- 1Laboratorio de Variación Biológica y Evolución, Departamento de Zoología, Escuela Nacional de Ciencias Biológicas, Instituto Politécnico Nacional, Mexico City, Mexico
- 2Laboratorio de Microbiología, Facultad de Zootecnia y Ecología, Universidad Autónoma de Chihuahua, Chihuahua, Mexico
- 3Department of Forest and Rangeland Stewardship, Warner College of Natural Resources, Colorado State University, Fort Collins, CO, United States
- 4Laboratorio de Ecología Microbiana, Departamento de Microbiología, Escuela Nacional de Ciencias Biológicas, Instituto Politécnico Nacional, Mexico City, Mexico
Dendroctonus-bark beetles are associated with microbes that can detoxify terpenes, degrade complex molecules, supplement and recycle nutrients, fix nitrogen, produce semiochemicals, and regulate ecological interactions between microbes. Females of some Dendroctonus species harbor microbes in specialized organs called mycetangia; yet little is known about the microbial diversity contained in these structures. Here, we use metabarcoding to characterize mycetangial fungi from beetle species in the Dendroctonus frontalis complex, and analyze variation in biodiversity of microbial assemblages between beetle species. Overall fungal diversity was represented by 4 phyla, 13 classes, 25 orders, 39 families, and 48 genera, including 33 filamentous fungi, and 15 yeasts. The most abundant genera were Entomocorticium, Candida, Ophiostoma-Sporothrix, Ogataea, Nakazawaea, Yamadazyma, Ceratocystiopsis, Grosmannia-Leptographium, Absidia, and Cyberlindnera. Analysis of α-diversity indicated that fungal assemblages of D. vitei showed the highest richness and diversity, whereas those associated with D. brevicomis and D. barberi had the lowest richness and diversity, respectively. Analysis of β-diversity showed clear differentiation in the assemblages associated with D. adjunctus, D. barberi, and D. brevicomis, but not between closely related species, including D. frontalis and D. mesoamericanus and D. mexicanus and D. vitei. A core mycobiome was not statistically identified; however, the genus Ceratocystiopsis was shared among seven beetle species. Interpretation of a tanglegram suggests evolutionary congruence between fungal assemblages and species of the D. frontalis complex. The presence of different amplicon sequence variants (ASVs) of the same genus in assemblages from species of the D. frontalis complex outlines the complexity of molecular networks, with the most complex assemblages identified from D. vitei, D. mesoamericanus, D. adjunctus, and D. frontalis. Analysis of functional variation of fungal assemblages indicated multiple trophic groupings, symbiotroph/saprotroph guilds represented with the highest frequency (∼31% of identified genera). These findings improve our knowledge about the diversity of mycetangial communities in species of the D. frontalis complex and suggest that minimal apparently specific assemblages are maintained and regulated within mycetangia.
Introduction
Dendroctonus bark beetles (Curculionidae: Scolytinae) are agents of disturbance in coniferous forest in North and Central America, and their populations occasionally outbreak to affect large areas of healthy forest with consequences for biodiversity, ecosystem and landscape degradation, and economic damage to timber resources (Six and Bracewell, 2015). Beetles breed in the inner bark and feed on the phloem of living trees of genera Pinus, Larix, Pseudotsuga, and Piceae (Family: Pinaceae). This strategy requires them to have metabolic and nutritional capabilities to overcome chemical defenses of trees and exploit a substrate rich in complex polysaccharides (e.g., cellulose, hemicellulose, starch, pectin) and other polymers (e.g., lignin) that are not readily available to them (Krokene, 2015; Chiu et al., 2019; Pace, 2019; Soto-Robles et al., 2021). Dendroctonus bark beetles are associated with facultative microbes (e.g., filamentous fungi, yeasts, bacteria) that may aid them in the tasks of detoxifying terpenes (DiGuistini et al., 2011; Adams et al., 2013; Xu et al., 2015; Dai et al., 2022), degrading complex molecules (Valiev et al., 2009; Cano-Ramírez et al., 2016; Briones-Roblero et al., 2017a), uric acid recycling and fixing nitrogen (Ayres et al., 2000; Bleiker and Six, 2007; Goodsman et al., 2012; Morales-Jiménez et al., 2012, 2013), producing semiochemicals (Boone et al., 2013; Cale et al., 2019), and regulating ecological interactions between symbionts and pathogens (Cardoza et al., 2006; Adams et al., 2009; Cheng et al., 2015, 2018; Xu et al., 2016).
Microbial communities are acquired by Dendroctonus beetles via feeding and contact during their development under the bark of trees and transported in the gut, on the integument, or in specialized secretory structures termed “mycetangia” (Vega and Biedermann, 2020). Mycetangia are compartmentalized cuticular spaces whose microenvironment changes throughout the beetle life cycle (Beaver, 1989; Six, 2012). These structures are common in ambrosia and bark beetles (Jordal and Cognato, 2012); however, in the genus Dendroctonus, they vary in their shape and location. For example, mycetangia occur as pits in D. pseudotsugae Hopkins, 1905 (Lewinsohn et al., 1994), pouches in the maxillary cardines in D. ponderosae Hopkins, 1902 and D. jeffreyi Hopkins, 1909 (Whitney and Farris, 1970), and as well-developed prothoracic sacs in the species of the Dendroctonus frontalis complex, including D. adjunctus Blandford, 1897, D. approximatus Dietz, 1890, D. barberi Hopkins, 1909, D. brevicomis LeConte, 1876, D. frontalis Zimmermann, 1868, D. mexicanus Hopkins, 1905, D. mesoamericanus Armendáriz-Toledano and Sullivan, 2015 and D. vitei Wood, 1974 (Barras and Perry, 1971; Yuceer et al., 2011; Six and Bracewell, 2015).
It is assumed that the mycetangia in bark beetles that feed on phloem evolved independently of nutritional requirements, unlike other insects that are nutritionally and physiologically dependent on fungi carried in these structures (Birkemoe et al., 2018; Vega and Biedermann, 2020). Mycetangia secrete specific amino acids, sterols and fatty acids that stimulate germination of fungal spores or hyphal growth (Barras and Perry, 1971; Happ et al., 1971; Yuceer et al., 2011; Six, 2012). These characteristics suggest that mycetangia should be highly selective and constrain the presence of microbes to specific taxa whose physiological capabilities are able to survive in this selective microenvironment (Beaver, 1989). However, it is also possible that phylogenetically unrelated microbes may have adapted to co-exist with symbiotic species within mycetangia (Six, 2012), and such adaptations could favor microbial dispersal within and between habitats.
It has been demonstrated that mycetangial microbes have a certain degree of specificity within Dendroctonus species (Harrington, 2005; Bracewell and Six, 2014; Birkemoe et al., 2018; Bracewell et al., 2018). Yet, few studies attempt to broadly evaluate composition of whole fungi and yeast assemblages, and culture-dependent approaches have limited taxonomic coverages. In this study, we comprehensively characterize the diversity of mycetangial assemblages from species in the D. frontalis complex using high-throughput next generation sequencing (NGS) of the internal transcribed spacer 2 region (ITS-2). Application of NGS has enhanced the characterization of microbial communities associated with Dendroctonus species and their host trees, and enables the evaluation of community change across developmental stages and growth conditions (e.g., field-grown whole adults, larvae, phloem, galleries, and laboratory-reared whole adults) (Durand et al., 2015, 2019; Dohet et al., 2016; Briones-Roblero et al., 2017b; Hernández-García et al., 2017; Cheng et al., 2018; Gonzalez-Escobedo et al., 2019). Here, we asked three fundamental questions about the diversity of microbes present in mycetangial structures: How diverse are the mycetangial assemblages of beetles in the D. frontalis complex? How specific are these assemblages to different beetle species? What are the potential functional roles of these assemblages? Accordingly, we test the following two respective hypotheses: (1) beetle species have similar mycetangial assemblages in terms of community composition, diversity, and trophic relationships, and (2) microbial assemblages show a high degree of specificity to beetle species given the dependence of these on filamentous fungi and the different environmental conditions and unique relationships with their host-trees. This study provides new insight into the biodiversity and function of bark beetle-fungal interactions and may have consequences for our understanding of complex multi-partner symbioses.
Materials and methods
Collection of insects
The species of the Dendroctonus frontalis complex (Lanier et al., 1988), including Dendroctonus adjunctus, D. barberi, D. brevicomis, D. frontalis, D. mesoamericanus, D. mexicanus, and D. vitei were collected in different localities from Mexico and United States (Table 1). Sterile forceps were used to directly remove pioneer adults from galleries built under the bark from five pine trees (∼15 m tall, ∼25 cm diameter) during the onset of colonization, but in the case from D. barberi and D. brevicomis beetles were collected from Lindgren funnel traps baited with endo-brevicomin (+) and α-pinene, and exo-brevicomin (+) and α-pinene (Synergy Canada Inc.), respectively. Insects collected in Mexico were stored and transported in sterile Magenta™ vessels GA-7 (Sigma-Aldrich, United States) at 4°C and processed immediately upon arrival at the laboratory, while those from the United States were shipped by air in vials of 70% alcohol. Species identification was carried out according to Armendáriz-Toledano and Zúñiga (2017).
All insects were superficially disinfected by serial immersion in the following solutions at 1 min intervals: detergent solution (10 mmol L–1 Tris-HCl pH 8, 1 mmol L–1 EDTA, 10 mmol L–1 NaCl, 1% SDS, 2% Triton X-100), 70% ethanol solution and sterile distilled water. The head and abdomen of all insects were removed and placed in a sterile phosphate-buffer solution (PBS pH 7.4; 137 mmol L–1 NaCl, 2.7 mmol L–1 KCl, 10 mmol L–1 NaHPO4, 2 mmol L–1 KH2PO4). Under a stereoscopic microscopy, the portion of the prothorax (callus pronotal) containing the complete female mycetangium was cleaned directly again with detergent solution and 96% ethanol, using a camelhair brush. To maintain sterile conditions, dissections and surface sterilizations were performed in a laminar flow hood. Lastly, three replicates of 50 mycetangia of each sample (150/species) were transferred into 1.5 mL microtubes containing 200 μL of sterile PBS and stored at −20°C until DNA extraction. For D. mexicanus and D. barberi there were four (200 total mycetangia) and two (100 total mycetangia) replicates analyzed.
DNA extraction, amplification, and sequencing
All samples were centrifuged for 5 min at 13,000 rpm to concentrate pronota, and supernatants were discarded. To each sample, 500 μL of lysis buffer (10 mM Tris-HCl pH 8, 1 mM EDTA, 10 mM NaCl, 1% SDS, 2% Triton X-100) were added, and later each pronotum set was macerated using sterile plastic pestles and vortexed with 0.2 g of zirconia beads for 1.5 min. Thereafter, 40 μL of Proteinase K (20 mg mL–1) were added and incubated at 60°C for 3 h. After incubation, 500 μL of chloroform-isoamyl alcohol (24:1 v/v) were added to the samples and mixed gently by inversion. The tubes were kept at −70°C for 1 h. Then 180 μL of 1% CTAB (hexadecyltrimethylammonium bromide) in 0.7 M NaCl and 80 μL of 5 M NaCl were added, and the samples were centrifuged for 5 min at 5,000 × g and the aqueous phase recovered in new sterile tubes. DNA was precipitated with 500 μL of 100% ethanol. DNA pellets were resuspended in sterile deionized water, and their concentration and purity were evaluated in a NanoDrop 2000c Spectrophotometer (Thermo Scientific, Wilmington, DE, United States). Universal primer pairs ITS3 (5′-GCA TCG ATG AAG AAC GCA GC-3′) and ITS4 (5′-TCC TCC GCT TAT TGA TAT GC-3′) were used to characterize the fungal assemblages of mycetangia samples. The ITS2 region was sequenced using paired-end 2 × 300 bp on an Illumina MiSeq sequencer at Macrogen Inc. (Seoul, Korea).
