- Department of Immunochemistry, Laboratory of Glycobiology, Hirszfeld Institute of Immunology and Experimental Therapy Polish Academy of Sciences, Wrocław, Poland
Glycosphingolipids (GSLs) are ubiquitous components of the cell membranes, found across several kingdoms of life, from bacteria to mammals, including humans. GSLs are a subclass of major glycolipids occurring in animal lipid membranes in clusters named “lipid rafts.” The most crucial functions of GSLs include signal transduction and regulation as well as participation in cell proliferation. Despite the mainstream view that pathogens rely on protein–protein interactions to survive and thrive in their hosts, many also target the host lipids. In particular, multiple pathogens produce adhesion molecules or toxins that bind GSLs. Attachment of pathogens to cell surface receptors is the initial step in infections. Many mammalian pathogens have evolved to recognize GSL-derived receptors. Animal glycosphingolipidomes consist of multiple types of GSLs differing in terminal glycan and ceramide structures in a cell or tissue-specific manner. Interspecies differences in GSLs dictate host specificity as well as cell and tissue tropisms. Evolutionary pressure exerted by pathogens on their hosts drives changes in cell surface glycoconjugates, including GSLs, and has produced a vast number of molecules and interaction mechanisms. Despite that abundance, the role of GSLs as pathogen receptors has been largely overlooked or only cursorily discussed. In this review, we take a closer look at GSLs and their role in the recognition, cellular entry, and toxicity of multiple bacterial, viral and fungal pathogens.
Introduction
Glycosphingolipids (GSLs) are amphipathic lipids consisting of hydrophilic glycan and hydrophobic ceramide (N-acylsphingosine) moieties. Ceramides comprise a sphingoid base (aminoalcohol) linked to a fatty acid through an amide bond (Figure 1A). Due to the variety of sugar structures (ranging from monosaccharide residues to branched glycan moieties), sphingoid and acyl chains, GSLs represent the largest group of sphingolipids (Merrill et al., 2007; Gault et al., 2010). These molecules sort with specific microdomains in the plasma membrane called lipid rafts. GSLs are classified into broad types based on carbohydrate composition. In animals, glucosylceramide is the cornerstone for the synthesis of all complex GSLs, with the largest containing over 20 sugar residues (found in the human placenta; Ladisch et al., 1995; Merrill et al., 2007; Ishibashi et al., 2009). Step-wise extension of these glycan moieties generates a series of neutral root structures that are commonly found in different species and tissues, including globo, isoglobo, lacto, neolacto, ganglio, gala, muco, arthro, and mollu (Figure 1B). These root structures serve as the basis for a widely used nomenclature system (in addition to traditional names). For example, a GSL blood group antigen historically called Forssman, which belongs to the globo series, is a globotetraosylceramide with α1,3-linked N-acetylgalactosamine or IV3-α-GalNAc-Gb4Cer (with the roman numeral indicating which sugar in the root structure, counting from the ceramide end, carries the substituent and the superscript indicating the linkage position on that sugar, in this case, C-3; Chester, 1997; Merrill et al., 2007). Traditionally, all acidic GSLs containing one or more sialic acid residues are called gangliosides, including the compounds based on other than ganglio root structures (Chester, 1997; Yu et al., 2009; Giussani et al., 2014). Lactosylceramide may also be directly mono-, di- or trisialylated, whence elongation of the ganglio root structure continues in the same way as for the unsialylated lactosylceramide, generating the 0-, a-, b- and c-series pathways of ganglioside synthesis, respectively (Merrill et al., 2007; Giussani et al., 2014).
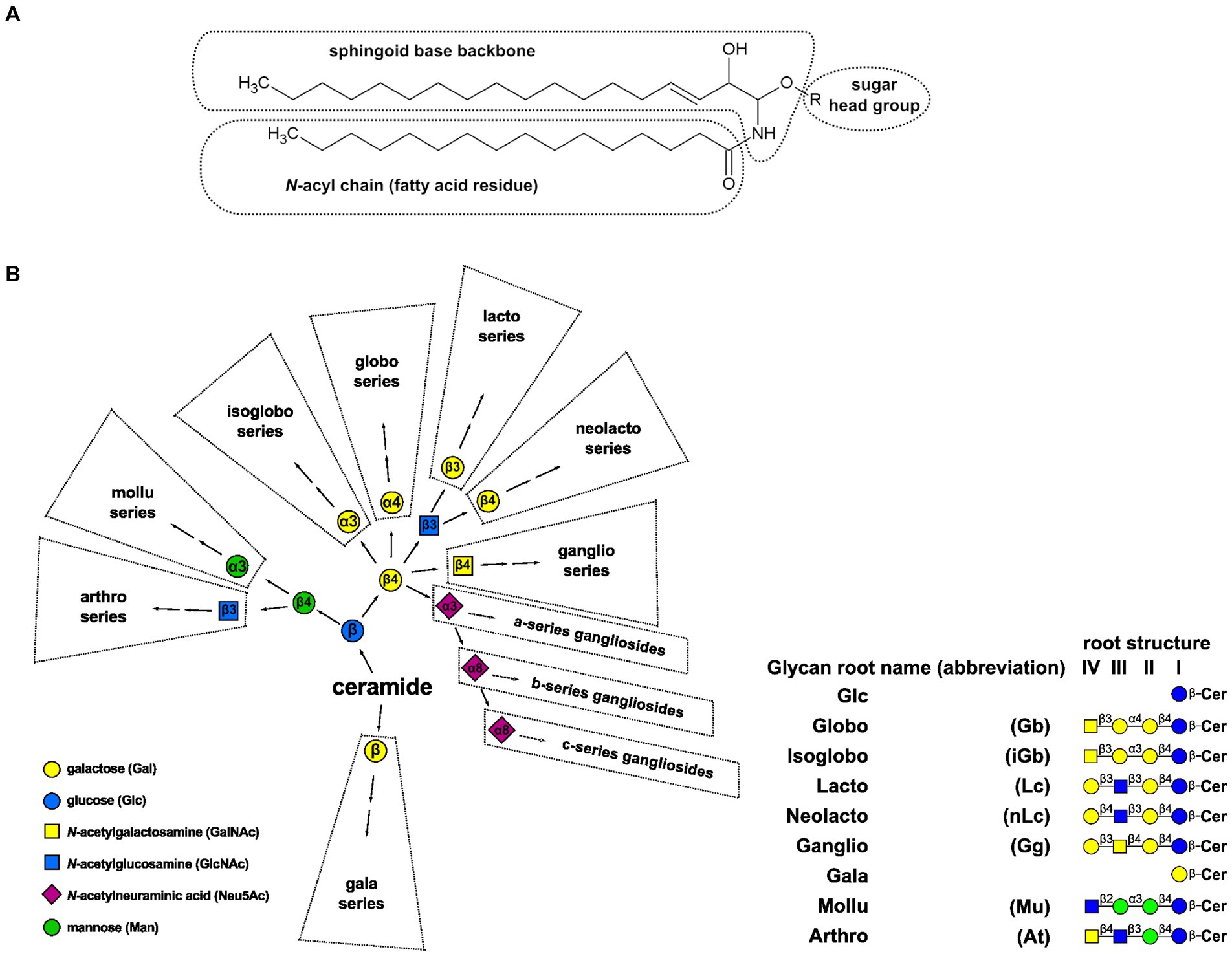
Figure 1. (A) Schematic representation of a GSL structure. Ceramide moiety comprises sphingoid backbone (based on sphingosine d18:1Δ4) and N-acyl chain. (B) A backbone relational depiction of major GSL synthesis pathways and an overview of glycan root structures. Names and symbols are presented on figure according to IUPAC (Merrill et al., 2007), while monosaccharide symbols are consistent with Varki and Sharon recommendations (Varki et al., 2017); Cer: ceramide.
Many viruses, bacteria and fungi produce adhesins or toxins that recognize GSLs. Cell surface GSLs provide pathogens with unique opportunities to invade and thrive in the host. Adhesins and toxins specifically recognize oligosaccharide chains of GSLs, which protrude beyond the cell membrane, but subsequent events and the fate of the microbe or toxin may depend on additional factors, such as density of GSL packaging in the host cell membrane, the cell membrane microenvironment, and other membrane components, including proteins, mostly transmembrane or GPI-anchored (Duncan et al., 2002; Taïeb et al., 2004). High-affinity binding of pathogens to GSL receptors involved in crucial functions in host cells such as signal transduction pathways or intracellular transport have not been thoroughly studied to date. The affinity linkage between microbial toxins or adhesins to host GSL receptors may affect the mechanism of toxicity or adhesion and the intracellular transport of pathogens or their virulence factors. The hydrophobic part of GSLs affects the conformation of the glycone part, and hence, it may affect the receptor functions of GSLs (Smith et al., 2004; D’Angelo et al., 2013). Recent studies have shown that the molecular ‘spacer’ function of cholesterol in lipid membranes could also affect the conformation and binding properties of GSLs. Some bacterial virulence factors (e.g., cholera toxins) bind to receptors located in condensed complexes with cholesterol in lipid membranes in vitro (Radhakrishnan et al., 2000).
Many pathogens recognize sugar antigens (Unione et al., 2017; Thompson et al., 2019), which has driven modifications of cell surface sugars by hosts as a means of defense. To hinder recognition of cell surface sugars by pathogens, hosts use three strategies:
1. The sugar antigen recognized by the pathogen is modified so that it is no longer recognized by the pathogen (Cserti and Dzik, 2007);
2. The sugar antigen recognized by the pathogen ceases to be produced altogether, e.g., via pseudogenization. One example is the disappearance of the Galα1 → 3Gal antigen, which, additionally, increases resistance to pathogens carrying that structure (Koike et al., 2002);
3. The sugar antigen becomes a decoy receptor that binds the pathogen but prevents it from entering. Examples include the A and B blood group antigens and the cholera toxin or sugar antigens from the human milk (A/B, Lewis) and rotaviruses (Anstee, 2010; El-Hawiet et al., 2015; Orczyk-Pawiłowicz and Lis-Kuberka, 2020).
Many GSL blood group antigens may act as a pathogen or toxin receptors or co-receptors. Here, we review bacterial, viral, and fungal pathogens or toxins, and the mechanisms whereby they hijack cell surface GSLs to invade, evade or incapacitate the host cells (summarized in Figures 2, 3; Table 1).

Table 1. Summary of described pathogen ligands, host receptors and relevance of these interactions in infectious diseases epidemiology.
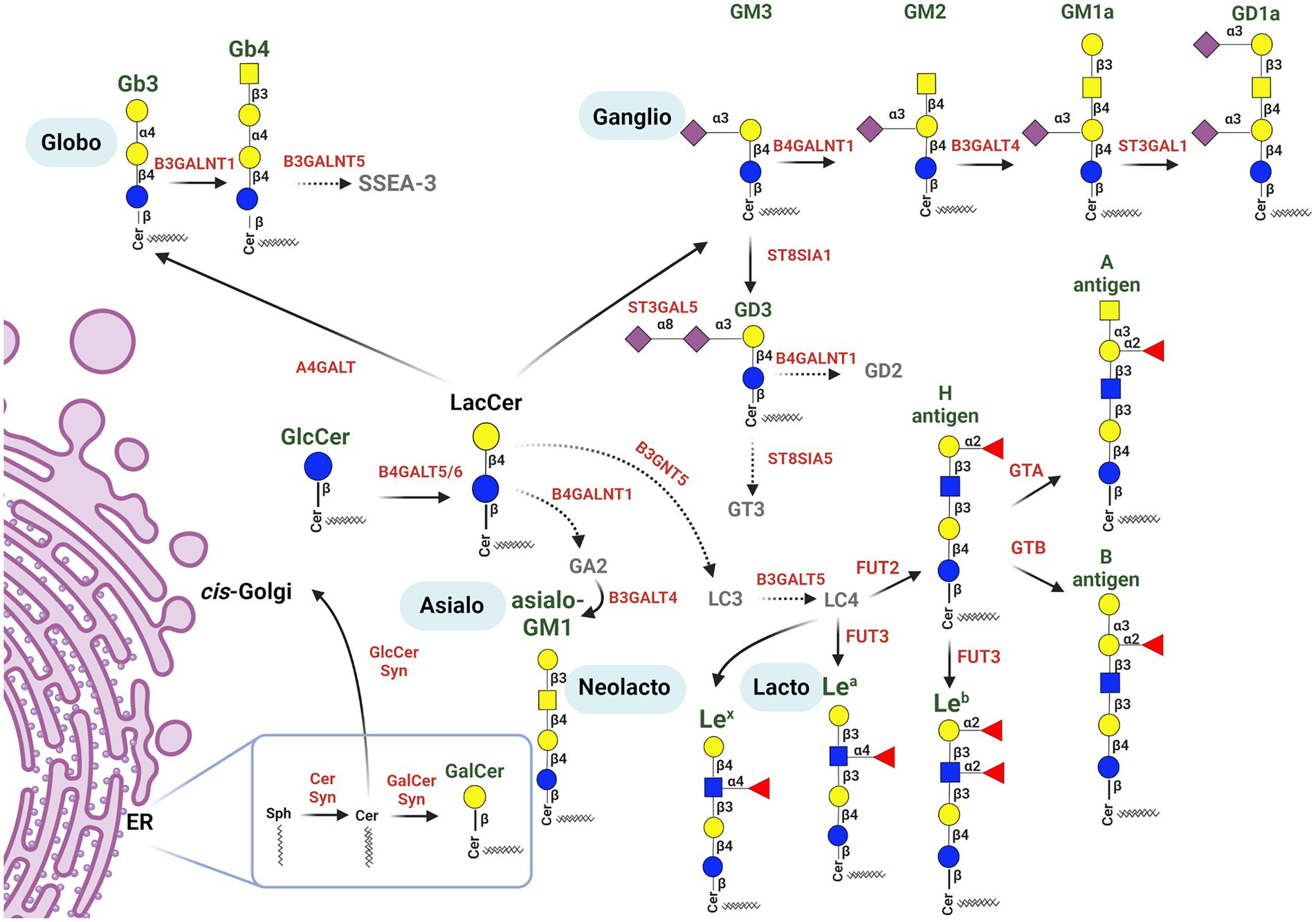
Figure 2. Schematic representation of major glycosphingolipid biosynthesis pathways, highlighting GSLs participating in pathogen binding. Biosynthesis of GSLs proceeds in the endomembrane system, beginning in the endoplasmic reticulum by ceramide synthesis from sphingoid base and acyl-CoA, and continues in the Golgi apparatus. Ceramide may be galactosylated in the ER (producing GalCer) or transported to the cis-Golgi to produce GlcCer and LacCer. LacCer is used for the production of more complex GSLs by specific Golgi-resident glycosyltransferases, creating several GSL series pathways. LacCer is utilized by (1) β1,4-N-acetylgalactosylaminyltransferase (B4GALNT1) initiating the asialo series; (2) β1,3-N-acetylglucosaminyltransferase (B3GNT5), producing Lc3, which is converted to Lc4, the precursor for the lacto and neolacto series GSLs; (3) α1,4-galactosyltransferase (A4GALT), producing Gb3, thus initiating the globo series pathway; (4) α2,3-sialyltransferase (ST3GAL5), producing GM3, which belongs to the ganglio series. The ABO histo-blood group antigens are subsequently formed from the H antigen (it is also the precursor of the Leb antigen, belonging to the Lewis blood group system) by specific GTs; α1,3-N-acetylgalactosyltransferase (A transferase) synthesizes the A antigen, while α1,3-galactosyltransferase (B transferase) synthesizes the B antigen. The Lea and Lex blood group antigens are created by fucosyltransferase 3. More complex glycan structures of ganglio-series GSLs are formed by a sequential action of different GTs, such as N-acetylgalactosaminyltransferases, galactosyltransferases and sialyltransferases, producing the GM2, GM1a and GD1a gangliosides. GM3 may be further processed by α2,8-sialyltransferase 1, forming GD3. GSLs in green play roles in interactions with pathogens. Glycosyltransferases involved in GSL synthesis are shown in red [Figure was created with BioRender.com].
