- 1Department of Biochemistry, Emory University School of Medicine, Atlanta, GA, United States
- 2Graduate Program in Biochemistry, Cell and Developmental Biology (BCDB), Graduate Division of Biological and Biomedical Sciences, Emory University, Atlanta, GA, United States
- 3Emory Antibiotic Resistance Center (ARC), Emory University, Atlanta, GA, United States
The tuberactinomycins are a family of cyclic peptide ribosome-targeting antibiotics with a long history of use as essential second-line treatments for drug-resistant tuberculosis. Beginning with the identification of viomycin in the early 1950s, this mini-review briefly describes tuberactinomycin structures and biosynthesis, as well as their past and present application in the treatment of tuberculosis caused by infection with Mycobacterium tuberculosis. More recent studies are also discussed that have revealed details of tuberactinomycin action on the ribosome as well as resistance mechanisms that have emerged since their introduction into the clinic. Finally, future applications of these drugs are considered in the context of their recent removal from the World Health Organization’s List of Essential Medicines.
Introduction
Antibiotics have been a critical component of modern medicine since their discovery early in the 20th century, offering effective treatments for otherwise potentially fatal bacterial infections (Fleming, 2001; Gelpi et al., 2015). These “miracle drugs” have also been pivotal to advances in modern medicine by enabling surgeries, organ transplants, chemotherapy, and other procedures requiring immunosuppression. The bacterial ribosome has provided fertile ground in nature and in the research laboratory for antibiotic development and is the target of many antibiotics in clinical use today (Figure 1A). However, resistance to these essential medicines among diverse human bacterial pathogens has now developed against almost all classes of antibiotics in clinical use (Wilson, 2014; Lin et al., 2018). Without action to counter the increasing prevalence of resistance, we face the alternative future of a “post-antibiotic world” where common infections and other diseases once again have a much greater mortality rate (World Health Organization, 2014).
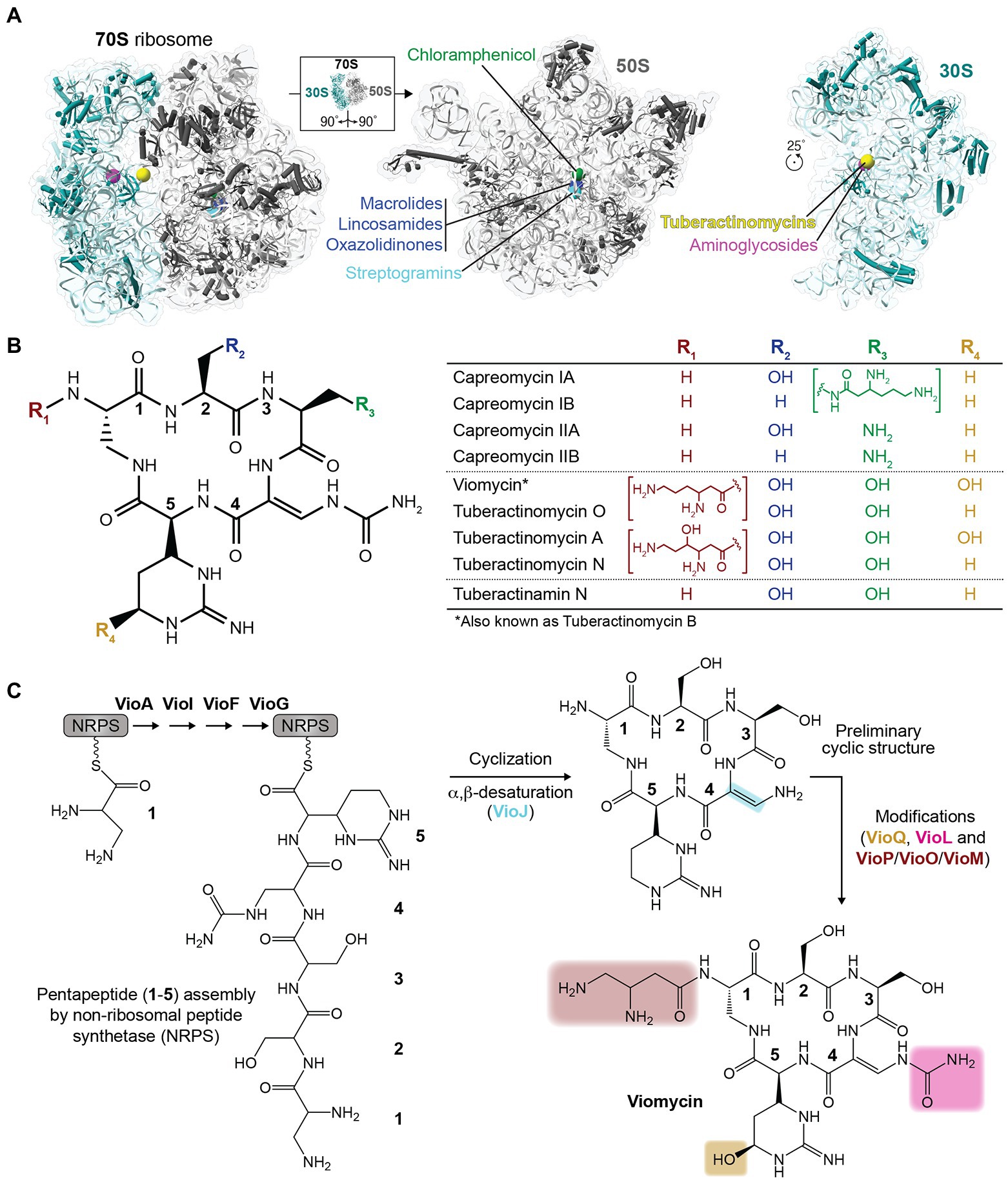
Figure 1. Ribosome-targeting antibiotics and chemical structures of the tuberactinomycins. (A) Sites of action of select clinically relevant ribosome-targeting antibiotics are shown on the structure of the intact Escherichia coli 70S ribosome (left) and individual subunits (right) rotated as indicated to show their subunit interfaces (PDB code 6LKQ). The indicated antibiotics target the 50S (large) subunit at the peptidyl transferase center and nascent peptide exit tunnel (chloramphenicol, lincosamides, oxazolidinones, streptogramins, and macrolides), or the 30S (small) subunit decoding center and nearby inter-subunit interface (aminoglycosides and tuberactinomycins). (B) Chemical structures of the tuberactinomycin antibiotics shown as the common pentapeptide core scaffold (left) and unique substituents at four variable positions (R1 to R4; as indicated in the table on the right). (C) Overview of the process of tuberactinomycin biosynthesis (shown for viomycin), including non-ribosomal peptide synthetase (NRPS) assembly of the pentapeptide and subsequent modification steps.
