- 1Fujian Key Laboratory on Conservation and Sustainable Utilization of Marine Biodiversity, Fuzhou Institute of Oceanography, Minjiang University, Fuzhou, China
- 2Department of Plant Pathology, Nanjing Agricultural University, Nanjing, China
- 3Institute of Plant Pathology, Fujian Agriculture and Forestry University, Fuzhou, China
- 4College of Chemistry and Life Sciences, Sichuan Provincial Key Laboratory for Development and Utilization of Characteristic Horticultural Biological Resources, Chengdu Normal University, Chengdu, China
- 5Department of Forest Mycology and Plant Pathology, Swedish University of Agricultural Sciences, Uppsala, Sweden
Effector genes, together with climatic and other environmental factors, play multifaceted roles in the development of plant diseases. Understanding the role of environmental factors, particularly climate conditions affecting the evolution of effector genes, is important for predicting the long-term value of the genes in controlling agricultural diseases. Here, we collected Phytophthora infestans populations from five locations along a mountainous hill in China and sequenced the effector gene Pi02860 from >300 isolates. To minimize the influence of other ecological factors, isolates were sampled from the same potato cultivar on the same day. We also expressed the gene to visualise its cellular location, assayed its pathogenicity and evaluated its response to experimental temperatures. We found that Pi02860 exhibited moderate genetic variation at the nucleotide level which was mainly generated by point mutation. The mutations did not change the cellular location of the effector gene but significantly modified the fitness of P. infestans. Genetic variation and pathogenicity of the effector gene were positively associated with the altitude of sample sites, possibly due to increased mutation rate induced by the vertical distribution of environmental factors such as UV radiation and temperature. We further found that Pi02860 expression was regulated by experimental temperature with reduced expression as experimental temperature increased. Together, these results indicate that UV radiation and temperature are important environmental factors regulating the evolution of effector genes and provide us with considerable insight as to their future sustainable action under climate and other environmental change.
Introduction
Plant diseases have deleterious effects on ecological integrity and agricultural productivity, greatly threatening natural sustainability and global food security. For example, damage caused by plant diseases such as chestnut blight and Dutch elm disease to primary and secondary forests not only reduces species richness and the associated ecological services (Martín et al., 2013) but has also restructured ecological landscapes in North America and Europe (Raum, 2020). From a social-economic perspective, 13–20% of global agricultural harvest (Burdon et al., 2020) corresponding to ~US$220 billion is lost due to plant disease epidemics annually. Moreover, it is of great concern that the ecological and societal impact of plant diseases may be further aggravated by global climate change (Cavicchioli et al., 2019).
Effector proteins, either directly secreted into the apoplast or translocated into host cells, play critical roles in the antagonistic interaction between hosts and pathogens (Lo Presti et al., 2015; Wang et al., 2017). They are mainly involved in the regulation of the host’s immunity system but also take part in nutrient uptake of pathogens from hosts (Uhse and Djamei, 2018). To broaden their host range and increase their successful colonization and reproduction, both expression level and profile of pathogen effector genes are strictly regulated during antagonistic interactions, and show waves of concerted expression at diverse stages of infection and reproduction according to the surrounding environment and genetic background of hosts (Wang et al., 2011; Soyer et al., 2014; Ren et al., 2020). For example, some effector genes are upregulated to promote host susceptibility (Boevink et al., 2016) and subdue the host immune system (Turnbull et al., 2017), while others are down regulated to avoid host recognition (Lo Presti et al., 2015).
It has been found that plant pathogens encode a large number of effectors in order to ensure successful colonization and reproduction by suppressing host defence immunity (Franceschetti et al., 2017). The specialized location of many effector genes in the gene-sparse, repeat-rich regions of the genome provide them with unique niches for quick evolution (Haas et al., 2009; Raffaele and Kamoun, 2012; Dong et al., 2015) and hence the ability to defeat host defence immunity involved in gene-for-gene interactions (Fry, 2008; Vleeshouwers et al., 2011). The arm race between host resistance genes and pathogen effector genes is particularly intense in agricultural ecosystems due to the strong directional selection generated by farming practices associated with crop intensification and genetic homogenization (Zhan et al., 2014, 2015). It is widely documented that pathogens in agricultural ecosystems are equipped with an array of mutation mechanisms to drive their escape from host immunity. For example, Phytophthora infestans Avr1 and Avr4 were removed from the effector repertoire by pseudogenization to escape the corresponding R1 and R4 detection in host (van Poppel et al., 2008; Shen et al., 2021; Waheed et al., 2021). Phytophthora infestans Avr2 escapes host immunity by protein disordering through which shifts the effector from an avirulent type to virulent type (Yang et al., 2020). On the other hand, P. infestans Avr3a overcomes R3a recognition by potato crop through point mutations in two residues (K80I103 to E80M103) where protein (effector)-protein (receptor) interactions take place (Boutemy et al., 2011; Yaeno et al., 2011).
As an important part of the disease triangle, climatic conditions can regulate the growth and development of pathogens and hosts as well as their interactions (Juroszek et al., 2020). In addition to host resistance and the biology of pathogens, recent studies have shown that the evolution and performance of effector genes are also affected by climate conditions. For example, the effector function of P. syringae AvrRpt2 to Arabidopsis RPS2 resistance disappeared when plants were grown for 3 weeks under 28°C (Wang et al., 2009). The strong association of annual mean temperature in the collection sites with nucleotide diversity and/or population differentiation of Avr1 (Shen et al., 2021), Avr2 (Yang et al., 2020), Avr3a (Yang et al., 2018) and Avr4 (Waheed et al., 2021) in P. infestans further supports that local air temperature could influence the evolution of effector genes.
Our knowledge about the evolution of effector genes is fragmented. Most research on this part of genome has focused on molecular characterization and functional analysis. Population genetic analysis of the spatial distribution of effector genes to understand their evolutionary history, mechanisms and future trajectory is limited. Climate changes in the planet have accelerated in the past decades due to the human activities including agricultural production. It is predicted that average air temperature would increase ~5°C by the end of the century (Tollefson, 2020). UV radiation, another important determinant of disease epidemics and pathogen evolution, is also expected to increase associated with the current wave of climate change due to the thinning ozone layer associated with emission of some industrial gases (Bais et al., 2015). Knowledge of how and to what extent these climate and climate related factors may impact the evolution and performance of effector genes is important to understand future ecological and agricultural sustainability under changing climates.
In this manuscript, we used a population genetic approach to study the evolution of Pi02860, one of confirmed effector gene in the interaction of P. infestans with potato host (Yang et al., 2016b) and how ecological factors may influence its evolution. P. infestans is one of the most widespread oomycete plant pathogens causing late blight disease of potato and tomato. Genome analysis revealed that P. infestans codes many effectors that are delivered into plant cells during infection where they interfere with host immunity (Haas et al., 2009). Pi02860 is one of the effectors that are specifically upregulated at ~2 days after infecting potato plants (Yang et al., 2016b). It is found that this effector significantly suppressed the cell death triggered by pathogen-associated molecular pattern INF1 and strongly enhanced the colonization ability of P. infestans (Yang et al., 2016b). It interacted with the host susceptibility protein NRL1 and enhanced the assembly of NRL1 with SWAP70, a positive regulator of immunity, to promote proteasome-mediated degradation of the latter and, thus, suppress immunity (Yang et al., 2016b; He et al., 2018).
The specific objectives of the study were to answer four questions: (i) what is the genetic variation of Pi02860? (ii) How is the genetic diversity generated? (iii) What are the pathogenicity and cellular consequences of the observed mutations? and (iv) how may climatic or climate-related factors such as UV radiation and air temperature affect the performance and spatial distribution of genetic variation in the effector gene? Altitude change provides a strong reflection of climatic gradients across short spatial distances, offering a great opportunity to study the influence of climate conditions particularly UV light and temperature on the biology and ecology of species (Körner, 2007). For example, UV intensity increases 10–20% and temperature decreases ~6.5°C for every 1,000 m elevation. To achieve our objectives, we sampled >300 isolates from five altitudinal sites along a mountainous hill and sequenced the effector gene Pi02860. Genetic variation, mechanisms responsible for the generation of this variation, cellular localization, altitudinal distribution and expression of the effector gene under different experimental temperatures were analysed. A total of 21 nucleotide haplotypes mainly generated by point mutations were found in the collections. The spatial distribution of the effector gene was associated with the altitude of collection sites and the expression of the effector was strongly regulated by experimental temperatures.
Materials and methods
Phytophthora infestans collections
Phytophthora infestans isolates were collected in a single day during the 2016 late blight epidemic season from a single potato cultivar (Hui-2) grown in five fields along along a mountainous hill located at Huize, Yunnan. The altitudes of the five fields ranged from 1,976 to 2,677 m and were designated as A–E from lower to higher altitude, respectively (Table 1). One hundred P. infestans infected plants each at least 1-m apart were selected from each field and one infected leaf was sampled from the upper canopy of each plant. The sampled leaves were transferred to the laboratory within 24 h for P. infestans isolation. A single isolate was secured from each sample, resulting in a collection of a total of 354 isolates with 59–87 isolates originating from each of the five fields. The isolates were purified three times by sequential transfers of a single sporangium to a fresh rye B plate supplemented with ampicillin (100 μg/ml) and rifampicin (50 μg/ml). Detailed information on the sample collection and P. infestans isolation can be found in our previous publications (Zhu et al., 2015; Yang et al., 2016a).
