- 1Shaanxi Centre of Stem Cells Engineering and Technology, College of Veterinary Medicine, Northwest A&F University, Shaanxi, China
- 2State Key Laboratory for Molecular and Developmental Biology, Institute of Genetics and Developmental Biology, Chinese Academy of Sciences, Beijing, China
Gut microbiota (GM) is a major component of the gastrointestinal tract. Growing evidence suggests that it has various effects on many distal organs including the male reproductive system in mammals. GM and testis form the gut-testis axis involving the production of key molecules through microbial metabolism or de novo synthesis. These molecules have nutrition, immunity, and hormone-related functions and promote the male reproductive system via the circulatory system. GM helps maintain the integral structure of testes and regulates testicular immunity to protect the spermatogenic environment. Factors damaging GM negatively impact male reproductive function, however, the related mechanism is unknown. Also, the correlation between GM and testis remains to be yet investigated. This review discusses the complex influence of GM on the male reproductive system highlighting the impact on male fertility.
Introduction
Gut microbiota (GM) is an indispensable regulator of host metabolism, immunity, and endocrine functions. Its composition, abundance, metabolites, and signaling pathways significantly impact organ development starting from the local intestine to distal organs. The metabolic outcomes of GM determine key processes like lipid and bile metabolism, vitamin and short-chain fatty acids production, pathogens resistance, DNA expression and detoxification (Walter and Ley, 2011). The genetic and chemical diversity of GM is far greater than that of the host genome as GM includes trillions of symbiotic bacteria, virus, and fungi in the intestine (Lam et al., 2022; Schupack et al., 2022). As for bacteria, intestinal microenvironment is mainly conducive to the growth of six major bacterial phyla, including Firmicutes, Bacteroides, Proteobacteria, Actinomycetes, Verrucomicrobia, and Fusobacterium (Eckburg et al., 2005). Among them, Bacteroides and Firmicutes account for >90% (Qin et al., 2010), and their proportions change dynamically during different stages of animal life. Although a large part of GM remains conserved, evidence suggests that the microbial abundance of GM changes dynamically at the species level depending on the host’s age or health conditions (Yatsunenko et al., 2012). These features allow GM to work much better in different phases/health conditions. Although GM is dynamic, it has some basic functions regulating immunity, metabolism, and nervous system impacting the general physical and mental health of the host (Adak and Khan, 2019).
In GM mediated digestion of nutrients, the main end product of carbohydrates is short-chain fatty acids (SCFAs), which play a role in the metabolism and circulation of glucose and lipid. Three kinds of SCFAs, propionate, acetate, and butyrate, have roles in maintaining intestinal integrity and relieving inflammation (Morrison and Preston, 2016). Amino acids and short peptides produced in the digestive tract after proteolysis are used by GM to synthesize other kinds of proteins. Moreover, GM can enzymatically decompose protein products to generate energy or produce signaling molecules to regulate the physiological state of the host (Figure 1; Nicholson et al., 2012). The GM balance is very critical to the homeostasis of the host’s immune system. Beneficial strains strengthen the tight junctions of the intestine. In case of disturbed GM, immune responses are generated in the local intestine, which can gradually advance to inflammatory bowel disease (IBD). The integrity of the intestine is impaired by inflammation induced by bacteria-produced lipopolysaccharides (LPS) and inflammatory cytokines, which can circulate and spread to other organs (Ulluwishewa et al., 2011).
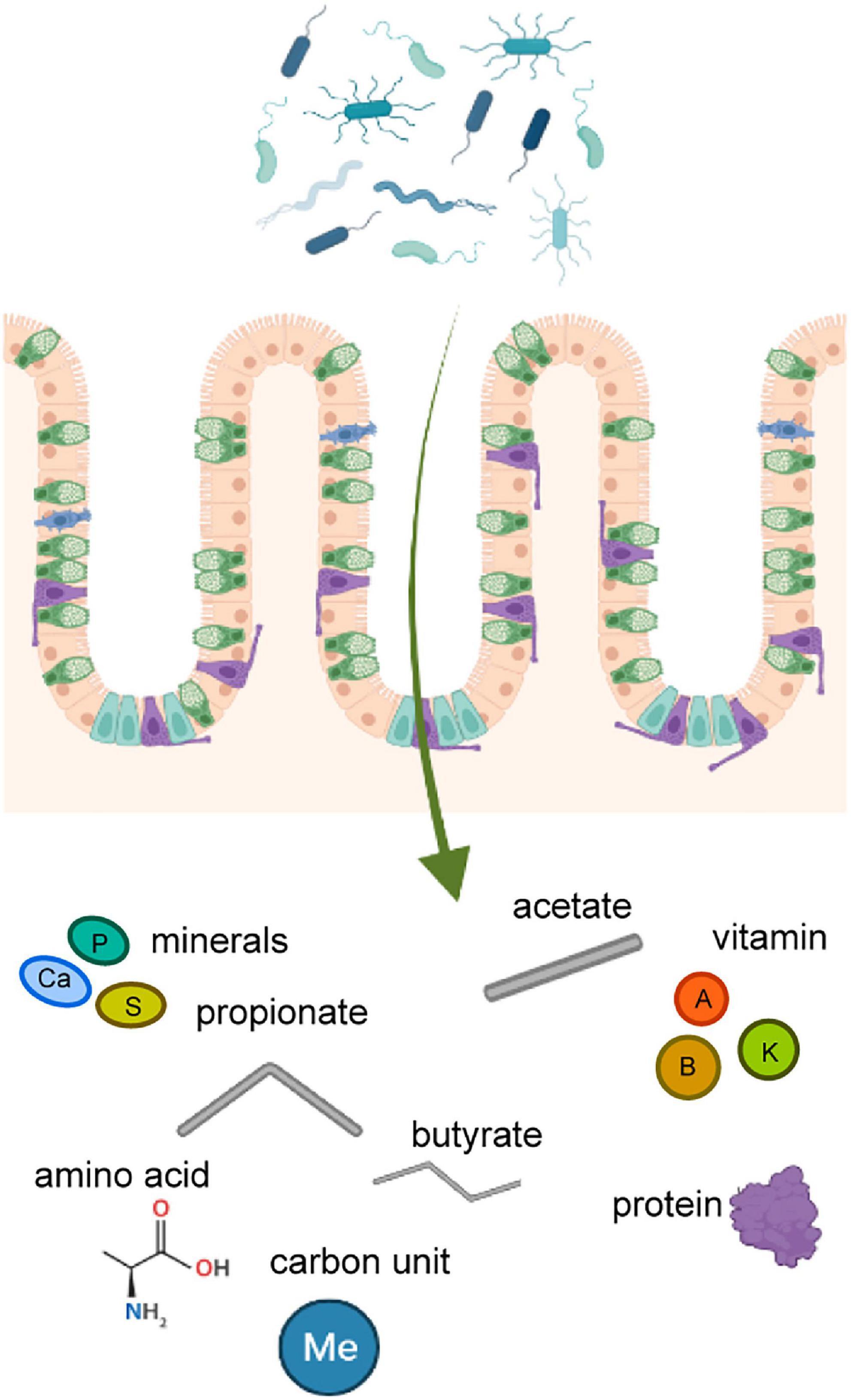
Figure 1. The nutrient substance produced by gut microbiota. Products secreted by gut microbiota in the intestine affect the distal parts of body through the circulatory system. These substances include amino acids, proteins, vitamins, minerals, fatty acids, and so on.
The health status or balance of the GM also affects the development and health of the male reproductive system of in mammals (Martinot et al., 2021). This effect could be positive or negative in nature (Guo et al., 2020; Liu et al., 2022). Certainly, the reproductive ability of male animals directly determines the survival and reproduction of organisms, which can become vulnerable at the time of imbalanced GM. Also, the testicles, wrapped in the scrotum outside the body cavity, are easily vulnerable to changes in the internal and external environment. Heat and cold stress, hormone levels and endocrine disruptors, dietary structure, exercise, growth and development, and congenital factors all have effects on the function of testis (Belloc et al., 2014; Tian et al., 2019; Qin et al., 2021). According to the World Health Organization (WHO), 15% of couples worldwide struggle with infertility, of which, 50% of cases of infertility are due to men having troubles such as varicoceles and azoospermia (Jensen et al., 2017; Wu et al., 2021).
The growth of the testis relies on germ and somatic cells. A mature testis produces sperm. Spermatozoa accumulate in the epididymis and are then discharged out of the penis through the deferens to complete the jaculation process. This process requires nutrients such as water, amino acids, lipids, carbohydrates, vitamins, and minerals. Germ cells achieve differentiation and maturation through the process of exchanging nutrients and metabolic wastes with Sertoli cells (Dance et al., 2015). In addition, the erection of the penis requires stimuli from various gas signaling molecules, which are majorly produced by cyclic metabolism in GM (Zmora et al., 2019). Recent studies found a small number of bacteria in testicles are similar to gut bacteria and semen can regulate certain male reproductive diseases (Altmae et al., 2019; Godia et al., 2020). From the perspective of the male reproductive system, the testes and penis protect the germ cells, while GM absorbs and metabolizes nutrients to ensure the functioning of male reproductive organs. This review aims to expound on the regulation of GM that in multiple ways promotes the growth and development of the male reproductive system.
