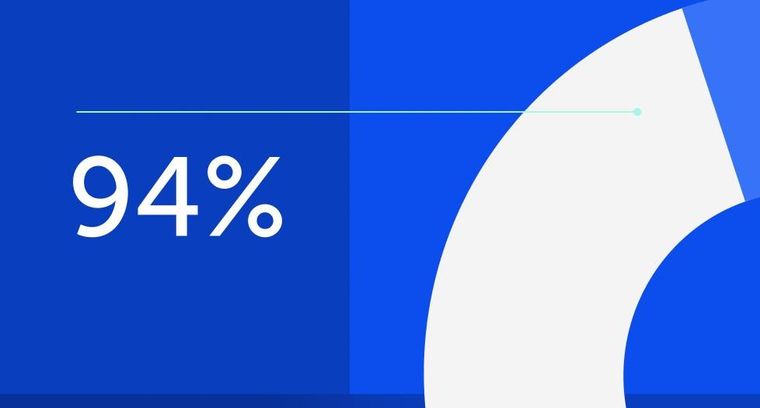
94% of researchers rate our articles as excellent or good
Learn more about the work of our research integrity team to safeguard the quality of each article we publish.
Find out more
REVIEW article
Front. Microbiol., 31 August 2022
Sec. Antimicrobials, Resistance and Chemotherapy
Volume 13 - 2022 | https://doi.org/10.3389/fmicb.2022.974984
Staphylococcus aureus (S. aureus) infections are often difficult to cure completely. One of the main reasons for this difficulty is that S. aureus can be internalized into cells after infecting tissue. Because conventional antibiotics and immune cells have difficulty entering cells, the bacteria can survive long enough to cause recurrent infections, which poses a serious burden in healthcare settings because repeated infections drastically increase treatment costs. Therefore, preventing and treating S. aureus internalization is becoming a research hotspot. S. aureus internalization can essentially be divided into three phases: (1) S. aureus binds to the extracellular matrix (ECM), (2) fibronectin (Fn) receptors mediate S. aureus internalization into cells, and (3) intracellular S. aureus and persistence into cells. Different phases require different treatments. Many studies have reported on different treatments at different phases of bacterial infection. In the first and second phases, the latest research results show that the cell wall-anchored protein vaccine and some microbial agents can inhibit the adhesion of S. aureus to host cells. In the third phase, nanoparticles, photochemical internalization (PCI), cell-penetrating peptides (CPPs), antimicrobial peptides (AMPs), and bacteriophage therapy can effectively eliminate bacteria from cells. In this paper, the recent progress in the infection process and the prevention and treatment of S. aureus internalization is summarized by reviewing a large number of studies.
S. aureus is a significant human pathogen that causes a variety of clinical illnesses. It is a prominent cause of bacteremia, infective endocarditis, osteoarthritis, skin and soft tissue infections, pleuropulmonary infections, and device-related infections (Tong et al., 2015). S. aureus infection has a significant socioeconomic impact in both developed and poor countries (Balasubramanian et al., 2017). For instance, a thorough analysis of skin and soft tissue infections between 2001 and 2009 revealed that hospitalized patients’ treatment expenditures in the United States ranged from $12,000 to $23,000 (Suaya et al., 2014). Sometimes, it is difficult to cure these infections completely, especially when they become chronic. The internalization of S. aureus by cells and bacterial biofilms is the major cause of persistent and difficult-to-treat infections (Richter et al., 2016). Recently, some studies have summarized the formation of and treatment strategies for biofilms in detail (Bhattacharya et al., 2015; Moormeier and Bayles, 2017; Suresh et al., 2019), and this paper will not elaborate any further on that topic.
There is growing evidence that S. aureus has the ability to invade and persist within eukaryotic cells. S. aureus has been found in many non-phagocytic cells, such as human osteoblast cell lines, normal chick osteoblasts, mouse fibroblasts, mouse renal cells, bovine mammary epithelial cells, and human bronchial epithelial cells (Alexander and Hudson, 2001; Richter et al., 2016). S. aureus intracellularity has been proposed as an immune-evasive strategy to avoid identification by professional phagocytes (Fraunholz and Sinha, 2012). One of the most challenging aspects of treating intracellular S. aureus infections is getting enough antibacterial medicines to the intracellular bacteria. Many antibiotics have limited cell membrane penetration (β-lactams and aminoglycosides), intracellular unabiding retention (fluoroquinolones and macrolides), insufficient intracellular distribution, and low intracellular concentration (Zhou et al., 2018). Therefore, there is an urgent demand to develop novel therapies to treat intracellular S. aureus infections. The process of S. aureus internalization can essentially be divided into three phases (Figure 1): (i) S. aureus binds to the extracellular matrix (ECM), (ii) fibronectin (Fn) receptors mediate S. aureus internalization into cells, and (iii) intracellular S. aureus and persistence into cells (Wen et al., 2020). We will use osteoblasts as an example to illustrate the process of S. aureus internalization.
Figure 1. The process of S. aureus internalization into cells and the potential treatments. The process of S. aureus internalization can essentially be divided into three phases: (1) S. aureus binds to the extracellular matrix (ECM), (2) fibronectin (Fn) receptors mediate S. aureus internalization into cells, and (3) intracellular S. aureus and persistence into osteoblasts. In addition to binding to the ECM, S. aureus can also directly stimulate osteoblasts through its PAMPs, resulting in a variety of cellular reactions. In the first and second phases, immunotherapy and some microbial agents can effectively interfere with bacterial adhesion. Besides, some agents could enhance the antimicrobial defense of the host cells by inducing the production of antimicrobial peptides and decreasing the secretion of inflammatory cytokines. In the third phase, some treatments such as nanoparticles, photochemical internalization, cell-penetrating peptides, antimicrobial peptides, and bacteriophage therapy have significant bactericidal effects against intracellular S. aureus.
S. aureus’s ability to infect bone, and more especially the osteoblast, is closely tied to its ability to bind the bone extracellular matrix (BEM) components (Heilmann, 2011). All of the proteins and glycans in the BEM are possible S. aureus binding sites. The extracellular matrix can foster the accumulation of S. aureus near osteoblasts. I collagen (Cn), bone sialoprotein, osteopontin, and fibronectin are the most studied because they interact directly with S. aureus (Josse et al., 2015). S. aureus binds to the BEM through its cell wall-anchored (CWA) proteins while contacting bone tissue in preparation for subsequent internalization. Cn represents approximately 90–95% of the organic fraction of the BEM, and the Cn adhesin (Cna) protein on the surface of S. aureus plays a function by adhering to Cn in the process of S. aureus infection in bone tissue (Patti et al., 1994). The N-terminal A domain (containing of N1, N2, and N3), the B repetitive sequence, the cell wall anchoring domain, and a brief cytoplasmic region comprise Cna proteins (Zong et al., 2005; Herman-Bausier et al., 2016). Cna primarily binds to Cn via a tightly wrapped mechanism (the “collagen hug” mechanism) (Herman-Bausier et al., 2016). The N1 and N2 domains of the N-terminal A domain (which has an IgG fold-like structure) and the B repetitive sequence are now known to be involved in this mechanism. S. aureus proteinaceous surface adhesins can be covalently attached to the cell wall peptidoglycan or surface-associated via various mechanisms such as ionic or hydrophobic interactions (Heilmann, 2011).
The capacity of fibronectin to bind with α5β1 integrin is now thought to be the most prevalent mechanism for S. aureus internalization in endothelial cells and osteoblasts (Khalil et al., 2007).
