- 1Department of Biology, College of Science, Taif University, Taif, Saudi Arabia
- 2Department of Biotechnology, College of Science, Taif University, Taif, Saudi Arabia
- 3Department of Plant Protection, College of Agricultural Engineering Science, University of Baghdad, Baghdad, Iraq
- 4College of Plant Protection, Fujian Agriculture and Forestry University, Fuzhou, China
- 5Shenzhen Key Laboratory of Marine Bioresource and Eco-environmental Sciences, College of Life Sciences and Oceanography, Shenzhen University, Shenzhen, Guangdong, China
- 6Department of Chemistry, College of Science, Taif University, Taif, Saudi Arabia
- 7Department of Plant Protection, College of Horticulture and Forestry, Agriculture University, Kota, India
- 8Department of Environmental Energy and Engineering, Kyonggi University, Suwon, South Korea
- 9Department of Medical Biotechnology and Integrative Physiology, Institute of Biotechnology, Saveetha School of Engineering, Saveetha Institute of Medical and Technical Sciences, Thandalam, Chennai, Tamil Nadu, India
The current work is aimed at isolating and identifying new Entomopathogenic bacterium (EPB) strains associated with Steinernema feltiae and assessing the EPB’s biocontrol potential on Aphis punicae and Aphis illinoisensis adults in the laboratory. From S. feltiae, five bacterial isolates were isolated and molecularly characterized. Lysinibacillus xylanilyticus strain TU-2, Lysinibacillus xylanilyticus strain BN-13, Serratia liquefaciens strain TU-6, Stenotrophomonas tumulicola strain T5916-2-1b, and Pseudochrobactrum saccharolyticum strain CCUG are the strains. Pathogenicity tests demonstrated that bacterial cells were more toxic against the two aphid species than bacterial cell-free supernatants. S. tumulicola strain T5916-2-1b cells and filtrate were reported to have the strongest potential to kill A. punicae and A. illinoisensis individuals within 6 h after treatment, with 100% mortality of both insects 24 and 48 h after treatment. Based on the results of the study, it looked like endogenous Steinernema-associated EPB could be used directly as a biocontrol agent for A. punicae and A. illinoisensis.
Introduction
Since several years, entomopathogenic nematodes (EPNs) from the Steinernematidae and Heterorhabditidae families have been investigated as biocontrol agents. This is because they have a wide range of hosts and host-seeking behavior, as well as mass rearing and are easily applicable with low cost. They also exhibit long-term efficacy, they are compatible with most chemicals, they are safe for the environment (for non-target organisms such as humans), their use reduces the amount of pesticide residues in food, they increase the activity of other natural enemies and they increase biodiversity in managed ecosystems (Gaugler and Kaya, 1990).
Gram-negative bacteria from the Enterobacteriaceae family, Photorhabdus sp. and Xenorhabdus sp., live in symbiotic associations with EPNs from the Heterorhabditis and Steinernema genera, respectively (Boemare et al., 1993; Sajnaga and Kazimierczak, 2020). Infective juveniles (IJs) of steinernematid and heterorhabditid nematodes carry the symbiotic bacteria in their midguts, which inhabit in the soil of various ecological systems (Dillman et al., 2012). The nematodes aggressively seek for insect hosts, the symbiotic Xenorhabdus sp. and Photorhabdus sp. are released into the hemocoel after entering by the insect’s mouth, anus, or spiracles, respectively (Salvadori et al., 2012). The symbiotic bacteria play a wide range of biological roles, the most important of which is to keep the pathobiome conditions in the polyxenic colonized insect cadaver and soil appropriately balanced for the EPN/EPB symbiotic complex (Ogier et al., 2020). The symbiotic bacteria then invade the insect’s haemolymph, destroy tissues, and explore a variety of immunosuppressive factors, such as toxin complexes, hydrolytic enzymes, hemolysins, and antimicrobial substances that kill the insect host in less than 48 h (Fang et al., 2011; Shi et al., 2017). Finally, in the insect host, the symbiotic bacteria multiply fast, causing septicaemia a process for turning insect cadavers into a suitable food source for nematode development and reproduction. Multiple recent investigations, however, when entomopathogenic partners were injected into insects alone, the results put a question mark on this hypothesis, they were found to exhibit decreased virulence or to be nonvirulent (Bisch et al., 2015; Kim et al., 2017; McMullen et al., 2017). Similarly (Ogier et al., 2020), revealed that the association between Steinernema and Xenorhabdus was not monoxenic, and that several Proteobacteria were found in the bacterial population associated with laboratory-reared IJs from Steinernema carpocapsae, S. feltiae, S. glaseri, and S. weiseri. They also observed that a dozen Proteobacteria species (Pseudomonas, Stenotrophomonas, Alcaligenes, Achromobacter, Pseudochrobactrum, Ochrobactrum, Brevundimonas, Deftia, and others) were found to be linked with the main symbiont (Xenorhabdus nematophila). Non-symbiotic bacteria are hypothesized to join IJ vectors via the cuticle or intercuticular region, where they eventually enter the insect haemocoel during IJ penetration.(Singh et al., 2014). In soil-dwelling Caenorhabditis elegans nematodes, Pseudomonas, Ochrobactrum, and Stenotrophomonas have been frequently identified (Dirksen et al., 2016). Likely, Proteobacteria are also the most common bacterial group found in plant root bacterial populations.(Hartman et al., 2017) and in soils covered with plants, such as the rhizosphere (Kumar et al., 2022).
Many insect pests affect the quality and yield of pomegranate and grapevine cultivation. The pomegranate aphid, Aphis punicae Passerini (Hemiptera: Aphididae), is a major pest attacks pomegranate crop around the world. Fruits, leaves, and inflorescences are consumed by both adults and nymphs. Pomegranate aphid infestation results in pale, curled leaves, slowed development, and dropped flowers, as well as the transmission of viral infections and the secretion of honey dew, which fungi survive in lowering crop quality and yield (Moawad and Al-Barty, 2011). Aphis illinoisensis (Shimer) is a grapevine pest that feeds on the lower surface of new leaves, young terminal shoots (Blackman and Eastop, 2011), and fruit clusters, causing some grape berries to fall off (Pfeiffer and Schultz, 1986). Pomegranate and grapevine aphids have been documented as invasive pests in southern European, North African, and Asian countries since the early 2000s (El-Gantiry et al., 2012; Salim et al., 2022). Unfortunately, aphids have a high reproductive potential, and using insecticides extensively to control them leads to resistance development. When aphicides are used heavily on pomegranates or grapevines, the remnants are mainly concentrated in the fruits. Contamination with pesticides is undesirable because these fruits are consumed fresh (Li and Han, 2004; Pertot et al., 2017). As a result, scientists are looking for new pesticides that are more efficient against pests, less hazardous to natural enemies, and less destructive to the environment (Fouad et al., 2018; Ga’al et al., 2021).
Root weevils, white grubs, root worms, cutworms, sciarid flies, and armyworms are among the pests that have been controlled by EPNs (Hazir et al., 2004). When tested in field and laboratory conditions, EPNs and/or entomopathogenic bacteria (EPB) have been shown to satisfactorily control mosquitoes, pomegranate aphids, cabbageworms, scarab beetles and cherry fruit flies (Herz et al., 2006; Alghamdi et al., 2017; Yooyangket et al., 2018; Elbrense et al., 2021). The gene encoding the protease inhibitor protein has been recognized and expressed in the symbiotic bacterium Xenorhabdus bovienii strains BJFS526 and Xbpi-1. This protein’s impact on the pea aphid Acyrthosiphon pisum was also investigated (Zeng et al., 2012; Jin et al., 2014). Xenorhabdus szentirmaii is a one-of-a-kind source of antimicrobial peptides that are effective against virtually all known phytopathogens (Fuchs et al., 2014; Fodor et al., 2022).
To date, in several countries, including Saudi Arabia, both EPNs and their associations have not been sufficiently examined in terms of their diversity and application. Considering all the plant protection perspectives, as well as climatic, geographic and regulation aspects, the most reasonable approach is to search for potential biological plant protection (EPN/EPB) agents native locally.
Therefore, various bacterial strains that could be used as suitable control organisms need to be assessed to develop a new biological control technique. The genetic diversity of Saudi Arabian and Egyptian EPN genotypes was examined using RAPD and ISSR markers after an EPN species, Steinernema sp., was isolated from the soil of pomegranate trees in Taif, Saudi Arabia (Aljuboori et al., 2022). Geographically, Taif is an elevated location in Saudi Arabia, with valleys, steep mountains, and agricultural plateaus. There is an abundance of potential insect hosts; thus, the diversity of EPNs and EPB is expected to be very rich in this region. The goals of this research was to identify EPB associated with Steinernema found in Taif, Saudi Arabia, and to assess their activity against the pomegranate and grapevine aphids, A. punicae and A. illinoisensis, under laboratory conditions. On the basis of these goals we hypothesized that EPB associated with Steinernema would be good controlling agents for the control of A. punicae and A. illinoisensis.
Materials and methods
Insects
Fresh leaves and buds of pomegranate and grapevine trees infested with A. punicae and A. illinoisensis, respectively, were harvested on the same experimental day from pomegranate and grapevine farms in Taif, Saudi Arabia.