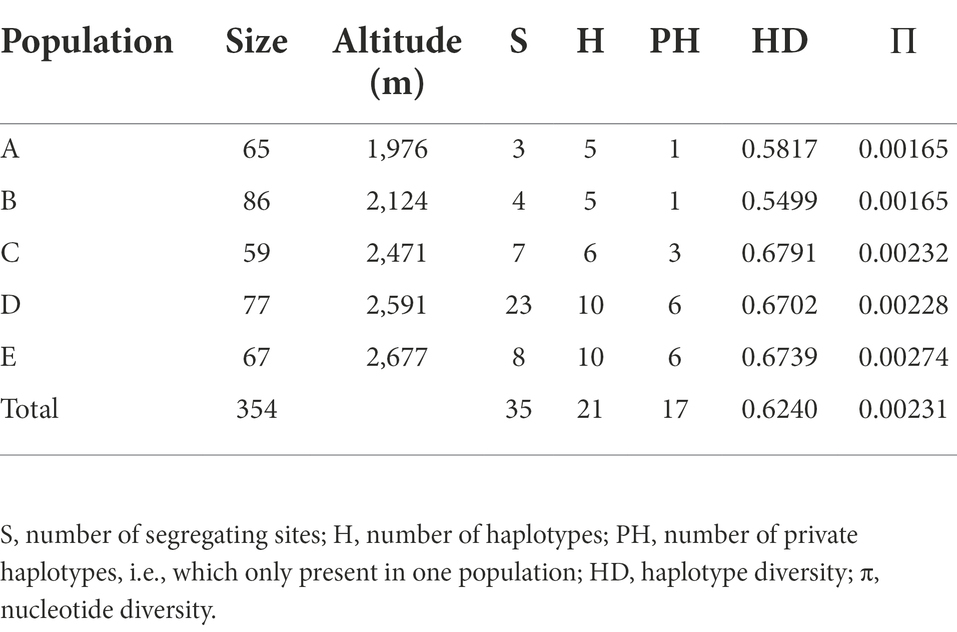
Table 1. Sample size, and genetic variation of Phytophthora infestans Pi02860 sequences collected from five altitudinal locations.
Pi02860 sequencing
Phytophthora infestans isolates were cultured on rye B agar at 18°C in the dark. Mycelia (~100 mg) were harvested after 15 days cultivation, transferred into sterile, 2 ml centrifuge tubes and lyophilized with a vacuum freeze dryer (Alpha1-2, Christ, Germany). The lyophilized mycelia were ground to powder with a mixer mill (MM400, Retsch, Germany). Total DNA was extracted using a Plant gDNA Miniprep Kit (GD 2611, Biomiga, China) according to the manufacturer’s instructions. The genomic DNA was suspended in 50 μl of ultrapure water and stored at −20°C until use.
A pair of Pi02860 specific primers (For: 5′-ACTCACCGTCACCCTCATTC-3′ and Rev: 5′-AACTTTGACTCCGACCGTTG-3′) were designed according to the conserved upstream and downstream of the reference sequence (PITG_02860) downloaded from NCBI and used to amplify the Pi02860 effector gene. PCR reactions were carried out in a 25 μl reaction volume using a thermal cycler (Applied Biosystems 2720). The reaction cocktail contained 1 × PCR buffer, 0.2 mM dNTPs, 1 unit of TransStart KD Plus DNA Polymerase, 0.2 μM of primers and 20 ng of template DNA. PCR amplification was started with an initial denaturation step of 94°C for 2 min, followed by 30 cycles of amplification at 95°C for 30 s, annealing at 55°C for 30 s, extension at 72°C for 50 s, and ended with a further extension cycle at 72°C for 10 min. The PCR products were separated by 1% agarose gel electrophoresis and sent to Sangon Biotech company for sequencing using an ABI3730 automated DNA sequencer (Applied Bio-Systems, United States).
Plant preparation
Nicotiana benthamiana and a universal susceptible potato variety Desiree were grown in a greenhouse at 20°C in 60% humidity. The greenhouse was supplemented with 16 h-light at the intensity of 120–150 μmol m−2 s−1, nutrient and water were supplied at necessary. Nicotiana benthamiana plants were used for agroinfiltration at 4–5 weeks old and potato leaves at 5–6 weeks old were used for P. infestans infection analysis.
Vector construction
After removing those with mutations in the start codon or translating to the same isoforms, 16 Pi02860 haplotypes without a signal peptide were amplified from genomic DNAs of corresponding P. infestans isolates. PCR products were ligated into pEarlyGate 104 (N-terminal GFP tag) using a Vazyme ClonExpressII One Step Cloning Kit, and then transformed into Agrobacterium tumefaciens strain AGL1. The Avr3a and INF1 constructs with N-terminal GFP tag were kindly provided by Dr. Qin He from Huazhong Agricultural University, Wuhan, China.
Agroinfiltration, infection assays, HR suppression and cellular localization
Agrobacterium tumefaciens strain AGL1 transformed with the vector constructed above was grown overnight in LB medium containing selective antibiotics at 28°C, pelleted, resuspended in infiltration buffer (10 mM MES, 10 mM MgCl2), and adjusted to the required optical density measured at 600 nm (OD600) before being infiltrated into N. benthamiana leaves (generally 0.005–0.01 for cellular localization purposes, 0.25 for infection assays, and 0.5 for hypersensitive reaction assays). For co-expression, agrobacterial cultures carrying the appropriate vector constructs were mixed prior to infiltration.
Phytophthora infestans strain 88,069 (Yang et al., 2021) was used for plant infection. It was cultured on rye agar at 18°C for 2 weeks before sporangia collection. The inoculum concentration was adjusted to 40,000 sporangia per ml, and 10 μl droplets were inoculated onto the abaxial side of leaves of intact N. benthamiana plants which were transiently expressed with Pi02860 haplotypes in sealed boxes. The average lesion diameter was pictured at 6–8 days after inoculation (DAIs).
Cellular localization of Pi02860 effectors was investigated on N. benthamiana plants by confocal imaging. In this analysis, N. benthamiana cells were imaged at 2 DAIs using a Nikon Ti-E inverted microscope with Plan Apo 40X/0.95 dry objective. GFP was excited by 488-nm light shed from an argon laser, and 500–530 nm emissions were captured. To minimize potential artifacts of ectopic protein expression, only single optical images from leaf cells expressing low levels of the protein fusions were collected and analysed.
Analyses of thermal-mediated Pi02860 expression
Fifteen P. infestans isolates with different Pi02860 haplotypes were inoculated onto the detached leaves of the universal susceptible potato cultivar at low (10°C), near optimum (18°C) (near optimum) and high (25°C) temperature of the pathogen infection (Yang et al., 2016a). Three 6-mm leaf disks were excised from the inoculation sites 2 DAIs. RNA was extracted from the leaf disks using the EasyPure plant RNA Kits (TransGen Biotech) according to the manufacturer’s instructions. RNA was quantified using a Nanodrop 1000 (Thermo Scientific) and cDNA was synthesized using TransScript One-Step gDNA Removal and cDNA Synthesis SuperMix (TransGen Biotech) according to the manufacturer’s instructions. QRT-PCR was performed using TransStart Top Green qPCR SuperMix (TransGen Biotech) and run on a QuantStudio 5 Real-Time PCR Instrument (Applied Biosystems) using QuantStudio Design & Analysis software v1.4.2 with specific primers (For: GTGTCGCCTGGTCTAATCC, Rev: TTCTCTCTTCATTGGCTTCG). The expression of the effector gene was analyzed using the ΔΔCt method (McLellan et al., 2013) normalized with the housekeeping gene ActinA.
Data analyses
Before analysis, all Pi02860 nucleotide sequences were visually assessed to remove potential artifacts (Yang et al., 2013). Pi02860 isoforms were deduced from nucleotide sequences and multiple sequence alignments were performed by the ClustalW implemented in MEGA 7.0.21 (Kumar et al., 2016). Nucleotide haplotypes were reconstructed with the PHASRE algorithm implemented in DnaSP 5.10 (Librado and Rozas, 2009). The DnaSP 5.10 program was also used to estimate haplotype diversity, nucleotide diversity, the rates of non-synonymous substitutions and synonymous substitutions. Haplotype and nucleotide diversities were estimated for each of the five populations (elevations) as well as the combined population by pooling the sequences for individual populations. A median joining (MJ) network illustrating genealogical relationships among haplotypes was generated using PopART 1.7 (Leigh and Bryant, 2015). The population scaled recombination rates were assessed by INTERVAL program implemented in LDHAT package at the interface of RDP4 (McVean et al., 2002, 2004). The mean lesion size (INF1 cell death suppression) in each population was calculated with the formula as follows:
where wi and pi represent the frequency and the observed lesion size (INF1 cell death suppression) of haplotype i and Y is the mean lesion size (INF1 cell death suppression) in the population. Associations of sequence diversity with biological characteristics of the effector gene and altitude were evaluated by Pearson’s correlation (Lawrence and Lin, 1989). Duncan’s multiple range and LSD tests (Ott and Longnecker, 2015) were applied to determine the differences in lesion size, INF1 induced cell death and gene expression using the SAS software. A Chi-square test for haplotype homogeneity among different altitudes was conducted by SPSS 19.0.
Results
Sequence variation in Pi02860 effector gene
A total of 35 variable sites were detected in the 354 full nucleotide sequences, representing 3–23 sites from each of the five populations (Table 1). These variable sites formed 21 nucleotide haplotypes. Nearly all sequence variations were generated by point mutations (Supplementary Table 1). Besides that, an early termination stop codon was generated by point mutation at the 107th nucleotide in Hap_8, causing truncation within the RXLR motif (Figure 1; Supplementary Table 1). Pseudo genes generated by deletions in the start codon were found in two isolates (Supplementary Table 1).
