- 1Malaria Molecular Epidemiology Unit, Institut Pasteur du Cambodge, Phnom Penh, Cambodia
- 2Center for Tropical and Emerging Global Diseases, University of Georgia, Athens, GA, United States
Plasmodium vivax, one species of parasite causing human malaria, forms a dormant liver stage, termed the hypnozoite, which activate weeks, months or years after the primary infection, causing relapse episodes. Relapses significantly contribute to the vivax malaria burden and are only killed with drugs of the 8-aminoquinoline class, which are contraindicated in many vulnerable populations. Development of new therapies targeting hypnozoites is hindered, in part, by the lack of robust methods to continuously culture and characterize this parasite. As a result, the determinants of relapse periodicity and the molecular processes that drive hypnozoite formation, persistence, and activation are largely unknown. While previous reports have described vastly different liver-stage growth metrics attributable to which hepatocyte donor lot is used to initiate culture, a comprehensive assessment of how different P. vivax patient isolates behave in the same lots at the same time is logistically challenging. Using our primary human hepatocyte-based P. vivax liver-stage culture platform, we aimed to simultaneously test the effects of how hepatocyte donor lot and P. vivax patient isolate influence the fate of sporozoites and growth of liver schizonts. We found that, while environmental factors such as hepatocyte donor lot can modulate hypnozoite formation rate, the P. vivax case is also an important determinant of the proportion of hypnozoites observed in culture. In addition, we found schizont growth to be mostly influenced by hepatocyte donor lot. These results suggest that, while host hepatocytes harbor characteristics making them more- or less-supportive of a quiescent versus growing intracellular parasite, sporozoite fating toward hypnozoites is isolate-specific. Future studies involving these host–parasite interactions, including characterization of individual P. vivax strains, should consider the impact of culture conditions on hypnozoite formation, in order to better understand this important part of the parasite’s lifecycle.
Introduction
Malaria remains a major public health challenge with an estimated 241 million cases in 2021 (World Health Organization, 2021). Among the parasite species responsible for human malaria, Plasmodium vivax is the most widely dispersed as well as the most resistant to elimination programs. This resilience is attributed to several features unique to P. vivax, including its ability to develop over a wider range of temperatures and, in particular, at lower thermal limits than Plasmodium falciparum. Additionally, P. vivax forms transmissible gametocytes faster, and has a shorter incubation period in the mosquito vector, than P. falciparum (MacDonald, 1957; World Health Organization, 1975; Bousema and Drakeley, 2011; Thomas et al., 2018). Critically, P. vivax parasites persist in the human host liver as hypnozoites (a dormant parasite liver stage) which activate weeks, months, or years after the primary infection, causing relapse episodes (White, 2011). Hypnozoites are insensitive to most antimalarials except 8-aminoquinolines, which are contraindicated in large sections of the population including pregnant women, younger children, and patients with glucose-6-phosphate dehydrogenase deficiency (Baird et al., 2018). Because in vitro culture of liver stages is dependent on limited access to P. vivax-infected mosquitoes, our understanding of P. vivax liver stages has considerably lagged in comparison to P. falciparum. Consequently, the determinants of relapse periodicity and the molecular processes that drive hypnozoite formation, persistence, and activation are still largely unknown.
Transmission of P. vivax occurs when sporozoites are injected into a new host by the bite of an infected Anopheles mosquito. Individual P. vivax sporozoites migrate to the liver, invade a hepatocyte, and form either a liver-schizont or hypnozoite. Plasmodium vivax schizonts mature within 9–12 days and release merozoites into the bloodstream, thereby initiating the primary blood-stage infection, while P. vivax hypnozoites are small, non-dividing forms that remain quiescent for various periods of time (Mueller et al., 2009). Frequencies of P. vivax relapses are highly variable, from 3 –4 weeks in the tropics to 8–10 months in temperate regions, and it remains unknown if the frequencies observed are determined by genetic or environmental factors (Mueller et al., 2009; Hulden and Hulden, 2011; White, 2011; White et al., 2014). Several factors influencing relapse frequency have been proposed such as sporozoite inoculum size, acquired immunity of the host, primary drug treatment regimens, co-infections, fever, hemolysis, seasonality, mosquito bites, and epigenetic control (Hulden and Hulden, 2011; White, 2011; Shanks and White, 2013; Dembele et al., 2014; Commons et al., 2019). However, a constant activation rate without external stimuli could also explain the frequencies observed (White et al., 2014). Yet, the prevalence of hypnozoite formation is rarely considered and difficult to directly ascertain in living systems (Bright et al., 2014; Mikolajczak et al., 2015).
The cellular interactions governing migration and invasion of sporozoites into hepatocytes are species-specific and only partially understood, rendering generalizations difficult (Manzoni et al., 2017; Dundas et al., 2019). Comparisons of infection rates in several human hepatocyte donor lots show that some lots are not supportive of either P. vivax or P. falciparum parasites, which could be a product of natural variation in hepatic surface receptors necessary to malaria parasite entry (Manzoni et al., 2017). Alternatively, the process for manufacturing cryo-plateable primary hepatocytes could affect cell phenotypes, including the aforementioned surface receptors, and alter hepatocyte permissiveness (Roth et al., 2018; Maher et al., 2021b). Host cell permissiveness is likely also modulated by the host cell environment as sufficient glycolytic and respiratory activities are needed to sustain the energy demands of an intracellular parasite (Kaushansky et al., 2013; Kaushansky and Kappe, 2015; Yang et al., 2021). As such, liver lobules perform different metabolic functions and have recently been shown to influence P. falciparum parasite preferences and growth in the host cell (Yang et al., 2021). Interestingly, a recent single-cell transcriptomic study of P. vivax liver stages did not show a clear pattern of infection in different hepatocyte subpopulations, although, it is unknown if zonally-differentiated hepatocytes remain fully differentiated ex vivo (Ruberto et al., 2022). Thus, human host hepatocyte characteristics are likely important factors in parasite development as well as potential determinants of the schizont or hypnozoite fate remaining to be discovered.
The density of individuals within a shared environment has a strong impact on individual fitness. For parasites such as Plasmodium spp., fitness depends on interactions with several organisms during their life cycle, including but not limited to, the human host, the mosquito vector, and co-infecting malaria parasites. The hosts represent ecological niches for co-infecting malaria parasites, which often consist of more than one parasite genotype (Read and Taylor, 2001; Bruce and Day, 2002). Therefore, individual parasites are in direct competition for resources, in indirect competition with shared exposure to immune responses, and potentially in direct interference between parasites, which can all affect virulence and transmission (Read and Taylor, 2001; Bruce and Day, 2002; Raberg et al., 2006; Metcalf et al., 2011). Although much work has been carried out on P. falciparum and to a lesser extent P. vivax asexual stages due to the availability of culture and analyses, these bottom-up and top-down mechanisms could also affect sporozoite fate akin to how crowding and inbreeding rate, among other factors, influence gametocyte fate and infectivity (Taylor et al., 1997; Reece et al., 2008; Bousema and Drakeley, 2011). Thus, during transmission from the vector to the human host, parasite competition could theoretically favor the production of hypnozoite-fated sporozoites to decrease future competition in the human host and increase the likelihood of relapse during the next high-transmission season.
Using our recently-developed primary human hepatocyte (PHH)-based 384-well P. vivax liver-stage culture platform (Roth et al., 2018; Maher et al., 2021b), we aimed at testing the effects of PHH donor lots and P. vivax cases on liver-stage parasites. While we have previously reported how sporozoites behave when infected into different PHH donor lots in this system, due to logistical challenges these studies relied on historical comparisons of sporozoites from only a single P. vivax isolate for each experimental run (Roth et al., 2018). This original approach cannot account for factors which are known to or likely affect parasite viability and phenotypes, such as the effect of an international shipment needed to send infected mosquitoes from an endemic area to a research laboratory, the health and genetic drift of a mosquito colony over time, the variation of different seedings of each donor lot of PHHs, specific lots of reagents such as culture media, the conditions of the laboratory environment and equipment, and which human operators perform dissection, infection, and media replacement during culture. To effectively remove or better control for these factors, this assessment relied on an experimental design in which the same four PHH donor lots, which were pre-validated to support P. vivax infection, were seeded into different wells of the same microtiter plates on the same day and infected with the same inoculum of sporozoites from three different P. vivax patient isolates. We used this design to confirm that PHH donor lots influence the total number of parasites and investigate if these lots specifically affected schizont growth and the proportion of hypnozoites observed. Additionally, to characterize other factors critical for establishing in vitro culture, we factored into our design an assessment of how the sporozoite inoculum size and P. vivax case impacted these three aspects (total number of parasites, schizont size, and proportion of hypnozoites).