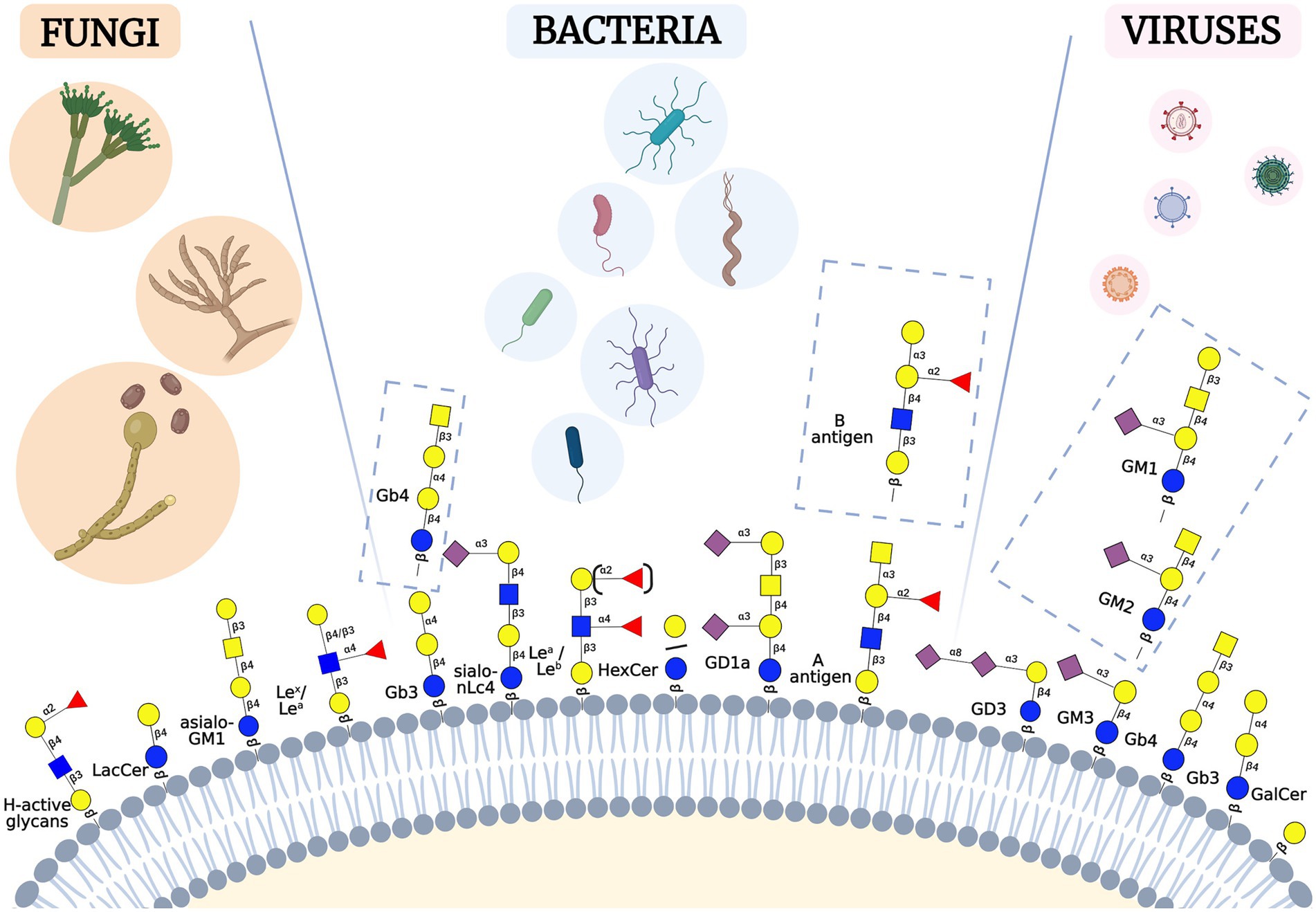
Figure 3. Schematic representation of selected GSLs involved in host-pathogen interactions. Fungi, bacteria and viruses may bind various types of GSLs residing in the human membranes. These GSLs belong to different GSL-series, such as asialo, lacto, neolacto, ganglio [Figure was created with BioRender.com].
Interaction between bacteria and host GSLs
Acinetobacter baumannii
Acinetobacter baumannii is a nosocomial pathogen typically causing pneumonia, but also urinary tract infections, skin infections and meningitis, mostly in hospitalized patients (Madar Johansson et al., 2020). Multiresistant strains of A. baumannii are a growing public health issue and they are on WHO’s high priority list of pathogens demanding urgency in development of new antimicrobial compounds. The outer membrane protein A (OmpA), the biofilm-associated protein (BAP), the Acinetobacter trimeric autotransporter adhesin (Ata) and the BAP-like proteins 1 and 2 (BLP-1 and BLP-2) were shown to take part in adhesion of bacterial cells to human epithelial cells and biofilm formation (Giannouli et al., 2013). Mass spectroscopy analysis of human small intestine glycosphingolipids recognized by A. baumannii proteins revealed lactotetraosylceramide (Galβ3GlcNAcβ3Galβ4Glcβ1Cer) and neolactotetraosylceramide (Galβ4GlcNAcβ3Galβ4Glcβ1Cer). The bacteria are also bound to lactotriaosylceramide (GlcNAcβ3Galβ4Glcβ1Cer), isoglobotriaosylceramide, gangliotriaosylceramide, gangliotetraosylceramide, galactosylceramide, lactosylceramide with phytosphingosine and/or hydroxy-fatty acids, indicating a N-acetylglucosamine moiety as a basic structure recognized by A. baumannii (Madar Johansson et al., 2020). Despite the high degree of homology with P. aeruginosa, LecA protein (PA-IL), LecA of A. baumannii does not bind to glycoconjugates with terminal Galα1 → 4Gal or Galα1 → 3Gal moieties (besides iGb3; Blanchard et al., 2008; Madar Johansson et al., 2020).
Campylobacter jejuni enteritis
Neurons of human peripheral nerves produce large amounts of gangliosides GM1 and GD1a. Strikingly the high level of expression of GM1 on the nodal membranes of human motor nerves has substantial implications for the persistence and severity of post-infectious complications (Yanaka et al., 2019). Most of the described strains of C. jejuni can also produce glycoconjugates (GM1-like or GD1a-like lipooligosaccharides) on the bacterial cell surface. Anti-GM1 IgG1 autoantibodies arising in response to C. jejuni infections may lead to complement-mediated motor nerve injury. The binding of anti-GM1 autoantibodies to peripheral motor nerves underlies Guillain–Barré syndrome (GBS), which is defined as a post-infectious autoimmune neuropathy with characteristic acute limb weakness (Lee et al., 2004; Prendergast et al., 2004). GBS follows C. jejuni infections in one-third of patients (Yamana et al., 2019). Moreover, GBS was identified in patients previously infected with: Mycoplasma pneumoniae, herpes simplex virus type 1 (HSV-1), and Epstein–Barr virus (Yuki, 1997; Sheikh et al., 1998; Tse et al., 2012).
Clostridium sp.
Clostridium botulinum produces seven serotypes of Botulinum neurotoxin (A-G), each of which is a single polypeptide of approximately 150 kDa consisting of two heavy and light chains linked by a disulfide bond. The carboxy part of the heavy chain participates in adhesion, while the N-terminal part mediates entry of the light chain (zinc endoprotease) into the host cell (Orrell et al., 2017). The toxin binds to its receptors on the terminal neurons of neuromuscular junctions. A number of studies indicate that the 16S and 19S complexes of the hemagglutinin complex may bind to specific GSLs. Thus, the C-type progenitor binds sialoparagloboside, ganglioside GM3 and paragloboside. The Type A progenitor recognizes GSLs with terminal galactose, particularly paragloboside and Gb3 and LacCer. Many studies indicate that a large number of BoNT serotypes require the presence of 1b-series gangliosides for the most effective binding to the target cell. The only exception is serotype G, which binds in the absence of gangliosides. Detailed studies have shown that ganglioside binding occurs through the C-terminus of a heavy chain, and removal of ten amino acid residues from its sequence abolishes the binding (Barth et al., 2004; Yowler and Schengrund, 2004).
Another strain of Clostridium sp., C. tetani produces the tetanus neurotoxin (TeNT). The toxin binds to vesicle-associated membrane protein-2 (VAMP-2), which inhibits neurotransmitter release in the central nervous system, and thus causes spastic paralysis and death. The molecular mechanism of TeNT entry into neurons is still unclear. Receptor binding occurs on presynaptic membrane of human α-motor neurons and involves gangliosides (Montecucco, 1986; Mocchetti, 2005). Interactions between TeNT and gangliosides have been well described in structural and biochemical studies in vitro and in vivo. Such interactions on the host cell surface required two molecules of gangliosides: an a-series ganglioside (GD1a, involving its lactose moiety and the TeNT W carbohydrate-binding pocket) and a b-series ganglioside (GT2, GD3, involving their sialic acids moieties and the TeNT R carbohydrate-binding pocket; Chen et al., 2009). TeNT cytotoxicity requires both receptors (Rummel et al., 2003; Chen et al., 2008, 2009).
Clostridium difficile toxin (CDT) consists of an enzymatic component with ADP-ribosyltransferase activity and a translocation/binding component. This binary actin-ADP-ribosylating toxin can depolymerize the actin cytoskeleton and induce formation of microtubule-based membrane protrusions (Barbieri et al., 2002). These activities enhance bacterial adhesion and colonization by virulent strains of C. difficile. The main glycosphingolipid receptor for this toxin is the ganglioside GM1 localized in lipid microdomains. Numerous studies have shown that composition of the lipid bilayer microenvironment, especially the presence of cholesterol, can favor membrane protrusions. Indeed, depletion of cholesterol from lipid microdomains abolishes the effects of the toxin and reduces membrane protrusions. The microenvironment of plasma membrane and composition of lipid bilayer are the main players in ligand-receptor interactions (Barth et al., 2004; Schwan et al., 2011).
Escherichia coli
Uropathogenic E.coli (UPEC) strains produce two types of adhesive surface organelles named Chaperone-usher (CU) fimbriae 1 and P, found in many Gram-negative bacteria (Wurpel et al., 2013). FimH, the adhesive subunit of type 1 fimbriae, binds uroplakins, which are mannosylated glycoproteins present on the bladder epithelial cells (urothelium). This is a key step in the invasion of urothelium during chronic E. coli cystitis (Mulvey et al., 1998). Type P fimbriae contain PapG subunits, which recognize Galα1 → 4Galβ residues in the globo-series GSLs on kidney epithelium. Some of these GSLs are histo-blood group antigens belonging to the P1PK blood group system formerly known as P, which is why the P fimbriae were so named (Söderhäll et al., 1997; Kaczmarek et al., 2014; Terlizzi et al., 2017). Pili F1C and S adhesins: SfaH and FocH bind sialylα2 → 3lactose and GalNAcβ1 → 4Galβ disaccharide in asialoceramides (Khan et al., 2000a,b). The Fm1H is an adhesin of F9 and binds Galβ1 → 3GalNAc (the TF antigen). Studies have shown that replacing 3 amino acids in 3 loops forming FimH and Fm1H caused a change in binding specificity of these adhesins from terminal α-D mannose to terminal β-D galactose or β-D GalNAc (Moonens and Remaut, 2017).
Enterotoxigenic Escherichia coli (ETEC) strains are the main cause of diarrhea in young children from developing countries. Adhesion and colonization of host intestinal epithelium depend on heat-stable and/or heat-labile enterotoxins. Fimbrial structures on bacterial cells’ surfaces are referred to as colonization factors (CFs) that mediate the adhesion of ETEC. Recently published studies indicate that ETEC can bind to numerous GSLs structures on host intestinal epithelium, e.g.: iGb3, nLc4, GgO3, GgO4, lactosylceramide with phytosphingosine and/or hydroxy fatty acids and glycosphingolipids with terminal HBGA determinants (Lea, Lex, and Ley; Jansson et al., 2006; Roussel et al., 2017).
Shiga toxin-producing Escherichia coli strains (STEC) can cause enterocolitis, bloody diarrhea, and, sometimes, a severe complication called hemolytic-uremic syndrome (HUS). Worldwide, STEC infects several million individuals annually, posing a growing threat to public health (Majowicz et al., 2014).
Shiga (Stx) toxins belong to the AB5 class of protein toxins (Fan et al., 2000). This group also includes pertussis toxin (Ptx), cholera toxin (Ctx), thermolabile enterotoxins produced by E.coli (LT-I and LT-II) and subtilase (SubAB; Paton et al., 2004; Johannes and Römer, 2010). The enzymatic activity responsible for cytotoxicity is located in the A subunit, which is often classified as a type II ribosome-inactivating protein (RIP) or ER-driven protein toxin (ERT, ER-routing protein toxin). The A subunit consists of the A1 and A2 fragments linked by a disulfide bridge. The pentamer of the B subunits forms a pore, containing the C-terminal fragment of the A subunit (Silva et al., 2017).
The pentamers of Shiga toxin B subunits bind GSLs of the globo series, showing a strong preference for the trisaccharide Galα1 → 4Galβ1 → 4Glc. Stx2e (a subtype pathogenic for pigs) also uses Gb4Cer (GalNAcβ1 → 3Galα1 → 4Galβ1 → 4Glc) as a receptor as well as Gb5Cer with the structure Galβ1 → 3GalNAcβ1 → 3Galα1 → 4Galβ1 → 4Glc and GalNAcα1 → 3GalNAcβ1 → 3Galα1 → 4Galβ1 → 4Glcβ1 → 1Cer also known as the Forssman antigen (Gallegos et al., 2012; Detzner et al., 2021). Recently, it was shown that Shiga toxin can also bind Galα1 → 4Gal structures on N-glycans and use them as functional receptors (Szymczak-Kulus et al., 2021). Severe complications of STEC infection in humans result from Shiga toxin-mediated damage to the endothelium. This triggers release of proinflammatory cytokines, including tumor necrosis factor alpha (TNF-α), which inadvertently exacerbates the cytotoxicity by enhancing the synthesis of Gb3 in the endothelium, creating a vicious circle (Foster et al., 2000).