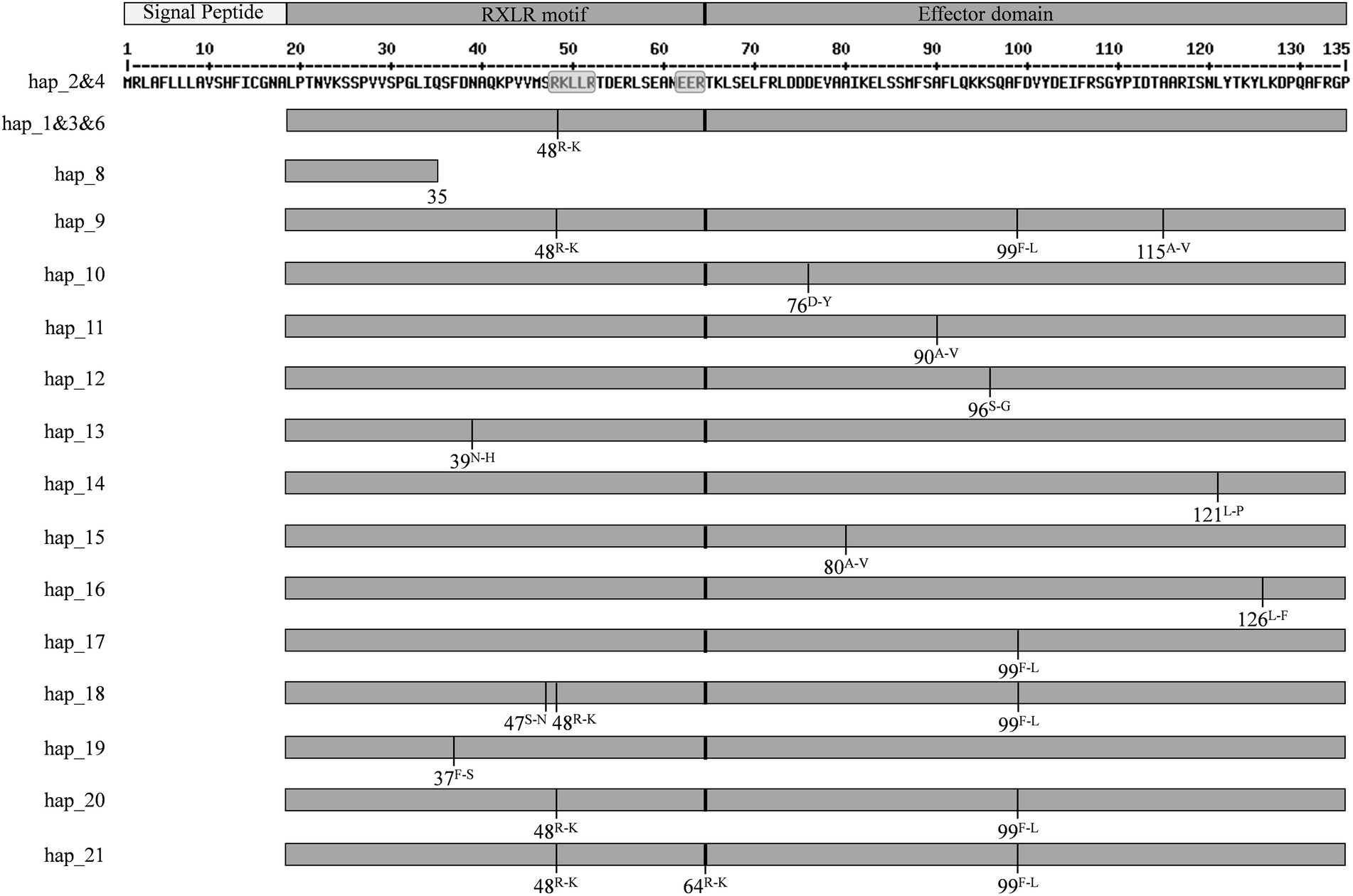
Figure 1. Sketch showing the Pi02860 structural domain and the mutation position in each of nucleotide haplotypes. Hap_5 and Hap_7 were removed due to a mutation in start codon.
Altitudinal distribution of Pi02860 gene
Among the 21 nucleotide haplotypes, Hap_1 to Hap_4 were major haplotypes with a frequency ranging from 0.00 to 62.79% in individual populations (Supplementary Table 2) and ranging from 2.26 to 54.24% in the combined population. Hap_1, Hap_2, and Hap_3 were observed in all five populations while Hap_4, was observed in four of the five populations (Figure 2). Other haplotypes were detected only once and were private to each population (Table 1; Figure 2). The pathogen populations from different altitudes varied significantly in the frequency of both nucleotide haplotypes (Supplementary Table 2) and isoforms (Supplementary Table 3) by the homogeneity test, with a value of p of 0.009 and 0.044, respectively. The haplotype diversity of nucleotide sequences in the five populations ranged from 0.5499 to 0.6791 with a mean of 0.6240 when sequences from the five sites were combined. Nucleotide diversity in the populations ranged from 0.00165 to 0.00274 with a grand mean of 0.00231 (Table 1). Populations collected from the lowest altitudes (A and B) had the least number of haplotypes and lowest haplotype nucleotide diversity while population E from the highest altitude displayed the greatest number of haplotypes and highest nucleotide diversity (Table 1).
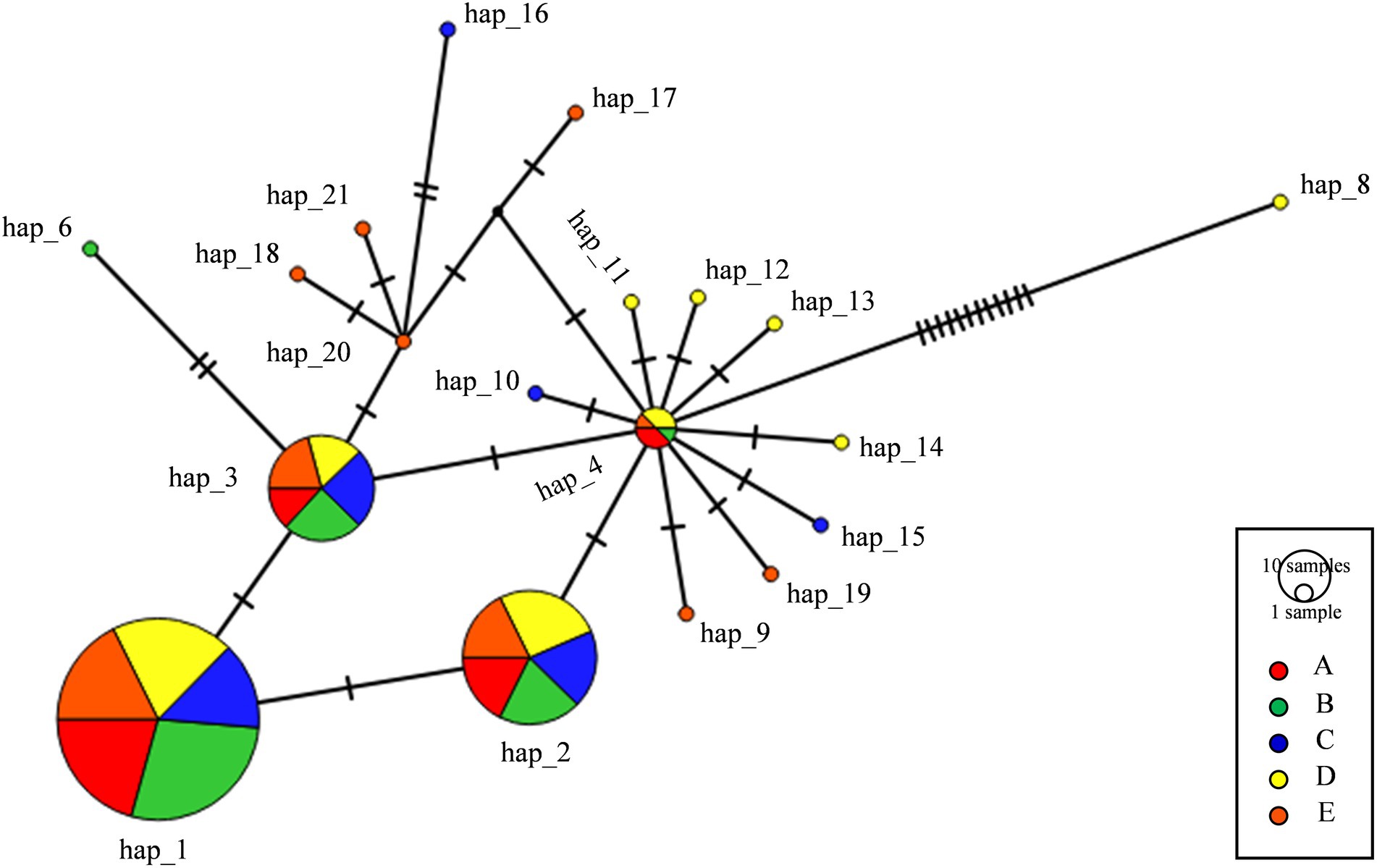
Figure 2. Haplotype network of Pi02860 gene. Nucleotide haplotypes are named with Hap followed by a number. Size of the circles indicates haplotype frequencies in the population. Colors correspond to the respective populations. Each tick mark represents a step of nucleotide substitution. Hap_5 and Hap_7 have a mutation in start codon and were removed from haplotype network construction.
Haplotype network
Hap_4 was identical to T30-4, the reference sequence. Hap_1, Hap_2 and Hap_3 had one SNP in 30T-C or 143G-A compared to the reference sequence (Supplementary Table 1). Due to some synonymous mutations, Hap_1 and Hap_3 were translated to the same amino acid isoform, accounting for 68.93% of the combined population while Hap_2 and Hap_4 were translated to another isoform, accounting for 26.27% of the combined population (Supplementary Table 3). All dominant haplotypes were one or two steps away from each other except Hap_8 which has an early termination at the 36th amino acid position and some rare haplotypes (Figure 2).
Association between sequence variation and altitude
Haplotype richness of Pi02860 was positively associated with altitude (p = 0.049, Figure 3A). The haplotype diversity and nucleotide diversity of the effector gene were also positively correlated with altitude, with a value of p of 0.037 and 0.013, respectively (Figures 3B,C). Both synonymous mutation (dS) and non-synonymous mutation (dN) rates were positively correlated with altitude, but only the correlation with synonymous mutation was significant (Figures 3D,E). Population recombination rate was negatively correlated with altitude but was not significant (Figure 3F).