Gut microbiota supports testis by metabolizing nutrients
Testes cannot de novo synthesize nutrients. The blood vessels in the testis transport nutrients, including those synthesized or metabolized by GM, from the digestive system to the testicular interstitium via the convoluted seminiferous tubules through Sertoli cells and their intercellular connections. Nutrients such as vitamins and minerals synthesized or metabolized by GM are essential for testes (Table 1). The changed microbiota may disturb the original nutritional structure and function of the testis.
Vitamin A
Vitamin A is an indispensable nutrient for the reproductive system and embryonic development. One of the metabolic forms of vitamin A is retinoic acid (RA), which prompts the stagnant spermatogonial stem cells in the G0/G1 stage in the embryo to initiate meiosis for differentiation into mature sperms. Vitamin A deficiency leads to the failure of type A spermatogonial stem cells differentiation into type A1; the stratified squamous keratinizing epithelium replaces epithelia of the prostate, epididymis, and seminal vesicle, slowing sperm production (Clagett-Dame and Knutson, 2011). In humans and mice, the expression of two genes related to spermatogenesis (Stra8 and Rec8) is promoted by RA. Without the expression of Stra8, undifferentiated spermatogonia are difficult to accumulate and differentiate, which causes the failure of meiosis. In Sertoli cells, RA binds to retinoic acid receptor (RAR) recruiting retinoid X receptors (RXRs), which promotes the transcription process. Interference of RAR or RXRs in Sertoli cells blocking the RA-RAR/RXR signaling causes the failure of the blood-testis barrier (BTB), which forbids sperms to mature and release from Sertoli cells (Schleif et al., 2022). GM plays an important role in regulating the intestinal absorption and metabolism of vitamin A. Proteins produced by Escherichia coli like RXRs and farnesoid X receptors have been linked to the transport of vitamin A to intestinal cells, where retinal dehydrogenase (RALDH) converts retinal into RA. Also, Clostridia directly modulates the RA concentration. Moreover, GM inhibits the activity of the cytochrome P450 (CYP) family of protein, which can degrade vitamin A. In addition, intestinal microbial enzymes promote the production of retinoic acid from b-carotene (Stacchiotti et al., 2021). In sheep, diet-induced metabolic disorders lead to the imbalance of GM reducing the production of bile acids and the absorption of vitamin A, a kind of fat-soluble vitamin. Consequently, it significantly increases the ratio of undifferentiated spermatogonia in the testis but decreases the number of mature sperms (Zhang et al., 2022).
Folic acid
Folic acid (vitamin B9) is necessary for DNA and RNA synthesis and methylation. It can affect chromatin structure by affecting histone methylation which is necessary for cell division. Folic acid in the diet improves semen quality and testicular tissue structure, especially if the animal is exposed to reproductive toxic substances. Folic acid helps germ cells to resist oxidative stress and inflammation to prevent DNA damage and apoptosis. Also, it protects the proliferation and differentiation of germ cells from the accumulation of oxidative substances (Rad et al., 2021). Methylenetetrahydrofolate reductase (MTHFR) is one of the key enzymes in folic acid metabolism, which participates in the biosynthesis of tetrahydro folic acid (THFA) and vitamin B12 and can re-methylate homocysteine to methionine, an essential amino acid. The low levels of these two vitamins lead to hyperhomocysteinemia, a disease related to the failure of in vitro fertilization (IVF) and decreased sperm density, vitality, and DNA integrity. The circulating homocysteine and degree of oxidative stress are positively correlated (Fowler, 2005). Human MTHFR gene polymorphisms 677CT and 1298AC can cause a 70% reduction in folate metabolism and hyperhomocysteinemia. MTHFR 677T allele is an important factor for male infertility in Asia. Folic acid treatment for 3 months can significantly alleviate the semen oxidative stress due to MTHFR 677TT gene carriers, and decrease malondialdehyde and sperm DNA breakage index, improving the natural pregnancy rate and live birth rate (Huang et al., 2020). Folic acid is obtained mainly from dietary supplements and bacterial synthesis. THFA is synthesized by intestinal bacteria from GTP, erythrose 4-phosphate, and phosphoenolpyruvate, which is directly absorbed through the proton-coupled folate transporter of colon cells and distributed through the circulatory system. Metagenomic analysis showed that Bacteroides fragilis and Prevotella copri of Bacteroidetes, Clostridium difficile, Lactobacillus plantarum, L. reuteri, L. delbrueckii ssp., bulgaricus and Streptococcus thermophilus of Firmicutes, part of Bifidobacterium spp. of Actinobacteria, Fusobacterium varium of Fusobacteria, and Salmonella enterica of Proteobacteria genera play a role in THFA synthesis (Yoshii et al., 2019). In GM, Lactobacillus and Bifidobacterium of GM are the main folic acid-producing and metabolizing bacteria (Wu et al., 2022). The production of folic acid can be detected in the culture system of human fecal microbiota in vitro. A study showed that oral Lactobacillus or Acidobacillus in cadmium-poisoned mice reduced testicular cadmium poisoning and promoted germ cell formation, which is a similar effect to folic acid supplementation (Kadry and Megeed, 2018).
Calcium
Calcium plays a decisive role in the fertilization process. It regulates sperm motility in mammals, which directly determines the occurrence of sperm-egg fusion. The process of sperm capacitation is dependent on the activation of the calcium ion channels on the sperm flagellum for sperm motility into the female reproductive tract (Vyklicka and Lishko, 2020). This chemotactic behavior determines acrosome reaction, including hyper activated motility (HAM) like progressive motility and flagellar asymmetric motility. For the acrosome reaction, the sperm needs a sustained increase in intracellular Ca2+ levels until F-actin is released from the plasma membrane. Ca2+ induces HAM by regulating F-actin, and its influx is mainly controlled by CatSper, which is a sperm-specific Ca2+ channel. Ca2+ influx can also produce cAMP through a cascade signal reaction promoting active protein kinase A (PKA) causing protein tyrosine phosphorylation. Ultimately, signal transduction in sperm is promoted. Calcium ions accumulate in the epididymis and prostate fluid against the concentration gradient, which is 2–3 times higher than that of the circulatory blood levels (Finkelstein et al., 2020). Blood calcium concentration is sustained by the dissolution of calcium salts via osteoclasts in bones. GM is the main regulator of mammalian bone mass, which regulates Ca2+ levels in the reproductive system by regulating the conversion between blood and bone calcium. In GM, Bifidobacteria and Lactobacillus affect the absorption of food calcium. The short-chain fatty acids (SCFAs) in the colon are the regulator of bone cell metabolism. GM produces SCFAs by decomposing dietary fiber. SCFAs reduce the formation of calcium phosphate and promote calcium absorption by lowering the intestine Ph (D’Amelio and Sassi, 2018). A study showed that the levels of IL-6, RANKL, and TNF-α in bone tissues decreased in germ-free mice lowering the number of osteoclasts than SPF mice. SCFAs increase calcium transport through regulations of signaling pathways. Additionally, SCFAs promote the synthesis of serotonin (5-HT), which interacts with bone cells via the activation of 5-HT1B receptors on pre-osteogenic cells to inhibit the proliferation of osteoblasts and reduce the formation of bone calcium. This ensures the blood calcium content (Sjogren et al., 2012). A study in GF (germ-free) mice showed an increase in bone mass, while the number of osteoclasts on the surface of bone decreased lowering the concentration of free Ca2+. Re-colonization of the GM in GF mice could normalize the bone mass (Ding K. et al., 2020). This modulating effect of GM on the calcium salt status either promotes or inhibits the survival and motility status of sperm.
Vitamin K
There are two sources of natural vitamin K, plant-derived phylloquinone (vitamin K1), and menaquinone (vitamin K2 or MK-n) produced by microorganisms. In mammals, GM synthesizes menaquinone and transports it through the circulatory system. Vitamin K1 must be converted into vitamin K2 to play important physiological functions such as blood coagulation, fibrinolysis, and bone homeostasis. A vitamin K-rich diet can improve the inflammatory resistance ability of the testes. It can also upregulate the cholesterol and steroid hormone synthase genes, such as Cyp11a, thereby increasing the concentration of serum testosterone. In the testicular inflammation rat model induced by LPS, inflammatory mediators such as Nuclear Factor kappa B (NF-κB) and pro-inflammatory factors reduced the transcriptional activity of steroidogenic factor 1 and cyclic AMP response element-binding protein that regulate Cyp11a. Consequently, the reduced expression of Cyp11a decreased inhibited the synthesis of testosterone in the testis. In all, vitamin K inhibited the activation of NF-κB, increased the expression of Cyp11a after LPS treatment, and reduced the inhibitory effect of inflammatory stimulation on testosterone synthesis (Takumi et al., 2011). Vitamin K, as a cofactor, helps γ-glutamyl carboxylase (GGCX) to carboxylate glutamic acid residues into γ-carboxyglutamic acid residues, which then activates vitamin K-dependent proteins. GGCX in testis may promote vitamin K-dependent γ-carboxylation of androgen receptor in Sertoli cell, which helps maintain the BTB structure, and facilitates the development of germ cells and sperm release (Shiba et al., 2021). In idiopathic non-obstructive azoospermia (iNOA) patients, vitamin K epoxide reductase complex subunit 1 (VKORC1), the substrate of vitamin K cycle metabolism, was found abnormally deleted in Leydig cells and extracellular matrix (Alfano et al., 2019). In addition, the relative proportion of vitamin K and D also significantly impact calcium metabolism affecting the development and motility of sperm (Khalil et al., 2021). Human or mice GM can add or reduce the side chain of dietary supplement vitamin K precursor to remodel to menaquinone 4, 10, 11, and 12 for further utilization (Ellis et al., 2021). GM like Bacteroides fragilis can produce vitamin K, mainly the menaquinones. MK4 promotes the genes related to testosterone synthesis. Also, MK7 works with Vitamin D to regulate the level of calcium (Stacchiotti et al., 2021).