Isolation of Steinernema-associated bacteria
In this study, EPN S. feltiae strain NYH (MTöth et al., 2005) was originated from the Laboratory of Fodor Andras, Pannonia University, Keszthely, Hungary. According to the Akhurst (1980) method modified by (Vitta et al., 2018), bacterial symbionts of S. feltiae were isolated from infective dauer juveniles (IJs) or from the haemolymph of deceased Galleria mellonella larvae that was infected with S. feltiae IJs. Briefly, to isolate EPB from EPN infective juveniles, IJs were collected and centrifuged three times using sterilized tap water after being obtained from Galleria white traps. Some were placed in sterile petri plates with a drop of physiological saline (M9) solution before being moved to 5% chlorox. Individuals were moved to a series of sterilized M9 drops after a 2-min incubation period, and then fractured with a sterile platinum wire. The drop of M9 was diluted and deposited onto an NBTA indicator plate [nutrient agar + triphenyl tetrazolium chloride (0.004%) and bromothymol blue (0.025%)] and incubated at 28°C for 48 h. For isolation of EPB from G. mellonella cadavers, the dead G. mellonella larvae were washed in 100% ethanol for 1 min to be surface-sterilized before being placed in a sterile Petri dish to dry. Following that, a sterile sharp needle was used to penetrate the third segment of the G. mellonella larvae’s head to allow an influx of the haemolymph containing symbiotic bacteria. The haemolymph samples were distributed and streaked over NBTA media using a sterile loop, as previously described. Bacteria were frequently cultured every 24 h until pure isolated colonies were acquired, and then stored at −80°C with 20% glycerol (v/v) for further study. To generate the cell-free conditioned filtrates or cell suspensions, in 5 ml of Luria-Bertani (LB) broth, one colony of every isolate of relevant bacteria was seeded and cultured overnight at 28°C shaking at 220 rpm. Furthermore, 100-ml culture aliquots were shaken at room temperature overnight before being introduced to flasks with 400 ml of the identical media and agitated at 200 rpm for 5 days. To obtain a cell-free filtrate, the supernatant was filtered by a 0.22 μm Millipore filter, then the pellet was resuspended in sterile distilled water. Following that the filtrate was kept at 4°C for subsequent dilution with sterile distilled water to get concentrations of 600, 400, 200, and 100 μl/ml. The bacterial cell suspension was adjusted at OD600 to 1.0 using a spectrophotometer. A 10-fold serial dilution spread plate was used, with a bacterial suspension concentration of 1 × 108 (CFU/ml). Each bacterial cell solution was diluted to obtain concentrations 108, 106, 104, and 102 CFU/ml.
Identification of Steinernema-associated bacteria
The genomic DNA of the isolated bacteria was extracted from the bacterial pellets using the Bacterial Genomic DNA Miniprep Kit (QIAprep Spin Miniprep Kit). The bacterial genomic DNA was stored at −20°C prior to use in a PCR. To identify bacterial species, PCR-based analysis and 16S rRNA gene sequencing (1,504 base pairs, bp) were completed using the inter-universal primers 785F (GGATTAGATACCCTGGTA) and 907R (CCGTCAATTCMTTTRAGTTT; Tailliez et al., 2006).
Phylogenetic tree analysis
To identify the bacterial species associated with S. feltiae, a comparison of the partially edited nucleotide sequences (16S rRNA) was performed using the BLASTN program from the NCBI. The 16S rRNA sequences were compared to the NCBI database “16S rRNA sequence (Bacteria and Archae).” The alignments of all 16S sequences were done operating the program of MUSCLE with 50 iterations and were presented in the CLC viewer. The evolutionary history was inferred using the most probability method supported the Tamura-Nei model (Tamura and Nei, 1993). The tree with the highest log likelihood (−1426.34) is shown. The proportion of trees within which the associated taxa clustered together is shown next to the branches. Initial tree(s) for the heuristic search were obtained automatically by applying Neighbor-Joining and BioNJ algorithms to a matrix of pairwise distances estimated using the most composite likelihood (MCL) approach and so selecting the topology with superior log likelihood value. Evolutionary analyses were performed employing MEGA7 (Kumar et al., 2018) with 1,000 bootstrap values.
Bioassay
The five Steinernema-associated bacterial isolates (cells or filtrates) were selected for use in the toxicity bioassay: Stenotrophomonas tumulicola strain T5916-2-1b (Isolate 7), Pseudochrobactrum saccharolyticum strain CCUG (Isolate 13), Lysinibacillus xylanilyticus strain BN-13 (Isolate 1), Serratia liquefaciens strain TU-6 (Isolate 6) and Lysinibacillus xylanilyticus strain TU-2 (Isolate 2). Accession numbers have been given in Table 1. The toxicity of the bacterial isolates was evaluated on A. punicae and A. illinoisensis via a topical application method described by Eidy et al. (2016), with slight modification. In brief, five Petri dishes (9 cm) lined with filter paper (Whatman number 2) were prepared for each bacterial cell or filtrate concentration, and then four Taify pomegranate or grapevine leaf discs with a diameter of 1.5 cm were cut out and placed on the filter paper in each dish to feed the aphids. Then, 5 μl of each concentration of bacterial cell suspension or supernatant was dropped directly onto the bodies of the aphids. The individual adult aphids were carefully relocated using a fine camel hairbrush onto the leaf discs in the Petri dishes. In the control conditions, insects were treated with the same volume of distilled water or sterile filtered LB media for each aphid species. After that, the Petri dishes were wrapped with Parafilm and held under laboratory conditions of 25 ± 1°C, 65 ± 3% relative humidity and a 12 l:12 D light–dark cycle. The mortality rate of the aphids was recorded after exposure to the bacterial suspensions or cells for 6, 12, 24 and 48 h. If an insect’s appendages did not move when pushed with a fine-point brush, it was declared dead. Each bioassay was performed with five replicates on different dates. The experiment was repeated twice. Furthermore, LC50 and LC90 values of both the EPB cells and filtrates were determined using Probit analysis (Finney, 1971).
Statistical analysis
A two-way variance analysis (ANOVA) was operated to evaluate the aphid mortality rate, followed by Duncan’s multiple range tests. The results were presented as mean ± standard error (M ± SE). The COSTAT program was used to conduct all analyses. (Version 6.400). Using SPSS Version 23, the values of LC50 and LC90, the 95% confidence limits of the lower and upper values, slope and intercept and the χ2 values of the tested EPB were t-tested (p < 0.05), where p-values less than 0.05 were significantly considered.
Results
Identification of EPBs by sequencing the 16S rRNA gene
A BLASTN search of the rRNA_type strains/16S_ribosomal_RNA database returned the following results. Isolate 1 showed 87.50% identity with Lysinibacillus fusiformis strain DSM 2898 (NR_042072.1) and with Lysinibacillus fusiformis strain NBRC 15717 (NR_112569.1). Similarly, Isolate 2 showed 88.15% identity with Lysinibacillus fluoroglycofenilyticus strain cmg86 (NR_148289.1) and with Lysinibacillus sphaericus strain DSM 28 (NR_042073.1), Bacillus ndiopicus strain FF3 (NR_149205.1), Lysinibacillus macrolides (NR_114920.1), Solibacillus isronensis (NR_115952.1) and Lysinibacillus boronitolerans (NR_041276.1). However, isolate 2 showed identity (98% similarity) with Lysinibacillus xylanilyticus strain TU-2. Isolate 6 showed 99.26% identity with Serratia liquefaciens strain ATCC 27592 (NR_122057.1), and Isolate 7 showed 99.56% identity with Stenotrophomonas maltophilia strain NBRC 14161 (NR_113648.1), Stenotrophomonas tumulicola strain T5916-2-1b (NR_148818.1) and Stenotrophomonas pavanii strain ICB 89 (NR_116793.1). Isolate 13 showed 88.26% identity with Pseudochrobactrum saccharolyticum (NR_042473.1).
The phylogenetic tree analysis results (Figure 1) validated our morphological identification as indicated that Isolate 7 belonged to a Stenotrophomonas tumulicola T5916-2-1b and that Isolate 6 belonged a Serratia liquefaciens strain TU-6. However, isolate 1 belonged to Lysinibacillus xylanilyticus strain BN-13. Whereas, isolate 2 were found to be closely related to Lysinibacillus xylanilyticus strain TU-2. Additionally, isolate 13 was found to be closely related to Pseudochrobactrum sp.