Quality control and taxonomic assignment
Raw paired-end reads were imported into Quantitative Insights Into Microbial Ecology QIIME2 v.2022.2 (Bolyen et al., 2019). Sequences were quality filtered, trimmed, denoised, and merged using DADA2 plugin (Callahan et al., 2016). Chimeric sequences, singletons, and doubletons were identified and removed via the consensus method in DADA2. Representative ASVs were aligned with MAFFT and used for phylogenetic reconstruction in FastTree using plugins alignment and phylogeny. A pretrained Naïve Bayes classifier based on UNITE database (Kõljalg et al., 2020)1 at 97% similarity threshold was applied to assign the taxonomy. The taxonomic identity of representative ASVs was manually corroborated in the GenBank2 and UNITE databases. Sequences belonging to the ITS2 of the insects, mites, and plants were discarded. Sequences of all libraries were normalized in MetagenomeSeq v.1.22.0 using the method of cumulative sum scaling (Paulson et al., 2013). Libraries were homogenized at the same sample size (through multiple rarefaction) considering the library with the lowest number of sequences.
Characterization of α- and β-diversity of mycetangial fungal assemblages
The sampling coverage was determined using rarefaction curves and the Good’s coverage estimator in QIIME2. The relative abundances of ASVs were visualized at the genus level in a heatmap developed in the CIMminer platform3 and associated to a dendrogram built by the UPGMA method using the Bray-Curtis index in QIIME2.
To calculate the α-diversity within these fungal assemblages, we estimated species richness using the observed ASV number and Chao1 index and species diversity with Shannon, Simpson, and Simpson’s Reciprocal indexes in QIIME2. Pairwise differences in the diversity indices between libraries were evaluated using Kruskal-Wallis test in QIIME2. Finally, to compare fungal assemblages in a multidimensional space, a principal coordinate analysis (PCoA) using Bray-Curtis dissimilarity matrix and an analysis of similarities (ANOSIM) were performed considering 999 random permutations in QIIME2.
A core mycobiome of mycetangia from the species of the D. frontalis complex was defined using two cut-offs in QIIME2. The first (strict core) consist in ASVs present > 70% of all libraries; the second is made up of ASVs present between 50 and 70% of libraries (relaxed core).
Specificity of fungal assemblages to beetle species
A maximum likelihood phylogeny was inferred in PhyML 3.0 in the ATGC Montpellier Bioinformatics platform for the species of the D. frontalis complex using mtDNA cytochrome oxidase I sequences deposited in NCBI GenBank (accession no. AF60001.1 D. adjunctus, AF06999.1 D. barberi, AF068002.1 D. brevicomis, AF067986.2 D. frontalis, AF067988.1 D. mexicanus, KT364536.1 D. mesoamericanus, KT364538.1 D. vitei). Sequence AF067999.1 of D. brevicomis from Colorado, United States was considered as D. barberi, because this species was recently removed from its synonymy with D. brevicomis based on morphological, molecular, and chemical ecology evidence which indicates that populations from Colorado, Nevada, Utah, Arizona, New Mexico, and Texas correspond to D. barberi (Bracewell et al., 2018; Valerio-Mendoza et al., 2019; Sullivan et al., 2021). Sequences were aligned with Clustal X v.2.1 (Larkin et al., 2007) and manually edited in Seaview v.4.0.5 (Gouy et al., 2010). The best nucleotide substitution model that fit the sequence set was determined in jModelTest v.2.1.10 (Darriba et al., 2012), being GTR + I according Akaike information criterion (k = 20, −Ln = 3018.98366, AIC = 6077.96732). A bootstrap test using 1000 pseudoreplicates was carried out to evaluate the robustness of the clusters. D. adjunctus was used as the outgroup. A dendrogram of fungal assemblages was built by the unweighted arithmetic average clustering method (UPGMA) in QIIME2 using Bray-Curtis dissimilarity index. The topologies of the bark beetles and fungal assemblages were reconciled in Jane v.4.0 (Conow et al., 2010), mixing general kinds of coevolutionary events, such as cospeciation, host-switching, duplication, etc., and finding the best reconstructions by minimizing the global cost. Two models were tested, edges (edge) and nodes (node) following different cost schemes using 100 generations and a population size of 50: Cospeciation = 0, Duplication = 1, Duplication and Host switching = 2, Loss = 1, Failure to diverge = 1. The tanglegram and randomizations of the tips of the trees and the fungal assemblage topology was reconstructed after 1000 generations and considering a population size of 1000. The significance of the association was between distance matrices was evaluated using Mantel test after 1000 permutations in PAST v.3.11 (Hammer et al., 2001).
Ecological network analysis and trophic mode
Fungal co-occurrence network from mycetangial assemblages from species of the D. frontalis complex was generated in MENA platform (Deng et al., 2012, accessed 29 March 2022)4 based on random matrix theory. For this analysis, we used those ASVs obtained after of the filter process and annotated to order, class or genus and whose reads numbers were > 20. Topological properties as modularity, clustering coefficient, average path length, graph density, and average degree of network were also estimated in MENA. The Spearman correlation test with a significance value of < 0.05 was used for the construction of co-occurrence network, which was visualized in Cytoscape v.3.9.1 (Shannon et al., 2003). To evaluate the empirical network and to identify mycobiota interactions that were due to non-random, a random network was generated from the empirical structure pattern of mycetangial assemblage and tested by power-law distribution. The random network was constructed based on the Maslov-Sneppen method in MENA, which kept numbers of nodes (mycetangial taxa) and edges (connections) unchanged, but rewired positions of all links in the network. Mycetangia networks from the species of the D. frontalis complex were manually extracted from the global network to visualize the ASVs co-occurrence in each bark beetle species. Lastly, the functional guild and trophic mode of the ASVs were searched using the FUNGuild database v.1.0 (Nguyen et al., 2016), and manually completed using specialized literature and USDA fungal Database (Farr and Rossman, 2015).
Results
Sequencing data
A total of 3,361,885 reads were obtained from the 21 libraries analyzed. From these, 426,410 were recovered after quality control and 288,143 were used for the analysis after rarefaction. The rarefaction curves analysis and values obtained with the Good’s coverage estimator (> 99%) indicated an appropriate sampling effort for all samples (Supplementary Figure 1). A total of 182 ASVs were defined, the lowest number of observed ASVs was 10 in D. barberi and the highest was 40 in D. mexicanus (Supplementary Table 1).
Composition of fungal assemblages
In the seven species of the D. frontalis complex, a total of four phyla, 13 classes, 25 orders, 39 families, and 48 genera were identified. Basidiomycota was the most abundant phylum with 50.86% of relative abundance (RA) in all samples, followed by Ascomycota (47.99%), Zygomycota (1.13%) and Mortierellomycota (< 0.01%). Basidiomycota was more abundant in D. barberi (RA = 99.28%), D. brevicomis (RA = 98.59%), D. frontalis (RA = 94.54%) and D. mesoamericanus (RA = 63.23%). Ascomycota was particularly abundant in D. adjunctus (RA = 99.98%), D. mexicanus (RA = 99.88%) and D. vitei (RA = 91.75%). Zygomycota and Mortierellomycota were only present in D. vitei (RA = 7.2%; RA = 0.04%). At the class level, Agaricomycetes was mainly abundant in all insect species, while Dothideomycetes, Eurotiomycetes, and Saccharomycetes were well represented in D. adjunctus and D. mexicanus (Figure 1A and Supplementary Figure 2A). In all libraries, the most identified orders were Corticiales, Saccharomycetales, and Ophiostomatales (Supplementary Figure 2B). Saccharomycetales and Ophiostomatales were mainly detected in D. adjunctus, D. mesoamericanus, D. mexicanus, and D. vitei, while Corticiales was present in high abundance in D. barberi, D. brevicomis, D. frontalis, and D. mesoamericanus (Supplementary Figure 2C).
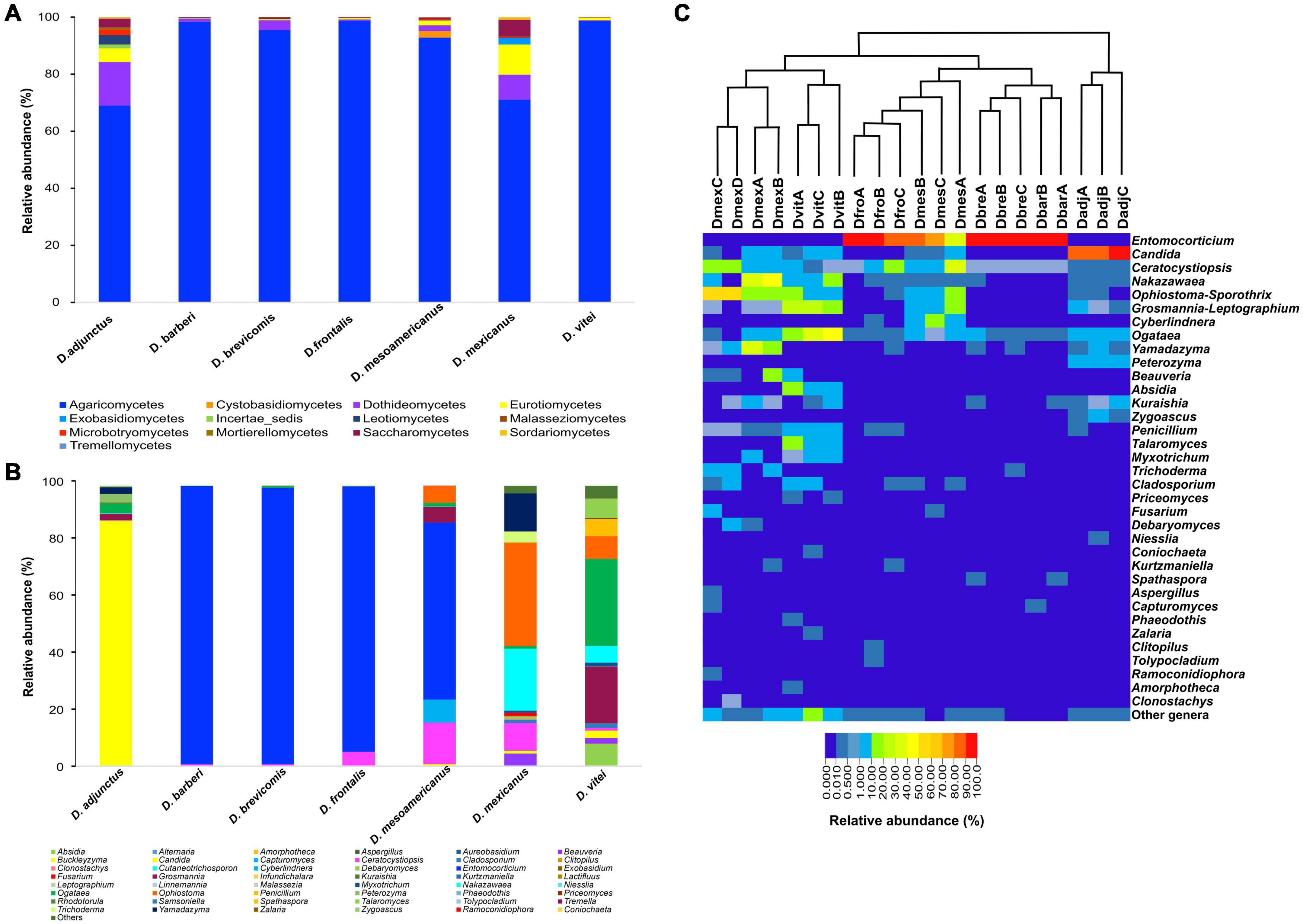
Figure 1. Fungal diversity and relative abundance of mycetangial fungi from the species of the D. frontalis complex. Biological replicates of each beetle species were grouped in (A,B), but not in (C). (A) Bar diagram illustrating mycetangial fungi at the class level. (B) Bar diagram showing mycetangial fungi at the genus level. (C) Heat map showing mycetangial fungi per library at the genus level by beetle species, the 21 libraries were grouped by the unweighted pair group method with arithmetic mean (UPGMA) using the Bray-Curtis dissimilarity matrix; red color represent high abundance and strong blue low abundance.