Fibronectin functions as a bridge between S. aureus and osteoblasts. On the one hand, S. aureus has two proteins on its surface that may bind to fibronectin: fibronectin-binding proteins A and B (FnBP A/B). Fibronectin, on the other hand, binds to osteoblasts via the α5β1 integrin (Hauck and Ohlsen, 2006). This “Fn bridge” enables S. aureus to enter osteoblasts via internalization. S. aureus mutants lacking FnBPs have trouble internalizing in host osteoblasts (Ahmed et al., 2001). Besides, cells missing the integrin β1 subunit do not internalize S. aureus in substantial numbers, highlighting the critical function of integrin α5β1 for the uptake process (Fowler et al., 2000). FnBPs bind to Fn via a tandem β-zipper structure, which causes a conformational shift in Fn, exposing a cryptic integrin-binding site in Fn, which then interacts with the α5β1 integrin with great affinity (Prystopiuk et al., 2018). Fn creates mechanically strong bridges between FnBPAs on the surface of S. aureus and purified integrins that can sustain substantially higher stresses (∼800 pN) than the conventional Fn-α5β1 integrin interaction (∼100 pN). This great mechanical stability lends itself to an invasion model in which binding of Fn to FnBPA via a -zipper results in force-induced unfolding and allosteric activation of FnIII domains. This exposes hidden integrin-binding sites, which engage in a robust, high-affinity contact with integrins (Liang et al., 2016). The local recruitment of structural proteins like tensin, vinculin, and zyxin as well as signaling enzymes like Src family protein tyrosine kinases (PTKs) and focal adhesion kinase (FAK) at the site of bacterial attachment is caused by bacteria-induced clustering of integrins. Multiple downstream effectors, including cortactin, are tyrosine phosphorylated as a result of FAK and Src’s joint activity. The effect of cortactin on cytoskeleton rearrangements via the Arp2/3 complex or the control of endocytosis by dynamin is most likely how it functions in the internalization of bacteria (Hauck and Ohlsen, 2006).
In addition to binding to the BEM, S. aureus can also directly stimulate osteoblasts through its pathogen-associated molecular patterns (PAMPs), resulting in a variety of cellular reactions. PAMPs can bind to pattern recognition receptors (PRR) on osteoblasts, such as toll-like receptors (TLR) and the tumor necrosis factor receptor 1 (TNFR-1). TLRs are a family of 13 mammalian members, each of which mediates an intrinsic signaling pathway and induces specific biological responses against microorganisms (Uematsu and Akira, 2006). TLR-2, TLR-4, and TLR-5 have been observed in osteoblasts. For S. aureus infections, TLR2, which can induce the release of antimicrobial peptides (AMPs) (Varoga et al., 2009), is an important relevant receptor involved in this process (Fournier, 2012). TLR2 can recognize ligands with a wide range of structural variations, including proteins, glycopolymers, peptidoglycans, lipoarabinomannan, and lipoproteins/lipopeptides (Oliveira-Nascimento et al., 2012). There is increasing evidence that lipoproteins play an important role in TLR2 activation by staphylococci (Fournier, 2012). TLR-4 and TLR-5 participate in responses against gram-negative bacteria by recognizing lipopolysaccharide and flagellin, respectively, but are not involved in the interaction between S. aureus and osteoblasts (Madrazo et al., 2003). According to current research, apart from PRRs like TLRs, S. aureus can also interact with epithelial cells and osteoblasts through the extracellular TNFR-1 receptor (Gomez et al., 2004; Claro et al., 2013). TNFR-1 interacts with the protein A of S. aureus, which can result in the production of cytokines, osteoblast death, or an imbalanced bone homeostasis (Claro et al., 2011, 2013). Besides, the iron-regulated surface determinant-B (IsdB) of S. aureus was involved in invasion, and IsdB most likely interacts with integrins that bind ligands with the RGD motif (Zapotoczna et al., 2013); however, the endocytic pathway has not been discovered.
In bone infection, S. aureus internalization in osteoblasts is crucial. Intracellular persistence process depends on the total number of infected cells (Bongiorno et al., 2020). This transformation from acute to chronic bone infection indicates the initiation of persistent infection caused by survival bacteria in cells. S. aureus can survive in osteoblasts following internalization due to two factors (Figure 1): vesicle escape and small-colony variant (SCV) formation. The co-localization of fluorescent intracellular S. aureus and a lysosomal-associated membrane marker in osteoblasts revealed the trafficking of live bacteria into late endosomal/lysosomal vesicles, implying that S. aureus survives inside the vesicles (Jauregui et al., 2013). The survival and proliferation of S. aureus within cells were via preventing combination of phagosome and lysosome, subversion autophagy, and others. A recent study shows that internalization of S. aureus is higher in macrophages than in osteoblasts, but the proportion of S. aureus that survives in osteoblasts is higher than that in macrophages (Hamza and Li, 2014). This disparity results from non-professional phagocytes’ incapacity to remove bacteria from vesicles, allowing S. aureus to persist in osteoblasts for long time (Hamza and Li, 2014).
At the same time, these surviving bacteria also transform into SCVs. The SCVs are a slow-growing bacterial subpopulation with abnormal colony shape on agar plates and atypical metabolic properties, which are related to the resistance of S. aureus, reinfection, and chronic infections. Such SCVs were produced mostly as a result of antibiotic-induced or spontaneous mutations in particular metabolic genes such as har, hemB, ctaA, and thyA (Chen et al., 2021). Approximately 70% of patients who had long-term antibiotic treatment have S. aureus SCV infection (Melter and Radojevic, 2010). SCVs have higher intracellular persistence and lower antibiotic susceptibility than wild-type bacteria (Tuchscherr et al., 2016). In vitro exposure to various antibiotics has been demonstrated to produce S. aureus SCVs from their parental strains (Zhang G. et al., 2018). The membrane potential of the SCV is lowered, which indirectly lowers the bactericidal efficacy of antimicrobial drugs, because transmembrane potential is necessary for the uptake of positively charged AMPs and antibiotics. In addition, the cell membrane is hydrophobic, yet most antibiotics are hydrophilic, making antibiotic entry into the cell problematic and allowing bacteria to evade the activity of most antibiotics. Furthermore, after escaping the original cells and infecting new cells, SCVs quickly revert to the wild-type, extremely toxic, invasive phenotype, which explains why chronic osteomyelitis patients suffer recurring infections.
The first and second phases of treatment focus on killing off free bacteria and preventing their adhesion and internalization into cells. Adhesion and internalization are immediate events. However, given the good bactericidal effect of conventional antibiotics on free S. aureus, the current research focuses on preventing the adhesion and internalization of bacteria. S. aureus adhesion to the ECM is achieved mainly by its cell wall-anchored (CWA) proteins. CWA proteins are essential virulence factors for the survival of S. aureus in the commensal state and during invasive infections. CWA proteins have a variety of functions, including adhesion and invasion of host cells and tissues, escape from immune responses, and biofilm formation (Foster et al., 2014). Therefore, CWA proteins have attracted much attention as therapeutic targets. Moreover, some microbiological agents have been shown to inhibit the adhesion of S. aureus. Because the bacteria are free in the first phase, traditional antibiotic use is also necessary.
