- 1Multimodal Laboratory Medicine Research Area, Unit of Human Microbiome, Bambino Gesù Children’s Hospital, IRCCS, Rome, Italy
- 2Department of Diagnostic and Laboratory Medicine, Unit of Microbiology and Diagnostic Immunology, Unit of Microbiomics and Multimodal Laboratory Medicine Research Area, Unit of Human Microbiome, Bambino Gesù Children’s Hospital, IRCCS, Rome, Italy
Chronic inflammation is a hallmark for a variety of disorders and is at least partially responsible for disease progression and poor patient health. In recent years, the microbiota inhabiting the human gut has been associated with not only intestinal inflammatory diseases but also those that affect the brain, liver, lungs, and joints. Despite a strong correlation between specific microbial signatures and inflammation, whether or not these microbes are disease markers or disease drivers is still a matter of debate. In this review, we discuss what is known about the molecular mechanisms by which the gut microbiota can modulate inflammation, both in the intestine and beyond. We identify the current gaps in our knowledge of biological mechanisms, discuss how these gaps have likely contributed to the uncertain outcome of fecal microbiota transplantation and probiotic clinical trials, and suggest how both mechanistic insight and -omics-based approaches can better inform study design and therapeutic intervention.
Introduction
Inflammation is a vital biological function evolved to protect the body from external pathogens and harmful or dying cells. Acute inflammation involves identification of the harmful material, emigration of neutrophils and other immune cells from the blood vessels to the offended tissue, and destroying or phagocytosing the culprit. This natural process is largely beneficial, resolves itself in a matter of hours or days, and is a necessary part of healing. However, when the complex machinery involved in mounting these defenses misinterprets these signals the body can respond inappropriately, resulting in a prolonged inflammatory state referred to as chronic inflammation, which is the hallmark of a wide array of disorders collectively and loosely referred to as inflammatory diseases. Uncovering the numerous players responsible for precipitating this inappropriate inflammatory response is an ongoing but necessary scientific challenge, as it is widely believed that combatting chronic inflammation is at the heart of alleviating many of the symptoms associated with these diseases. One such player that is gaining great attention in recent years is the ecosystem of bacteria, viruses, fungi, and other microbes that inhabit the gut, collectively referred to as the gut microbiota (GM).
The gut harbors hundreds of commensal species that perform a wide array of necessary biological functions and whose presence is vital to human survival (Quigley, 2013). Furthermore, these species live in a balanced ecosystem which, when unbalanced, can have negative consequences on human health, a state known as dysbiosis. The body of evidence suggesting a link between dysbiosis of the GM and inflammatory disorders is vast and has been extensively reviewed in many pathological contexts, such as inflammatory bowel disease (IBD) (Kostic et al., 2014; Putignani et al., 2016; Glassner et al., 2020), cancer (Gagnière et al., 2016; Chen et al., 2017; Lucas et al., 2017; Saus et al., 2019; Cheng et al., 2020), rheumatic arthritis (Scher et al., 2016; Konig, 2020; Zaiss et al., 2021), psoriasis (Myers et al., 2019; Olejniczak-Staruch et al., 2021), metabolic diseases (Manco et al., 2010; del Chierico et al., 2014; Nobili et al., 2019; Montanari et al., 2021), food allergies (Vernocchi et al., 2015; Berni Canani et al., 2019; Bunyavanich and Berin, 2019; Shu et al., 2019), and neuropsychiatric disorders (Petra et al., 2015; Koopman and el Aidy, 2017; Peirce and Alviña, 2019; Ristori et al., 2019, 2020; Rutsch et al., 2020). However, many studies investigating the putative role of the GM in inflammatory disease have been limited to identifying dysbiosis in the gut in affected patients. For example, patients with IBD have been found to have reduced diversity of the GM overall (Manichanh et al., 2006; Pascal et al., 2017; Kowalska-Duplaga et al., 2019; lo Presti et al., 2019; Putignani et al., 2021), as well as either expanded or diminished populations of specific bacterial genera/species (Table 1). Patterns of dysbiosis in the gut have emerged for many inflammatory diseases that are also not intestinal in origin, leading to a bacterial signature of chronic inflammation in multiple pathological contexts (Table 2). While these studies are strongly suggestive, whether the bacteria in question actually drive disease progression, or whether they are simply markers for disease-related dysbiosis, is still a matter of debate.
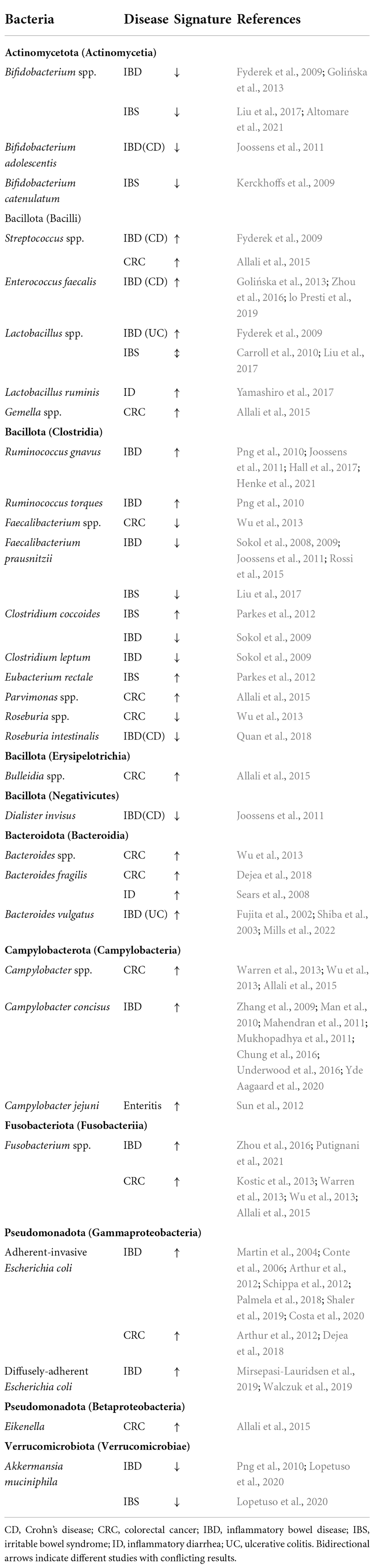
Table 1. Summary of bacterial genera/species signatures associated with inflammatory diseases, organized as Phylum (Class).