The tuberactinomycins are one example of an important class of ribosome-targeting antibiotics with a long history of clinical use (Anonymous, 1973). Although recently replaced by alternative oral therapies on the World Health Organization (WHO) recommended treatments for active tuberculosis (TB), the tuberactinomycins viomycin and capreomycin remain potent anti-mycobacterial agents. Use of these drugs as second-line treatments was partly designed as a strategy to limit the development of resistance (Bartz et al., 1951; Sutton et al., 1966), but Mycobacterium tuberculosis resistance to tuberactinomycins has nonetheless emerged and threatens the efficacy of these antibiotics.
Beginning with their initial discovery and structural characterization in the early 1950s, here, we describe current knowledge on tuberactinomycin biosynthesis, mechanisms of action on the ribosome, and clinically relevant resistance mechanisms that have emerged. Finally, viewed through this lens, we speculate on the future potential use (s) of these and similar antibiotics.
Chemical structure and biosynthesis of the tuberactinomycin antibiotics
Like many antibiotics in use today, tuberactinomycins are natural products of soil-dwelling bacteria. Viomycin was first isolated from Streptomyces puniceus in 1951, and was quickly demonstrated to have potent antibiotic activity against M. tuberculosis (Bartz et al., 1951; Finlay et al., 1951). Subsequent discoveries completed the currently recognized tuberactinomycin antibiotic family: tuberactinomycins A, B, and N (also known as enviomycin), and O from Streptomyces griseoverticillatus var. tuberacticus in 1968; capreomycin from Streptomyces capreolus (now Saccharothrix mutabilis subspecies capreolus) in 1960; and tuberactinamine N, isolated in 1975 (Herr et al., 1960, 1961; Herr and Redstone, 1966; Nagata et al., 1968; Wakamiya et al., 1970; Ando et al., 1971; Yoshioka et al., 1971; Wakamiya and Shiba, 1975). Subsequent work to isolate the individual tuberactinomycins A, B, N, and O revealed that tuberactinomycin B and viomycin were the same compound (Noda et al., 1972). Capreomycin was also initially identified as a mixture of four components (capreomycins IA, IB, IIA, and IIB), which were subsequently isolated and the differences in their core ring substituents characterized (Herr et al., 1960, 1961; Herr and Redstone, 1966; Figure 1B). Notably, however, capreomycin went into clinical use 2 years later (in 1973) as a mixture of all four components (Anonymous, 1973).
Structurally, the tuberactinomycin antibiotics are defined by a conserved cyclic pentapeptide core ring derived from non-ribosomally synthesized pentapeptides, which are cyclized and modified in a series of subsequent reactions to produce the final active compound (Figure 1C). The ~36.3 kb viomycin biosynthesis gene cluster in Streptomyces vinaceus contains 20 open reading frames (ORFs) encoding all components necessary for the biosynthesis, regulation, export, and activation of viomycin (Thomas et al., 2003; Barkei et al., 2009). First, the cyclic pentapeptide core (common to all tuberactinomycins) is assembled by VioA, VioI, VioF, and VioG using at least one L-serine and at least one of the non-proteinogenic amino acids 2,3-diaminopropionate, L-capreomycidine, and β-ureidodehydroalanine. VioJ then desaturates part of the ring and additional modifications are added by VioL (carbamoylation), VioM/VioO (N-acylation), and VioQ (hydroxylation). Other genes within the biosynthetic cluster are not directly associated with the building of viomycin, but perform other essential steps such as the synthesis of specialized amino acid building blocks (VioP). The cluster also includes a viomycin resistance gene (vph, encoding viomycin phosphotransferase) to protect the producing bacterium from self-intoxication.
The capreomycin biosynthetic gene cluster in S. mutabilis subspecies capreolus has also been characterized and comprises 33 ORFs (Felnagle et al., 2007). Of these 33 ORFs, only 19 are proposed to be involved with the production of capreomycin, while the function of the other 14 ORFs remains unknown. These ORFs have no sequence similarity to genes that suggest an obvious role in capreomycin biosynthesis and sequence analyses of the viomycin and capreomycin gene clusters show that homology exists only between the already-identified ORFs (Felnagle et al., 2007). The capreomycin biosynthesis gene cluster encodes three different resistance enzymes: cph (the direct homolog of viomycin phosphotransferase vph), cac (a putative capreomycin acetyltransferase), and cmnU (proposed to encode a 16S rRNA m1A1408 aminoglycoside-resistance methyltransferase; Skinner and Cundliffe, 1980; Thiara and Cundliffe, 1995). cac is required in addition to cph, as the latter enzyme modifies a hydroxyl group present in capreomycin IA and IIA but absent in IB and IIB; cac is proposed to modify an amino group common to all four capreomycin molecules. Why the capreomycin biosynthesis cluster also encodes the rRNA modification enzyme CmnU is less clear, though it is possible that this additional target-based resistance mechanism alleviates residual toxicity in the modified capreomycin product of cph or cac or, alternatively, against a currently unknown secondary metabolite that is produced in the process of capreomycin but not viomycin biosynthesis.