Helicobacter pylori
Helicobacter pylori is the best-known pathogen of the human gastrointestinal tract, with half of the human population being infected. Helicobacter pylori colonizes the gastric mucosa. Prolonged infection may increase the risk of gastric cancer (Díaz et al., 2018). One of the H. pylori adhesins BabA binds Leb from group O individuals fivefold greater than Leb from group A individuals (Anstee, 2010; Benktander et al., 2012). In A and B blood type individuals, these glycotopes are hidden under the α1,3-linked residues of GalNAc or Gal, respectively, and BabA binds A and B antigens only weakly. Gastric mucosa of type O individuals is more susceptible to the binding of adhesin (Lingwood, 1999). During chronic infections, H. pylori upregulates the host β3GnT5 gene expression, which leads to increased synthesis of the Lex structure. In addition, the pathogen produces SabA, which is an adhesin that binds Lex and Lea glycoproteins (Kościelak, 2012; Jin et al., 2018). Both adhesins belong to the Helicobacter Outer Membrane Proteins family (HOPs), which also includes LabA. LabA recognizes the GalNAcβ1 → 4GlcNac structure, which is characteristic of the gastric mucosa glycoproteins. The HOP protein family have autotransporter-like architecture. The BabA and SabA adhesion ectodomains have similar topologies: 7 α-helices (4 + 3 system). In addition, BabA has a four-twisted β-sheet structure that comprises the binding site for the Leb antigen (Moonens and Remaut, 2017). Results published by Teneberg and co-workers show that lactotetraosylceramide and lactosylceramide may also act as receptors for H. pylori (Teneberg et al., 1997, 2002; Angström et al., 1998; Roche et al., 2007).
Pseudomonas aeruginosa
Pseudomonas aeruginosa is a pathogen that can attack the cell in a variety of ways. Factors contributing to bacterial invasion include GSLs, which affect the adhesion of P. aeruginosa to non-phagocytic cells. LecA, a homotetrameric galactophilic lectin located on the outer bacterial membrane binds GSLs (Kuhaudomlarp et al., 2020). Interaction of LecA with Gb3 produces large monolayer vesicles in the membrane enabling bacteria to enter the host cell (Blanchard et al., 2008; Kuhaudomlarp et al., 2020). This mechanism has been termed the “lipid zipper” and it depends on the composition of the lipid bilayer (Siukstaite et al., 2021). Cholesterol in lipid rafts stabilizes the Lec-A induced domains, and, with a sufficiently high density of Gb3, triggers a lipid zipper resulting in cell invasion. Blocking Lec-A/Gb3 interaction results in reduced invasion of Pseudomonas aeruginosa, and is a promising strategy for drug development (Eierhoff et al., 2014).
Vibrio cholerae
Vibrio cholerae is the causative agent of cholera, a life-threatening diarrhoeal disease. The bacteria colonize the human small intestine and secrete their major virulence factor called cholera toxin (CT; Ali et al., 2016; Deen et al., 2020). CT is a member of the AB5 group of bacterial toxins, composed of one catalytically active A subunit (responsible for the cytotoxicity) and a homopentamer of the receptor-binding B subunits (Bharati and Ganguly, 2011). Originally, it was believed that the B subunit binds GM1 on the apical surface of intestinal epithelial cells. However, the role of GM1 is controversial because GM1 is rare in the human gastrointestinal tract (Holmgren et al., 1975; Wands et al., 2015). Recent studies suggest that fucosylated blood group antigens (e.g., Lex) may function as secondary receptors important for cellular uptake of the toxin and toxicity, but the mechanism remains unclear (Heim et al., 2019; Patry et al., 2019).
Interaction between viruses and host GSLs
HCMV
Human cytomegalovirus (HCMV) is a double-stranded linear DNA β-herpesvirus that typically causes a mild respiratory illness. The mechanism by which the virus infects cells is not fully understood. The infection consists of many stages and involves glycoconjugates. Heparin sulfate on the cell membrane was proposed to initiate binding of the virus. Most molecules reported in the literature as HCMV receptors are glycoproteins (Fernández-Moreno et al., 2021). However, HCMV shows a binding reactivity to SGGLs (sulfated glucuronyl glycosphingolipids), especially to SGLPG (sulfated glucuronyl lactosaminylparagloboside). Inhibition of SGGLs affects both the expression of the IE gene and the plaque formation by HCMV, indicating that the binding of HCMV to the sulfated carbohydrate epitope in SGGL plays an important role in the initial stages of infection. Notably, HCMV binds more strongly to SGLPG than to SGPG. Also, SGLPG had a stronger inhibitory effect than SGPG on HCMV infection. The lactosamine repeats (-3Galβ1 → 4GlcNAc1-)2 of SGLPG, in addition to the 3-sulfated glucuronyl moiety, may be important for recognition by HCMV. Lactosamine repeats could also play a role in preferential binding of HCMV to nLc6Cer compared to nLc4Cer (Ogawa-Goto et al., 1998).
HIV
The genome of HIV comprises two plus (+) sense single RNA strands (Frankel and Young, 1998). The virus contains viral p17 matrix protein (MA), integrase (IN), and the viral protein R (Vpr) helping with viral RNA reverse transcription into double-stranded complementary DNA (cDNA) in the host cell cytoplasm following transport to the cell nucleus where it is integrated into the host cell genome; an envelope as well as a protein core (Lingwood, 2011).
The role of GSLs as receptors in HIV infection is complex. The viral adhesive protein gp120 forms a highly glycosylated trimeric complex on the viral membrane. The first GSL identified as a receptor for the gp120 protein was galactosylceramide and its sulfatide. Other receptors include GM3, GD3, and Gb3. Notably, gp120 shows binding preferences for different GSLs depending on the strain (GM3 for R5X4 HIV, Gb3 for HIV X4; Viard et al., 2003; Puryear and Gummuluru, 2012). The GSL binding site and the chemokine receptor are in the same loop (V3). The cholesterol/GSL ratio in lipid rafts may play a role in HIV infection. Densely packed lipids hinder the initial interaction of the viral protein with the receptor on the host cell (Liao et al., 2001). One of the viral proteins, called Nef, leads to downregulation of CD4 by directing it to a degradation pathway associated with the endoplasmic reticulum (Binette et al., 2007). Gp120 binding to GalCer triggers events leading to infection of CD4-negative cells. Modification of the lipid moiety in GalCer affects binding of the virus to CD4 +/− cells with varying degrees of inhibition. Gp120-GSL interactions may be influenced by the octamer of a conservative peptide from the V3 loop. The GSL binding site is at the center of the chemokine receptor binding in the gp120 protein (Liao et al., 2001). Lund and co-workers showed that a soluble Gb3 (adamantylGb3) analogue may bind gp120 and thus inhibit viral infection in vitro. These inhibition properties were tested for HIV X4, R5 strains, and resistant HIV-1 strains. A similar effect was demonstrated for other Gb3 analogues. Inhibition of infection may be due to blockage of the chemokine receptor binding site(Lund et al., 2006; Lingwood, 2011). An inverse relationship between the amount of Gb3 and HIV infection was shown for individuals with different genotypes in the P1PK blood group system. Lymphocytes lacking Gb3 (the null genotype called p) were the most sensitive, while T cells from the P1k individuals were the most resistant (Lund et al., 2009).
Human Parvovirus B19
The Parvoviridae is a single-stranded DNA small icosahedral viral family divided into Parvovirinae and Densovirinae subfamilies which can infect mammalian and invertebrate hosts, respectively. These subfamilies are further divided into five genera. Parvovirus B19 is classified as Erythrovirus (Thompson et al., 2019).
Human Parvovirus B19 was identified in the serum of an infected patient in 1975 (Brown and Young, 1995). Infection with the B19 parvovirus can cause erythema infectiosum (the Fifth disease), but also arthropathy (joint disease), fetal loss, and anemia. The main cause of anemia following B19 infection is binding of viral particles to erythroid precursors which leads to their destruction. Globoside (Gb4) was identified as the main receptor necessary for parvovirus B19 binding, but not competent for viral particles entry into host cells. For this, B19 requires another receptor: α5β1 integrin. However, this protein co-receptor is not present on mature red blood cells, which highly express Gb4, so B19 can bind but not enter mature red cells (Weigel-Kelley et al., 2003; Bieri and Ros, 2019; Thompson et al., 2019; Bieri et al., 2021). In addition to erythrocytes, B19 can infect several immune cells, including macrophages, B cells, T cells and follicular dendritic cells (Takahashi et al., 1998). Ku80 may play a role as a co-receptor, which mediates binding and entry of the virus (Munakata et al., 2005). Extensive studies on the role of Gb4 and other P1PK blood group system antigens in parvovirus B19 entry revealed that only cells from individuals with p phenotype are not susceptible to the virus. That finding gave rise to the hypothesis that the minimum glycan moiety for virus binding is GluNAc/GalNAcβ1→3Gal structure (Taube et al., 2010).
Noroviruses
Caliciviruses are family of a small, single-stranded positive-sense RNA, non-enveloped and icosahedral viruses. The family consists of five major genera: Norovirus (humans, pigs and mice; Cao et al., 2007; Caddy et al., 2014), Sapovirus (human and pigs), Vesivirus (cats), Lagovirus (rabbits) and Nebovirus (cattle; Stuart and Brown, 2007; Kim et al., 2014). This viral family requires glycans for attachment and host cell entry.
Noroviruses infect human, bovine, and canine gastrointestinal epithelia, as well as RHDV (Rabbit Hemorrhagic Disease Virus), which target hepatocytes. All utilize human blood group-associated antigens (HBGAs) as cell-surface receptors (Huang et al., 2003; Hutson et al., 2003). On the other hand, GI-specific murine norovirus (MNV) and porcine sapovirus bind sialic acids mostly on O-glycans and some gangliosides (Ossiboff et al., 2007; Taube et al., 2010; Haga et al., 2016).
The activity of FUT genes in relation to expression of HBGA (secretor-type Fucα1→2Gal modifications) and levels of Lewis antigens (Fucα1→3/4GlcNAc) are associated with norovirus infection (Nasir et al., 2012; Almand et al., 2017; Someya, 2022). Localization of P2 (the most variable region of the P protein containing the carbohydrate-binding motif) dimers on the viral particle surface has prompted intensive research because of potential interactions with branches of glycans. The universality of these epitopes among mammalian species and the potential for emergence of new P2 variants raise concerns about interspecies transmission (Chassaing et al., 2020).
Polyomaviruses
Polyomaviruses constitute a small family of double-stranded DNA, non-enveloped icosahedral viruses. The family of Polyomaviridae is divided into four genera: α-polyomavirus, β-polyomavirus, γ-polyomavirus, and δ-polyomavirus.
In humans, polyomavirus infections are common but mostly asymptomatic. They can be dangerous for immuno-compromised patients, in whom the infection symptoms may be severe and possibly lead to neoplasms and cancer (Neu et al., 2009; Maginnis et al., 2015).
The outer capsid of polyomavirus consists of pentamers of the VP1 coat protein with the glycan (particularly sialoglycan)-binding domain in most species (O’Hara et al., 2014).
Terminal NeuAcα2→3Galβ1→3GalNAc moiety is recognized by mouse polyomavirus (MPyV) and can be found in both gangliosides (e.g., GD1a) and glycoproteins (O-linked), although the contribution of these receptors in natural infections is still being examined (Tsai et al., 2003). Site-specific mutation at position 91 in VP1 allows binding the receptors with an additional NeuAc in the structure NeuAcα2→3Galβ1→3(NeuAcα2→6)GalNAc, which in some studies is associated with a decreased tumorigenic phenotype (O’Hara et al., 2014). BKV and JCV are well-recognized human polyomaviruses, identified in the same year. Their names were created from the initials of the patients from whom they were isolated (Gardner et al., 1971; Padgett et al., 1971). Both BKV and JCV polyomaviruses (HPyV-1 and HPyV-2, respectively) bind NeuAcα2→3Gal and NeuAcβ2→6Gal structures present in glycans of various ganglioside and glycoproteins from cells and tissues infected by these viruses (urogenital tissue, lymphocytes, renal cells, and astrocytes; Ströh et al., 2015). Merkel Cell Polyomavirus (MCPyV) is a new polyomavirus isolated from aggressive skin cancer, Merkel cell carcinoma (MCC; Gaynor et al., 2007). In recent reports, it was shown that only GT1b, but not GD1a or GD1b bind to MCPyV VP1 protein, suggesting that both α2,3-linked terminal and α2,8-linked internal sialic acids of GT1b are crucial for the interaction (Erickson et al., 2009). Another member of the family, SV40 virus is highly specific for the GM1 ganglioside, with NeuGc instead of NeuAc, but the interaction is relatively weak. Studies have shown that during the attachment viral molecules interact not only with GM1 but also with co-receptor the class I major histocompatibility complex (MHC-I) proteins and entering to cells in a caveolin-dependent or -independent manner (Pelkmans et al., 2001; Damm et al., 2005). Recognition of terminal NeuGc prevents SV40 infection of the human host (Neu et al., 2008).
Rotaviruses
The Reoviridae (divided into two subfamilies: Sedoreovirinae and Spinareovirinae) are double-stranded RNA viruses, with complex, multilayered and icosahedral capsids. The most studied genera are Rotavirus (Sedoreovirinae subfamily) and Orthoreovirus (also named ‘reovirus,’ Spinareovirinae subfamily), which consist of large spike proteins projecting fivefold from the icosahedral (Settembre et al., 2011). The terminal domains of those spike proteins VP8 in rotavirus (Taube et al., 2010) and δ1 (Reiter et al., 2011) bind glycans (Sun et al., 2016).
Infections with rotaviruses (family Reoviridae) are the main cause of diarrhoea in young mammals. Rotaviral infections cause over 500,000 deaths in developing countries and are a frequent cause of childhood morbidity in industrialized countries. Moreover, infections result in significant losses in agriculture (diarrhoea in calf, pig, and poultry production). The infection involves two steps: viral recognition of and binding to the villus tip cells of the small intestine. Receptor analogs may inhibit the binding to host cells (Arias et al., 2008; Mukherjee and Chawla-Sarkar, 2011; Thompson et al., 2019). Human rotavirus (RV) infections are mostly caused by groups A and, to a lesser extent, C of RVs. Group A includes two types of strains: neuraminidase (NA) - sensitive and NA – insensitive, which is determined by the binding affinity of VP8* domain to terminal sialic acid moieties on the host cell surface carbohydrates (Delorme et al., 2001; Haselhorst et al., 2009; Kim et al., 2017). HBGAs, in particular A, H, and Lewis (Leb) antigens, appear to be the main receptors. Some studies suggest that secretor individuals are more susceptible to RV infections than nonsecretors. In the case of some RV strains (P[4], P[8]), nonsecretors develop neutralizing antibodies. Suggesting that they may be affected by those strains (Ayouni et al., 2015; Rodríguez-Díaz et al., 2017). It has been hypothesized that the higher frequency of infections among individuals with blood group A may signify evolution of RV in response to receptor changes in the host. These changes (e.g., αGal-to-GalNAc binding switch) have occurred under selection pressure in many animal species (Zhao et al., 2020).