Some studies have attempted to demonstrate a degree of causality between dysbiosis and disease in human studies by different statistical means, such as Mendelian randomization analyses, and other computational models (Sanna et al., 2019; Lv et al., 2021). Others have attempted to address these concerns by employing both preclinical and germ-free murine models. In mice, transplantation of the GM of a preclinical model of colorectal cancer into germ-free mice resulted in intestinal inflammation and tumorigenesis, which was reversed upon treatment with antibiotics (Zackular et al., 2013). Another study found that monocolonization of a germ-free, colitis-susceptible mouse model with Escherichia coli NC101 was sufficient to induce intestinal inflammation and tumorigenesis (Arthur et al., 2012). In a study using bacteria from humans, a tumor-prone mouse model co-infected with Escherichia coli and Bacteroides fragilis strains isolated from patients with familial adenomatous polyposis had a stronger pro-inflammatory response, greater tumor growth, and higher mortality rates than mice infected with either strain alone (Dejea et al., 2018). Beyond the gut, fecal microbiota transplantation between mouse models and the modulation of the GM with either pro- or antibiotics was sufficient to shape the hepatic inflammatory environment and either promote or suppress liver carcinogenesis (Schneider et al., 2022). In humans, similar bacterial species were found to characterize patients who responded positively to hepatic cancer treatment (Ponziani et al., 2022). In these studies, the causal link between gut dysbiosis and inflammatory diseases has at least been strengthened by these methods.
However, there have also been times when studies designed to uncover a causal link between dysbiosis and pathology have not supported the hypothesis that these specific bacteria are drivers of disease progression. It has been demonstrated that not taking confounding variables, such as diet, weight, alcohol consumption, and tobacco use into consideration when matching patients with healthy controls can lead to exaggerated and artifactual findings of dysbiosis (Vujkovic-Cvijin et al., 2020). Consistently, one large metagenomics study performed in Australia concluded that the variability in the GM between patients with autism and healthy controls was due to other confounding variables, such as age and diet, rather than being directly related to autism spectrum disorder (Yap et al., 2021). Furthermore, despite the numerous studies associating the prevalence of Campylobacter spp. with inflammatory bowel disease in both adults and children (Table 1) and a meta-analysis predicting the contrary (Castaño-Rodríguez et al., 2017), a longitudinal case study of a Danish cohort found that patients with Campylobacter concisus- or Campylobacter jejuni-positive stool samples were not at increased risk of developing IBD (Nielsen et al., 2019), nor was there an increased prevalence of Campylobacter concisus in a study of British children presenting with de novo IBD (Hansen et al., 2013). Despite these disappointing results, it is important to note that these studies do not completely exclude the possibility of a causal link between Campylobacter spp. and IBD. Even if the presence of these bacteria in the gut does not increase the risk of disease onset, it is still possible that they are responsible for sustaining the chronic inflammation that is initiated by other means, and are therefore still active participants in the pathology. These kinds of studies demonstrate why a better understanding of biological mechanisms can better inform our interpretation of clinical data.
Taken together, these studies have led to a general consensus that the GM can in fact have a direct effect on human health, and at least participate in the progression of various inflammatory diseases. However, while some of these studies have strengthened the evidence of a causal link between the GM and disease progression, much mechanistic insight into how the GM directly affects inflammation is still lacking. In this review, we focus specifically on what is known about the biological mechanisms employed by microbes in the gut to influence inflammation, identify the current gaps in our knowledge, and discuss how a deeper understanding of mechanistic insight can shape future clinical study design and therapeutic strategies.