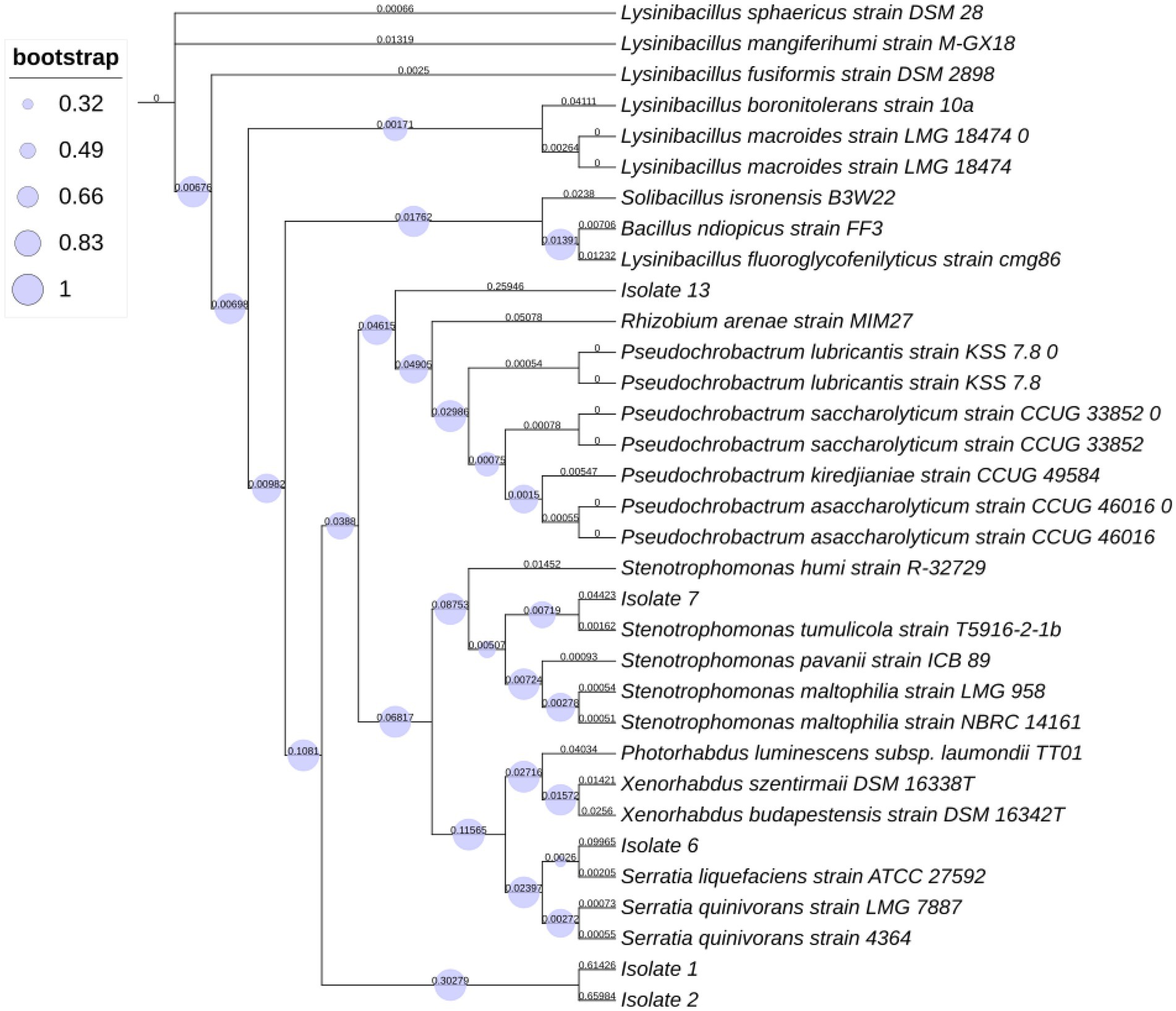
Figure 1. Phylogenetic tree represents distances based on the 16S rRNA sequences acquired from isolates within insect pathogenic nematodes Steinernema feltiae.
Insecticidal activity
Toxicity of bacterial cell suspensions to Aphis illinoisensis and Aphis punicae
The data presented in Table 2 show that the mortality rate of A. illinoisensis adults varied from 0 to 100% after topical application of five bacterial cell suspensions at four concentrations for four exposure times. These results confirmed that the efficiency of the bacterial isolates was directly associated with concentration (Table 2). The data show that cells from all of the bacterial isolates had a significant impact on the mortality rates of A. illinoisensis adults to some extent (p < 0.05), as they caused various levels of mortality in the grapevine aphid (p < 0.05). Table 2 also shows that the A. illinoisensis adults were susceptible (p < 0.05) to all of the bacterial isolates at all tested concentrations and exposure periods. Average mortality rates of 77 and 45.8% were recorded for S. tumulicola strain T5916-2-1b and S. liquefaciens strain TU-6, respectively. Bacterial cells of Lysinibacillus xylanilyticus strain BN-13 were less effective, inducing a mean adult mortality rate of 12.8% (p < 0.05). When individuals were exposed to 108 CFU/ml of S. tumulicola for 24 and 48 h, the greatest A. illinoisensis mortality rate (100%) was detected. There was also a real correlation between mortality rate and exposure time (p < 0.05). As a result, the mortality rate increased dramatically as the exposure period increased., and an adult mortality rate of 44.2% was recorded 48 h post-exposure. High mortality rates were associated with high concentrations of S. tumulicola and S. liquefaciens at all tested times, and considerable mortality rates were associated with L. xylanilyticus strain TU-2. In contrast to the bacterial cells of S. tumulicola causing significant aphid mortality at all tested times when at a low concentration (102 CFU/ml), the mortality rate associated with L. xylanilyticus strain BN-13. Was not significantly different from that of the control treatments (absence of bacteria-derived products), specifically with a 6-h exposure (0%).
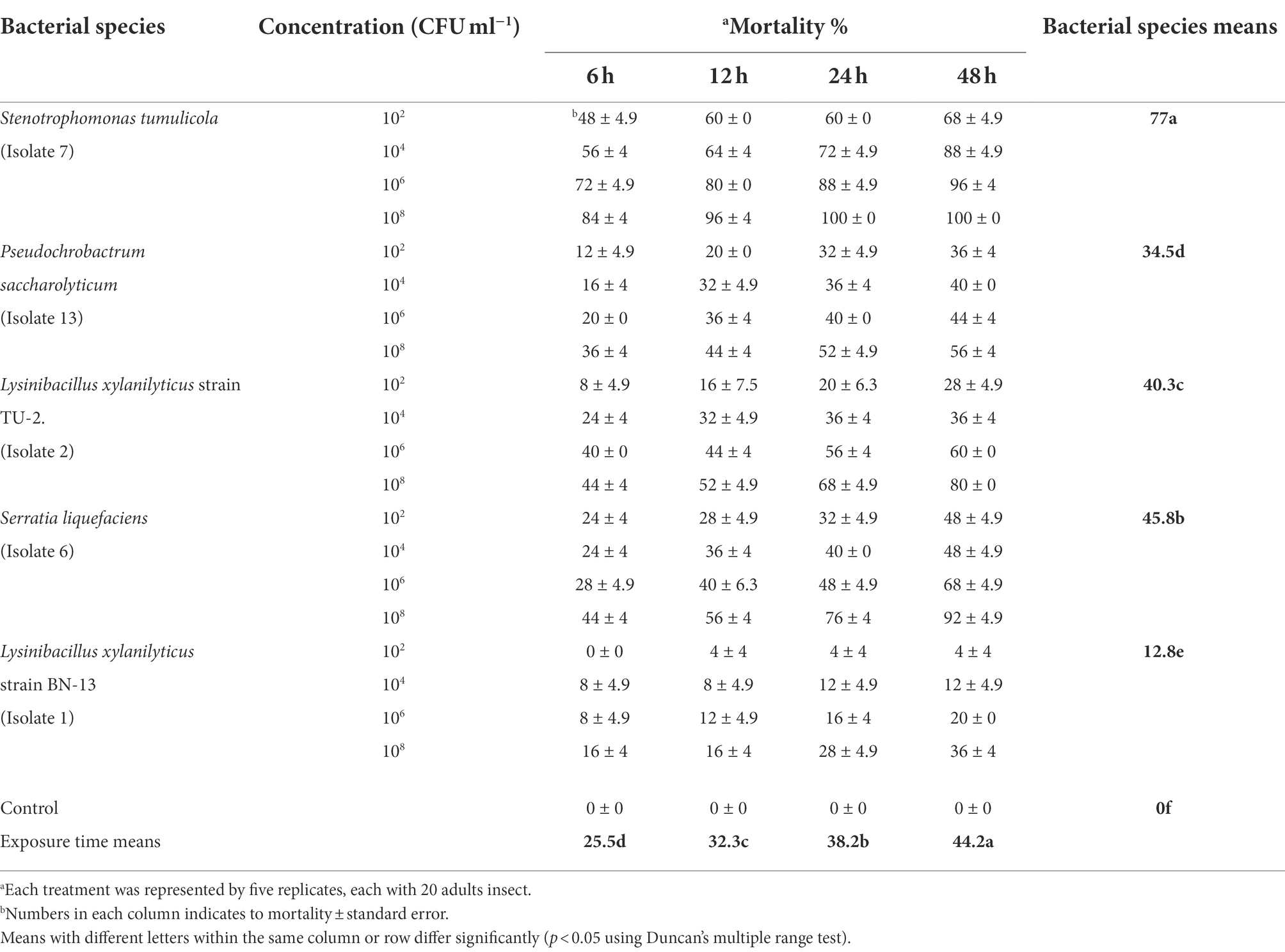
Table 2. Toxicity of five bacterial species cells against the grapevine aphid, Aphis illinoisensis under laboratory condition.