70S ribosome binding and tuberactinomycin mechanism of action
Tuberactinomycins inhibit the process of bacterial translation, i.e., protein synthesis by the ribosome. Specifically, the bactericidal effect of this class of antibiotics is derived from their capacity to block the process of translocation, or movement of the mRNA-tRNA pairs following peptide bond formation to position the next three-nucleotide codon within the aminoacyl tRNA binding site (A site) for decoding (Modolfll and Vazquez, 1977). During this step, the deacylated tRNA is moved from the peptidyl tRNA site (P site) to the exit site (E site), while the A-site tRNA, now carrying the nascent polypeptide chain, is moved to the P site. Normally, the movement of the tRNAs is driven by elongation factor G (EF-G) and hydrolysis of GTP. Viomycin binds the ribosome in the pre-translocation state, stabilizing 16S nucleotides A1492 and A1493 in their active conformation and preventing the backward movement of the 30S body and head domain (Holm et al., 2019; Belardinelli et al., 2021). Viomycin has been shown to prevent translocation for a minimum of ~45 s (longer with higher viomycin concentration), thereby inhibiting overall translation and hindering essential cell processes (Holm et al., 2016). While a detailed discussion is beyond the scope of this focused mini-review, it is also noteworthy that the tuberactinomycins have overlapping binding sites (see Figure 1), mechanisms of action, and susceptibility to bacterial resistance mechanisms with other ribosome-targeting antibiotics (Maus et al., 2005; Wilson, 2014; Lin et al., 2018; Holm et al., 2019).
Optimal tuberactinomycin binding to the 70S ribosome, and thus the anti-mycobacterial activity of these antibiotics, is dependent on intrinsic ribose 2’-OH methylation incorporated by a single Class I S-adenosyl-L-methionine (SAM)-dependent housekeeping methyltransferase TlyA, encoded by the tlyA gene. Two subfamilies of TlyA are known, TlyAI which methylates 23S rRNA nucleotide C1920 (E. coli numbering; C2158 in M. tuberculosis) and dual specificity TlyAII which methylates C1920 and 16S rRNA nucleotide C1409 (C1402 in M. tuberculosis; Johansen et al., 2006; Monshupanee et al., 2012). TlyAII is conserved in mycobacteria, while TlyAI is possessed by other diverse bacterial species, including Thermus thermophilus which is used extensively in ribosome structural studies, but not in E. coli. As a result, E. coli is intrinsically less susceptible to tuberactinomycins (Johansen et al., 2006; Monshupanee et al., 2012), and M. tuberculosis clinical resistance can arise through loss of TlyA activity, as discussed further below.
Structures of viomycin and capreomycin bound to 70S ribosomes from several bacterial species have been determined via X-ray crystallography or cryogenic electron microscopy (cryo-EM) and these antibiotics share a common binding site located primarily in the ribosome 30S subunit decoding center and contacting the adjacent 30S/50S subunit interface between 16S rRNA helix 44 (h44) and 23S rRNA Helix 69 (H69; Stanley et al., 2010; Yang et al., 2017; Figure 2A). This binding site is largely conserved when either capreomycin or viomycin are bound to ribosomes from different bacterial species. Additionally, both drugs bind to the ribosome in a very similar manner whether the 70S complex is in the classical or rotated state (i.e., the relative orientation of the subunits to each other at different points in a cycle of translation; Stanley et al., 2010; Yang et al., 2017; Zhang et al., 2020). Structural analyses of the E. coli 70S-viomycin complex identified four additional sites: one exclusively on the 30S subunit near ribosomal protein S12 (Figure 2B; Vio#2) when the ribosome is in either a classical or rotated state, and a triple cluster at a distinct inter-subunit site which appears unique to the rotated state (Figure 2B; Vio#3 to #5; Zhang et al., 2020).
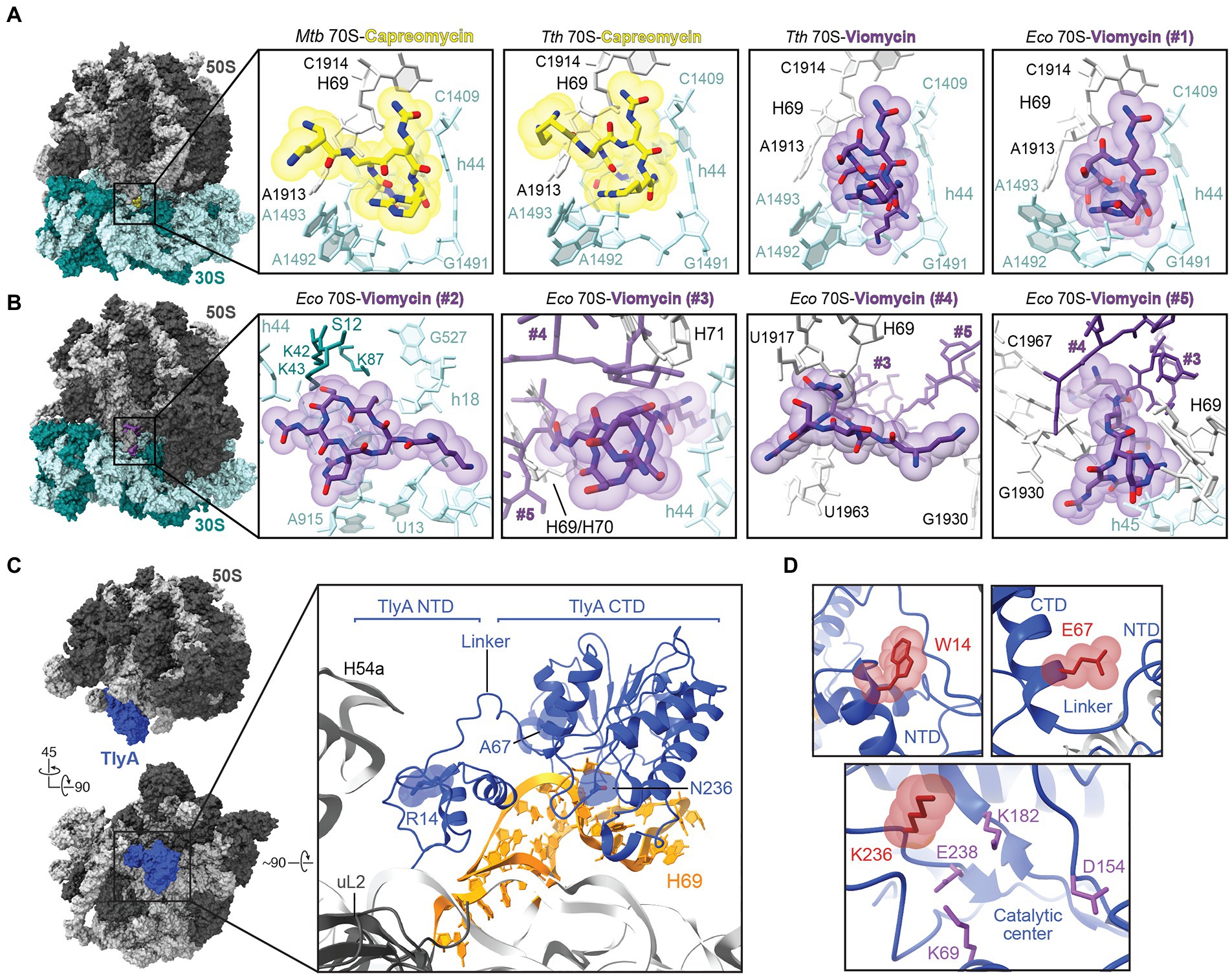
Figure 2. Molecular basis of tuberactinomycin action and resistance. (A) Overview of the Mycobacterium tuberculosis (Mtb) 70S structure (PDB code 5 V93) with the capreomycin (yellow) binding site indicated within the boxed region. Zoomed-in views are also shown of the primary tuberactinomycin antibiotic binding site at the 30S and 50S interface for (left to right): capreomycin bound to the M. tuberculosis 70S (PDB code 5 V93) and T. thermophilus (Tth) 70S (PDB code 4V7M), and for viomycin (purple) bound to T. thermophilus 70S (PDB code 4V7L) and E. coli (Eco) 70S (PDB code 6LKQ). For ease of comparison, nucleotides are labeled with E. coli numbering where shown. (B) Overview of the E. coli 70S-viomycin complex and zoomed-in views of the four additional viomycin binding sites, #2 to #5, identified in this structure (PDB code 6LKQ). Note that viomycin molecules at sites #3-#5 make interactions with each other and are shown in each view but with only the focused viomycin shown with atom coloring. (C) Two views of the structure of the mycobacterial 50S subunit-TlyA complex (left). In the top view, the 50S subunit is shown in the same orientation as in the 70S ribosome views of panels A and B. Also highlighted in the zoomed-in view are TlyA (blue), its rRNA binding site on H69, and three amino acid substitutions leading to loss of TlyA activity from missense tlyA mutations in M tuberculosis clinical isolates. (D) Zoomed-in views of the sites of amino acid substitution shown with the residue arising from clinical resistance mutation (red).