Bacterial invasion of intestinal epithelial cells can drive inflammation
One of the most direct ways that intestinal bacteria can precipitate a pro-inflammatory response is by invading the cells in their environment. In vitro models of the intestinal epithelial barrier have shown that many bacteria, such as diffusely adherent Escherichia coli, Shigella dysenteriae, Fusobacterium varium, Bacteroides vulgatus, and Clostridium clostridioforme, adhere to and are internalized by intestinal epithelial cells, whereupon they stimulate the secretion of tumor necrosis factor-α (TNF-α) (Ohkusa et al., 2009; Gopal et al., 2017; Walczuk et al., 2019). One study found that Campylobacter concisus, as well as other Campylobacter species associated with Crohn’s disease, were also able to invade human intestinal epithelial cells in vitro, avoid being phagocytized, and induce inflammation (Man et al., 2010). Most intriguingly, this invasion was significantly increased upon co-treatment with either TNF-α or interferon-γ (IFN-γ), suggesting that Campylobacter species are more virulent in an environment that is already inflamed (Man et al., 2010). In light of the Danish study finding no significant increase in the risk of developing IBD in Campylobacter concisus-positive patients (Nielsen et al., 2019), these studies taken together support the hypothesis that C. concisus influences IBD progression by sustaining a pro-inflammatory response initiated by other factors. Another (not necessarily exclusive) explanation is the high variability in virulence and intracellular survival between different C. concisus strains, with those isolated from patients with chronic IBD having an invasive potential of up to 500 times those isolated from healthy controls (Kaakoush et al., 2011; Deshpande et al., 2013), as well as an increased ability to evade the autophagic process (Burgos-Portugal et al., 2014). Furthermore, the most invasive Campylobacter concisus strains isolated from patients were also found to express exotoxin 9, a gene with a domain that has homologues in many viruses and is thought to contribute to their virulence (Kaakoush et al., 2011). Another possibility for differences in virulence is the expression of zonula occludens toxin (zot), which is only found in a subset of C. concisus strains and which alone can induce a pro-inflammatory response, cause epithelial barrier damage, and initiate cell death in vitro (Mahendran et al., 2016). Genetic manipulation of different C. concisus strains could shed further light on which genes most affect their ability to invade, avoid autophagy, and induce inflammation in human cells. Finally, it is important to note that characterization of the GM rarely resolves down to the species level, let alone strain. Longitudinal studies, such as the ones conducted on Danish adults and British children, which fail to take differences between strains into consideration, may have missed an association between C. concisus infection and IBD due to this inherent variability. With recent advances in -omics methods, human studies would ideally include a deeper characterization of the GM to avoid masking such effects.
Similarly, Campylobacter jejuni is also associated with intestinal inflammation, enteritis, and colorectal cancer and has long been known to be able to invade the colonic mucosa in humans (van Spreeuwel et al., 1985). Infection of a colitis-susceptible mouse model with C. jejuni activated mammalian target of rapamycin (mTOR) signaling, intestinal inflammation, neutrophil infiltration, and severe colitis, which could be reversed by administering rapamycin and activating autophagy (Lippert et al., 2009; Sun et al., 2012). C. jejuni also expresses surface lipooligosaccharides (LOS), which mimick human gangliosides, the structure of which varies between strains and are important to the bacteria’s invasive potential and survival both in vitro and in vivo (Guerry et al., 2002; Louwen et al., 2008; Naito et al., 2010). Genetic manipulation of C. jejuni demonstrated that their ability to invade host cells was also strongly correlated with the production of chemotaxis protein CheY and, to a lesser extent, the energy taxis protein CetA (Bouwman et al., 2014). Some strains also produce the genotoxin cytolethal distending toxin, which was shown to be necessary for C. jejuni-induced tumorigenesis in germ-free mice, though its absence did not impair its ability to invade host cells (Bouwman et al., 2014; He et al., 2019). Given the many different molecular players involved in the virulence, pro-inflammatory potential and host response to C. jejuni infection, deeper strain-level characterization of the GM in human patients could shed more light on the association between C. jejuni and inflammatory diseases.
Bacterial cell-surface molecules can independently influence inflammation
Bacteria can express a variety of molecules on their cell surface, which can aid in motility, intercellular communication, and otherwise influence their microenvironment. In the context of intestinal inflammation, many of these extracellular molecules can also aid in the bacterium’s ability to invade host cells. Cytolethal distending toxin produced by C. jejuni, for example, is composed of three subunits, two of which bind lipid rafts on the cell membrane, allowing the third to translocate across and induce inflammation and apoptosis (Lin et al., 2011). However, numerous studies have shown that these molecules can modulate the inflammatory response even when administered without the bacteria themselves, demonstrating that these molecules have an independent role in inflammation uncoupled from their role in aiding bacterial adherence or invasion. Flagellin, the protein that forms the filament of the bacterial flagellum, was one of the first molecules to be shown to have this effect. Salmonella-derived flagellin was found to have a dose-dependent pro-inflammatory effect in mice, even causing death at the highest doses, despite being administered without the pathogen itself (Ciacci-Woolwine et al., 1998; Eaves-Pyles et al., 2001). Later, it was demonstrated that Salmonella-derived flagellin activated the NLRC4 inflammasome by neuronal apoptosis inhibitory proteins (NAIPs) (Miao et al., 2010; Bouwman et al., 2014). Since then, similar inflammatory responses have been reported to be elicited by flagellin derived from other bacterial strains, such as commensal E. coli strains (Rhee et al., 2005) and enterohemorrhagic E. coli (Lewis et al., 2016). Presumably, these molecules can elicit a pro-inflammatory response because the immune system has learned to recognize a foreign invader from its cell surface, and thus mounts a defense as soon as the intruder is sensed and before they have time to invade host cells and do damage. However, despite being a highly conserved protein across the prokaryotic kingdom, not all bacterial-derived flagellin proteins elicit this same effect. Flagellin derived from Roseburia intestinalis, unlike that from pathogenic bacteria, actually has a protective effect on colon epithelial cells in vitro and protects from colitis in vivo by upregulating the lncRNA HIF1A-AS2, which in turn suppresses the pro-inflammatory response (Quan et al., 2018; Wu et al., 2020).