The data in Table 3 confirmed that the bacterial cells of S. tumulicola and S. liquefaciens were the most effective against A. illinoisensis 48 h after treatment, with LC50 values of 2.75 × 10 and 6.76 × 103 CFU/ml and LC90 values of 8.13 × 103 and 3.24 × 107 CFU/ml, respectively. Table 3 also shows that the cells of L. xylanilyticus strain TU-2 were the third most virulent and that Pseudochrobactrum saccharolyticum strain CCUG and L. xylanilyticus strain BN-13 were the least efficient against A. illinoisensis after different exposure durations with LC50 values of 7.41 × 104, 1.26 × 107 and 4.37 × 109 CFU/ml, respectively. For the A. illinoisensis population, the highest degree of homogeneity was found in S. tumulicola and S. liquefaciens with slope values of 2.95 and 2.33, respectively, and the other tested bacterial cell species exhibited low slope values, indicating heterogeneity in the aphid response to these bacterial isolates (Table 3).
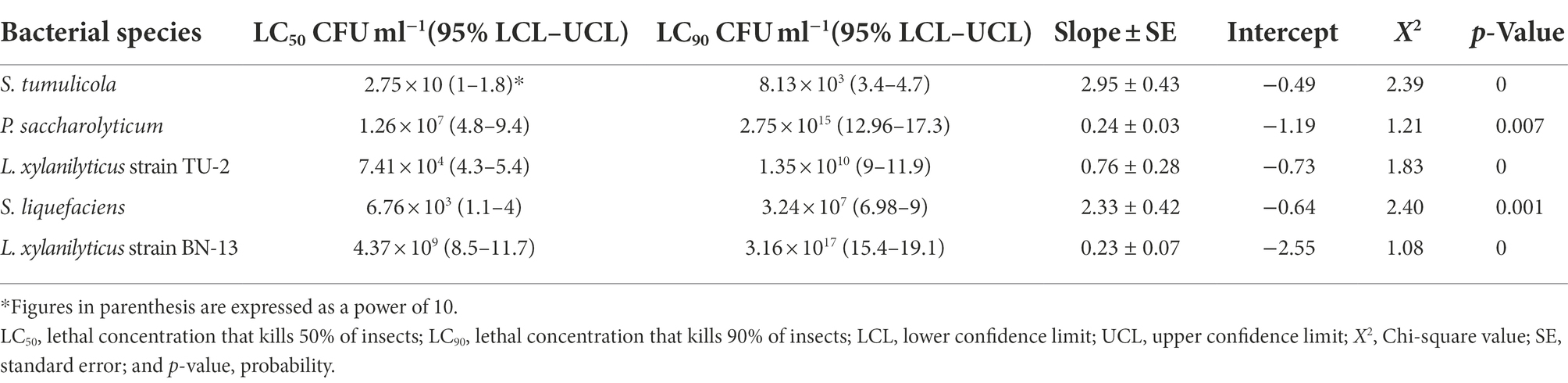
Table 3. Aphicidal activity of five bacterial species cells against A. illinoisensis after 48 h of exposure.
The toxic activity data of five Steinernema-associated bacterial species against A. punicae under laboratory conditions are presented in Table 4. These EPB cells were found to have a significant effect on adult aphid mortality (p < 0.05). Adult lethality was significantly greater in the S. tumulicola isolate (82%) than in the other isolates. Individual mortality rates increased substantially as bacterial cell concentration and exposure time increased (p < 0.05). On aphid infection, there was a strong interaction between EPB species, bacterial cell concentration, and exposure period (p = 0.0113), whereas the interaction between bacterial cell concentration and exposure time was insignificant (p = 0.5263). The maximum mortality rate (100%) was observed with individuals exposed to 108 CFU/ml of S. tumulicola, 6–48 h post treatment (compared to treatment with distilled water), and the lowest mortality rate (4%) was recorded with the adults exposed to 102 CFU/ml of L. xylanilyticus strain BN-13, 6 h after the treatment (Table 4).
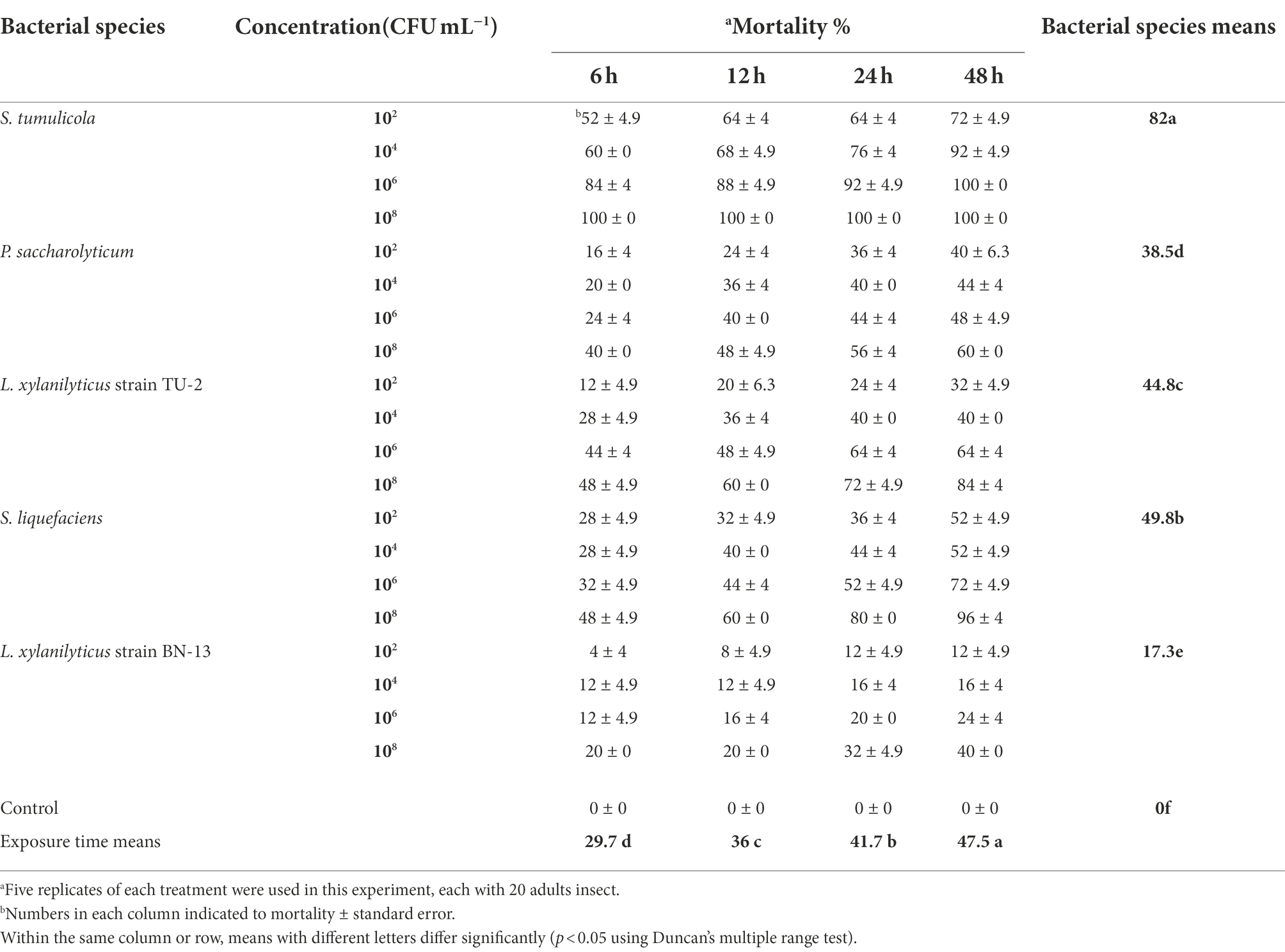
Table 4. Aphicidal activity of five bacterial species cells on the pomegranate aphid, A. punicae under laboratory condition.
As shown in Table 5, of all the tested bacterial species, S. tumulicola cells were the most effective in terms of toxicity against A. punicae adults 48 h after treatment, with an LC50 of 2.69 × 101 CFU/ml and an LC90 of 1.55 × 103 CFU/ml. In comparison, S. liquefaciens cells recorded an LC50 of 2.34 × 103 and an LC90 of 1.75 × 107 CFU/ml (Table 4), and L. xylanilyticus strain BN-13 cells recorded higher LC50 and LC90 values of 1.55 × 109 and 1.35 × 1018 CFU/ml, respectively. It was also clear that S. tumulicola and S. liquefaciens isolates exhibited high slope values (3.68 and 2.56), which indicates homogeneity in the pomegranate aphid response to these bacteria (Table 4).
