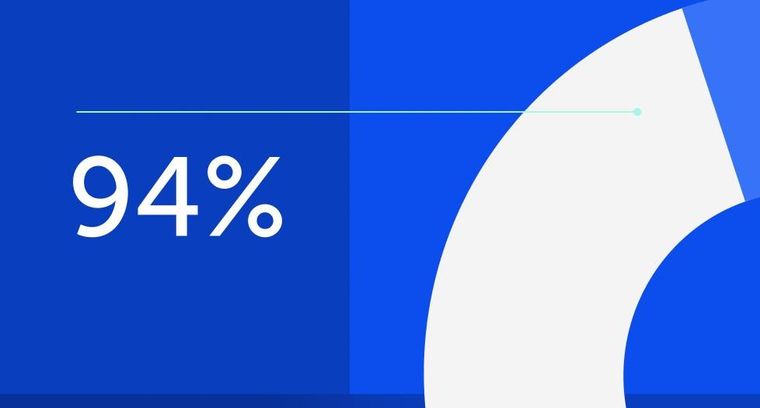
94% of researchers rate our articles as excellent or good
Learn more about the work of our research integrity team to safeguard the quality of each article we publish.
Find out more
REVIEW article
Front. Microbiol., 11 August 2022
Sec. Microbiotechnology
Volume 13 - 2022 | https://doi.org/10.3389/fmicb.2022.966226
This article is part of the Research TopicBiotechnological Advances in Bioremediation of Heavy Metal Polluted SoilsView all 9 articles
Heavy metal contamination in soils endangers humans and the biosphere by reducing agricultural yield and negatively impacting ecosystem health. In recent decades, this issue has been addressed and partially remedied through the use of “green technology,” which employs metal-tolerant plants to clean up polluted soils. Furthermore, the global climate change enhances the negative effects of climatic stressors (particularly drought, salinity, and extreme temperatures), thus reducing the growth and metal accumulation capacity of remediating plants. Plant growth-promoting bacteria (PGPB) have been widely introduced into plants to improve agricultural productivity or the efficiency of phytoremediation of metal-contaminated soils via various mechanisms, including nitrogen fixation, phosphate solubilization, phytohormone production, and biological control. The use of metal-tolerant plants, as well as PGPB inoculants, should hasten the process of moving this technology from the laboratory to the field. Hence, it is critical to understand how PGPB ameliorate environmental stress and metal toxicity while also inducing plant tolerance, as well as the mechanisms involved in such actions. This review attempts to compile the scientific evidence on this topic, with a special emphasis on the mechanism of PGPB involved in the metal bioremediation process [plant growth promotion and metal detoxification/(im)mobilization/bioaccumulation/transformation/translocation] and deciphering combined stress (metal and climatic stresses) tolerance.
Soil contaminated with heavy metals has become a serious worldwide problem due to geologic and anthropogenic activities, such as mining, fossil fuel combustion, application of agrochemicals, and so on. As heavy metals are non-biodegradable and extremely persistent in the environment, they can easily accumulate in different foods. Metal contamination of various foods, such as crops, meat, fish, milk, and eggs, threatens food safety. Metals contaminate agricultural soils, irrigation water, plants, and animals, resulting in their incorporation into the food chain and posing a significant threat to human health and ecosystems (Abdel-Rahman, 2022). The major sources of heavy metals and their harmful effects are summarized in Table 1. There are currently numerous methods for controlling heavy metal pollution. The advantages and disadvantages of different techniques are summarized in Table 2. Traditional remediation technologies for contaminated soil, such as cleaning, heat treatment, electrochemistry, and amendment application, often have complex processes that easily destroy soil structure and fertility. They are ineffective for treating both low concentration and large-scale heavy metal contamination in soils. Phytoremediation is a potential solution for remediating metal-polluted soils since it is a cost-effective plant-based approach (Ma et al., 2016a). During global climate change scenarios, plants are more severely and frequently subjected to episodes of climatic stress, such as high temperature, drought, and salinity, limiting their growth and performance. Furthermore, the direct (e.g., competition of ions) or indirect (e.g., alteration of soil physicochemical-biological properties) impact of climate change on metal bioavailability in soils may impede plant adaptation, making them more susceptible to stress and thus limiting the widespread application of phytoremediation (Rajkumar et al., 2013). Plant beneficial microorganisms (PBM), particularly plant growth-promoting bacteria (PGPB) create symbiotic relationships with plants, alleviating the toxicity of heavy metals, promoting multimodal tolerance of plants to metals and climatic stresses, and affecting the bioavailability of metals in soils (Ma et al., 2016b). For instance, PGPB can alleviate metal toxicity and alter metal bioavailability in soils through metal biosorption, bioaccumulation, redox reaction, mobilization, precipitation, and transformation (Ma et al., 2016a). They can also provide plants with multiplex tolerance to a variety of climatic stresses (such as drought and high salinity) by producing 1-aminocyclopropane-1-carboxylate deaminase (ACCD), siderophore, and phytohormone, and dissolving insoluble mineral nutrients (such as nitrogen, phosphorus, and potassium). These PGPB strains could also protect plants from phytopathogens by producing antibiotics and inducing induced systemic resistance (Grover et al., 2021). Understanding the interaction between plants and PBM has lots of potential for accelerating metal phytoremediation under various environmental stressors (e.g., salinity, drought, and extreme temperature). There have been very few investigations on plant-microbe associations for bioremediation of metal-polluted soils under climatic stresses.
Table 2. Advantages and disadvantages of the available remediation techniques for metal-contaminated soils.
The current review has discussed the underlying mechanisms of PGPB involved in the heavy metal bioremediation in response to metal alone or in combination with climatic conditions (e.g., drought, salt, and heat) have been discussed. The main objective is to provide an overview of recent advances in developing PGPB-assisted phytoremediation under various climatic stresses, including the strategies to improve remediating plants tolerance and biomass, metal detoxification, bioaccumulation, transformation, and translocation activities. This review also emphasizes the commercial application of PGPB to improve phytoremediation efficiency.
Plant growth-promoting bacteria are a type of bacteria that may colonize rhizosphere soils and plant tissues and stimulate plant growth through various plant growth-promoting (PGP) activities under different conditions (Hashem et al., 2019). PGPB can improve plant abiotic and biotic stress tolerance by directly modulating phytohormone levels and facilitating resource acquisition, and/or indirectly by protecting plants against phytopathogens through the production of antibiotics and siderophores (Backer et al., 2018). They may act as free-living or rhizosphere bacteria (that form specific symbiotic relationships with roots), endophytic bacteria (that can colonize plant interior tissues), Rhizobia spp., and cyanobacteria (Glick et al., 2012). Figure 1 shows the type of plant growth-promoting bacteria. They all use the same PGP methods (direct and indirect); however, there are distinctions among these bacteria. Endophytic bacteria are more valuable in real-world applications than rhizobacteria because of their stable living environment and closer contact with plants for nutrient supply (Afzal et al., 2019). It has been reported that a group of metal-resistant PGPB, such as Pseudomonas, Arthrobacter, Agrobacterium, Bacillus, Azoarcus, Azospirillum, Azotobacter, Burkholderia, Klebsiella, Alcaligenes, Serratia, Rhizobium, and Enterobacter species have great potential to promote the growth of various plants in the metal-contaminated environments (Enebe and Babalola, 2018). These metal-resistant PGPB were found to enhance plant metal tolerance by improving detoxification rates of plants, enzymes secreted by plant roots, and soil pH modification (Guo et al., 2020). Moreover, certain metal-resistant PGPB can also alter metal mobility and bioavailability, and consequently plant usage rate by releasing chelating agents, acidification, and redox changes (Verma and Kuila, 2019). Therefore, these metal-resistant PGPB strains can be used as a suitable candidate for metal phytoremediation to minimize the adverse impact of metals and enhance metal accumulation capacity of plants. A number of metal-resistant PGPB have been reported to improve plant bioaccumulation/phytoextraction capacity through the secretion of siderophores and organic acids, which improve metal bioavailability by reducing soil pH (Manoj et al., 2020). In contrast, some metal-resistant PGPB can release polymeric substances (such as glomalin and polysaccharides) that speed up metal phytostabilization by limiting their mobility (Ma et al., 2016a).
Figure 1. Type of plant growth-promoting bacteria (Glick et al., 2012).
However, microbes have a strong dependence on the environment, and changes in environmental conditions can modulate the diversity, abundance, and functioning of bacteria (Afzal et al., 2019). Sánchez-Marañón et al. (2017) studied bacterial communities in eight soils selected along a soil-forming gradient and found that distinct bacterial distributions were positively connected to organic carbon, water-stable aggregates, porosity, water, and acidity. Furthermore, endophytic microbiota can be influenced by the age, genotype, nutritional status, and geographical location of the host plants (Ahmed et al., 2020). Carvalhais et al. (2013) confirmed that the transcriptional changes of B. amyloliquefaciens caused by the nutrient-deficient corn exudates were significantly correlated with the concentrations of amino acids, aspartic acid, valine, and glutamic acid in the root exudates. Furthermore, differences in microbial communities can result from host plant preferences for stress conditions. Wu et al. (2020a) reported the growth of B. subtilis at high salinity reduces the cell expansion pressure due to the passage of water through an osmotic gradient. Similarly, Xu et al. (2018) also demonstrated that drought delayed the development of early root microbiota in Sorghum bicolor. Nevertheless, inoculation of climatic stress-resistant PGPB has great potential to increase plant resistance/tolerance to such environmental stresses. Bruno et al. (2020) pointed out that Bacillus cereus improved the growth of S. bicolor and its phytoremediation potential in Cr-contaminated soil at elevated atmospheric temperature by producing siderophores and indole-3-acetic acid (IAA).