Fungal ligands binding GSLs
Fungal infections are a global health problem, with increasing incidence worldwide, particularly in patients with immune dysfunctions. It is estimated that over 1 billion people may be affected by fungal infections, including the most commonly detected aspergillosis (caused by Aspergillus spp.) and candidiasis (as a result of Candida spp. invasion), with an estimated mortality of 1 million annually (Lass-Flörl et al., 2021). Fungal pathogens bind many receptors, including glycosphingolipids. The most significant and the best described GSL receptor is lactosylceramide (Galβ1 → 4Glcβ-Cer) which is bound by the most ubiquitous fungi, such as Cryptococcus neoformans, Candida albicans, Saccharomyces cerevisiae and yeast phase of Histoplasma capsulatum and Sporothrix schenckii (Jimenez-Lucho et al., 1990). It was demonstrated that a clinically relevant fungus C. neoformans, responsible for cryptococcosis (May et al., 2016), strongly binds to LacCer-rich brain cells and cultured human glioma cells. Moreover, liposomes containing LacCer inhibited the fungi binding, while glucosylceramide and free lactose did not, confirming the involvement of LacCer in pathogen binding (Jimenez-Lucho et al., 1990). The binding of C. neoformans to LacCer is affected by the structure of the ceramide moiety. Semisynthetic lactosylceramide (named DL-dihydrolactocerebroside) with a short-chain fatty acid is not recognized by the fungal ligands. In addition, the terminal galactose residue of LacCer is essential for binding. Gal-terminated LacCer is crucial for C. neoformans binding because the LacCer precursor (glucosylceramide) and other lactosyl residue-containing neutral and acidic GSLs were not recognized by this pathogen (Jimenez-Lucho et al., 1990).
Candida spp.
Candida spp. are a type of yeast that belong to Saccharomycetaceae family, which causes an array of infectious diseases collectively called candidiasis (Gow et al., 2011; Hani et al., 2015). Over 90% of infections are caused by five fungi: C. albicans (the major pathogen), C. glabrata, C. tropicalis, C. parapsilosis and C. krusei (Bongomin et al., 2017). These yeasts can recognize both non-GSL (stratherin, hydroxyapatite, fibrinogen and fibronectin; Johansson et al., 2000) and GSL receptors (LacCer, asialo-GM1, Lea and H-active glycans; May et al., 1989; Jimenez-Lucho et al., 1990; Brassart et al., 1991; Yu et al., 1994; Johansson et al., 2000; Miyauchi et al., 2007). According to Jemeneza-Lucho LacCer is recognized by C. albicans, but these results were not confirmed in other studies (Yu et al., 1994). LacCer may be bound by Candida spp. or serve as a pattern recognition receptor to neutrophils in response to β-glucan, a component of Candida spp. cell wall (Sato et al., 2006). Generally, GSLs are recognized by lectin-like adhesins, whose specificity differs between strains and the recognition is influenced by growth conditions (Tosh and Douglas, 1992; Cameron and Douglas, 1996). Fucose-binding lectin is the best-studied Candida spp. adhesin. Oligosaccharides with terminal Fucα1 → 2Gal (O/H blood group antigen) were able to decrease Candida spp. adhesion to the epithelial cells, in contrast to internally fucosylated Lewis blood group antigens that did not show an inhibitory effect (Critchley and Douglas, 1987; Tosh and Douglas, 1992; Cameron and Douglas, 1996; Johansson et al., 2000). Other studies shed new light on the role of GSL-derived compounds in host membrane. Notably, GSLs in lipid rafts significantly influence the clearance of fungal pathogens by affecting interactions between Candida spp. and immune cells (in particular macrophages and neutrophils). Neutrophils are crucial immune cells involved in Candida spp. pathogenesis, because they are responsible for clearance of the pathogen. Moreover, macrophages contain LacCer in their own plasma membrane, raising the possibility of interaction with Candida spp. (Bryan et al., 2015; Tafesse et al., 2015).
It was demonstrated in several studies, that Lewis and ABO blood group phenotypes may be associated with susceptibility to Candida spp. The secretor/nonsecretor status (Lewis phenotypes) can influence vaginal candidiasis caused by C. albicans (Hilton et al., 1995; Kulkarni and Venkatesh, 2004). It was shown that Le(a-b-) phenotype was linked to recurrent vaginitis in women (Hilton et al., 1995), but when comparing Le(a + b-) with Le(a-b+) individuals there is no significant difference. Kulkarni and Venkatesh (2004) found correlation between Candida spp. and ABO phenotype and demonstrated that vaginal candidiasis occurs predominating in nonsecretors. It may be explained by the lack of ABO antigens in the body fluids of nonsecretors, which facilitates the attachment of C. albicans to the epithelial cells. Animal model studies support that secretor status may be protective against C. albicans vaginitis (Hurd and Domino, 2004; Domino et al., 2009), which may be also related to modulation of host–microbe interactions by estrogen, a key hormone upregulating FUT2 expression (responsible for secretor status; Fidel et al., 2000; Domino et al., 2009). Data on the role of ABO and Lewis secretor/nonsecretor status in oral candidiasis are conflicting (Aly et al., 1991; Ben-Aryeh et al., 1995). The proportion of nonsecretor status was significantly higher in patients with chronic hyperplastic candidiasis compared to the control subjects (Lamey et al., 1988). A small Indian study comparing ABO type and Candida spp. incidence in patients with duodenal ulcers (Singh et al., 1990) showed that C. albicans most frequently colonized patients with the O blood group, with higher incidence of nonsecretor status among the patients with peptic ulcers (Burford-Mason et al., 1993).
Histoplasma capsulatum
Histoplasma capsulatum is a dimorphic fungus responsible for histoplasmosis, one of the most frequently occurring invasive fungal pulmonary infections (Gnat et al., 2021; Souza et al., 2021). This pathogen produces a cell surface 60 kDa heat shock protein (HSP60) which recognizes complement receptor 3 (CR3) on macrophages, leading to H. capsulatum HSP60 internalization. The interactions between immune cells and H. capsulatum depend on lipid microdomains on the cell surface, and the presence of sialylated GSLs, such as GM1, which is required for recruitment of CR3 into the cell membrane (Lin et al., 2010). Impaired adhesion in the absence of GM1 may be caused by two proposed mechanisms: (1) disorganization of lipid microdomains results in decreased association between macrophages and H. capsulatum or (2) GM1 may be a co-receptor in the initial steps of host-H. capsulatum interactions (Guimarães et al., 2019; Souza et al., 2021). In addition, LacCer, a key GSL in Src family tyrosine kinase Lyn-dependent signaling pathway, plays a crucial role in H. capsulatum pathogenesis by regulating cytoskeleton remodeling (Nakayama et al., 2013).
Paracoccidioides brasiliensis
Paracoccidioides brasiliensis is a pathogenic dimorphic fungus that may cause paracoccidioidomycosis. According to Ywazaki et al. (2011) P. brasiliensis may bind GalCer, LacCer, CTH (Galα1 → 4Galα1 → 4Glcα1 → 1Cer), GD3, GM1 and GD1a. Another study showed that ganglioside GM1 localizes in lipid rafts of epithelial cells and may serve as receptor for this fungus (Maza et al., 2008). Also, GM3 occurring in lung fibroblast is involved in P. brasiliensis binding and/or infection (Ywazaki et al., 2011). Removal of N-acetylneuraminic acid from human alveolar cells resulted in decreased adhesion of P. brasiliensis conidia to these cells, suggesting that sialic acids may play a significant role in pathomechanism (González et al., 2008). Moreover, disruption of lipid rafts using cholesterol-sequestering agents (methyl-β-cyclodextrin, MβCD, or nystatin) hindered P. brasiliensis interaction with the cells (Maza et al., 2008). In addition, malate synthase can mediate P. brasiliensis interaction with mammalian cells because it acts as an adhesin (da Silva Neto et al., 2009).
Pneumocystis jirovecii
Pneumocystis jirovecii is a pathogen responsible for opportunistic pneumonia in immunocompromised patients (Dellière et al., 2020) and children (Zakrzewska et al., 2021), with a relatively high mortality rate (Hahn et al., 2003). The main cell surface protein involved in host cell binding and internalization is β-glucan, a cell wall component containing 1,3-linked β-d-glucopyranosyl residues and variable amounts of 1,6-linked β-d-pyranosyl side chains, which may be recognized by LacCer in S. cerevisiae (Zimmerman et al., 1998). Hahn et al. (2003) found that blocking LacCer using a specific anti-LacCer antibody markedly decreased the release of macrophage inflammatory protein (MIP-2). Similar results were obtained using GSLs inhibitors (N-butyldeoxyno jirimycin and d-threo-1-phenyl-2-decanoylamino-3-morpholino-1-propanol-HCl), suggesting that LacCer may also play a significant role in inducing β-glucan-induced inflammatory signaling of P. jirovecii pathogenesis (Hahn et al., 2003; Souza et al., 2021).
Discussion and future perspectives
Glycosphingolipids play several important roles in cell–cell interactions and communication in complex, multicellular organisms. The role of GSLs in mammals was studied by knock-outs of specific glycosyltransferases, mostly participating in initial steps of GSL biosynthesis (Yamashita et al., 1999, 2003; Kumagai et al., 2010; Wu et al., 2011; Allende and Proia, 2014). On the single-cell level, GSLs are involved in proliferation, apoptosis, endocytosis and cell migration, and thus they play a role in cancer progression (including breast, lung, colorectal and melanoma; Zhuo et al., 2018). This makes GSLs attractive targets for anti-tumor therapies (Kroll et al., 2020), including immunotherapy (Yu et al., 2020).
Autoimmunity induced by antigen mimicry, best described in Campylobacter jejuni infections, presents a unique challenge. Autoantibody responses against GM1 destroy host peripheral nerve components, leading to acute motor axonal neuropathy (AMAN) or acute motor-sensory axonal neuropathy (an axonal form of GBS, based on the absence/presence of sensory involvement). One GBS variant, Miller Fisher syndrome, is characterized by gait ataxia, areflexia, and acute ophthalmoplegia (Lee et al., 2004; Brezovska et al., 2011; Koike and Katsuno, 2021). Molecular mechanisms driving the development of autoantibodies and host complement activation remain incompletely understood.
The importance of GSLs as receptors is linked to their localization in lipid rafts in the plasma membrane. Networks of lipid rafts and other membrane elements (mainly membrane-associated proteins) influence host-pathogen interactions by affecting pathogen or toxin sequestration and cell entry. Shiga and cholera toxins enter the host cells via GSL receptors, but successful entry and cytotoxicity depend on multiple factors, including the composition of plasma membrane, organization of lipid and protein components, clustering of GSL receptors, and actin remodeling. Membrane microdomains called lipid rafts contain a special subset of proteins (e.g., G proteins) and lipids (cholesterol, GPI-anchored proteins, and glycolipids in the outer leaflet, and unsaturated phospholipids and caveolin in the inner leaflet). Many studies suggested that entry through lipid raft components helps pathogens evade host immune responses and lysosomal fusion. Disruption of lipid rafts (e.g., depletion of cholesterol) inhibits or stimulates signaling pathways. The ceramide moiety of GSLs also impacts their receptor functions. For example, Shiga toxins bind more strongly to Gb3 with long-chain fatty acid (C20-C24) compared to shorter ones (C12-C14). Additionally, Gb3 isoforms with saturated fatty acid chains show lower affinity to Stx compared to unsaturated chains. These elements of the receptor chemistry, distribution of receptors in the cell membrane as well as the configuration and number of receptor binding sites on the ligands determine whether the toxin can induce negative membrane curvature and tubular plasma membrane intussusception necessary for cellular uptake (Römer et al., 2007, 2010; Johannes and Römer, 2010; Pezeshkian et al., 2016; Johannes and Verhelst, 2021).
The mechanisms whereby pathogens and toxins engage GSLs to thrive in the host or induce cytotoxicity are more challenging to understand than the traditional protein–protein interaction paradigm (Kulkarni et al., 2021). The multiple examples of infections or pathologies involving GSLs point to untapped potential and call for unorthodox approaches in search of new treatments.
Author contributions
AB, KM and RK wrote the manuscript. RK and MC reviewed and edited the manuscript. KM prepared the figures. All authors have read and agreed to the published version of the manuscript. All authors contributed to the article and approved the submitted version.
Funding
This research was funded by the National Science Centre of Poland, OPUS project 2018/31/B/NZ6/01828 (MC).
Conflict of interest
The authors declare that the research was conducted in the absence of any commercial or financial relationships that could be construed as a potential conflict of interest.
Publisher’s note
All claims expressed in this article are solely those of the authors and do not necessarily represent those of their affiliated organizations, or those of the publisher, the editors and the reviewers. Any product that may be evaluated in this article, or claim that may be made by its manufacturer, is not guaranteed or endorsed by the publisher.
Abbreviations
FUT, Fucosyltransferase; GalCer, Galactosylceramide; Gb3, Globotriaosylceramide; Gb4, Globotetraosylceramide; GgO3, Gangliotriaosylceramide; GgO4, Gangliotetraosylceramide; GlcCer, Glucosylceramide; GM1, Monosialotetrahexosylganglioside; HBGAs, Human Blood Group Associated Antigens; LPS, Lipopolysaccharide; SGGLs, Sulfated Glucuronyl Glycosphingolipids; SGLPG, Sulfated Glucuronyl Lactosaminylparagloboside; SGPG, Sulfated Glucuronyl Paragloboside; CTH, Ceramide Trihexoside.
References
Ahsan, N. (ed). (2006). Polyomaviruses and human diseases. New York, N.Y.: Georgetown, Tex: Springer Science+Business Media; Eurekah.com/Landes Bioscience.
Aigal, S., Claudinon, J., and Römer, W. (2015). Plasma membrane reorganization: A glycolipid gateway for microbes. Biochimica et Biophysica Acta (BBA) - Molecular Cell Research 1853, 858–871. doi: 10.1016/j.bbamcr.2014.11.014
Ali, M., Debes, A. K., Luquero, F. J., Kim, D. R., Park, J. Y., Digilio, L., et al. (2016). Potential for controlling cholera using a ring vaccination strategy: re-analysis of data from a cluster-randomized clinical trial. PLoS Med. 13:e1002120. doi: 10.1371/journal.pmed.1002120
Allende, M. L., and Proia, R. L. (2014). Simplifying complexity: genetically resculpting glycosphingolipid synthesis pathways in mice to reveal function. Glycoconj. J. 31, 613–622. doi: 10.1007/s10719-014-9563-5
Almand, E. A., Moore, M. D., and Jaykus, L.-A. (2017). Norovirus binding to ligands Beyond Histo-blood group antigens. Front. Microbiol. 8:2549. doi: 10.3389/fmicb.2017.02549
Aly, F. Z., Blackwell, C. C., MacKenzie, D. A., Weir, D. M., Elton, R. A., Cumming, C. G., et al. (1991). Chronic atrophic oral candidiasis among patients with diabetes mellitus--role of secretor status. Epidemiol. Infect. 106, 355–363. doi: 10.1017/S0950268800048500
Angström, J., Teneberg, S., Milh, M. A., Larsson, T., Leonardsson, I., Olsson, B.-M., et al. (1998). The lactosylceramide binding specificity of Helicobacter pylori. Glycobiology 8, 297–309. doi: 10.1093/glycob/8.4.297
Anstee, D. J. (2010). The relationship between blood groups and disease. Blood 115, 4635–4643. doi: 10.1182/blood-2010-01-261859
Arias, C. F., Guerrero, C. A., Méndez, E., Zárate, S., Isa, P., Espinosa, R., et al. (2008). “Early events of rotavirus infection: The search for the receptor(s)” in Novartis Foundation Symposia. eds. D. Chadwick and J. A. Goode (Chichester, UK: John Wiley & Sons, Ltd), 47–63.