Toxicity of bacterial filtrates to Aphis illinoisensis and Aphis punicae
The same tendency was observed when the influence of bacterial filtrate on the mortality of A. illinoisensis adults was examined (Table 6). According to these findings, individual mortality was also found to be highly influenced by bacterial species, filtrate concentration, and exposure time (p < 0.05). S. tumulicola exceeded all of the other tested bacteria in A. illinoisensis mortality at all concentrations and exposure periods tested. S. tumulicola induced an adult mortality rate of 73.5%, and isolates S. liquefaciens, L. xylanilyticus strain TU-2, P. saccharolyticum and L. xylanilyticus strain BN-13 induced mortality rates of 41.8, 36.8%, 30.5 and 9%, respectively. A. illinoisensis adults were significantly killed by a bacterial cell-free suspension at 600 μl/ml (46.2%; p < 0.05). Exposure time significantly affected the mortality rate (p < 0.05); a 41% mortality rate was observed 48 h post-exposure. As appeared in Table 2, aphid mortality (means ± SE) ranged from 44 to 100%, 20 to 88%, 4 to 76%, 8 to 52, and 0 to 32%, respectively (p < 0.05) when S. tumulicola, S. liquefaciens, L. xylanilyticus strain TU-2, P. saccharolyticum and L. xylanilyticus strain BN-13 were applied at 100–600 μl/ml. Furthermore, the cell-free supernatant of L. xylanilyticus strain BN-13 did not cause any mortality at 100 μl/ml at any tested times, and initiates toxicity (4% mortality) up to 32% when tested at 200–600 μl/ml (Table 6).
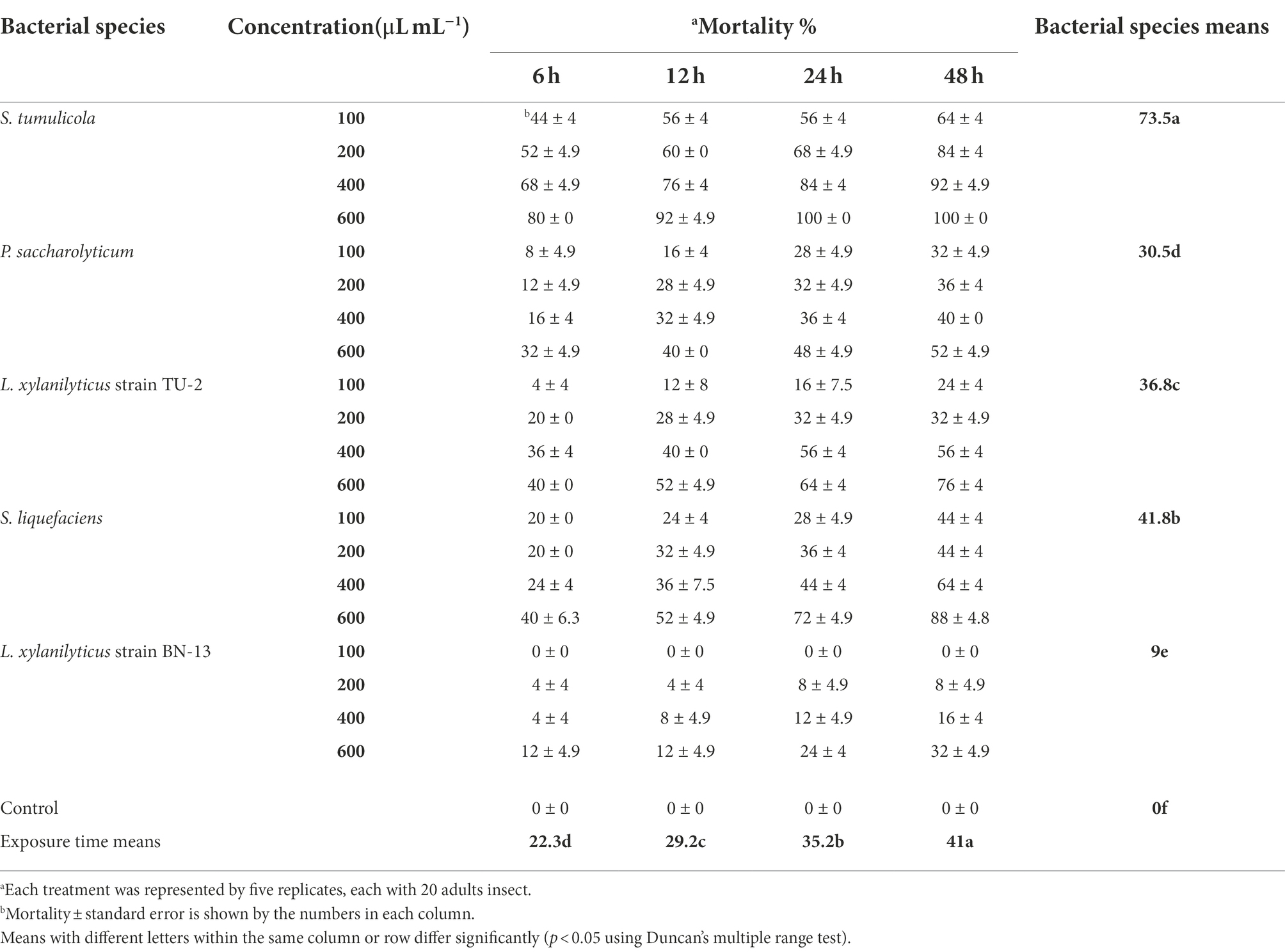
Table 6. Aphicidal activity of five bacterial species filtrates on the grapevine aphid, A. illinoisensis under laboratory condition.
Likewise, the data presented in Table 7 clarified that the filtrate of S. tumulicola isolate was more effective against grapevine aphid adults than the filtrates of all other tested isolates; the LC50 and LC90 at 48 h after treatment were 70.3 and 271.1 μl/ml, respectively. It’s also worth mentioning that L. xylanilyticus strain BN-13 and P. saccharolyticum isolates, having values of LC50 (958.8 and 586.5 μl/ml) and LC90 values of 3,318 and 1888 μl/ml, respectively, after 48 h were the least effective (Table 7). The highest degree of homogeneity for A. illinoisensis was observed for S. tumulicola (slope value of 2.38), and Low slope values were recorded for the other bacterial filtrate concentrations examined, indicating that the grapevine aphid response to these concentrations was heterogeneous (Table 7).
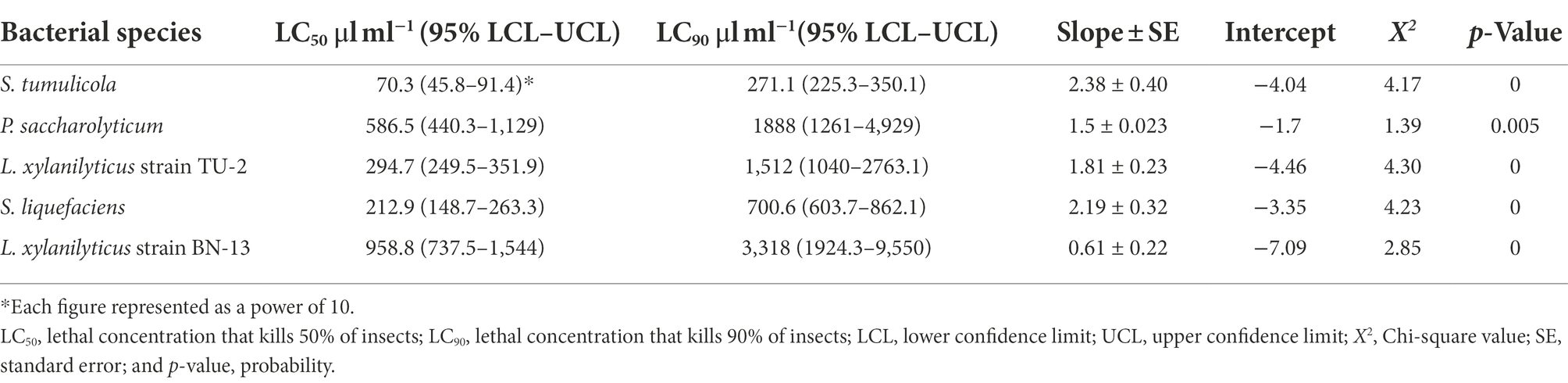
Table 7. Toxicity of five bacterial species filtrates against A. illinoisensis after 48 h of exposure.
The mortality rates of A. punicae were statistically significant (p < 0.05) at all concentrations from 6 to 48 h (Table 8). The data’s regression analysis revealed that mortality of A. punicae adults significantly increased with bacterial concentration (R2 = 0.938; p < 0.05). When they were treated with 600 and 100 μl/ml, respectively, they had the highest (49.3%) and lowest (23.3%) mortality rates. Among all tested bacterial species, S. tumulicola was the most toxic: it induced a 77.3% mortality rate in A. punicae adults, whereas S. liquefaciens, L. xylanilyticus strain TU-2, P. saccharolyticum and L. xylanilyticus strain BN-13 isolates induced mortality rates of 45.8, 40.8, 34.5 and 13.3%, respectively. The overall mortality of the A. punicae adults after treatment with 100–600 μl/ml of S. tumulicola filtrate ranged from 48 to 100%, while it ranged from 24 to 92% for S. liquefaciens, and from 8 to 80% for L. xylanilyticus strain TU-2. In contrast, it ranged from 0 and 36% and from 12 to 56% for L. xylanilyticus strain BN-13 and P. saccharolyticum, respectively. Table 8 shows that the A. punicae adults were extremely vulnerable (p < 0.05) to both the S. tumulicola and S. liquefaciens filtrates, since they recorded 100 and 92% mortality 48 h post treatment. S. tumulicola induced 100% individual mortality at 24 and 48 h post-treatment, and S. liquefaciens induced 76 and 92% mortality at 600 μl/ml at the same exposure times, respectively. There was also an increase in adult mortality as the bacterial filtrate concentration and exposure time increased (Table 8).