At the common site on the 30S shared by capreomycin and viomycin, antibiotic binding affects the positioning of 16S rRNA nucleotides A1492 and A1493, and 23S rRNA nucleotide A1913 (E. coli numbering), which surround the A-site tRNA in the ribosomal decoding center. Flipping of A1492 and A1493 from h44 is established as a mechanism by which the ribosome interrogates the mRNA-tRNA pairing by contacting the minor groove of the codon-anticodon pairing (Ogle et al., 2001). Together with repositioning of A1913 to hydrogen bond with the A-site tRNA, the interactions of these nucleotides likely result in the observed increased tRNA affinity for the A site, hindering of subunit movement during translocation, and promotion of back translocation in the presence of tuberactinomycins (Peske et al., 2004; Szaflarski et al., 2008; Holm et al., 2016, 2019; Belardinelli et al., 2021). Consistent with this site of action for the tuberactinomycins, selection of capreomycin resistance in T. thermophilus identified mutations or deletions at A1913 and the adjacent U1915 at the tip of H69 (Monshupanee et al., 2008). Further, these rRNA changes did not affect C1920 modification by TlyA suggesting a direct effect on the drug binding site structure.
While binding at the common site (Figure 2A; Vio#1) can explain much of the observed action of viomycin and is supported by the above noted mutational analyses, identification of additional binding sites for this drug (though, to date, not for capreomycin; Figure 2B; Vio#2–5) open the possibility of additional contributions to its mechanism of action. As noted by Zhang et al. in their report on the 70S-viomycin complex, identification of essentially equivalent viomycin locations in the A site in both the classical and rotated state calls into question the role of drug binding at this site in hindering translocation (Zhang et al., 2020). Alternatively, the triple cluster of viomycin molecules at the subunit interface may be the origin of inhibition of subunit dissociation and inter-subunit movement, as it is observed only in the rotated state (Figure 2B). This possibility is supported by earlier single molecule studies suggesting multiple binding sites (Feldman et al., 2010), and the proximity of one of the three viomycins (Vio#3) to the C1920 ribose which is modified by TlyA. However, the high concentration of viomycin (0.5 mM)—which may be necessary to form the unusual triple cluster of drug molecules—and the absence of TlyA-encoded modifications at C1409 and C1920 in the E. coli ribosomes used for these studies, leave some uncertainty over the contribution of these binding sites to viomycin action. On the other hand, the previously identified common binding site (Figures 2A,B; Vio#1) cannot readily explain the specific dependence of viomycin and capreomycin activity on C1920 methylation distant from this site. The influence of ribose methylation on C1920 sugar pucker and H69 helical structure influencing the architecture of the common binding at the tip of H69 site has recently been proposed (Laughlin et al., 2022), but further studies are needed to tease apart these important molecular details of tuberactinomycin action on the ribosome.
Clinical use of tuberactinomycins and mechanisms of resistance
According to the WHO, TB was the second-leading cause of death from a single infectious agent worldwide in 2020 (behind COVID-19), resulting in approximately 1.5 million deaths (World Health Organization, 2021). Further, of an estimated 9.9 million individuals who developed TB in 2020, 7.5% of those tested for drug resistance indicated infection with either a multi (MDR)-, pre-extensively, or extensively drug-resistant strain of M. tuberculosis. Tuberactinomycins, and capreomycin in particular, have a long history in the treatment of these drug-resistant cases of TB (World Health Organization, 2016), with its inclusion on the WHO Model List of Essential Medicines reflecting this global importance (World Health Organization, 2017). However, these drugs suffer side effects, including ototoxicity and nephrotoxicity, and were thus not recommended for use in children or adult patients with mild forms of TB (World Health Organization, 2016). Additionally, as noted earlier, with the wider availability of all-oral treatments, the WHO revised guidelines around the treatment of MDR-TB in 2018, and currently recommends against the use of capreomycin (which is an injectable agent; World Health Organization, 2018). However, with their retained anti-mycobacterial efficacy, the tuberactinomycins may still play an important role in the treatment of TB or other bacterial infectious diseases, particularly if limitations due to side effects can be overcome with new generations of drugs. As such, defining the basis of resistance mechanisms which have already emerged through clinical use of these drugs will also be an important future consideration.