At the genus level, 33 filamentous fungi and 15 yeasts were identified (Figure 1B). The most abundant genera found in the 21 libraries were Entomocorticium, Candida, Ophiostoma-Sporothrix, Ogataea, Nakazawaea, Yamadazyma, Ceratocystiopsis, Grosmannia-Leptographium, Absidia, and Cyberlindnera. Other genera had relative abundances < 1% and low prevalence in all bark beetle species (Figures 1B,C). The genus Entomocorticium was dominant (RA = 50.95%) in the libraries from D. barberi, D. brevicomis, D. frontalis, and D. mesoamericanus. Other taxa with relative abundances between 13 and 87% were Candida in D. adjunctus, Ogataea in D. vitei; Ophiostoma-Sporothrix, Nakazawaea, and Yamadazyma in D. mexicanus; Ceratocystiopsis in D. mesoamericanus and Grosmannia-Leptographium in D. vitei. Lastly, the genera Nakazawaea, Ophiostoma-Sporothrix, Penicillium, Talaromyces, and Grosmannia-Leptographium, showed low relative abundances between 4.5 and 20% in D. vitei, Ophiostoma-Sporothrix in D. mesoamericanus, and Ceratocystiopsis in D. frontalis and D. mexicanus.
α and β-diversity of fungal assemblages
The fungal assemblages of D. vitei showed the highest richness (Chao1 = 29 ± 6.08) and diversity (Shannon = 3.28 ± 0.27; Simpson = 0.83 ± 0.04), while the fungal assemblages of D. brevicomis had the lowest values of richness (Chao1 = 9 ± 1.00) and D. barberi had the lowest diversity (Shannon = 0.43 ± 0.01; Simpson = 0.12 ± 0.01). The number of dominant species in the fungal assemblages varied from five in D. vitei to one in D. barberi and D. frontalis (Table 2). Kruskal-Wallis test showed significant differences in richness (Chao1) and diversity (Shannon, Simpson) in at least one pair of the fungal assemblages from the species of the D. frontalis complex, except in D. barberi which did not show differences with other assemblage (Table 2). Highlighting the fungal assemblage from D. brevicomis as the most different in richness, and those from D. frontalis, D. mexicanus, and D. vitei as the most diverse (Table 2).
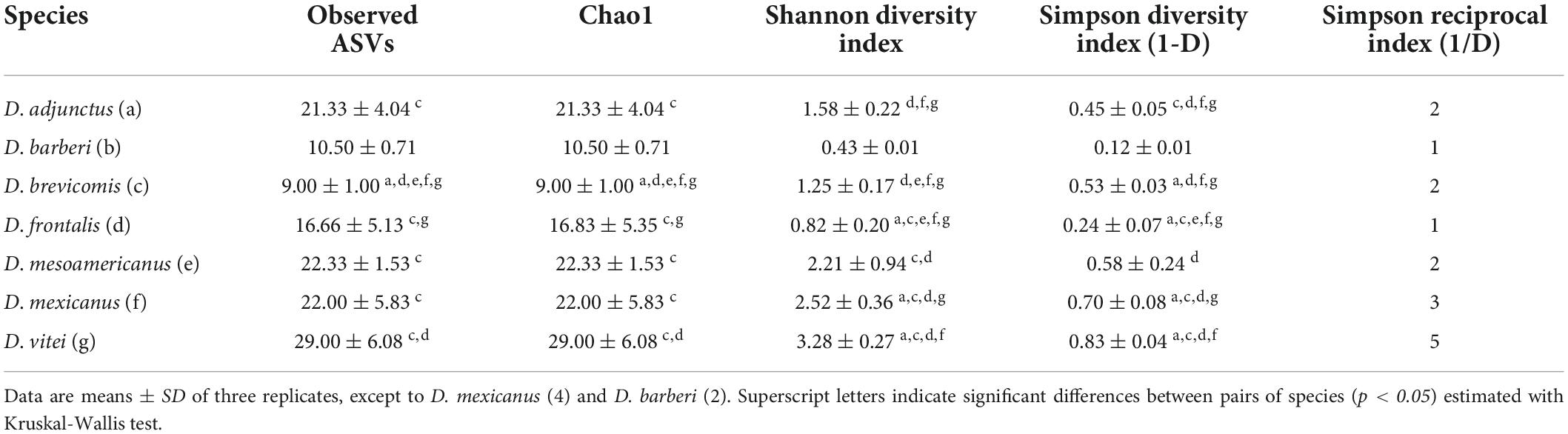
Table 2. Amplicon sequence variant (ASVs) richness and diversity indexes of the mycetangial fungi assemblages from the species of the Dendroctonus frontalis complex.
Significant differences (ANOSIM, p = 0.001) were found between the mycetangial assemblages of the different species of the D. frontalis complex using Bray-Curtis dissimilarity matrix (Figure 2A). The first three coordinates of the PCoA explained 61.55% of the total observed variation (Figure 2B). A clear spatial segregation of libraries corresponding to D. adjunctus, D. barberi, and D. brevicomis species was observed in the multidimensional space, but not between close species D. frontalis and D. mesoamericanus, as well as D. mexicanus and D. vitei.
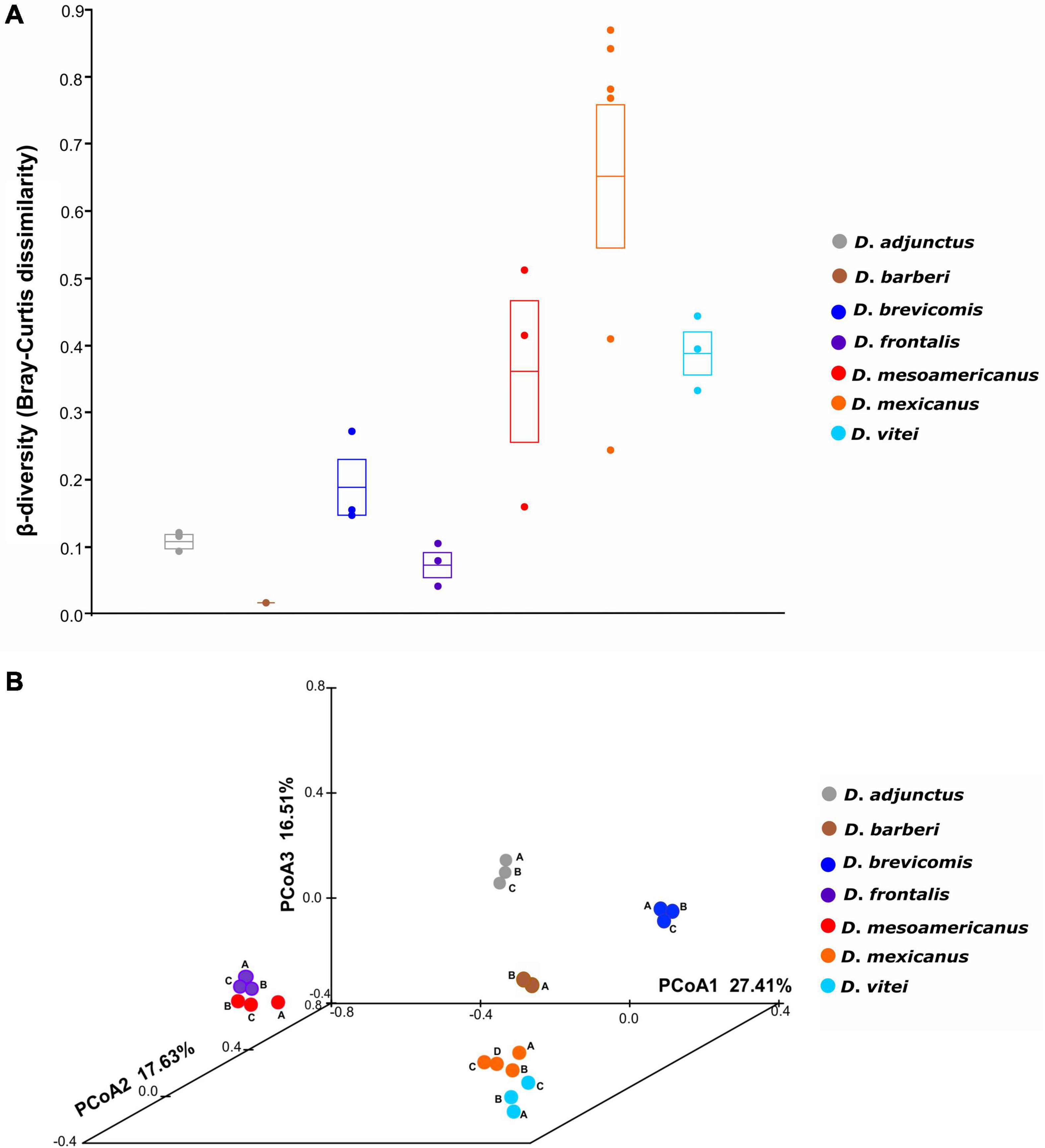
Figure 2. Beta-diversity and principal coordinate analysis (PCoA) based on Bray-Curtis dissimilarity. (A) Significant differences among in the mycangial fungal communities from the species of the D. frontalis complex (ANOSIM, p = 0.001). The circles represent pairwise Bray-Curtis dissimilarity values between biological replicates from species of the D. frontalis complex; the transversal line and ends in the box represent the mean and its standard error. (B) PCoA plot depicting variation in a multidimensional space of mycetangial fungal assemblages from the species of the D. frontalis complex; the first three PCoA coordinates explained 61.55% of the total observed variation; dots of the same color represent biological replicates (A, B, C) of the same bark beetle species, except for D. mexicanus (A, B, C, D) and D. barberi (A, B).
The absence of a strict core was evident because only the genus Ceratocystiopsis was shared among 21 libraries of seven species (represented by different ASVs). Only two genera, Nakazawaea and Ogataea, were found in the relaxed core, because they were recovered in at least 70% of libraries, but also represented with different ASVs.
Fungal assemblages and their association with the species of the Dendroctonus frontalis complex
The tanglegram showed a significant global congruence between fungal assemblages and species of the D. frontalis complex (Figures 3A,B). Four cospeciation events and four duplication and host switch events were inferred. The first cospeciation event was between D. mexicanus and D. vitei; the second between D. mesoamericanus and D. frontalis; the third between groups D. mexicanus + D. vitei and D. mesoamericanus + D. frontalis; and the last between D. barberi and D. brevicomis. The four events of duplication and host switch were distributed between D. frontalis and D. mesoamericanus (2), between groups D. mesoamericanus + D. frontalis and D. barberi + D. brevicomis (1), and the last between D. adjunctus and the group of D. mexicanus + D. vitei + D. mesoamericanus + D. frontalis (1). Topological reconciliation showed that the species of the D. frontalis complex has potentially exclusive mycetangial assemblages, because the ASVs are not completely shared. The reconciliation of topologies (p = 0.01) and association between distances matrices from bark beetles and mycetangial assemblages was statistically significant (Mantel test r = 0.7093, p = 0.003).