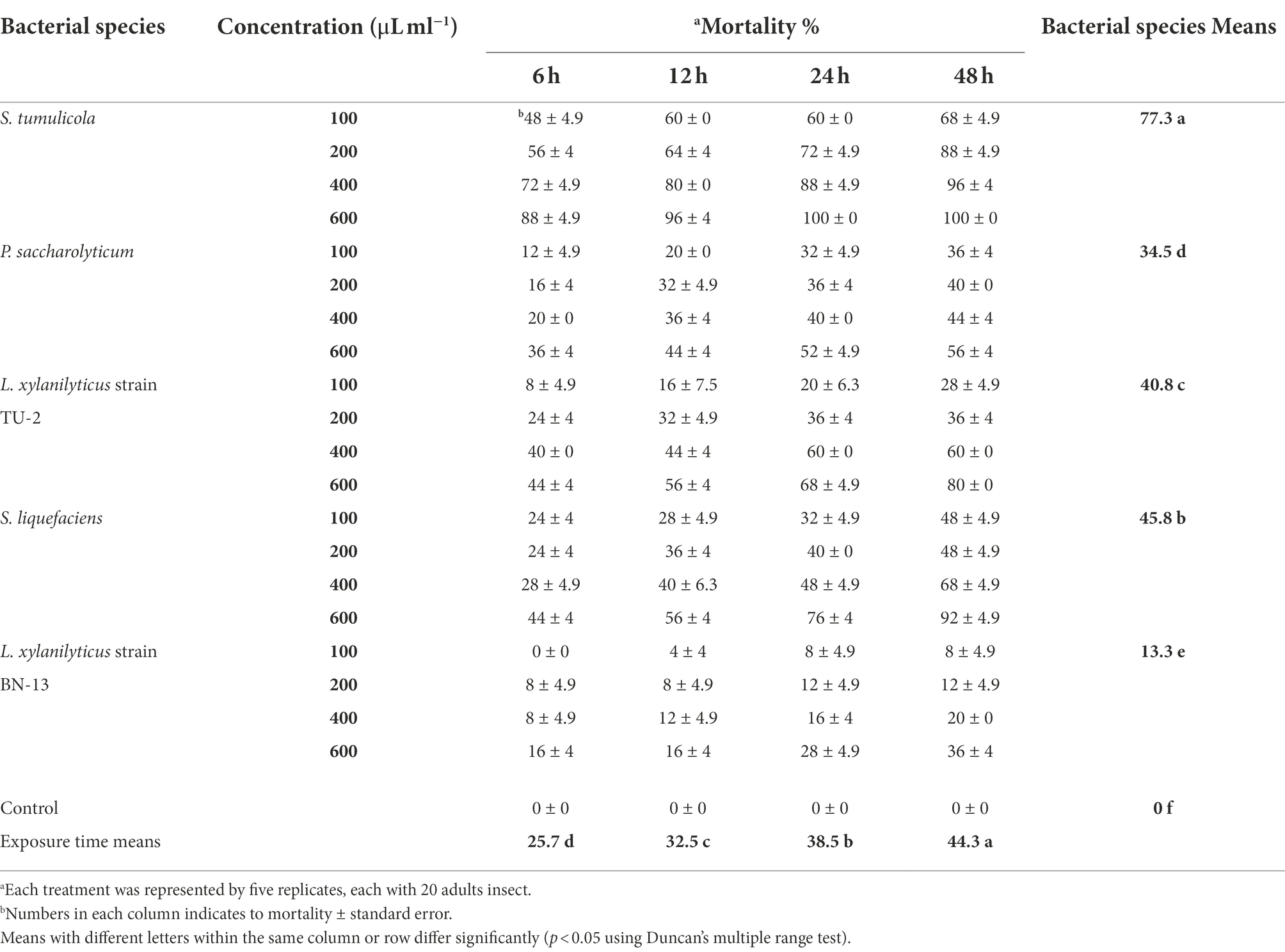
Table 8. Aphicidal activity of five bacterial species filtrates on the pomegranate aphid, A. punicae under laboratory condition.
The LC50 and LC90 values for each isolate at 48 h after treatment against A. punicae adults are shown in Table 9. The LC50 and LC90 values for S. tumulicola were 65.4 and 218.6 μl/ml. The LC50 and LC90 values for isolates S. liquefaciens, L. xylanilyticus strain TU-2, P. saccharolyticum and L. xylanilyticus strain BN-13 were 212.9 and 587.5 μl/ml, 312.1 and 755.3 μl/ml, 483.4 and 1,406.2 μl/ml, and 784.2 and 1,808.3 μl/ml, respectively. In addition, the A. punicae individuals exhibited different degrees of homogeneity in response to the tested bacterial filtrates. The slope values ranged from 0.59 to 2.45 (Table 9). Additionally, S. tumulicola had the highest degree of homogeneity for A. punicae, with a slope value of 2.45.
Discussion
The first phase of this research resulted in the successful recovery and isolation of EPB from S. feltiae. Five bacterial isolates associated with EPN S. feltiae were identified. These findings are consistent with and add to those previously reported by (Alotaibi et al., 2021 and Noureldeen et al., 2022), who isolated Xenorhabdus sp. and Photorhabdus sp. from the EPNs Steinernema sp. and Heterorhabditis sp., respectively, in the same region and recorded their complex activities against Meloidogyne incognita, which infects pomegranate under greenhouse conditions. The five isolated species of Steinernema-associated bacteria found here were molecularly identified and termed isolates; based on phylogenetic tree analysis, L. xylanilyticus strain TU-2, L. xylanilyticus strain BN-13, S. tumulicola, S. liquefaciens and P. saccharolyticum. In the present study, we found that these five isolates were symbiotically associated with S. feltiae, which were discovered for the first time in Saudi Arabia. The obtained data are in agreement with those earlier stated by (Ogier et al., (2020), on four species of Steinernema and their associated bacteria. In that study, two species, Pseudomonas chlororaphis and Pseudomonas protegens, which are often members of the associated microbiota possibly, engaged in Steinernema’s parasitic lifecycle, exhibited entomopathogenic capabilities, implying a role in the virulence and pathobiome membership of Steinernema. Also our results are confirmed by (Moawad and Al-Barty, (2011), Dirksen et al., (2016), Hartman et al., (2017), and Lacerda Júnior et al., (2017).
Regarding the insecticidal activity, it was clear that the five bacterial isolates were more effective on the pomegranate aphid under laboratory conditions than on the grapevine aphid, which showed low susceptibility. It was also clear that S. tumulicola and S. liquefaciens could control the pomegranate and grapevine aphids. The obtained results also accomplished that S. tumulicola isolate was better than S. liquefaciens against both A. punicae and A. illinoisensis adults; however, A. illinoisensis was more resistant. S. tumulicola isolate either cells or filtrates, showed aphicidal activity against A. punicae and A. illinoisensis systematically or through direct contact, causing lethality to the adult stage of both aphid species, and the accumulative mortality approached 100% 48 h following treatment. These results were in accordance with those of (Alotaibi et al., 2021) who observed that the two Xenorhabdus bacterial species EMA and EMC causing significant higher E. ceratoniae larvae mortality than that caused by the Photorhabdus species TT01. In a recent study conducted by Elbrense et al., (2021), it was discovered that H. bacteriophora and its symbiont, Photorhabdus sp., were more virulent against Pieris rapae and Pentodon algerinus. Our findings are in conformity with the results of (Jabeen et al., 2018), who measured the amount of bacterial chitinases produced by S. maltophilia and their termiticidal potential, and (Amer et al., 2021), who claimed that S. maltophilia had antimicrobial activity against a variety of multidrug-resistant bacteria and fungi. Likewise, Rhizoctonia solani, a phytopathogen, was inhibited by S. maltophilia, probably due to antibiosis and the production of lytic enzymes that eliminate fungi (Berg, 1996). Following research, it was shown that the synthesis of novel bioactive compounds is mostly due to S. maltophilia’s metabolic diversity, including molecules that could be employed in bio-control against microbes and insects (Ribitsch et al., 2012). Overall, bacterial isolates are showing more toxicity toward the aphids than the bacterial cell free extract. This is most likely because during the extraction process, significant metabolites of bacterial crude cell extract were lost, or they were never present. Second, various EPB species have variable protein densities, which is why different EPB results have varied. It has been established that the protein encoding density of several Xenorhabdus species varies greatly (Kim et al., 2017) While the crude cell extract was shown to be less effective than other fractions of EPB, the data clearly show that it contains certain harmful proteins that remain there after centrifugation and are responsible for death. However, the relationship between biological processes and temperature is also real and significant.