The microbiome is essential for plant growth and function, particularly PGPB play a key role in plant growth regulation via phytohormone production, plant nutrient acquisition, and abiotic and biotic stress alleviation, which enable plants to tolerate high concentrations of heavy metals and thus better survive in challenging conditions. Figure 2 depicts the mechanism of the synergistic effect of PGPB on the phytoremediation of metal-contaminated soils. PGPB could promote plant growth directly and/or indirectly under metal stress. The direct plant growth promotion by PGPB involves producing phytohormones (e.g., auxin, cytokinin, gibberellin, abscisic acid, and ethylene), or facilitating plant nutrient uptake (e.g., nitrogen, phosphorus, potassium, etc.; Figure 2A). Since the interaction between the antibacterial activity of PGPB and nutrient competition inhibits the growth of pathogenic bacteria, the production of antibacterial compounds and the coexistence of pathogens enhance ISR and indirectly promote plant growth (Figure 2B). One or more of these mechanisms can be used by specific PGPB to enhance plant resistance to environmental stresses. PGPB also can adsorb metals through coordinate, chelate, and ion exchange. Under climatic stresses, PGPB can also effectively change metal bioavailability through mobilization, stabilization, and transformation, thereby improving bioremediation efficiency and reducing the climatic stress effect by regulating the antioxidant enzyme activity and ion balance in plants (Figure 2C).
Figure 2. Mechanism of the synergistic effects of PGPB on the bioremediation of metal-contaminated soils. (A) Direct mechanism of plant growth promotion; (B) Indirect mechanism of plant growth promotion; (C) Mechanism of PGPB affecting metal under abiotic stress.
The biomass of remediating plants and soil metal bioavailability are key factors influencing phytoremediation efficiency. Nitrogen, phosphorus, potassium, and other minerals are essential nutrients for plant growth. Although there are many phosphorus and potassium elements present in soils, most of them are insoluble and not bioavailable for plants. The existence of metals can aggravate the loss of nutrients in soils, making them unable to be effectively absorbed and utilized by plants (Ashraf et al., 2017; Ahemad, 2019). Metals can also have a significant impact on plant growth and development. Many studies have shown that when plants are grown under metal stress conditions, the membrane system of plants is damaged, and then the structure and function of organelles are affected, and various physiological and biochemical processes (such as chlorophyll content, photosynthesis rate, biomass reduction, and so on) in their tissues are impaired (Zhu et al., 2020). In metal-contaminated soil, PGPB can improve plant tolerance to such stresses (metal and other climatic stresses) and stimulate plant growth by maintaining nutrient status and adjusting phytohormonal balance through the production of plant growth regulators. Several studies have indicated that PGPB contributes significantly to phytohormone production, which can not only regulate plant growth, regular development, and physiological processes and but also control biological and non-biological stress responses (Afzal et al., 2019; Hewage et al., 2020). Chen et al. (2017) reported that under the stress of Zn and Cd, Pseudomonas fluorescens can promote the growth and physiological indicators (above ground chlorophyll and enzyme activity) of Sedum alfredii by producing IAA, and improve plant Cd absorption by regulating the expression and transport genes of Cd. Furthermore, some IAA-producing PGPB could increase plant uptake of nutrients and water and reduce the stress effects of salt and drought on plants by changing the roots system architecture (Etesami and Maheshwari, 2018). Pan et al. (2019) demonstrated the potential of abscisic acid (ABA)-producing B. subtilis to minimize Cd accumulation in Arabidopsis thaliana. A number of bacteria with gibberellin acid production capacity alleviate metal toxicity by reducing Cd uptake and lipid peroxidation, altering hormonal balance, and regulating activities of proteases, catalase, and peroxidase (Etesami, 2018). Moreover, PGPB containing ACCD can help plants to cleave the synthesis ethylene precursor 1-aminocyclopropane-1-carboxylate by metabolizing it into α-butanone acid and ammonia, thereby alleviating the ethylene level in plants and improving their climatic stress tolerance (Vejan et al., 2016).
Microorganisms can also improve plant growth directly via nitrogen fixation, phosphorus dissolution, and potassium dissolution (Ashraf et al., 2017). Stenotrophomonas rhizophila and B. amyloliquefaciens were able to fix nitrogen, thus providing abundant nitrogen to Brassica napus (Liu et al., 2021). Besides, certain PGPB like Klebsiella variicola can convert insoluble phosphate to soluble forms through the secretion of enzymes (phosphonates and C–P lyases) and organic acids (citric acid oxalic, fumaric, and malic; Manoj et al., 2020), thereby improving the phosphorus availability in the rhizosphere under metal stress. Potassium-solubilizing bacteria can form biofilms on the surface of rhizosphere minerals by producing capsular polysaccharides, hydroxyl anions, iron carriers, and extracellular enzymes, as well as dissolve K-containing minerals in soils and effectively release K by synthesizing organic and inorganic acids (Etesami and Maheshwari, 2018). Many recent investigations have revealed that a number of PGPB, namely Pseudomonas, Bacillus, Klebsiella, and Pantoea, can release K from insoluble minerals such as mica and illite through a variety of mechanisms (including acidolysis, chelation, exchange reactions, and complexolysis), including the production of organic acids (Bakhshandeh et al., 2017). Some microorganisms can absorb iron from the siderophore-Fe complex through chelation degradation and release of iron, direct uptake of siderophore-Fe complex and ligand exchange (Ma et al., 2016b). Agarwal et al. (2020) found that Staphylococcus warneri GL1, B. velezensis GL3, GL5, and GMC2, isolated from Gnetum gnemon, are able to secrete various siderophores with high affinity for Fe3+, effectively inhibiting the growth of Ralstonia solanacearum in the rhizosphere.
Over the past 2 decades, there has been a better understanding of antibiotics as the basis of the biological control mechanism of PGPB and a variety of antibiotics have been identified such as amphibious steroids, 2,4-diacetylphloroglucinol, hydrogen cyanide (HCN), oomycin A, phenazine, pyoluteorin, pyrrolidin tensin, and troponin (Compant et al., 2005). Pseudomonas fluorescens can inhibit the root rot of Nicotiana tabacum caused by Thielaviopsis basicola by synthesizing pyocyanin and 2,4-diacetyl fluorescein (Morales-Cedeño et al., 2021). Competition is one of the important mechanisms of PGPB’s resistance, including nutrition competition and locus competition. Through high-density colonization in plant rhizosphere or tissues, PGPB competes with indigenous microorganisms in the same micro-environment for oxygen, nutrition, and space (Compant et al., 2005). Induced systemic resistance (ISR) is the term being used for microbe-mediated induce plant resistance to infection by pathogenic fungi, bacteria, viruses, nematodes, and pests (Manoj et al., 2020). ISR is a central mechanism for Pseudomonas, Trichoderma, and Bacillus to protect plant against various pathogens (Saeed et al., 2021). In addition, lipopolysaccharide, flagellum and siderophores produced by PGPB can also cause ISR in plants (Ahmed et al., 2020). Inoculation with hydrogen cyanide-producing Brevibacterium casei MH8a significantly increased the biomass and accumulation of Cd, Zn, and Cu in Sinapis alba (Plociniczak et al., 2016). This is probably due to the potential of bacterial HCN to enhance plant growth and metal mobilization (Manoj et al., 2020). Recently, the release of stress-related volatile compounds was also found to increase plant biomass, yield, and survival under water stress (Etesami and Glick, 2020).
Climatic stresses have a significant impact on metal bioavailability. For instance, extreme temperatures can disrupt the nutrient and metal pathway by dissolving organic matter, decomposing microbial cells and destroying soil aggregates, and altering metal bioavailability, absorption, and distribution in plant tissues (Rajkumar et al., 2013). Li et al. (2011) found that higher temperature increased Cd accumulation in roots while decreased root elongation of Triticum aestivum in Cd-contaminated soils. This is because higher temperatures increased Cd toxicity to plant roots by increasing Cd accumulation and changing the subcellular distribution of Cd. Furthermore, changes in soil moisture can affect soil pH, EH, calcium carbonate, soluble organic content, and the electrochemical characteristics of the soil surface, all of which have an indirect impact on metal distribution in the soil solid–liquid phase and thus metal bioavailability. Pascual et al. (2004) noticed lower bioavailability of Zn, Cu, Mn, and Ni concentrations in soils when water was scarce. This was ascribed to a lower rate of soil mineralization as a result of drought stress. In response, increased metal deposition in the leaves has the ability to improve drought stress resistance and delay the negative consequences by reducing stratum corneum transpiration or increasing osmotic pressure in cells (Rajkumar et al., 2013).
Heavy metals are commonly present in both bioavailable and non-bioavailable forms in soils. The mobility and solubility of metals in soils are considered to be important factors influencing plant extraction efficiency (Ma et al., 2016b). Metal-resistant PGPB can mobilize metals and thus increase metal availability in the soil environment by secreting various organic acids (such as oxalic acid, acetic acid, and citric acid) and biosurfactants. Wu et al. (2018) noted that the inoculation of Buttiauxella sp. SaSR13 increased the content of root exudates (especially malic and oxalic acids) of S. alfredii, resulting in significant increases in the bioavailability and plant uptake of Cd. Additionally, biosurfactants can promote the entry of hydrophobic contaminants into the aqueous phase by solubilizing and micellizing the contaminants. These micelles and dissolved contaminants allow metal removal via soil washing or make them easily absorbed by plants (Akbari et al., 2018). Therefore, biosurfactant-producing microorganisms present in contaminated soils can effectively enhance metal mobility (Lal et al., 2018). San Martín et al. (2021) demonstrated that rhamnolipid-producing Pseudomonas Y3-B1A achieved a maximum vanadium removal efficiency of 85.5%.