Materials and methods
Clinical isolates and collection of Plasmodium vivax sporozoites
Blood samples were collected from symptomatic P. vivax patients at local health facilities in Mondulkiri province (eastern Cambodia) from 2018 to 2021. Clinical isolate collection and research procedures were reviewed and approved by the Cambodian National Ethics Committee for Health Research (approval number: 100NECHR, 113 NECHR, 104 NECHR). The protocols conform to the Helsinki Declaration on ethical principles for medical research involving human subjects (version 2002) and informed written consent was obtained for all volunteers, or their parent or legal guardian for participants under 18 years old. Patients presenting signs of severe malaria, infected with non-vivax malaria parasites, under 5 years of age, pregnant, or lactating were excluded from the collection. Following informed consent from eligible study participants, venous blood samples were collected by venipuncture into heparin-containing tubes. Immediately after collection, treatment was provided by local health staff according to Cambodia National Malaria Treatment Guidelines. Clinical isolates were immediately prepared for feeding to Anopheles dirus mosquitoes in a secure insectary as previously described (Maher et al., 2021b). Following a P. vivax gametocyte-containing blood meal, An. dirus mosquitoes were maintained on a 10% sucrose +0.05% para-aminobenzoic solution. Mosquitoes found positive for P. vivax oocysts at 6 days post feeding were transported to the IPC facility in Phnom Penh, Cambodia where salivary glands were aseptically dissected into RPMI without sodium bicarbonate on 16–21 days post-infection (dpi).
Liver-stage infection
Primary human hepatocytes (PHH) were seeded 2 days prior to infection (except for Experiment 1 for which they were seeded 3 days prior) and cultured as previously described (Maher et al., 2021b). Infection was performed by diluting freshly-dissected sporozoites into culture media with antibiotics (gentamicin, penicillin, streptomycin, neomycin), adding 20 μl sporozoite-media mixture to each well, and centrifugation of the 384-well plate at 200 RCF for 5 min at room temperature. Media was changed with fresh culture media containing antibiotics the day after infection and every 2–3 days thereafter. At 8 or 12 dpi (depending on the experiment protocol, see below) cultures were fixed for 15 min at room temperature with 4% paraformaldehyde in PBS. Fixed cultures were stained with recombinant mouse anti-P. vivax Upregulated in Infectious Sporozoites-4 antibody (UIS4; Schafer et al., 2018) diluted 1:25,000, followed by rabbit anti-mouse Alexa Fluor488-conjugated antibody (Invitrogen™, catalog number: A11029) diluted 1:1000. Cultures were then counterstained with 10 μg/ml Hoechst 33342 (Invitrogen™, catalog number: H21492) to detect parasite and host cell nuclear DNA. Automated High Content Imaging was carried out with a 20× objective on a ImageXpress Confocal Micro (Molecular Devices) or 4× objective on a Lionheart (BioTek). Liver-stage parasite were quantified for number and growth area per well and per parasite using built-in cellular analysis and quantification software (MetaXpress for ImageXpress or Gen5 for Lionheart). Hypnozoites were defined as brightly UIS4-stained round forms (ratio of maximum and minimum widths of each form >0.6) with under 150 μm2 total area and a bright prominence in the parasitophorous vacuole membrane. Schizonts were defined as brightly-UIS4-stained forms with greater than 150 μm2 total area (Maher et al., 2021a,b).
Experiment 1: Testing simultaneously different PHH donor lots, Plasmodium vivax cases, and sporozoite inoculum sizes
Four PHH donor lots, UBV, BGW, HHR, and OTW, were infected with three different P. vivax cases (C1 to C3) at eight sporozoite densities ranging from 12 × 103 to 30 × 103 per well in 3 × 103 increments (Supplementary Figure 1). Six technical replicate wells per condition were used. Cultures were fixed at eight dpi.
Experiment 2: Comparison of eight PHH donor lots and influence of sporozoite inoculum size
Eight PHH donor lots, BGW, BPB, DQQ, ERR, HDS, HLY, IRZ, and ZPE, were infected with one P. vivax case at six sporozoite densities ranging from 5 × 103 to 30 × 103 per well in 5 × 103 increments. Four technical replicate wells per conditions were used. Cultures were fixed at eight dpi.
Experiment 3: Comparison of 51 Plasmodium vivax cases using one PHH donor lot
Negative control wells containing 0.1% v/v DMSO from our large P. vivax liver-stage drug screening database (Maher et al., 2021a) were used to compare results from 51 P. vivax cases used to infect 132 assay plates. All assay plates were seeded with PHH donor lot BGW and comprised 106 plates with and 26 plates without 1-aminobenzotriazole (ABT) treatment, a protocol condition used to limit phase I hepatic metabolism of unoptimized test compounds (Maher et al., 2021b). The number of hypnozoites and schizonts was averaged over 16 or 24 wells DMSO control wells depending on the plate map. The majority of cultures were seeded with PHHs 2 days prior to infection with sporozoites, although for some infections cultures were initiated 1 or 3 days prior to infection due to logistical constraints. All cultures were fixed at twelve dpi.
Statistical analyses
Experiment 1: Testing different PHH donor lots, Plasmodium vivax cases, and sporozoite inoculum sizes
The total number of parasites was analyzed using a Generalized Linear Mixed Model (GLMM) with a zero-truncated negative binomial error structure. In this GLMM, PHH donor lot (4 levels: BGW, HHR, OTW, and UBV), P. vivax case (3 levels: C1, C2, and C3), and sporozoite inoculum size (12 × 103, 15 × 103, 17 × 103, 19 × 103, 21 × 103, 24 × 103, 27 × 103 and 30 × 103) were coded as fixed categorical factors, the log of number of nuclei was coded as an offset to account for the number of PHH per well, and well nested in plate were coded as random factors to account for repeated measurements of the same infection conditions and plate effects. The proportion of hypnozoites was analyzed using a similar GLMM with a binomial error structure and individual well ID was added as a random factor to improve the model fit.
The schizont size (individual measurement of all schizonts present in the well) was Box-Cox transformed and analyzed using a GLMM with a Gaussian distribution. In this model, PHH donor lot, P. vivax case, sporozoite inoculum size, and all two-way interactions were coded as fixed categorical factors and well nested in plate were coded as random factors.
Experiment 2: Comparison of eight PHH donor lots and influence of sporozoite inoculum size or seed density
The total number of parasites and proportion of hypnozoites were analyzed using GLMMs with a zero-truncated negative binomial error structure and a binomial error structure, respectively. In these GLMMs, PHH donor lot and sporozoite inoculum size were coded as fixed categorical factors, the log of number of nuclei was coded as an offset to account for the number of PHHs per well, and replicate wells were coded as random factors to account for repeated measurements of the same infection conditions.
Experiment 3: Comparison of 51 Plasmodium vivax cases using one PHH donor lot
The total number of parasites and proportion of hypnozoites were analyzed using univariate GLMMs with a zero-truncated negative binomial error structure and a binomial error structure, respectively. In these GLMMs, the log of the average number of nuclei was coded as an offset to account for the number of PHHs per well, and P. vivax case was coded as a random factor to account for measurements of assay plates infected with the same P. vivax case. We investigated the effects of sporozoite inoculum size, PHH age at infection, assay version, season, and patient sex. In addition, a data subset of 37 plates for which the visit count was known (that is, the number of times the same patient visited the clinic, which was either 2, 3, 5, or 6 visits) was used to investigate the effect of multiple visits on the proportion of hypnozoites using a univariate GLMM with a binomial structure.
Model selection was used with the stepwise removal of terms, followed by likelihood ratio tests (LRT). Term removals that significantly reduced explanatory power (p < 0.05) were retained in the minimal adequate model (Crawley, 2007). All analyses were performed in R v. 4.0.3 (R Core Team, 2020). Results are presented as mean ± standard error (SE) and proportion ± confidence interval (CI).
Results
Experiment 1: Testing different PHH donor lots, Plasmodium vivax cases, and sporozoite inoculum sizes
In this experiment, four PHH donor lots were infected with three different P. vivax cases at eight sporozoite densities. The average number of parasites per well was significantly influenced by the PHH donor lot ( = 1023.78, p < 0.0001; Figure 1A), with BGW supporting the highest mean parasite per well (260.85 ± 9.64), followed by UBV (162.12 ± 6.09), HHR (123.48 ± 4.74) and OTW (79.28 ± 3.65). The number of parasites was also influenced by the P. vivax case ( = 13.67, p = 0.001; Figure 1B) with P. vivax case 3 having the highest number of parasites per well (173.95 ± 8.68), followed by P. vivax case 1 (168.98 ± 7.64) and P. vivax case 2 (126.36 ± 4.77). As expected, the average number of parasites per well increased with the sporozoite inoculum size ( = 228.37, p < 0.0001; Figure 1C), increasing from 89.97 ± 6.76 parasites per well when 12 × 103 sporozoite were inoculated to 211.94 ± 16.66 parasites per well with 30 × 103 sporozoites.