Conclusion
In current study, we found that these five bacteria isolates were associated with S. feltiae, and be identified as the first recorded at Taif, Saudi Arabia. The effectiveness of these bacteria was examined against the two important insect pests of pomegranate and grapevine, A. punicae and A. illinoisensis. Our results offer a reliable base for promising biocontrol methods and agents that could be used in managing piercing-sucking insects. Further investigations are needed, especially regarding other associated microorganisms for developing new environmentally friendly insect pests control toward suitable agricultural production. In the future, the inoculation of these bacteria can be used directly as biocontrol agents or they can be used in combination with other available methods of biocontrol for better managements of insect pests.
Data availability statement
The data presented in the study are deposited in the NCBI repository, accession number OP001649, OP002058, OP002063, OP578131, and OP578132.
Author contributions
SSA and AN: conceptualization and writing—original draft. HD, SSA, and AN: data curation. HD, AN, SJ and AA-B: formal analysis. SSA, AA, and AN: investigation. HD, AN, MZ, and AA-B: methodology. SSA: project administration. SSA, SA, BA, and AN: resources. HD and AA: validation. AN and BA: visualization. SSA, LK, AB, UK, and AN: writing—review and editing. All authors contributed to the article and approved the submitted version.
Funding
The current work was funded by Taif University Researchers Supporting Project number (TURSP-2020/295), Taif university, Taif, Saudi Arabia.
Acknowledgments
The authors extend their appreciation to Taif University for funding current work by Taif University Researchers Supporting Project number (TURSP-2020/295), Taif University, Taif, Saudi Arabia.
Conflict of interest
The authors declare that the research was conducted in the absence of any commercial or financial relationships that could be construed as a potential conflict of interest.
Publisher’s note
All claims expressed in this article are solely those of the authors and do not necessarily represent those of their affiliated organizations, or those of the publisher, the editors and the reviewers. Any product that may be evaluated in this article, or claim that may be made by its manufacturer, is not guaranteed or endorsed by the publisher.
References
Akhurst, R. (1980). Morphological and functional dimorphism in Xenorhabdus spp., bacteria symbiotically associated with the insect pathogenic nematodes Neoaplectana and Heterorhabditis. Microbiology 121, 303–309.
Alghamdi, A., El-Deen, A. N., Al-Barty, A., and Hassan, M. (2017). Toxicity of some plant extracts and entomopathogenic nematode against pomegranate aphid, Aphis punicae under laboratory condition. Annu. Res.Rev. Biol. 21, 1–6. doi: 10.9734/ARRB/2017/38575
Aljuboori, F. K., Ibrahim, B. Y., and Mohamed, A. H. (2022). Biological control of the complex disease of rhizoctonia solani and root-knot nematode meloidogyne javanica on chickpea by Glomus spp. and Pseudomonas sp. Iraqi J. Agric. Sci. 53, 669–676.
Alotaibi, S. S., Darwish, H., Alharthi, S., Alghamdi, A., Noureldeen, A., Fallatah, A. M., et al. (2021). Control potentials of three Entomopathogenic bacterial isolates for the carob moth, Ectomyelois ceratoniae (Lepidoptera: Pyralidae) in pomegranates. Agriculture 11:1256. doi: 10.3390/agriculture11121256
Amer, A., Hamdy, B., Mahmoud, D., Elanany, M., Rady, M., Alahmadi, T., et al. (2021). Antagonistic activity of bacteria isolated from the Periplaneta americana L. gut against some multidrug-resistant human pathogens. Antibiotics 10:294. doi: 10.3390/antibiotics10030294
Berg, R. D. (1996). The indigenous gastrointestinal microflora. Trends Microbiol. 4, 430–435. doi: 10.1016/0966-842X(96)10057-3
Bisch, G., Pagès, S., McMullen Ii, J. G., Stock, S. P., Duvic, B., Givaudan, A., et al. (2015). Xenorhabdus bovienii CS03, the bacterial symbiont of the entomopathogenic nematode Steinernema weiseri, is a non-virulent strain against lepidopteran insects. J. Invertebr. Pathol. 124, 15–22. doi: 10.1016/j.jip.2014.10.002
Blackman, R. L., and Eastop, V. F. (2011). Additions and amendments to “aphids on the World’s plants”. Zootaxa 2774, 57–68. doi: 10.11646/zootaxa.2774.1.3
Boemare, N., Akhurst, R., and Mourant, R. (1993). DNA relatedness between Xenorhabdus spp.(Enterobacteriaceae), symbiotic bacteria of entomopathogenic nematodes, and a proposal to transfer Xenorhabdus luminescens to a new genus, Photorhabdus gen. Nov. Int. J. Syst. Evol. Microbiol. 43, 249–255.
Dillman, A. R., Guillermin, M. L., Lee, J. H., Kim, B., Sternberg, P. W., and Hallem, E. A. (2012). Olfaction shapes host–parasite interactions in parasitic nematodes. Proc. Natl. Acad. Sci. 109, E2324–E2333. doi: 10.1073/pnas.1211436109
Dirksen, P., Marsh, S. A., Braker, I., Heitland, N., Wagner, S., Nakad, R., et al. (2016). The native microbiome of the nematode Caenorhabditis elegans: gateway to a new host–microbiome model. BMC Biol. 14, 1–16. doi: 10.1186/s12915-016-0258-1
Eidy, M., Rafiee-Dastjerdi, H., Zargarzadeh, F., Golizadeh, A., and Mahdavi, V. (2016). Pathogenicity of the entomopathogenic fungi Beauveria bassiana (Balsamo) and Verticillium lecanii (Zimmerman) against aphid Macrosiphum rosae, Linnaeus (Hemiptera: Aphididae) under laboratory conditions. Jord. J. Biol. Sci. 9, 25–28. doi: 10.12816/0027004
Elbrense, H., Elmasry, A., Seleiman, M. F., Al-Harbi, M. S., El-Raheem, A., and Ahmed, M. (2021). Can symbiotic bacteria (Xenorhabdus and Photorhabdus) be more efficient than their Entomopathogenic nematodes against Pieris rapae and Pentodon algerinus larvae? Biology 10:999. doi: 10.3390/biology10100999
El-Gantiry, A., El-Heneidy, A., Mousa, S., and Adly, D. (2012). Aphis illinoisensis Shimer (Hemiptera: Aphididae) a recent invasive aphid species in Egypt. Egypt. J. Biol. Pest Control 22:225.
Fang, X., Li, Z., Wang, Y., and Zhang, X. (2011). In vitro and in vivo antimicrobial activity of Xenorhabdus bovienii YL002 against Phytophthora capsici and Botrytis cinerea. J. Appl. Microbiol. 111, 145–154. doi: 10.1111/j.1365-2672.2011.05033.x
Fodor, A., Gualtieri, M., Zeller, M., Tarasco, E., Klein, M. G., Fodor, A. M., et al. (2022). Type strains of Entomopathogenic nematode-symbiotic bacterium species, Xenorhabdus szentirmaii (EMC) and X. budapestensis (EMA), are exceptional sources of non-ribosomal templated, large-target-spectral, thermotolerant-antimicrobial peptides (by both), and Iodinin (by EMC). Pathogens 11:342. doi: 10.3390/pathogens11030342
Fouad, H., Hongjie, L., Hosni, D., Wei, J., Abbas, G., Ga’al, H., et al. (2018). Controlling Aedes albopictus and Culex pipiens pallens using silver nanoparticles synthesized from aqueous extract of Cassia fistula fruit pulp and its mode of action. Artif. Cells, Nanomed. Biotechnol. 46, 558–567. doi: 10.1080/21691401.2017.1329739
Fuchs, S. W., Grundmann, F., Kurz, M., Kaiser, M., and Bode, H. B. (2014). Fabclavines: bioactive peptide–polyketide-Polyamino hybrids from Xenorhabdus. Chembiochem 15, 512–516. doi: 10.1002/cbic.201300802
Ga’al, H., Yang, G., Fouad, H., Guo, M., and Mo, J. (2021). Mannosylerythritol lipids mediated biosynthesis of silver nanoparticles: an eco-friendly and operative approach against chikungunya vector Aedes albopictus. J. Clust. Sci. 32, 17–25. doi: 10.1007/s10876-019-01751-0
Gaugler, R., and Kaya, H. K. (1990). Entomopathogenic Nematodes in Biological Control. Boca Raton, FL: CRC Press.
Hartman, K., Van Der Heijden, M. G., Roussely-Provent, V., Walser, J.-C., and Schlaeppi, K. (2017). Deciphering composition and function of the root microbiome of a legume plant. Microbiome 5, 1–13. doi: 10.1186/s40168-016-0220-z
Hazir, S., Kaya, H. K., Stock, S. P., and Keskin, N. (2004). Entomopathogenic nematodes (Steinernematidae and Heterorhabditidae) for biological control of soil pests. Turk. J. Biol. 27, 181–202.