Gut microbiota regulates the immune microenvironment of testis
Testes are immune privilege organs. Notably, the male haploid germ cells are not produced until the time of puberty, a long time after birth, which makes these new cells prone to the self-immune system (Qu et al., 2020). Therefore, these germ cells, which are self-antigens, are isolated from the environment to prevent attacks from the immune system. The seminiferous tubules are surrounded by a basement membrane, which is composed of supporting cells and intercellular connections in the blood-testis barrier, specialization of basal exoplasm, and muscle-like tubule cells. The seminiferous tubules create independent cavities, which block the attack from the immune system. Sertoli cells also phagocytose and digest apoptotic germ cells and their remnants to prevent autoimmunity. Androgens synthesized by interstitial cells, corticosterone secreted by testicular macrophages, and prostaglandins, activin, and 25-hydroxycholesterol present in the interstitium inhibit the function of macrophages in the testis. The secretion of corticosterone induces the differentiation of macrophages into immunosuppressive M2 type, promotes the secretion of anti-inflammatory cytokine IL-10, inhibits the expression of TNFα, IL-6, and other pro-inflammatory factors, and reduces the level of the immune response (Wang et al., 2017). The regulatory T lymphocytes (Treg cells) present in the testis upregulate the anti-inflammatory factors IL-10, IL-35, and TGF-β, creating an immunosuppressive microenvironment. A higher number of effector T cells over Treg cells in the testis disturbs the immune-suppressed environment and the autoimmune response is activated (Jacobo, 2018). Although GM promotes maintenance of the immune privileged microenvironment of testis in multiple ways, it can also break it in adverse situation (Figure 2).
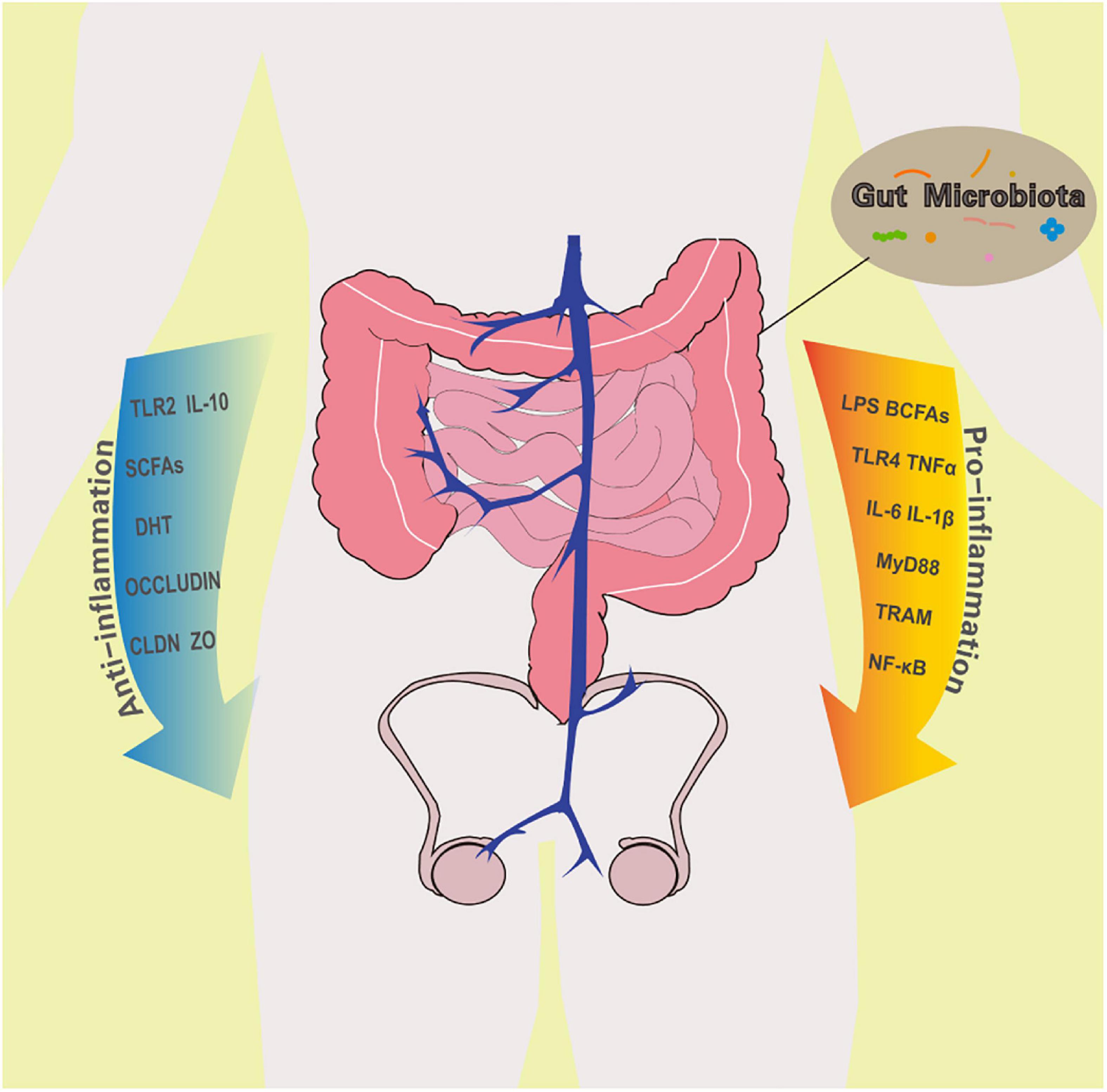
Figure 2. The effect of gut microbiota on testes immune privileged microenvironment. A healthy GM promotes anti-inflammatory cells and factors. However, when abnormal bacteria multiply in large numbers, they increase the concentration of pro-inflammatory molecules in the intestine and body fluids. Both positive and negative changes in GM affect the status of the testicular immune microenvironment. TLR2, Toll-like receptor 2; IL-10, Interleukin-10; SCFAs, short chain fatty acids; DHT, dihydrotestosterone; CLDN, claudins; ZO, Zona occludens; LPS, lipopolysaccharide; BCFAs, branched chain fatty acids; TLR4, Toll-like receptor 4; TNFα, tumor necrosis factor α; IL-6, Interleukin-6; IL-1β, Interleukin-1β; MyD88, myeloid differentiation factor 88; TRAM, translocation associated membrane protein; NF-κB: nuclear factor kappa B.
Gut microbiota and peripheral immunity
The crosstalk between GM and the peripheral immune system influences the balance of pro- and anti-inflammatory cells and maintains the tolerance of the immune exemption department in testis. Bacteroides Fragilis produces Polysaccharide A, activates TLR2 signal to induce the production of Foxp3 + Treg cells, promotes the secretion of anti-inflammatory factor IL-10, and inhibits the effect of pro-inflammatory Th17 cells. All this enhances the organ resistance against inflammation (Round and Mazmanian, 2010). SCFAs, an important product of gut microbes, especially butyrate, can facilitate peripheral naive CD4 + T cells of extrathymus to differentiate into Foxp3 + Treg cells (Arpaia et al., 2013). Also, butyric acid promotes the differentiation of M2 macrophages (Ji et al., 2016). SCFAs inhibit the NF-κB pathway by inhibiting lipopolysaccharide-induced macrophages to produce nitric oxide and pro-inflammatory cytokines TNFα, IL-1β, and IL-6 while promoting the secretion of IL-10 (Liu et al., 2012). Finally, the produced immune cells reach the testis via mesenteric the lymph system, hepatic portal vein and testicular artery, and affect the immune microenvironment of the testis. In the case of disturbed GM, the secretion of pro-inflammatory factors increases activating macrophages and dendritic cells in the testis. When these innate immune cells enter the epididymis, sperms are recognized as non-self substances attacked, affecting their survival and function (Zheng et al., 2021).
Gut microbiota and androgen
GM regulates the development of gonads through the gut-brain axis, promotes androgen synthesis, and protects the testicular immune tolerance. Androgens ensure the level of Treg cells, inhibit the proliferation of NK cells, and also protect the structure of BTB to prevent pathogenic substances (Kabbesh et al., 2021). A study showed that gut microbes have a strong ability to promote testosterone levels. In adult mice, the level of dihydrotestosterone (DHT) in feces is >20 times higher than that in serum (Collden et al., 2019). Furthermore, compared with sterile mice, the normal concentration of free DHT in the intestine of normal mice was higher. Also, the levels of testosterone, serum gonadotropins luteinizing hormone (LH), and follicle-stimulating hormone (FSH) were higher in the testes of normal mice or mice colonized with probiotics than those in sterile mice. In addition, genes controlling the testosterone production in GF mice such as Hsd3b1, Hsd17b11, Cyp11a1, and INSL3 were down regulated (Al-Asmakh et al., 2014). GM-produced LPS and pro-inflammatory factors degrade testicular IkB and promote the expression of upstream kinase IKK, which promotes nuclear translocation of NF-κB and inhibits transcription. Phosphorylated NF-κB inhibits the transcription of SF-1 and CREB in testis decreasing the expression of steroid producing gene Cyp11a and testosterone levels. This process can be reversed by increasing the colonization of GM synthesizing vitamin K (Takumi et al., 2011).