Metal-resistant PGPB can also mobilize metal through biomethylation, leading to their volatilization. Certain PGPB can transfer methyl groups to metals (Pb and Se, etc.) to form methylated metal compounds with altered volatility, solubility, and toxicity (Ahemad, 2019). Microbial methylation of arsenic (As) raises trace levels of As species like monomethylarsinic acid and dimethylarsinic acid (DMA) in soils. Methylated As is absorbed by plant roots more slowly than inorganic As. DMA does not form compounds with plant chelating agents and is not quenched in vacuoles, allowing for efficient DMA transport inside the plants (Bali and Sidhu, 2021). Zhang et al. (2015) also proposed that As trioxide S-adenosylmethionine methyltransferase of Pseudomonas alcaligenes play a major role in the methylation and detoxification of As (III), which can be used in the bioremediation of As-contaminated environment.
The combination of metals with extracellular substances (e.g., anionic functional groups and extracellular polymers) can reduce metal bioavailability in soils, therefore reducing metal absorption or migration to aboveground plant parts. For instance, many metals can be efficiently immobilized in soils by combining with anionic functional groups on the cell surface (e.g., mercapto, carboxyl, hydroxyl, sulfonate, amine, and amide groups; Jacob et al., 2018). These substances reduce metal toxicity by forming complexes or effective barriers around cells (Ahemad, 2019). Some PGPB can also reduce metal bioavailability in soils through precipitation, alkalization, and complexation processes. The inorganic acids secreted by PGPB (e.g., hydrogen sulfide, bicarbonate, and phosphate) can also react rapidly with certain dissolved metals (Cu, Fe, Zn, and Pb) to form insoluble precipitates (Ma et al., 2016b).
Extracellular polymer substances (EPS) produced by PGPB are biosynthetic polymers composed mainly of polysaccharides, proteins, uronic acid, hummus, lipids, and other compounds. A previous study noted that bacterial EPS play a variety of biological activities in microbes and plants (Manoj et al., 2020). EPS generated by PGPB was shown to bind firmly to potentially harmful trace elements and capture precipitated metal sulfides and oxides to form organic metal complexes, enhancing resistance to toxic trace elements (Etesami and Maheshwari, 2018). The role of PGPB in the biosorption of Cs+ was confirmed by the production of EPS and biofilm formation of Nocardiopsis sp. (Sivaperumal et al., 2018). Silambarasan et al. (2019b) discovered that Rhodotorula sp. CAH2 could survive up to 6 mmol L−1 Al and produce EPS consisting of glucose, mannose, and galactose even under multiple stress conditions (salt and drought), along with the yield increasing as the stress level increased.
Phosphate solubilized by PGPB can also precipitate metals as metal accumulation in bacterial biomass is mediated by phosphatases that release inorganic phosphates from supplied organophosphate donor molecules (e.g., glycerol 2-phosphate) and metal cations precipitate on the biomass as phosphates (Gadd, 2004). In addition, microbial-induced carbonate precipitation (MICP) has been proposed as a viable bioremediation approach for metal immobilization. In MICP, carbonates can bind to the metals (e.g., Pb2+ and Cu2+) on the surface, after which these metal elements change from soluble forms to insoluble forms, thus reducing their toxicity (Tamayo-Figueroa et al., 2019). The MICP caused by B. pasteurii ATCC 11859 maintained the microbial growth while reducing the available Pb content in the soil, resulting in a decrease in Pb extraction and available Pb content by 76.34 and 41.65%, respectively (Chen et al., 2021).
The valence state of the metal determines its toxicity. The oxidation–reduction process of metal by bacteria results in various chemical transformations of metal, affecting their shape and mobility in soils, which is regarded as an essential detoxifying mechanism (Ma et al., 2016b). Metal ions’ redox reactions can be regulated by PGPB via cell metabolism, and metals could be converted into non-bioavailable states in the rhizosphere to reduce their toxic effects on plants (Sharma, 2021). It has been found that variation in metal-reducing bacteria can catalyze the reduction reaction and use metal to replace Fe3+ and S0 as terminal electron receptors in anaerobic respiration (Yin et al., 2019).
Enzyme-mediated reduction in toxic metals to less destructive forms is another popular strategy for reducing metal toxicity, which helps to improve microbial resistance to metal ions. These enzymes cleave bonds and use the energy generated by biochemical reactions to assist in the transfer of electrons from one compound to another (Voica et al., 2016). Harmful pollutants are eventually oxidized to innocuous molecules as a result of these processes. Furthermore, these enzymes aid in the humification of various phenolic compounds produced by lignin degradation in the soil environment, as well as the detoxification of various xenobiotics, such as aniline or phenolic compounds via chemical interactions (Jacob et al., 2018). Giovanella et al. (2016) found that Pseudomonas B50A removed 86% of Hg in the medium. This is probably due to the fact that Hg (II) reductase produced by strain B50A could effectively reduce Hg (II) to Hg (0) to reduce its toxicity.
The metal detoxification processes induced by PGPB have a significant influence on phytoremediation efficiency. Metal biosorption and bioaccumulation by bacteria, as well as the synthesis of plant hormones, ACCD, and other secretions, have all been proven to improve plant resistance to metals (Etesami and Maheshwari, 2018; Yaashikaa et al., 2021). Biosorption and bioaccumulation of inorganic and organic pollutants are determined by interaction traits of biomass and concentration of pollutants. In biosorption, the contaminants adhere to the surface of the cell wall, whereas in bioaccumulation, the contaminants accumulate within the cells (Ma et al., 2016b; Priyadarshanee and Das, 2021). Huang et al. (2020a) found that B. subtilis had significant biosorption potential, since it adsorbed about 10–20 mg L−1 concentration of Cd2+. This type of biosorption phenomenon can reduce the pollutants (especially metals) toxicity to plants. In another study, Sedlakova-Kadukova et al. (2019) proved that Streptomyces K11 isolated from alkaline brown mud disposal site considerably reduced the Zn toxicity through extracellular accumulation and chelation, which are related to its Zn tolerance and high bioaccumulation efficiency.
The use of genetically engineered microorganisms (GEMs) is also a promising strategy to clean up metal-polluted soils. Transgenic methods are not only used to convert functional genes but also to elevate particularly recognized promoters to existing gene functions connected with metal accumulation/translocation/detoxification mechanisms and introduce them to target bacteria (Pratush et al., 2018). As a low molecular weight, cysteine-rich protein, metallothioneins (MTs) found in many bacteria have the ability to bind metals and form complex biochemical structures (Venegas-Rioseco et al., 2022). Some gene transformation experiments have convincingly demonstrated that MTS produced by PGPB (e.g., P. putida and Mycobacterium tuberculosis) can improve plant tolerance to metals (Mierek-Adamska et al., 2017; Nanda et al., 2019). Li et al. (2021) proved that E. coli cells expressing SUMO-ShMT3 bioaccumulated Cd2+, Cu2+, and Zn2+. The biofilm-forming marine bacterium P. aeruginosa N6P6 possessing the bmtA gene resisted a variety of metals (e.g., Pb, Cd, Hg, Cr, and Zn; Kumari and Das, 2019).
Since several elements in the promoter region of the metals responsive gene can be activated by plant hormones and growth regulators, the relationship between these regulator compounds and metal chelator phytochelatins is very important, which are in the first line of heavy metal defense mechanism is critical (Pal et al., 2018). Phytochelatins are enzymatically synthesized from glutathione by phytochelatin synthase activity in the presence of metal and their synthesis also initiates/transforms the entities of metal anions (Ag, Au, Cd, Cu, Hg, Pb, and Zn) and cations (As; Ozyigit et al., 2020). de Souza and Vicente (2020) proved that recombinant E. coli clones expressing the synthetic phytochelatin EC20 have higher Cd2+ biosorption capacity and tolerance than that without EC20. Heavy-metal ATPases (HMA), a subfamily of P-type HMA transporters, are found in a wide range of microorganisms. The energy released by ATP hydrolysis is mostly used to power the transmembrane transport of some metal ions, such as Ag+, Zn2+, Cd2+, Cu2+, and Ni2+ (Yang et al., 2022). Begum et al. (2019) showed that HSP70 and HMA3 genes (a member of the HMA family) were highly expressed in Panicum virgatum inoculated with P grimontii and P. vagans under Cd stress, resulting in an increase in the biomass and IAA yield in inoculated plants, but a decrease in Cd accumulation. Both the natural resistance-associated macrophage protein family and the yellow streak-like transporters are also responsible for the absorption, transport, and detoxification of transition metals (Chowdhury et al., 2021; Yang et al., 2022).
The application of these GEMs is a very effective method to remove pollutants from the environment. However, the application of GEMs can affect the natural ecosystem, posing risks to the environment (Hussain et al., 2018). GEMs are considered a competitive alien species to the ecosystem and their introduction may reduce microbial biodiversity in the ecosystem. Besides, they may have adverse effects on human health, causing cancer and other genetic diseases (Saravanan et al., 2022). Undoubtedly, GEMs have potential ecological risks, but it is possible to find an efficient way to implement GEMs in the bioremediation of metal-polluted soils in the future through technical safeguards and innovation. For instance, some countries have issued necessary guidelines for assessing and monitoring the risks of GEMs in the environment, emphasizing risk stratification when applying GEMs for bioremediation (Wu et al., 2021). There have been recent attempts to design and track GEMs including the development of a set of criteria for the utilization of GEMs (Ezezika and Singer, 2010). In addition, another containment approach mainly involves designing “suicidal GEMs,” when the pollutants are degraded, the killer gene is activated and the GEMs are then eradicated (Rebello et al., 2021).