Other bacterial–cell surface molecules have also been found to have potent anti-inflammatory effects. In mice, strains of both Bifidobacterium breve and Bacillus subtilis that produce a surface-associated exopolysaccharide are able to evade the host’s immune system, suppress inflammation and thus help protect against Citrobacter rodentium-induced enteric insults via TLR4 signaling (Fanning et al., 2012; Jones et al., 2014). Faecalibacterium prausnitzii, a bacterial species typically underrepresented in patients with inflammatory diseases (Table 1), also has anti-inflammatory effects in experimental models. In both in vitro culture and a preclinical mouse model of colitis, two different F. prausnitzii strains were able to attenuate the pro-inflammatory response and protect against disease progression (Rossi et al., 2015). Interestingly, the F. prausnitzii strain HTF-F, which produces an extracellular polymeric matrix (EPM), was the most successful of the two strains at protecting against inflammation, and treatment with the EPM on its own was sufficient to elicit a protective, anti-inflammatory response (Rossi et al., 2015).
Similarly, Bifidobacterium longum 3564, formerly known as Bifidobacterium infantis, can attenuate the pro-inflammatory response elicited by pathogenic bacteria both in vitro and in vivo, not by interfering with their ability to bind and invade intestinal cells (O’Hara et al., 2006), but rather by directly interfering with chemokine secretion and the subsequent pro-inflammatory response to infection (O’Mahony et al., 2008; Sibartie et al., 2009; Scully et al., 2013). This strain was also found to be able to suppress pro-inflammatory chemokines released upon exposure to Salmonella typhimurium, Clostridium difficile, or Mycobacterium paratuberculosis in vitro (Sibartie et al., 2009), as well as protect against ovalbumin respiratory allergy–induced inflammation in mice (Lyons et al., 2010). It was subsequently demonstrated that this strain differs from other Bifidobacterium longum strains in the expression of a unique cell surface exopolysaccharide (sEPS) (Altmann et al., 2016). Not only was this sEPS shown to be crucial in the protective and anti-inflammatory properties of B. longum 35624, but removing the gene encoding for this sEPS actually caused the strain to induce a pro-inflammatory response in the lungs when delivered intranasally to mouse models of inflammatory diseases, although polysaccharide-negative strains were not sufficient to induce chronic inflammation and colitis in healthy mice (Schiavi et al., 2016). Since its discovery, studies have shown that treatment with this sEPS alone was sufficient to alleviate inflammation and protect against symptoms in preclinical mouse models of respiratory allergies (Schiavi et al., 2018), osteoporosis (Wallimann et al., 2021) and in mice infected with a lethal influenza virus (Groeger et al., 2020). These anti-inflammatory patterns were also replicated in both healthy volunteers and multiple patient groups fed with B. longum 35624 (Konieczna et al., 2012; Groeger et al., 2013; Zaharuddin et al., 2019), though clinical trials involving treatment with sEPS itself are still lacking.
Although F. prausnitzii and Bifidobacterium spp. are usually underrepresented in pathological contexts, and thus are almost always considered to be “healthy” bacteria, some bacterial species can have opposing effects on inflammation depending on their extracellular chemistry. For example, polysaccharide A (PSA) produced by B. fragilis is sufficient to reverse the CD4+ T cell deficiency and abnormal lymphoid organogenesis found in germ-free mice, indicating an essential role for an extracellular molecule produced by commensal bacteria in the gut in immune system development (Mazmanian et al., 2005). PSA is also sufficient to suppress interleukin-17 and protect from experimental colitis in a mouse model infected with Helicobacter hepaticus, again by acting on CD4+ T cell populations (Mazmanian et al., 2008). A follow-up study further elucidated that B. fragilis-produced PSA regulates intestinal inflammation by mediating the conversion of CD4+ T cells into Foxp3+ T cells, which release anti-inflammatory cytokines and protect the intestinal mucosa. Finally, B. fragilis can both prevent and cure colitis in mouse models by releasing PSA in outer membrane vesicles, stimulating plasmacytoid dendritic cells to act in concert with CD4+ T cells and conferring immunoprotection (Round and Mazmanian, 2010; Shen et al., 2012; Dasgupta et al., 2014). Since these discoveries, PSA has also been shown to confer a protective effect on mouse models of demyelinating disease (Ochoa-Repáraz et al., 2010), viral encephalitis (Ramakrishna et al., 2019), and adverse drug reactions to voriconazole (Wang et al., 2021). As of this time, no clinical studies have been conducted in humans to address whether or not PSA-positive B. fragilis could have a protective effect. One possible reason is that, as in the case of B. fragilis, the same species of bacteria can have opposing effects on inflammation and disease progression, and thus a great amount of caution must be taken in designing such studies. Apart from PSA, some B. fragilis strains can also secrete a tumorigenic toxin referred to as Bacteroides fragilis toxin (BFT), and strains producing this toxin can cause colitis and tumorigenesis in mice (Wu et al., 2009). In humans, PSA-positive B. fragilis were significantly reduced in isolates from human patients with IBD, and B. fragilis subpopulations expressing BFT were less likely to also be PSA-positive (Blandford et al., 2019), though no human studies have been conducted to address the causality of either BFT or PSA on disease state. Differences in administration between studies are also a variable that should not be underestimated. For example, one study in a mouse model of type 1 diabetes showed that, while oral administration of heat-killed B. fragilis had a protective, anti-inflammatory effect, intravenous injection, or oral administration under enhanced gut permeability conditions, actually aggravated the symptoms (Sofi et al., 2019). Notably, B. fragilis lacking in PSA had no effect on disease progression, no matter the method by which it was administered (Sofi et al., 2019). Although this further strengthens the evidence for PSA as the driver for Bacteroides fragilis-mediated changes, it also suggests that PSA can elicit unforeseen consequences if found in the wrong anatomical compartment. Given the dual role that B. fragilis can have on inflammation, and given the beneficial effects of PSA in multiple preclinical mouse models, a perhaps safer therapeutic strategy could be to investigate whether administration of PSA alone is safe, well-tolerated, and anti-inflammatory in humans as well.