Tuberactinomycins bind at a similar location on the ribosome to the aminoglycosides kanamycin and amikacin, which have also been used as second-line drugs for the treatment of TB, and cross-resistance between these drug classes in M. tuberculosis has been observed (Maus et al., 2005; Jugheli et al., 2009; Akbergenov et al., 2011). Clinical isolates of tuberactinomycin-resistant M. tuberculosis typically arise through mutation of the genes encoding either rRNA or TlyA. Sites of resistance mutations in 16S rRNA cluster around the drug binding site at the subunit interface (Figure 2A) and include the TlyA target nucleotide in h44 (C1409), its base pairing partner (G1491), and several other nearby residues in the ribosome decoding center (Maus et al., 2005; Akbergenov et al., 2011; Phelan et al., 2019; Olawoye et al., 2021). Deletion of 23S rRNA nucleotide A1916 in the loop of H69 has also been observed in a capreomycin-resistance clinical M. tuberculosis isolate (Johansen et al., 2006).
Mutations in tlyA leading to clinical resistance include both nonsense (premature stop codon) and missense mutations that result in amino acid substitutions that eliminate TlyA enzymatic activity (Johansen et al., 2006; Monshupanee et al., 2012; Li et al., 2019; Phelan et al., 2019). The TlyA structure comprises an amino-terminal domain (NTD), which adopts a ribosomal protein S4-like fold, and a Class I methyltransferase carboxyl-terminal domain (CTD), connected by a short linker which is important for cosubstrate SAM binding within the CTD (Witek et al., 2017). Recent structural studies have elucidated both the full-length structure of TlyA and the molecular basis for recognition and modification of one of its two substrates, C1920 on the 50S subunit (Figure 2C; Laughlin et al., 2022). TlyA interaction with the 50S subunit exclusively exploits contacts with 23S rRNA at H69 and the adjacent rRNA junction, via a contiguous surface spanning both protein domains which is enriched in basic residues. The NTD appears critical for correct substrate recognition as substitution of either of two conserved arginine residues (Arg6 and Arg20), distant from the enzyme active site, eliminates C1920 ribose methylation. These residues act in concert to recognize the unique 23S rRNA structure at the base of H69. Similarly, critical residues were also identified in the CTD, including Phe157 which appears to stabilize a “flipped” conformation of C1920 for optimal orientation of the ribose 2’-OH for modification. Mutations in tlyA that inactivate TlyA and lead to clinical capreomycin resistance have been identified in both protein domains and can be rationalized based on these recent structural insights (Figure 2D). For example, a R14W substitution (Phelan et al., 2019) likely results in capreomycin resistance by disruption of the NTD structure and thus critical contacts to 23S rRNA made by the nearby Arg6 and Arg20. Another common mutation found in resistant M. tuberculosis results in a lysine substitution of Gln236 in the TlyA CTD (Walker et al., 2018); this residue immediately follows a short loop that envelops the flipped C1920 base and additionally results in placement of a basic side chain close to residue Glu238 which has been proposed to play an important role in catalysis (Figure 2D; Arenas et al., 2011). Finally, a third clinical capreomycin-resistance mutation was identified which results in an A67E substitution at a site distant from both the catalytic center and NTD region critical for rRNA (Phelan et al., 2019; Laughlin et al., 2022). How this change impacts TlyA function is less clear, but the larger charged residue would likely disrupt a hydrophobic pocket occupied by Trp62 of the TlyA inter-domain linker (Figure 2D), which was previously shown to be important for SAM binding and which could also influence correct NTD/CTD association or inter-domain communication during substrate recognition (Witek et al., 2017; Laughlin et al., 2022).
While these recent studies have elucidated much about TlyA’s mechanism of action and the basis for its inactivation by mutations in drug-resistant M. tuberculosis, several open questions remain. In the case of dual specificity enzymes (TlyAII) like that of M. tuberculosis, how the enzyme adapts to recognize and modify its target nucleotide in the 30S is currently not known in detail. However, a significantly overlapping molecular surface of the TlyA NTD and CTD is again critical, but with distinct dependencies on functionally critical residues compared to 50S subunit modification (Laughlin et al., 2022). In terms of clinical resistance, a broader question is why M. tuberculosis and many other bacteria maintain TlyA given its contribution to tuberactinomycin activity on the ribosome. Removal of endogenous TlyA activity appears to incur little cost to fitness, but may result in a reduction of functional 70S ribosomes (Salamaszynska-Guz et al., 2014; Freihofer et al., 2016). Identification of a critical function for TlyA—perhaps unrelated to rRNA modification—or conditions under which it is revealed will require further careful investigation.
Conclusion and future perspectives
Tuberactinomycins are a valuable class of ribosome-targeting antibiotics that have played an important role in treating drug-resistant TB. Despite current recommendations against their use, retained tuberactinomycin efficacy leaves open the possibility for future applications, especially if new derivatives can be obtained which counter the emerging resistance mechanisms and overcome toxicity issues. Previous studies have demonstrated that the addition of various compounds to cultures of bacteria expressing the biosynthetic gene cluster can alter the products of antibiotic production resulting in new compounds based on the tuberactinomycin core (Morse et al., 1997). Additionally, engineering of the biosynthetic cluster or alteration of supplied building blocks could provide starting points for further evolution of this drug family using semi-synthetic chemical approaches. In addition to providing new alternatives to treat TB, an expanded tuberactinomycin family would likely find application in the fight against other antibiotic-resistant bacterial infections. For example, viomycin has been shown to be effective vancomycin-resistant enterococci and MRSA (Dirlam et al., 1997; Linde et al., 1997). Additionally, capreomycin has been shown in cell culture to have anti-viral activity against SARS-CoV2 despite its previous use as strictly an antibiotic (Kumar et al., 2021). Combined with efforts to reduce unwanted side effects, future efforts to expand the tuberactinomycin drug class may offer a fruitful avenue to much-needed treatments for infectious diseases.