The use of PGPB in bioremediation has become more and more popular due to their abilities to detoxify and degrade toxins and promote plant growth. Silambarasan et al. (2020) demonstrated that the inoculation with Pseudomonas citronellolis SLP6 improved bud and root growth (length, fresh, and dried biomass), chlorophyll content, antioxidant enzyme activity, and Cu uptake in roots and shoots under Cu and Cu+ salt stress. Interestingly, they concluded that the P. citronellolis SLP6 amalgamation could be an effective approach for phytostabilization in Cu-contaminated saline soils. Accordingly, Bruno et al. (2021) reported that the inoculation of multi-metal (MM) and increased atmospheric temperature (IAT) tolerant B. cereus TCU11 significantly improved the growth and phytoextraction (Pb, Zn, Ni, Cu, and Cd) potential of Zea mays in metal-contaminated soils. However, most PGPB applications in metal bioremediation are done in pot or greenhouse experiments, with in situ investigations in field conditions being rare. The field experiment conducted by Ren et al. (2019) showed that B. cepacia J62 increased the contents of ascorbic acid and glutathione in B. napus and reduced the oxidative stress caused by metals. The successful colonization contributed to increasing the biomass and the total Cu absorption (67.91%). Prapagdee and Khonsue (2015) found that the Cd accumulation in the root, ground tissue, and whole plant increased by 1.2-, 1.4-, and 1.1-fold, respectively, after inoculation of Arthrobacter sp. with Ocimum gratissimum for 2 months in the field conditions. The applications of PGPB in metal phytoremediation in the past 5 years are summarized in Table 3.
Furthermore, the use of microbial agents in soil bioremediation via bioaugmentation techniques would be extremely beneficial to the industrialization of microbial inoculum. Surprisingly, it also boosted the commercialization and market demand for various microbial inoculations. Microbial agents are abundant in high-activity beneficial PGPB, such as N-fixing bacteria and K-dissolving bacteria. These microorganisms’ metabolic activities can effectively reduce metal concentrations and toxicity in the environment (Ma et al., 2016a). Notably, B. subtilis has been produced and sold under the trade names RhizoVital® and FZB24® TB for use in alleviating environmental stress and promoting plant growth (Ngalimat et al., 2021). Pseudomonas fluorescens has also been used to produce commercial inoculants under the trade names Conquer and Victus (Suyal et al., 2016). Moreover, some inoculant products currently on the market contain several different microorganisms (O’Callaghan et al., 2020). Wang et al. (2020) noted that the combination of Enterobacter sp. and Comamonas sp. can efficiently fix Cd. In addition, various inorganic materials (mainly clay and talc), organic materials (peat, charcoal, and plant waste materials), and polymers (polysaccharides, protein, and synthetic polymers) were used as carriers for PGPB encapsulation (Szopa et al., 2022). Alginate hydrogels seem to be a successful commercial product. Su et al. (2021) noted that the maximum adsorption capacity of Cu on multilayer calcium alginate beads containing diatom bacteria and B. Subtilis can reach 141.34 mg g−1. Bacillus strains immobilized in alginate macrobeads were also found to enhance drought stress adaptation of Guinea grass (Mendoza-Labrador et al., 2021). Tu et al. (2020) used corn stalk biochar and Pseudomonas sp. as materials and illustrated the stabilization mechanism of biochar-loaded microbial inoculum on Cd-and Cu-contaminated soils.
This review has addressed the mechanism of PGPB promoting plant growth and enhancing plant resistance under biotic and abiotic stress and the function in response to metal bioavailability and toxicity. This has significant scientific and practical implications for the use of PGPB in the phytoremediation of metal-contaminated soils as well as an understanding of the interaction between external pressure factors and biological processes. However, due to the nature of PGPB themselves, there are limitations in their utilization process. First, the genetic stability of PGPB is poor and easy to change, making them difficult to remove all pollutants. Second, there is a competitive survival relationship between PGPB and indigenous microbes, and eventually, these PGPB strains may be eliminated due to competitive failure. Finally, PGPB are easily affected by other factors such as external environment temperature, soil pH, and so on, thereby hampering the bioremediation efficiency. More research is needed to better understand the interaction between major factors namely metal, soil, microorganisms and plants.
We should pay close attention to how PGPB is employed in actual soil rehabilitation. Soil pH, humidity, and other environmental parameters could affect the efficiency of bioremediation. Compare with the studies of PGPB strain under laboratory conditions, the research on the field and in situ remediation experiments of PGPB strain under different environmental conditions is still very scarce.
The effects of different inoculation or application methods of PGPB on the phytoremediation efficiency of metal-polluted soil were explored. The appropriate inoculation method not only can change the soil nutritional status and directly affect PGPB survival and colonization efficiency but also can indirectly affect metal bioavailability in soils by changing the quantity and composition of root exudates of host plants.
There is a need to strengthen the utilization and safe disposal of post-remediation materials. Some bioaccumulating plants could produce certain harmful biomass after soil remediation. If these dry materials cannot be effectively treated, the original significance of bioremediation will be compromised. Therefore, the follow-up treatment of bioremediation technology and the recovery and treatment of metal in soils also have great research value.
It is necessary to develop additional research to analyze and anticipate how metals may influence plant development, metal accumulation, and ecophysiological responses in soils as a result of global climate change. The exact repercussions of climate change on plant–metal interactions in the future are difficult to anticipate due to the complicated interactions between various metals. Furthermore, much of the research lacks information on the behavioral dynamics and metabolomics of PGPB under environmental stresses. As a result, we need to improve our understanding of rhizosphere micro-ecological processes at the molecular level and choose the best couple of plants and PGPB to provide theoretical direction for long-term pollution decontamination.
YM developed the ideas and wrote the manuscript, and was the project sponsor. YW wrote the first draft of the manuscript. YM, MN, XS, XC, ZL, and DN revised the manuscript. All authors contributed to the article and approved the submitted version.
This work is carried out at the College of Resources and Environment, Southwest University, supported by the Fundamental Research Funds for the Central Universities (No. SWU 020010), the Natural Science Foundation of Chongqing (No. cstc2021jcyj-msxmX0827), and Chongqing Returned Overseas Students’ Entrepreneurship and Innovation Support Program (No. cx2021001).
The authors declare that the research was conducted in the absence of any commercial or financial relationships that could be construed as a potential conflict of interest.
All claims expressed in this article are solely those of the authors and do not necessarily represent those of their affiliated organizations, or those of the publisher, the editors and the reviewers. Any product that may be evaluated in this article, or claim that may be made by its manufacturer, is not guaranteed or endorsed by the publisher.
Abdelkrim, S., Jebara, S. H., and Jebara, M. (2018). Antioxidant systems responses and the compatible solutes as contributing factors to lead accumulation and tolerance in Lathyrus sativus inoculated by plant growth promoting rhizobacteria. Ecotoxicol. Environ. Saf. 166, 427–436. doi: 10.1016/j.ecoenv.2018.09.115
Abdel-Rahman, G. N. (2022). Heavy metals, definition, sources of food contamination, incidence, impacts and remediation: a literature review with recent updates. Egypt. J. Chem. 65, 419–437. doi: 10.21608/EJCHEM.2021.80825.4004
Afzal, I., Shinwari, Z. K., Sikandar, S., and Shahzad, S. (2019). Plant beneficial endophytic bacteria: mechanisms, diversity, host range and genetic determinants. Microbiol. Res. 221, 36–49. doi: 10.1016/j.micres.2019.02.001