Recombinant CWA proteins were recently used as the specific vaccines against S. aureus infection mainly through antibody-mediated protective immunity (Foster et al., 2014). After being injected into the body, the recombinant CWA protein causes the body to produce antibodies that attack specific CWA proteins on S. aureus, thereby inhibiting their function. Many specific vaccines have been developed against various CWA proteins, but most have not yet been subjected to clinical testing. Clumping factor A (ClfA), which is produced by most clinical isolates of S. aureus, has been regarded as an important vaccine candidate (Scully et al., 2015; Li et al., 2016). It has been shown to provide partial protection against S. aureus infections including lethal bloodstream infections and septic arthritis (Josefsson et al., 2001; Scully et al., 2015; Schneewind and Missiakas, 2019). Similarly, the corresponding vaccines that target clumping factor B (ClfB), or S. aureus surface protein X (SasX) have all demonstrated certain abilities to reduce infectivity and/or colonization by S. aureus (Liu et al., 2015; Lacey et al., 2019).
However, given the myriad of virulence factors produced by the pathogen, the effect of combinations of CWA antigens is superior to that of a single antigen (Delfani et al., 2016), which supports the use of a combination of antigens in vaccines for future clinical trials (Foster et al., 2014). Yang L. et al. (2016) designed the novel chimeric vaccine IsdB151–277ClfA33–213 (IC), which is based on the immune-dominant areas of the iron surface determinant B (IsdB) and ClfA. This vaccine induced higher protection in an S. aureus sepsis model compared with the single components alone and showed broad immune protection against several clinical S. aureus isolates. A surface protein vaccine (containing ClfA, fibrinogen binding protein B, serine-aspartate repeat D, and SpA) raised antigen-specific immune responses that protected leukopenic mice against S. aureus bloodstream infections (Rauch et al., 2014). Whereas, some active immunization techniques have not yet proven effective in humans. In a Phase III experiment, an IsdB vaccine that was protective in animals failed to protect patients from major infections following cardiothoracic surgery for unknown reasons (Fowler et al., 2013). There are some possible reasons for the negative findings of clinical trials. Preclinical findings with antigens evaluated in clinical trials were probably exaggerated by vaccine producers. Furthermore, because all human S. aureus vaccines developed to date have only targeted one antigen, they are unlikely to protect against complex bacterial infections. Finally, new generation adjuvants, which may be essential in boosting antibody formation and guiding the T-cell response toward the proper profile of cytokine release, were not present in the vaccines (Bagnoli et al., 2012).
Other monoclonal antibodies have also shown good antimicrobial effects. Yang Y. et al. (2016) demonstrated that 2H7, a protective monoclonal antibody targeting the conserved domain of S. aureus surface protein A (SasA), could recognize wild-type S. aureus and promote the opsonophagocytic killing of S. aureus. Besides, Tkaczyk et al. (2016) tested a monoclonal antibody combination targeting alpha toxin (AT) and ClfA that neutralized AT-mediated cytotoxicity, blocked fibrinogen binding by ClfA, prevented bacterial agglutination, targeted the bacteria for opsonophagocytic killing, and provided broad isolate coverage in a lethal-bacteremia mode.
Recently, some microbiological agents have shown excellent effects in inhibiting the invasion of S. aureus. Bouchard et al. (2013) demonstrated that Lactobacillus casei reduced S. aureus Newbould 305 and RF122 internalization by 60–80% without modifying cell viability and morphology. An extracellular anti-inflammatory drug, serratiopeptidase, can reduce the invasion and internalization of S. aureus to osteoblasts by 75% (Papa et al., 2013; Selan et al., 2017). In an in vitro and in vivo experiment, Wang X. et al. (2018) found that the plectasin derivatives MP1102/NZ2114 had a good effect on intracellular S. aureus clearance. The inhibition of S. aureus internalization by microbiological agents likely involves such means: (a) direct effect on S. aureus, including coaggregation, as observed for vaginal lactobacilli (Younes et al., 2012); (b) inhibition of S. aureus virulence expression, including major virulence regulators (Papa et al., 2013).
Some agents could enhance the antimicrobial defense of the host cells by inducing the production of AMPs and decreasing the secretion of inflammatory cytokines. Sodium butyrate has been shown to increase the expression of tracheal antimicrobial peptide (TAP), β-defensin, and inducible nitric oxide synthase (iNOS) mRNAs in bovine mammary epithelial cells (bMECs), as well as the production of nitric oxide (Ochoa-Zarzosa et al., 2009). Short-chain fatty acids (propionic and hexanoic) have similar actions. Propionic and hexanoic reduced bacterial internalization into bMECs, which ranged 27–55 and 39–65%, respectively. And they up-regulated TAP mRNA expression; however, bovine neutrophil β-defensin 5 (BNBD5) mRNA expression was not modified or was down-regulated (Alva-Murillo et al., 2012). Besides, bMECs treated with 17β-Estradiol (E2) (50 pg/mL, 24 h) reduced S. aureus internalization (∼50%). E2 also decreased the secretion of TNF-α and IL-1β as well as IL-6 production. Furthermore, E2 also increased the expression of AMPs DEFB1, BNBD5, and psoriasin S100A7 (Medina-Estrada et al., 2016).
After S. aureus adheres to the cell surface, it begins to be internalized into the host cell. The S. aureus internalization was an active process that was mainly mediated by the FnBPs and integrin α5β1 of host (Sinha et al., 2000; Hauck and Ohlsen, 2006; Alva-Murillo et al., 2014) cells. There are two main results after S. aureus invades host cells: (a) virulence factors produced by the bacteria or the inflammatory reaction induced by the bacteria cause most host cells to rapidly lyse and die; and (b) some S. aureus bacteria transform from the wild type into a less toxic small colony variant type and live in host cells for a long time (Proctor et al., 2014). At present, more research is aimed at this phase of treatment, and many types of treatments have been demonstrated to have a good bactericidal effect on intracellular S. aureus.
The effectiveness of nanoparticles loaded with antibiotics to treat bacterial infections has been studied for many years due to their excellent characteristics including their nano size, surface charge, and large specific surface area. Nanoparticles have contributed to great progress in research on antibacterial biofilms, and they can effectively penetrate thick biofilms and bacterial membranes and disrupt these membranes to kill bacteria (Mu et al., 2016; Mihu et al., 2017). Furthermore, because nanoparticles can effectively penetrate the cell membrane and improve the concentration and bactericidal activity of antibiotics in cells, the field of nanotechnology provides novel approaches for tackling internalized S. aureus (Maya et al., 2012; Mu et al., 2016). Given the different types of nanoparticle carriers, the corresponding bactericidal effect is not the same.
Nanoparticles can improve the permeability and accumulation of their payload drug within cells. Increased cellular uptake and the subsequent controlled release of the nanoparticle-adsorbed antibiotics can effectively enhance their antibacterial effects, which makes them more effective for treating intracellular infection (Zhou et al., 2018). Silver nanoparticles (AgNPs) and their combination with antibiotics have demonstrated high extracellular and intracellular bacterial killing abilities and present unique aspects for potential clinical applications (Kang et al., 2019). Maya et al. (2012) developed biocompatible, 200-nm-sized tetracycline-encapsulated O-carboxymethyl chitosan nanoparticles (Tet-O-CMC Nps). These Tet-O-CMC Nps were capable of delivering Tet intracellularly and showed a sixfold increase in antibacterial activity over that of free Tet against intracellular S. aureus. To improve the efficiency of delivery and target specificity, Yang Y. et al. (2016) reported a unique intracellular antibiotic delivery nanoparticle that is composed of (a) a mesoporous silica nanoparticle core loaded with gentamicin, (b) an infected microenvironment (bacterial toxin)-responsive lipid bilayer surface shell, and (c) the bacteria-targeting peptide ubiquicidin (UBI29.41), which is immobilized on the lipid bilayer shell surface. BI29.41 showed high sensitivity, specificity, and accuracy for detecting bacterial infection.