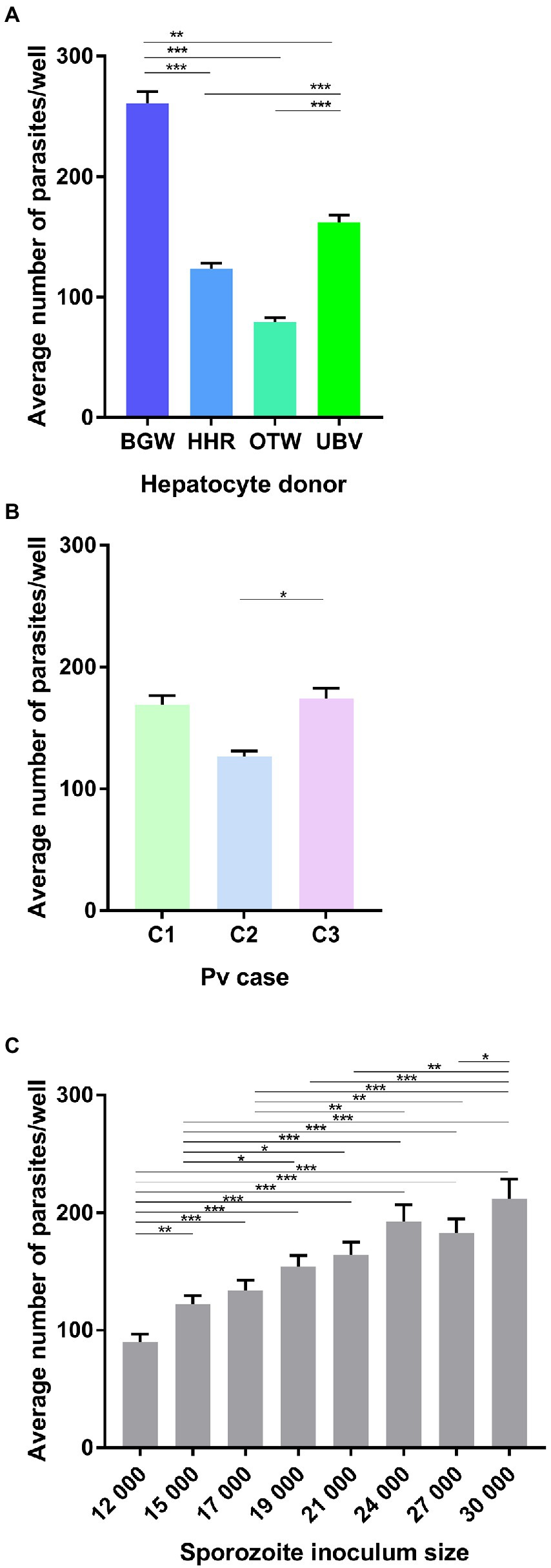
Figure 1. Analysis of the average number of Plasmodium vivax parasites per well. Data include all parasites quantified from Experiment 1 in which four PHH donor lots (UBV, BGW, HHR, and OTW) were infected with three different P. vivax cases (C1–C3) at eight sporozoite densities ranging from 12 × 103 to 30 × 103 per well and fixed at 8 days post-infection. Data are categorized by (A) PHH donor lot, (B) P. vivax isolate, and (C) sporozoite inoculum size. Asterisks indicate significant differences (Post hoc Tukey’s pairwise comparisons, ***p < 0.0001, **p < 0.001, *p < 0.05). Bars represent ± SE.
The proportion of hypnozoites per well was significantly influenced by the PHH donor lot ( = 589.50, p < 0.0001; Figure 2A) with UBV supporting the highest proportion (65.11 ± 0.31%), followed by BGW (63.97 ± 0.31%), OTW (54.37 ± 0.32%), and HHR (52.36 ± 0.33%). The three P. vivax cases investigated had significantly different proportion of hypnozoites, ranging from 57.9 ± 0.32% in P. vivax case 1, to 61.8 ± 0.32% in P. vivax case 2 and 62.89 ± 0.31% in P. vivax case 3 ( = 50.73, p < 0.0001; Figure 2B). The proportion of hypnozoites also showed a small increase as the sporozoite inoculum size increased, from 58.6 ± 0.32% when 12 × 103 sporozoite were inoculated to 63.68 ± 0.31% with 30 × 103 sporozoites ( = 20.09, p = 0.005; Figure 2C).
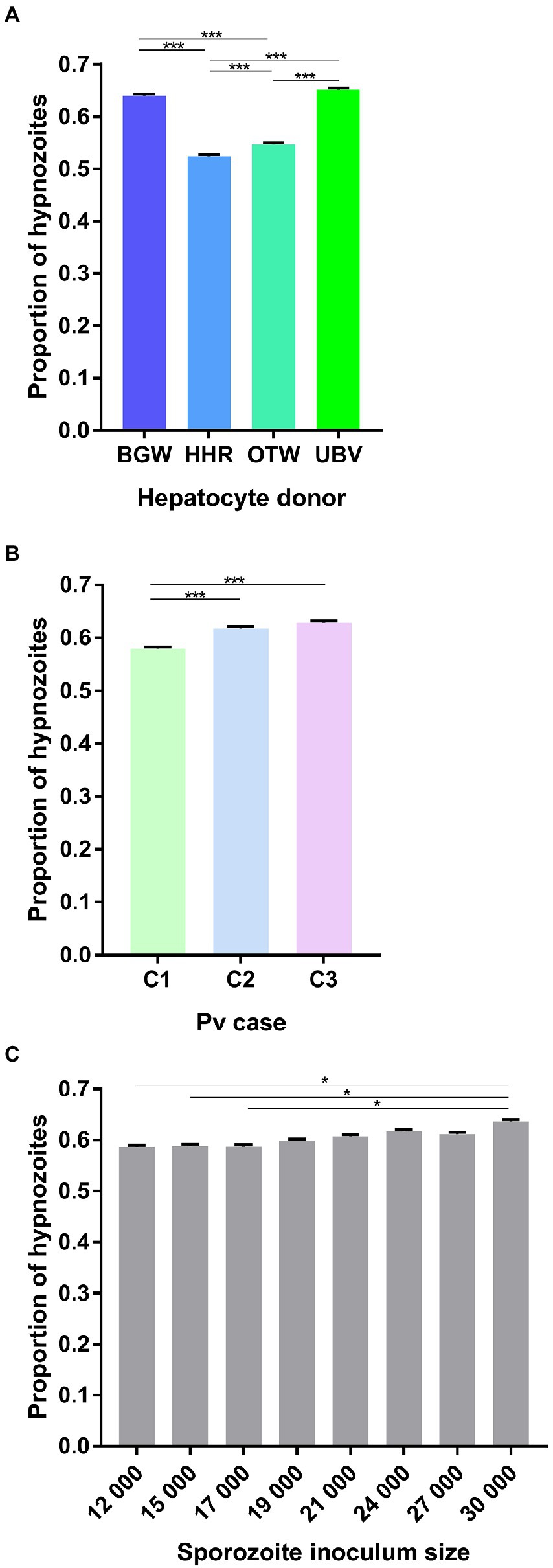
Figure 2. Analysis of the average proportion of P. vivax hypnozoites per well. Data include all parasites quantified from Experiment 1 in which four PHH donor lots (UBV, BGW, HHR, and OTW) were infected with three different P. vivax cases (C1 to C3) at eight sporozoite densities ranging from 12 × 103 to 30 × 103 per well and fixed at 8 days post-infection. Data are categorized by (A) PHH donor lot, (B) P. vivax isolate, and (C) sporozoite inoculum size. Asterisks indicate significant differences (Post hoc Tukey’s pairwise comparisons, ***p < 0.0001, *p < 0.05). Bars represent ±95% CI.
The schizont size was significantly affected by the PHH donor lot ( = 4369.66, p < 0.0001; Figure 3A) with an average schizont size of 1195.46 ± 8.25 μm2 for HHR, followed by OTW (1021.61 ± 9.16 μm2), UBV (443.49 ± 2.94 μm2), and BGW (441.85 ± 2.83 μm2). Schizonts were on average larger in the infection from P. vivax case 3 (770.31 ± 6.41 μm2), followed by P. vivax case 1 (678.02 ± 4.81 μm2) and P. vivax case 2 (663.75 ± 5.39 μm2; = 28.63, p < 0.0001; Figure 3B). The average size of schizonts negatively correlated to the sporozoite inoculum size ( = 119.15, p < 0.0001; Figure 3C). There was a significant interaction of P. vivax case and PHH donor lot ( = 71.60, p < 0.0001; Figure 3D) such that lots providing the largest or smallest average schizont size were not always the same across infections with the three P. vivax cases.