Ayouni, S., Sdiri-Loulizi, K., de Rougemont, A., Estienney, M., Ambert-Balay, K., Aho, S., et al. (2015). Rotavirus P[8] infections in persons with secretor and nonsecretor phenotypes, Tunisia. Emerg. Infect. Dis. 21, 2055–2058. doi: 10.3201/eid2111.141901
Barbé, L., Le Moullac-Vaidye, B., Echasserieau, K., Bernardeau, K., Carton, T., Bovin, N., et al. (2018). Histo-blood group antigen-binding specificities of human rotaviruses are associated with gastroenteritis but not with in vitro infection. Sci. Rep. 8:12961. doi: 10.1038/s41598-018-31005-4
Barbieri, J. T., Riese, M. J., and Aktories, K. (2002). Bacterial toxins that modify the actin cytoskeleton. Annu. Rev. Cell Dev. Biol. 18, 315–344. doi: 10.1146/annurev.cellbio.18.012502.134748
Barth, H., Aktories, K., Popoff, M. R., and Stiles, B. G. (2004). Binary bacterial toxins: biochemistry, biology, and applications of common Clostridium and Bacillus proteins. Microbiol. Mol. Biol. Rev. 68, 373–402. doi: 10.1128/MMBR.68.3.373-402.2004
Barros, M. B., de Almeida Paes, R., and Schubach, A. O. (2011). Sporothrix schenckii and Sporotrichosis. Clin. Microbiol. Rev. 24, 633–654. doi: 10.1128/CMR.00007-11
Benedict, K., and Mody, R. K. (2016). Epidemiology of Histoplasmosis Outbreaks, United States, 1938–2013. Emerg. Infect. Dis. 22, 370–378. doi: 10.3201/eid2203.151117
Ben-Aryeh, H., Blumfield, E., Szargel, R., Laufer, D., and Berdicevsky, I. (1995). Oral Candida carriage and blood group antigen secretor status. Mycoses 38, 355–358. doi: 10.1111/j.1439-0507.1995.tb00064.x
Benktander, J., Ångström, J., Breimer, M. E., and Teneberg, S. (2012). Redefinition of the carbohydrate binding specificity of Helicobacter pylori BabA adhesin. J. Biol. Chem. 287, 31712–31724. doi: 10.1074/jbc.M112.387654
Bharati, K., and Ganguly, N. K. (2011). Cholera toxin: a paradigm of a multifunctional protein. Indian J. Med. Res. 133, 179–187.
Bieri, J., Leisi, R., Bircher, C., and Ros, C. (2021). Human parvovirus B19 interacts with globoside under acidic conditions as an essential step in endocytic trafficking. PLoS Pathog. 17:e1009434. doi: 10.1371/journal.ppat.1009434
Bieri, J., and Ros, C. (2019). Globoside is dispensable for parvovirus B19 entry but essential at a Postentry step for productive infection. J. Virol. 93, e00972–e00919. doi: 10.1128/JVI.00972-19
Binette, J., Dubé, M., Mercier, J., Halawani, D., Latterich, M., and Cohen, E. A. (2007). Requirements for the selective degradation of CD4 receptor molecules by the human immunodeficiency virus type 1 Vpu protein in the endoplasmic reticulum. Retrovirology 4:75. doi: 10.1186/1742-4690-4-75
Blanchard, B., Nurisso, A., Hollville, E., Tétaud, C., Wiels, J., Pokorná, M., et al. (2008). Structural basis of the preferential binding for globo-series glycosphingolipids displayed by Pseudomonas aeruginosa lectin I. J. Mol. Biol. 383, 837–853. doi: 10.1016/j.jmb.2008.08.028
Bongomin, F., Gago, S., Oladele, R. O., and Denning, D. W. (2017). Global and multi-National Prevalence of fungal diseases-estimate precision. J. Fungi. 3:E57. doi: 10.3390/jof3040057
Brassart, D., Woltz, A., Golliard, M., and Neeser, J. R. (1991). In vitro inhibition of adhesion of Candida albicans clinical isolates to human buccal epithelial cells by Fuc alpha 1,2Gal beta-bearing complex carbohydrates. Infect. Immun. 59, 1605–1613. doi: 10.1128/iai.59.5.1605-1613.1991
Brezovska, K., Panovska, A. P., Grozdanova, A., Suturkova, L., Basta, I., and Apostolski, S. (2011). Immunoreactivity of glycoproteins isolated from human peripheral nerve and Campylobacter jejuni (O: 19). J. Neurosci. Rural Pract. 2, 125–129. doi: 10.4103/0976-3147.83576
Brown, K. E., and Young, N. S. (1995). Parvovirus B19 infection and hematopoiesis. Blood Rev. 9, 176–182. doi: 10.1016/0268-960X(95)90023-3
Bruyand, M., Mariani-Kurkdjian, P., Gouali, M., de Valk, H., King, L. A., Le Hello, S., et al. (2018). Hemolytic uremic syndrome due to Shiga toxin-producing Escherichia coli infection. Médecine et Maladies Infectieuses 48, 167–174. doi: 10.1016/j.medmal.2017.09.012
Bryan, A. M., Del Poeta, M., and Luberto, C. (2015). Sphingolipids as regulators of the phagocytic response to fungal infections. Mediat. Inflamm. 2015:640540. doi: 10.1155/2015/640540
Burford-Mason, A. P., Willoughby, J. M. T., and Weber, J. C. P. (1993). Association between gastrointestinal tract carriage of Candida, blood group O, and nonsecretion of blood group antigens in patients with peptic ulcer. Dig. Dis. Sci. 38, 1453–1458. doi: 10.1007/BF01308603
Buuck, S., Smith, K., Fowler, R. C., Cebelinski, E., Lappi, V., Boxrud, D., et al. (2016–2017). Epidemiology of Enterotoxigenic Escherichia coli infection in Minnesota. Epidemiol Infect 148:e206. doi: 10.1017/S0950268820001934
Caddy, S., Breiman, A., le Pendu, J., and Goodfellow, I. (2014). Genogroup IV and VI canine noroviruses interact with histo-blood group antigens. J. Virol. 88, 10377–10391. doi: 10.1128/JVI.01008-14
Cameron, B. J., and Douglas, L. J. (1996). Blood group glycolipids as epithelial cell receptors for Candida albicans. Infect. Immun. 64, 891–896. doi: 10.1128/iai.64.3.891-896.1996
Cao, S., Lou, Z., Tan, M., Chen, Y., Liu, Y., Zhang, Z., et al. (2007). Structural basis for the recognition of blood group trisaccharides by norovirus. J. Virol. 81, 5949–5957. doi: 10.1128/JVI.00219-07
Capece, G., and Gignac, E. (2022). “Norovirus,” in StatPearls (Treasure Island (FL): StatPearls Publishing). Available at: http://www.ncbi.nlm.nih.gov/books/NBK513265/
Cawthraw, S. A., Feldman, R. A., Sayers, A. R., and Newell, D. G. (2002). Long-term antibody responses following human infection with Campylobacter jejuni. Clin. Exp. Immunol. 130, 101–106. doi: 10.1046/j.1365-2249.2002.01966.x
CDC Botulism | Epidemiological Overview for Clinicians (2019). Available at: https://www.emergency.cdc.gov/agent/botulism/clinicians/epidemiology.asp
Chan, S. C. W., Chung, H. Y., Lau, C. S., and Li, P. H. (2021). Epidemiology, mortality and effectiveness of prophylaxis for Pneumocystis jiroveci pneumonia among rheumatic patients: a territory-wide study. Ann. Clin. Microbiol. Antimicrob. 20:78. doi: 10.1186/s12941-021-00483-2
Chassaing, M., Boudaud, N., Belliot, G., Estienney, M., Majou, D., de Rougemont, A., et al. (2020). Interaction between norovirus and Histo-blood group antigens: A key to understanding virus transmission and inactivation through treatments? Food Microbiol. 92:103594. doi: 10.1016/j.fm.2020.103594
Chen, C., Baldwin, M. R., and Barbieri, J. T. (2008). Molecular basis for tetanus toxin coreceptor interactions. Biochemistry 47, 7179–7186. doi: 10.1021/bi800640y
Chen, C., Fu, Z., Kim, J.-J. P., Barbieri, J. T., and Baldwin, M. R. (2009). Gangliosides as high affinity receptors for tetanus neurotoxin. J. Biol. Chem. 284, 26569–26577. doi: 10.1074/jbc.M109.027391
Chester, M. A. (1997). Nomenclature of glycolipids (IUPAC recommendations 1997). Pure Appl. Chem. 69, 2475–2488. doi: 10.1351/pac199769122475
Critchley, I. A., and Douglas, L. J. (1987). Role of glycosides as epithelial cell receptors for Candida albicans. J. Gen. Microbiol. 133, 637–643. doi: 10.1099/00221287-133-3-637
Cserti, C. M., and Dzik, W. H. (2007). The ABO blood group system and plasmodium falciparum malaria. Blood 110, 2250–2258. doi: 10.1182/blood-2007-03-077602
D’Angelo, G., Capasso, S., Sticco, L., and Russo, D. (2013). Glycosphingolipids: synthesis and functions. FEBS J. 280, 6338–6353. doi: 10.1111/febs.12559
da Silva Neto, B. R., De Fátima Silva, J., Mendes-Giannini, M. J. S., Lenzi, H. L., de Almeida Soares, C. M., and Pereira, M. (2009). The malate synthase of Paracoccidioides brasiliensis is a linked surface protein that behaves as an anchorless adhesin. BMC Microbiol. 9:272. doi: 10.1186/1471-2180-9-272
Damm, E.-M., Pelkmans, L., Kartenbeck, J., Mezzacasa, A., Kurzchalia, T., and Helenius, A. (2005). Clathrin-and caveolin-1-independent endocytosis: entry of simian virus 40 into cells devoid of caveolae. J. Cell Biol. 168, 477–488. doi: 10.1083/jcb.200407113
Deen, J., Mengel, M. A., and Clemens, J. D. (2020). Epidemiology of cholera. Vaccine 38, A31–A40. doi: 10.1016/j.vaccine.2019.07.078
Dellière, S., Gits-Muselli, M., Bretagne, S., and Alanio, A. (2020). Outbreak-causing Fungi: pneumocystis jirovecii. Mycopathologia 185, 783–800. doi: 10.1007/s11046-019-00408-w
Delorme, C., Brüssow, H., Sidoti, J., Roche, N., Karlsson, K. A., Neeser, J. R., et al. (2001). Glycosphingolipid binding specificities of rotavirus: identification of a sialic acid-binding epitope. J. Virol. 75, 2276–2287. doi: 10.1128/JVI.75.5.2276-2287.2001
Detzner, J., Klein, A.-L., Pohlentz, G., Krojnewski, E., Humpf, H.-U., Mellmann, A., et al. (2021). Primary human renal proximal tubular epithelial cells (pHRPTEpiCs): Shiga toxin (Stx) glycosphingolipid receptors, Stx Susceptibility, and Interaction with Membrane Microdomains. Toxins 13:529. doi: 10.3390/toxins13080529
Díaz, P., Valenzuela Valderrama, M., Bravo, J., and Quest, A. F. G. (2018). Helicobacter pylori and Gastric Cancer: Adaptive Cellular Mechanisms Involved in Disease Progression. Front. Microbiol. 9:5. doi: 10.3389/fmicb.2018.00005
Domino, S. E., Hurd, E. A., Thomsson, K. A., Karnak, D. M., Holmén Larsson, J. M., Thomsson, E., et al. (2009). Cervical mucins carry alpha(1,2)fucosylated glycans that partly protect from experimental vaginal candidiasis. Glycoconj. J. 26, 1125–1134. doi: 10.1007/s10719-009-9234-0
Duncan, M. J., Shin, J.-S., and Abraham, S. N. (2002). Microbial entry through caveolae: variations on a theme. Cell. Microbiol. 4, 783–791. doi: 10.1046/j.1462-5822.2002.00230.x
Eierhoff, T., Bastian, B., Thuenauer, R., Madl, J., Audfray, A., Aigal, S., et al. (2014). A lipid zipper triggers bacterial invasion. Proc. Natl. Acad. Sci. U. S. A. 111, 12895–12900. doi: 10.1073/pnas.1402637111
El-Hawiet, A., Kitova, E. N., and Klassen, J. S. (2015). Glycan Recognition Recognition of human milk oligosaccharides by bacterial exotoxins. Glycobiology 25, 845–854. doi: 10.1093/glycob/cwv025
Epidemiology of Helicobacter pylori - Sonnenberg (2022). Alimentary Pharmacology & Therapeutics-Wiley Online Library. doi: 10.1111/apt.16592
Erickson, K. D., Garcea, R. L., and Tsai, B. (2009). Ganglioside GT1b is a putative host cell receptor for the Merkel cell polyomavirus. J. Virol. 83, 10275–10279. doi: 10.1128/JVI.00949-09
Fan, E., Merritt, E. A., Verlinde, C. L. M. J., and Hol, W. G. J. (2000). AB(5) toxins: structures and inhibitor design. Current opinion in structural biology 10, 680–686. doi: 10.1016/S0959-440X(00)00152-4
Fatima, R., and Aziz, M. (2022). “Enterohemorrhagic Escherichia Coli,” in StatPearls (Treasure Island (FL): StatPearls Publishing). Available at: http://www.ncbi.nlm.nih.gov/books/NBK519509/
Fernández-Moreno, R., Torre-Cisneros, J., and Cantisán, S. (2021). Human cytomegalovirus (HCMV)-encoded microRNAs: potential biomarkers and clinical applications. RNA Biol. 18, 2194–2202. doi: 10.1080/15476286.2021.1930757
Fidel, P. L., Cutright, J., and Steele, C. (2000). Effects of reproductive hormones on experimental vaginal candidiasis. Infect. Immun. 68, 651–657. doi: 10.1128/IAI.68.2.651-657.2000
Florek, M., Nawrot, U., Korzeniowska-Kowal, A., Włodarczyk, K., Wzorek, A., Woźniak-Biel, A., et al. (2021). An analysis of the population of Cryptococcus neoformans strains isolated from animals in Poland, in the years 2015-2019. Sci. Rep. 11:6639. doi: 10.1038/s41598-021-86169-3