Agarwal, H., Dowarah, B., Baruah, P. M., Bordoloi, K. S., Krishnatreya, D. B., and Agarwala, N. (2020). Endophytes from Gnetum gnemon L. can protect seedlings against the infection of phytopathogenic bacterium Ralstonia solanacearum as well as promote plant growth in tomato. Microbiol. Res. 238:126503. doi: 10.1016/j.micres.2020.126503
Ahemad, M. (2019). Remediation of metalliferous soils through the heavy metal resistant plant growth promoting bacteria: paradigms and prospects. Arab. J. Chem. 12, 1365–1377. doi: 10.1016/j.arabjc.2014.11.020
Ahmed, A., Munir, S., He, P. F., Li, Y. M., He, P. B., Wu, Y. X., et al. (2020). Biocontrol arsenals of bacterial endophyte: an imminent triumph against clubroot disease. Microbiol. Res. 241:126565. doi: 10.1016/j.micres.2020.126565
Akbari, S., Abdurahman, N. H., Yunus, R. M., Yunus, R. M., Fayaz, F., and Alara, O. R. (2018). Biosurfactants—a new frontier for social and environmental safety: a mini review. Biotechnol. Res. Int. 2, 81–90. doi: 10.1016/j.biori.2018.09.001
Akhtar, M. J., Ullah, S., Ahmad, I., Rauf, A., Nadeem, S. M., Khan, M. Y., et al. (2018). Nickel phytoextraction through bacterial inoculation in Raphanus sativus. Chemosphere 190, 234–242. doi: 10.1016/j.chemosphere.2017.09.136
Ali, A., Guo, D., Li, Y. M., Shaheen, S. M., Wahid, F., Antoniadis, V., et al. (2021). Streptomyces pactum addition to contaminated soils improved soil quality and plant growth and enhanced metals phytoextraction by wheat in a green remediation trial. Chemosphere 273:129692. doi: 10.1016/j.chemosphere.2021.129692
Ashraf, M. A., Hussain, I., Rasheed, R., Iqbal, M., Riaz, M., and Arif, M. S. (2017). Advances in microbe-assisted reclamation of heavy metal contaminated soils over the last decade: A review. J. Environ. Manag. 198, 132–143. doi: 10.1016/j.jenvman.2017.04.060
Backer, R., Rokem, J. S., Ilangumaran, G., Lamont, J., Praslickova, D., Ricci, E., et al. (2018). Plant growth-promoting rhizobacteria: context, mechanisms of action, and roadmap to commercialization of biostimulants for sustainable agriculture. Front. Plant Sci. 9:1473. doi: 10.3389/fpls.2018.01473
Bakhshandeh, E., Pridashti, H., and Lendeh, K. S. (2017). Phosphate and potassium-solubilizing bacteria effect on the growth of rice. Ecol. Eng. 103, 164–169. doi: 10.1016/j.ecoleng.2017.03.008
Balali-Mood, M., Naseri, K., Tahergorabi, Z., Khazdair, M. R., and Sadeghi, M. (2021). Toxic mechanisms of five heavy metals: mercury, lead, chromium, cadmium, and arsenic. Front. Pharmacol. 12:643972. doi: 10.3389/fphar.2021.643972
Bali, A. S., and Sidhu, G. P. S. (2021). Arsenic acquisition, toxicity and tolerance in plants—From physiology to remediation: a review. Chemosphere 283:131050. doi: 10.1016/j.chemosphere.2021.131050
Banik, A., Pandya, P., Patel, B., Rathod, C., and Dangar, M. (2018). Characterization of halotolerant, pigmented, plant growth promoting bacteria of groundnut rhizosphere and its in-vitro evaluation of plant-microbe protocooperation to withstand salinity and metal stress. Sci. Total Environ. 630, 231–242. doi: 10.1016/j.scitotenv.2018.02.227
Begum, N., Hu, Z. Y., Cai, Q. S., and Lou, L. Q. (2019). Influence of PGPB inoculation on HSP70 and HMA3 gene expression in switchgrass under cadmium stress. Plants Basel. 8, 504. doi: 10.3390/plants8110504
Bruno, L. B., Anbuganesan, V., Karthik, C., Tripti,, Kumar, A., Banu, J. R., et al. (2021). Enhanced phytoextraction of multi-metal contaminated soils under increased atmospheric temperature by bioaugmentation with plant growth promoting Bacillus cereus. J. Environ. Manag. 289:112553. doi: 10.1016/j.jenvman.2021.112553
Bruno, L. B., Karthik, C., Ma, Y., Kadirvelu, K., Freitas, H., and Rajkumar, M. (2020). Amelioration of chromium and heat stresses in Sorghum bicolor by Cr6+ reducing-thermotolerant plant growth promoting bacteria. Chemosphere 244:125521. doi: 10.1016/j.chemosphere.2019.125521
Burges, A., Epelde, L., Blanco, F., Becerril, J. M., and Garbisu, C. (2017). Ecosystem services and plant physiological status during endophyte-assisted phytoremediation of metal contaminated soil. Sci. Sci. Total. Environ. 584-585, 329–338. doi: 10.1016/j.scitotenv.2016.12.146
Carvalhais, L. C., Dennis, P. G., Fan, B., Fedoseyenko, D., Kierul, K., Becker, A., et al. (2013). Linking plant nutritional status to plant-microbe interactions. PLoS One 8:e68555. doi: 10.1371/journal.pone.0068555
Chen, M. J., Li, Y. F., Jiang, X. R., Zhao, D. R., Liu, X. F., Zhou, J. L., et al. (2021). Study on soil physical structure after the bioremediation of Pb pollution using microbial-induced carbonate precipitation methodology. J. Hazard. Mater. 411:125103. doi: 10.1016/j.jhazmat.2021.125103
Chen, B., Luo, S., Wu, Y. J., Ye, J. Y., Wang, Q., Xu, X. M., et al. (2017). The effects of the endophytic bacterium Pseudomonas fluorescens Sasm 05 and IAA on the plant growth and cadmium uptake of Sedum alfredii hance. Front. Microbiol. 8:2538. doi: 10.3389/fmicb.2017.02538
Cheng, C., Wang, R., Sun, L. J., He, L. Y., and Sheng, X. F. (2021). Cadmium-resistant and arginine decarboxylase-producing endophytic Sphingomonas sp. C40 decreases cadmium accumulation in host rice (Oryza sativa Cliangyou 513). Chemosphere 275:130109. doi: 10.1016/j.chemosphere.2021.130109
Chowdhury, R., Nallusamy, S., Shanmugam, V., Loganathan, A., Muthurajan, R., Sivathapandian, S. K., et al. (2021). Genome-wide understanding of evolutionary and functional relationships of rice yellow stripe-Like (YSL) transporter family in comparison with other plant species. Biologia 77, 39–53. doi: 10.1007/s11756-021-00924-5
Compant, S., Duffy, B., Nowak, J., Clément, C., and Barka, E. A. (2005). Use of plant growth-promoting bacteria for biocontrol of plant diseases: principles, mechanisms of action, and future prospects. Appl. Environ. Microbiol. 71, 4951–4959. doi: 10.1128/AEM.71.9.4951-4959.2005
de Souza, C. B., and Vicente, E. J. (2020). Expression of synthetic phytochelatin EC20 in E. Coli increases its biosorption capacity and cadmium resistance. Biosci. J. 36, 619–627. doi: 10.14393/BJ-v36n2a2020-42463
Dhaliwal, S. S., Singh, J., Taneja, P. K., and Mandal, A. (2020). Remediation techniques for removal of heavy metals from the soil contaminated through different sources: a review. Environ. Sci. Pollut. Res. 27, 1319–1333. doi: 10.1007/s11356-019-06967-1
Enebe, M. C., and Babalola, O. O. (2018). The influence of plant growth-promoting rhizobacteria in plant tolerance to abiotic stress: a survival strategy. Appl. Microbiol. Biotechnol. 102, 7821–7835. doi: 10.1007/s00253-018-9214-z
Etesami, H. (2018). Bacterial mediated alleviation of heavy metal stress and decreased accumulation of metals in plant tissues: mechanisms and future prospects. Ecotoxicol. Environ. Saf. 147, 175–191. doi: 10.1016/j.ecoenv.2017.08.032
Etesami, H., and Glick, B. R. (2020). Halotolerant plant growth–promoting bacteria: prospects for alleviating salinity stress in plants. Environ. Exp. Bot. 178:104124. doi: 10.1016/j.envexpbot.2020.104124
Etesami, H., and Maheshwari, D. K. (2018). Use of plant growth promoting rhizobacteria (PGPRs) with multiple plant growth promoting traits in stress agriculture: action mechanisms and future prospects. Ecotoxicol. Environ. Saf. 156, 225–246. doi: 10.1016/j.ecoenv.2018.03.013
Ezezika, O. A., and Singer, P. A. (2010). Genetically engineered oil-eating microbes for bioremediation: prospects and regulatory challenges. Technol. Soc. 32, 331–335. doi: 10.1016/j.techsoc.2010.10.010
Gadd, G. M. (2004). Microbial influence on metal mobility and application for bioremediation. Geoderma 122, 109–119. doi: 10.1016/j.geoderma.2004.01.002
Ghosh, P. K., Maiti, T. K., Pramanik, K., Ghosh, S. K., Mitra, S., and De, T. K. (2018). The role of arsenic resistant Bacillus aryabhattai MCC3374 in promotion of rice seedlings growth and alleviation of arsenic phytotoxicity. Chemosphere 211, 407–419. doi: 10.1016/j.chemosphere.2018.09.109
Giovanella, P., Cabral, L., Bento, F. M., Gianello, C., and Camargo, F. A. O. (2016). Mercury (II) removal by resistant bacterial isolates and mercuric (II) reductase activity in a new strain of Pseudomonas sp B50A. New Biotechnol. 33, 216–223. doi: 10.1016/j.nbt.2015.05.006
Glick, B. R. (2012). Plant growth-promoting bacteria: mechanisms and applications. Scientifica 2012:963401. doi: 10.6064/2012/963401
Gong, Y. Y., Zhao, D. Y., and Wang, Q. L. (2018). An overview of field-scale studies on remediation of soil contaminated with heavy metals and metalloids: technical progress over the last decade. Water Res. 147, 440–460. doi: 10.1016/j.watres.2018.10.024
Grover, M., Bodhankar, S., Sharma, A., Sharma, P., Singh, J., and Nain, L. (2021). PGPR mediated alterations in root traits: way toward sustainable crop production. Front. Sustain. Food Syst. 4:618230. doi: 10.3389/fsufs.2020.618230