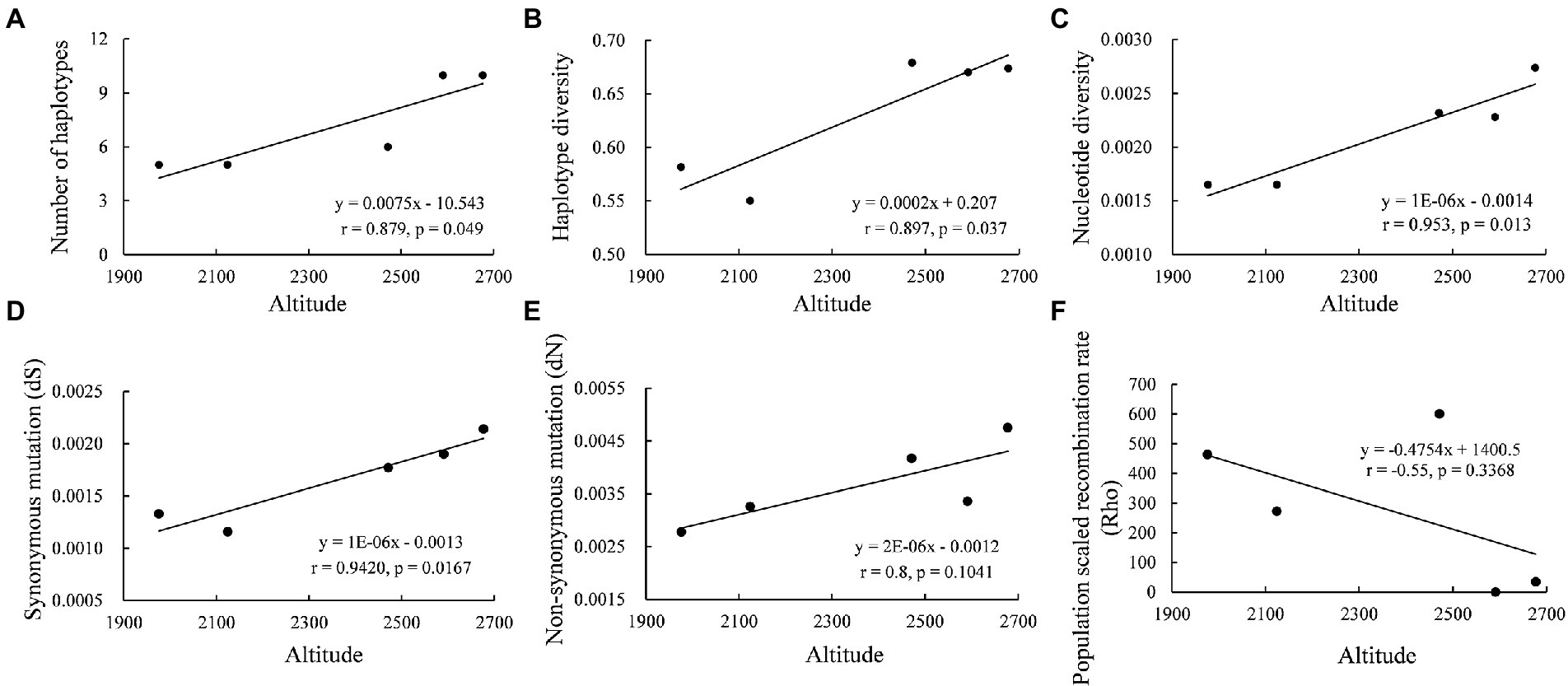
Figure 3. Correlations between the altitude of the collection sites of the pathogen and the genetic variation of Pi02860 gene: (A) the number of haplotypes; (B) haplotype diversity; (C) nucleotide diversity; (D) synonymous mutation; (E) nonsynonymous mutation; (F) population scaled recombination rate of Pi02860 and altitude.
The subcellular localization of Pi02860 haplotypes
Sixteen vectors were constructed to transiently express Pi02860 haplotypes without a signal peptide in N. benthamiana for subcellular localization analysis. Two of the 21 haplotypes which are considered as pseudogenes due to a point mutation in the start codon were removed from the cellular localization analysis. Three haplotypes (Hap_1, Hap_3, and Hap_6) translate into one identical isoform and two haplotypes (Hap_2 and Hap_4) translate into another identical isoform (Figure 1). Only one vector was constructed for each of these two isoforms. Confocal images showed that non-synonymous mutation at these nucleotide sites (37th, 39th, 47th, 48th, 64th, 76th, 80th, 90th, 96th, 99th, 115th, 121st and 126th) did not change the subcellular localization of the Pi02860 effector. All GFP-tagged Pi02860 haplotypes were found to localize in the cytoplasm and nucleus (Figure 4).
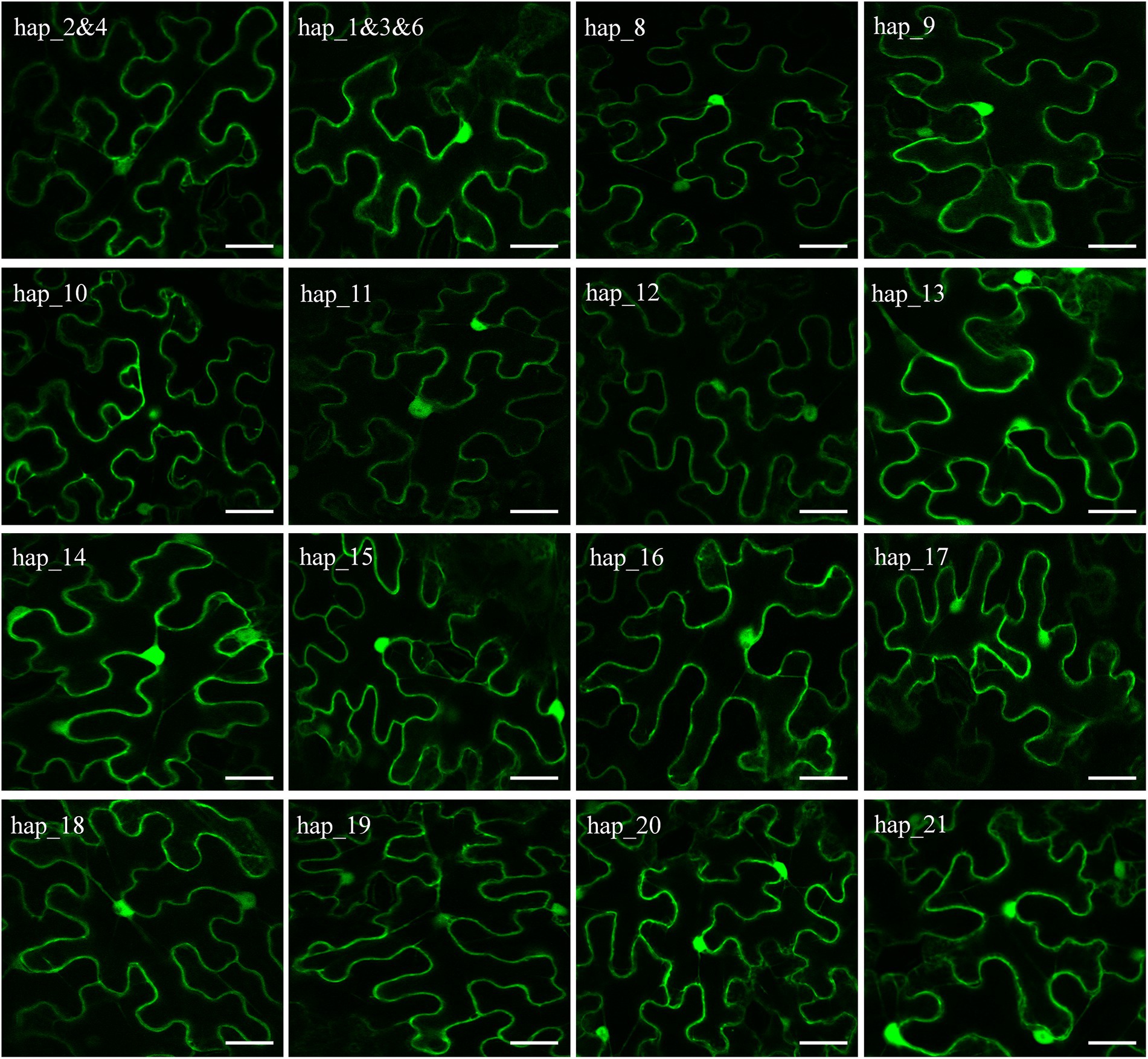
Figure 4. Confocal images showing that GFP-Pi02860 effectors are localized in the cytoplasm and nucleus. Scale bars are 20 μm.
Virulence analysis of Pi02860 haplotypes
GFP-tagged expression revealed all Pi02860 haplotypes including the truncated haplotype promoted the lesion size of co-expressed host N. benthamiana and there was also a significant difference in pathogenicity among the effector haplotypes (Figure 5). Hap_2 and Hap_4, which translate into isoform identical to the wild type Pi02860 effector (T30-4), produced lesions larger than Hap_8, Avr3a and the negative control (EV). Hap_1, Hap_3 and Hap_6 which have one SNP in 48K-R compared to the wild type Pi02860 effector, as well as other haplotypes except Hap_8, produced larger lesions than Hap_2 and Hap _4 did. Hap_8, which has a terminal truncation starting at the 36th amino acid position, produced significantly smaller lesions than other haplotypes including Avr3a (Figure 5). The altitude of collection site was negatively associated with the lesion size of the pathogen but was not significant (Figure 6A).