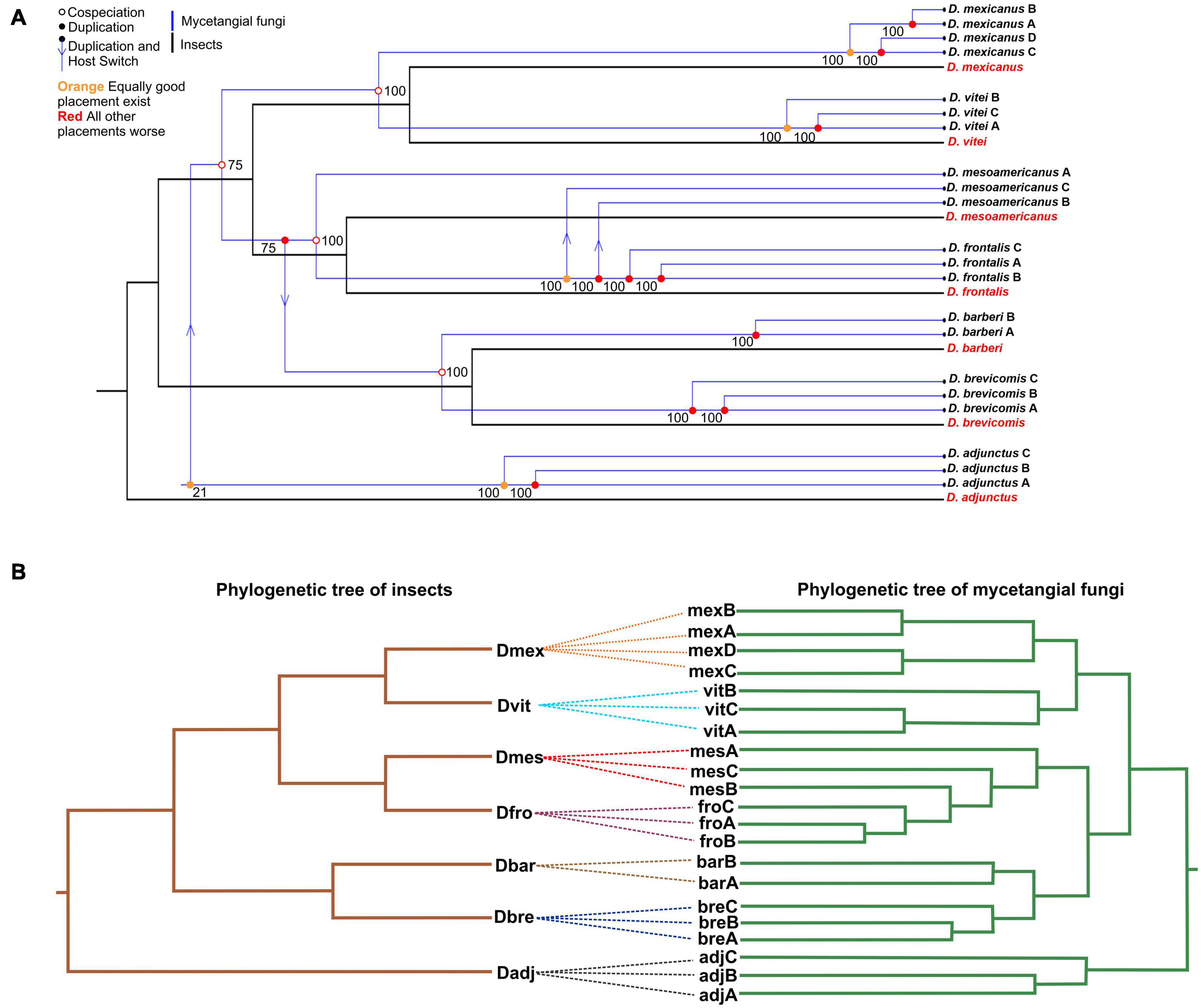
Figure 3. The tanglegram of fungal assemblages and species of the D. frontalis complex. (A) Topological reconciliation of bark beetles and fungal assemblages. Four cospeciation events were found, the first between D. mexicanus and D. vitei; the second between D. mesoamericanus and D. frontalis; the third between groups D. mexicanus + D. vitei and D. mesoamericanus + D. frontalis; and the last between D. barberi and D. brevicomis. Four duplication and host switch events were detected between D. frontalis and D. mesoamericanus (2), between groups D. mesoamericanus + D. frontalis and D. barberi + D. brevicomis (1), and the last between D. adjunctus and the group of D. mexicanus + D. vitei + D. mesoamericanus + D. frontalis (1). The reconciliation of topologies (p = 0.01) and association between distance matrices (Mantel test r = 0.7093, p = 0.003) were significant. (B) ML-phylogeny from the species of the D. frontalis complex inferred using mtDNA COI sequence (AF60001.1 D. adjunctus, AF06999.1 D. barberi, AF068002.1 D. brevicomis, AF067986.2 D. frontalis, AF067988.1 D. mexicanus, KT364536.1 D. mesoamericanus, KT364538.1 D. vitei). The model best was GTR + I sensu Akaike criterion (k = 20, −Ln = 3018.98366, AIC = 6077.96732). Bootstrap values are shown upper nodes. D. adjunctus was used as outgroup. Dendrogram of fungal assemblages built using UPGMA and Bray-Curtis dissimilarity index.
Ecological network analysis of mycetangial assemblages
From 182 ASVs recovered on a total of 288,143 sequences in the DNA metabarcoding analysis, only 105 had > 20 reads. The association of these 105 ASVs showed a network containing 62 nodes connected by 295 links, of which 50.85% were positive connections (e.g., mutualistic or commensalistic interactions) and 49.15% were negative connections (e.g., antagonistic interactions). Nodes were represented by one class, one order, 11 genera of filamentous fungi and 7 yeasts. Four modules were integrated in the global network. The module 0 included ASVs from D. mexicanus and D. vitei, represented by four and six filamentous fungi genera, respectively, and two yeasts genera each one. The module 1 was integrated by ASVs from D. adjunctus represented by one filamentous fungi genus and five yeasts genera. The module 2 was formed by ASVs from D. barberi and D. brevicomis, represented by two and one filamentous fungi genera, respectively, and one yeast each one. Lastly, the module 3 was formed by ASVs from D. frontalis and D. mesoamericanus, defined by two and four filamentous fungi genera and one and three yeasts, respectively. The ASVs belonging to Ogataea (Oga1) and Grosmannia (Gro1) had > 10 interactions with ASVs of others modules (Figure 4). The connectivity distribution in this network followed the power-law model (R2 = 0.013). The values of modularity (M = 0.589), average connectivity (avgK = 9.516), average path distance (GD = 2.329), and average clustering coefficient (avgCC = 0.778) were significantly higher than the random network values (M = 0.218, GD = 2.061, avgCC = 0.241), suggesting that the mycetangial ASVs assemblage was non-random (p < 0.05) (Figure 4). Individual networks were integrated by different ASV numbers. For example, D. brevicomis, D. barberi, and D. mexicanus had < 9 ASVs, whereas D. adjunctus, D. frontalis, D. mesoamericanus, and D. vitei had ≥ 10. These networks were almost entirely integrated by different ASVs, with a few exceptions, including the ASVs of Cer1, Ent1,5, Oga1,6, Oph1, Gro1, and Yam3, which were shared among the bark beetle species (Figure 5 and Supplementary Table 2). Based on the number of positive and negative interactions (edges), the dominant condition of some ASVs in the individual networks was evident, highlighting those from the genus Candida (Can) in the D. adjunctus network; Entomocorticium (Ent) in the networks of D. barberi, D. brevicomis, and D. frontalis; Ceratocystiopsis (Cer), Yamadazyma (Yam), and Talaromyces (Tal) in the networks of D. mesoamericanus, D. mexicanus, and D. vitei, respectively (Figure 5 and Supplementary Table 2). All these results suggest that the fungal assemblage networks associated to the species of the D. frontalis complex was non-randomly distributed and had a highly connected topological structure.
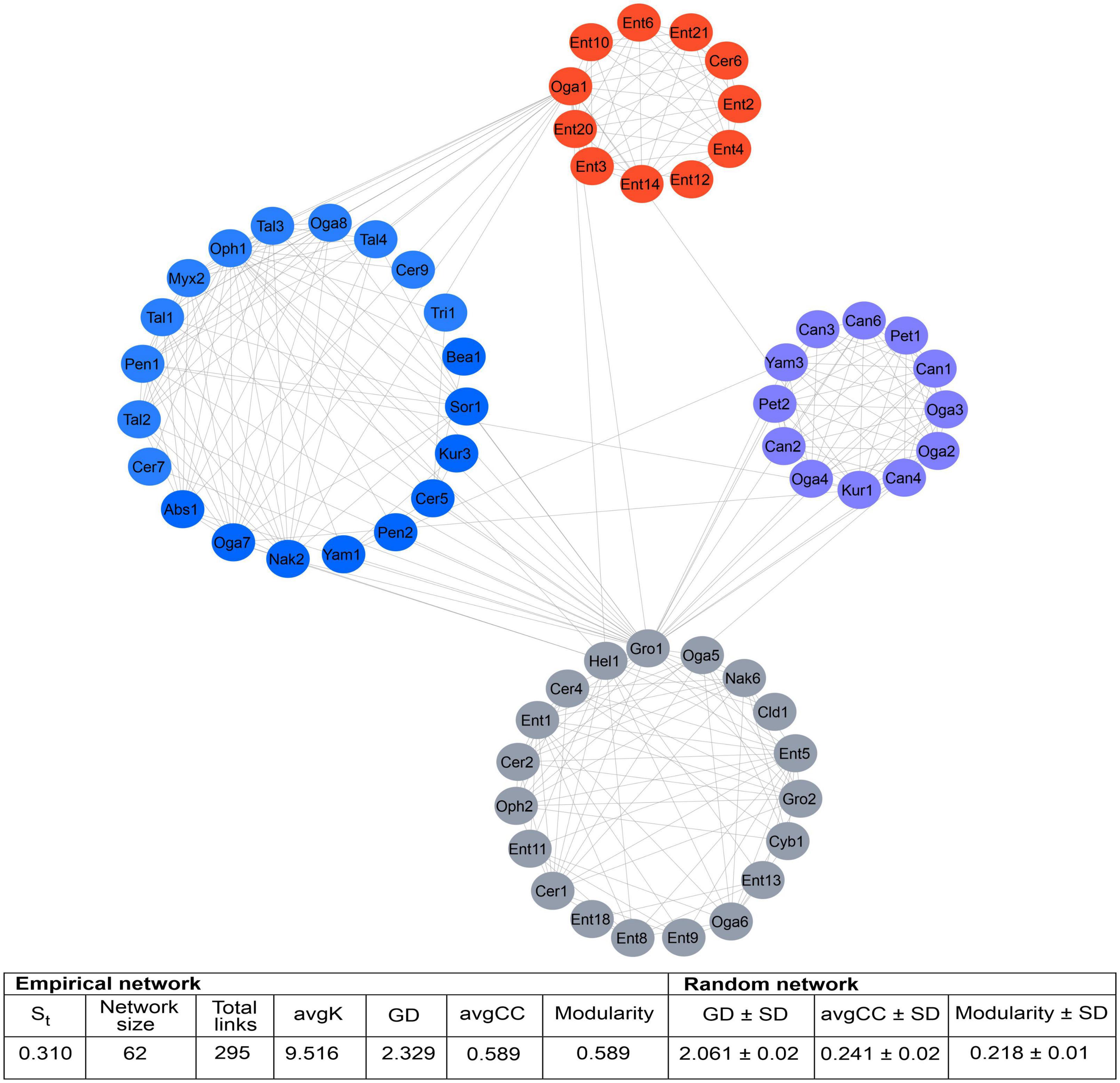
Figure 4. Global network of the mycetangial fungi assemblages from the species of the D. frontalis complex. It was built with 105 ASVs whose reads > 20 reads, which include to one class, one order,13 genera of filamentous fungi and 9 yeasts. ASVs showed a network contained 62 nodes connected by 295 edges, of which 50.85% were positive connections (mutualistic or commensalistic interactions) and 49.15% negative connections (antagonistic interactions). Four modules integrated the general network. The module 0 included the ASVs from D. mexicanus and D. vitei, the module 1 the ASVs from D. adjunctus, the module 2 the ASVs from D. barberi and D. brevicomis, and the module 3 the ASVs from D. frontalis and D. mesoamericanus. The connectivity distributions in this general network followed the power-law model (R2 = 0.013). The values of modularity, average connectivity, average path distance, and average clustering coefficient were significantly higher that of a random network (p < 0.05) and they are shown in the framework under the figure. Filamentous Fungi: Abs, Absidia; Bea, Beauveria; Cer, Ceratocystiopsis; Cld, Cladosporium; Ent, Entomocorticium; Gro, Grosmannia-Leptographium; Hel, Helotiales; Myx, Myxotrichum; Oph, Ophiostoma-Sporothrix; Pen, Penicillium; Sor, Sordariomycetes; Tal, Talaromyces; Tri, Trichoderma. Yeast: Can, Candida; Cyb, Cyberlindnera; Kur, Kuraishia; Nak, Nakazawaea; Oga, Ogataea; Pet, Peterozyma; Yam, Yamadazyma.