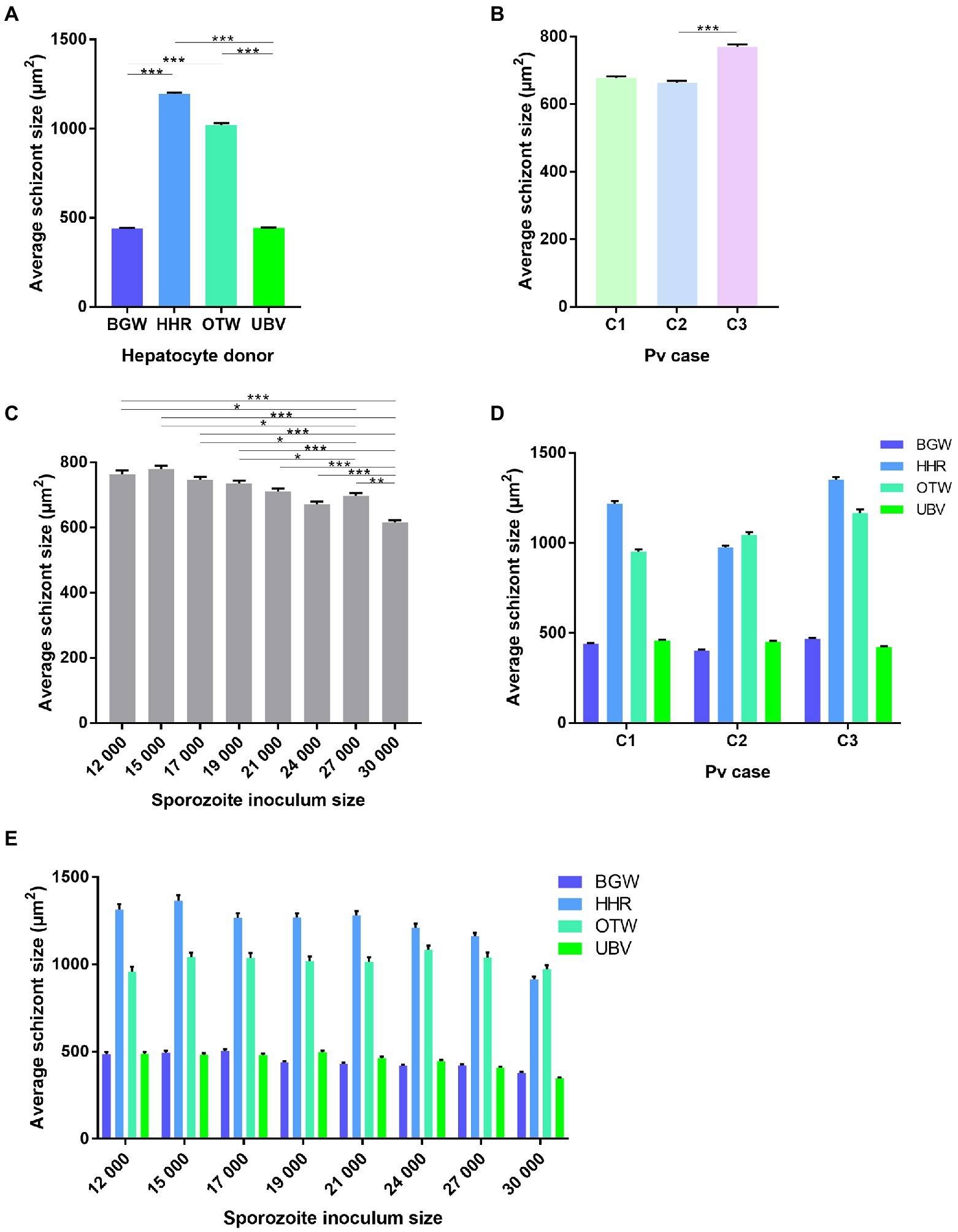
Figure 3. Analysis of the average size of P. vivax schizonts by PHH donor lot and P. vivax case. Data include all parasites from Experiment 1 in which four PHH donor lots (UBV, BGW, HHR, and OTW) were infected with three different P. vivax cases (C1–C3) at eight sporozoite densities ranging from 12 × 103 to 30 × 103 per well and fixed at 8 days post-infection. Data are categorized by (A) PHH donor lot, (B) P. vivax isolate, (C) sporozoite inoculum size, (D) P. vivax isolate and PHH donor lot, and (E) sporozoite inoculum size and PHH donor lot. Asterisks indicate significant differences (Post hoc Tukey’s pairwise comparisons, ***p < 0.0001, **p < 0.001, *p < 0.05). Bars represent ± SE.
The differences in average schizont sizes between PHH donor lots tended to decrease as the sporozoite inoculum size increased; this effect was most apparent between lots HHR and OTW, which produced the largest schizonts of the four lots (PHH lots – sporozoite inoculum size interaction: = 42.84, p = 0.0033; Figure 3E). The interaction between P. vivax case and sporozoite inoculum size was not significant ( = 19.42, p = 0.15). Overall, UBV and BGW harbored a large proportion of small schizonts whereas HHR and OTW harbored similar proportions of schizonts of different size classes (Supplementary Figure 2). We did not investigate further a correlation between the total number of parasites in the well and the average schizont sizes as the data were segregated with UBV and BGW forming one group and OTW and HHR forming another group (Supplementary Figure 3).
Experiment 2: Comparisons of eight PHH donor lots and influence of sporozoite inoculum size
Eight different PHH donor lots were seeded and infected with six different sporozoite inoculums from a single P. vivax case. The average number of parasites per well was significantly influenced by the PHH donor lot ( = 196.01, p < 0.0001; Figure 4A) and the sporozoite inoculum size ( = 192.24, p < 0.0001; Figure 4B). The proportion of hypnozoites was significantly influenced by the PHH donor lot ( = 413.37, p < 0.0001; Figure 4C) but not by the sporozoite inoculum size ( = 11.026, p = 0.051; Figure 4D).
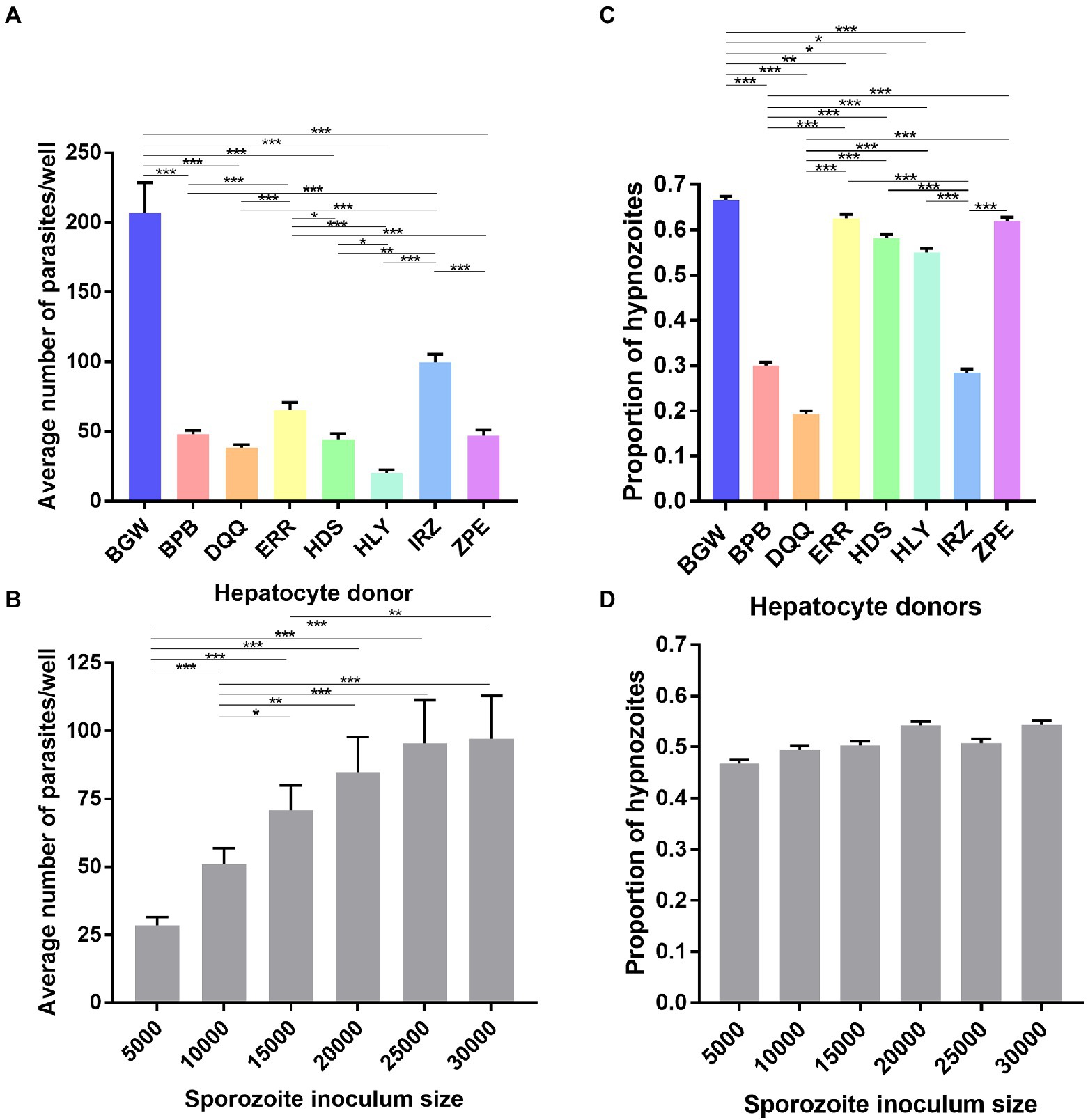
Figure 4. Growth metrics of liver-stage parasites following infection of eight PHH donor lots with one P. vivax case. Data include all parasites quantified from Experiment 2 in which eight PHH donor lots (BGW, BPB, DQQ, ERR, HDS, HLY, IRZ, ZPE) were infected with one P. vivax case at six sporozoite densities ranging from 5 × 103 to 30 × 103 per well. The average number P. vivax parasites per well is shown categorized by (A) PHH donor lot and (B) sporozoite inoculum size. The average proportion of P. vivax hypnozoites per well is shown categorized by (C) PHH donor lots and (D) sporozoite inoculum size. Asterisks indicate significant differences (Post hoc Tukey’s pairwise comparisons, ***p < 0.0001, **p < 0.001, *p < 0.05). Bars represent ± SE (A,B) or ± 95% CI (C,D).