Due to their unique biologic performance, nanoparticles armed with antimicrobial agents are used as a potential weapon against S. aureus infection and have demonstrated more advantages than the conventional preparations. However, study on nanosystems’ ability to treat S. aureus infections is still ongoing, and we must contend with issues like reasonable large-scale production and the premature release of nanoparticles. Besides, there are currently few nanoparticles designed to treat the SCV phenotype of S. aureus. New approaches for the production of nanoparticles should be established in order to eradicate the SCV phenotype (Zhou et al., 2018).
Photochemical internalization (PCI) is a physical-targeting technique in which amphoteric photosensitizers located in endocytic vesicles undergo a series of chemical reactions based on light excitation at a specific wavelength. This in turn causes the vesicle to burst, and the large molecules in the vesicle are released into the cytoplasm. PCI has been shown to facilitate entry into the cytoplasm of most large molecules, including nucleic acids and proteins, and other molecules that cannot easily penetrate cell membranes (Jerjes et al., 2020a,b; Sosic et al., 2020).
Zhang X. et al. (2018) demonstrated for the first time that PCI can effectively enhance the cytosolic release of antibiotics from endocytic vesicles after internalization, thus providing a good clearance effect of intracellular S. aureus. First, antibiotics, together with photosensitizers, are internalized through endocytosis or phagocytosis. This process encases the internal antibiotics in vesicles. The photosensitizer is localized in the membrane of the intracellular vesicle, and the drug may be isolated inside the vesicle. Upon illumination, these photosensitizer-bound membranes are disrupted, causing the drugs to be released from the vesicles into the cytoplasm and allowing them to reach their intracellular targets. Thus, with PCI, a lower antibiotic dose can be used for treating (intracellular) staphylococcal infection. Whereas, there are relatively few studies about PCI for treating intracellular S. aureus at present, and more research is needed in the future to overcome issues such as PCI toxicity.
CPPs are a family of various peptides, typically comprising 5–30 amino acids, that can pass through tissue and cell membranes (Guidotti et al., 2017). These peptides have been extensively shown to be capable of transporting a wide variety of biologically active conjugates (cargoes) into cells including proteins, peptides, DNAs, siRNAs, and small drugs, and thus they are considered efficient drug delivery vehicles (Mahmood et al., 2016). Cargoes can be conjugated to CPPs either by covalent bonds or by non-covalent complex formation (Guidotti et al., 2017). The mechanism of how CPPs transport cargoes from outside the cell to inside the cell has been extensively studied in recent years, but it is still not completely clear. Nonetheless, these mechanisms of entry can be roughly divided into two categories: energy-independent direct penetration of the plasma membrane and energy-dependent endocytosis; most CPPs and CPP–cargo conjugates enter cells via endocytosis (Guidotti et al., 2017). However, precisely determining the transmembrane pathway of a CPP is not that simple. Even for the same CPP, the transmembrane efficiency and pathway may be affected by the concentration, temperature, and cell type (Fretz et al., 2007).
Randhawa et al. (2016) investigated the effects of two CPPs (P3 and P8) in combination with four antibiotics (viz. oxacillin, erythromycin, norfloxacin, and vancomycin) against MRSA strains. They found that this combination of CPPs and antibiotics showed high toxicity against MRSA compared with antibiotics alone. In addition to binding with antibiotics, CCIs can cooperate with some molecules to create an intracellular bactericidal effect. Peptidoglycan hydrolases (PGHs) have good bactericidal activity against both drug-sensitive and -resistant S. aureus bacteria. Based on this finding, Rohrig et al. (2020) reported that synergistically active PGH-CPP cocktails reduced both intracellular and drug-resistant S. aureus. JDlys is a cell wall hydrolase (also called lysin) derived from staphylococcus phage JD007. The results of an experiment showed that CPP-JDlys can enter keratinocytes and effectively eliminate intracellular MRSA. In further experiments in mice, CPP-JDlys efficiently inhibited the proliferation of MRSA in murine skin and thus shortened the course of wound healing (Wang Z. et al., 2018). However, the clinical effectiveness of CPPs as drug delivery vehicles is hampered by a number of characteristics. The biggest obstacles for CPP-based medications are physiological instability, a lack of selectivity, and low efficacy (Kim et al., 2021).
AMPs, which are small proteins with potent antibacterial, antiviral, and antifungal activity, are widely found in nature. More than 3,100 different AMPs have been described thus far (Lazzaro et al., 2020). AMPs are secreted by cells in the body as the first barrier of defense against microbial invasion. Some of these AMPs are present constitutively while others may be induced in response to infection (Alcayaga-Miranda et al., 2017). AMPs have a positive charge, which attracts them to the generally negatively charged membranes of bacteria; this results in pore formation and membrane perturbation, leakage of cellular components, and cell death (Stallmann et al., 2006; Lazzaro et al., 2020).
Recently, some short synthetic AMPs have demonstrated highly effective intracellular antimicrobial activity. Bormann et al. (2017) reported that short artificial AMPs that contain three arginine residues and one lysine residue might be responsible for the effective cell penetration observed and killing of internalized bacteria without harming the host cells. Cathelicidin LL-37 is amphiphilic in nature and is comprised of hydrophobic and hydrophilic residues aligned on opposite sides of the peptides. Noore et al. (2013) found that LL-37 was more effective in killing extra- and intracellular S. aureus than commonly used conventional antibiotics. However, the cell-penetrating mechanism of AMPs is not fully understood and might occur by direct translocation, endocytotic uptake, or the formation of inverted micelles (Bormann et al., 2017). Besides, natural AMPs are difficult to produce because of their low yield and undesirable impurities. Many AMPs have high antibacterial activity, but some of them have undesirable properties that make them unsuitable for clinical use (Biswaro et al., 2018).
In addition to the more advanced treatments studied above, other new approaches have emerged. A phage is a type of bacteria-targeting virus that has extremely high specificity and is easy to apply. A specific phage can only kill the targeted bacteria but does not affect the normal bacteria and body cells (Grunenwald et al., 2018). Phages infect their specific bacterial hosts and during the lytic (or virulent) lifestyle highjack the machinery of the host cell to replicate and ultimately destroy the host, thus simultaneously producing progeny and killing the host. When used as a bactericidal drug, phages have the following characteristics (Nikolich and Filippov, 2020): (a) phages are effective against multi-drug resistant pathogens and can therefore be used in combination with antibiotics, often with synergistic effects; (b) phages are highly specific and usually infect only one bacterium or subgroup, so they have little effect on the normal microflora; (c) a phage is a self-replicating drug because it can replicate on the target bacteria and concentrate precisely where the pathogen cells at the site of infection must be destroyed; (d) since phages coevolve with their hosts, they can adapt to newly emergent resistant strains of the host bacterium; (e) phages are usually weak immunogens; thus, adverse immunologic responses are unlikely; and (f) when the targeted bacteria disappear, the phage disappears and does not remain in the body.