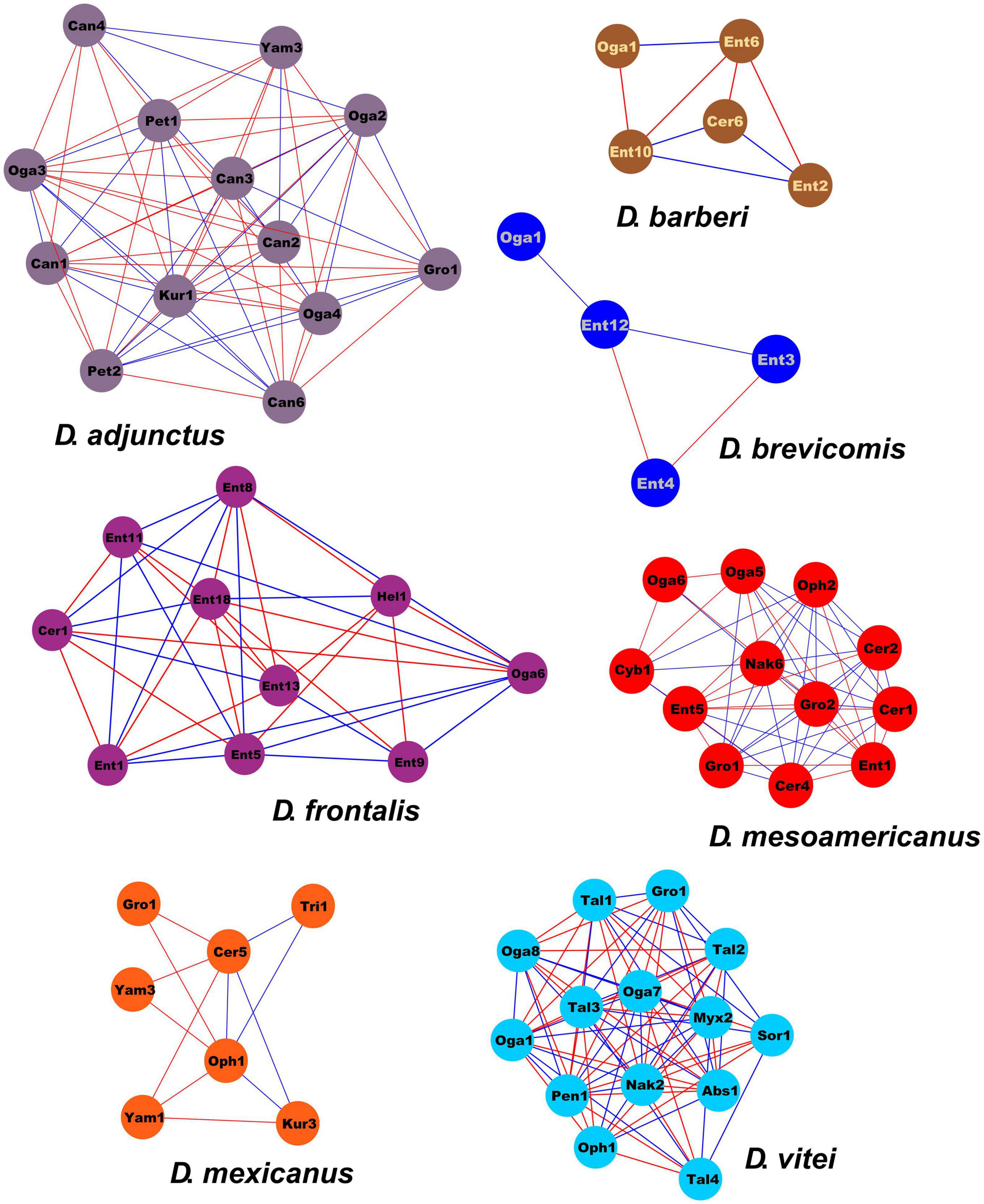
Figure 5. Individual network extracted from global network of the mycetangial fungi from each species of the D. frontalis complex species. The nodes are colored in each module according to the bark beetle species from which ASVs were identified. Individual networks were integrated by different ASV numbers. Blue lines indicate positive interactions (mutualism or commensalism), while a red lines indicates negative interactions (antagonism). Filamentous Fungi: Abs, Absidia; Bea, Beauveria; Cer, Ceratocystiopsis; Cld, Cladosporium; Ent, Entomocorticium; Gro, Grosmannia-Leptographium; Hel, Helotiales; Myx, Myxotrichum; Oph, Ophiostoma-Sporothrix; Pen, Penicillium; Sor, Sordariomycetes; Tal, Talaromyces; Tri, Trichoderma. Yeast: Can, Candida; Cyb, Cyberlindnera; Kur, Kuraishia; Nak, Nakazawaea; Oga, Ogataea; Pet, Peterozyma; Yam, Yamadazyma.
The total ASVs (182) were assigned into seven trophic modes at the following frequencies: symbiotroph/saprotroph (30.77%), followed by saprotroph (27.47%), pathotroph (19.79%), pathotroph/saprotroph/symbiotroph (11.54%), pathotroph/saprotroph (3.85%), pathotroph/symbiotroph (2.75%), symbiotroph (1.65%), and unassociated (2.20%). The guild of these fungi and yeast genera fell into the categories plant or animal pathogen, animal symbionts, wood saprotrophs, and less often lichen and fungal parasite (Supplementary Table 3).
Discussion
This is the first comprehensive study on the mycobiome of pronotal mycetangia from the species of the Dendroctonus frontalis complex. Based on Good’s coverage (>99%) and rarefaction curves, sampling effort was adequate. Our findings show the presence of fungal assemblages in the mycetangia of these beetles integrated both by filamentous fungi and yeasts, which varies among bark beetle species. This diversity is apparently limited to 33 genera of filamentous fungi and 15 genera of yeasts belonging to phyla Basidiomycota (50.86%), Ascomycota (47.99%), Zygomycota (1.13%), and Mortierellomycota (<0.01%) using ITS2 region. These results should be interpreted cautiously, because the intraspecific variation among ribosomal unit of the cluster rRNA loci, which has multiple copies in the fungi genome of the same species, could inflate the estimates of ASV richness and its abundance (Taylor et al., 2016; Lavrinienko et al., 2021). Thus, the inclusion of additional molecular markers would facilitate a better characterization of mycetangial assemblages.
The results indicate that the phylum Basidiomycota was more abundant than Ascomycota, in contrast to results reported for other bark beetles (Harrington, 2005), ambrosial insects (Henriques et al., 2006), and other Coleoptera (Biedermann and Vega, 2020) where Ascomycota is the dominant phylum. The presence of Mucoromycota (= Zygomycota) and Mortierellomycota are reported for the first time to be associated with the mycetangium from the species of the D. frontalis complex. Zygomycota has also been reported from the gut and galleries of D. armandi and D. valens in China, where they are invasive (Hu et al., 2015) and Mortierellomycota from galleries and epimycobiota of other Coleoptera (Wang et al., 2020; Held et al., 2021). Likewise, the basidiomycetes Entomocorticium, and ascomycetes Candida, Ophiostoma-Sporothrix, Ogataea, Nakazawaea, Yamadazyma, Ceratocystiopsis, Grosmannia-Leptographium, and Cyberlindnera were abundant in mycetangia.
Analysis of α-diversity revealed that the composition of fungal assemblages, as well as the relative abundance of their members, vary widely among species of the D. frontalis complex. Mycetangial assemblages of D. adjunctus, D. mexicanus, D. mesoamericanus, and D. vitei were more diverse than D. barberi, D. brevicomis, and D. frontalis. Significant changes in the assemblage structure are evident, as exemplified by Entomocorticium that is abundant and apparently exclusive to D. barberi, D. brevicomis, D. frontalis, and D. mesoamericanus, whereas Ogataea and Grosmannia-Leptographium are more prevalent in D. vitei, but Candida was the most abundant in D. adjunctus. Other genera such as Ceratocystiopsis and Ophiostoma-Sporothrix are also abundant, but not exclusive of any beetle species indicating that some fungal taxa are highly specific to their host beetles whereas others are likely generalists. Alternatively, some fungal associations may predate the evolutionary divergence of beetle taxa and may have been retained as symbionts across that divergence.
Differences in α-diversity of the fungal assemblages across beetle species may be due to several factors. For example, the development and ultrastructural organization of the mycetangium varies among species in the D. frontalis complex (Barras, 1967; Happ et al., 1971; Yuceer et al., 2011). This could indicate variable microenvironmental conditions associated with the mycetangia of different beetle species, including selective factors such as gas exchange, temperature, and redox potential (Six and Bentz, 2007; Bleiker and Six, 2009; Addison et al., 2013; Dysthe et al., 2015; Six and Klepzig, 2021). In addition, mycetangial glandular cells provide molecules to regulate the growth of specific fungi and bacteria (Francke-Grossman, 1967; Barras and Perry, 1971; Happ et al., 1971; Yuceer et al., 2011) or produce chemical secretions that inhibit the growth of unspecific fungi or favor the presence of symbiotic fungi (Schneider and Rudinsky, 1969; Happ et al., 1971; Paine and Birch, 1983).