Experiment 3: Comparison of 51 Plasmodium vivax cases using one PHH donor lot
One PHH donor lot (BGW) was used to seed 132 assay plates infected with 51 P. vivax cases. The total number of parasites per well was influenced by the P. vivax case ( = 137.5, p < 0.0001; Figure 5A) but not by the sporozoite inoculum size ( = 3.40, p = 0.065, Supplementary Figure 4A). The average total number of parasites per well was influenced by the PHH age at infection ( = 11.954, p = 0.002, Supplementary Figure 4B). However, post-hoc comparisons showed only a significantly lower total number of parasites in PHHs infected at day 1 compared to day two post-seeding (81.83 ± 9.62 vs. 136.24 ± 7.12 respectively; Tukey’s post-hoc test: p = 0.001, all other comparisons being non-significant). The average total number of parasites per well was not influenced by the presence of ABT ( = 0.14, p = 0.71, Supplementary Figure 4C), the season ( = 0.41, p = 0.52, Supplementary Figure 4D), nor the patient sex ( = 0.02, p = 0.88, Supplementary Figure 4F). The proportion of hypnozoites was influenced by the P. vivax case ( = 197.58, p < 0.0001; Figure 5B) but not by the sporozoite inoculum size ( = 0.69, p = 0.40, Supplementary Figure 5A). The proportion of hypnozoites was also influenced by the PHH age at infection ( = 9.92, p = 0.007, Supplementary Figure 5B). However, post-hoc comparisons showed only a significantly lower proportion of hypnozoites in PHHs infected at day three compared to day one post-seeding (57.3 ± 0.7% vs. 63.3 ± 0.7%; Tukey’s post-hoc test: p = 0.006, all other comparisons being non-significant). The proportion of hypnozoites was not affected by the presence of ABT ( = 2.99, p = 0.08, Supplementary Figure 5C), the season (dry: 60.1 ± 0.7% vs. rainy: 66.6 ± 0.7%; = 3.5, p = 0.06, Supplementary Figure 5D) nor the patient sex ( = 0.10, p = 0.75, Supplementary Figure 5E). The proportion of hypnozoites was not significantly influenced by the number of visits to the clinic a patient already experienced ( = 1.36, p = 0.24, Supplementary Figure 5F).
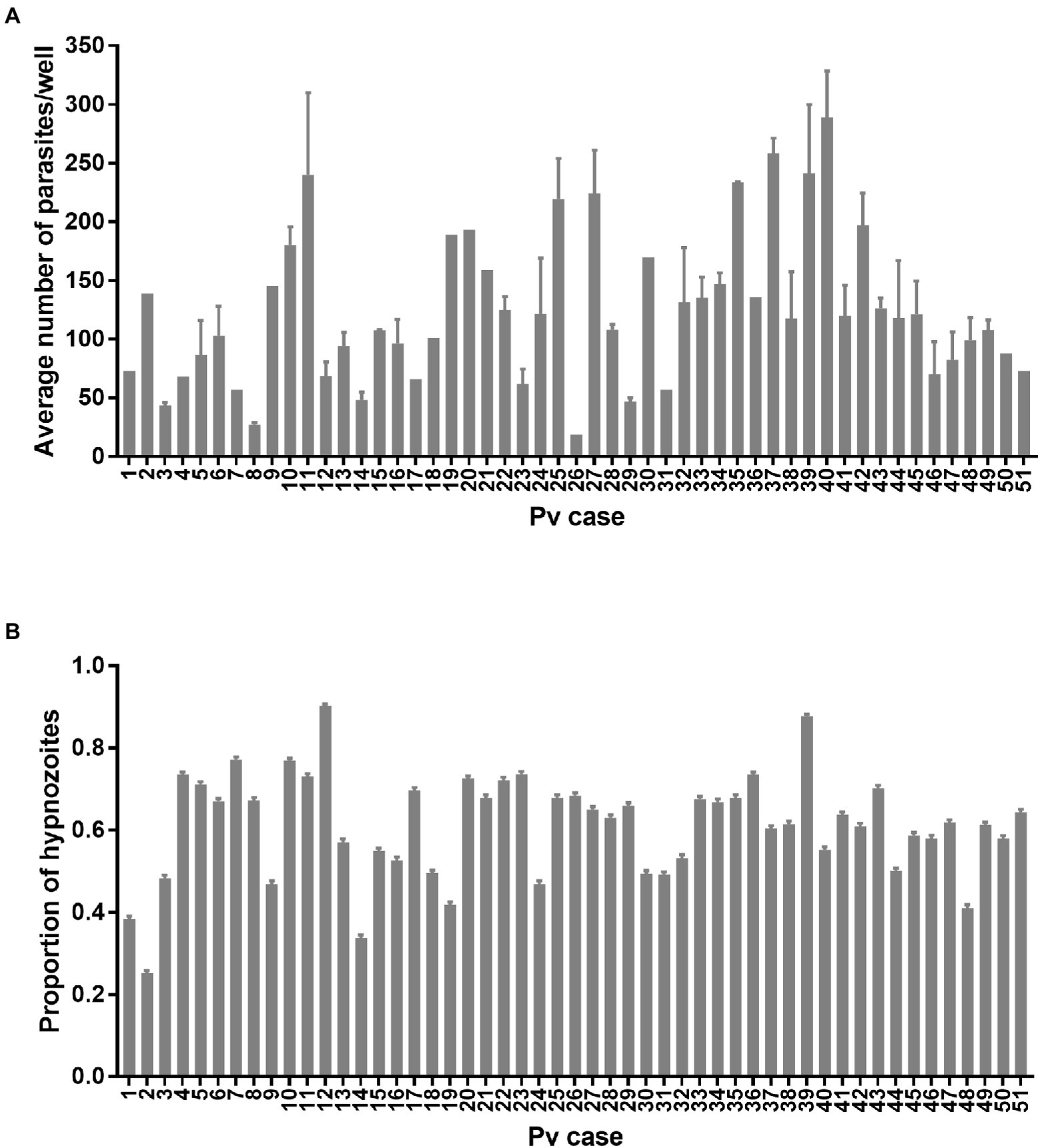
Figure 5. Growth metrics of P. vivax liver-stage parasites from 51 cases. (A) Average number of parasites per well and (B) proportion of hypnozoites from the 0.1% DMSO control wells of 132 assay plates initiated from 51 P. vivax cases for liver-stage drug discovery and development. Bars represent ±SE (A) or ±95% CI (B).