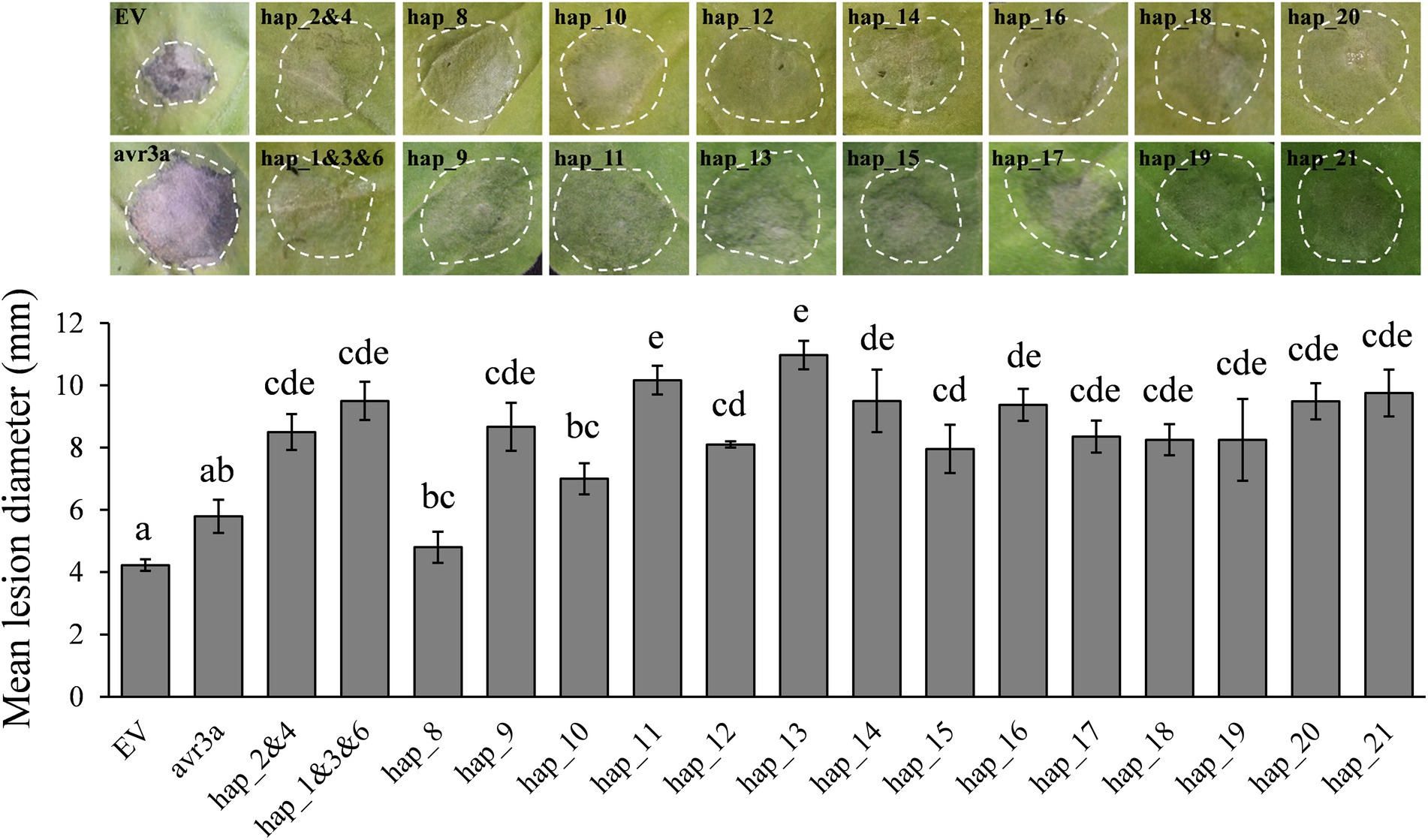
Figure 5. Transient overexpression of Pi02860 haplotypes in N. benthamiana increases P. infestans lesion diameter to various degrees compared with free GFP. Bottom: statistical test; Top: representative images of pathogen expression. EV and avr3a are negative and positive controls, respectively. Hap_1 to Hap_21 are the codes of 21 haplotypes detected. Hap_2 and 4 are deduced into the same isoform and Hap_1, 3 and 6 are deduced into another the same isoform, thus only one vector was constructed for each of the two haplotype groups. Results shown are derived from the mean of at least three independent biological replicates.
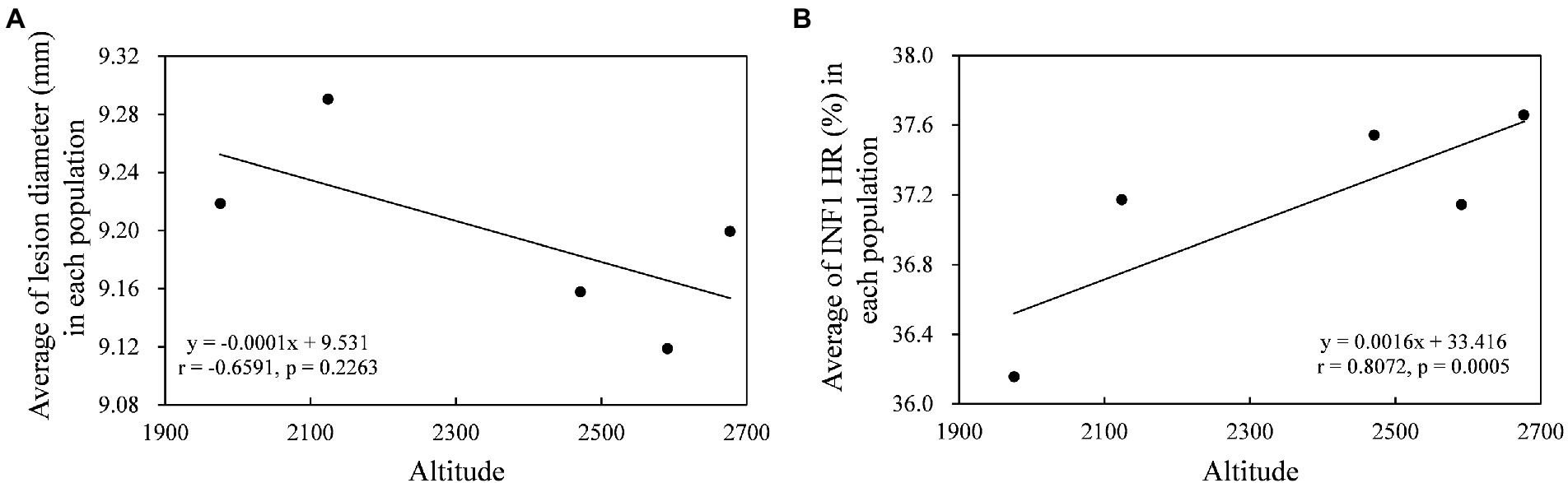
Figure 6. Correlation between the altitude of the collection sites and lesion size (A) and INF1 suppression (B) of pathogen population.
Pi02860 haplotypes varied significantly in suppressing INF1 cell death (Figure 7). Although there was no significantly correlation, the suppression of INF1 cell death caused by effector haplotypes largely mirrored the lesion sizes they produced on N. benthamiana (Figures 5, 7). In this expression analysis, Hap_2 and Hap _4 had the strongest suppression on cell death elicited by INF1, followed by Hap_1 Hap _3 and Hap _6, the most dominant Pi02860 effector haplotypes. Hap_8 had nearly no effect on INF1 cell death suppression. The average of INF1 cell death suppression in the P. infestans populations was positively and significantly correlated with altitude (Figure 6B).
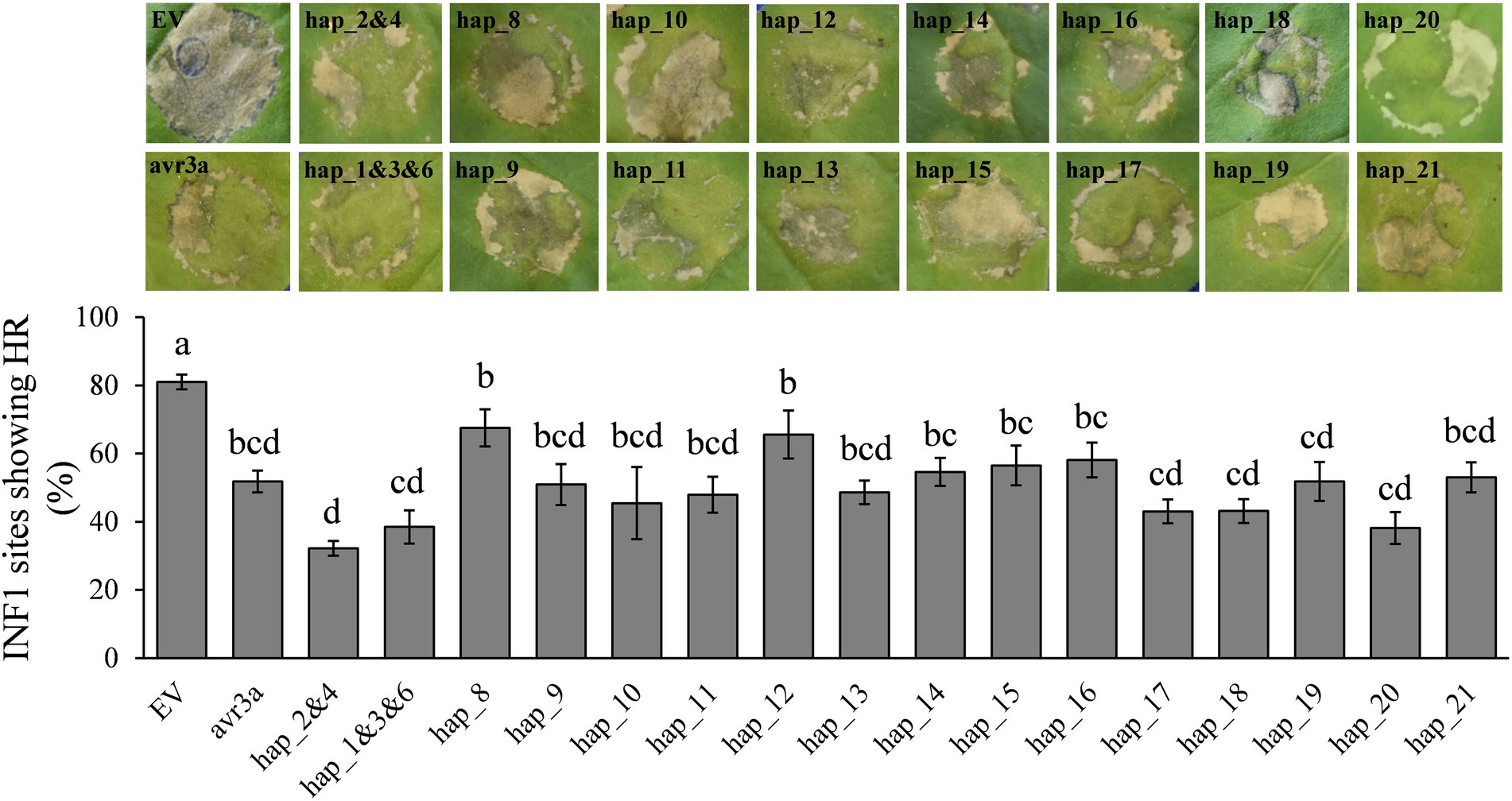
Figure 7. Transient overexpression of GFP-Pi02860 haplotypes in N. benthamiana suppresses the HR (hypersensitive response) triggered by the elicitin INF1. Top: images of pathogen expression: Bottom: statistical test. EV and avr3a are negative and positive controls, respectively. Hap_1 to Hap_21 are the codes of 21 haplotypes detected. Hap_2 and 4 are deduced into the same isoform and Hap_1, 3 and 6 are deduced into another the same isoform, thus only one vector was constructed for each of the two haplotype groups. Results shown are derived from the mean of at least three independent biological replicates.