Foster, G. H., Armstrong, C. S., Sakiri, R., and Tesh, V. L. (2000). Shiga toxin-induced tumor necrosis factor alpha expression: requirement for toxin enzymatic activity and monocyte protein kinase C and protein tyrosine kinases. Infect. Immun. 68, 5183–5189. doi: 10.1128/IAI.68.9.5183-5189.2000
Frankel, A. D., and Young, J. A. (1998). HIV-1: fifteen proteins and an RNA. Annu. Rev. Biochem. 67, 1–25. doi: 10.1146/annurev.biochem.67.1.1
Fu, Y., Luo, Y., and Grinspan, A. M. (2021). Epidemiology of community-acquired and recurrent Clostridioides difficile infection. Therap. Adv. Gastroenterol 14:17562848211016248. doi: 10.1177/17562848211016248
Gallegos, K. M., Conrady, D. G., Karve, S. S., Gunasekera, T. S., Herr, A. B., and Weiss, A. A. (2012). Shiga toxin binding to glycolipids and glycans. PLoS One 7:e30368. doi: 10.1371/journal.pone.0030368
Gardner, S. D., Field, A. M., Coleman, D. V., and Hulme, B. (1971). New human papovavirus (B.K.) isolated from urine after renal transplantation. Lancet 1, 1253–1257. doi: 10.1016/s0140-6736(71)91776-4
Gault, C. R., Obeid, L. M., and Hannun, Y. A. (2010). “An overview of sphingolipid metabolism: from synthesis to breakdown,” in Sphingolipids as Signaling and Regulatory Molecules (United States: Springer)
Gaynor, A. M., Nissen, M. D., Whiley, D. M., Mackay, I. M., Lambert, S. B., Wu, G., et al. (2007). Identification of a novel polyomavirus from patients with acute respiratory tract infections. PLoS Pathog. 3:e64. doi: 10.1371/journal.ppat.0030064
Giannouli, M., Antunes, L. C. S., Marchetti, V., Triassi, M., Visca, P., and Zarrilli, R. (2013). Virulence-related traits of epidemic Acinetobacter baumannii strains belonging to the international clonal lineages I-III and to the emerging genotypes ST25 and ST78. BMC Infect. Dis. 13:282. doi: 10.1186/1471-2334-13-282
Giussani, P., Tringali, C., Riboni, L., Viani, P., and Venerando, B. (2014). Sphingolipids: key regulators of apoptosis and pivotal players in cancer drug resistance. Int. J. Mol. Sci. 15, 4356–4392. doi: 10.3390/ijms15034356
Gnat, S., Łagowski, D., Nowakiewicz, A., and Dyląg, M. (2021). A global view on fungal infections in humans and animals: infections caused by dimorphic fungi and dermatophytoses. J. Appl. Microbiol. 131, 2688–2704. doi: 10.1111/jam.15084
González, A., Gómez, B. L., Diez, S., Hernández, O., Restrepo, A., Hamilton, A. J., et al. (2005). Purification and partial characterization of a Paracoccidioides brasiliensis protein with capacity to bind to extracellular matrix proteins. Infect Immun. 73, 2486–2495. doi: 10.1128/IAI.73.4.2486-2495.2005
González, A., Caro, E., Muñoz, C., Restrepo, A., Hamilton, A. J., and Cano, L. E. (2008). Paracoccidioides brasiliensis conidia recognize fibronectin and fibrinogen which subsequently participate in adherence to human type II alveolar cells: involvement of a specific adhesin. Microb. Pathog. 44, 389–401. doi: 10.1016/j.micpath.2007.11.001
Gow, N. A. R., van de Veerdonk, F. L., Brown, A. J. P., and Netea, M. G. (2011). Candida albicans morphogenesis and host defence: discriminating invasion from colonization. Nat. Rev. Microbiol. 10, 112–122. doi: 10.1038/nrmicro2711
Guimarães, A. J., de Cerqueira, M. D., Zamith-Miranda, D., Lopez, P. H., Rodrigues, M. L., Pontes, B., et al. (2019). Host membrane glycosphingolipids and lipid microdomains facilitate Histoplasma capsulatum internalisation by macrophages. Cell. Microbiol. 21:e12976. doi: 10.1111/cmi.12976
Haga, K., Fujimoto, A., Takai-Todaka, R., Miki, M., Doan, Y. H., Murakami, K., et al. (2016). Functional receptor molecules CD300lf and CD300ld within the CD300 family enable murine noroviruses to infect cells. Proc. Natl. Acad. Sci. U. S. A. 113, E6248–E6255. doi: 10.1073/pnas.1605575113
Hahn, P. Y., Evans, S. E., Kottom, T. J., Standing, J. E., Pagano, R. E., and Limper, A. H. (2003). Pneumocystis carinii cell wall beta-glucan induces release of macrophage inflammatory protein-2 from alveolar epithelial cells via a lactosylceramide-mediated mechanism. J. Biol. Chem. 278, 2043–2050. doi: 10.1074/jbc.M209715200
Hani, U., Shivakumar, H. G., Vaghela, R., Osmani, R. A. M., and Shrivastava, A. (2015). Candidiasis: a fungal infection--current challenges and progress in prevention and treatment. Infect. Disord. Drug Targets 15, 42–52. doi: 10.2174/1871526515666150320162036
Haselhorst, T., Fleming, F. E., Dyason, J. C., Hartnell, R. D., Yu, X., Holloway, G., et al. (2009). Sialic acid dependence in rotavirus host cell invasion. Nat. Chem. Biol. 5, 91–93. doi: 10.1038/nchembio.134
Heim, J. B., Hodnik, V., Heggelund, J. E., Anderluh, G., and Krengel, U. (2019). Crystal structures of cholera toxin in complex with fucosylated receptors point to importance of secondary binding site. Sci. Rep. 9:12243. doi: 10.1038/s41598-019-48579-2
Hilton, E., Chandrasekaran, V., Rindos, P., and Isenberg, H. D. (1995). Association of recurrent candidal vaginitis with inheritance of Lewis blood group antigens. J. Infect. Dis. 172, 1616–1619. doi: 10.1093/infdis/172.6.1616
HIV/AIDS (2021). Available at: https://www.who.int/news-room/fact-sheets/detail/hiv-aids
Holmgren, J., Lönnroth, I., Månsson, J., and Svennerholm, L. (1975). Interaction of cholera toxin and membrane GM1 ganglioside of small intestine. Proc. Natl. Acad. Sci. U. S. A. 72, 2520–2524. doi: 10.1073/pnas.72.7.2520
Huang, P., Farkas, T., Marionneau, S., Zhong, W., Ruvoën-Clouet, N., Morrow, A. L., et al. (2003). Noroviruses bind to human ABO, Lewis, and secretor Histo-blood group antigens: identification of 4 distinct strain-specific patterns. J. Infect. Dis. 188, 19–31. doi: 10.1086/375742
Hurd, E. A., and Domino, S. E. (2004). Increased susceptibility of secretor factor gene Fut2-null mice to experimental vaginal candidiasis. Infect. Immun. 72, 4279–4281. doi: 10.1128/IAI.72.7.4279-4281.2004
Hurdiss, D. L., Frank, M., Snowden, J. S., Macdonald, A., and Ranson, N. A. (2018). The Structure of an Infectious Human Polyomavirus and Its Interactions with Cellular Receptors. Structure 26, 839–847.e3. doi: 10.1016/j.str.2018.03.019
Hutson, A. M., Atmar, R. L., Marcus, D. M., and Estes, M. K. (2003). Norwalk virus-Like particle Hemagglutination by binding to H Histo-blood group antigens. J. Virol. 77, 405–415. doi: 10.1128/JVI.77.1.405-415.2003
Ishibashi, Y., Nagamatsu, Y., Meyer, S., Imamura, A., Ishida, H., Kiso, M., et al. (2009). Transglycosylation-based fluorescent labeling of 6-gala series glycolipids by endogalactosylceramidase. Glycobiology 19, 797–807. doi: 10.1093/glycob/cwp051
Jansson, L., Tobias, J., Lebens, M., Svennerholm, A.-M., and Teneberg, S. (2006). The major subunit, CfaB, of colonization factor antigen i from enterotoxigenic Escherichia coli is a glycosphingolipid binding protein. Infect. Immun. 74, 3488–3497. doi: 10.1128/IAI.02006-05
Jimenez-Lucho, V., Ginsburg, V., and Krivan, H. C. (1990). Cryptococcus neoformans, Candida albicans, and other fungi bind specifically to the glycosphingolipid lactosylceramide (Gal beta 1-4Glc beta 1-1Cer), a possible adhesion receptor for yeasts. Infect. Immun. 58, 2085–2090. doi: 10.1128/iai.58.7.2085-2090.1990
Jin, C., Barone, A., Borén, T., and Teneberg, S. (2018). Helicobacter pylori–binding nonacid glycosphingolipids in the human stomach. J. Biol. Chem. 293, 17248–17266. doi: 10.1074/jbc.RA118.004854
Johannes, L., and Römer, W. (2010). Shiga toxins from cell biology to biomedical applications. Nat. Rev. Microbiol. 8, 105–116. doi: 10.1038/nrmicro2279
Johannes, L., and Verhelst, S. (2021). The cellular and chemical biology of endocytic trafficking and intracellular delivery-The GL-Lect hypothesis. Molecules 26:3299. doi: 10.3390/molecules26113299
Johansson, I., Bratt, P., Hay, D. I., Schluckebier, S., and Strömberg, N. (2000). Adhesion of Candida albicans, but not Candida krusei, to salivary statherin and mimicking host molecules. Oral Microbiol. Immunol. 15, 112–118. doi: 10.1034/j.1399-302x.2000.150207.x
Jong, A., Wu, C. H., Chen, H. M., Luo, F., Kwon-Chung, K. J., Chang, Y. C., et al. (2007). Identification and characterization of CPS1 as a hyaluronic acid synthase contributing to the pathogenesis of Cryptococcus neoformans infection. Eukaryot Cell. 6, 1486–1496. doi: 10.1128/EC.00120-07
Jong, A., Wu, C. H., Gonzales-Gomez, I., Kwon-Chung, K. J., Chang, Y. C., Tseng, H. K., et al. (2012). Hyaluronic acid receptor CD44 deficiency is associated with decreased Cryptococcus neoformans brain infection. J. Biol. Chem. 287, 15298–15306. doi: 10.1074/jbc.M112.353375
Kaczmarek, R., Buczkowska, A., Mikołajewicz, K., Krotkiewski, H., and Czerwinski, M. (2014). P1PK, GLOB, and FORS blood group systems and GLOB collection: biochemical and clinical aspects. Do we understand it all yet? Transfus. Med. Rev. 28, 126–136. doi: 10.1016/j.tmrv.2014.04.007
Khan, A. S., Kniep, B., Oelschlaeger, T. A., Van Die, I., Korhonen, T., and Hacker, J. (2000a). Receptor structure for F1C fimbriae of Uropathogenic Escherichia coli. Infect. Immun. 68, 3541–3547. doi: 10.1128/IAI.68.6.3541-3547.2000
Khan, A. S., Mühldorfer, I., Demuth, V., Wallner, U., Korhonen, T. K., and Hacker, J. (2000b). Functional analysis of the minor subunits of S fimbrial adhesin (SfaI) in pathogenic Escherichia coli. Mol. Gen. Genet. 263, 96–105. doi: 10.1007/PL00008680
Kim, D.-S., Hosmillo, M., Alfajaro, M. M., Kim, J.-Y., Park, J.-G., Son, K.-Y., et al. (2014). Both α2,3-and α2,6-linked sialic acids on O-linked glycoproteins act as functional receptors for porcine Sapovirus. PLoS Pathog. 10:e1004172. doi: 10.1371/journal.ppat.1004172
Kim, J.-Y., Kim, D.-S., Seo, J.-Y., Park, J.-G., Alfajaro, M. M., Soliman, M., et al. (2017). Glycan-specificity of four neuraminidase-sensitive animal rotavirus strains. Vet. Microbiol. 207, 159–163. doi: 10.1016/j.vetmic.2017.06.016
Koike, C., Fung, J. J., Geller, D. A., Kannagi, R., Libert, T., Luppi, P., et al. (2002). Molecular basis of evolutionary loss of the alpha 1,3-galactosyltransferase gene in higher primates. J. Biol. Chem. 277, 10114–10120. doi: 10.1074/jbc.M110527200
Koike, H., and Katsuno, M. (2021). Emerging infectious diseases, vaccines and Guillain–Barré syndrome. Clin. Exp. Neuroimmunol. 12, 165–170. doi: 10.1111/cen3.12644
Kolbrink, B., Scheikholeslami-Sabzewari, J., Borzikowsky, C., von Samson-Himmelstjerna, F. A., Ullmann, A. J., Kunzendorf, U., et al. (2022). Evolving epidemiology of pneumocystis pneumonia: Findings from a longitudinal population-based study and a retrospective multi-center study in Germany. Lancet Reg. Health Eur. 18:100400. doi: 10.1016/j.lanepe.2022.100400
Koromyslova, A., Tripathi, S., Morozov, V., Schroten, H., and Hansman, G. S. (2017). Human norovirus inhibition by a human milk oligosaccharide. Virology 508, 81–89. doi: 10.1016/j.virol.2017.04.032
Kościelak, J. (2012). The hypothesis on function of glycosphingolipids and ABO blood groups revisited. Neurochem. Res. 37, 1170–1184. doi: 10.1007/s11064-012-0734-0
Kroll, A., Cho, H. E., and Kang, M. H. (2020). Antineoplastic agents targeting sphingolipid pathways. Front. Oncol. 10:833. doi: 10.3389/fonc.2020.00833
Kuhaudomlarp, S., Gillon, E., Varrot, A., and Imberty, A. (2020). LecA (PA-IL): A galactose-binding lectin from Pseudomonas aeruginosa. Methods Mol. Biol. 2132, 257–266. doi: 10.1007/978-1-0716-0430-4_25
Kulkarni, D. G., and Venkatesh, D. (2004). Non-secretor status; a predisposing factor for vaginal candidiasis. Indian J. Physiol. Pharmacol. 48, 225–229.
