, P., and Kumar, P. (2018). ‘Green’ synthesis of metals and their oxide nanoparticles: applications for environmental remediation. J. Nanobiotechnol. 16, 1–24. doi: 10.1186/s12951-018-0408-4
Singh, S., Gupta, A., Waswani, H., Prasad, M., and Ranjan, R. (2022). “Impact of pesticides on the ecosystem,” in Agrochemicals in Soil and Environment. eds. M. Naeem, J. F. J. Bremont, A. A. Ansari, and S. S. Gill (Singapore: Springer), 157–181.
Singh, R., Manickam, N., Mudiam, M. K. R., Murthy, R. C., and Misra, V. (2013). An integrated (nano-bio) technique for degradation of γ-HCH contaminated soil. J. Hazard. Mater. 258-259, 35–41. doi: 10.1016/j.jhazmat.2013.04.016
Singh, M. P., Vishwakarma, S. K., and Srivastava, A. K. (2013). Bioremediation of direct blue 14 and extracellular ligninolytic enzyme production by white rot fungi: Pleurotus sp. Biomed. Res. Int. 2013, 1–4. doi: 10.1155/2013/180156
Singh, B. K., Walker, A., Morgan, J. A. W., and Wright, D. J. (2004). Biodegradation of chlorpyrifos by Enterobacter strain B-14 and its use in bioremediation of contaminated soils. Appl. Environ. Microbiol. 70, 4855–4863. doi: 10.1128/AEM.70.8.4855-4863.2004
Souri, Z., Karimi, N., Sarmadi, M., and Rostami, E. (2017). Salicylic acid nanoparticles (SANPs) improve growth and phytoremediation efficiency of Isatis cappadocica Desv., under as stress. IET Nanobiotechnol. 11, 650–655. doi: 10.1049/iet-nbt.2016.0202
Souza, F. L., Saéz, C., Llanos, J., Lanza, M. R., Cañizares, P., and Rodrigo, M. A. (2016). Solar-powered electrokinetic remediation for the treatment of soil polluted with the herbicide 2, 4-D. Electrochim. Acta 190, 371–377. doi: 10.1016/j.electacta.2015.12.134
Sultana, N., Hossain, S. M., Mohammed, M. E., Irfan, M. F., Haq, B., Faruque, M. O., et al. (2020). Experimental study and parameters optimization of microalgae based heavy metals removal process using a hybrid response surface methodology-crow search algorithm. Sci. Rep. 10, 1–15. doi: 10.1038/s41598-020-72236-8
Suman, J., Uhlik, O., Viktorova, J., and Macek, T. (2018). Phytoextraction of heavy metals: a promising tool for clean-up of polluted environment? Front. Plant Sci. 9:1476. doi: 10.3389/fpls.2018.01476
Sun, S., Sidhu, V., Rong, Y., and Zheng, Y. (2018). Pesticide pollution in agricultural soils and sustainable remediation methods: a review. Curr. Pollut. Rep. 4, 240–250. doi: 10.1007/s40726-018-0092-x
Sunny, N. E., Kaviya, A., and Kumar, S. V. (2022). “Mechanistic approach on the synthesis of metallic nanoparticles from microbes,” in Agri-waste and Microbes for Production of Sustainable Nanomaterials. eds. K. Abd-Elsalam, R. Periakaruppan, and S. Rajeshkumar (Elsevier), 577–602.