Gut microbiota and blood-testis barrier
A healthy GM improves the integrity of the BTB by upregulating intercellular connections and reducing permeability. The BTB is composed of Sertoli cells and adhesion junction (AJ) and tight junction (TJ) proteins between the cells, such as occludin, claudins (CLDN), JAM, Zona occludens (ZO-1, ZO-2, ZO-3) (Mruk and Cheng, 2015). GM promotes the development of Sertoli cells and their tight junctions, thereby ensuring the formation of seminiferous tubules and the safety of the microenvironment. In 15–16 days old prepubertal mice, SPF mice showed more complete seminiferous tubule development and lumen formation than GF mice. Due to underdeveloped Sertoli cells and low quantity, the lumen of the seminiferous tubules of GF mice was more atresia, showing no attachment of mature luminal co-germ cells. The expression of adhesion links and tight junction proteins, such as occludin, ZO-2, and E-cadherin, was also lower in GF mice. The re-colonization of probiotics in the intestines of GF mice improved the above situation. Due to the loss of Sertoli cells and their intercellular connections, the BTB of GF mice showed higher permeability than SPF mice. The Evans Blue (EB) perfusion test showed higher fluorescence intensity in the seminiferous tubules of GF mice, while after probiotics colonization, the fluorescence only appeared in the interstitium outside the seminiferous tubules (Al-Asmakh et al., 2014). The colonization with normal GM promotes the development of the BTB by improving the secretion of androgen. Testosterone binds to the testosterone receptor on Sertoli cells and promotes the expression of Claudin3 protein in Sertoli cells to increase the tightness of the BTB (Meng et al., 2005). Bacterial translocation induced by abnormal intestinal permeability leads to oxidative stress, activates testicular LPS/TLR4, and transfers NF-κB and mitogen-activated protein kinase to the nucleus through the MyD88 and TRAM pathways. This activates the innate immunity damaging testicular endothelium and the BTB (Wang and Xie, 2022).
Gut microbiota and testicular immune environment
The altered composition of GM can change gut permeability and immune status through its metabolites, endotoxins, and pro-inflammatory factors, thereby, affecting the immune environment of testis and damaging the reproductive system. A study showed that boars with highly abnormal sperm rates and lower semen utilization contained higher plasma endotoxin and pro-inflammatory factors such as TNF- α and IL-6, and lower anti-inflammatory factor such as IL-10 (Guo et al., 2020). Also, the concentration of fecal branch chain fatty acids (BCFAs), and the markers of proteolysis in the colon were significantly higher than that in boars with high-quality semen. Meanwhile, zonulin and diamineoxidase, which destroy the integrity of the intestine, were also higher in the plasma of boars with low-quality semen. Eventually, the study found that Sphingobium, a genus of bacteria that destroys the integrity of the intestine, was enriched in the GM of boars with low-quality semen. The abundance of Sphingobium had a strong positive correlation with plasma endotoxin. simultaneously, the abundance of gram-negative Proteobacteria in the intestine of boars also increased with low semen utilization. BCFAs, the product of abnormal protein breakdown by Proteobacteria, showed higher enrichment in the feces of boars with poor semen quality, which is an indicator of increased intestinal permeability. Proteobacteria use amino acids to produce BCFAs while other toxic metabolites are produced in the process. This suggests that higher levels of Sphingobium and Proteobacteria in the intestine may cause inflammatory responses decreasing semen quality. Increased intestinal permeability promotes LPS leakage into the blood, activates Toll-like receptors, and triggers the immune system to produce IL-6 and TNFα and other pro-inflammatory factors causing immune attacks on the testis (Vaarala et al., 2008; El-Baz et al., 2021). Eventually, the sperm cell membrane in such boars is damaged by lipid peroxidation, the vitality is reduced, and the damage to sperm DNA increases. Also, testosterone synthesis is reduced lowering reproductive ability.
Gut microbiota regulates testis by releasing signaling molecules
The growth, development, and functional regulation of the male reproductive system are also affected by various signaling molecules. For example, 5-hydroxytryptamine (5-HT, serotonin), γ-aminobutyric acid (GABA), and dopamine can regulate androgen levels and the process of sperm capacitation. Nitric oxide (NO), carbon monoxide (CO), hydrogen sulfide (H2S), and sulfur dioxide (SO2) are important signal molecules synthesized from arginine, glycine, and cysteine, respectively (Table 2). These activate guanylate cyclase to produce cGMP, which regulates vascular smooth muscle cell relaxation, hemodynamics, neurotransmission, and cell metabolism through cGMP-dependent protein kinases. H2S is also an important regulator of nerve function and endothelium-dependent relaxation, regulating membrane KATP channel stimulation and intracellular cAMP signal transmission. In addition, NH3 is the main product of amino acid catabolism in bacteria and profoundly affects the function of neurons and the vascular system through glutamine-dependent inhibition of NO synthesis (Li et al., 2009).
GABA and 5-HT
GM has been shown to produce various neurotransmitters, such as GABA, 5-HT, dopamine, and norepinephrine by metabolizing proteins and amino acids or by de novo synthesis (Dai et al., 2015). Experiments in mammals show that a large number of neurotransmitters molecules produced by GM play a role in maintaining and changing the physiological functions of animals (Strandwitz, 2018; Huang and Wu, 2021). The presence of 5-HT in the testis balances the production of androgens. In rat interstitial cells, 5-HT binds to 5-HT2 receptors to stimulate the secretion of corticotropin-releasing factor (CRF), which inhibits the synthesis of cAMP and gonadotropin-induced androgen (Tinajero et al., 1993). 5-HT (four times a day, 10 mg/kg) injected into the abdominal cavity of rats reduced the weight and volume of the testis, and lowered the concentration of inhibin and serum testosterone (Hedger et al., 1995). Also, 5-HT inhibits ejaculation and adjusts penile flaccidity and detumescence via the control of vascular resistance, blood pressure, hemostasis and platelet function. 5-HT binding to 5-HT2C and 5-HT1B receptors increases ejaculatory latency and delays orgasm, while 5-HT binding to 5-HT1A receptor decreases ejaculatory latency. The testis itself can produce endogenous 5-HT, while the rest is mainly used from the peripheral circulation (Berger et al., 2009). The gut is the main source of 5-HT; >90% of the total 5-HT is gut-derived, which is transported to the whole body through platelets. Enterochromaffin cells (ECs), mucosal mast cells, and myenteric neuron cells mainly synthesize 5-HT in the intestine. Studies have shown that nearly 10% of ECs synthesis peripheral 5-HT rely on GM. The concentration of serum 5-HT in adult GF mice decreased, and correspondingly, the concentration of 5-HT in the colon and feces decreased significantly. Spore-forming microbes (Sp) from the healthy mouse and human microbiota promote local and peripheral 5-HT concentration through its metabolites to promote the expression of tryptophan hydroxylase 1 (Tph1), an important gene for 5-HT synthesis in ECs (Yano et al., 2015). Cellular components of Clostridium ramosum have also been shown to stimulate host ECs to secrete 5-HT and modify the colonic stem cells to differentiate into lineages that secrete 5-HT (Mandic et al., 2019). Some bacteria in culture, including Corynebacterium spp., Streptococcus spp. and Escherichia coli, were reported to synthesize 5-HT (Yano et al., 2015). Damage GM induces local inflammation, which lowers the number of 5-HT transporters (Stasi et al., 2019). A study showed that male Brandt’s voles reared in high density stress environments exhibited a higher abundance of Streptococcus and E. coli in the intestine, which possibly increases the serum cortisol and 5-HT concentrations. Both of these increased the serum testosterone levels of Brandt’s voles via the hypothalamic-pituitary-gonadal axis making the animal more aggressive (Liu et al., 2020).
The GM genome metabolism model showed that Bacteroides, Parabacter and E. coli actively express GABA. Also, the isolation and culture of Lactobacillus and Bifidobacterium in the intestine could produce GABA. The GABA concentration is related to the process of sperm capacitation in the vagina. GABA promotes the tyrosine phosphorylation of sperm protein, which is an indicator of sperm capacitation. GABA also promotes the acrosome reaction, which is inhibited by selective GABA receptor antagonists (Kurata et al., 2019). A study in hamsters showed that GABA reduces the excessive activation of sperm by inhibiting the binding of 5-HT to 5-HT2 receptors, thereby co-regulating sperm activation with 5-HT (Fujinoki and Takei, 2017). GABA can also regulate the sexual behavior of male mammals. Treatment with Moxidectin, an anthelmintic drug, in rats lowered their libido and sexual behavior by reducing GABA secretion, which hindered penile erection (Rodrigues-Alves et al., 2008).