Discussion
The determinants of relapse periodicity and the molecular processes that drive hypnozoite formation, persistence, and activation are still largely unknown. Three non-mutually exclusive hypotheses have been proposed regarding the determinants of hypnozoite formation: predetermination during the sporozoite development in the mosquito, fate determination as sporozoites progress from mosquito salivary glands to the hepatocytes, and fate determination after infecting the host hepatocyte (reviewed in Schäfer et al., 2021). We found that both the PHH donor lot and the P. vivax case influenced the proportion of hypnozoites observed (Figures 2, 4). This suggests that the proportion of hypnozoites is strain-dependent as previously shown in a humanized mouse model (Mikolajczak et al., 2015), and as suggested by historical data on the heterogeneity of P. vivax strains (Boyd and Kitchen, 1937, 1948; Boyd, 1940; Schwartz et al., 1950). Indeed, the frequency and number of relapses are highly variable and the patterns of infections have been shown to vary with the geographic origin of the parasites (Boyd and Kitchen, 1937, 1948; Boyd, 1940; Schwartz et al., 1950). Intrinsic variations in susceptibility to 8-aminoquinolines have also been shown with, for example, higher doses of primaquine required to eliminate the Chesson strain compared to the St. Elizabeth strain (Ehrman et al., 1945; Edgcomb et al., 1950; Garrison et al., 1952; Cooper et al., 1953; Alving et al., 1955). Although laboratory conditions have some variability, the large differences in hypnozoite proportions observed with infections from 51 P. vivax cases using one PHH donor lot over time further support this hypothesis. Interestingly, a recent report suggests sporozoites found in mosquito salivary glands do not seem to present two distinct transcriptional signatures corresponding to future hypnozoites and schizonts (Ruberto et al., 2021b), further suggesting that transcriptional changes responsible for the fate of sporozoites could occur in the host hepatocyte or be epigenetically-controlled (Schäfer et al., 2021).
The different PHH donor lots we tested showed large variation in both the quantity and ratio of hypnozoites to schizonts after infection. We performed two experiments comparing multiple PHH donor lots; the first with four lots previously identified as highly supportive of P. vivax infection (Figure 1A), and another with seven lots supporting various levels of P. vivax infection in comparison to the highly-supportive lot BGW (Figure 4A). These results suggest host hepatocytes harbor characteristics making them more- or less-supportive of a quiescent versus growing intracellular parasite. Indeed, recent studies have shown that liver-stage parasites must form and maintain a delicate interface with the host hepatocyte’s lysosomes, with failure leading to parasite death (Niklaus et al., 2019). This survivability factor is one example of many possible host–parasite interactions that could explain both the net parasite and ratio differences we noted across PHH donor lots. Another explanation for the infection rate differences is that, while sporozoites are traversing hepatocytes, there may sense the suitability of a particular hepatocyte prior to switching from traversal to invasion, which is distinctly marked by formation of a parasitophorous vacuole membrane (reviewed in (Sinnis and Coppi, 2007)). Such a tropism has been described for P. yoelii and P. falciparum sporozoites preferentially infecting polyploid hepatocytes (Austin et al., 2014). Regarding the fate of sporozoites in these different donor lots, it has been shown that P. vivax hypnozoites are susceptible to several antimalarials for the first few days post-hepatocyte infection (Gural et al., 2018; Posfai et al., 2020). During this time, the parasite’s cytoplasmic compartment and membranes grow to several times the volume of a sporozoite and begin to incorporate host proteins such as aquaporin 3, indicating sporozoites must establish quiescence, and are not immediately quiescent, following infection (Posfai et al., 2020). Likewise, recent reports of the earliest known markers of liver schizogony, including DNA synthesis, division of the parasite nucleus, and expression of liver-specific protein 2 at 3 days post-hepatocyte infection, suggest that commitment to schizogony may not occur immediately after hepatocyte infection (Mikolajczak et al., 2015; Gupta et al., 2019; Botnar et al., 2022). As there seems to be ample time for a cell-cycle checkpoint to prevent DNA synthesis as liver forms are established, we speculate that, over the first 24–48 h post-infection, sporozoites may be able to sense, or at least be influenced by, the intracellular environment of the hepatocyte, and then respond to specific conditions or stimuli by forming either a hypnozoite or schizont. Yet another possible explanation for the different infection rate and hypnozoite ratio noted across PHH donor lots could be the composition of hepatocytes in each lot from either zones 1, 2, or 3 of the liver lobule. Liver lobules perform specific metabolic functions and display different levels of glycolysis and cellular respiration (Kaushansky et al., 2013; Kaushansky and Kappe, 2015; Yang et al., 2021). Recently, a study of PHHs infected with P. falciparum demonstrated that cultured PHHs are comprised different ratios of cells from each zone and zonal differentiation as important for liver-stage development. In our recent report of single-cell RNA-sequencing of P. vivax liver stages and host hepatocytes, we did look for an infection and fating preference for sporozoites infected into a culture of various hepatocyte subpopulations; however, we found no clear infection pattern or preference (Ruberto et al., 2022). Further deciphering the components of the host cell environment as allowing or favoring hypnozoites versus schizont formation would help better understand the mechanisms of dormancy.
In Cambodia, vivax malaria is less-frequently transmitted during the dry season, when the population of the Anopheline vector is at its lowest level. During this time, it would be advantageous for any vivax parasites that do transmit to form hypnozoites, such that the blood-stage infections resulting from transmission would occur after the end of the dry season. Such a mechanism has been described for strains of long-latency vivax malaria such as those formerly prevalent in northern latitudes (Huldén and Heliövaara, 2008). However, seasonal variation in Cambodia might not be strong enough to select for long-latency strains. It has also been shown that, for some strains, once relapses begin after a period of latency, they are frequent, indicating cessation of latency is also programmed (White, 2011). In this study, we had the unique opportunity to quantify the formation of hypnozoites and schizonts of P. vivax isolates from patients during the dry season as well as from the same patients visiting the clinic for malaria therapies multiple times. While we did not find an apparent effect of the same patient recurrently visiting the clinic nor of the season on the proportion of hypnozoites, we did find that some of the 51 cases exhibited remarkably high hypnozoite ratios, indicating genotypes encoding for hypnozoite formation do persist in the population and likely factor into latency and ongoing transmission. As we were not able to collect enough parasite material for DNA or RNA sequencing to further characterize these unique cases, future studies could combine hepatocyte donor lot panels with a multi-omics approach to better understand these genotype–phenotype relationships.
A crowding effect could influence sporozoites to become hypnozoites to avoid competition and increase the chance of transmission by opportunistically causing a relapse after the primary blood infection and subsequent immune response. To look for such a crowding effect on sporozoite fating we performed two experiments with an inoculum gradient culminating with a highest inoculum of 30 × 103 sporozoites per well, or a relatively large multiplicity of infection of >2 sporozoites for each PHH. As expected, the number of parasites increased positively with the sporozoite inoculum size in these two first experiments, resulting in an apparent plateau representing saturation (Figures 1C, 4B), However, we observed only a small influence of the sporozoite inoculum size on the proportion of hypnozoites in only one experiment out of three which suggest that this factor is not likely a strong determinant of sporozoite fating. In our third experiment, we further analyzed the effect of inoculum size and found it did not influence the infection rate of 51 P. vivax cases used for a routine drug screening program. Thus, the sporozoite inoculum size influences the infection rate with some modulations, which could be due to an intrinsic P. vivax case difference in infectivity or to the sporozoite development status. Indeed, salivary gland dissections result in the collection of all sporozoite available, not only the mature sporozoites which would have migrated within the saliva during a natural mosquito-bite infection. Therefore, developmental heterogeneity of sporozoites could explain the different infection rates observed across the P. vivax cases used (Ruberto et al., 2021a,b). Comparing hypnozoite ratios in hepatocyte infections resulting from dissections of one batch of infected mosquitoes over several days would help resolve an effect of sporozoite loiter time in the vector.
We found that schizont growth was strongly influenced by the PHH donor lot. Interestingly, two patterns were observed in P. vivax infections independent from a parasite density effect: PHH donor lots supporting a large proportion of small schizonts and few large schizonts versus lots supporting a homogenous distribution of schizont sizes. Future studies comparing the net production of merozoites would help determine if the two different strategies result in different parasite loads. Similarly, investigating the individual cell metabolic activities of these two classes of donor lot would help our understanding of the factors driving schizont development (Supplementary Table 1).
Elegant studies have shown the first relapses in life are genetically homologous and that the parasites causing relapses in a vivax malaria patient were likely caused by hypnozoites from meiotic sibling sporozoites from the oocyst phase of the lifecycle (Imwong et al., 2012; Bright et al., 2014). These studies suggest genetic crosses could be used to further investigate the determinates of sporozoite fating under controlled laboratory conditions. Such studies would be remarkably informative if methods are ever established for in vitro propagation and experimental transmission of P. vivax strains. In lieu of such an experimental system, P. vivax strains with distinct relapse phenotypes can be propagated and transmitted from experimental infections of nonhuman primates [reviewed in (Joyner et al., 2015)], or perhaps humanized mice (Schäfer et al., 2020). These systems would allow interrogation of P. vivax sporozoite fating with either wild-type or transgenic parasites strains and do so with experimental replication with parasites of the same genetic background. This report utilizes a well-controlled experimental design to identify and measure the relative effect of factors influencing sporozoite invasion and development in a hepatocyte culture system and, having used patient isolates to generate sporozoites, serves as a natural reference point as investigators focus on understanding hypnozoite biology using these alternative model systems.
Data availability statement
The original contributions presented in the study are included in the article/Supplementary material, further inquiries can be directed to the corresponding authors.