Through genetic engineering, modified phages can be used for targeted drug delivery and new material assembly, thus extending the field of phage therapy and further expanding the medical community’s understanding of phages (Pires et al., 2016). Kim et al. (2012) found that efficient internalization and cytosolic localization of 3D8 VL transbody-displayed phages provides a useful tool for the intracellular delivery of polar macromolecules. This study also proves that phage internalization occurs via a physiological endocytotic mechanism through specific cell surface receptors rather than non-specific transcytotic pathways. By fluorescent labeling of the phage and S. aureus, the phage was shown to penetrate bovine mammary epithelial cells and remove S. aureus from the cells (Zhang et al., 2017). Phages can also enter macrophages by infecting MRSA, killing the intracellular MRSA, and significantly reducing the cytotoxic damage caused by MRSA (Capparelli et al., 2007; Kaur et al., 2014). Previous studies have shown a synergistic effect between bacteriophages and antibiotics or CCP (Gutierrez et al., 2018; Wang Z. et al., 2018). JDlys is a cell wall hydrolase (also called lysin) derived from Staphylococcus phage JD007. CPPTat-JDlys, a fusion of CPPTat to JDlys, was able to effectively eliminate intracellular MRSA bacteria and alleviate the inflammatory response and cell damage caused by MRSA (Wang Z. et al., 2018). Similarly, the combination of lytic protein CF-301 and daptomycin and endolysin MR-10 and minocycline was found to significantly increase survival from bacteremia in mice (Gutierrez et al., 2018). However, up to now, the phage therapy has limitations related to the bacterial resistance to phage (Caflisch et al., 2019), quality and safety requirements, stability of phage preparations, fast phage screening methods, and unsatisfactory regulatory framework (Pires et al., 2020). More research is needed in the future to refine phage therapy.
Staphylococcus aureus internalization can be divided into three different phases, each with its own characteristics. Once S. aureus is internalized into cells, it is difficult to treat even with antibiotics. In the first and second phases, immunotherapy and some microbial agents can effectively interfere with bacterial adhesion. In third phase, nanoparticles, photochemical internalization, cell penetrating peptides, antimicrobial peptides, and bacteriophage therapy can effectively eliminate bacteria from cells. However, it is worth noting that most of these treatments are only in the experimental phase at present and have not entered the clinical trial phase. Significant efforts must be made to verify the therapeutic effect and safety of these drugs.
JL designed the study, collected the data, and wrote the manuscript. QW and FG critically revised the work. LA contributed to the writing. TY contributed to the review of the manuscript. All authors contributed to the article and approved the submitted version.
This study was supported by the National Natural Science Foundation of China (Grant No. 31970090).
The authors declare that the research was conducted in the absence of any commercial or financial relationships that could be construed as a potential conflict of interest.
All claims expressed in this article are solely those of the authors and do not necessarily represent those of their affiliated organizations, or those of the publisher, the editors and the reviewers. Any product that may be evaluated in this article, or claim that may be made by its manufacturer, is not guaranteed or endorsed by the publisher.
Ahmed, S., Meghji, S., Williams, R. J., Henderson, B., Brock, J. H., and Nair, S. P. (2001). Staphylococcus aureus fibronectin binding proteins are essential for internalization by osteoblasts but do not account for differences in intracellular levels of bacteria. Infect. Immun. 69, 2872–2877. doi: 10.1128/IAI.69.5.2872-2877.2001
Alcayaga-Miranda, F., Cuenca, J., and Khoury, M. (2017). Antimicrobial activity of mesenchymal stem cells: Current status and new perspectives of antimicrobial peptide-based therapies. Front. Immunol. 8:339. doi: 10.3389/fimmu.2017.00339
Alexander, E. H., and Hudson, M. C. (2001). Factors influencing the internalization of Staphylococcus aureus and impacts on the course of infections in humans. Appl. Microbiol. Biotechnol. 56, 361–366. doi: 10.1007/s002530100703
Alva-Murillo, N., Lopez-Meza, J. E., and Ochoa-Zarzosa, A. (2014). Nonprofessional phagocytic cell receptors involved in Staphylococcus aureus internalization. Biomed Res. Int. 2014:538546. doi: 10.1155/2014/538546
Alva-Murillo, N., Ochoa-Zarzosa, A., and Lopez-Meza, J. E. (2012). Short chain fatty acids (propionic and hexanoic) decrease Staphylococcus aureus internalization into bovine mammary epithelial cells and modulate antimicrobial peptide expression. Vet. Microbiol. 155, 324–331. doi: 10.1016/j.vetmic.2011.08.025
Bagnoli, F., Bertholet, S., and Grandi, G. (2012). Inferring reasons for the failure of Staphylococcus aureus vaccines in clinical trials. Front. Cell. Infect. Microbiol. 2:16. doi: 10.3389/fcimb.2012.00016
Balasubramanian, D., Harper, L., Shopsin, B., and Torres, V. J. (2017). Staphylococcus aureus pathogenesis in diverse host environments. Pathog. Dis. 75:ftx005.
Bhattacharya, M., Wozniak, D. J., Stoodley, P., and Hall-Stoodley, L. (2015). Prevention and treatment of Staphylococcus aureus biofilms. Expert Rev. Anti Infect. Ther. 13, 1499–1516.
Biswaro, L. S., Da, C. S. M., Rezende, T., Dias, S. C., and Franco, O. L. (2018). Antimicrobial peptides and nanotechnology. Recent Advances and Challenges. Front. Microbiol. 9:855. doi: 10.3389/fmicb.2018.00855
Bongiorno, D., Musso, N., Lazzaro, L. M., Mongelli, G., Stefani, S., and Campanile, F. (2020). Detection of methicillin-resistant Staphylococcus aureus persistence in osteoblasts using imaging flow cytometry. Microbiologyopen 9:e1017. doi: 10.1002/mbo3.1017
Bormann, N., Koliszak, A., Kasper, S., Schoen, L., Hilpert, K., Volkmer, R., et al. (2017). A short artificial antimicrobial peptide shows potential to prevent or treat bone infections. Sci. Rep. 7:1506.
Bouchard, D. S., Rault, L., Berkova, N., Le Loir, Y., and Even, S. (2013). Inhibition of Staphylococcus aureus invasion into bovine mammary epithelial cells by contact with live Lactobacillus casei. Appl. Environ. Microbiol. 79, 877–885. doi: 10.1128/AEM.03323-12
Caflisch, K. M., Suh, G. A., and Patel, R. (2019). Biological challenges of phage therapy and proposed solutions: A literature review. Expert Rev. Anti. Infect. Ther. 17, 1011–1041. doi: 10.1080/14787210.2019.1694905
Capparelli, R., Parlato, M., Borriello, G., Salvatore, P., and Iannelli, D. (2007). Experimental phage therapy against Staphylococcus aureus in mice. Antimicrob. Agents Chemother. 51, 2765–2773.
Chen, J., Zhou, H., Huang, J., Zhang, R., and Rao, X. (2021). Virulence alterations in Staphylococcus aureus upon treatment with the sub-inhibitory concentrations of antibiotics. J. Adv. Res. 31, 165–175. doi: 10.1016/j.jare.2021.01.008
Claro, T., Widaa, A., McDonnell, C., Foster, T. J., O’Brien, F. J., and Kerrigan, S. W. (2013). Staphylococcus aureus protein a binding to osteoblast tumour necrosis factor receptor 1 results in activation of nuclear factor kappa B and release of interleukin-6 in bone infection. Microbiology (Reading). 159(Pt 1), 147–154. doi: 10.1099/mic.0.063016-0
Claro, T., Widaa, A., O’Seaghdha, M., Miajlovic, H., Foster, T. J., O’Brien, F. J., et al. (2011). Staphylococcus aureus protein A binds to osteoblasts and triggers signals that weaken bone in osteomyelitis. PLoS One 6:e18748. doi: 10.1371/journal.pone.0018748.