Nitric oxide and hydrogen sulfide
Arginine amino acid has nutritional effects on male reproductive function. Although bacteria in the small intestine can decompose arginine and affect the use of arginine by the reproductive system, some bacteria such as Lactobaillus spp., Bifidobacterium spp., Staphylococcus aureus, Bacillus spp. affect the NO production via arginine metabolism (Dai et al., 2015). NO synthesizing bacteria Bacillus and Paenibacillus were found in the GM of obese girls, and the NO synthesis was positively correlated with the level of FSH (Li Y. et al., 2021). Physiological levels of NO, a signaling molecule, also play an important role in the male reproductive system. In the brain, NO promotes the release of neurotransmitters to maintain libido and the secretion of luteinizing hormone-releasing hormone (LHRH) and GnRH to increase sex hormone levels. In testis, NO dilates blood vessels, allowing the testes to regulate local temperature. In the reproductive system, NO is released at the nerve endings of the cavernous body to activate guanosine cyclase. Activated guanylate cyclase produces cGMP to relax the vascular smooth muscle congesting the corpus cavernosum which leads to penis erection (Gratzke et al., 2010). H2S can also act as a physiological vasodilator, which directly affects erectile function. A study showed an increase in penis length after H2S injection into the penile cavernous body; the efficiency of penis lengthening was similar to the effect of 20 μg prostaglandin E1 (D’Emmanuele di Villa Bianca et al., 2011). Intestinal sulfate-reducing bacteria (SRB) such as Desulfovibrio spp., can use H2, lactic acid, and acetate as electron donors, and sulfate or sulfite as electron acceptors to produce H2S. There are also some bacteria in large intestines, such as E. coli, Salmonella enterica, Clostridium spp., and Enterobacter aerogenes, that can metabolize sulfur-containing amino acids to produce H2S (Gibson et al., 1993; Ran et al., 2019).
Sex hormone
The type and abundance of gut microbes can affect the level of sex hormones in animals. Bacterial overgrowth in the small intestine may trigger an increase in intestinal permeability and systemic circulation, and a decrease in serum testosterone, which impairs testicular function (Tremellen and Pearce, 2020). This effect may be achieved by interfering with the steroid cycle metabolism and affecting the hormone-HPG axis. The level of sex steroid hormones is related to the composition and diversity of gut microbes. Individuals with more diverse gut microbes have higher levels of sex steroids (Shin et al., 2019). Estrogens (such as estradiol), progesterone, and their receptor exist in male sexual glands maintain male fertility. In women, estrogen production requires GM-secreted β-glucuronidase to covert conjugated estrogens to deconjugated forms. The increase in the abundance of β-glucuronidase-producing bacteria can promote in the level of circulating estrogen. A study showed that the α diversity of GM negatively correlates with the concentration of estradiol and positively correlates with the proportion of estrogen metabolites in the urine of women, however, the same needs to be verified in men and male animals (Qi et al., 2021). As mentioned earlier, GM can alter the expression of steroid-producing genes HSD3β1, Cyp11a, etc., which changes the levels of sex hormones (Takumi et al., 2011; Ding N. et al., 2020). Compared with SPF male mice, GF male mice had lower serum levels of testosterone (T), LH, and FSH, however, colonizing their intestine with probiotics significantly increased the serum levels of these hormones (Al-Asmakh et al., 2014). A study in the O-PLS mice model showed that testosterone levels were positively associated with Prevotellaceae, Cytophagaceae, Fibrobacteriaceae, Sphingobacteriaceae, and Idiomarinaceae, and negatively associated with Actinobacteria, Proteobacteria, Firmicutes and Verrucomicrobia phylum (Markle et al., 2013). In adolescent males, the level of testosterone was found to be associated with Adlercreutzia, Ruminococcus, Dorea, Clostridium and Parabacteroides genus (Yuan et al., 2020). Besides, a part of GM converts androgen precursors into active androgens (Pernigoni et al., 2021). GM promotes the deglucuronidation of testosterone and DHT, and increases the levels of free testosterone and DHT, which contribute to the development of secondary sexual characteristics in male animals. GC-MS (Gas chromatography-tandem mass spectrometry) analysis revealed that the intestinal levels of free testosterone and DHT were higher in segments with a high microbial density such as the cecum and colon than in a low microbial density segment such as the proximal small intestine. The free DHT level in feces is >70 times higher than in serum. Compared to normal mice, the concentration of free DHT is much lower in the distal intestine of GF mice, which contained a lot of glucuronidated T and DHT (Collden et al., 2019). Transplantation of fecal microbes from high-fat diet mice were into the intestine of normal mice increased the intestinal abundance of Bacteroidaceae and Prevotellaceae in the transplanted mice decreasing the expression of the Hsd3β1 gene encoding DHT synthase in testis (Ding N. et al., 2020).
Conclusion and perspectives
GM metabolizes nutrients in animals regulating their immune state. GM has great research value for its effect on far distal organs. Experimental and clinical evidence from different species indicate that the main ways through which microbiota affects the development and function of the reproductive system include: providing nutrients like SCFAs, vitamins, and minerals to transform the function and gene expression status of the reproductive system, regulating the testicular immune microenvironment, controlling physiological processes through signal transduction, and affecting hormone levels (Dai et al., 2015; Li et al., 2017; Li X. et al., 2021; Zhang et al., 2022). The metabolic processes of GM provide crucial nutrients such as vitamins and minerals to the reproductive system; and regulate the development and functions of testes to maintain their immune privileged state. GF animals, which had no microbial abundance in the gut, exhibited decreased testosterone levels and abnormal BTB structure than the normal ones (Al-Asmakh et al., 2014). An altered GM negatively affects the function of the testis under on various stresses or the influence of toxic substances (Liu et al., 2022). Instead of providing nutritional molecules and support to the reproductive system, the abnormal microbiota produces pro-inflammatory factors and creates an oxidative environment that disrupts the spermatogenic process in the testis (Tian et al., 2019; Ding N. et al., 2020; Zhao et al., 2020). The effect of GM on distal organs is a fascinating prospect that requires more research. It may also provide a new promising way to regulate reproduction. Improving dietary structure, recolonizing healthy fecal microbes, and supplementing health products like probiotics have been shown to alleviate infertility in men and male animals, which further proves that altering the composition of GM can regulate the physiological functions of the testis, or even reverse the alterations to the aging effect on reproductive system (Poutahidis et al., 2014; Xie et al., 2019). Studies have shown that the decreased number of germ cells and low-quality semen in high-fat diet male animals are largely induced by GM disturbances which cause an accumulation of harmful metabolites such as sphingosine. Remodeling their GM by feeding melatonin or transplanting alginate oligosaccharides-improved fecal microbiota effectively alleviates the above conditions (Hao et al., 2022a; Sun et al., 2022). Zhang C. et al. (2021), Zhang P. et al. (2021), and Hao et al. (2022b) also found that transplantation of fecal microbiota from mice supplemented with alginate oligosaccharide to mice treated with busulfan or streptozotocin (a type 1 diabetes inducer) could rescue germ cell loss and improve semen quality through metabolic pathways. Although GM metabolites have an impact on fertility, basic phenomena yet remain to be defined completely. The physiological changes and specific consequences of this phenomenon are difficult to quantify, track and locate in real-time. For now, it is unknown how many metabolites from the circulatory system pass the BTB directly affect the male reproductive system. Existing research trends indicate that using multi-omics technology can delineate the interactions between GM and the host organs/tissues (Tilocca et al., 2020). With the establishment of gene expression profiles and metabolomics, researchers can now locate the transverse spatial organization and longitudinal phase states of GM (Tropini et al., 2017; Mars et al., 2020). The intricate networks between GM as well as the breaking and rebuilding of microbial balance are other research challenges. In the following research, scholars need to pay attention to the effect of partial and/or the entire function of the GM on toward the reproductive capacity in males and design a series of microbial complex agents to promote or inhibit fertility without affecting normal health (Alfano et al., 2018). Research targeting the treatment and development of GM will generate more emphasis in the near future to improve the health status of humans and animals.
Author contributions
HC wrote the manuscript. SP investigated and supervised the manuscript. XC and DQ designed the tables and figures and edited the manuscript. JH, YDL, and YL edited the manuscript. All authors read and approved the final version of the manuscript.
Funding
This work was supported by the National Natural Sciences Foundation of China (32072815), the Technology Innovation Leading Program of Shaanxi Province (2020QFY10), the Program of Shaanxi Province Science and Technology Innovation Team (2019TD-036), and the Fundamental Research Funds for the Central Universities (2452020157).
Conflict of interest
The authors declare that the research was conducted in the absence of any commercial or financial relationships that could be construed as a potential conflict of interest.
Publisher’s note
All claims expressed in this article are solely those of the authors and do not necessarily represent those of their affiliated organizations, or those of the publisher, the editors and the reviewers. Any product that may be evaluated in this article, or claim that may be made by its manufacturer, is not guaranteed or endorsed by the publisher.