Guo, J.K., and Muhammad, H., Lv, X., Wei, T., Ren, X.H., and Jia, H.L., , Atif, S., and Hua, L. (2020). Prospects and applications of plant growth promoting rhizobacteria to mitigate soil metal contamination: a review. Chemosphere 246: 125823. doi: 10.1016/j.chemosphere.2020.125823
Hashem, A., Tabassum, B., and Abd Allah, E. F. (2019). Bacillus subtilis: a plant-growth promoting rhizobacterium that also impacts biotic stress. Saudi J. Biol. Sci. 26, 1291–1297. doi: 10.1016/j.sjbs.2019.05.004
He, X. M., Xu, M. J., Wei, Q. P., Tang, M. Y., Guan, L. K., Lou, L. Q., et al. (2020). Promotion of growth and phytoextraction of cadmium and lead in Solanum nigrum L. mediated by plant-growth-promoting rhizobacteria. Ecotoxicol. Environ. Saf. 205:111333. doi: 10.1016/j.ecoenv.2020.111333
Hewage, K. A. H., Yang, J. F., Wang, D., Hao, G. F., Yang, G. F., and Zhu, J. K. (2020). Chemical manipulation of abscisic acid signaling: a new approach to abiotic and biotic stress management in agriculture. Adv. Sci. 7:2001265. doi: 10.1002/advs.202001265
Huang, H. J., Jia, Q. Y., Jing, W. X., Dahms, H. U., and Wang, L. (2020a). Screening strains for microbial biosorption technology of cadmium. Chemosphere 251:126428. doi: 10.1016/j.chemosphere.2020.126428
Huang, H. M., Zhao, Y. L., Fan, L., Jin, Q., Yang, G. Y., and Xu, Z. G. (2020b). Improvement of manganese phytoremediation by Broussonetia papyrifera with two plant growth promoting (PGP) Bacillus species. Chemosphere 260:127614. doi: 10.1016/j.chemosphere.2020.127614
Hussain, I., Aleti, G., Naidu, R., Puschenreiter, M., Mahmood, Q., Rahman, M. M., et al. (2018). Microbe and plant assisted-remediation of organic xenobiotics and its enhancement by genetically modified organisms and recombinant technology: a review. Sci. Total Environ. 628-629, 1582–1599. doi: 10.1016/j.scitotenv.2018.02.037
Jacob, J. M., Karthik, C., Saratale, R. G., Kumar, S. S., Prabakar, D., Kadirvelu, K., et al. (2018). Biological approaches to tackle heavy metal pollution: a survey of literature. J. Environ. Manag. 217, 56–70. doi: 10.1016/j.jenvman.2018.03.077
Jain, D., Kour, R., Bhojiya, A. A., Meena, R. H., Singh, A., Mohanty, S. R., et al. (2020). Zinc tolerant plant growth promoting bacteria alleviates phytotoxic effects of zinc on maize through zinc immobilization. Sci. Rep. 10, 13865–13164. doi: 10.1038/s41598-020-70846-w
Jeyasundar, P. G. S. A., Ali, A., Azeem, M., Li, Y. M., Guo, D., Sikdar, A., et al. (2021). Green remediation of toxic metals contaminated mining soil using bacterial consortium and Brassica juncea. Environ. Pollut. 277:116789. doi: 10.1016/j.envpol.2021.116789
Ke, T., Guo, G. Y., Liu, J. R., Zhang, C., Tao, Y., Wang, P. P., et al. (2021). Improvement of the cu and cd phytostabilization efficiency of perennial ryegrass through the inoculation of three metal-resistant PGPR strains. Environ. Pollut. 271:116314. doi: 10.1016/j.envpol.2020.116314
Kim, K. H., Kabir, E., and Jahan, S. A. (2016). A review on the distribution of hg in the environment and its human health impacts. J. Hazard. Mater. 306, 376–385. doi: 10.1016/j.jhazmat.2015.11.031
Kumari, S., and Das, S. (2019). Expression of metallothionein encoding gene bmt A in biofilm-forming marine bacterium Pseudomonas aeruginosa N6P6 and understanding its involvement in Pb (II) resistance and bioremediation. Environ. Sci. Pollut. Res. 26, 28763–28774. doi: 10.1007/s11356-019-05916-2
Lal, S., Ratna, S., Ben Said, O., and Kumar, R. (2018). Biosurfactant and exopolysaccharide-assisted rhizobacterial technique for the remediation of heavy metal contaminated soil: an advancement in metal phytoremediation technology. Environ. Technol. Innov. 10, 243–263. doi: 10.1016/j.eti.2018.02.011
Li, X. F., Ren, Z. M., Crabbe, M. J. C., Wang, L., and Ma, W. L. (2021). Genetic modifications of metallothionein enhance the tolerance and bioaccumulation of heavy metals in Escherichia coli. Ecotoxicol. Environ. Saf. 222:112512. doi: 10.1016/j.ecoenv.2021.112512
Li, C. F., Zhou, K. H., Qin, W. Q., Tian, C. J., Qi, M., Yan, X. M., et al. (2019). A review on heavy metals contamination in soil: effects, sources, and remediation techniques. Soil Sediment Contam. 28, 380–394. doi: 10.1080/15320383.2019.1592108
Li, D. D., Zhou, D. M., Wang, P., and Li, L. Z. (2011). Temperature affects cadmium-induced phytotoxicity involved in subcellular cadmium distribution and oxidative stress in wheat roots. Ecotoxicol. Environ. Saf. 74, 2029–2035. doi: 10.1016/j.ecoenv.2011.06.004
Liu, Y., Gao, J., Bai, Z. H., Wu, S. H., Li, X. L., Wang, N., et al. (2021). Unraveling mechanisms and impact of microbial recruitment on oilseed rape (Brassica napus L.) and the rhizosphere mediated by plant growth-promoting rhizobacteria. Microorganisms 9:161. doi: 10.3390/microorganisms9010161
Liu, L. W., Li, W., Song, W. P., and Guo, M. X. (2018). Remediation techniques for heavy metal-contaminated soils: principles and applicability. Sci. Total Environ. 633, 206–219. doi: 10.1016/j.scitotenv.2018.03.161
Liu, S. M., Yang, B., Liang, Y. S., Xiao, Y. H., and Fang, J. (2020). Prospect of phytoremediation combined with other approaches for remediation of heavy metal-polluted soils. Environ. Sci. Pollut. Res. 27, 16069–16085. doi: 10.1007/s11356-020-08282-6
Ma, Y., Oliveira, R. S., Freitas, H., and Zhang, C. (2016a). Biochemical and molecular mechanisms of plant-microbe-metal interactions: relevance for phytoremediation. Front. Plant Sci. 7:918. doi: 10.3389/fpls.2016.00918
Ma, Y., Rajkumar, M., Moreno, A., Zhang, C., and Freitas, H. (2017). Serpentine endophytic bacterium Pseudomonas azotoformans ASS1 accelerates phytoremediation of soil metals under drought stress. Chemosphere 185, 75–85. doi: 10.1016/j.chemosphere.2017.06.135
Ma, Y., Rajkumar, M., Zhang, C., and Freitas, H. (2016b). Beneficial role of bacterial endophytes in heavy metal phytoremediation. J. Environ. Manag. 174, 14–25. doi: 10.1016/j.jenvman.2016.02.047
Ma, H., Wei, M. Y., Wang, Z. R., Hou, S. Y., Li, X. D., and Xu, H. (2020). Bioremediation of cadmium polluted soil using a novel cadmium immobilizing plant growth promotion strain Bacillus sp. TZ5 loaded on biochar. J. Hazard. Mater. 388:122065. doi: 10.1016/j.jhazmat.2020.122065
Mahdi, I., Fahsi, N., Hafidi, M., Benjelloun, S., Allaoui, A., and Biskri, L. R. (2021). Phosphate solubilizing Bacillus atrophaeus GQJK17 S8 increases quinoa seedling, withstands heavy metals, and mitigates salt stress. Sustain. For. 13:3307. doi: 10.3390/su13063307
Mallick, I., Bhattacharyya, C., Mukherji, S., Dey, D., Sarkar, S. C., Mukhopadhyay, U. K., et al. (2018). Effective rhizoinoculation and biofilm formation by arsenic immobilizing halophilic plant growth promoting bacteria (PGPB) isolated from mangrove rhizosphere: a step towards arsenic rhizoremediation. Sci. Total Environ. 610-611, 1239–1250. doi: 10.1016/j.scitotenv.2017.07.234
Manoj, S. R., Karthik, C., Kadirvelu, K., Arulselvi, P. I., Shanmugasundaram, T., Bruno, B., et al. (2020). Understanding the molecular mechanisms for the enhanced phytoremediation of heavy metals through plant growth promoting rhizobacteria: a review. J. Environ. Manag. 254:109779. doi: 10.1016/j.jenvman.2019.109779
Mello, I. S., Targanski, S., Pietro-Souza, W., Stachack, F. F. F., Terezo, A. J., and Soares, M. A. N. (2020). Endophytic bacteria stimulate mercury phytoremediation by modulating its bioaccumulation and volatilization. Ecotoxicol. Environ. Saf. 202:110818. doi: 10.1016/j.ecoenv.2020.110818
Mendoza-Labrador, J., Romero-Perdomo, F., Abril, J., Hernandez, J. P., Uribe-Velez, D., and Buitrago, R. B. (2021). Bacillus strains immobilized in alginate macrobeads enhance drought stress adaptation of Guinea grass. Rhizosphere 19:100385. doi: 10.1016/j.rhisph.2021.100385
Mierek-Adamska, A., Tylman-Mojzeszek, W., Znajewska, Z., and Dabrowska, G. B. (2017). Bacterial metallothioneins. Postep. Mifrobiol. 56, 171–179. doi: 10.21307/PM-2017.56.2.171
Morales-Cedeño, L. R., Orozco-Mosqueda, M. D., Loeza-Lara, P. D., Parra-Cota, F. I., de los Santos-Villalobos, S., and Santoyo, G. (2021). Plant growth-promoting bacterial endophytes as biocontrol agents of pre-and post-harvest diseases: fundamentals, methods of application and future perspectives. Microbiol. Res. 242:126612. doi: 10.1016/j.micres.2020.126612
Mousavi, S. M., Motesharezadeh, B., Hosseini, H. M., Alikhani, H., and Zolfaghari, A. A. (2018). Root-induced changes of Zn and Pb dynamics in the rhizosphere of sunflower with different plant growth promoting treatments in a heavily contaminated soil. Ecotoxicol. Environ. Saf. 147, 206–216. doi: 10.1016/j.ecoenv.2017.08.045
Nanda, M., Kumar, V., and Sharma, D. K. (2019). Multimetal tolerance mechanisms in bacteria: the resistance strategies acquired by bacteria that can be exploited to 'clean-up' heavy metal contaminants from water. Aquat. Toxicol. 212, 1–10. doi: 10.1016/j.aquatox.2019.04.011