Ethics statement
The studies involving human participants were reviewed and approved by Cambodian National Ethics Committee for Health Research. Written informed consent to participate in this study was provided by all volunteers or the participants’ legal guardian/next of kin for participants under 18 years old.
Author contributions
AV, JP, and SM: conceptualization. AV: data curation, formal analysis, and visualization. AV, BW, SM, and DK: funding acquisition, project administration, and resources. JP, CC, AV, and SM: investigation. AV and SM: writing of original draft and preparation. All authors contributed to the article and approved the submitted version.
Funding
This work was supported by Medicines for Malaria Venture (RD/2017/0042 to BW and AV, RD/16/1082 and RD/15/0022 to SM and DK) and the Bill & Melinda Gates Foundation (OPP1023601 to DK).
Acknowledgments
We thank the patients of Mondulkiri Province, Cambodia, for participating in this study. We thank Tim Moeller of BioIVT for providing the panel of PHH donor lots used in Experiment 2 and metabolism data for all lots. High content imaging data were obtained in the Biomedical Microscopy Core at the University of Georgia, supported by the Georgia Research Alliance.
Conflict of interest
The authors declare that the research was conducted in the absence of any commercial or financial relationships that could be construed as a potential conflict of interest.
Publisher’s note
All claims expressed in this article are solely those of the authors and do not necessarily represent those of their affiliated organizations, or those of the publisher, the editors and the reviewers. Any product that may be evaluated in this article, or claim that may be made by its manufacturer, is not guaranteed or endorsed by the publisher.
Supplementary material
The Supplementary material for this article can be found online at: https://www.frontiersin.org/articles/10.3389/fmicb.2022.976606/full#supplementary-material
References
Alving, A. S., Arnold, J., Hockwald, R. S., Clayman, C. B., Dern, R. J., Beutler, E., et al. (1955). Potentiation of the curative action of primaquine in vivax malaria by quinine and chloroquine. J. Lab. Clin. Med. 46, 301–306.
Austin, L. S., Kaushansky, A., and Kappe, S. H. (2014). Susceptibility to Plasmodium liver stage infection is altered by hepatocyte polyploidy. Cell. Microbiol. 16, 784–795. doi: 10.1111/cmi.12282
Baird, J. K., Battle, K. E., and Howes, R. E. (2018). Primaquine ineligibility in anti-relapse therapy of Plasmodium vivax malaria: the problem of G6PD deficiency and cytochrome P-450 2D6 polymorphisms. Malar. J. 17, 42. doi: 10.1186/s12936-018-2190-z
Botnar, A., Lawrence, G., Maher, S. P., Vantaux, A., Witkowski, B., Shiau, J. C., et al. (2022). Alkyne modified purines for assessment of activation of Plasmodium vivax hypnozoites and growth of pre-erythrocytic and erythrocytic stages in Plasmodium spp. Int. J. Parasitol. doi: 10.1016/j.ijpara.2022.03.003 [Epub ahead of print].
Bousema, T., and Drakeley, C. (2011). Epidemiology and infectivity of Plasmodium falciparum and Plasmodium vivax gametocytes in relation to malaria control and elimination. Clin. Microbiol. Rev. 24, 377–410. doi: 10.1128/CMR.00051-10
Boyd, M. F. (1940). On strains or races of the malaria parasites. Am. J. Trop. Med. s1-20, 69–80. doi: 10.4269/ajtmh.1940.s1-20.69
Boyd, M. F., and Kitchen, S. F. (1937). Recurring clinical activity in infections with the McCoy strain of Plasmodium vivax. Am. J. Trop. Med. s1-17, 833–843.
Boyd, M. F., and Kitchen, S. F. (1948). On the homogeneity or heterogeneity of Plasmodium vivax infections acquired in highly endemic regions. Am. J. Trop. Med. s1-28, 29–34. doi: 10.4269/ajtmh.1948.s1-28.29
Bright, A. T., Manary, M. J., Tewhey, R., Arango, E. M., Wang, T., Schork, N. J., et al. (2014). A high resolution case study of a patient with recurrent Plasmodium vivax infections shows that relapses were caused by meiotic siblings. PLoS Negl. Trop. Dis. 8, e2882. doi: 10.1371/journal.pntd.0002882
Bruce, M. C., and Day, K. P. (2002). Cross-species regulation of malaria parasitaemia in the human host. Curr. Opin. Microbiol. 5, 431–437. doi: 10.1016/S1369-5274(02)00348-X
Commons, R. J., Simpson, J. A., Thriemer, K., Hossain, M. S., Douglas, N. M., Humphreys, G. S., et al. (2019). Risk of plasmodium vivax parasitaemia after Plasmodium falciparum infection: a systematic review and meta-analysis. Lancet Infect. Dis. 19, 91–101. doi: 10.1016/S1473-3099(18)30596-6
Cooper, W. C., Myatt, A. V., Hernandez, T., Jeffery, G. M., and Coatney, G. R. (1953). Studies in human malaria: XXXI. Comparison of Primaquine, Isopentaquine, SN-3883, and Pamaquine as curative agents against Chesson strain vivax malaria. Am. J. Trop. Med. Hyg. 2, 949–957. doi: 10.4269/ajtmh.1953.2.949
Dembele, L., Franetich, J. F., Lorthiois, A., Gego, A., Zeeman, A. M., Kocken, C. H., et al. (2014). Persistence and activation of malaria hypnozoites in long-term primary hepatocyte cultures. Nat. Med. 20, 307–312. doi: 10.1038/nm.3461
Dundas, K., Shears, M. J., Sinnis, P., and Wright, G. J. (2019). Important extracellular interactions between Plasmodium Sporozoites and host cells required for infection. Trends Parasitol. 35, 129–139. doi: 10.1016/j.pt.2018.11.008
Edgcomb, J. H., Arnold, J., Yount, E. H., Jr Alving, A. S., Eichelberger, L., Jeffery, G. M., et al. (1950). Primaquine, SN 13272, a new curative agent in vivax malaria; a preliminary report. J. Natl. Malar. Soc. 9, 285–292.
Ehrman, F. C., Ellis, J. M., and Young, M. D. (1945). Plasmodium vivax Chesson strain. Science 101, 377. doi: 10.1126/science.101.2624.377.a
Garrison, P. L., Hankey, D. D., Coker, W. G., Donovan, W. N., Jastremski, B., Coatney, G. R., et al. (1952). Cure of Korean vivax malaria with pamaquine and primaquine. J. Am. Med. Assoc. 149, 1562–1563. doi: 10.1001/jama.1952.72930340021010a
Gupta, D. K., Dembele, L., Voorberg-van der Wel, A., Roma, G., Yip, A., Chuenchob, V., et al. (2019). The Plasmodium liver-specific protein 2 (LISP2) is an early marker of liver stage development. elife 8, e43362. doi: 10.7554/eLife.43362
Gural, N., Mancio-Silva, L., Miller, A. B., Galstian, A., Butty, V. L., Levine, S. S., et al. (2018). In vitro culture, drug sensitivity, and Transcriptome of Plasmodium vivax Hypnozoites. Cell Host Microbe 23, 395–406.e4. doi: 10.1016/j.chom.2018.01.002
Huldén, L., and Heliövaara, K. (2008). Natural relapses in vivax malaria induced by Anopheles mosquitoes. Malar. J. 7, 64. doi: 10.1186/1475-2875-7-64
Hulden, L., and Hulden, L. (2011). Activation of the hypnozoite: a part of Plasmodium vivax life cycle and survival. Malar. J. 10, 90. doi: 10.1186/1475-2875-10-90
Imwong, M., Boel, M. E., Pagornrat, W., Pimanpanarak, M., McGready, R., Day, N. P., et al. (2012). The first Plasmodium vivax relapses of life are usually genetically homologous. J. Infect. Dis. 205, 680–683. doi: 10.1093/infdis/jir806
Joyner, C., Barnwell, J. W., and Galinski, M. R. (2015). No more monkeying around: primate malaria model systems are key to understanding Plasmodium vivax liver-stage biology, hypnozoites, and relapses. Front. Microbiol. 6, 145. doi: 10.3389/fmicb.2015.00145
Kaushansky, A., and Kappe, S. H. (2015). Selection and refinement: the malaria parasite's infection and exploitation of host hepatocytes. Curr. Opin. Microbiol. 26, 71–78. doi: 10.1016/j.mib.2015.05.013
Kaushansky, A., Metzger, P. G., Douglass, A. N., Mikolajczak, S. A., Lakshmanan, V., Kain, H. S., et al. (2013). Malaria parasite liver stages render host hepatocytes susceptible to mitochondria-initiated apoptosis. Cell Death Dis. 4, e762. doi: 10.1038/cddis.2013.286
Maher, S. P., Vantaux, A., Chaumeau, V., Chua, A. C. Y., Cooper, C. A., Andolina, C., et al. (2021a). Probing the distinct chemosensitivity of Plasmodium vivax liver stage parasites and demonstration of 8-aminoquinoline radical cure activity in vitro. Sci. Rep. 11, 19905. doi: 10.1038/s41598-021-99152-9
Maher, S. P., Vantaux, A., Cooper, C. A., Chasen, N. M., Cheng, W. T., Joyner, C. J., et al. (2021b). A phenotypic screen for the liver stages of Plasmodium vivax. Bio-protocol. 11:e4253. doi: 10.21769/BioProtoc.4253
Manzoni, G., Marinach, C., Topçu, S., Briquet, S., Grand, M., Tolle, M., et al. (2017). Plasmodium P36 determines host cell receptor usage during sporozoite invasion. elife 6:e25903. doi: 10.7554/eLife.25903
Metcalf, C. J. E., Graham, A. L., Huijben, S., Barclay, V. C., Long, G. H., Grenfell, B. T., et al. (2011). Partitioning regulatory mechanisms of within-host malaria dynamics using the effective propagation number. Science 333, 984–988. doi: 10.1126/science.1204588
Mikolajczak, S. A., Vaughan, A. M., Kangwanrangsan, N., Roobsoong, W., Fishbaugher, M., Yimamnuaychok, N., et al. (2015). Plasmodium vivax liver stage development and hypnozoite persistence in human liver-chimeric mice. Cell Host Microbe 17, 526–535. doi: 10.1016/j.chom.2015.02.011
Mueller, I., Galinski, M. R., Baird, J. K., Carlton, J. M., Kochar, D. K., Alonso, P. L., et al. (2009). Key gaps in the knowledge of Plasmodium vivax, a neglected human malaria parasite. Lancet Infect. Dis. 9, 555–566. doi: 10.1016/S1473-3099(09)70177-X
Niklaus, L., Agop-Nersesian, C., Schmuckli-Maurer, J., Wacker, R., Grünig, V., and Heussler, V. T. (2019). Deciphering host lysosome-mediated elimination of Plasmodium berghei liver stage parasites. Sci. Rep. 9, 7967. doi: 10.1038/s41598-019-44449-z
Posfai, D., Maher, S. P., Roesch, C., Vantaux, A., Sylvester, K., Péneau, J., et al. (2020). Plasmodium vivax liver and blood stages recruit the druggable host membrane channel aquaporin-3. Cell Chem. Biol. 27, 719–727.e5. doi: 10.1016/j.chembiol.2020.03.009
R Core Team. (2020). A Language and Environment for Statistical Computing. Vienna: R Foundation for Statistical Computing.