Herz, A., Köppler, K., Vogt, H., Elias, E., Peters, A., and Katz, P. (2006). Biological control of the cherry fruit fly, Rhagoletis cerasi L.(Diptera, Tephritidae) by use of entomopathogenic nematodes: first experiences towards practical implementation. In Ecofruit-12th International Conference on Cultivation Technique and Phytopathological Problems in Organic Fruit-Growing: Proceedings to the Conference, 31st January to 2nd February 2006, Weinsberg/Germany (pp. 67–72).
Jabeen, F., Hussain, A., Manzoor, M., Younis, T., Rasul, A., and Qazi, J. I. (2018). Potential of bacterial chitinolytic, Stenotrophomonas maltophilia, in biological control of termites. Egypt. J. Biol. Pest Control 28, 1–10. doi: 10.1186/s41938-018-0092-6
Jin, D., Zeng, F., Dong, S., and Zhang, H. (2014). Effects of a protease inhibitor protein from Xenorhabdus bovienii on physiology of pea aphid (Acyrthosiphon pisum). Pestic. Biochem. Physiol. 108, 86–91. doi: 10.1016/j.pestbp.2013.12.010
Kim, I.-H., Aryal, S. K., Aghai, D. T., Casanova-Torres, Á. M., Hillman, K., Kozuch, M. P., et al. (2017). The insect pathogenic bacterium Xenorhabdus innexi has attenuated virulence in multiple insect model hosts yet encodes a potent mosquitocidal toxin. BMC Genom. 18, 1–25. doi: 10.1186/s12864-017-4311-4
Kumar, S., Stecher, G., Li, M., Knyaz, C., and Tamura, K. (2018). Mega X: molecular evolutionary genetics analysis across computing platforms. Mol. Biol. Evol. 35, 1547–1549. doi: 10.1093/molbev/msy096
Kumar, U., Saqib, H. S. A., Islam, W., Prashant, P., Patel, N., Chen, W., et al. (2022). Landscape Composition and Soil Physical–Chemical Properties Drive the Assemblages of Bacteria and Fungi in Conventional Vegetable Fields. Microorganisms 10:1202.
Li, F., and Han, Z. (2004). Mutations in acetylcholinesterase associated with insecticide resistance in the cotton aphid, Aphis gossypii glover. Insect Biochem. Mol. Biol. 34, 397–405. doi: 10.1016/j.ibmb.2004.02.001
McMullen, J. G., Ii, R. M., Ogier, J.-C., Pagès, S., Gaudriault, S., and Stock, S. P. (2017). Variable virulence phenotype of Xenorhabdus bovienii (γ-Proteobacteria: Enterobacteriaceae) in the absence of their vector hosts. Microbiology 163, 510–522. doi: 10.1099/mic.0.000449
Moawad, S. S., and Al-Barty, A. M. (2011). Evaluation of some medicinal and ornamental plant extracts toward pomegranate aphid, Aphis punicae (Passerini) under laboratory conditions. Afr. J. Agric. Res. 6, 2425–2429. doi: 10.5897/AJAR11.294
MTöth, E., Márialigeti, K., Fodor, A., Lucskai, A., and Farkas, R. (2005). Evaluation of efficacy of entomopathogenic nematodes against larvae of Lucilia sericata (Meigen, 1826) (Diptera: Calliphoridae). Acta Vet. Hung. 53, 65–71. doi: 10.1556/avet.53.2005.1.7
Noureldeen, A., Kumar, U., Asad, M., Darwish, H., Alharthi, S., Fawzy, M., et al. (2022). Aphicidal activity of five plant extracts applied singly or in combination with entomopathogenic bacteria, Xenorhabdus budapestensis against rose aphid, Macrosiphum rosae (Hemiptera: Aphididae). J. King Saud Univ. Sci. 34:102306. doi: 10.1016/j.jksus.2022.102306
Ogier, J.-C., Frayssinet, M., and Gaudriault, S. (2020). Entomopathogenic nematode-associated microbiota: from monoxenic paradigm to pathobiome. Microbiome 8, 1–17. doi: 10.1186/s40168-020-00800-5
Pertot, I., Caffi, T., Rossi, V., Mugnai, L., Hoffmann, C., Grando, M., et al. (2017). A critical review of plant protection tools for reducing pesticide use on grapevine and new perspectives for the implementation of IPM in viticulture. Crop Prot. 97, 70–84. doi: 10.1016/j.cropro.2016.11.025
Pfeiffer, D. G., and Schultz, P. B. (1986). Major Insect and Mite Pests of Grape in Virginia. Virginia: Virginia Cooperative Extension Service.
Ribitsch, D., Heumann, S., Karl, W., Gerlach, J., Leber, R., Birner-Grünberger, R., et al. (2012). Extracellular serine proteases from Stenotrophomonas maltophilia: screening, isolation and heterologous expression in E. coli. J. Biotechnol. 157, 140–147. doi: 10.1016/j.jbiotec.2011.09.025
Salim, F. D., Ibrahim, K. M., and Yousif, W. H. (2022). The effectiveness of extract the seed of pomegranate in healing the wound induced inrabbits skin. Iraqi J. Agric. Sci. 53, 265–271.
Sajnaga, E., and Kazimierczak, W. (2020). Evolution and taxonomy of nematode-associated entomopathogenic bacteria of the genera Xenorhabdus and Photorhabdus: an overview. Symbiosis 80, 1–13. doi: 10.1007/s13199-019-00660-0
Salvadori, J. D. M., Defferrari, M. S., Ligabue-Braun, R., Lau, E. Y., Salvadori, J. R., and Carlini, C. R. (2012). Characterization of entomopathogenic nematodes and symbiotic bacteria active against Spodoptera frugiperda (Lepidoptera: Noctuidae) and contribution of bacterial urease to the insecticidal effect. Biol. Control 63, 253–263. doi: 10.1016/j.biocontrol.2012.08.002
Shi, D., An, R., Zhang, W., Zhang, G., and Yu, Z. (2017). Stilbene derivatives from Photorhabdus temperata SN259 and their antifungal activities against phytopathogenic fungi. J. Agric. Food Chem. 65, 60–65. doi: 10.1021/acs.jafc.6b04303
Singh, S., Reese, J. M., Casanova-Torres, Á. M., Goodrich-Blair, H., and Forst, S. (2014). Microbial population dynamics in the hemolymph of Manduca sexta infected with Xenorhabdus nematophila and the entomopathogenic nematode Steinernema carpocapsae. Appl. Environ. Microbiol. 80, 4277–4285. doi: 10.1128/AEM.00768-14
Tailliez, P., Pages, S., Ginibre, N., and Boemare, N. (2006). New insight into diversity in the genus Xenorhabdus, including the description of ten novel species. Int. J. Syst. Evol. Microbiol. 56, 2805–2818. doi: 10.1099/ijs.0.64287-0
Tamura, K., and Nei, M. (1993). Estimation of the number of nucleotide substitutions in the control region of mitochondrial DNA in humans and chimpanzees. Mol. Biol. Evol. 10, 512–526.
Vitta, A., Thimpoo, P., Meesil, W., Yimthin, T., Fukruksa, C., Polseela, R., et al. (2018). Larvicidal activity of Xenorhabdus and Photorhabdus bacteria against Aedes aegypti and Aedes albopictus. Asian Pac. J. Trop. Biomed. 8:31. doi: 10.4103/2221-1691.221134
Yooyangket, T., Muangpat, P., Polseela, R., Tandhavanant, S., Thanwisai, A., and Vitta, A. (2018). Identification of entomopathogenic nematodes and symbiotic bacteria from Nam Nao National Park in Thailand and larvicidal activity of symbiotic bacteria against Aedes aegypti and Aedes albopictus. PLoS One 13:e0195681. doi: 10.1371/journal.pone.0195681
Keywords: entomopathogenic bacteria, molecular identification, Aphis punicae, Aphis illinoisensis, biological control
Citation: Baazeem A, Alotaibi SS, Khalaf LK, Kumar U, Zaynab M, Alharthi S, Darwish H, Alghamdi A, Jat SK, Al-Barty A, Albogami B, Noureldeen A and Ravindran B (2022) Identification and environment-friendly biocontrol potential of five different bacteria against Aphis punicae and Aphis illinoisensis (Hemiptera: Aphididae). Front. Microbiol. 13:961349. doi: 10.3389/fmicb.2022.961349
Edited by:
Pankaj Bhatt, Purdue University, United StatesReviewed by:
Kalpana Bhatt, Gurukul Kangri Vishwavidyalaya, IndiaSaurabh Gangola, Graphic Era Hill University, India
Copyright © 2022 Baazeem, Alotaibi, Khalaf, Kumar, Zaynab, Alharthi, Darwish, Alghamdi, Jat, Al-Barty, Albogami, Noureldeen and Ravindran. This is an open-access article distributed under the terms of the Creative Commons Attribution License (CC BY). The use, distribution or reproduction in other forums is permitted, provided the original author(s) and the copyright owner(s) are credited and that the original publication in this journal is cited, in accordance with accepted academic practice. No use, distribution or reproduction is permitted which does not comply with these terms.
*Correspondence: Ahmed Noureldeen, YS5ub3VyZWxkZWVuQHR1LmVkdS5zYQ==; Balasubramani Ravindran, a2FsYW1yYXZpQGdtYWlsLmNvbQ==