Nejad, Z. D., Jung, M. C., and Kim, K. H. (2018). Remediation of soils contaminated with heavy metals with an emphasis on immobilization technology. Environ. Geochem. Health 40, 927–953. doi: 10.1007/s10653-017-9964-z
Ngalimat, M. S., Yahaya, R. S. R., Baharudin, M. M. A. A., Yaminudin, S. M., Karim, M., Ahmad, S. A., et al. (2021). A review on the biotechnological applications of the operational group Bacillus amyloliquefaciens. Microorganisms 9:613. doi: 10.3390/microorganisms9030614
O’Callaghan, M., Ballard, R. A., and Wright, D. (2020). Soil microbial inoculants for sustainable agriculture: limitations and opportunities. Soil Use Manag. 38, 1340–1369. doi: 10.1111/sum.12811
Ozyigit, I. I., Can, H., and Dogan, I. (2020). Phytoremediation using genetically engineered plants to remove metals: a review. Environ. Chem. Lett. 19, 669–698. doi: 10.1007/s10311-020-01095-6
Pal, M., Janda, T., and Szalai, G. (2018). Interactions between plant hormones and thiol-related heavy metal chelators. Plant Growth Regul. 85, 173–185. doi: 10.1007/s10725-018-0391-7
Pan, W., Lu, Q., Xu, Q. R., Zhang, R. R., Li, H. Y., Yang, Y. H., et al. (2019). Abscisic acid-generating bacteria can reduce cd concentration in pakchoi grown in Cd-contaminated soil. Ecotoxicol. Environ. Saf. 177, 100–107. doi: 10.1016/j.ecoenv.2019.04.010
Pascual, I., Antolín, M. C., García, C., Polo, A., and Sánchez-Díaz, M. (2004). Plant availability of heavy metals in a soil amended with a high dose of sewage sludge under drought conditions. Biol. Fertil. Soils 40, 291–299. doi: 10.1007/s00374-004-0763-1
Peng, W. H., Li, X. M., Song, J. X., Jiang, W., Liu, Y. Y., and Fan, W. H. (2018). Bioremediation of cadmium-and zinc-contaminated soil using Rhodobacter sphaeroides. Chemosphere 197, 33–41. doi: 10.1016/j.chemosphere.2018.01.017
Plociniczak, T., Sinkkonen, A., Romantschuk, M., Sułowicz, S., and Piotrowska-Seget, Z. (2016). Rhizospheric bacterial strain Brevibacterium casei MH8a colonizes plant tissues and enhances cd, Zn, cu phytoextraction by white mustard. Front. Plant Sci. 7:101. doi: 10.3389/fpls.2016.00101
Pramanik, K., Mandal, S., Banerjee, S., Ghosh, A., Maiti, T. K., and Mandal, N. C. (2021). Unraveling the heavy metal resistance and biocontrol potential of Pseudomonas sp. K32 strain facilitating rice seedling growth under Cd stress. Chemosphere 274:129819. doi: 10.1016/j.chemosphere.2021.129819
Prapagdee, B., and Khonsue, N. (2015). Bacterial-assisted cadmium phytoremediation by Ocimum gratissimum L. in polluted agricultural soil: a field trial experiment. J. Environ. Sci. Technol. 12, 3843–3852. doi: 10.1007/s13762-015-0816-z
Pratush, A., Kumar, A., and Hu, Z. (2018). Adverse effect of heavy metals (As, Pb, hg, and Cr) on health and their bioremediation strategies: a review. Int. Microbiol. 21, 97–106. doi: 10.1007/s10123-018-0012-3
Priyadarshanee, M., and Das, S. (2021). Biosorption and removal of toxic heavy metals by metal tolerating bacteria for bioremediation of metal contamination: a comprehensive review. J. Environ. Chem. Eng. 9:104686. doi: 10.1016/j.jece.2020.104686
Rajendran, S., Priya, T. A. K., Khoo, K. S., Hoang, T. K. A., Ng, H. S., Munawaroh, H. S. H., et al. (2021). A critical review on various remediation approaches for heavy metal contaminants removal from contaminated soils. Chemosphere 287:132369. doi: 10.1016/j.chemosphere.2021.132369
Rajkumar, M., Prasad, M. N. V., Swaminathan, S., and Freitas, H. (2013). Climate change driven plant–metal–microbe interactions. Environ. Int. 53, 74–86. doi: 10.1016/j.envint.2012.12.009
Raklami, A., Oufdou, K., Tahiri, A. I., Mateos-Naranjo, E., Navarro-Torre, S., Rodriguez-Llorente, I. D., et al. (2019). Safe cultivation of Medicago sativa in metal-polluted soils from semi-arid regions assisted by heat-and metallo-resistant PGPR. Microorganisms 7:212. doi: 10.3390/microorganisms7070212
Rebello, S., Nathan, V. K., Sindhu, R., Binod, P., Awasthi, M. K., and Pandey, A. (2021). Bioengineered microbes for soil health restoration: present status and future. Bioengineered 12, 12839–12853. doi: 10.1080/21655979.2021.2004645
Ren, X. M., Guo, S. J., Tian, W., Chen, Y., Han, H., Chen, E., et al. (2019). Effects of plant growth-promoting bacteria (PGPB) inoculation on the growth, antioxidant activity, cu uptake, and bacterial community structure of rape (Brassica napus L.) grown in cu-contaminated agricultural soil. Front. Microbiol. 10:1455. doi: 10.3389/fmicb.2019.01455
Rizvi, A., and Khan, M. S. (2017). Biotoxic impact of heavy metals on growth, oxidative stress and morphological changes in root structure of wheat (Triticum aestivum L.) and stress alleviation by Pseudomonas aeruginosa strain CPSB1. Chemosphere 185, 942–952. doi: 10.1016/j.chemosphere.2017.07.088
Saeed, Q., Wang, X. K., Haider, F. U., Kucerik, J., Mumtaz, M. Z., Holatko, J., et al. (2021). Rhizosphere bacteria in plant growth promotion, biocontrol, and bioremediation of contaminated sites: a comprehensive review of effects and mechanisms. Int. J. Mol. Sci. 22, 10529. doi: 10.3390/ijms221910529
San Martín, Y. B., León, H. F. T., Rodríguez, A. A., Marqués, A. M., and López, M. I. S. (2021). Rhamnolipids application for the removal of vanadium from contaminated sediment. Curr. Microbiol. 78, 1949–1960. doi: 10.1007/s00284-021-02445-5
Sánchez-Marañón, M., Miralles, I., Aguirre-Garrido, J. F., Anguita-Maeso, M., Millán, V., Ortega, R., et al. (2017). Changes in the soil bacterial community along a pedogenic gradient. Sci. Rep. 7:14593. doi: 10.1038/s41598-017-15133-x
Saravanan, A., Kumar, P. S., Ramesh, B., and Srinivasan, S. (2022). Removal of toxic heavy metals using genetically engineered microbes: molecular tools, risk assessment and management strategies. Chemosphere 298:134341. doi: 10.1016/j.chemosphere.2022.134341
Sarwar, N., Imran, M., Shaheen, M. R., Ishaque, W., Kamran, M. A., Matloob, A., et al. (2017). Phytoremediation strategies for soils contaminated with heavy metals: modifications and future perspectives. Chemosphere 171, 710–721. doi: 10.1016/j.chemosphere.2016.12.116
Sedlakova-Kadukova, J. Kopcakova, A., Gresakova, L., Pristas, P., and Pristas, P. (2019). Bioaccumulation and biosorption of zinc by a novel Streptomyces K11 strain isolated from highly alkaline aluminium brown mud disposal site. Ecotoxicol. Environ. Saf. 167, 204–211. doi: 10.1016/j.ecoenv.2018.09.123
Sharma, P. (2021). Efficiency of bacteria and bacterial assisted phytoremediation of heavy metals: an update. Bioresour. Technol. 328:124835. doi: 10.1016/j.biortech.2021.124835
Silambarasan, S., Logeswari, P., Cornejo, P., Abrahamc, J., and Valentine, A. (2019a). Simultaneous mitigation of aluminum, salinity and drought stress in Lactuca sativa growth via formulated plant growth promoting Rhodotorula mucilaginosa CAM4. Ecotoxicol. Environ. Saf. 180, 63–72. doi: 10.1016/j.ecoenv.2019.05.006
Silambarasan, S., Logeswari, P., Cornejo, P., and Kannan, V. R. (2019b). Evaluation of the production of exopolysaccharide by plant growth promoting yeast Rhodotorula sp. strain CAH2 under abiotic stress conditions. Int. J. Biol. Macromol. 121, 55–62. doi: 10.1016/j.ijbiomac.2018.10.016
Silambarasan, S., Logeswari, P., Valentine, A., and Cornejo, P. (2019c). Role of Curtobacterium herbarum strain CAH5 on aluminum bioaccumulation and enhancement of Lactuca sativa growth under aluminum and drought stresses. Ecotoxicol. Environ. Saf. 183:109573. doi: 10.1016/j.ecoenv.2019.109573
Silambarasan, S., Logeswari, P., Valentine, A., Cornejo, P., and Kannan, V. R. (2020). Pseudomonas citronellolis strain SLP6 enhances the phytoremediation efficiency of Helianthus annuus in copper contaminated soils under salinity stress. Plant Soil 457, 241–253. doi: 10.1007/s11104-020-04734-7
Singh, A., and Prasad, S. M. (2015). Remediation of heavy metal contaminated ecosystem: an overview on technology advancement. J. Environ. Sci. Technol. 12, 353–366. doi: 10.1007/s13762-014-0542-y
Sivaperumal, P., Kamala, K., and Rajaram, R. (2018). Adsorption of cesium ion by marine actinobacterium Nocardiopsis sp. 13H and their extracellular polymeric substances (EPS) role in bioremediation. Environ. Sci. Pollut. Res. 25, 4254–4267. doi: 10.1007/s11356-017-0818-0
Su, C., Sun, X. J., Mu, Y. Z., Li, P. W., Li, J., Fan, P. S., et al. (2021). Multilayer calcium alginate beads containing diatom biosilica and bacillus subtilis as microecologics for sewage treatment. Carbohydr. Polym. 256:117603. doi: 10.1016/j.carbpol.2020.117603
Suyal, D. C., Soni, R., Sai, S., and Goel, R. (2016). “Microbial inoculants as biofertilizer,” in Microbial Inoculants in Sustainable Agricultural Productivity. eds. D. Singh, H. Singh, and R. Prabha (New Delhi: Springer).