Kulkarni, R., Wiemer, E. A. C., and Chang, W. (2021). Role of lipid rafts in pathogen-host interaction - A Mini review. Front. Immunol. 12:815020. doi: 10.3389/fimmu.2021.815020
Kumagai, T., Sato, T., Natsuka, S., Kobayashi, Y., Zhou, D., Shinkai, T., et al. (2010). Involvement of murine β-1,4-galactosyltransferase V in lactosylceramide biosynthesis. Glycoconj. J. 27, 685–695. doi: 10.1007/s10719-010-9313-2
Ladisch, S., Hasegawa, A., Li, R., and Kiso, M. (1995). Immunosuppressive activity of chemically synthesized gangliosides. Biochemistry 34, 1197–1202. doi: 10.1021/bi00004a012
Lamey, P.-J., Darwaza, A., Fisher, B. M., Samaranayake, L. P., Macfarlane, T. W., and Frier, B. M. (1988). Secretor status, candidal carriage and candidal infection in patients with diabetes mellitus. J. Oral Pathol. Med. 17, 354–357. doi: 10.1111/j.1600-0714.1988.tb01549.x
Lass-Flörl, C., Samardzic, E., and Knoll, M. (2021). Serology anno 2021-fungal infections: from invasive to chronic. Clin. Microbiol. Infect. 27, 1230–1241. doi: 10.1016/j.cmi.2021.02.005
Lee, G., Jeong, Y., Wirguin, I., Hays, A. P., Willison, H. J., and Latov, N. (2004). Induction of human IgM and IgG anti-GM1 antibodies in transgenic mice in response to lipopolysaccharides from Campylobacter jejuni. J. Neuroimmunol. 146, 63–75. doi: 10.1016/j.jneuroim.2003.10.045
Li, W., Liu, L., Tao, R., Zheng, X., Li, H., and Shang, S. (2020). Epidemiological characteristics of human cytomegalovirus infection and glycoprotein H genotype in Chinese children. Pediatrics & Neonatology 61, 63–67. doi: 10.1016/j.pedneo.2019.06.010
Liao, Z., Cimakasky, L. M., Hampton, R., Nguyen, D. H., and Hildreth, J. E. (2001). Lipid rafts and HIV pathogenesis: host membrane cholesterol is required for infection by HIV type 1. AIDS Res. Hum. Retrovir. 17, 1009–1019. doi: 10.1089/088922201300343690
Lin, J.-S., Huang, J.-H., Hung, L.-Y., Wu, S.-Y., and Wu-Hsieh, B. A. (2010). Distinct roles of complement receptor 3, Dectin-1, and sialic acids in murine macrophage interaction with Histoplasma yeast. J. Leukoc. Biol. 88, 95–106. doi: 10.1189/jlb.1109717
Lingwood, C. A. (1999). Glycolipid receptors for verotoxin and Helicobacter pylori: role in pathology. Biochim. Biophys. Acta (BBA) - Mol. Basis Dis. 1455, 375–386. doi: 10.1016/S0925-4439(99)00062-9
Lingwood, C. A. (2011). Glycosphingolipid functions. Cold Spring Harb. Perspect. Biol. 3:a004788. doi: 10.1101/cshperspect.a004788
Logan, L. K., Gandra, S., Trett, A., Weinstein, R. A., and Laxminarayan, R. (2018). Acinetobacter baumannii Resistance Trends in Children in the United States, 1999–2012. J. Pediatric Infect Dis. Soc. 8, 136–142. doi: 10.1093/jpids/piy018
Lund, N., Branch, D. R., Mylvaganam, M., Chark, D., Ma, X.-Z., Sakac, D., et al. (2006). A novel soluble mimic of the glycolipid, globotriaosyl ceramide inhibits HIV infection. AIDS 20, 333–343. doi: 10.1097/01.aids.0000206499.78664.58
Lund, N., Olsson, M. L., Ramkumar, S., Sakac, D., Yahalom, V., Levene, C., et al. (2009). The human P(k) histo-blood group antigen provides protection against HIV-1 infection. Blood 113, 4980–4991. doi: 10.1182/blood-2008-03-143396
Macri, A., and Crane, J. S. (2022). “Parvoviruses,” in StatPearls (Treasure Island (FL): StatPearls Publishing). Available at: http://www.ncbi.nlm.nih.gov/books/NBK482245/
Madar Johansson, M., Azzouz, M., Häggendal, B., Säljö, K., Malmi, H., Zavialov, A., et al. (2020). Glycosphingolipids recognized by Acinetobacter baumannii. Microorganisms 8:612. doi: 10.3390/microorganisms8040612
Maginnis, M. S., Nelson, C. D. S., and Atwood, W. J. (2015). JC polyomavirus attachment, entry, and trafficking: unlocking the keys to a fatal infection. J. Neuro-Oncol. 21, 601–613. doi: 10.1007/s13365-014-0272-4
Majowicz, S. E., Scallan, E., Jones-Bitton, A., Sargeant, J. M., Stapleton, J., Angulo, F. J., et al. (2014). Global incidence of human Shiga toxin–producing Escherichia coli infections and deaths: A systematic review and knowledge synthesis. Foodborne Pathog. Dis. doi: 10.1089/fpd.2013.1704
May, S. J., Blackwell, C. C., and Weir, D. M. (1989). Lewis a blood group antigen of non-secretors: a receptor for candida blastospores. FEMS Microbiol. Immunol. 1, 407–409. doi: 10.1111/j.1574-6968.1989.tb02429.x
May, R. C., Stone, N. R. H., Wiesner, D. L., Bicanic, T., and Nielsen, K. (2016). Cryptococcus: from environmental saprophyte to global pathogen. Nat. Rev. Microbiol. 14, 106–117. doi: 10.1038/nrmicro.2015.6
Maza, P. K., Straus, A. H., Toledo, M. S., Takahashi, H. K., and Suzuki, E. (2008). Interaction of epithelial cell membrane rafts with Paracoccidioides brasiliensis leads to fungal adhesion and Src-family kinase activation. Microbes Infect. 10, 540–547. doi: 10.1016/j.micinf.2008.02.004
Merrill, A. H., Wang, M. D., Park, M., and Sullards, M. C. (2007). (Glyco)sphingolipidology: an amazing challenge and opportunity for systems biology. Trends Biochem. Sci. 32, 457–468. doi: 10.1016/j.tibs.2007.09.004
Miyauchi, M., Giummelly, P., Yazawa, S., and Okawa, Y. (2007). Adhesion of Candida albicans to HeLa cells: studies using polystyrene beads. Biol. Pharm. Bull. 30, 588–590. doi: 10.1248/bpb.30.588
Mocchetti, I. (2005). Exogenous gangliosides, neuronal plasticity and repair, and the neurotrophins. Cell. Mol. Life Sci. 62, 2283–2294. doi: 10.1007/s00018-005-5188-y
Montecucco, C. (1986). How do tetanus and botulinum toxins bind to neuronal membranes? Trends Biochem. Sci. 11, 314–317. doi: 10.1016/0968-0004(86)90282-3
Moonens, K., and Remaut, H. (2017). Evolution and structural dynamics of bacterial glycan binding adhesins. Curr. Opin. Struct. Biol. 44, 48–58. doi: 10.1016/j.sbi.2016.12.003
Mukherjee, A., and Chawla-Sarkar, M. (2011). Rotavirus infection: A perspective on epidemiology, genomic diversity and vaccine strategies. Indian J. Virol. 22, 11–23. doi: 10.1007/s13337-011-0039-y
Mulvey, M. A., Lopez-Boado, Y. S., Wilson, C. L., Roth, R., Parks, W. C., Heuser, J., et al. (1998). Induction and evasion of host defenses by type 1-piliated uropathogenic Escherichia coli. Science 282, 1494–1497. doi: 10.1126/science.282.5393.1494
Munakata, Y., Saito-Ito, T., Kumura-Ishii, K., Huang, J., Kodera, T., Ishii, T., et al. (2005). Ku80 autoantigen as a cellular coreceptor for human parvovirus B19 infection. Blood 106, 3449–3456. doi: 10.1182/blood-2005-02-0536
Nakayama, H., Ogawa, H., Takamori, K., and Iwabuchi, K. (2013). GSL-enriched membrane microdomains in innate immune responses. Arch. Immunol. Ther. Exp. 61, 217–228. doi: 10.1007/s00005-013-0221-6
Nasir, W., Frank, M., Koppisetty, C. A. K., Larson, G., and Nyholm, P.-G. (2012). Lewis histo-blood group α1,3/α1,4 fucose residues may both mediate binding to GII.4 noroviruses. Glycobiology 22, 1163–1172. doi: 10.1093/glycob/cws084
Neu, U., Stehle, T., and Atwood, W. J. (2009). The Polyomaviridae: contributions of virus structure to our understanding of virus receptors and infectious entry. Virology 384, 389–399. doi: 10.1016/j.virol.2008.12.021
Neu, U., Woellner, K., Gauglitz, G., and Stehle, T. (2008). Structural basis of GM1 ganglioside recognition by simian virus 40. Proc. Natl. Acad. Sci. U. S. A. 105, 5219–5224. doi: 10.1073/pnas.0710301105
Norja, P., Lassila, R., and Makris, M. (2012). Parvovirus transmission by blood products - a cause for concern? British journal of haematology 159, 385–393. doi: 10.1111/BJH.12060
Norovirus Worldwide | CDC (2022). Available at: https://www.cdc.gov/norovirus/trends-outbreaks/worldwide.html
O’Hara, S. D., Stehle, T., and Garcea, R. (2014). Glycan receptors of the Polyomaviridae: structure, function, and pathogenesis. Curr. Opin. Virol. 7, 73–78. doi: 10.1016/j.coviro.2014.05.004
Ogawa-Goto, K., Arao, Y., Ito, Y., Ogawa, T., Abe, T., Kurata, T., et al. (1998). Binding of human cytomegalovirus to sulfated glucuronyl glycosphingolipids and their inhibitory effects on the infection. J. Gen. Virol. 79, 2533–2541. doi: 10.1099/0022-1317-79-10-2533
Okuda, T., Tokuda, N., Numata, S. I., Ito, M., Ohta, M., Kawamura, K., et al. (2006). Targeted disruption of Gb3/CD77 synthase gene resulted in the complete deletion of globo-series glycosphingolipids and loss of sensitivity to verotoxins. JBC. doi: 10.1074/jbc.M600057200
Orczyk-Pawiłowicz, M., and Lis-Kuberka, J. (2020). The impact of dietary Fucosylated oligosaccharides and glycoproteins of human Milk on infant well-being. Nutrients 12:1105. doi: 10.3390/nu12041105
Orrell, K. E., Zhang, Z., Sugiman-Marangos, S. N., and Melnyk, R. A. (2017). Clostridium difficile toxins A and B: receptors, pores, and translocation into cells. Crit. Rev. Biochem. Mol. Biol. 52, 461–473. doi: 10.1080/10409238.2017.1325831
Ossiboff, R. J., Sheh, A., Shotton, J., Pesavento, P. A., and Parker, J. S. L. (2007). Feline caliciviruses (FCVs) isolated from cats with virulent systemic disease possess in vitro phenotypes distinct from those of other FCV isolates. J. Gen. Virol. 88, 506–517. doi: 10.1099/vir.0.82488-0
Padgett, B. L., Walker, D. L., ZuRhein, G. M., Eckroade, R. J., and Dessel, B. H. (1971). Cultivation of papova-like virus from human brain with progressive multifocal leucoencephalopathy. Lancet 1, 1257–1260. doi: 10.1016/s0140-6736(71)91777-6
Pappas, P. G., Tellez, I., Deep, A. E., Nolasco, D., Holgado, W., and Bustamante, B. (2000). Sporotrichosis in Peru: description of an area of hyperendemicity. Clin. Infect. Dis. 30, 65–70. doi: 10.1086/313607
Paton, A. W., Srimanote, P., Talbot, U. M., Wang, H., and Paton, J. C. (2004). A new family of potent AB 5 Cytotoxins produced by Shiga toxigenic Escherichia coli. J. Exp. Med. J. Exp. Med. 200, 35–46. doi: 10.1084/jem.20040392
Patry, R. T., Stahl, M., Perez-Munoz, M. E., Nothaft, H., Wenzel, C. Q., Sacher, J. C., et al. (2019). Bacterial AB5 toxins inhibit the growth of gut bacteria by targeting ganglioside-like glycoconjugates. Nat. Commun. 10:1390. doi: 10.1038/s41467-019-09362-z
Pelkmans, L., Kartenbeck, J., and Helenius, A. (2001). Caveolar endocytosis of simian virus 40 reveals a new two-step vesicular-transport pathway to the ER. Nat. Cell Biol. 3, 473–483. doi: 10.1038/35074539
Pezeshkian, W., Gao, H., Arumugam, S., Becken, U., Bassereau, P., Florent, J.-C., et al. (2016). Mechanism of Shiga Toxin Clustering on Membranes. ACS Nano 11, 314–324. doi: 10.1021/acsnano.6b05706
Pinkbook: Tetanus | CDC (2021). Available at: https://www.cdc.gov/vaccines/pubs/pinkbook/tetanus.html
Prendergast, M. M., Tribble, D. R., Baqar, S., Scott, D. A., Ferris, J. A., Walker, R. I., et al. (2004). In vivo phase variation and serologic response to Lipooligosaccharide of Campylobacter jejuni in experimental human infection. Infect. Immun. 72, 916–922. doi: 10.1128/IAI.72.2.916-922.2004
Puryear, W. B., and Gummuluru, S. (2012). “Role of glycosphingolipids in dendritic cell-mediated HIV-1 trans-infection” in HIV interactions with dendritic cells advances in experimental medicine and biology. eds. L. Wu and O. Schwartz (New York, NY: Springer), 131–153.
Radhakrishnan, A., Anderson, T. G., and McConnell, H. M. (2000). Condensed complexes, rafts, and the chemical activity of cholesterol in membranes. Proc. Natl. Acad. Sci. U. S. A. 97, 12422–12427. doi: 10.1073/pnas.220418097
Ramamurthy, T., Mutreja, A., Weill, F.-X., Das, B., Ghosh, A., and Nair, G. B. (2019). Revisiting the Global Epidemiology of Cholera in Conjunction With the Genomics of Vibrio cholerae. Frontiers in Public Health 7. doi: 10.3389/fpubh.2019.00203
Rawat, S. S., Johnson, B. T., and Puri, A. (2005). Sphingolipids: Modulators of HIV-1 Infection and Pathogenesis. Biosci. Rep. 25, 329–343. doi: 10.1007/s10540-005-2894-5
Reiter, D. M., Frierson, J. M., Halvorson, E. E., Kobayashi, T., Dermody, T. S., and Stehle, T. (2011). Crystal structure of reovirus attachment protein σ1 in complex with sialylated oligosaccharides. PLoS Pathog. 7:e1002166. doi: 10.1371/journal.ppat.1002166
Reynolds, D., and Kollef, M. (2021). The Epidemiology and Pathogenesis and Treatment of Pseudomonas aeruginosa Infections: An Update. Drugs 81, 2117–2131. doi: 10.1007/s40265-021-01635-6
Roche, N., Ilver, D., Ångström, J., Barone, S., Telford, J. L., and Teneberg, S. (2007). Human gastric glycosphingolipids recognized by Helicobacter pylori vacuolating cytotoxin VacA. Microbes Infect. 9, 605–614. doi: 10.1016/j.micinf.2007.01.023
Rodríguez-Díaz, J., García-Mantrana, I., Vila-Vicent, S., Gozalbo-Rovira, R., Buesa, J., Monedero, V., et al. (2017). Relevance of secretor status genotype and microbiota composition in susceptibility to rotavirus and norovirus infections in humans. Sci. Rep. 7:45559. doi: 10.1038/srep45559
Römer, W., Berland, L., Chambon, V., Gaus, K., Windschiegl, B., Tenza, D., et al. (2007). Shiga toxin induces tubular membrane invaginations for its uptake into cells. Nature 450, 670–675. doi: 10.1038/nature05996
Römer, W., Pontani, L.-L., Sorre, B., Rentero, C., Berland, L., Chambon, V., et al. (2010). Actin dynamics drive membrane reorganization and scission in clathrin-independent endocytosis. Cell 140, 540–553. doi: 10.1016/j.cell.2010.01.010
Roussel, C., Cordonnier, C., Livrelli, V., Wiele, T. V.de, and Blanquet-Diot, S. (2017). “Enterotoxigenic and Enterohemorrhagic Escherichia coli: survival and modulation of virulence in the human gastrointestinal tract,” in Escherichia coli - recent advances on physiology, pathogenesis and biotechnological applications, ed. A. Samie (London: InTech).