Author contributions
ZL conducted a review of relevant literature and prepared a first draft of the manuscript. ZL and GC wrote sections of the final manuscript. Both authors contributed to the article and approved the submitted version.
Funding
Our research on TlyA is supported by NIH/NIAID awards T32-AI106699 (to ZL) and R01-AI088025 (to GC).
Acknowledgments
We thank Christine M. Dunham and William M. Shafer for comments on the manuscript draft.
Conflict of interest
The authors declare that the research was conducted in the absence of any commercial or financial relationships that could be construed as a potential conflict of interest.
Publisher’s note
All claims expressed in this article are solely those of the authors and do not necessarily represent those of their affiliated organizations, or those of the publisher, the editors and the reviewers. Any product that may be evaluated in this article, or claim that may be made by its manufacturer, is not guaranteed or endorsed by the publisher.
References
Akbergenov, R., Shcherbakov, D., Matt, T., Duscha, S., Meyer, M., Wilson, D. N., et al. (2011). Molecular basis for the selectivity of antituberculosis compounds capreomycin and viomycin. Antimicrob. Agents Chemother. 55, 4712–4717. doi: 10.1128/AAC.00628-11
Ando, T., Matsuura, K., Izumi, R., Noda, T., Take, T., Nagata, A., et al. (1971). Studies on tuberactinomycin 2. Isolation and properties of tuberactinomycin-N, a new tuberactinomycin group antibiotic. J. Antibiot. 24:680. doi: 10.7164/antibiotics.24.680
Anonymous, (1973). Evaluation of a new antituberculous agent. Capreomycin sulfate (capastat sulfate). JAMA 223, 179–180. doi: 10.1001/jama.1973.03220020045016
Arenas, N. E., Salazar, L. M., Soto, C. Y., Vizcaino, C., Patarroyo, M. E., Patarroyo, M. A., et al. (2011). Molecular modeling and in silico characterization of Mycobacterium tuberculosis TlyA: possible misannotation of this tubercle bacilli-hemolysin. BMC Struct. Biol. 11, 16. doi: 10.1186/1472-6807-11-16
Barkei, J. J., Kevany, B. M., Felnagle, E. A., and Thomas, M. G. (2009). Investigations into viomycin biosynthesis by using heterologous production in Streptomyces lividans. Chembiochem 10, 366–376. doi: 10.1002/cbic.200800646
Bartz, Q. R., Ehrlich, J., Mold, J. D., Penner, M. A., and Smith, R. M. (1951). viomycin, a new tuberculostatic antibiotic. American Rev. Tuberculosis 63, 4–6. doi: 10.1164/art.1951.63.1.4
Belardinelli, R., Sharma, H., Peske, F., and Rodnina, M. V. (2021). Perturbation of ribosomal subunit dynamics by inhibitors of tRNA translocation. RNA 27, 981–990. doi: 10.1261/rna.078758.121
Dirlam, J. P., Belton, A. M., Birsner, N. C., Brooks, R. R., Chang, S. P., Chandrasekaran, R. Y., et al. (1997). Cyclic homopentapeptides 1. Analogs of tuberactinomycins and capreomycin with activity against vancomycin-resistant enterococci and Pasteurella. Bioorg. Med. Chem. Lett. 7, 1139–1144. doi: 10.1016/S0960-894X(97)00186-8
Feldman, M. B., Terry, D. S., Altman, R. B., and Blanchard, S. C. (2010). Aminoglycoside activity observed on single pre-translocation ribosome complexes. Nat. Chem. Biol. 6:244. doi: 10.1038/nchembio0310-244c
Felnagle, E. A., Rondon, M. R., Berti, A. D., Crosby, H. A., and Thomas, M. G. (2007). Identification of the biosynthetic gene cluster and an additional gene for resistance to the antituberculosis drug capreomycin. Appl. Environ. Microbiol. 73, 4162–4170. doi: 10.1128/AEM.00485-07
Finlay, A. C., Hobby, G. L., Hochstein, F., Lees, T. M., Lenert, T. F., Means, J. A., et al. (1951). Viomycin a new antibiotic active against mycobacteria. American Rev. Tuberculosis 63, 1–3. doi: 10.1164/art.1951.63.1.1
Fleming, A. (2001). On the antibacterial action of cultures of a penicillium, with special reference to their use in the isolation of B. influenzae. 1929. Bull. World Health Organ. 79, 780–790.
Freihofer, P., Akbergenov, R., Teo, Y., Juskeviciene, R., Andersson, D. I., and Bottger, E. C. (2016). Nonmutational compensation of the fitness cost of antibiotic resistance in mycobacteria by overexpression of tlyA rRNA methylase. RNA 22, 1836–1843. doi: 10.1261/rna.057257.116
Gelpi, A., Gilbertson, A., and Tucker, J. D. (2015). Magic bullet: Paul Ehrlich, Salvarsan and the birth of venereology. Sex. Transm. Infect. 91, 68–69. doi: 10.1136/sextrans-2014-051779
Herr, E. B. Jr., Haney, G. E., and Pittenger, G. E. (1961). “A New Peptide Antibiotic” in 140th Meeting of the American Chemical Society (Geneva: World Health Organization).
Herr, E. B. Jr., Haney, G. E., Pittenger, G. E., and Higgens, C. E. (1960). Isolation and characterization of a new peptide antibiotic. Proceed. Indiana Acad. Sci. 69:134.