Szopa, D., Mielczarek, M., Skrzypczak, D., Izydorczyk, G., Mikula, K., Chojnacka, K., et al. (2022). Encapsulation efficiency and survival of plant growth-promoting microorganisms in an alginate-based matrix-A systematic review and protocol for a practical approach. Ind. Crop. Prod. 181:114846. doi: 10.1016/j.indcrop.2022.114846
Tamayo-Figueroa, D. P., Castillo, E., and Brandão, P. F. B. (2019). Metal and metalloid immobilization by microbiologically induced carbonates precipitation. World J. Microbiol. Biotechnol. 35:58. doi: 10.1007/s11274-019-2626-9
Tanwir, K., Javed, M. T., Abbas, S., Shahid, M., Akram, M. S., Chaudhary, H. J., et al. (2021). Serratia sp. CP-13 alleviates Cd toxicity by morpho-physio-biochemical improvements, antioxidative potential and diminished cd uptake in Zea mays L. cultivars differing in Cd tolerance. Ecotoxicol. Environ. Saf. 208:111584. doi: 10.1016/j.ecoenv.2020.111584
Tu, C., Wei, J., Guan, F., Liu, Y., Sun, Y. H., and Luo, Y. M. (2020). Biochar and bacteria inoculated biochar enhanced Cd and Cu immobilization and enzymatic activity in a polluted soil. Environ. Pollut. 137:105576. doi: 10.1016/j.envint.2020.105576
Vardhan, K. H., Kumar, P. S., and Panda, R. C. (2019). A review on heavy metal pollution, toxicity and remedial measures: current trends and future perspectives. J. Mol. Liq. 290:111197. doi: 10.1016/j.molliq.2019.111197
Vejan, P., Abdullah, R., Khadiran, T., Ismail, S., and Boyce, A. N. (2016). Role of plant growth promoting rhizobacteria in agricultural sustainability—a review. Molecules 21:573. doi: 10.3390/molecules21050573
Venegas-Rioseco, J., Ginocchio, R., and Ortiz-Calderon, C. (2022). Increase in phytoextraction potential by genome editing and transformation: a review. Plants Basel 11, 86. doi: 10.3390/plants11010086
Verma, S., and Kuila, A. (2019). Bioremediation of heavy metals by microbial process. Environ. Technol. Innov. 14:100369. doi: 10.1016/j.eti.2019.100369
Vishnupradeep, R., Bruno, L. B., Taj, Z., Rajkumar, M., Challabathula, D., Karthik, C., et al. (2022). Plant growth promoting bacteria improve growth and phytostabilization potential of Zea mays under chromium and drought stress by altering photosynthetic and antioxidant responses. Environ. Technol. Innov. 25:102154. doi: 10.1016/j.eti.2021.102154
Voica, D. M., Bartha, L., Banciu, H. L., and Oren, A. (2016). Heavy metal resistance in halophilic Bacteria and Archaea. FEMS Microbiol. Lett. 363:fnw146. doi: 10.1093/femsle/fnw146
Wang, X., Hu, K., Xu, Q., Lu, L. F., Liao, S. J., and Wang, G. J. (2020). Immobilization of cd using mixed Enterobacter and Comamonas bacterial reagents in pot experiments with Brassica rapa L. Environ. Sci. Technol. 54, 15731–15741. doi: 10.1021/acs.est.0c03114
Wang, Q., Zhang, W. J., He, L. Y., and Sheng, X. F. (2018). Increased biomass and quality and reduced heavy metal accumulation of edible tissues of vegetables in the presence of Cd-tolerant and immobilizing Bacillus megaterium H3. Ecotoxicol. Environ. Saf. 148, 269–274. doi: 10.1016/j.ecoenv.2017.10.036
Wu, C., Li, F., Yi, S. W., and Ge, F. (2021). Genetically engineered microbial remediation of soils co-contaminated by heavy metals and polycyclic aromatic hydrocarbons: advances and ecological risk assessment. J. Environ. Manag. 296:113185. doi: 10.1016/j.jenvman.2021.113185
Wu, K. R., Luo, J. P., Li, J. X., An, Q. L., Yang, X. E., Liang, Y. C., et al. (2018). Endophytic bacterium Buttiauxella sp SaSR13 improves plant growth and cadmium accumulation of hyperaccumulator Sedum alfredii. Environ. Sci. Pollut. Res. 25, 21844–21854. doi: 10.1007/s11356-018-2322-6
Wu, Y. J., Ma, L. Y., Liu, Q. Z., Vestergård, M., Topalovic, O., Wang, Q., et al. (2020b). The plant-growth promoting bacteria promote cadmium uptake by inducing a hormonal crosstalk and lateral root formation in a hyperaccumulator plant Sedum alfredii. J. Hazard. Mater. 395:122661. doi: 10.1016/j.jhazmat.2020.122661
Wu, T. Y., Wu, X. Q., Xu, X. Q., Kong, W. L., and Wu, F. (2020a). Salt tolerance mechanism and species identification of the plant rhizosphere bacterium JYZ-SD2. Curr. Microbiol. 77, 388–395. doi: 10.1007/s00284-019-01835-0
Xu, L., Naylor, D., Dong, Z. B., Simmons, T., Pierroz, G., Hixson, K. K., et al. (2018). Drought delays development of the sorghum root microbiome and enriches for monoderm bacteria. Proc. Nat. Acad. Sci. U.S.A. 115, E4284–E4293. doi: 10.1073/pnas.1717308115
Yaashikaa, P. R., Kumar, P. S., Saravanan, A., and Vo, D. V. N. (2021). Advances in biosorbents for removal of environmental pollutants: a review on pretreatment, removal mechanism and future outlook. J. Hazard. Mater. 420:126596. doi: 10.1016/j.jhazmat.2021.126596
Yang, Z., Yang, F., Liu, J. L., Wu, H. T., Yang, H., Shi, Y., et al. (2022). Heavy metal transporters: functional mechanisms, regulation, and application in phytoremediation. Sci. Total Environ. 809:151099. doi: 10.1016/j.scitotenv.2021.151099
Yin, K., Wang, Q. N., Lv, M., and Chen, L. X. (2019). Microorganism remediation strategies towards heavy metals. Chem. Eng. J. 360, 1553–1563. doi: 10.1016/j.cej.2018.10.226
You, L. X., Zhang, R. R., Dai, J. X., Lin, Z. T., Li, Y. P., Herzberg, M., et al. (2021). Potential of cadmium resistant Burkholderia contaminans strain ZCC in promoting growth of soy beans in the presence of cadmium. Ecotoxicol. Environ. Saf. 211:111914. doi: 10.1016/j.ecoenv.2021.111914
Zerrouk, I. Z., Benchabane, M., Khelifi, L., Yokawa, K., Ludwig-Müller, J., and Balusk, F. (2016). A Pseudomonas strain isolated from date-palm rhizospheres improves root growth and promotes root formation in maize exposed to salt and aluminum stress. J. Plant Physiol. 191, 111–119. doi: 10.1016/j.jplph.2015.12.009
Zerrouk, I. Z., Rahmoune, B., Khelifi, L., Mounir, K., Baluska, F., and Ludwig-Muller, J. (2019). Algerian Sahara PGPR confers maize root tolerance to salt and aluminum toxicity via ACC deaminase and IAA. Acta Physiol. Plant. 41, 9. doi: 10.1007/s11738-019-2881-2
Zhang, J., Cao, T. T., Tang, Z., Shen, Q. R., Rosen, B. P., and Zhao, F. J. (2015). Arsenic methylation and volatilization by arsenite S-Adenosylmethionine methyltransferase in Pseudomonas alcaligenes NBRC14159. Appl. Environ. Microbiol. 81, 2852–2860. doi: 10.1128/AEM.03804-14
Keywords: plant growth-promoting bacteria, metal bioavailability, metal detoxification, climatic stresses, bioremediation
Citation: Wang Y, Narayanan M, Shi X, Chen X, Li Z, Natarajan D and Ma Y (2022) Plant growth-promoting bacteria in metal-contaminated soil: Current perspectives on remediation mechanisms. Front. Microbiol. 13:966226. doi: 10.3389/fmicb.2022.966226
Received: 10 June 2022; Accepted: 13 July 2022;
Published: 11 August 2022.
Edited by:
Deniz Yildirim, Çukurova University, TurkeyReviewed by:
Dilek Alagöz, Çukurova University, TurkeyCopyright © 2022 Wang, Narayanan, Shi, Chen, Li, Natarajan and Ma. This is an open-access article distributed under the terms of the Creative Commons Attribution License (CC BY). The use, distribution or reproduction in other forums is permitted, provided the original author(s) and the copyright owner(s) are credited and that the original publication in this journal is cited, in accordance with accepted academic practice. No use, distribution or reproduction is permitted which does not comply with these terms.
*Correspondence: Ying Ma, Y2F0aHltYXlpbmdAaG90bWFpbC5jb20=
Disclaimer: All claims expressed in this article are solely those of the authors and do not necessarily represent those of their affiliated organizations, or those of the publisher, the editors and the reviewers. Any product that may be evaluated in this article or claim that may be made by its manufacturer is not guaranteed or endorsed by the publisher.
Research integrity at Frontiers
Learn more about the work of our research integrity team to safeguard the quality of each article we publish.