References
Adak, A., and Khan, M. R. (2019). An insight into gut microbiota and its functionalities. Cell. Mol. Life Sci. 76, 473–493. doi: 10.1007/s00018-018-2943-4
Al-Asmakh, M., Stukenborg, J. B., Reda, A., Anuar, F., Strand, M. L., Hedin, L., et al. (2014). The gut microbiota and developmental programming of the testis in mice. PLoS One 9:e103809. doi: 10.1371/journal.pone.0103809
Alfano, M., Ferrarese, R., Locatelli, I., Ventimiglia, E., Ippolito, S., Gallina, P., et al. (2018). Testicular microbiome in azoospermic men-first evidence of the impact of an altered microenvironment. Hum. Reprod. 33, 1212–1217. doi: 10.1093/humrep/dey116
Alfano, M., Pederzoli, F., Locatelli, I., Ippolito, S., Longhi, E., Zerbi, P., et al. (2019). Impaired testicular signaling of vitamin A and vitamin K contributes to the aberrant composition of the extracellular matrix in idiopathic germ cell aplasia. Fertil. Steril. 111, 687–698. doi: 10.1016/j.fertnstert.2018.12.002
Altmae, S., Franasiak, J. M., and Mandar, R. (2019). The seminal microbiome in health and disease. Nat. Rev. Urol. 16, 703–721. doi: 10.1038/s41585-019-0250-y
Arpaia, N., Campbell, C., Fan, X., Dikiy, S., van der Veeken, J., deRoos, P., et al. (2013). Metabolites produced by commensal bacteria promote peripheral regulatory T-cell generation. Nature 504, 451–455. doi: 10.1038/nature12726
Belloc, S., Cohen-Bacrie, M., Amar, E., Izard, V., Benkhalifa, M., Dalleac, A., et al. (2014). High body mass index has a deleterious effect on semen parameters except morphology: Results from a large cohort study. Fertil. Steril. 102, 1268–1273. doi: 10.1016/j.fertnstert.2014.07.1212
Berger, M., Gray, J. A., and Roth, B. L. (2009). The expanded biology of serotonin. Annu. Rev. Med. 60, 355–366. doi: 10.1146/annurev.med.60.042307.110802
Clagett-Dame, M., and Knutson, D. (2011). Vitamin A in reproduction and development. Nutrients 3, 385–428. doi: 10.3390/nu3040385
Collden, H., Landin, A., Wallenius, V., Elebring, E., Fandriks, L., Nilsson, M. E., et al. (2019). The gut microbiota is a major regulator of androgen metabolism in intestinal contents. Am. J. Physiol. Endocrinol. Metab. 317, E1182–E1192. doi: 10.1152/ajpendo.00338.2019
Dai, Z., Wu, Z., Hang, S., Zhu, W., and Wu, G. (2015). Amino acid metabolism in intestinal bacteria and its potential implications for mammalian reproduction. Mol. Hum. Reprod. 21, 389–409. doi: 10.1093/molehr/gav003
D’Amelio, P., and Sassi, F. (2018). Gut microbiota, Immune system, and bone. Calcif. Tissue Int. 102, 415–425. doi: 10.1007/s00223-017-0331-y
Dance, A., Thundathil, J., Wilde, R., Blondin, P., and Kastelic, J. (2015). Enhanced early-life nutrition promotes hormone production and reproductive development in Holstein bulls. J. Dairy Sci. 98, 987–998. doi: 10.3168/jds.2014-8564
D’Emmanuele di Villa Bianca, R., Sorrentino, R., Mirone, V., and Cirino, G. (2011). Hydrogen sulfide and erectile function: A novel therapeutic target. Nat. Rev. Urol. 8, 286–289. doi: 10.1038/nrurol.2011.45
Ding, K., Hua, F., and Ding, W. (2020). Gut microbiome and osteoporosis. Aging Dis. 11, 438–447. doi: 10.14336/AD.2019.0523
Ding, N., Zhang, X., Zhang, X. D., Jing, J., Liu, S. S., Mu, Y. P., et al. (2020). Impairment of spermatogenesis and sperm motility by the high-fat diet-induced dysbiosis of gut microbes. Gut 69, 1608–1619. doi: 10.1136/gutjnl-2019-319127
Eckburg, P. B., Bik, E. M., Bernstein, C. N., Purdom, E., Dethlefsen, L., Sargent, M., et al. (2005). Diversity of the human intestinal microbial flora. Science 308, 1635–1638. doi: 10.1126/science.1110591
El-Baz, A. M., Shata, A., Hassan, H. M., El-Sokkary, M. M. A., and Khodir, A. E. (2021). The therapeutic role of lactobacillus and montelukast in combination with metformin in diabetes mellitus complications through modulation of gut microbiota and suppression of oxidative stress. Int. Immunopharmacol. 96:107757. doi: 10.1016/j.intimp.2021.107757
Ellis, J. L., Karl, J. P., Oliverio, A. M., Fu, X., Soares, J. W., Wolfe, B. E., et al. (2021). Dietary vitamin K is remodeled by gut microbiota and influences community composition. Gut Microbes 13, 1–16. doi: 10.1080/19490976.2021.1887721
Finkelstein, M., Etkovitz, N., and Breitbart, H. (2020). Ca(2+) signaling in mammalian spermatozoa. Mol. Cell. Endocrinol. 516:110953. doi: 10.1016/j.mce.2020.110953
Fowler, B. (2005). Homocysteine: Overview of biochemistry, molecular biology, and role in disease processes. Semin. Vasc. Med. 5, 77–86. doi: 10.1055/s-2005-872394
Fujinoki, M., and Takei, G. L. (2017). gamma-Aminobutyric acid suppresses enhancement of hamster sperm hyperactivation by 5-hydroxytryptamine. J. Reprod. Dev. 63, 67–74. doi: 10.1262/jrd.2016-091
Gibson, G. R., Macfarlane, G. T., and Cummings, J. H. (1993). Sulphate reducing bacteria and hydrogen metabolism in the human large intestine. Gut 34, 437–439. doi: 10.1136/gut.34.4.437
Godia, M., Ramayo-Caldas, Y., Zingaretti, L. M., Darwich, L., Lopez, S., Rodriguez-Gil, J. E., et al. (2020). A pilot RNA-seq study in 40 pietrain ejaculates to characterize the porcine sperm microbiome. Theriogenology 157, 525–533. doi: 10.1016/j.theriogenology.2020.08.001
Gratzke, C., Angulo, J., Chitaley, K., Dai, Y. T., Kim, N. N., Paick, J. S., et al. (2010). Anatomy, physiology, and pathophysiology of erectile dysfunction. J. Sex. Med. 7, 445–475. doi: 10.1111/j.1743-6109.2009.01624.x
Guo, L., Wu, Y., Wang, C., Wei, H., Tan, J., Sun, H., et al. (2020). Gut microbiological disorders reduce semen utilization rate in duroc boars. Front. Microbiol. 11:581926. doi: 10.3389/fmicb.2020.581926
Hao, Y., Feng, Y., Yan, X., Chen, L., Ma, X., Tang, X., et al. (2022a). Gut microbiota-testis axis: FMT mitigates high-fat diet-diminished male fertility via improving systemic and testicular metabolome. Microbiol. Spectr. 10:e0002822. doi: 10.1128/spectrum.00028-22
Hao, Y., Feng, Y., Yan, X., Chen, L., Zhong, R., Tang, X., et al. (2022b). Gut microbiota-testis axis: FMT improves systemic and testicular micro-environment to increase semen quality in type 1 diabetes. Mol. Med. 28:45. doi: 10.1186/s10020-022-00473-w
Hedger, M. P., Khatab, S., Gonzales, G., and de Kretser, D. M. (1995). Acute and short-term actions of serotonin administration on the pituitary-testicular axis in the adult rat. Reprod. Fertil. Dev. 7, 1101–1109. doi: 10.1071/RD9951101
Huang, F., and Wu, X. (2021). Brain neurotransmitter modulation by gut microbiota in anxiety and depression. Front. Cell. Dev. Biol. 9:649103. doi: 10.3389/fcell.2021.649103
Huang, W. J., Lu, X. L., Li, J. T., and Zhang, J. M. (2020). Effects of folic acid on oligozoospermia with MTHFR polymorphisms in term of seminal parameters, DNA fragmentation, and live birth rate: A double-blind, randomized, placebo-controlled trial. Andrology 8, 110–116. doi: 10.1111/andr.12652