Delfani, S., Mohabati, M. A., Imani, F. A., Amani, J., and Emaneini, M. (2016). Protection of mice against Staphylococcus aureus infection by a recombinant protein ClfA-IsdB-Hlg as a vaccine candidate. Med. Microbiol. Immunol. 205, 47–55. doi: 10.1007/s00430-015-0425-y
Foster, T. J., Geoghegan, J. A., Ganesh, V. K., and Hook, M. (2014). Adhesion, invasion and evasion: The many functions of the surface proteins of Staphylococcus aureus. Nat. Rev. Microbiol. 12, 49–62. doi: 10.1038/nrmicro3161
Fournier, B. (2012). The function of TLR2 during staphylococcal diseases. Front. Cell. Infect. Microbiol. 2:167. doi: 10.3389/fcimb.2012.00167
Fowler, T., Wann, E. R., Joh, D., Johansson, S., Foster, T. J., and Hook, M. (2000). Cellular invasion by Staphylococcus aureus involves a fibronectin bridge between the bacterial fibronectin-binding MSCRAMMs and host cell beta1 integrins. Eur. J. Cell. Biol. 79, 672–679. doi: 10.1078/0171-9335-00104
Fowler, V. G., Allen, K. B., Moreira, Moustafa, M., Isgro, F., Boucher, H. W., et al. (2013). Effect of an investigational vaccine for preventing Staphylococcus aureus infections after cardiothoracic surgery: A randomized trial. JAMA 309, 1368–1378. doi: 10.1001/jama.2013.3010
Fraunholz, M., and Sinha, B. (2012). Intracellular Staphylococcus aureus: Live-in and let die. Front. Cell. Infect. Microbiol. 2:43. doi: 10.3389/fcimb.2012.00043
Fretz, M. M., Penning, N. A., Al-Taei, S., Futaki, S., Takeuchi, T., Nakase, I., et al. (2007). Temperature-, concentration- and cholesterol-dependent translocation of L- and D-octa-arginine across the plasma and nuclear membrane of CD34+ leukaemia cells. Biochem. J. 403, 335–342. doi: 10.1042/BJ20061808
Gomez, M. I., Lee, A., Reddy, B., Muir, A., Soong, G., Pitt, A., et al. (2004). Staphylococcus aureus protein a induces airway epithelial inflammatory responses by activating TNFR1. Nat. Med. 10, 842–848. doi: 10.1038/nm1079
Grunenwald, C. M., Bennett, M. R., and Skaar, E. P. (2018). Nonconventional Therapeutics against Staphylococcus aureus. Microbiol. Spectr. 6:10.1128.
Guidotti, G., Brambilla, L., and Rossi, D. (2017). Cell-penetrating peptides: From basic research to clinics. Trends Pharmacol. Sci. 38, 406–424.
Gutierrez, D., Fernandez, L., Rodriguez, A., and Garcia, P. (2018). Are phage lytic proteins the secret weapon to kill Staphylococcus aureus? mBio 9, e1923–e1917. doi: 10.1128/mBio.01923-17
Hamza, T., and Li, B. (2014). Differential responses of osteoblasts and macrophages upon Staphylococcus aureus infection. BMC Microbiol. 14:207. doi: 10.1186/s12866-014-0207-5.
Hauck, C. R., and Ohlsen, K. (2006). Sticky connections: Extracellular matrix protein recognition and integrin-mediated cellular invasion by Staphylococcus aureus. Curr. Opin. Microbiol. 9, 5–11.
Herman-Bausier, P., Valotteau, C., Pietrocola, G., Rindi, S., Alsteens, D., Foster, T. J., et al. (2016). Mechanical strength and inhibition of the Staphylococcus aureus collagen-binding protein cna. mBio. 7, e1529–e1516. doi: 10.1128/mBio.01529-16
Jauregui, C. E., Mansell, J. P., Jepson, M. A., and Jenkinson, H. F. (2013). Differential interactions of Streptococcus gordonii and Staphylococcus aureus with cultured osteoblasts. Mol. Oral. Microbiol. 28, 250–266. doi: 10.1111/omi.12022
Jerjes, W., Hamdoon, Z., Berg, K., Hogset, A., and Hopper, C. (2020a). Apparent Complete Response of a treatment refractory and recurrent squamous cell carcinoma lesion to photochemical internalization: A clinical case study. Photochem. Photobiol. 96, 680–683. doi: 10.1111/php.13210
Jerjes, W., Theodossiou, T. A., Hirschberg, H., Hogset, A., Weyergang, A., Selbo, P. K., et al. (2020b). Photochemical internalization for intracellular drug delivery. From basic mechanisms to clinical research. J. Clin. Med. 9:528.
Josefsson, E., Hartford, O., O’Brien, L., Patti, J. M., and Foster, T. (2001). Protection against experimental Staphylococcus aureus arthritis by vaccination with clumping factor A, a novel virulence determinant. J. Infect. Dis. 184, 1572–1580. doi: 10.1086/324430
Josse, J., Velard, F., and Gangloff, S. C. (2015). Staphylococcus aureus vs. Osteoblast: Relationship and consequences in osteomyelitis. Front. Cell. Infect. Microbiol. 5:85. doi: 10.3389/fcimb.2015.00085
Kang, J., Dietz, M. J., Hughes, K., Xing, M., and Li, B. (2019). Silver nanoparticles present high intracellular and extracellular killing against Staphylococcus aureus. J. Antimicrob. Chemother. 74, 1578–1585. doi: 10.1093/jac/dkz053
Kaur, S., Harjai, K., and Chhibber, S. (2014). Bacteriophage-aided intracellular killing of engulfed methicillin-resistant Staphylococcus aureus (MRSA) by murine macrophages. Appl. Microbiol. Biotechnol. 98, 4653–4661. doi: 10.1007/s00253-014-5643-5
Khalil, H., Williams, R. J., Stenbeck, G., Henderson, B., Meghji, S., and Nair, S. P. (2007). Invasion of bone cells by Staphylococcus epidermidis. Microbes Infect. 9, 460–465.
Kim, A., Shin, T. H., Shin, S. M., Pham, C. D., Choi, D. K., Kwon, M. H., et al. (2012). Cellular internalization mechanism and intracellular trafficking of filamentous M13 phages displaying a cell-penetrating transbody and TAT peptide. PLoS One 7:e51813. doi: 10.1371/journal.pone.0051
Kim, G. C., Cheon, D. H., and Lee, Y. (2021). Challenge to overcome current limitations of cell-penetrating peptides. Biochim. Biophys. Acta Proteins Proteom. 1869:140604.
Lacey, K. A., Mulcahy, M. E., Towell, A. M., Geoghegan, J. A., and McLoughlin, R. M. (2019). Clumping factor B is an important virulence factor during Staphylococcus aureus skin infection and a promising vaccine target. PLoS Pathog. 15:e1007713. doi: 10.1371/journal.ppat.1007713.
Lazzaro, B. P., Zasloff, M., and Rolff, J. (2020). Antimicrobial peptides: Application informed by evolution. Science. 368:eaau5480.