Differences found in β-diversity are due to the absence of both dominant and marginal members in assemblages (Figure 1B). This explains the absence of a strict core mycobiome for species of the D. frontalis complex as a whole. On another hand, the presence of a relaxed minimal core consisting of Ceratocystiopsis, Nakazawaea, and Ogataea, along with the presumed exclusivity of the assemblages observed in the tanglegram, suggest that a core for individual species may exist. This hypothesis could be tested in future studies incorporating samples of these bark beetles from other localities, as the distribution of both insects and symbionts is not random but depending on ecological opportunities and selective pressures in geographic space, which eventually could modify the interaction among them, as well as the integration of minimal assemblages or the membership of some symbionts within the core mycobiome.
Members of this core may be playing a relevant ecological role and complex. For example, Ceratocystiopsis is a pathogenic fungus to pines and has been considered important to overcome the defensive system of healthy trees during beetle attacks, potentially facilitating successful colonization (Harrington, 1993). However, phytopathogenicity is likely not the evolutionary driver behind these associations and pathogenic fungi may be rarer associates than ecologically neutral symbionts (Six and Wingfield, 2011). Complex ecological roles might not be exclusive of the filamentous fungi; for instance, because it has demonstrated that Ogataea yeast can favor the growth of filamentous fungi, but also inhibit other fungi (Davis et al., 2011). Thus, collectively, the functions developed by the core members may have crucial importance in the life cycle of bark beetle species.
Despite their abilities to perform diverse functions to the benefit of these insects, the overrepresentation of certain fungi (e.g., Entomocorticium, Ophiostoma-Sporothrix, Ceratocystiopsis, and Grosmannia-Leptographium) in the species of the D. frontalis complex suggests that they are not randomly acquired even though they are transmitted horizontally, and their spores are passively incorporated into mycetangia (Beaver, 1989). This is also applicable to yeasts (e.g., Ogataea, Candida, Nakazawaea, Yamadazyma, Cyberlindnera) whose acquisition is horizontal, as suggested by their ubiquity in all developmental stages and body structures including mycetangia of these and other bark beetles (Rivera et al., 2009; Davis, 2015). The FUNGuild analysis showed that almost all filamentous fungi recorded in this study (e.g., Ophiostoma-Sporothrix, Grosmannia-Leptographium, Beauveria) are pathotrophs, whereas yeasts and some filamentous fungi (e.g., Ogataea, Kuraishia, Nakazawaea, Yamadazyma, Cyberlindnera, Peterozyma, Zygoascus, Entomocorticium, Ceratocystiopsis, Penicillium) are symbiotrophs or saprotrophs. Independently of their trophic mode and horizontal transmission, the nested structure of the global ecological network (i.e., it was more nested than the random network), in which few interacting species are compartmentalized into modules, suggesting that minimal assemblages with relatively high species redundancy are maintained, regulated, and structured within the mycetangia of these insects (Figure 4 and Supplementary Figure 3).
Although several studies have tested interaction between microbes isolated from the mycetangium (Scott et al., 2008; Oh et al., 2009; Davis et al., 2011), to our knowledge it is unknown how these assemblages are regulated and stabilized over space and time. The action of antibiotics, antifungals, mycotoxins and volatile organic compounds produced by fungi, yeasts, and bacteria, as well as by chemical secretions produced by mycetangial glands, or the immune system of ambrosia and bark beetles could be factors involved in this self-regulation (Nakashima et al., 1982; Scott et al., 2008; Oh et al., 2009; Shi and Sun, 2010). Other factors that could influence the regulation of mycetangial fungal assemblages include beneficial (mutualism, commensalism), competitive or neutral interactions of their members within integuments, as well as their potential functional roles, which could vary geographically with shifting environmental and biotic pressures.
Thus, the observed sporadic occurrence of some fungi or yeasts, as well as the possible loss of overrepresented members suggest that they are incidental or transient members of mycetangial assemblages, which could result in replacement by another member with a similar ecological niche (Six, 2012, 2019; Hofstetter et al., 2015; Bracewell et al., 2018). This functional redundancy could help to stabilize ecological role of mycetangial assemblages and guarantee the regulation of their members over space and time.
Lastly, the positive correlation between the similarity of the fungi assemblage composition and the phylogenetic relatedness of species of the D. frontalis complex observed in the tanglegram, suggest an ecological pattern of phylosymbiosis (Figure 3). This pattern, as suggested by events associated to tanglegram (cospeciation and duplication and host switch), could have been generated through the diversification from the species of the D. frontalis complex, as it was recently demonstrated in the case of mycetangial fungi associated to D. brevicomis and D. barberi (Bracewell et al., 2018). However, at shared habitats such as mycetangia, it cannot be discarded that this pattern could be raised and structured by habitat selection, where the abiotic and biotic environment into mycetangia could determinate the composition and specificity of the assemblages. Further research is required to test whether the phylosymbiosis corresponds with diversification event of these bark beetles or well if it is result non-adaptive host filtering (Groussin et al., 2020).
In summary, these findings improve our knowledge of the diversity of mycetangial assemblages in species of the D. frontalis complex. The isolation and culture of some fungal species has helped to clarify the nature of the relationship of individual members with Dendroctonus species, as well as the implications they have on the biology, ecology, and evolution of these insects. Our results add to this literature and suggest that specific minimal assemblages are maintained, regulated, and structured within the mycetangium of these insects. Analyzing these assemblages using an integrated approach, such as we do here, helps to further clarify the nutritional, physiological, and ecological roles of their members, as well as their potential interactions. This approach is a useful first step in better understanding these systems. Further work in this area is needed to analyze and better understand integral functional aspects, such as metabolite level and composition, metatranscriptomic and/or metaproteomic response of these communities within the mycetangium of their hosts, and multispecific interactions in vitro and in vivo.
Data availability statement
The datasets presented in this study can be found in National Center for Biotechnology Information (NCBI) Bioproject database under accession number: PRJNA813135.
Author contributions
KV-O, FR-O, and GZ conceived the work. KV-O performed the experiment. KV-O, RP-M, RG-E, TD, KS, FR-O, and GZ interpreted the result, performed the draft, and final edition of the manuscript. All authors contributed and approved the final manuscript.
Funding
This study was funded by the Instituto Politécnico Nacional SIP 20211009. This work was part of KV-O MsC thesis. She was fellow CONACYT (891748) and Member of the Program of Beca de Estímulo Institutional de Formación de Investigadores del Instituto Politécnico Nacional (BEIFI-IPN).
Acknowledgments
Brian T. Sullivan (USDA Forest Service, Southern Research Station, Pineville, LA), Stephen L. Clark (USDA Forest Service, Forest Health Protection, Texas Zone), Andrea R. Hefty (Forest Service, Forest Health Protection, Southern CA Shared Service Area), Andrew Graves (USDA Forest Service, Forest Health Protection, New Mexico Zone), Monica Gaylord (USDA Forest Service, Forest Health Protection R3, Arizona Zone), Danielle Malesky (Forest Service Forest Health Protection Northern and Intermountain Regions) kindly provide material from D. brevicomis and D. barberi for this study. Gerardo Cuellar Rodríguez (Universidad Autónoma de Nuevo León), Francisco Bonilla Torres (CONAFOR), Efraín Hernández (CONAFOR), and José Villa Castillo (CONAP-Parque Nacional Volcán Nevado de Colima) for their assistance in the field. Mario Favila Castillo and Jorge Macias Samano for their comment about this manuscript.
Conflict of interest
The authors declare that the research was conducted in the absence of any commercial or financial relationships that could be construed as a potential conflict of interest.
Publisher’s note
All claims expressed in this article are solely those of the authors and do not necessarily represent those of their affiliated organizations, or those of the publisher, the editors and the reviewers. Any product that may be evaluated in this article, or claim that may be made by its manufacturer, is not guaranteed or endorsed by the publisher.
Supplementary material
The Supplementary Material for this article can be found online at: https://www.frontiersin.org/articles/10.3389/fmicb.2022.969230/full#supplementary-material
Footnotes
- ^ https://unite.ut.ee
- ^ https://blast.ncbi.nlm.nih.gov/Blast.cgi
- ^ https://discover.nci.nih.gov/cimminer/home.do
- ^ http://ieg4.rccc.ou.edu/MENA/main.cgi
References
Adams, A. S., Aylward, F. O., Adams, S. M., Erbilgin, N., Aukema, B. H., Currie, C. R., et al. (2013). Mountain pine beetles colonizing historical and naive host trees are associated with a bacterial community highly enriched in genes contributing to terpene metabolism. Appl. Environ. Microbiol. 79, 3468–3475. doi: 10.1128/AEM.00068-13
Adams, A. S., Currie, C. R., Cardoza, Y., Klepzig, K. D., and Raffa, K. F. (2009). Effects of symbiotic bacteria and tree chemistry on the growth and reproduction of bark beetle fungal symbionts. Can. J. For. Res. 36, 64–72. doi: 10.1139/X09-034
Addison, A. L., Powell, J. A., Six, D. L., Moore, M., and Bentz, B. J. (2013). The role of temperature variability in stabilizing the mountain pine beetle-fungus mutualism. J. Theor. Biol. 335, 40–50. doi: 10.1016/j.jtbi.2013.06.012
Armendáriz-Toledano, F., and Zúñiga, G. (2017). Illustrated key to species of genus Dendroctonus (Coleoptera: Curculionidae) occurring in Mexico and Central America. J. Insect Sci. 17, 1–15. doi: 10.1093/jisesa/iex009