Jacobo, P. (2018). The role of regulatory T Cells in autoimmune orchitis. Andrologia 50:e13092. doi: 10.1111/and.13092
Jensen, C. F. S., Ostergren, P., Dupree, J. M., Ohl, D. A., Sonksen, J., and Fode, M. (2017). Varicocele and male infertility. Nat. Rev. Urol. 14, 523–533. doi: 10.1038/nrurol.2017.98
Ji, J., Shu, D., Zheng, M., Wang, J., Luo, C., Wang, Y., et al. (2016). Microbial metabolite butyrate facilitates M2 macrophage polarization and function. Sci. Rep. 6:24838. doi: 10.1038/srep24838
Kabbesh, H., Riaz, M. A., Jensen, A. D., Scheiner-Bobis, G., and Konrad, L. (2021). Long-term maintenance of viable adult rat sertoli cells able to establish testis barrier components and function in response to androgens. Cells 10:2405. doi: 10.3390/cells10092405
Kadry, M. O., and Megeed, R. A. (2018). Probiotics as a complementary therapy in the model of cadmium chloride toxicity: Crosstalk of beta-catenin, BDNF, and StAR signaling pathways. Biol. Trace Elem. Res. 185, 404–413. doi: 10.1007/s12011-018-1261-x
Khalil, Z., Alam, B., Akbari, A. R., and Sharma, H. (2021). The medical benefits of vitamin K2 on calcium-related disorders. Nutrients 13:691. doi: 10.3390/nu13020691
Kurata, S., Hiradate, Y., Umezu, K., Hara, K., and Tanemura, K. (2019). Capacitation of mouse sperm is modulated by gamma-aminobutyric acid (GABA) concentration. J. Reprod. Dev. 65, 327–334. doi: 10.1262/jrd.2019-008
Lam, S., Bai, X., Shkoporov, A. N., Park, H., Wu, X., Lan, P., et al. (2022). Roles of the gut virome and mycobiome in faecal microbiota transplantation. Lancet Gastroenterol. Hepatol. 7, 472–484. doi: 10.1016/S2468-1253(21)00303-4
Li, H., Qi, T., Huang, Z. S., Ying, Y., Zhang, Y., Wang, B., et al. (2017). Relationship between gut microbiota and type 2 diabetic erectile dysfunction in Sprague-Dawley rats. J. Huazhong Univ. Sci. Technol. Med. Sci. 37, 523–530. doi: 10.1007/s11596-017-1767-z
Li, X., Bazer, F. W., Gao, H., Jobgen, W., Johnson, G. A., Li, P., et al. (2009). Amino acids and gaseous signaling. Amino Acids 37, 65–78. doi: 10.1007/s00726-009-0264-5
Li, X., Cheng, W., Shang, H., Wei, H., and Deng, C. (2021). The interplay between androgen and gut microbiota: Is there a microbiota-gut-testis axis. Reprod. Sci. 29, 1674–1684. doi: 10.1007/s43032-021-00624-0
Li, Y., Shen, L., Huang, C., Li, X., Chen, J., Li, S. C., et al. (2021). Altered nitric oxide induced by gut microbiota reveals the connection between central precocious puberty and obesity. Clin. Transl. Med. 11:e299. doi: 10.1002/ctm2.299
Liu, J. B., Chen, K., Li, Z. F., Wang, Z. Y., and Wang, L. (2022). Glyphosate-induced gut microbiota dysbiosis facilitates male reproductive toxicity in rats. Sci. Total Environ. 805:150368. doi: 10.1016/j.scitotenv.2021.150368
Liu, J., Huang, S., Li, G., Zhao, J., Lu, W., and Zhang, Z. (2020). High housing density increases stress hormone- or disease-associated fecal microbiota in male Brandt’s voles (Lasiopodomys brandtii). Horm. Behav. 126:104838. doi: 10.1016/j.yhbeh.2020.104838
Liu, T., Li, J., Liu, Y., Xiao, N., Suo, H., Xie, K., et al. (2012). Short-chain fatty acids suppress lipopolysaccharide-induced production of nitric oxide and proinflammatory cytokines through inhibition of NF-kappaB pathway in RAW264.7 cells. Inflammation 35, 1676–1684. doi: 10.1007/s10753-012-9484-z
Mandic, A. D., Woting, A., Jaenicke, T., Sander, A., Sabrowski, W., Rolle-Kampcyk, U., et al. (2019). Clostridium ramosum regulates enterochromaffin cell development and serotonin release. Sci. Rep. 9:1177. doi: 10.1038/s41598-018-38018-z
Markle, J. G., Frank, D. N., Mortin-Toth, S., Robertson, C. E., Feazel, L. M., Rolle-Kampczyk, U., et al. (2013). Sex differences in the gut microbiome drive hormone-dependent regulation of autoimmunity. Science 339, 1084–1088. doi: 10.1126/science.1233521
Mars, R. A. T., Yang, Y., Ward, T., Houtti, M., Priya, S., Lekatz, H. R., et al. (2020). Longitudinal Multi-omics reveals subset-specific mechanisms underlying irritable bowel syndrome. Cell 182, 1460–1473.e17. doi: 10.1016/j.cell.2020.08.007
Martinot, E., Thirouard, L., Holota, H., Monrose, M., Garcia, M., Beaudoin, C., et al. (2021). Intestinal microbiota defines the GUT-TESTIS axis. Gut 71, 844–845. doi: 10.1136/gutjnl-2021-324690
Meng, J., Holdcraft, R. W., Shima, J. E., Griswold, M. D., and Braun, R. E. (2005). Androgens regulate the permeability of the blood-testis barrier. Proc. Natl. Acad. Sci. U.S.A. 102, 16696–16700. doi: 10.1073/pnas.0506084102
Morrison, D. J., and Preston, T. (2016). Formation of short chain fatty acids by the gut microbiota and their impact on human metabolism. Gut Microbes 7, 189–200. doi: 10.1080/19490976.2015.1134082
Mruk, D. D., and Cheng, C. Y. (2015). The mammalian blood-testis barrier: Its biology and regulation. Endocr. Rev. 36, 564–591. doi: 10.1210/er.2014-1101
Nicholson, J. K., Holmes, E., Kinross, J., Burcelin, R., Gibson, G., Jia, W., et al. (2012). Host-gut microbiota metabolic interactions. Science 336, 1262–1267. doi: 10.1126/science.1223813
Pernigoni, N., Zagato, E., Calcinotto, A., Troiani, M., Mestre, R. P., Cali, B., et al. (2021). Commensal bacteria promote endocrine resistance in prostate cancer through androgen biosynthesis. Science 374, 216–224. doi: 10.1126/science.abf8403
Poutahidis, T., Springer, A., Levkovich, T., Qi, P., Varian, B. J., Lakritz, J. R., et al. (2014). Probiotic microbes sustain youthful serum testosterone levels and testicular size in aging mice. PLoS One 9:e84877. doi: 10.1371/journal.pone.0084877
Qi, X., Yun, C., Pang, Y., and Qiao, J. (2021). The impact of the gut microbiota on the reproductive and metabolic endocrine system. Gut Microbes 13, 1–21. doi: 10.1080/19490976.2021.1894070
Qin, D. Z., Cai, H., He, C., Yang, D. H., Sun, J., He, W. L., et al. (2021). Melatonin relieves heat-induced spermatocyte apoptosis in mouse testes by inhibition of ATF6 and PERK signaling pathways. Zool. Res. 42, 514–524. doi: 10.24272/j.issn.2095-8137.2021.041
Qin, J., Li, R., Raes, J., Arumugam, M., Burgdorf, K. S., Manichanh, C., et al. (2010). A human gut microbial gene catalogue established by metagenomic sequencing. Nature 464, 59–65. doi: 10.1038/nature08821
Qu, N., Ogawa, Y., Kuramasu, M., Nagahori, K., Sakabe, K., and Itoh, M. (2020). Immunological microenvironment in the testis. Reprod. Med. Biol. 19, 24–31. doi: 10.1002/rmb2.12293
Rad, I., Saberi, A., Koochakzadeh-Nematollahi, N. S., Habibzadeh, V., Salarkia, E., Amanollahi, S., et al. (2021). The effects of folic acid on testicular histology, sperm quality, and spermatogenesis indices following 3,4-methylenedioxymethamphetamine exposure in adult male rats. Addict. Health 13, 36–44.