Li, X., Wang, X., Thompson, C. D., Park, S., Park, W. B., and Lee, J. C. (2016). Preclinical efficacy of clumping factor a in prevention of Staphylococcus aureus infection. mBio 7, e2215–e2232. doi: 10.1128/mBio.02232-15
Liang, X., Garcia, B. L., Visai, L., Prabhakaran, S., Meenan, N. A., Potts, J. R., et al. (2016). Allosteric regulation of fibronectin/alpha5beta1 interaction by fibronectin-binding MSCRAMMs. PLoS One 11:e159118. doi: 10.1371/journal.pone.0159118
Liu, Q., Du, X., Hong, X., Li, T., Zheng, B., He, L., et al. (2015). Targeting surface protein SasX by active and passive vaccination to reduce Staphylococcus aureus colonization and infection. Infect. Immun. 83, 2168–2174. doi: 10.1128/IAI.02951-14
Madrazo, D. R., Tranguch, S. L., and Marriott, I. (2003). Signaling via Toll-like receptor 5 can initiate inflammatory mediator production by murine osteoblasts. Infect. Immun. 71, 5418–5421. doi: 10.1128/IAI.71.9.5418-5421.2003
Mahmood, A., Prufert, F., Efiana, N. A., Ashraf, M. I., Hermann, M., Hussain, S., et al. (2016). Cell-penetrating self-nanoemulsifying drug delivery systems (SNEDDS) for oral gene delivery. Expert Opin. Drug Deliv. 13, 1503–1512. doi: 10.1080/17425247.2016.1213236
Maya, S., Indulekha, S., Sukhithasri, V., Smitha, K. T., Nair, S. V., Jayakumar, R., et al. (2012). Efficacy of tetracycline encapsulated O-carboxymethyl chitosan nanoparticles against intracellular infections of Staphylococcus aureus. Int. J. Biol. Macromol. 51, 392–399. doi: 10.1016/j.ijbiomac.2012.06.009
Medina-Estrada, I., Lopez-Meza, J. E., and Ochoa-Zarzosa, A. (2016). Anti-inflammatory and antimicrobial effects of estradiol in bovine mammary epithelial cells during Staphylococcus aureus internalization. Mediators Inflamm. 2016:6120509. doi: 10.1155/2016/6120509
Melter, O., and Radojevic, B. (2010). Small colony variants of Staphylococcus aureus–review. Folia Microbiol. (Praha) 55, 548–558.
Mihu, M. R., Cabral, V., Pattabhi, R., Tar, M. T., Davies, K. P., Friedman, A. J., et al. (2017). sustained nitric oxide-releasing nanoparticles interfere with methicillin-resistant Staphylococcus aureus adhesion and biofilm formation in a rat central venous catheter model. Antimicrob. Agents Chemother. 61, e2020–e2016. doi: 10.1128/AAC.02020-16
Moormeier, D. E., and Bayles, K. W. (2017). Staphylococcus aureus biofilm: A complex developmental organism. Mol. Microbiol. 104, 365–376. doi: 10.1111/mmi.13634
Mu, H., Tang, J., Liu, Q., Sun, C., Wang, T., and Duan, J. (2016). Potent antibacterial nanoparticles against biofilm and intracellular bacteria. Sci. Rep. 6:18877.
Nikolich, M. P., and Filippov, A. A. (2020). Bacteriophage therapy: Developments and directions. Antibiotics (Basel) 9:135.
Noore, J., Noore, A., and Li, B. (2013). Cationic antimicrobial peptide LL-37 is effective against both extra- and intracellular Staphylococcus aureus. Antimicrob. Agents Chemother. 57, 1283–1290. doi: 10.1128/AAC.01650-12
Ochoa-Zarzosa, A., Villarreal-Fernandez, E., Cano-Camacho, H., and Lopez-Meza, J. E. (2009). Sodium butyrate inhibits Staphylococcus aureus internalization in bovine mammary epithelial cells and induces the expression of antimicrobial peptide genes. Microb. Pathog. 47, 1–7. doi: 10.1016/j.micpath.2009.04.006
Oliveira-Nascimento, L., Massari, P., and Wetzler, L. M. (2012). The role of TLR2 in infection and immunity. Front. Immunol. 3:79. doi: 10.3389/fimmu.2012.00079
Papa, R., Artini, M., Cellini, A., Tilotta, M., Galano, E., Pucci, P., et al. (2013). A new anti-infective strategy to reduce the spreading of antibiotic resistance by the action on adhesion-mediated virulence factors in Staphylococcus aureus. Microb. Pathog. 63, 44–53. doi: 10.1016/j.micpath.2013.05.003
Patti, J. M., Bremell, T., Krajewska-Pietrasik, D., Abdelnour, A., Tarkowski, A., Ryden, C., et al. (1994). The Staphylococcus aureus collagen adhesin is a virulence determinant in experimental septic arthritis. Infect. Immun. 62, 152–161. doi: 10.1128/iai.62.1.152-161.1994
Pires, D. P., Cleto, S., Sillankorva, S., Azeredo, J., and Lu, T. K. (2016). Genetically Engineered Phages: A Review of Advances over the Last Decade. Microbiol. Mol. Biol. Rev. 80, 523–543.
Pires, D. P., Costa, A. R., Pinto, G., Meneses, L., and Azeredo, J. (2020). Current challenges and future opportunities of phage therapy. FEMS Microbiol. Rev. 44, 684–700.
Proctor, R. A., Kriegeskorte, A., Kahl, B. C., Becker, K., Loffler, B., and Peters, G. (2014). Staphylococcus aureus Small Colony Variants (SCVs): A road map for the metabolic pathways involved in persistent infections. Front. Cell. Infect. Microbiol. 4:99. doi: 10.3389/fcimb.2014.00099.
Prystopiuk, V., Feuillie, C., Herman-Bausier, P., Viela, F., Alsteens, D., Pietrocola, G., et al. (2018). Mechanical forces guiding Staphylococcus aureus cellular invasion. Acs Nano. 12, 3609–3622. doi: 10.1021/acsnano.8b00716
Randhawa, H. K., Gautam, A., Sharma, M., Bhatia, R., Varshney, G. C., Raghava, G. P., et al. (2016). Cell-penetrating peptide and antibiotic combination therapy: A potential alternative to combat drug resistance in methicillin-resistant Staphylococcus aureus. Appl. Microbiol. Biotechnol. 100, 4073–4083. doi: 10.1007/s00253-016-7329-7
Rauch, S., Gough, P., Kim, H. K., Schneewind, O., and Missiakas, D. (2014). Vaccine protection of leukopenic mice against Staphylococcus aureus bloodstream infection. Infect. Immun. 82, 4889–4898. doi: 10.1128/IAI.02328-14
Richter, E., Harms, M., Ventz, K., Nolker, R., Fraunholz, M. J., Mostertz, J., et al. (2016). Quantitative proteomics reveals the dynamics of protein phosphorylation in human bronchial epithelial cells during internalization, phagosomal escape, and intracellular replication of Staphylococcus aureus. J. Proteome Res. 15, 4369–4386. doi: 10.1021/acs.jproteome.6b00421
Rohrig, C., Huemer, M., Lorge, D., Luterbacher, S., Phothaworn, P., Schefer, C., et al. (2020). Targeting hidden pathogens: Cell-penetrating enzybiotics eradicate intracellular drug-resistant Staphylococcus aureus. mBio 11, e209–e220. doi: 10.1128/mBio.00209-20
Schneewind, O., and Missiakas, D. (2019). Sortases, surface proteins, and their roles in Staphylococcus aureus disease and vaccine development. Microbiol. Spectr. 7:10.1128. doi: 10.1128/microbiolspec.PSIB-0004-2018
Scully, I. L., Timofeyeva, Y., Keeney, D., Matsuka, Y. V., Severina, E., McNeil, L. K., et al. (2015). Demonstration of the preclinical correlate of protection for Staphylococcus aureus clumping factor A in a murine model of infection. Vaccine 33, 5452–5457. doi: 10.1016/j.vaccine.2015.08.029
Selan, L., Papa, R., Ermocida, A., Cellini, A., Ettorre, E., Vrenna, G., et al. (2017). Serratiopeptidase reduces the invasion of osteoblasts by Staphylococcus aureus. Int. J. Immunopathol. Pharmacol. 30, 423–428. doi: 10.1177/0394632017745762
Sinha, B., Francois, P., Que, Y. A., Hussain, M., Heilmann, C., Moreillon, P., et al. (2000). Heterologously expressed Staphylococcus aureus fibronectin-binding proteins are sufficient for invasion of host cells. Infect. Immun. 68, 6871–6878. doi: 10.1128/IAI.68.12.6871-6878.2000
Sosic, L., Selbo, P. K., Kotkowska, Z. K., Kundig, T. M., Hogset, A., and Johansen, P. (2020). Photochemical internalization: Light paves way for new cancer chemotherapies and vaccines. Cancers (Basel) 12:165. doi: 10.3390/cancers12010165
Stallmann, H. P., Faber, C., Nieuw, A. A., and Wuisman, P. I. (2006). Antimicrobial peptides: Review of their application in musculoskeletal infections. Injury. 37(Suppl. 2), S34–S40.