Thermal-mediated expression polymorphisms in Pi02860 effector gene
QRT-PCR analysis of Pi02860 expression at 2DAIs on susceptible potato plants showed that the transcription accumulation varied substantially among isolates with different effector haplotypes and among experimental temperatures (Figure 8). In all but isolate DQS9-14 of Hap_2, AH8-7 of Hap_3, DQS9-1 of Hap_12 and BKY-2 of Hap_6, Pi02860 effector was expressed mostly at 10°C, followed by at 18°C. The effector was least expressed at 25°C. At 18°C, the nearly optimum infection temperature of the pathogen, the expression of Pi02860 gene in seven isolates was upregulated >3 times with the highest upregulation of ~20 times in isolate BHYW-9 of Hap_1 whereas the expression in other isolates was <2 times (Figure 8). At 10°C, seven isolates were upregulated for ~20 times with the most upregulation of >300 times in isolate CHZ-62 of Hap_11. At 25°C, only three isolates were upregulated >2 times.
Discussion
Due to their critical roles in host-pathogen interactions, pathogen effectors are important proteins regulating disease initiation and epidemics in natural and agricultural ecosystems (Uhse and Djamei, 2018). Population genetic analysis of effector genes would provide useful insights on how these important proteins evolve and what ecological functions they play. In addition to their roles in regulating host pathogen interaction, it has been documented that effector genes also have other ecological functions such as antimicrobial activities (Snelders et al., 2020). To maintain these multifaceted functions, effector genes are expected to have a higher evolvability equipped by high genetic variation for quick adaptation to constantly changing biotic and abiotic environments. Indeed, previous studies have reported that the genetic variation in effector genes is higher than many other functional genes in P. infestans and other pathogen species, possibly due to a synergic effect of recombination (Yang et al., 2018; Dale et al., 2019), plasticity (Timilsina et al., 2020) and multiple mutation mechanisms including pseudogenization, point mutation, deletion, insertion and truncation (Shen et al., 2021; Waheed et al., 2021). The physical locations of many effector genes in the sparse regions of genomes enriching with transposable elements provide unique opportunity for mutations to occur with a minimum fitness penalty to pathogen species (Haas et al., 2009; Dong et al., 2015). In this study, we found >20 nucleotide haplotypes in ~350 Pi02860 sequences analysed. This is much higher than the genetic variation of other functional genes in this species. For example, we only identified < 10 nucleotide haplotypes in the 165 eEF1a sequences (Wang et al., 2021b) and two nucleotide haplotypes in the 140 ATP6 sequences (Zhang et al., 2017). However, the genetic variation found in Pi02860 is much lower than other effector genes of the pathogen such as Avr1, Avr2, Avr3a and Avr4 (Yang et al., 2018, 2020; Shen et al., 2021; Waheed et al., 2021). In addition, only point mutation and deletion were found in the current study. On the other hand, many variation mechanisms including pseudogenization, point mutation, deletion, insertion and truncation were found in Avr1, Avr2, Avr3a and Avr4 of P. infestans.
These differences in genetic variation and mutation mechanisms between the Pi02860 and other effector genes of P. infestans may reflect differences in interactions with host resistance genes. The corresponding resistance genes of effectors Avr1, Avr2, Avr3a and Avr4 in our previous publications have been used commercially over a wide spatial scale in the past decades. Due to the constant interaction with host immunity systems, it is expected that this group of effector genes have become equipped with higher genetic variation to maintain the pathogen’s co-evolutionary pace with the host. On the other hand, Pi02860 is only a candidate effector gene with some documented functions (Yang et al., 2016b; He et al., 2018) of regulating host immunity responses. No corresponding resistance gene has been identified in the potato host or has been used commercially. In this case, standing genetic variation in the effector gene is not essential for reproduction and transmission of the pathogen and therefore would be gradually purged out either due to random genetic drift (Lynch et al., 2016) or genetic load (Kirkpatrick and Jarne, 2000) incurred to the pathogen in its presence. It is documented that plant pathogens have optimized their effector reservoirs over their co-evolution journey with their hosts. Some effectors play a more important and common role in pathogen virulence than others. This group of effectors are more conserved in sequence structure and are regard as core effectors (Chepsergon et al., 2021). It is possible that Pi02860 is also one of such core effectors.
We found mutations in the effector domain of Pi02860 did not change its subcellular localization. The effectors were only found in the nucleus and cytoplasm, confirming that Pi02860 is a cytoplasmic effector (Yang et al., 2016b). However, mutations in this region dramatically affect the fitness of the pathogen as indicated by the statistical difference among the nucleotide haplotypes in their ability to suppress the host’s immunity response and the amount of disease they produced (Figures 5, 7). Overall, these mutations reduce the ability of the pathogen to inhibit host immunity responses, leading to less disease even though the positive correlation between the two parameters is not significant (Figure 6). This reduction in immunity suppression is more obvious for the haplotype with the C-terminal truncation (Hap_8), further suggesting that Pi02860 may be a core effector which is crucial for P. infestans pathogenicity and its loss from the genome may lead to a severe fitness penalty to the pathogen.
In addition to host resistance, other ecological factors may also contribute to the evolution of effector genes. Previously, it was reported that microbial community structure in the plant root rhizosphere can affect the evolutionary outcome of effector genes in a plant pathogen (Bever et al., 2012). Here, we found that abiotic factors may also contribute to the evolution of the effector gene in P. infestans. Genetic variation of Pi02860 in terms of haplotype richnesss, haplotype diversity and nucleotide diversity increased as the altitude of the sample collection sites increased (Figures 2B,C, 3A). This spatial pattern of genetic variation is also linked to the fitness of the pathogen (Figure 6). Mutation rate, particularly the synonymous mutation rate, also increased as the altitude of the collection site increased (Figures 3D–F). Because no resistance gene corresponding to Pi02860 was used in the fields and the isolates included in the study were sampled from the same potato variety on the same day at the same region, the observed difference in the genetic variation among altitudinal Pi02860 effector is unlikely to have been caused by natural selection for host adaptation. Rather, it more likely reflects an elevated mutation rate caused by the vertical distribution of climatic conditions such as UV radiation and temperature. UV radiation is a major mutagenic agent which promotes the generation of genetic variation in species by inducing structural change of genetic material in the genome. For example, the mutation rate in bacteria increased hundreds of times after exposed to UV radiation (Shibai et al., 2017). In clear skies, UV radiation increases by 10–20% in response to a 1,000 m increase in altitude (Blumthaler et al., 1992; Schmucki and Philipona, 2002; Narayanan et al., 2010).
Temperature is an important environmental factor with crucial impacts on nearly all biological and evolutionary processes of species (Townsley and Yildiz, 2015; Huot et al., 2017; Garbelotto et al., 2021). Recently, it was reported that temperature can regulate the population genetic structure and evolution of effector genes (Velásquez et al., 2018; Waheed et al., 2021). In the current study, we found the expression of Pi02860 was regulated by experimental temperatures. The effector gene demonstrated a higher expression level at a lower temperature (10°C) compared to the near optimum infection temperature of the pathogen (~19°C), and the expression level was further reduced as the experimental temperature increased to 25°C (Figure 8). Air temperature at the earth surface is negatively correlated with altitude (Legates and Willmott, 1990) and it is possible that changes in temperature may also contribute to the observed association of genetic variation in Pi02860 with altitude. Recombination increases genetic variation of species by reshuffling genetic material among genomes and reducing the purging effect of natural selection (Gossmann et al., 2014). Both UV radiation and temperature can affect the recombination rate of species (Boyko et al., 2005; Shibai et al., 2017). Recombination events regulated by vertical distributions of temperature may also contribute to the observed association of genetic variation with altitude, but this hypothesis is rejected by the association analysis between recombination rate and the altitudes of collection sites. We found neither putative recombination events by the seven algorithms (RDP, GENECONV, Bootscan, MaxChi, Chimaera, SiScan and 3Seq) embedded in the RDP4 suite (Martin et al., 2015) nor association between recombination rate and altitude. However, intragenic recombination has been documented in several other effector genes of the pathogen sampled from the region (Yang et al., 2020; Shen et al., 2021; Waheed et al., 2021).
Many genetic and ecological factors influence the generation and maintenance of genetic variation. To minimize this effect, we sampled from the same potato variety along a hill on the same day. Our results indicate that in additional to host, climatic conditions associated with altitudinal distribution also contribute to the evolution of effector gene Pi02860. It has to point out that only one effector was reported in the current manuscript, further studies involving more effector genes and pathogen species are needed to confirm the altitudinal pattern. However, our results are complementary to other evolutionary phenomena that also show altitudinal effect on pathogen evolution at both the genic and organismic level (Santini and Ghelardini, 2015; Wang et al., 2021a). Further studies involving more effector genes are needed and may therefore, have many important implications for future agricultural and ecological sustainability caused by ongoing climate changes.
Data availability statement
The Pi02860 haplotype sequence data presented in the study has been deposited in NCBI. The GenBank accession numbers of hap_1, 2, 3, 4, 6, 9, 10, 11, 12, 13, 14, 15, 16, 17, 18, 19, 20, 21 are OP222576, OP222577, OP222578, OP222579, OP222580, OP222581, OP222582, OP222583, OP222584, OP222585, OP222586, OP222587, OP222588, OP222589, OP222590, OP222591, OP222592, and OP222593. The GenBank accession numbers of hap_5, 7, 8 are OP222594, OP222595, and OP222596.
Author contributions
L-NY and HO performed the experiments, analysed data and wrote the manuscript. ON, AW, and Y-PW collected pathogen isolates. HF and WL genotyped pathogen isolates. JZ conceived, designed and supervised the experiments, analysed the data and wrote the manuscript. All authors contributed the article and approved the submitted version.
Funding
This work was supported by the National Natural Science Foundation of China (grant no. 31901861).
Acknowledgments
We thank Jeremy Burdon for comments and language proofreading.