Raberg, L., de Roode, J. C., Bell, A. S., Stamou, P., Gray, D., and Read, A. F. (2006). The role of immune-mediated apparent competition in genetically diverse malaria infections. Am. Nat. 168, 41–53. doi: 10.1086/505160
Read, A. F., and Taylor, L. H. (2001). The ecology of genetically diverse infections. Science 292, 1099–1102. doi: 10.1126/science.1059410
Reece, S. E., Drew, D. R., and Gardner, A. (2008). Sex ratio adjustment and kin discrimination in malaria parasites. Nature 453, 609–614. doi: 10.1038/nature06954
Roth, A., Maher, S. P., Conway, A. J., Ubalee, R., Chaumeau, V., Andolina, C., et al. (2018). A comprehensive model for assessment of liver stage therapies targeting Plasmodium vivax and Plasmodium falciparum. Nat. Commun. 9, 1837. Epub 2018/05/11. doi: 10.1038/s41467-018-04221-9
Ruberto, A. A., Bourke, C., Merienne, N., Obadia, T., Amino, R., and Mueller, I. (2021a). Single-cell RNA sequencing reveals develop mental heterogeneity among Plasmodium berghei sporozoites. Sci. Rep. 11, 4127. doi: 10.1038/s41598-021-82914-w
Ruberto, A. A., Bourke, C., Vantaux, A., Maher, S. P., Jex, A., Witkowski, B., et al. (2021b). Single-cell RNA sequencing of Plasmodium vivax sporozoites reveals stage- and species-specific transcriptomic signatures. PLoS Negl. Trop Dis. 16:e0010633. doi: 10.1371/journal.pntd.0010633
Ruberto, A. A., Maher, S. P., Vantaux, A., Joyner, C. J., Bourke, C., Balan, B., et al. (2022). Single-cell RNA profiling of Plasmodium vivax-infected hepatocytes reveals parasite- and host- specific transcriptomic signatures and therapeutic targets. Front. Cell Infect. Microbiol. 12:986314. doi: 10.3389/fcimb.2022.986314
Schafer, C., Dambrauskas, N., Steel, R. W., Carbonetti, S., Chuenchob, V., Flannery, E. L., et al. (2018). A recombinant antibody against Plasmodium vivax UIS4 for distinguishing replicating from dormant liver stages. Malar. J. 17, 370. doi: 10.1186/s12936-018-2519-7
Schäfer, C., Roobsoong, W., Kangwanrangsan, N., Bardelli, M., Rawlinson, T. A., Dambrauskas, N., et al. (2020). A humanized mouse model for Plasmodium vivax to test interventions that block liver stage to blood stage transition and blood stage infection. iScience 23, 101381. doi: 10.1016/j.isci.2020.101381
Schäfer, C., Zanghi, G., Vaughan, A. M., and Kappe, S. H. I. (2021). Plasmodium vivax latent liver stage infection and relapse: biological insights and new experimental tools. Annu. Rev. Microbiol. 75, 87–106. doi: 10.1146/annurev-micro-032421-061155
Schwartz, R., Mansuy, M. M., and John, W. C. (1950). Current concepts of malaria: with a review of five hundred and ten admissions to a veterans hospital. A.M.A. Arch. Intern. Med. 86, 837–856. doi: 10.1001/archinte.1950.00230180042004
Shanks, G. D., and White, N. J. (2013). The activation of vivax malaria hypnozoites by infectious diseases. Lancet Infect. Dis. 13, 900–906. doi: 10.1016/S1473-3099(13)70095-1
Sinnis, P., and Coppi, A. (2007). A long and winding road: the Plasmodium sporozoite's journey in the mammalian host. Parasitol. Int. 56, 171–178. doi: 10.1016/j.parint.2007.04.002
Taylor, L. H., Walliker, D., and Read, A. F. (1997). Mixed-genotype infections of the rodent malaria Plasmodium chabaudi are more infectious to mosquitoes than single-genotype infections. Parasitology 115, 121–132. doi: 10.1017/S0031182097001145
Thomas, S., Ravishankaran, S., Justin, N., Asokan, A., Kalsingh, T. M. J., Mathai, M. T., et al. (2018). Microclimate variables of the ambient environment deliver the actual estimates of the extrinsic incubation period of Plasmodium vivax and Plasmodium falciparum: a study from a malaria-endemic urban setting, Chennai in India. Malar. J. 17, 201. doi: 10.1186/s12936-018-2342-1
White, N. J. (2011). Determinants of relapse periodicity in Plasmodium vivax malaria. Malar. J. 10, 297. doi: 10.1186/1475-2875-10-297
White, M. T., Karl, S., Battle, K. E., Hay, S. I., Mueller, I., and Ghani, A. C. (2014). Modelling the contribution of the hypnozoite reservoir to Plasmodium vivax transmission. elife 3:e04692. doi: 10.7554/eLife.04692
World Health Organization. (1975). WHO Manual on Practical Entomology in Malaria, Part II. Geneva, Switzerland.
Keywords: Plasmodium vivax, malaria, hypnozoite, hepatocyte, relapse
Citation: Vantaux A, Péneau J, Cooper CA, Kyle DE, Witkowski B and Maher SP (2022) Liver-stage fate determination in Plasmodium vivax parasites: Characterization of schizont growth and hypnozoite fating from patient isolates. Front. Microbiol. 13:976606. doi: 10.3389/fmicb.2022.976606
Edited by:
Abhisheka Bansal, Jawaharlal Nehru University, IndiaReviewed by:
Dennis Shanks, The University of Queensland, AustraliaTejram Sahu, Johns Hopkins University, United States
Melanie Shears, University of Washington, United States
Copyright © 2022 Vantaux, Péneau, Cooper, Kyle, Witkowski and Maher. This is an open-access article distributed under the terms of the Creative Commons Attribution License (CC BY). The use, distribution or reproduction in other forums is permitted, provided the original author(s) and the copyright owner(s) are credited and that the original publication in this journal is cited, in accordance with accepted academic practice. No use, distribution or reproduction is permitted which does not comply with these terms.
*Correspondence: Amélie Vantaux, YW1lbGllLnZhbnRhdXhAZ21haWwuY29t; Steven P. Maher, c3RldmVuLm1haGVyQHVnYS5lZHU=