Tan, W., Peralta-Videa, J. R., and Gardea-Torresdey, J. L. (2018). Interaction of titanium dioxide nanoparticles with soil components and plants: current knowledge and future research needs–a critical review. Environ. Sci. Nano 5, 257–278. doi: 10.1039/C7EN00985B
Tang, A., Wang, B., Liu, Y., Li, Q., Tong, Z., and Wei, Y. (2015). Biodegradation and extracellular enzymatic activities of Pseudomonas aeruginosa strain GF31 on β-cypermethrin. Environ. Sci. Pollut. Res. 22, 13049–13057. doi: 10.1007/s11356-015-4545-0
Teodoro, M., Briguglio, G., Fenga, C., and Costa, C. (2019). Genetic polymorphisms as determinants of pesticide toxicity: recent advances. Toxicol. Rep. 6, 564–570. doi: 10.1016/j.toxrep.2019.06.004
Thatoi, H., Behera, B. C., and Mishra, R. R. (2013). Ecological role and biotechnological potential of mangrove fungi: a review. Mycology 4, 54–71. doi: 10.1080/21501203.2013.785448
Torres-Badia, M., Solar-Malaga, S., Serrano, R., Garcia-Marin, L. J., and Bragado, M. J. (2022). The adverse impact of herbicide roundup ultra plus in human spermatozoa plasma membrane is caused by its surfactant. Sci. Rep. 12, 1–15. doi: 10.1038/s41598-022-17023-3
Upadhyaya, H., Panda, S. K., Bhattacharjee, M. K., and Dutta, S. (2010). Role of arbuscular mycorrhiza in heavy metal tolerance in plants: prospects for phytoremidiation. J. Phytology 2, 16–27.
Usman, M., Farooq, M., Wakeel, A., Nawaz, A., Cheema, S. A., Ur Rehman, H., et al. (2020). Nanotechnology in agriculture: current status, challenges and future opportunities. Sci. Total Environ. 721:137778. doi: 10.1016/j.scitotenv.2020.137778
Usman, M., Jellali, S., Anastopoulos, I., Charabi, Y., Hameed, B. H., and Hanna, K. (2022). Fenton oxidation for soil remediation: a critical review of observations in historically contaminated soils. J. Hazard. Mater. 424:127670. doi: 10.1016/j.jhazmat.2021.127670
Vacondio, B., Birolli, W. G., Ferreira, I. M., Seleghim, M. H., Gonçalves, S., Vasconcellos, S. P., et al. (2015). Biodegradation of pentachlorophenol by marine-derived fungus Trichoderma harzianum CBMAI 1677 isolated from ascidian Didemnun ligulum. Biocatal. Agric. Biotechnol. 4, 266–275. doi: 10.1016/j.bcab.2015.03.005
Vahabi, K., Mansoori, G. A., and Karimi, S. (2011). Biosynthesis of silver nanoparticles by fungus Trichoderma reesei (a route for large-scale production of AgNPs). Insci J. 1, 65–79. doi: 10.5640/insc.010165
Varma, A., and Khanuja, M. (2017). Role of nanoparticles on plant growth with special emphasis on Piriformospora indica: a review. Nanosci. Plant–Soil Syst. 48, 387–403. doi: 10.1007/978-3-319-46835-8_14
Vogel, G. (2017). Where have all the insects gone? Science 356, 576–579. doi: 10.1126/science.356.6338.576
Vicente, F., Santos, A., Sagüillo, E. G., Martínez-Villacorta, Á. M., Rosas, J. M., and Romero, A. (2012). Diuron abatement in contaminated soil using Fenton-like process. Chem. Eng. J. 183, 357–364.
Wang, H., Kim, B., and Wunder, S. L. (2015). Nanoparticle-supported lipid bilayers as an in situ remediation strategy for hydrophobic organic contaminants in soils. Environ. Sci. Technol. 49, 529–536. doi: 10.1021/es504832n
Wang, T. C., Qu, G., Li, J., and Liang, D. (2014). Evaluation and optimization of multi-channel pulsed discharge plasma system for soil remediation. Vacuum 103, 72–77. doi: 10.1016/j.vacuum.2013.12.012
Wang, T. C., Qu, G., Li, J., and Lu, N. (2013). Kinetics studies on pentachlorophenol degradation in soil during pulsed discharge plasma process. J. Electrostat. 71, 994–998.
Yadav, A., Kon, K., Kratosova, G., Duran, N., Ingle, A. P., and Rai, M. (2015). Fungi as an efficient mycosystem for the synthesis of metal nanoparticles: progress and key aspects of research. Biotechnol. Lett. 37, 2099–2120. doi: 10.1007/s10529-015-1901-6
Yadav, K. K., Singh, J. K., Gupta, N., and Kumar, V. J. J. M. E. S. (2017). A review of nano-bioremediation technologies for environmental cleanup: a novel biological approach. J. Mater. Environ. Sci. 8, 740–757.