Conflict of interest
The authors declare that the research was conducted in the absence of any commercial or financial relationships that could be construed as a potential conflict of interest.
The reviewer RV declared a shared affiliation with one of the authors JZ to the handling editor at the time of review.
Publisher’s note
All claims expressed in this article are solely those of the authors and do not necessarily represent those of their affiliated organizations, or those of the publisher, the editors and the reviewers. Any product that may be evaluated in this article, or claim that may be made by its manufacturer, is not guaranteed or endorsed by the publisher.
Supplementary material
The Supplementary material for this article can be found online at: https://www.frontiersin.org/articles/10.3389/fmicb.2022.972928/full#supplementary-material
References
Bais, A. F., McKenzie, R. L., Bernhard, G., Aucamp, P. J., Ilyas, M., Madronich, S., et al. (2015). Ozone depletion and climate change: impacts on UV radiation. Photochem. Photobiol. Sci. 14, 19–52. doi: 10.1039/C4PP90032D
Bever, J. D., Platt, T. G., and Morton, E. R. (2012). Microbial population and community dynamics on plant roots and their feedbacks on plant communities. Annu. Rev. Microbiol. 66, 265–283. doi: 10.1146/annurev-micro-092611-150107
Blumthaler, M., Ambach, W., and Rehwald, W. (1992). Solar UV-A and UV-B radiation fluxes at two alpine stations at different altitudes. Theor. Appl. Climatol. 46, 39–44. doi: 10.1007/BF00866446
Boevink, P. C., McLellan, H., Gilroy, E. M., Naqvi, S., He, Q., Yang, L., et al. (2016). Oomycetes seek help from the plant: Phytophthora infestans effectors target host susceptibility factors. Mol. Plant 9, 636–638. doi: 10.1016/j.molp.2016.04.005
Boutemy, L. S., King, S. R., Win, J., Hughes, R. K., Clarke, T. A., Blumenschein, T. M., et al. (2011). Structures of phytophthora RXLR effector proteins a conserved but adaptable fold underpins functional diversity. J. Biol. Chem. 286, 35834–35842. doi: 10.1074/jbc.M111.262303
Boyko, A., Filkowski, J., and Kovalchuk, I. (2005). Homologous recombination in plants is temperature and day-length dependent. Mutat. Res. Fundam. Mol. Mech. Mutagen. 572, 73–83. doi: 10.1016/j.mrfmmm.2004.12.011
Burdon, J. J., Barrett, L., Yang, L. N., He, D. C., and Zhan, J. (2020). Maximizing world food production through disease control. Bioscience 70, 126–128. doi: 10.1093/biosci/biz149
Cavicchioli, R., Ripple, W. J., Timmis, K. N., Azam, F., Bakken, L. R., Baylis, M., et al. (2019). Scientists’ warning to humanity: microorganisms and climate change. Nat. Rev. Microbiol. 17, 569–586. doi: 10.1038/s41579-019-0222-5
Chepsergon, J., Motaung, T. E., and Moleleki, L. N. (2021). “Core” RxLR effectors in phytopathogenic oomycetes: a promising way to breeding for durable resistance in plants? Virulence 12, 1921–1935. doi: 10.1080/21505594.2021.1948277
Dale, A. L., Feau, N., Everhart, S. E., Dhillon, B., Wong, B., Sheppard, J., et al. (2019). Mitotic recombination and rapid genome evolution in the invasive forest pathogen Phytophthora ramorum. mBio 10, e02452-18. doi: 10.1128/mBio.02452-18
Dong, S., Raffaele, S., and Kamoun, S. (2015). The two-speed genomes of filamentous pathogens: waltz with plants. Curr. Opin. Genet. Dev. 35, 57–65. doi: 10.1016/j.gde.2015.09.001
Franceschetti, M., Maqbool, A., Jiménez-Dalmaroni, M. J., Pennington, H. G., Kamoun, S., and Banfield, M. J. (2017). Effectors of filamentous plant pathogens: commonalities amid diversity. Microbiol. Mol. Biol. Rev. 81, e00066-16. doi: 10.1128/MMBR.00066-16
Fry, W. (2008). Phytophthora infestans: the plant (and R gene) destroyer. Mol. Plant Pathol. 9, 385–402. doi: 10.1111/j.1364-3703.2007.00465.x
Garbelotto, M., Schmidt, D., and Popenuck, T. (2021). Pathogenicity and infectivity of Phytophthora ramorum vary depending on host species, infected plant part, inoculum potential, pathogen genotype, and temperature. Plant Pathol. 70, 287–304. doi: 10.1111/ppa.13297
Gossmann, T. I., Santure, A. W., Sheldon, B. C., Slate, J., and Zeng, K. (2014). Highly variable recombinational landscape modulates efficacy of natural selection in birds. Genome Biol. Evol. 6, 2061–2075. doi: 10.1093/gbe/evu157
Haas, B. J., Kamoun, S., Zody, M. C., Jiang, R. H., Handsaker, R. E., Cano, L. M., et al. (2009). Genome sequence and analysis of the Irish potato famine pathogen Phytophthora infestans. Nature 461, 393–398. doi: 10.1038/nature08358
He, Q., Naqvi, S., McLellan, H., Boevink, P. C., Champouret, N., Hein, I., et al. (2018). Plant pathogen effector utilizes host susceptibility factor NRL1 to degrade the immune regulator SWAP70. Proce. Natl. Acad. Sci. 115, E7834–E7843. doi: 10.1073/pnas.1808585115
Huot, B., Castroverde, C. D. M., Velásquez, A. C., Hubbard, E., Pulman, J. A., Yao, J., et al. (2017). Dual impact of elevated temperature on plant defence and bacterial virulence in Arabidopsis. Nat. Commun. 8, 1808–1812. doi: 10.1038/s41467-017-01674-2
Juroszek, P., Racca, P., Link, S., Farhumand, J., and Kleinhenz, B. (2020). Overview on the review articles published during the past 30 years relating to the potential climate change effects on plant pathogens and crop disease risks. Plant Pathol. 69, 179–193. doi: 10.1111/ppa.13119
Kirkpatrick, M., and Jarne, P. (2000). The effects of a bottleneck on inbreeding depression and the genetic load. Am. Nat. 155, 154–167. doi: 10.1086/303312
Körner, C. (2007). The use of ‘altitude’ in ecological research. Trends Ecol. Evol. 22, 569–574. doi: 10.1016/j.tree.2007.09.006
Kumar, S., Stecher, G., and Tamura, K. (2016). MEGA7: molecular evolutionary genetics analysis version 7.0 for bigger datasets. Mol. Biol. Evol. 33, 1870–1874. doi: 10.1093/molbev/msw054
Lawrence, I., and Lin, K. (1989). A concordance correlation coefficient to evaluate reproducibility. Biometrics 45, 255–268. doi: 10.2307/2532051
Legates, D. R., and Willmott, C. J. (1990). Mean seasonal and spatial variability in global surface air temperature. Theor. Appl. Climatol. 41, 11–21. doi: 10.1007/BF00866198
Leigh, J. W., and Bryant, D. (2015). Popart: full-feature software for haplotype network construction. Methods Ecol. Evol. 6, 1110–1116. doi: 10.1111/2041-210X.12410
Librado, P., and Rozas, J. (2009). DnaSP v5: a software for comprehensive analysis of DNA polymorphism data. Bioinformatics 25, 1451–1452. doi: 10.1093/bioinformatics/btp187
Lo Presti, L., Lanver, D., Schweizer, G., Tanaka, S., Liang, L., Tollot, M., et al. (2015). Fungal effectors and plant susceptibility. Annu. Rev. Plant Biol. 66, 513–545. doi: 10.1146/annurev-arplant-043014-114623
Lynch, M., Ackerman, M. S., Gout, J. F., Long, H., Sung, W., Thomas, W. K., et al. (2016). Genetic drift, selection and the evolution of the mutation rate. Nat. Rev. Genet. 17, 704–714. doi: 10.1038/nrg.2016.104
Martin, D. P., Murrell, B., Golden, M., Khoosal, A., and Muhire, B. (2015). RDP4: detection and analysis of recombination patterns in virus genomes. Virus Evol. 1:vev003. doi: 10.1093/ve/vev003
Martín, J. A., Witzell, J., Blumenstein, K., Rozpedowska, E., Helander, M., Sieber, T. N., et al. (2013). Resistance to Dutch elm disease reduces presence of xylem endophytic fungi in elms (Ulmus spp.). PLoS One 8:e56987. doi: 10.1371/journal.pone.0056987
McLellan, H., Boevink, P. C., Armstrong, M. R., Pritchard, L., Gomez, S., Morales, J., et al. (2013). An RxLR effector from Phytophthora infestans prevents re-localisation of two plant NAC transcription factors from the endoplasmic reticulum to the nucleus. PLoS Pathog. 9:e1003670. doi: 10.1371/journal.ppat.1003670
McVean, G, Awadalla, P., and Fearnhead, P. (2002). A coalescent-based method for detecting and estimating recombination from gene sequences. Genetics 160, 1231–1241. doi: 10.1093/genetics/160.3.1231
McVean, G. A., Myers, S. R., Hunt, S., Deloukas, P., Bentley, D. R., and Donnelly, P. (2004). The fine-scale structure of recombination rate variation in the human genome. Science 304, 581–584. doi: 10.1126/science.1092500
Narayanan, D. L., Saladi, R. N., and Fox, J. L. (2010). Ultraviolet radiation and skin cancer. Int. J. Dermatol. 49, 978–986. doi: 10.1111/j.1365-4632.2010.04474.x
Ott, R. L., and Longnecker, M. T. (2015). An introduction to statistical methods and data analysis Cengage Learning. Boston: Duxbury Press.