Herr, E. B. Jr., and Redstone, M. O. (1966). Chemical and physical characterization of capreomycin. Ann. N. Y. Acad. Sci. 135, 940–946. doi: 10.1111/j.1749-6632.1966.tb45535.x
Holm, M., Borg, A., Ehrenberg, M., and Sanyal, S. (2016). Molecular mechanism of viomycin inhibition of peptide elongation in bacteria. Proc. Natl. Acad. Sci. U. S. A. 113, 978–983. doi: 10.1073/pnas.1517541113
Holm, M., Mandava, C. S., Ehrenberg, M., and Sanyal, S. (2019). The mechanism of error induction by the antibiotic viomycin provides insight into the fidelity mechanism of translation. elife 8:6124. doi: 10.7554/eLife.46124
Johansen, S. K., Maus, C. E., Plikaytis, B. B., and Douthwaite, S. (2006). Capreomycin binds across the ribosomal subunit interface using tlyA-encoded 2 '-O-methylations in 16S and 23S rRNAs. Mol. Cell 23, 173–182. doi: 10.1016/j.molcel.2006.05.044
Jugheli, L., Bzekalava, N., De Rijk, P., Fissette, K., Portaels, F., and Rigouts, L. (2009). High level of cross-resistance between kanamycin, amikacin, and capreomycin among Mycobacterium tuberculosis isolates from Georgia and a close relation with mutations in the rrs gene. Antimicrob. Agents Chemother. 53, 5064–5068. doi: 10.1128/AAC.00851-09
Kumar, S., Singh, B., Kumari, P., Kumar, P. V., Agnihotri, G., Khan, S., et al. (2021). Identification of multipotent drugs for COVID-19 therapeutics with the evaluation of their SARS-CoV2 inhibitory activity. Comput. Struct. Biotechnol. J. 19, 1998–2017. doi: 10.1016/j.csbj.2021.04.014
Laughlin, Z. T., Nandi, S., Dey, D., Zelinskaya, N., Witek, M. A., Srinivas, P., et al. (2022). 50S subunit recognition and modification by the Mycobacterium tuberculosis ribosomal RNA methyltransferase TlyA. Proc. Natl. Acad. Sci. U. S. A. 119:e2120352119. doi: 10.1073/pnas.2120352119
Li, Q., Gao, H., Zhang, Z., Tian, Y., Liu, T., Wang, Y., et al. (2019). Mutation and transmission profiles of second-line drug resistance in clinical isolates of drug-resistant Mycobacterium tuberculosis from Hebei province, China. Front. Microbiol. 10:1838. doi: 10.3389/fmicb.2019.01838
Lin, J., Zhou, D., Steitz, T. A., Polikanov, Y. S., and Gagnon, M. G. (2018). Ribosome-targeting antibiotics: modes of action, mechanisms of resistance, and implications for drug design. Annu. Rev. Biochem. 87, 451–478. doi: 10.1146/annurev-biochem-062917-011942
Linde, R. G., Birsner, N. C., Chandrasekaran, R. Y., Clancy, J., Howe, R. J., Lyssikatos, J. P., et al. (1997). Cyclic homopentapeptides 3. Synthetic modifications to the capreomycins and tuberactinomycins: Compounds with activity against methicillin-resistant Staphylococcus aureus and vancomycin-resistant Enterococci. Bioorg. Med. Chem. Lett. 7, 1149–1152. doi: 10.1016/S0960-894X(97)00188-1
Maus, C. E., Plikaytis, B. B., and Shinnick, T. M. (2005). Molecular analysis of cross-resistance to capreomycin, kanamycin, amikacin, and viomycin in Mycobacterium tuberculosis. Antimicrob. Agents Chemother. 49, 3192–3197. doi: 10.1128/AAC.49.8.3192-3197.2005
Modolfll, J., and Vazquez, D. (1977). The inhibition of ribosomal translocation by viomycin. Eur. J. Biochem. 81, 491–497. doi: 10.1111/j.1432-1033.1977.tb11974.x
Monshupanee, T., Gregory, S. T., Douthwaite, S., Chungjatupornchai, W., and Dahlberg, A. E. (2008). Mutations in conserved helix 69 of 23S rRNA of Thermus thermophilus that affect capreomycin resistance but not posttranscriptional modifications. J. Bacteriol. 190, 7754–7761. doi: 10.1128/JB.00984-08
Monshupanee, T., Johansen, S. K., Dahlberg, A. E., and Douthwaite, S. (2012). Capreomycin susceptibility is increased by TlyA-directed 2'-O-methylation on both ribosomal subunits. Mol. Microbiol. 85, 1194–1203. doi: 10.1111/j.1365-2958.2012.08168.x
Morse, B. K., Brown, M. S., Gagne, J. W., Mcarthur, H. A. L., Mccormick, E. L., Murphy, T. K., et al. (1997). Production of tuberactinamine A by Streptomyces griseoverticillatus var. tuberacticus NRRL 3482 fed with (S)-2-aminoethyl-L-cysteine. J. Antibiot. 50, 698–700. doi: 10.7164/antibiotics.50.698
Nagata, A., Ando, T., Izumi, R., Sakakibara, H., and Take, T. (1968). Studies on tuberactinomycin (tuberactin), a new antibiotic. I. Taxonomy of producing strain, isolation and characterization. J. Antibiot. (Tokyo) 21, 681–687. doi: 10.7164/antibiotics.21.681
Noda, T., Take, T., Nagata, A., Wakamiya, T., and Shiba, T. (1972). Chemical studies on tuberactinomycin. 3. The chemical structure of viomycin (tuberactinomycin B). J. Antibiot. (Tokyo) 25, 427–428. doi: 10.7164/antibiotics.25.427
Ogle, J. M., Brodersen, D. E., Clemons, W. M. Jr., Tarry, M. J., Carter, A. P., and Ramakrishnan, V. (2001). Recognition of cognate transfer RNA by the 30S ribosomal subunit. Science 292, 897–902. doi: 10.1126/science.1060612
Olawoye, I. B., Uwanibe, J. N., Kunle-Ope, C. N., Davies-Bolorunduro, O. F., Abiodun, T. A., Audu, R. A., et al. (2021). Whole genome sequencing of clinical samples reveals extensively drug resistant tuberculosis (XDR TB) strains from the Beijing lineage in Nigeria. West Africa. Sci Rep 11, 17387. doi: 10.1038/s41598-021-96956-7
Peske, F., Savelsbergh, A., Katunin, V. I., Rodnina, M. V., and Wintermeyer, W. (2004). Conformational changes of the small ribosomal subunit during elongation factor G-dependent tRNA-mRNA translocation. J. Mol. Biol. 343, 1183–1194. doi: 10.1016/j.jmb.2004.08.097