Several studies in genetically susceptible germ-free mouse models have elucidated a causal link between E. faecalis and IBD by demonstrating that the colonization of the alimentary tract with this single species led to chronic inflammation and disease (Balish and Warner, 2002). A follow-up study in the same model demonstrated that this effect was dependent on the matrix metalloprotease gelatinase, which contributes to chronic inflammation by compromising epithelial barrier integrity (Steck et al., 2011). Surprisingly, however, in a mouse model of IBD, heat-killed E. faecalis was found to alleviate inflammation and partially mitigate disease progression (Choi et al., 2019). While the authors of the study demonstrated a dose-dependent decrease in the expression of pro-inflammatory cytokines upon administration of heat-killed E. faecalis, they did not address what specific compound was responsible for this anti-inflammatory effect. Despite this lack of mechanistic knowledge, this study strongly suggests that E. faecalis can also produce a different compound that has therapeutic potential and is yet another example of how further investigation into the anti-inflammatory effects of bacteria-derived molecules could uncover novel therapeutic strategies, especially where probiotic intervention would be inappropriate.
Ruminococcus gnavus is another species often associated with inflammatory bowel diseases, presumably due to its ability to degrade human secretory mucin and thus alter its intestinal microenvironment to make it more favorable to a pro-inflammatory bacterial signature (Table 1 and Png et al., 2010). This bacterial species also produce a glucorhamnan polysaccharide which, when administered alone, can itself induce a potent inflammatory response in vitro via TLR4 signaling (Henke et al., 2019; Haynie et al., 2021). On the other hand, a study comparing R. gnavus isolates from patients with IBD found that some strains possessed a thick polysaccharide capsule. Bacterial strains lacking this protective capsule induced a strong pro-inflammatory response both in vitro and in mice, while those possessing it did not, although whether the polysaccharide capsule impacted the bacteria’s mucolytic abilities was not investigated (Henke et al., 2021). These studies further highlight the need for biological mechanistic insight into the relationship between microbes and disease, especially when trying to interpret correlative clinical studies, or isolating bacterial strains to use in therapeutic scenarios.
Bacterial metabolites produced in the gut can influence inflammation elsewhere
In addition to expressing molecules on their cell surface, bacteria in the intestine also produce metabolites, which can have implications that are far-reaching throughout the human body. Arguably, the most studied of these metabolites are short chain fatty acids (SCFAs), such as acetate, propionate, and butyrate, which are produced upon bacterial fermentation of dietary fiber and which largely influence inflammation via binding to G-protein-coupled receptors (Nogal et al., 2021). The almost ubiquitous trend of reduced Bifidobacterium spp. found in the GM of patients with chronic inflammation (Table 1) is often also correlated with a decrease in SCFAs, and which have had some success in alleviating symptoms of IBD (Kanauchi et al., 2002). In mouse models of colitis, asthma, and arthritis, SCFAs attenuated chronic inflammation by directly binding the G-protein-coupled receptor 43 (GPR43) and provoking a strong anti-inflammatory response, indicating that bacteria-derived metabolites can also alleviate inflammation far from the organ in which they live (Maslowski et al., 2009). In mice, Bifidobacterium lactis probiotic Probio-M8 reduced Aβ-plaque burden and improved cognition in a mouse model of Alzheimer’s disease (Cao et al., 2021). In humans, patients suffering from ulcerative colitis, psoriasis, or chronic fatigue syndrome all had reduced inflammatory markers when fed B. infantis 35624 compared with placebo-fed controls, indicating that these far-reaching anti-inflammatory effects are also reproducible in human subjects (Groeger et al., 2013).
Clostridium butyricum, most studied for its production of the SCFA butyrate, has also successfully protected both mouse models and human patients suffering from IBS-induced inflammation, as well as a mouse model of intestinal cancer, when administered as a probiotic (Sun et al., 2018; Zhao et al., 2019; Chen et al., 2020a). Beyond the gut, probiotic use of C. butyricum was also found to be protective against inflammation in mouse models of acute pancreatitis (Pan et al., 2019a), atherosclerosis (Chen et al., 2020b), metabolic disorders (Stoeva et al., 2021), and multiple sclerosis (Chen et al., 2019). While probiotic use of C. butyricum is believed to act on inflammation at many different levels, from “correcting” dysbiosis in the intestine to reducing intestinal leakage and beyond (Stoeva et al., 2021), the administration of sodium butyrate alone has also demonstrated an anti-inflammatory effect conferred directly by this bacterial metabolite. In a mouse model of Crohn’s disease, sodium butyrate feeding before TNBS-induced colitis significantly protected mice from inflammation and intestinal barrier dysfunction by binding G-protein-coupled Receptor 109 A (GPR109A), inhibiting histone deacetylases and suppressing pro-inflammatory pathways (Chen et al., 2018; Dou et al., 2020). Furthermore, butyrate produced by bacteria in the gut can enter the bloodstream, travel to multiple organs, and even cross the blood–brain barrier (Liu et al., 2020). Sodium butyrate feeding has thus also been shown to have an anti-inflammatory effect and protect against symptoms in mouse models of depression (Qiu et al., 2020), pancreatitis (Pan et al., 2019b), kidney disease (Felizardo et al., 2019), and obesity (Hong et al., 2016). Interestingly, sodium butyrate supplementation had an opposite effect when administered during gestation, with rats born to butyrate-fed mothers presenting with insulin resistance and increased skeletal fat accumulation (Huang et al., 2017), suggesting that SCFA metabolism during pregnancy can have very different consequences on health and disease outcome.