Raffaele, S., and Kamoun, S. (2012). Genome evolution in filamentous plant pathogens: why bigger can be better. Nat. Rev. Microbiol. 10, 417–430. doi: 10.1038/nrmicro2790
Raum, S. (2020). Land-use legacies of twentieth-century forestry in the UK: a perspective. Landsc. Ecol. 35, 2713–2722. doi: 10.1007/s10980-020-01126-1
Ren, F., Yan, D. H., Wu, G., Sun, X., Song, X., and Li, R. (2020). Distinctive gene expression profiles and effectors consistent with host specificity in two formae speciales of Marssonina brunnea. Front. Microbiol. 11:276. doi: 10.3389/fmicb.2020.00276
Santini, A., and Ghelardini, L. (2015). Plant pathogen evolution and climate change. CABI Rev. 2015, 1–8. doi: 10.1079/PAVSNNR201510035
Schmucki, D. A., and Philipona, R. (2002). Ultraviolet radiation in the Alps: the altitude effect. Opt. Eng. 41, 3090–3095. doi: 10.1117/1.1516820
Shen, L. L., Wheed, A., Wang, Y. P., Nkurikiyimfura, O., Wang, Z. H., Yang, L. N., et al. (2021). Multiple mechanisms drive the evolutionary adaptation of Phytophthora infestans effector Avr1 to host resistance. J. Fungi 7, 789. doi: 10.3390/jof7100789
Shibai, A., Takahashi, Y., Ishizawa, Y., Motooka, D., Nakamura, S., Ying, B. W., et al. (2017). Mutation accumulation under UV radiation in Escherichia coli. Sci. Rep. 7, 14531–14512. doi: 10.1038/s41598-017-15008-1
Snelders, N. C., Rovenich, H., Petti, G. C., Rocafort, M., van den Berg, G. C., Vorholt, J. A., et al. (2020). Microbiome manipulation by a soil-borne fungal plant pathogen using effector proteins. Nat. Plants 6, 1365–1374. doi: 10.1038/s41477-020-00799-5
Soyer, J. L., El Ghalid, M., Glaser, N., Ollivier, B., Linglin, J., Grandaubert, J., et al. (2014). Epigenetic control of effector gene expression in the plant pathogenic fungus Leptosphaeria maculans. PLoS Genet. 10:e1004227. doi: 10.1371/journal.pgen.1004227
Timilsina, S., Potnis, N., Newberry, E. A., Liyanapathiranage, P., Iruegas-Bocardo, F., White, F. F., et al. (2020). Xanthomonas diversity, virulence and plant–pathogen interactions. Nat. Rev. Microbiol. 18, 415–427. doi: 10.1038/s41579-020-0361-8
Tollefson, J. (2020). How hot will earth get by 2100? Nature 580, 443–445. doi: 10.1038/d41586-020-01125-x
Townsley, L., and Yildiz, F. H. (2015). Temperature affects c-di-GMP signalling and biofilm formation in Vibrio cholerae. Environ. Microbiol. 17, 4290–4305. doi: 10.1111/1462-2920.12799
Turnbull, D., Yang, L., Naqvi, S., Breen, S., Welsh, L., Stephens, J., et al. (2017). RXLR effector AVR2 up-regulates a brassinosteroid-responsive bHLH transcription factor to suppress immunity. Plant Physiol. 174, 356–369. doi: 10.1104/pp.16.01804
Uhse, S., and Djamei, A. (2018). Effectors of plant-colonizing fungi and beyond. PLoS Pathog. 14:e1006992. doi: 10.1371/journal.ppat.1006992
van Poppel, P. M., Guo, J., van de Vondervoort, P. J., Jung, M. W., Birch, P. R., Whisson, S. C., et al. (2008). The Phytophthora infestans avirulence gene Avr4 encodes an RXLR-dEER effector. Mol. Plant-Microbe Interact. 21, 1460–1470. doi: 10.1094/MPMI-21-11-1460
Velásquez, A. C., Castroverde, C. D. M., and He, S. Y. (2018). Plant–pathogen warfare under changing climate conditions. Curr. Biol. 28, R619–R634. doi: 10.1016/j.cub.2018.03.054
Vleeshouwers, V. G., Raffaele, S., Vossen, J. H., Champouret, N., Oliva, R., Segretin, M. E., et al. (2011). Understanding and exploiting late blight resistance in the age of effectors. Annu. Rev. Phytopathol. 49, 507–531. doi: 10.1146/annurev-phyto-072910-095326
Waheed, A., Wang, Y. P., Nkurikiyimfura, O., Li, W. Y., Liu, S. T., Lurwanu, Y., et al. (2021). Effector Avr4 in Phytophthora infestans escapes host immunity mainly through early termination. Front. Microbiol. 12:646062. doi: 10.3389/fmicb.2021.646062
Wang, Y., Bao, Z., Zhu, Y., and Hua, J. (2009). Analysis of temperature modulation of plant defense against biotrophic microbes. Mol. Plant Microbe Interact. 22, 498–506. doi: 10.1094/MPMI-22-5-0498
Wang, S., Boevink, P. C., Welsh, L., Zhang, R., Whisson, S. C., and Birch, P. R. (2017). Delivery of cytoplasmic and apoplastic effectors from Phytophthora infestans haustoria by distinct secretion pathways. New Phytol. 216, 205–215. doi: 10.1111/nph.14696
Wang, Q., Han, C., Ferreira, A. O., Yu, X., Ye, W., Tripathy, S., et al. (2011). Transcriptional programming and functional interactions within the Phytophthora sojae RXLR effector repertoire. Plant Cell 23, 2064–2086. doi: 10.1105/tpc.111.086082
Wang, Y. P., Waheed, A., Liu, S. T., Li, W. Y., Nkurikiyimfura, O., Lurwanu, Y., et al. (2021a). Altitudinal heterogeneity of UV adaptation in Phytophthorainfestans is associated with the spatial distribution of a DNA repair gene. J. Fungi 7, 245. doi: 10.3390/jof7040245
Wang, Y. P., Wu, E. J., Lurwanu, Y., Ding, J. P., He, D. C., Waheed, A., et al. (2021b). Evidence for a synergistic effect of post-translational modifications and genomic composition of eEF-1α on the adaptation of Phytophthora infestans. Ecol. Evol. 11, 5484–5496. doi: 10.1002/ece3.7442
Yaeno, T., Li, H., Chaparro-Garcia, A., Schornack, S., Koshiba, S., Watanabe, S., et al. (2011). Phosphatidylinositol monophosphate-binding interface in the oomycete RXLR effector AVR3a is required for its stability in host cells to modulate plant immunity. Proc. Natl. Acad. Sci. 108, 14682–14687. doi: 10.1073/pnas.1106002108
Yang, X., Chockalingam, S. P., and Aluru, S. (2013). A survey of error-correction methods for next-generation sequencing. Brief. Bioinform. 14, 56–66. doi: 10.1093/bib/bbs015
Yang, L. N., Liu, H., Duan, G. H., Huang, Y. M., Liu, S., Fang, Z. G., et al. (2020). The Phytophthora infestans AVR2 effector escapes R2 recognition through effector disordering. Mol. Plant Microbe Interact. 33, 921–931. doi: 10.1094/MPMI-07-19-0179-R
Yang, L. N., Liu, H., Wang, Y. P., Seematti, J., Grenville-Briggs, B. J., Wang, Z., et al. (2021). Pathogen-mediated stomatal opening: A previously overlooked pathogenicity strategy in the oomycete pathogen Phytophthora infestans. Front. Plant Sci. 12:668797. doi: 10.3389/fpls.2021.668797
Yang, L., McLellan, H., Naqvi, S., He, Q., Boevink, P. C., Armstrong, M., et al. (2016b). Potato NPH3/RPT2-like protein StNRL1, targeted by a Phytophthora infestans RXLR effector, is a susceptibility factor. Plant Physiol. 171, 645–657. doi: 10.1104/pp.16.00178
Yang, L., Ouyang, H. B., Fang, Z. G., Zhu, W., Wu, E. J., Luo, G. H., et al. (2018). Evidence for intragenic recombination and selective sweep in an effector gene of Phytophthora infestans. Evol. Appl. 11, 1342–1353. doi: 10.1111/eva.12629
Yang, L. N., Zhu, W., Wu, E. J., Yang, C., Thrall, P. H., Burdon, J. J., et al. (2016a). Trade-offs and evolution of thermal adaptation in the Irish potato famine pathogen Phytophthora infestans. Mol. Ecol. 25, 4047–4058. doi: 10.1111/mec.13727
Zhan, J., Thrall, P. H., and Burdon, J. J. (2014). Achieving sustainable plant disease management through evolutionary principles. Trends Plant Sci. 19, 570–575. doi: 10.1016/j.tplants.2014.04.010
Zhan, J., Thrall, P. H., Papaïx, J., Xie, L., and Burdon, J. J. (2015). Playing on a pathogen's weakness: using evolution to guide sustainable plant disease control strategies. Annu. Rev. Phytopathol. 53, 19–43. doi: 10.1146/annurev-phyto-080614-120040
Zhang, J. F., Wu, E. J., Luo, H. H., Zhu, W., and Zhan, J. (2017). Correlation analyses between ATP6 sequences of Phytophthora infestans and geographical factors. Jiangsu J. Agric. Sci. 45, 75–78. doi: 10.15889/j.issn.1002-1302.2017.08.021
Keywords: adaptation, agriculture, population genetics, plant pathogen, virulence factor, climate change, natural selection, molecular evolution
Citation: Yang L-N, Ouyang H, Nkurikiyimfura O, Fang H, Waheed A, Li W, Wang Y-P and Zhan J (2022) Genetic variation along an altitudinal gradient in the Phytophthora infestans effector gene Pi02860. Front. Microbiol. 13:972928. doi: 10.3389/fmicb.2022.972928
Edited by:
Qunqing Wang, Shandong Agricultural University, ChinaReviewed by:
Ramesh Raju Vetukuri, Swedish University of Agricultural Sciences, SwedenWeilin Cao, Shandong Agricultural University, China
Junliang Yin, Yangtze University, China
Copyright © 2022 Yang, Ouyang, Nkurikiyimfura, Fang, Waheed, Li, Wang and Zhan. This is an open-access article distributed under the terms of the Creative Commons Attribution License (CC BY). The use, distribution or reproduction in other forums is permitted, provided the original author(s) and the copyright owner(s) are credited and that the original publication in this journal is cited, in accordance with accepted academic practice. No use, distribution or reproduction is permitted which does not comply with these terms.
*Correspondence: Li-Na Yang, eWlrZXNodTExMTRAMTI2LmNvbQ==; Jiasui Zhan, Smlhc3VpLnpoYW5Ac2x1LnNl