Yang, J., Cao, W., and Rui, Y. (2017). Interactions between nanoparticles and plants: phytotoxicity and defense mechanisms. J. Plant Interact. 12, 158–169. doi: 10.1080/17429145.2017.1310944
Ye, M., Sun, M., Hu, F., Kengara, F. O., Jiang, X., Luo, Y., et al. (2014). Remediation of organochlorine pesticides (OCPs) contaminated site by successive methyl-β-cyclodextrin (MCD) and sunflower oil enhanced soil washing–Portulaca oleracea L. cultivation. Chemosphere 105, 119–125. doi: 10.1016/j.chemosphere.2013.12.058
Yunoki, A., Tsuchiya, E., Fukui, Y., Fujii, A., and Maruyama, T. (2014). Preparation of inorganic/organic polymer hybrid microcapsules with high encapsulation efficiency by an electrospray technique. ACS Appl. Mater. Interfaces 6, 11973–11979. doi: 10.1021/am503030c
Zaranyika, M. F., Matimati, E., and Mushonga, P. (2020). Degradation kinetics of DDT in tropical soils: a proposed multi-phase zero order kinetic model that takes into account evaporation, hydrolysis, photolysis, microbial degradation and adsorption by soil particulates. Sci. Afri. 9:e00467. doi: 10.1016/j.sciaf.2020.e00467
Zhang, M., Zhang, K., De Gusseme, B., Verstraete, W., and Field, R. (2014). The antibacterial and anti-biofouling performance of biogenic silver nanoparticles by lactobacillus fermentum. Biofouling 30, 347–357. doi: 10.1080/08927014.2013.873419
Zhang, H., Zhou, H., Bai, J., Li, Y., Yang, J., Ma, Q., et al. (2019). Biosynthesis of selenium nanoparticles mediated by fungus Mariannaea sp. HJ and their characterization. Colloids Surf. A Physicochem. Eng. Asp. 571, 9–16. doi: 10.1016/j.colsurfa.2019.02.070
Zhou, H., Sun, Q., Wang, X., Wang, L., Chen, J., Zhang, J., et al. (2014). Removal of 2, 4-dichlorophenol from contaminated soil by a heterogeneous ZVI/EDTA/air Fenton-like system. Sep. Purif. Technol. 132, 346–353. doi: 10.1016/j.seppur.2014.05.037
Keywords: pesticides, bioremediation, nano-bioremediation, microbial degradation, environment
Citation: Singh Y and Saxena MK (2022) Insights into the recent advances in nano-bioremediation of pesticides from the contaminated soil. Front. Microbiol. 13:982611. doi: 10.3389/fmicb.2022.982611
Received: 30 June 2022; Accepted: 16 September 2022;
Published: 19 October 2022.
Edited by:
Pankaj Bhatt, Purdue University, United StatesReviewed by:
Kalpana Bhatt, Gurukul Kangri Vishwavidyalaya, IndiaCopyright © 2022 Singh and Saxena. This is an open-access article distributed under the terms of the Creative Commons Attribution License (CC BY). The use, distribution or reproduction in other forums is permitted, provided the original author(s) and the copyright owner(s) are credited and that the original publication in this journal is cited, in accordance with accepted academic practice. No use, distribution or reproduction is permitted which does not comply with these terms.
*Correspondence: Mumtesh Kumar Saxena, bXVtdGVzaHNheGVuYUBnbWFpbC5jb20=
Disclaimer: All claims expressed in this article are solely those of the authors and do not necessarily represent those of their affiliated organizations, or those of the publisher, the editors and the reviewers. Any product that may be evaluated in this article or claim that may be made by its manufacturer is not guaranteed or endorsed by the publisher.
Research integrity at Frontiers
Learn more about the work of our research integrity team to safeguard the quality of each article we publish.