Ayres, M. P., Wilkens, R. T., Ruel, J. J., Lombardero, M. J., and Vallery, E. (2000). Nitrogen budgets of phloem-feeding bark beetles with and without symbiotic fungi. Ecology 81, 2198–2221. doi: 10.2307/177108
Barras, S. J. (1967). Thoracic mycangium of Dendroctonus frontalis (Coleoptera: Scolytidae) is synonymous with a secondary female character. Ann. Entomol. Soc. Am. 60, 486–487. doi: 10.1093/aesa/60.2.486
Barras, S. J., and Perry, T. (1971). Gland cells and fungi associated with prothoracic mycangium of Dendroctonus adjunctus. Ann. Entomol. Soc. Am. 64, 123–126. doi: 10.1093/aesa/64.1.123
Beaver, R. (1989). “Insect–fungus relationships in the bark and ambrosia beetles,” in Insect-fungus interactions, eds N. Wilding, N. M. Collins, P. M. Hammond, and J. F. Webber (San Diego, CA: Academic Press), 121–143. doi: 10.1016/C2009-0-02797-4
Biedermann, P. H. W., and Vega, F. E. (2020). Ecology and evolution of insect-fungus mutualisms. Annu. Rev. Entomol. 65, 431–455. doi: 10.1146/annurev-ento-011019-024910
Birkemoe, T., Jacobsen, R. M., Sverdrup-Thygeson, A., and Biedermann, P. H. W. (2018). “Insect-fungus interactions in dead wood systems,” in Saproxylic insects. Zoological monographs, ed. M. Ulyshen (Cham: Springer), 377–427. doi: 10.1007/978-3-319-75937-1_12
Bleiker, K. P., and Six, D. L. (2007). Dietary benefits of fungal associates to an eruptive herbivore: potential implications of multiple associates on host population dynamics. Environ. Entomol. 36, 1384–1396. doi: 10.1093/ee/36.6.1384
Bleiker, K. P., and Six, D. L. (2009). Effects of water potential and solute on the growth and interactions of two fungal symbionts of the mountain pine beetle. Mycol. Res. 113, 3–15. doi: 10.1016/j.mycres.2008.06.004
Bolyen, E., Rideout, J. R., Dillon, M. R., Bokulich, N. A., Abnet, C. C., Al-Ghalith, G. A., et al. (2019). Reproducible, interactive, scalable and extensible microbiome data science using QIIME 2. Nat. Biotechnol. 37, 852–857. doi: 10.1038/s41587-019-0209-9
Boone, C. K., Keefover-Ring, K., Mapes, A. C., Adams, A. S., Bohlmann, J., and Raffa, K. F. (2013). Bacteria associated with a tree-killing insect reduce concentrations of plant defense compounds. J. Chem. Ecol. 39, 1003–1006. doi: 10.1007/s10886-013-0313-0
Bracewell, R. R., and Six, D. L. (2014). Broadscale specificity in a bark beetle-fungal symbiosis: a spatio-temporal analysis of the mycangial fungi of the western pine beetle. Microb. Ecol. 68, 859–870. doi: 10.1007/s00248-014-0449-7
Bracewell, R. R., Vanderpool, D., Good, J. M., and Six, D. L. (2018). Cascading speciation among mutualists and antagonists in a tree–beetle –fungi interaction. Proc. R. Soc. B. 285:20180694. doi: 10.1098/rspb.2018.0694
Briones-Roblero, C. I., Rodríguez-Díaz, R., Santiago-Cruz, J. A., Zúñiga, G., and Rivera-Orduña, F. N. (2017a). Degradation capacities of bacteria and yeasts isolated from the gut of Dendroctonus rhizophagus (Curculionidae: Scolytinae). Folia. Microbiol. 62, 1–9. doi: 10.1007/s12223-016-0469-4
Briones-Roblero, C. I., Hernández-García, J. A., Gonzalez-Escobedo, R., Soto-Robles, L. V., Rivera-Orduña, F. N., and Zúñiga, G. (2017b). Structure and dynamics of the gut bacterial microbiota of the bark beetle, Dendroctonus rhizophagus (Curculionidae: Scolytinae) across their life stages. PLoS One 12:e0175470. doi: 10.1371/journal.pone.0175470
Cale, J. A., Ding, R., Wang, F., Rajabzadeh, R., and Erbilgin, N. (2019). Ophiostomatoid fungi can emit the bark beetle pheromone verbenone and other semiochemicals in media amended with various pine chemicals and beetle-released compounds. Fungal Ecol. 39, 285–295. doi: 10.1016/j.funeco.2019.01.003
Callahan, B. J., McMurdie, P. J., Rosen, M. J., Han, A. W., Johnson, A. J. A., and Holmes, S. P. (2016). DADA2: High-resolution sample inference from Illumina amplicon data. Nat. Methods. 13, 581–583. doi: 10.1038/nmeth.3869
Cano-Ramírez, C., Santiago-Hernández, A., Rivera-Orduña, F. N., García-Huante, Y., Zúñiga, G., and Hidalgo-Lara, M. E. (2016). Expression, purification and characterization of an endoglucanase from Serratia proteamaculans CDBB-1961, isolated from the gut of Dendroctonus adjunctus (Coleoptera: Scolytinae). AMB Express 6:63. doi: 10.1186/s13568-016-0233-9
Cardoza, Y. J., Klepzig, K. D., and Raffa, K. F. (2006). Bacteria in oral secretions of an endophytic insect inhibit antagonistic fungi. Ecol. Entomol. 31, 636–645. doi: 10.1111/j.1365-2311.2006.00829.x
Cheng, C., Wickham, J. D., Chen, L., Xu, D., Lu, M., and Sun, J. (2018). Bacterial microbiota protects an invasive bark beetle from a pine defensive compound. Microbiome 6:132. doi: 10.1186/s40168-018-0518-0
Cheng, C., Zhou, F., Lu, M., and Sun, J. (2015). Inducible pine rosin defense mediates interactions between an invasive insect-fungal complex and newly acquired sympatric fungal associates. Integr. Zool. 10, 453–464. doi: 10.1111/1749-4877.12138
Chiu, C. C., Keeling, C. I., Henderson, H. M., and Bohlmann, J. (2019). Functions of mountain pine beetle cytochromes P450 CYP6DJ1, CYP6BW1 and CYP6BW3 in the oxidation of pine monoterpenes and diterpene resin acids. PLoS One 14:e0216753. doi: 10.1371/journal.pone.0216753
Conow, C., Fielder, D., Ovadia, Y., and Libeskind-Hadas, R. (2010). Jane: a new tool for the cophylogeny reconstruction problem. Algorithms Mol. Biol. 5:16. doi: 10.1186/1748-7188-5-16
Dai, L., Li, H., Zheng, J., and Chen, H. (2022). Transcriptome analyses of the Chinese white pine beetle-fungal symbiont Leptographium qinlingensis under terpene stress or growth on host pine sawdust. Symbiosis 86, 17–31. doi: 10.1007/s13199-021-00822-z
Darriba, D., Taboada, G. L., Doallo, R., and Posada, D. (2012). jModelTest 2: more models, new heuristics and parallel computing. Nat. Methods. 9:772. doi: 10.1038/nmeth.2109
Davis, T. S. (2015). The ecology of yeasts in the bark beetle holobiont: a century of research revisited. Microb. Ecol. 69, 723–732. doi: 10.1007/s00248-014-0479-1
Davis, T. S., Hofstetter, R. W., Foster, J. T., Foote, N. E., and Keim, P. (2011). Interactions between the yeast Ogataea pini and filamentous fungi associated with the western pine beetle. Microb. Ecol. 61, 626–634. doi: 10.1007/s00248-010-9773-8
Deng, Y., Jiang, Y. H., Yang, Y., He, Z., Luo, F., and Zhou, J. (2012). Molecular ecological network analyses. BMC Bioinform. 13:113. doi: 10.1186/1471-2105-13-113
DiGuistini, S., Wang, Y., Liao, N. Y., Taylor, G., Tanguay, P., Feau, N., et al. (2011). Genome and transcriptome analyses of the mountain pine beetle-fungal symbiont Grosmannia clavigera, a lodgepole pine pathogen. Proc. Natl. Acad. Sci. U.S.A. 108, 2504–2509. doi: 10.1073/pnas.1011289108
Dohet, L., Gregoire, J. C., Berasategui, A., Kaltenpoth, M., and Biedermann, P. H. (2016). Bacterial and fungal symbionts of parasitic Dendroctonus bark beetles. FEMS Microbiol. Ecol. 92:fiw129. doi: 10.1093/femsec/fiw129
Durand, A. A., Bergeron, A., Constant, P., Buffet, J.-P., Déziel, E., and Guertin, C. (2015). Surveying the endomicrobiome and ectomicrobiome of bark beetles: the case of Dendroctonus simplex. Sci. Rep. 5:17190. doi: 10.1038/srep17190
Durand, A. A., Constant, P., Deziel, E., and Guertin, C. (2019). The symbiotic complex of Dendroctonus simplex: implications in the beetle attack and its life cycle. Bull. Entomol. Res. 109, 723–732. doi: 10.1017/S0007485319000051
Dysthe, J. C., Bracewell, R., and Six, D. L. (2015). Temperature effects on growth of fungal symbionts of the western pine beetle, Dendroctonus brevicomis. Fungal Ecol. 17, 62–68. doi: 10.1016/j.funeco.2015.05.010
Farr, D. F., and Rossman, A. Y. (2015). Fungal Databases. Systematic Mycology and Microbiology Laboratory. Available online at: https://nt.ars-grin.gov/fungaldatabases/ (accessed June 7, 2022).
Francke-Grossman, H. (1967). “Ectosymbiosis in wood-inhabiting insects,” in Symbiosis, ed. S. M. Henry (New York, NY: Academic Press), 141–205.
Gonzalez-Escobedo, R., Briones-Roblero, C. I., López, M. F., Rivera-Orduña, F. N., and Zúñiga, G. (2019). Changes in the microbial community of Pinus arizonica saplings after being colonized by the bark beetle Dendroctonus rhizophagus (Curculionidae: Scolytinae). Microb. Ecol. 78, 102–112. doi: 10.1007/s00248-018-1274-1
Goodsman, D. W., Erbilgin, N., and Lieffers, V. J. (2012). The impact of phloem nutrients on overwintering mountain pine beetles and their fungal symbionts. Environ. Entomol. 41, 478–486. doi: 10.1603/EN11205
Gouy, M., Guindon, S., and Gascuel, O. (2010). SeaView version 4: a multiplatform graphical user interface for sequence alignment and phylogenetic tree building. Mol. Biol. Evol. 27, 221–224. doi: 10.1093/molbev/msp259
Groussin, M., Mazel, F., and Alm, E. J. (2020). Co-evolution and co-speciation of host-gut bacteria systems. Cell Host Microbe. 28, 12–22. doi: 10.1016/j.chom.2020.06.013
Hammer, Ø., Harper, D. A. T., and Ryan, P. D. (2001). Past: paleontological statistics software package for education and data analysis. Palaeontol. Electron 4, 1–9.
Happ, G. M., Happ, C. M., and Barras, S. J. (1971). Fine structure of the prothoracic mycangium, a chamber for the culture of symbiotic fungi, in the southern pine beetle, Dendroctonus frontalis. Tissue Cell. 3, 295–308. doi: 10.1016/s0040-8166(71)80024-1
Harrington, T. C. (1993). “Diseases of conifers caused by species of Ophiostoma and Leptographium,” in Ceratocystis and Ophiostoma: taxonomy, ecology, and pathogenicity, eds M. J. Wingfield, K. A. Seifert, and J. F. Webber (St. Paul, MN: APS Press), 161–172.
Harrington, T. C. (2005). “Ecology and evolution of mycophagous bark beetles and their fungal partners,” in Ecological and evolutionary advances in insect-fungal associations, eds F. E. Vega and M. Blackwell (New York, NY: Oxford University Press), 257–291.
Held, B. W., Simeto, S., Rajtar, N. N., Cotton, A. J., Showalter, D. N., Bushley, K. E., et al. (2021). Fungi associated with galleries of the emerald ash borer. Fungal Biol. 125, 551–559. doi: 10.1016/j.funbio.2021.02.004
Henriques, J., Inácio, M. L., and Sousa, E. (2006). Ambrosia fungi in the insect-fungi symbiosis in relation to cork oak decline. Rev. Iberoam. Micol. 23, 185–188. doi: 10.1016/s1130-1406(06)70041-9
Hernández-García, J. A., Briones-Roblero, C. I., Rivera-Orduña, F. N., and Zúñiga, G. (2017). Revealing the gut bacteriome of Dendroctonus bark beetles (Curculionidae: Scolytinae): diversity, core members and co-evolutionary patterns. Sci. Rep. 7:13864. doi: 10.1038/s41598-017-14031-6
Hofstetter, R. W., Dinkins-Bookwalter, J., Davis, T. S., and Klepzig, K. D. (2015). “Symbiotic associations of bark beetles,” in Bark beetles: biology and ecology of native and invasive species, eds F. E. Vega and R. W. Hofstetter (San Diego, CA: Elsevier), 305–350.