Ran, S., Mu, C., and Zhu, W. (2019). Diversity and community pattern of sulfate-reducing bacteria in piglet gut. J. Anim. Sci. Biotechnol. 10:40. doi: 10.1186/s40104-019-0346-5
Rodrigues-Alves, P. S., Lebrun, I., Florio, J. C., Bernardi, M. M., and Spinosa Hde, S. (2008). Moxidectin interference on sexual behavior, penile erection and hypothalamic GABA levels of male rats. Res. Vet. Sci. 84, 100–106. doi: 10.1016/j.rvsc.2007.04.003
Round, J. L., and Mazmanian, S. K. (2010). Inducible Foxp3+ regulatory T-cell development by a commensal bacterium of the intestinal microbiota. Proc. Natl. Acad. Sci. U.S.A. 107, 12204–12209. doi: 10.1073/pnas.0909122107
Schleif, M. C., Havel, S. L., and Griswold, M. D. (2022). Function of Retinoic acid in development of male and female gametes. Nutrients 14:1293. doi: 10.3390/nu14061293
Schupack, D. A., Mars, R. A. T., Voelker, D. H., Abeykoon, J. P., and Kashyap, P. C. (2022). The promise of the gut microbiome as part of individualized treatment strategies. Nat. Rev. Gastroenterol. Hepatol. 19, 7–25. doi: 10.1038/s41575-021-00499-1
Shiba, S., Ikeda, K., Horie-Inoue, K., Azuma, K., Hasegawa, T., Amizuka, N., et al. (2021). Vitamin K-dependent gamma-glutamyl carboxylase in sertoli cells is essential for male fertility in mice. Mol. Cell. Biol. 41, e404–e420. doi: 10.1128/MCB.00404-20
Shin, J. H., Park, Y. H., Sim, M., Kim, S. A., Joung, H., and Shin, D. M. (2019). Serum level of sex steroid hormone is associated with diversity and profiles of human gut microbiome. Res. Microbiol. 170, 192–201. doi: 10.1016/j.resmic.2019.03.003
Sjogren, K., Engdahl, C., Henning, P., Lerner, U. H., Tremaroli, V., Lagerquist, M. K., et al. (2012). The gut microbiota regulates bone mass in mice. J. Bone Miner. Res. 27, 1357–1367. doi: 10.1002/jbmr.1588
Stacchiotti, V., Rezzi, S., Eggersdorfer, M., and Galli, F. (2021). Metabolic and functional interplay between gut microbiota and fat-soluble vitamins. Crit. Rev. Food Sci. Nutr. 61, 3211–3232. doi: 10.1080/10408398.2020.1793728
Stasi, C., Sadalla, S., and Milani, S. (2019). The relationship between the serotonin metabolism, gut-microbiota and the gut-brain axis. Curr. Drug Metab. 20, 646–655. doi: 10.2174/1389200220666190725115503
Strandwitz, P. (2018). Neurotransmitter modulation by the gut microbiota. Brain Res. 1693, 128–133. doi: 10.1016/j.brainres.2018.03.015
Strandwitz, P., Kim, K. H., Terekhova, D., Liu, J. K., Sharma, A., Levering, J., et al. (2019). GABA-modulating bacteria of the human gut microbiota. Nat. Microbiol. 4, 396–403. doi: 10.1038/s41564-018-0307-3
Sun, Y., Sun, P., Hu, Y., Shan, L., Geng, Q., Gong, Y., et al. (2022). Elevated testicular apoptosis is associated with elevated sphingosine driven by gut microbiota in prediabetic sheep. BMC Biol. 20:121. doi: 10.1186/s12915-022-01326-y
Takumi, N., Shirakawa, H., Ohsaki, Y., Ito, A., Watanabe, T., Giriwono, P. E., et al. (2011). Dietary vitamin K alleviates the reduction in testosterone production induced by lipopolysaccharide administration in rat testis. Food Funct. 2, 406–411. doi: 10.1039/c1fo10058k
Tian, X., Yu, Z., Feng, P., Ye, Z., Li, R., Liu, J., et al. (2019). Lactobacillus plantarum TW1-1 alleviates diethylhexylphthalate-induced testicular damage in mice by modulating gut microbiota and decreasing inflammation. Front. Cell. Infect. Microbiol. 9:221. doi: 10.3389/fcimb.2019.00221
Tilocca, B., Pieroni, L., Soggiu, A., Britti, D., Bonizzi, L., Roncada, P., et al. (2020). Gut-brain axis and neurodegeneration: State-of-the-art of meta-omics sciences for microbiota characterization. Int. J. Mol. Sci. 21:4045. doi: 10.3390/ijms21114045
Tinajero, J. C., Fabbri, A., Ciocca, D. R., and Dufau, M. L. (1993). Serotonin secretion from rat Leydig cells. Endocrinology 133, 3026–3029. doi: 10.1210/endo.133.6.8243331
Tremellen, K., and Pearce, K. (2020). Small intestinal bacterial overgrowth (SIBO) as a potential cause of impaired spermatogenesis. Gut 69, 2058–2059. doi: 10.1136/gutjnl-2020-320766
Tropini, C., Earle, K. A., Huang, K. C., and Sonnenburg, J. L. (2017). The gut microbiome: Connecting spatial organization to function. Cell Host Microbe. 21, 433–442. doi: 10.1016/j.chom.2017.03.010
Ulluwishewa, D., Anderson, R. C., McNabb, W. C., Moughan, P. J., Wells, J. M., and Roy, N. C. (2011). Regulation of tight junction permeability by intestinal bacteria and dietary components. J. Nutr. 141, 769–776. doi: 10.3945/jn.110.135657
Vaarala, O., Atkinson, M. A., and Neu, J. (2008). The “perfect storm” for type 1 diabetes: The complex interplay between intestinal microbiota, gut permeability, and mucosal immunity. Diabetes 57, 2555–2562. doi: 10.2337/db08-0331
Vyklicka, L., and Lishko, P. V. (2020). Dissecting the signaling pathways involved in the function of sperm flagellum. Curr. Opin. Cell. Biol. 63, 154–161. doi: 10.1016/j.ceb.2020.01.015
Walter, J., and Ley, R. (2011). The human gut microbiome: Ecology and recent evolutionary changes. Annu. Rev. Microbiol. 65, 411–429. doi: 10.1146/annurev-micro-090110-102830
Wang, M., Fijak, M., Hossain, H., Markmann, M., Nusing, R. M., Lochnit, G., et al. (2017). Characterization of the micro-environment of the testis that shapes the phenotype and function of testicular macrophages. J. Immunol. 198, 4327–4340. doi: 10.4049/jimmunol.1700162
Wang, Y., and Xie, Z. (2022). Exploring the role of gut microbiome in male reproduction. Andrology 10, 441–450. doi: 10.1111/andr.13143
Wu, J., Zhao, Y., Wang, X., Kong, L., Johnston, L. J., Lu, L., et al. (2022). Dietary nutrients shape gut microbes and intestinal mucosa via epigenetic modifications. Crit. Rev. Food Sci. Nutr. 62, 783–797. doi: 10.1080/10408398.2020.1828813
Wu, X., Lin, D., Sun, F., and Cheng, C. Y. (2021). Male infertility in humans: An Update on Non-Obstructive Azoospermia (NOA) and Obstructive Azoospermia (OA). Adv. Exp. Med. Biol. 1288, 161–173. doi: 10.1007/978-3-030-77779-1_8
Xie, C., Bian, Y., Feng, H., Zhao, Y., Wang, L., Li, Y., et al. (2019). Reversal of ciprofloxacin-induced testosterone reduction by probiotic microbes in mouse testes. Gen. Comp. Endocrinol. 284:113268. doi: 10.1016/j.ygcen.2019.113268
Yano, J. M., Yu, K., Donaldson, G. P., Shastri, G. G., Ann, P., Ma, L., et al. (2015). Indigenous bacteria from the gut microbiota regulate host serotonin biosynthesis. Cell 161, 264–276. doi: 10.1016/j.cell.2015.02.047
Yatsunenko, T., Rey, F. E., Manary, M. J., Trehan, I., Dominguez-Bello, M. G., Contreras, M., et al. (2012). Human gut microbiome viewed across age and geography. Nature 486, 222–227. doi: 10.1038/nature11053
Yoshii, K., Hosomi, K., Sawane, K., and Kunisawa, J. (2019). Metabolism of Dietary and microbial Vitamin B family in the regulation of host immunity. Front. Nutr. 6:48. doi: 10.3389/fnut.2019.00048
Yuan, X., Chen, R., Zhang, Y., Lin, X., and Yang, X. (2020). Gut microbiota: Effect of pubertal status. BMC Microbiol. 20:334. doi: 10.1186/s12866-020-02021-0
Zhang, C., Xiong, B., Chen, L., Ge, W., Yin, S., Feng, Y., et al. (2021). Rescue of male fertility following faecal microbiota transplantation from alginate oligosaccharide-dosed mice. Gut 70, 2213–2215. doi: 10.1136/gutjnl-2020-323593
Zhang, P., Feng, Y., Li, L., Ge, W., Yu, S., Hao, Y., et al. (2021). Improvement in sperm quality and spermatogenesis following faecal microbiota transplantation from alginate oligosaccharide dosed mice. Gut 70, 222–225. doi: 10.1136/gutjnl-2020-320992
Zhang, T., Sun, P., Geng, Q., Fan, H., Gong, Y., Hu, Y., et al. (2022). Disrupted spermatogenesis in a metabolic syndrome model: The role of vitamin A metabolism in the gut-testis axis. Gut 71, 78–87. doi: 10.1136/gutjnl-2020-323347
Zhao, T. X., Wei, Y. X., Wang, J. K., Han, L. D., Sun, M., Wu, Y. H., et al. (2020). The gut-microbiota-testis axis mediated by the activation of the Nrf2 antioxidant pathway is related to prepuberal steroidogenesis disorders induced by di-(2-ethylhexyl) phthalate. Environ. Sci. Pollut. Res. Int. 27, 35261–35271. doi: 10.1007/s11356-020-09854-2
Zheng, W., Zhang, S., Chen, X., Jiang, S., Li, Z., and Li, M. (2021). Case report: Dendritic cells and macrophages capture sperm in chronically inflamed human epididymis. Front. Immunol. 12:629680. doi: 10.3389/fimmu.2021.629680
Keywords: gut microbiota, testis, male fertility, gut-testis axis, probiotics
Citation: Cai H, Cao X, Qin D, Liu Y, Liu Y, Hua J and Peng S (2022) Gut microbiota supports male reproduction via nutrition, immunity, and signaling. Front. Microbiol. 13:977574. doi: 10.3389/fmicb.2022.977574
Received: 24 June 2022; Accepted: 02 August 2022;
Published: 18 August 2022.
Edited by:
Karolina Skonieczna-Żydecka, Pomeranian Medical University, PolandReviewed by:
Agata Mulak, Wrocław Medical University, PolandYong Zhao, Chinese Academy of Agricultural Sciences (CAAS), China
Copyright © 2022 Cai, Cao, Qin, Liu, Liu, Hua and Peng. This is an open-access article distributed under the terms of the Creative Commons Attribution License (CC BY). The use, distribution or reproduction in other forums is permitted, provided the original author(s) and the copyright owner(s) are credited and that the original publication in this journal is cited, in accordance with accepted academic practice. No use, distribution or reproduction is permitted which does not comply with these terms.
*Correspondence: Sha Peng, cGVuZ3NoYWN4aEBud2FmdS5lZHUuY24=
†These authors have contributed equally to this work