Suaya, J. A., Mera, R. M., Cassidy, A., O’Hara, P., Amrine-Madsen, H., Burstin, S., et al. (2014). Incidence and cost of hospitalizations associated with Staphylococcus aureus skin and soft tissue infections in the United States from 2001 through 2009. BMC Infect. Dis. 14:296. doi: 10.1186/1471-2334-14-296
Suresh, M. K., Biswas, R., and Biswas, L. (2019). An update on recent developments in the prevention and treatment of Staphylococcus aureus biofilms. Int. J. Med. Microbiol. 309, 1–12.
Tkaczyk, C., Hamilton, M. M., Sadowska, A., Shi, Y., Chang, C. S., Chowdhury, P., et al. (2016). targeting alpha toxin and clfa with a multimechanistic monoclonal-antibody-based approach for prophylaxis of serious Staphylococcus aureus disease. mBio 7, e528–e516. doi: 10.1128/mBio.00528-16
Tong, S. Y., Davis, J. S., Eichenberger, E., Holland, T. L., and Fowler, V. J. (2015). Staphylococcus aureus infections: Epidemiology, pathophysiology, clinical manifestations, and management. Clin. Microbiol. Rev. 28, 603–661.
Tuchscherr, L., Kreis, C. A., Hoerr, V., Flint, L., Hachmeister, M., Geraci, J., et al. (2016). Staphylococcus aureus develops increased resistance to antibiotics by forming dynamic small colony variants during chronic osteomyelitis. J. Antimicrob. Chemother. 71, 438–448. doi: 10.1093/jac/dkv371
Uematsu, S., and Akira, S. (2006). Toll-like receptors and innate immunity. J. Mol. Med. (Berl). 84, 712–725.
Varoga, D., Wruck, C. J., Tohidnezhad, M., Brandenburg, L., Paulsen, F., Mentlein, R., et al. (2009). Osteoblasts participate in the innate immunity of the bone by producing human beta defensin-3. Histochem. Cell Biol. 131, 207–218. doi: 10.1007/s00418-008-0522-8
Wang, X., Wang, X., Teng, D., Mao, R., Hao, Y., Yang, N., et al. (2018). Increased intracellular activity of MP1102 and NZ2114 against Staphylococcus aureus in vitro and in vivo. Sci. Rep. 8:4204. doi: 10.1038/s41598-018-22245-5
Wang, Z., Kong, L., Liu, Y., Fu, Q., Cui, Z., Wang, J., et al. (2018). A phage lysin fused to a cell-penetrating peptide kills intracellular methicillin-resistant Staphylococcus aureus in keratinocytes and has potential as a treatment for skin infections in mice. Appl. Environ. Microbiol. 84, e380–e318. doi: 10.1128/AEM.00380-18
Wen, Q., Gu, F., Sui, Z., Su, Z., and Yu, T. (2020). The process of osteoblastic infection by Staphylococcus aureus. Int. J. Med. Sci. 17, 1327–1332.
Yang, L., Cai, C., Feng, Q., Shi, Y., Zuo, Q., Yang, H., et al. (2016). Protective efficacy of the chimeric Staphylococcus aureus vaccine candidate IC in sepsis and pneumonia models. Sci. Rep. 6:20929. doi: 10.1038/srep20929
Yang, Y., Qian, M., Yi, S., Liu, S., Li, B., Yu, R., et al. (2016). Monoclonal antibody targeting Staphylococcus aureus surface protein a (sasa) protect against Staphylococcus aureus sepsis and peritonitis in mice. PLoS One 11:e149460. doi: 10.1371/journal.pone.0149460
Younes, J. A., van der Mei, H. C., van den Heuvel, E., Busscher, H. J., and Reid, G. (2012). Adhesion forces and coaggregation between vaginal Staphylococci and lactobacilli. PLoS One 7:e36917. doi: 10.1371/journal.pone.0036917
Zapotoczna, M., Jevnikar, Z., Miajlovic, H., Kos, J., and Foster, T. J. (2013). Iron-regulated surface determinant B (IsdB) promotes Staphylococcus aureus adherence to and internalization by non-phagocytic human cells. Cell. Microbiol. 15, 1026–1041.
Zhang, G., Zhao, Y., Hayes, A. J., Psaltis, A. J., Wormald, P. J., and Vreugde, S. (2018). Staphylococcus aureus small colony variants: Prevalence in chronic rhinosinusitis and induction by antibiotics. Allergy 73, 2403–2405. doi: 10.1111/all.13580
Zhang, L., Sun, L., Wei, R., Gao, Q., He, T., Xu, C., et al. (2017). Intracellular Staphylococcus aureus control by virulent bacteriophages within MAC-T bovine mammary epithelial cells. Antimicrob. Agents Chemother. 61:e1990–e1916. doi: 10.1128/AAC.01990-16
Zhang, X., de Boer, L., Heiliegers, L., Man-Bovenkerk, S., Selbo, P. K., Drijfhout, J. W., et al. (2018). Photochemical internalization enhances cytosolic release of antibiotic and increases its efficacy against Staphylococcal infection. J. Control. Release 283, 214–222. doi: 10.1016/j.jconrel.2018.06.004
Zhou, K., Li, C., Chen, D., Pan, Y., Tao, Y., Qu, W., et al. (2018). A review on nanosystems as an effective approach against infections of Staphylococcus aureus. Int. J. Nanomedicine 13, 7333–7347.
Keywords: Staphylococcus aureus, bacterial persistence, internalization, nanoparticles, cell-penetrating peptides
Citation: Li J, Wen Q, Gu F, An L and Yu T (2022) Non-antibiotic strategies for prevention and treatment of internalized Staphylococcus aureus. Front. Microbiol. 13:974984. doi: 10.3389/fmicb.2022.974984
Received: 01 July 2022; Accepted: 08 August 2022;
Published: 31 August 2022.
Edited by:
Giovanna Batoni, University of Pisa, ItalyReviewed by:
Dafne Bongiorno, University of Catania, ItalyCopyright © 2022 Li, Wen, Gu, An and Yu. This is an open-access article distributed under the terms of the Creative Commons Attribution License (CC BY). The use, distribution or reproduction in other forums is permitted, provided the original author(s) and the copyright owner(s) are credited and that the original publication in this journal is cited, in accordance with accepted academic practice. No use, distribution or reproduction is permitted which does not comply with these terms.
*Correspondence: Tiecheng Yu, eXV0Y0BqbHUuZWR1LmNu
Disclaimer: All claims expressed in this article are solely those of the authors and do not necessarily represent those of their affiliated organizations, or those of the publisher, the editors and the reviewers. Any product that may be evaluated in this article or claim that may be made by its manufacturer is not guaranteed or endorsed by the publisher.
Research integrity at Frontiers
Learn more about the work of our research integrity team to safeguard the quality of each article we publish.