Rummel, A., Bade, S., Alves, J., Bigalke, H., and Binz, T. (2003). Two carbohydrate binding sites in the H(CC)-domain of tetanus neurotoxin are required for toxicity. J. Mol. Biol. 326, 835–847. doi: 10.1016/S0022-2836(02)01403-1
Sato, T., Iwabuchi, K., Nagaoka, I., Adachi, Y., Ohno, N., Tamura, H., et al. (2006). Induction of human neutrophil chemotaxis by Candida albicans-derived beta-1,6-long glycoside side-chain-branched beta-glucan. J. Leukoc. Biol. 80, 204–211. doi: 10.1189/jlb.0106069
Schwan, C., Nölke, T., Kruppke, A. S., Schubert, D. M., Lang, A. E., and Aktories, K. (2011). Cholesterol-and sphingolipid-rich microdomains are essential for microtubule-based membrane protrusions induced by Clostridium difficile transferase (CDT). J. Biol. Chem. 286, 29356–29365. doi: 10.1074/jbc.M111.261925
Settembre, E. C., Chen, J. Z., Dormitzer, P. R., Grigorieff, N., and Harrison, S. C. (2011). Atomic model of an infectious rotavirus particle. EMBO J. 30, 408–16. doi: 10.1038/emboj.2010.322
Sheikh, K. A., Nachamkin, I., Ho, T. W., Willison, H. J., Veitch, J., Ung, H., et al. (1998). Campylobacter jejuni lipopolysaccharides in Guillain-Barré syndrome: molecular mimicry and host susceptibility. Neurology 51, 371–378. doi: 10.1212/WNL.51.2.371
Silva, C. J., Brandon, D. L., Skinner, C. B., and He, X. (2017). Shiga Toxins. Cham: Springer International Publishing.
Singh, S., Singh, N., Talwar, P., Kochhar, R., Mehta, S. K., and Jatava, L. (1990). Candida albicans and ABO blood groups. J. Assoc. Physicians India 38, 448–449.
Siukstaite, L., Imberty, A., and Römer, W. (2021). Structural diversities of lectins binding to the glycosphingolipid Gb3. Front. Mol. Biosci. 8:704685. doi: 10.3389/fmolb.2021.704685
Smith, D., Lord, J., Roberts, L., and Johannes, L. (2004). Glycosphingolipids as toxin receptors. Semin. Cell Dev. Biol. 15, 397–408. doi: 10.1016/j.semcdb.2004.03.005
Söderhäll, M., Normark, S., Ishikawa, K., Karlsson, K., Teneberg, S., Winberg, J., et al. (1997). Induction of protective immunity after escherichia coli bladder infection in primates. Dependence of the globoside-specific P-fimbrial tip adhesin and its cognate receptor. J. Clin. Invest. 100, 364–372. doi: 10.1172/JCI119542
Someya, Y. (2022). Lewis b antigen is a common ligand for genogroup I norovirus strains. FEBS Open Bio. doi: 10.1002/2211-5463.13455
Souza, T. N., Valdez, A. F., Rizzo, J., Zamith-Miranda, D., Guimarães, A. J., Nosanchuk, J. D., et al. (2021). Host cell membrane microdomains and fungal infection. Cell. Microbiol. 23:e13385. doi: 10.1111/cmi.13385
Ströh, L. J., Maginnis, M. S., Blaum, B. S., Nelson, C. D. S., Neu, U., Gee, G. V., et al. (2015). The greater affinity of JC polyomavirus capsid for α2,6-linked Lactoseries Tetrasaccharide c than for other Sialylated Glycans is a major determinant of infectivity. J. Virol. 89, 6364–6375. doi: 10.1128/JVI.00489-15
Stuart, A. D., and Brown, T. D. K. (2007). Alpha2,6-linked sialic acid acts as a receptor for feline calicivirus. J. Gen. Virol. 88, 177–186. doi: 10.1099/vir.0.82158-0
Sun, X., Guo, N., Li, D., Jin, M., Zhou, Y., Xie, G., et al. (2016). Binding specificity of P[8] VP8* proteins of rotavirus vaccine strains with histo-blood group antigens. Virology 495, 129–135. doi: 10.1016/j.virol.2016.05.010
Szymczak-Kulus, K., Weidler, S., Bereznicka, A., Mikolajczyk, K., Kaczmarek, R., Bednarz, B., et al. (2021). Human Gb3/CD77 synthase produces P1 glycotope-capped N-glycans, which mediate Shiga toxin 1 but not Shiga toxin 2 cell entry. J. Biol. Chem. doi: 10.1016/j.jbc.2021.100299
Tafesse, F. G., Rashidfarrokhi, A., Schmidt, F. I., Freinkman, E., Dougan, S., Dougan, M., et al. (2015). Disruption of sphingolipid biosynthesis blocks phagocytosis of Candida albicans. PLoS Pathog. 11:e1005188. doi: 10.1371/journal.ppat.1005188
Taïeb, N., Yahi, N., and Fantini, J. (2004). Rafts and related glycosphingolipid-enriched microdomains in the intestinal epithelium: bacterial targets linked to nutrient absorption. Adv. Drug Deliv. Rev. 56, 779–794. doi: 10.1016/j.addr.2003.09.007
Takahashi, Y., Murai, C., Shibata, S., Munakata, Y., Ishii, T., Ishii, K., et al. (1998). Human parvovirus B19 as a causative agent for rheumatoid arthritis. Proc. Natl. Acad. Sci. U. S. A. 95, 8227–8232. doi: 10.1073/pnas.95.14.8227
Taube, S., Jiang, M., and Wobus, C. E. (2010). Glycosphingolipids as receptors for non-enveloped viruses. Viruses 2, 1011–1049. doi: 10.3390/v2041011
Teneberg, S., Leonardsson, I., Karlsson, H., Jovall, P.-A., Angstrom, J., Danielsson, D., et al. (2002). Lactotetraosylceramide, a novel glycosphingolipid receptor for Helicobacter pylori, present in human gastric epithelium. J. Biol. Chem. 277, 19709–19719. doi: 10.1074/jbc.M201113200
Teneberg, S., Miller-Podraza, H., Lampert, H. C., Evans, D. J., Evans, D. G., Danielsson, D., et al. (1997). Carbohydrate binding specificity of the neutrophil-activating protein of Helicobacter pylori. J. Biol. Chem. 272, 19067–19071. doi: 10.1074/jbc.272.30.19067
Terlizzi, M. E., Gribaudo, G., and Maffei, M. E. (2017). UroPathogenic Escherichia coli (UPEC) infections: virulence factors, bladder responses, antibiotic, and non-antibiotic antimicrobial strategies. Front. Microbiol. 8:1566. doi: 10.3389/fmicb.2017.01566
Thompson, A. J., de Vries, R. P., and Paulson, J. C. (2019). Virus recognition of glycan receptors. Curr. Opin. Virol. 34, 117–129. doi: 10.1016/j.coviro.2019.01.004
Tosh, F. D., and Douglas, L. J. (1992). Characterization of a fucoside-binding adhesin of Candida albicans. Infect. Immun. 60, 4734–4739. doi: 10.1128/iai.60.11.4734-4739.1992
Tsai, B., Gilbert, J. M., Stehle, T., Lencer, W., Benjamin, T. L., and Rapoport, T. A. (2003). Gangliosides are receptors for murine polyoma virus and SV40. EMBO J. 22, 4346–4355. doi: 10.1093/emboj/cdg439
Tse, A. C. T., Cheung, R. T. F., Ho, S. L., and Chan, K. H. (2012). Guillain-Barré syndrome associated with acute hepatitis E infection. J. Clin. Neurosci. 19, 607–608. doi: 10.1016/j.jocn.2011.06.024
Unione, L., Gimeno, A., Valverde, P., Calloni, I., Coelho, H., Mirabella, S., et al. (2017). Glycans in infectious diseases. A molecular recognition perspective. Curr. Med. Chem. 24:702. doi: 10.2174/0929867324666170217093702
Varki, A., Cummings, R. D., Esko, J. D., Stanley, P., Hart, G. W., Aebi, M., et al. (2017). Essentials of glycobiology, 3rd Edition [Internet].
Viard, M., Parolini, I., Rawat, S. S., Fecchi, K., Sargiacomo, M., Puri, A., et al. (2003). The role of glycosphingolipids in HIV signaling, entry and pathogenesis. Glycoconj. J. 20, 213–222. doi: 10.1023/B:GLYC.0000024253.48791.d9
Wands, A. M., Fujita, A., McCombs, J. E., Cervin, J., Dedic, B., Rodriguez, A. C., et al. (2015). Fucosylation and protein glycosylation create functional receptors for cholera toxin. elife 4:e09545. doi: 10.7554/eLife.09545
Weigel-Kelley, K. A., Yoder, M. C., and Srivastava, A. (2003). Alpha5beta1 integrin as a cellular coreceptor for human parvovirus B19: requirement of functional activation of beta1 integrin for viral entry. Blood 102, 3927–3933. doi: 10.1182/blood-2003-05-1522
Wu, G., Lu, Z.-H., Kulkarni, N., Amin, R., and Ledeen, R. W. (2011). Mice lacking major brain gangliosides develop parkinsonism. Neurochem. Res. 36, 1706–1714. doi: 10.1007/s11064-011-0437-y
Wurpel, D. J., Beatson, S. A., Totsika, M., Petty, N. K., and Schembri, M. A. (2013). Chaperone-usher fimbriae of Escherichia coli. PLoS One 8:e52835. doi: 10.1371/journal.pone.0052835
Yamana, M., Kuwahara, M., Fukumoto, Y., Yoshikawa, K., Takada, K., and Kusunoki, S. (2019). Guillain-Barré syndrome and related diseases after influenza virus infection. Neurol. Neuroimmunol. Neuroinflamm. 6:e575. doi: 10.1212/NXI.0000000000000575
Yamashita, T., Hashiramoto, A., Haluzik, M., Mizukami, H., Beck, S., Norton, A., et al. (2003). Enhanced insulin sensitivity in mice lacking ganglioside GM3. Proc. Natl. Acad. Sci. U. S. A. 100, 3445–3449. doi: 10.1073/pnas.0635898100
Yamashita, T., Wada, R., Sasaki, T., Deng, C., Bierfreund, U., Sandhoff, K., et al. (1999). A vital role for glycosphingolipid synthesis during development and differentiation. Proc. Natl. Acad. Sci. U. S. A. 96, 9142–9147. doi: 10.1073/pnas.96.16.9142
Yanaka, S., Yogo, R., Watanabe, H., Taniguchi, Y., Satoh, T., Komura, N., et al. (2019). On-membrane dynamic interplay between anti-GM1 IgG antibodies and complement component C1q. Int. J. Mol. Sci. 21:147. doi: 10.3390/ijms21010147
Yowler, B. C., and Schengrund, C.-L. (2004). Glycosphingolipids—sweets for botulinum neurotoxin. Glycoconj. J. 21, 287–293. doi: 10.1023/B:GLYC.0000046271.64647.fd
Yu, J., Hung, J.-T., Wang, S.-H., Cheng, J.-Y., and Yu, A. L. (2020). Targeting glycosphingolipids for cancer immunotherapy. FEBS Lett. 594, 3602–3618. doi: 10.1002/1873-3468.13917
Yu, L., Lee, K. K., Sheth, H. B., Lane-Bell, P., Srivastava, G., Hindsgaul, O., et al. (1994). Fimbria-mediated adherence of Candida albicans to glycosphingolipid receptors on human buccal epithelial cells. Infect. Immun. 62, 2843–2848. doi: 10.1128/iai.62.7.2843-2848.1994
Yu, R. K., Nakatani, Y., and Yanagisawa, M. (2009). The role of glycosphingolipid metabolism in the developing brain. J. Lipid Res. 50, S440–S445. doi: 10.1194/jlr.R800028-JLR200
Yuki, N. (1997). Molecular mimicry between gangliosides and lipopolysaccharides of Campylobacter jejuni isolated from patients with Guillain-Barré syndrome and Miller Fisher syndrome. J. Infect. Dis. 176, S150–S153. doi: 10.1086/513800
Ywazaki, C. Y., Maza, P. K., Suzuki, E., Takahashi, H. K., and Straus, A. H. (2011). Role of host glycosphingolipids on Paracoccidioides brasiliensis adhesion. Mycopathologia 171, 325–332. doi: 10.1007/s11046-010-9376-4
Zakrzewska, M., Roszkowska, R., Zakrzewski, M., and Maciorkowska, E. (2021). Pneumocystis pneumonia: still a serious disease in children. J. Mother Child. 23, 159–162. doi: 10.34763/devperiodmed.20192303.159162
Zhao, D., Liu, Y., Huang, P., Xia, M., Li, W., Tan, M., et al. (2020). Histo-blood group antigens as divergent factors of groups A and C rotaviruses circulating in humans and different animal species. Emerg. Microb. Infect. 9, 1609–1617. doi: 10.1080/22221751.2020.1782270
Zhou, X., Nakashima, K., Ito, M., Zhang, X., Sakai, S., Feng, C., et al. (2020). Prevalence and viral loads of polyomaviruses BKPyV, JCPyV, MCPyV, TSPyV and NJPyV and hepatitis viruses HBV, HCV and HEV in HIV-infected patients in China. Sci. Rep. 10:17066. doi: 10.1038/s41598-020-74244-0
Zhuo, D., Li, X., and Guan, F. (2018). Biological roles of aberrantly expressed glycosphingolipids and related enzymes in human Cancer development and progression. Front. Physiol. 9:466. doi: 10.3389/fphys.2018.00466
Zimmerman, J. W., Lindermuth, J., Fish, P. A., Palace, G. P., Stevenson, T. T., and DeMong, D. E. (1998). A novel carbohydrate-glycosphingolipid interaction between a beta-(1-3)-glucan immunomodulator, PGG-glucan, and lactosylceramide of human leukocytes. J. Biol. Chem. 273, 22014–22020. doi: 10.1074/jbc.273.34.22014
Keywords: glycosphingolipids, bacteria, viruses, fungi, blood groups antigens
Citation: Bereznicka A, Mikolajczyk K, Czerwinski M and Kaczmarek R (2022) Microbial lectome versus host glycolipidome: How pathogens exploit glycosphingolipids to invade, dupe or kill. Front. Microbiol. 13:958653. doi: 10.3389/fmicb.2022.958653
Edited by:
Fabrizio Chiodo, National Research Council (CNR), ItalyReviewed by:
Anne Imberty, Centre National de la Recherche Scientifique (CNRS), FranceLeonardo Nimrichter, Federal University of Rio de Janeiro, Brazil
Copyright © 2022 Bereznicka, Mikolajczyk, Czerwinski and Kaczmarek. This is an open-access article distributed under the terms of the Creative Commons Attribution License (CC BY). The use, distribution or reproduction in other forums is permitted, provided the original author(s) and the copyright owner(s) are credited and that the original publication in this journal is cited, in accordance with accepted academic practice. No use, distribution or reproduction is permitted which does not comply with these terms.
*Correspondence: Radoslaw Kaczmarek, cmFkb3NsYXcua2Fjem1hcmVrQGhpcnN6ZmVsZC5wbA==; Marcin Czerwinski, bWFyY2luLmN6ZXJ3aW5za2lAaGlyc3pmZWxkLnBs