Phelan, J. E., O'sullivan, D. M., Machado, D., Ramos, J., Oppong, Y. E. A., Campino, S., et al. (2019). Integrating informatics tools and portable sequencing technology for rapid detection of resistance to anti-tuberculous drugs. Genome Med. 11:41. doi: 10.1186/s13073-019-0650-x
Salamaszynska-Guz, A., Taciak, B., Kwiatek, A., and Klimuszko, D. (2014). The Cj0588 protein is a Campylobacter jejuni RNA methyltransferase. Biochem. Biophys. Res. Commun. 448, 298–302. doi: 10.1016/j.bbrc.2014.04.104
Skinner, R. H., and Cundliffe, E. (1980). Resistance to the antibiotics viomycin and capreomycin in the Streptomyces species which produce them. J. Gen. Microbiol. 120, 95–104. doi: 10.1099/00221287-120-1-95
Stanley, R. E., Blaha, G., Grodzicki, R. L., Strickler, M. D., and Steitz, T. A. (2010). The structures of the anti-tuberculosis antibiotics viomycin and capreomycin bound to the 70S ribosome. Nat. Struct. Mol. Biol. 17, 289–293. doi: 10.1038/nsmb.1755
Sutton, W. B., Gordee, R. S., Wick, W. E., and Stanfield, L. (1966). In vitro and in vivo laboratory studies on the antituberculous activity of capreomycin. Ann. N. Y. Acad. Sci. 135, 947–959. doi: 10.1111/j.1749-6632.1966.tb45536.x
Szaflarski, W., Vesper, O., Teraoka, Y., Plitta, B., Wilson, D. N., and Nierhaus, K. H. (2008). New features of the ribosome and ribosomal inhibitors: non-enzymatic recycling, misreading and back-translocation. J. Mol. Biol. 380, 193–205. doi: 10.1016/j.jmb.2008.04.060
Thiara, A. S., and Cundliffe, E. (1995). Analysis of two capreomycin-resistance determinants from Streptomyces capreolus and characterization of the action of their products. Gene 167, 121–126. doi: 10.1016/0378-1119(95)00702-4
Thomas, M. G., Chan, Y. A., and Ozanick, S. G. (2003). Deciphering tuberactinomycin biosynthesis: isolation, sequencing, and annotation of the viomycin biosynthetic gene cluster. Antimicrob. Agents Chemother. 47, 2823–2830. doi: 10.1128/AAC.47.9.2823-2830.2003
Wakamiya, T., and Shiba, T. (1975). Chemical studies on tuberactinomycin.8. isolation of tuberactinamine-N, cyclic peptide moiety of tuberactinomycin-N and conversion of tuberactinomycin-N to O. J. Antibiot. 28, 292–297. doi: 10.7164/antibiotics.28.292
Wakamiya, T., Shiba, T., Kaneko, T., Sakakibara, H., Take, T., and Abe, J. N. (1970). Chemical studies on tuberactinomycin. 1. Structure of tuberactidine, guanidino amino acid component. Tetrahedron Lett. 11, 3497–3500. doi: 10.1016/S0040-4039(01)98511-9
Walker, T. M., Merker, M., Knoblauch, A. M., Helbling, P., Schoch, O. D., Van Der Werf, M. J., et al. (2018). A cluster of multidrug-resistant Mycobacterium tuberculosis among patients arriving in Europe from the Horn of Africa: A molecular epidemiological study. Lancet Infect. Dis. 18, 431–440. doi: 10.1016/S1473-3099(18)30004-5
Wilson, D. N. (2014). Ribosome-targeting antibiotics and mechanisms of bacterial resistance. Nat. Rev. Microbiol. 12, 35–48. doi: 10.1038/nrmicro3155
Witek, M. A., Kuiper, E. G., Minten, E., Crispell, E. K., and Conn, G. L. (2017). A Novel motif for S-adenosyl-l-methionine binding by the ribosomal RNA methyltransferase TlyA from Mycobacterium tuberculosis. J. Biol. Chem. 292, 1977–1987. doi: 10.1074/jbc.M116.752659
World Health Organization (2014). "Antimicrobial Resistance Global Report and Surveillance ". (Geneva: World Health Organization).
World Health Organization (2016). "WHO treatment guidelines for drug-resistant tuberculosis ". (Geneva: World Health Organization).
World Health Organization (2017). "WHO Model List of Essential Medicines ". 20th Edn. (Geneva: World Health Organization).
World Health Organization (2018). "Global Tuberculosis Report 2018 ". (Geneva: World Health Organization).
World Health Organization (2021). "Global Tuberculosis Report 2021 ". (Geneva: World Health Organization).
Yang, K., Chang, J. Y., Cui, Z., Li, X., Meng, R., Duan, L., et al. (2017). Structural insights into species-specific features of the ribosome from the human pathogen Mycobacterium tuberculosis. Nucleic Acids Res. 45, 10884–10894. doi: 10.1093/nar/gkx785
Yoshioka, H., Aoki, T., Goko, H., Nakatsu, K., Noda, T., Sakakiba, H., et al. (1971). Chemical studies on tuberactinomycin. 2. Structure of tuberactinomycin-O. Tetrahedron Lett. 12, 2043–2046. doi: 10.1016/S0040-4039(01)96775-9
Keywords: 70S ribosome, antibiotic resistance, mycobacteria, capreomycin, viomycin, methyltransferase, tuberculosis antibiotics, rRNA modification
Citation: Laughlin ZT and Conn GL (2022) Tuberactinomycin antibiotics: Biosynthesis, anti-mycobacterial action, and mechanisms of resistance. Front. Microbiol. 13:961921. doi: 10.3389/fmicb.2022.961921
Edited by:
Gregor Blaha, University of California, Riverside, United StatesReviewed by:
Sean R. Connell, Biocruces Bizkaia Health Research Institute, SpainYury S. Polikanov, University of Illinois at Chicago, United States
Copyright © 2022 Laughlin and Conn. This is an open-access article distributed under the terms of the Creative Commons Attribution License (CC BY). The use, distribution or reproduction in other forums is permitted, provided the original author(s) and the copyright owner(s) are credited and that the original publication in this journal is cited, in accordance with accepted academic practice. No use, distribution or reproduction is permitted which does not comply with these terms.
*Correspondence: Graeme L. Conn, Z2Nvbm5AZW1vcnkuZWR1