Faecalibacterium prausnitzii is another butyrate-producing commensal species found in abundance in the healthy human intestine, with strain-specific differences in butyrate production that correlate with its anti-inflammatory strength (Martín et al., 2017). Studies have found that the treatment of preclinical rodent models of colitis with both the bacteria itself and its culture supernatant has an anti-inflammatory effect, suggesting that secreted molecules, likely butyrate, are responsible for conferring protection against colitis (Qiu et al., 2013; Martín et al., 2014). Similarly, both living and dead preparations of Faecalibacterium prausnitzii could alleviate inflammation in a mouse model of asthma, also by directly modulating SCFA production (Hu et al., 2021). F. prausnitzii has also been used successfully as an anti-inflammatory prophylactic in a mouse model of pelvic radiation disease (Lapiere et al., 2020) and in a rat model of depression and anxiety (Hao et al., 2019). These studies, together with an almost universal signature of decreased F. prausnitzii populations in the GM of patients with inflammatory diseases, have made it an excellent candidate for future human studies and its production as a probiotic, though results in clinical trials are still lacking.
Other SCFAs have also been used, either singularly or together, in mouse models of inflammation beyond the gut. In a preclinical model of non-alcoholic steatohepatitis sodium acetate, sodium butyrate, and sodium propionate were all individually found to protect against inflammation and disease progression, although sodium acetate was the most successful of the three (Deng et al., 2020). Another study in mice fed with a high-fat diet found that the combinatorial effect of acetate and propionate was more effective than butyrate as a suppressor of inflammation, increased body weight, and diabetes (Mandaliya et al., 2021). Methyl acetate, on the other hand, was able to suppress inflammatory cell infiltration in the central nervous system, thereby protecting the spinal cord from demyelination and improving the health of a mouse model of multiple sclerosis (Xie et al., 2021), while ethyl acetate alleviated inflammation and rheumatoid arthritis in rats (Ikram et al., 2021). Propionate has also successfully been used to suppress inflammation and improve disease outcomes in mouse models of colitis (Bajic et al., 2020), atherosclerosis (Haghikia et al., 2022), and hypertensive cardiovascular damage (Bartolomaeus et al., 2019). Taken together, these studies suggest a system-wide anti-inflammatory effect of bacterially derived SCFAs, providing exciting new possibilities for therapy.
Interestingly, the anti-inflammatory properties of SCFA production have not always been associated with a beneficial outcome. One study employing both metagenomic and metabolomic profiling demonstrated elevated levels of both SCFAs and SCFA-producing bacteria in patients with non-alcoholic fatty liver disease and hepatocellular carcinoma (Behary et al., 2021). Furthermore, bacterial extracts from patients elicited an immunosuppressive response in peripheral blood mononuclear cell preparations, suggesting that, by dampening the immune system, GM-derived SCFAs can create a permissive landscape for cancerous cells to prosper by evading immune checkpoints (Behary et al., 2021). Studies like these highlight the importance of comprehending both the biological context and the mechanisms by which the GM can influence disease, to predict potential unintended consequences of modulating the GM in response to pathology.
In addition to dietary fiber, bacteria in the gut also participate in the metabolism of the amino acid tryptophan, producing metabolites such as indole, skatole, and tryptamine derivatives (Gasaly et al., 2021). These metabolites also have been found to regulate the gut microbial community, and intestinal immunity, and can have a systemic-wide effect on inflammation via binding to the xenobiotic receptor AhR (Zelante et al., 2013; Gasaly et al., 2021). In mice, Lactobacillus-derived tryptophan metabolites protect the intestinal mucosa and suppress inflammation via IL-22 (Zelante et al., 2013). In vitro, the Bifidobacterium-derived tryptophan metabolite indole-3-lactic acid alone was found to be able to suppress TNF-α and IL-8 in chemically stressed gut epithelial cells (Ehrlich et al., 2020). Beyond the gut, tryptophan metabolites have been found to suppress inflammation via AHR signaling in the central nervous system (Rothhammer et al., 2016) and have been found to have beneficial effects on diabetes and metabolic syndromes by regulating the microRNA miR-181 family (Galligan, 2018; Virtue et al., 2019). In humans, tryptophan metabolites in the serum are negatively correlated with disease activity in patients with IBD (Nikolaus et al., 2017) and with waist-to-hip ratio and systemic inflammation in people infected with HIV (Gelpi et al., 2020). However, a diet high in dietary fiber has been shown to have a beneficial effect on children with obesity and people with IBD (Zhang et al., 2015; Fritsch et al., 2021), and while this diet also increased tryptophan metabolites (among many other things), clinical trials investigating the effect of tryptophan catabolism specifically on chronic inflammation are still lacking.
Modulation of the gut microbiota in human trials has led to mixed results
Fecal microbiota transplantation (FMT) is a clinical practice by which fecal samples from healthy donors are transplanted into patients with severe dysbiosis. While this method is widely used to treat severely ill patients with C. difficile infections, the emerging importance of GM health in other pathological contexts has led to clinical trials of FMT in many different inflammatory diseases (Table 3). However, given the lack of deep -omics-driven characterization of the GM in clinical settings, as well as the gaps in mechanistic knowledge that still exist in our understanding of how the GM modulates inflammation, it is understandable that therapeutic intervention via FMT has not always resulted in clear-cut success (Table 3). In the case of ulcerative colitis, FMT generally has an approximately 30% success rate, and some success has also been noted for patients with cancer and cirrhosis (Table 3). However, despite the fact that FMT can protect from intestinal inflammation, insulin resistance, and weight gain in a preclinical mouse model of diabetes and diabetic kidney disease, clinical trials of FMT to treat obesity and insulin resistance have not been successful (Bastos et al., 2022 and Table 3).