Hu, X., Li, M., and Chen, H. (2015). Community structure of gut fungi during different developmental stages of the Chinese white pine beetle (Dendroctonus armandi). Sci. Rep. 5, 8411. doi: 10.1038/srep08411
Jordal, B. H., and Cognato, A. I. (2012). Molecular phylogeny of bark and ambrosia beetles reveals multiple origins of fungus farming during periods of global warming. BMC Evol. Biol. 12:133. doi: 10.1186/1471-2148-12-133
Kõljalg, U., Nilsson, H. R., Schigel, D., Tedersoo, L., Larsson, K.-H., May, T. W., et al. (2020). The taxon hypothesis paradigm—on the unambiguous detection and communication of taxa. Microorganims 8:1910. doi: 10.3390/microorganisms8121910
Krokene, P. (2015). “Conifer defense and resistance to bark beetles,” in Bark beetles: biology and ecology of native and invasive species, eds F. E. Vega and R. W. Hofstetter (San Diego, CA: Elsevier), 177–207.
Lanier, G. N., Hendrichs, J. P., and Flores, J. E. (1988). Biosystematics of the Dendroctonus frontalis (Coleoptera: Scolytidae) Complex. Ann. Ent. Soc. Am 81, 403–418.
Larkin, M. A., Blackshields, G., Brown, N. P., Chenna, R., McGettigan, P. A., McWilliam, H., et al. (2007). Clustal W and Clustal X version 2.0. Bioinformatics 23, 2947–2948. doi: 10.1093/bioinformatics/btm404
Lavrinienko, A., Jernfors, T., Koskimäki, J. J., Pirttilä, A. M., and Watts, P. C. (2021). Does intraspecific variation in rDNA copy number affect analysis of microbial communities? Trends Microbiol. 29, 19–27. doi: 10.1016/j.tim.2020.05.019
Lewinsohn, D., Lewinsohn, E., Bertagnolli, C. L., and Patridge, A. D. (1994). Blue-stain fungi and their transport structures on the Douglas-fir beetle. Can. J. Forest Res. 24, 2275–2283. doi: 10.1139/x94-292
Morales-Jiménez, J., Vera-Ponce de León, A., García-Domínguez, A., Martínez-Romero, E., Zúñiga, G., and Hernández-Rodríguez, C. (2013). Nitrogen-fixing and uricolytic bacteria associated with the gut of Dendroctonus rhizophagus and Dendroctonus valens (Curculionidae: Scolytinae). Microb. Ecol. 66, 200–210. doi: 10.1007/s00248-013-0206-3
Morales-Jiménez, J., Zúñiga, G., Ramírez-Saad, H. C., and Hernández-Rodríguez, C. (2012). Gut-associated bacteria throughout the life cycle of the bark beetle Dendroctonus rhizophagus Thomas and Bright (Curculionidae: Scolytinae) and their cellulolytic activities. Microb. Ecol. 64, 268–278. doi: 10.1007/s00248-011-9999-0
Nakashima, T., Iizawa, T., Ogura, K., Maeda, M., and Tanaka, T. (1982). Isolation of some microorganisms associated with five species of ambrosia beetles and two kinds of antibiotics produced by Xv-3 strain in these isolates. J. Fac. Agr. Hokkaido Univ. 61, 60–72.
Nguyen, N. H., Song, Z., Bates, S. T., Branco, S., Tedersoo, L., Menke, J., et al. (2016). FUNGuild: an open annotation tool for parsing fungal community datasets by ecological guild. Fungal Ecol 20, 241–248. doi: 10.1016/j.funeco.2015.06.006
Oh, D. C., Scott, J. J., Currie, C. R., and Clardy, J. (2009). Mycangimycin, a polyene peroxide from a mutualist Streptomyces sp. Org. Lett. 11, 633–636. doi: 10.1021/ol802709x
Pace, M. R. (2019). “Phloem: Cell Types, Structure, and Commercial Uses,” in Plant scince - structure, anatomy and morphogenesis in plants cultured in vivo and in vitro. eds A. Gonzalez, M. Rodriguez, and N. G. Săglam (London: IntechOpen), doi: 10.5772/intechopen.88162
Paine, T. D., and Birch, M. C. (1983). Acquisition and maintenance of mycangial fungi by Dendroctonus brevicomis LeConte (Coleoptera: Scolytidae). Environ. Entomol. 12, 1384–1386. doi: 10.1093/ee/12.5.1384
Paulson, J. N., Pop, M., and Bravo, H. C. (2013). MetagenomeSeq: Statistical analysis for sparse high-throughput sequencing. Bioconductor package. Available online at: http://www.cbcb.umd.edu/software/metagenomeSeq (accessed June 7, 2022).
Rivera, F. N., González, E., Gómez, Z., López, N., Hernández-Rodríguez, C., Berkov, A., et al. (2009). Gut-associated yeast in bark beetles of the genus Dendroctonus Erichson (Coleoptera: Curculionidae: Scolytinae). Biol. J. Linn. Soc. 98, 325–342. doi: 10.1111/j.1095-8312.2009.01289.x
Schneider, I. A., and Rudinsky, J. A. (1969). Mycetangial glands and their seasonal changes in Gnathotrichus retusus and G. sulcatus. Ann. Entomol. Soc. Am. 62, 39–43. doi: 10.1093/aesa/62.1.39
Scott, J. J., Oh, D. C., Yuceer, M. C., Klepzig, K. D., Clardy, J., and Currie, C. R. (2008). Bacterial protection of beetle-fungus mutualism. Science 322:63. doi: 10.1126/science.1160423
Shannon, P., Markiel, A., Ozier, O., Baliga, N. S., Wang, J. T., Ramage, D., et al. (2003). Cytoscape: a software environment for integrated models of biomolecular interaction networks. Genome Res. 13, 2498–2504. doi: 10.1101/gr.1239303
Shi, Z.-H., and Sun, J.-H. (2010). Immunocompetence of the red turpentine beetle, Dendroctonus valens LeConte (Coleoptera: Curculionidae: Scolytinae): variation between developments stages and sexes in populations of China. J. Insect Physiol. 56, 1696–1701. doi: 10.1016/j.jinsphys.2010.06.013
Six, D. L. (2012). Ecological and evolutionary determinants of bark beetle-fungus symbioses. Insects 3, 339–366. doi: 10.3390/insects3010339
Six, D. L. (2019). A major symbiont shift supports a major niche shift in a clade of tree killing bark beetles. Ecol. Entomol. 45, 190–201. doi: 10.1111/een.12786
Six, D. L., and Bentz, B. J. (2007). Temperature determines symbiont abundance in a multipartite bark beetle-fungus ectosymbiosis. Microb. Ecol. 54, 112–118. doi: 10.1007/s00248-006-9178-x
Six, D. L., and Bracewell, R. (2015). “Dendroctonus,” in Bark beetles: biology and ecology of native and invasive species, eds F. E. Vega and R. W. Hofstetter (San Diego, CA: Elsevier), 305–350.
Six, D. L., and Klepzig, K. D. (2021). Context dependency in bark beetle-fungus mutualisms revisited: assessing potential shifts in interaction outcomes against varied genetic, ecological, and evolutionary backgrounds. Front. Microbiol. 12:682187. doi: 10.3389/fmicb.2021.682187
Six, D. L., and Wingfield, M. J. (2011). The role of phytopathogenicity in bark beetle-fungus symbioses: a challenge to the classic paradigm. Annu. Rev. Entomol. 56, 255–272. doi: 10.1146/annurev-ento-120709-144839
Soto-Robles, L. V., López, M. F., Torres-Banda, V., Cano-Ramírez, C., Obregón-Molina, G., and Zúñiga, G. (2021). The bark beetle Dendroctonus rhizophagus (Curculionidae: Scolytinae) has digestive capacity to degrade complex substrates: functional characterization and heterologous expression of an α-amylase. Int. J. Mol. Sci. 22:36. doi: 10.3390/ijms22010036
Sullivan, B. T., Grady, A. M., Hofstetter, R. W., Pureswaran, D. S., Brownie, C., Cluck, D., et al. (2021). Evidence for semiochemical divergence between sibling bark beetle species: Dendroctonus brevicomis and Dendroctonus barberi. J. Chem. Ecol. 47, 10–27. doi: 10.1007/s10886-020-01233-y
Taylor, D. L., Walters, W. A., Lennon, N. J., Bochicchio, J., Krohn, A., Caporaso, J. G., et al. (2016). Accurate estimation of fungal diversity and abundance through improved lineage-specific primers optimized for illumina amplicon sequencing. Appl. Environ. Microbiol. 82, 7217–7226. doi: 10.1128/AEM.02576-16
Valerio-Mendoza, O., García-Roman, J., Becerril, M., Armendáriz-Toledano, F., Cuéllar-Rodríguez, G., Negrón, J. F., et al. (2019). Cryptic species discrimination in western pine beetle, Dendroctonus brevicomis LeConte (Curculionidae: Scolytinae), based on morphological characters and geometric morphometrics. Insects 10:377. doi: 10.3390/insects10110377
Valiev, A., Ogel, Z. B., and Klepzig, D. D. (2009). Analysis of cellulase and polyphenol oxidase production by southern pine beetle associated fungi. Symbiosis 49, 37–42. doi: 10.1007/s13199-009-0007-0
Vega, F. E., and Biedermann, P. H. W. (2020). On interactions, associations, mycetangia, mutualists and symbiotes in insect-fungus symbioses. Fungal Ecol. 44:100909. doi: 10.1016/j.funeco.2019.100909
Wang, H. M., Liu, F., Zhang, S. F., Kong, X. B., Lu, Q., and Zhang, Z. (2020). Epibiotic fungal communities of three Tomicus spp. infesting pines in southwestern China. Microorganisms 8:15. doi: 10.3390/microorganisms8010015
Whitney, H. S., and Farris, S. H. (1970). Maxillary mycangium in the mountain pine beetle. Science 167, 54–55. doi: 10.1126/science.167.3914.54
Xu, L. T., Lu, M., and Sun, J. H. (2016). Invasive bark beetle-associated microbes degrade a host defensive monoterpene. Insect. Sci. 23, 183–190. doi: 10.1111/1744-7917.12255
Xu, L., Lou, Q., Cheng, C., Lu, M., and Sun, J. (2015). Gut-associated bacteria of Dendroctonus valens and their involvement in verbenone production. Microb. Ecol. 70, 1012–1023. doi: 10.1007/s00248-015-0625-4
Keywords: mycetangia, mycobiome, ITS, symbiosis, bark beetles, Dendroctonus
Citation: Vazquez-Ortiz K, Pineda-Mendoza RM, González-Escobedo R, Davis TS, Salazar KF, Rivera-Orduña FN and Zúñiga G (2022) Metabarcoding of mycetangia from the Dendroctonus frontalis species complex (Curculionidae: Scolytinae) reveals diverse and functionally redundant fungal assemblages. Front. Microbiol. 13:969230. doi: 10.3389/fmicb.2022.969230
Received: 16 June 2022; Accepted: 18 August 2022;
Published: 16 September 2022.
Edited by:
Claudia S. L. Vicente, University of Évora, PortugalReviewed by:
Pepijn Wilhelmus Kooij, Universidade Estadual Paulista, BrazilAri Mikko Hietala, Norwegian Institute of Bioeconomy Research (NIBIO), Norway
Copyright © 2022 Vazquez-Ortiz, Pineda-Mendoza, González-Escobedo, Davis, Salazar, Rivera-Orduña and Zúñiga. This is an open-access article distributed under the terms of the Creative Commons Attribution License (CC BY). The use, distribution or reproduction in other forums is permitted, provided the original author(s) and the copyright owner(s) are credited and that the original publication in this journal is cited, in accordance with accepted academic practice. No use, distribution or reproduction is permitted which does not comply with these terms.
*Correspondence: Flor N. Rivera-Orduña, Zmxvcl8xNDEzQGhvdG1haWwuY29t; Gerardo Zúñiga, Y2Fwb3RlenVAaG90bWFpbC5jb20=