In most cases, the side effects of FMT consist of mild to moderate forms of gastrointestinal discomfort. Unfortunately, some of the other risks associated with FMT are, while uncommon, still serious enough to be noteworthy. In the case of ulcerative colitis, some patients receiving FMT have experienced worsening colitis, colectomy, or pneumonia (Paramsothy et al., 2017; Costello et al., 2019; Quagliariello et al., 2020), and one patient receiving FMT for chronic diarrhea developed adhesion ileus (Harsch and Konturek, 2019). Perplexingly, despite the lack of clinical evidence of an effect of FMT on obesity (Table 3), one case study reported a C. difficile-infected patient experiencing rapid and inexplicable weight gain after receiving FMT from her own overweight daughter (Alang and Kelly, 2015). In one tragic case, failure to adequately screen the donor material for drug-resistant pathogens led to bacteremia and the death of one recipient of FMT (DeFilipp et al., 2019). While the most severe of these adverse effects can be overcome by a more rigorous screening of donor samples for infectious pathogens, it is still clear that there are additional, currently unknown variables at play when patients undergo FMT. These unknown variables, combined with a broad spectrum of administration methods, dosages, donor–patient matching criteria, and evaluation of transplant success have all likely contributed to the uncertain outcome of FMT in treating inflammatory diseases. With the recent increase in interest in FMT as a therapeutic option for multiple syndromes, it is clear that a certain amount of standardization in sample screening, storage, and administration is necessary for a more robust clinical outcome (Cammarota et al., 2019). However, it is also apparent that more profound knowledge of the biological mechanisms by which the GM modulate inflammation, as well as a deeper -omics-based characterization of both patient and donor fecal samples, could help bring us closer to clinical success (Figure 1).
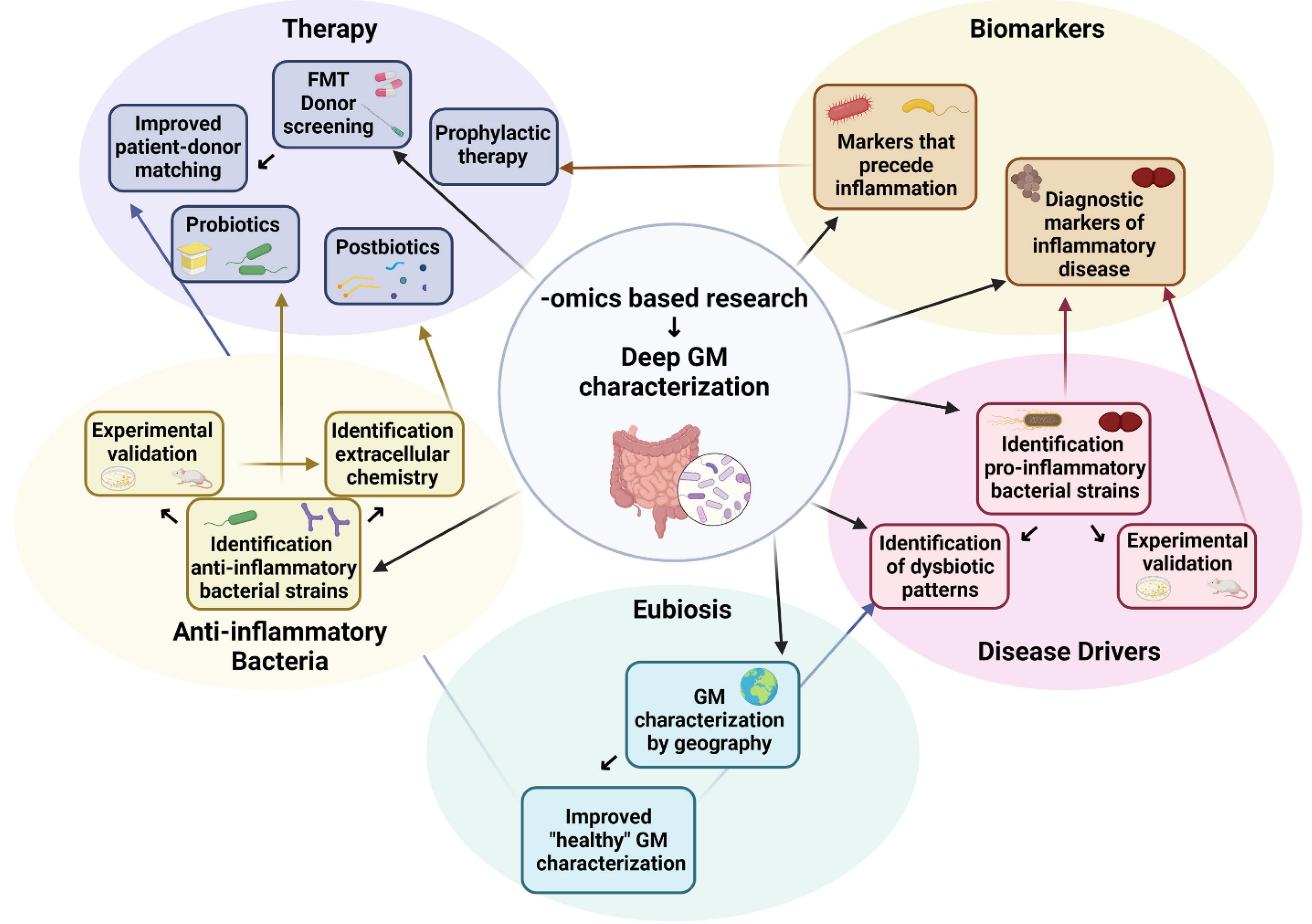
Figure 1. How omics-based research can inform and improve study design and therapeutic strategies. Created with BioRender.com.
Probiotic and metabolite intervention in patients with inflammatory diseases
While FMT has had some success in treating patients with inflammatory diseases, the complexity of the GM has also led to a number of confounding variables that can mask a successful outcome and that have been difficult to identify. With new advances in mechanistic insight, some clinical studies have been able to investigate probiotic intervention in inflammatory diseases, which can be more cost-effective, less invasive, and simpler to evaluate for safety and side effects. In the gut, probiotics were found to reduce disease severity and inflammatory markers in patients with IBS (Xu et al., 2021), as well as suppress inflammation in the intestine of adults and children with cystic fibrosis (Coffey et al., 2020) and in HIV-infected patients receiving antiretroviral medication (d’Ettorre et al., 2015). Beyond the gut, probiotics have also been found to alleviate inflammation and ameliorate symptoms of diseases of various etiology. In the context of metabolic syndromes, such as obesity, non-alcoholic fatty liver disease, type-2 diabetes, gestational diabetes, chronic kidney disease, and insulin resistance, numerous clinical studies have found a beneficial effect of probiotic intervention on inflammatory biomarkers, body mass index (BMI), intrahepatic fat, and insulin sensitivity (Sáez-Lara et al., 2016; Hajifaraji et al., 2018; Ahn et al., 2019; Vlachou et al., 2020; Shirvani-Rad et al., 2021; Zheng et al., 2021). Taken together, these studies suggest that the failures of FMT intervention in metabolic syndromes were due more to issues in methodology, rather than the result of the GM not being a driver of metabolic diseases. On the other hand, studies investigating probiotics as a treatment for neuropsychiatric disorders have been less conclusive. For example, while a meta-analysis of five clinical trials found that probiotic intervention decreased inflammatory biomarkers and improved cognitive impairment in patients with Alzheimer’s disease and mild cognitive impairment (Den et al., 2020), a different meta-analysis concluded that there is insufficient evidence to support the use of probiotics as a co-treatment for dementia (Krüger et al., 2021). Furthermore, probiotics had little to no impact in a clinical trial of people with mild to moderate depression, although a lack of an effect on the GM after probiotic use suggests that this may have been due to an insufficient dosage (Chahwan et al., 2019). Similarly, one meta-analysis suggested that Lactobacillus-laced probiotics were an effective adjunct treatment for patients with chronic periodontal disease (Matsubara et al., 2016), while another found the statistical evidence to this effect to be unreliable (Yanine et al., 2013).
So far, the biological evidence has uncovered specific bacterial species as of particular importance in suppressing inflammation in the intestine, such as lactic acid–producing bacteria and Bifidobacteria (Saez-Lara et al., 2015). Therefore, many clinical studies have focused on treatment with one or two bacterial strains instead of employing highly variable multi-strain probiotics. For example, Lactobacillus casei alleviated symptoms of acute diarrhea in affected children (Lai et al., 2019), while Bifidobacterium longum NCC3001 had no effect on IBS symptoms but did alleviate depression in patients with IBS (Pinto-Sanchez et al., 2017). In the brain, Lactobacillus plantarum PS128 seems to have a synergistic effect with oxytocin to help social cognition responses in patients with autism (Kong et al., 2021), while Bifidobacterium breve had some positive effects on memory in elderly patients with mild cognitive impairment (Xiao et al., 2020). Furthermore, probiotics in general and a combination Bifidobacterium bifidum BGN4 and Bifidobacterium longum BORI in particular reduced chronic low-grade inflammation and promoted mental flexibility in healthy elderly adults (Custodero et al., 2018; Kim et al., 2021), though no effect on inflammation or mood was found when depressed patients were treated with Lactobacillus helveticus and B. longum (Romijn et al., 2017). Bifidobacterium lactis was also found to help as an adjuvant therapy in reducing inflammation in patients with asthma (Liu et al., 2021). While this approach addresses issues of high variability between multi-strain probiotics, in some cases, treatment with a single strain is not sufficient to elicit the desired robust clinical outcome. For example, while one small study employing Bifidobacterium-fermented milk showed promising results in patients with ulcerative colitis (Ishikawa et al., 2003) and despite the strong preclinical data described above, a meta-analysis of five studies in patients with IBS reported that B. longum 35624 was only efficient in alleviating symptoms when administered with other probiotic bacteria (Yuan et al., 2017).
Despite being less expensive and invasive, clinical studies with probiotic therapy have suffered from many of the same issues as those with FMT, including small patient cohorts, high variability in dosage, and inconsistent monitoring of the GM response to probiotic use. Furthermore, while probiotic treatment is associated with fewer recorded adverse effects than FMT, and despite having been found to have had some success in modulating inflammation in patients with gastric cancer (Yang et al., 2022), probiotic intervention has been linked to rare cases of sepsis in patients with cancer, thus requiring more caution in clinical trials involving the immunocompromised (Redman et al., 2014).
In addition to probiotics, clinical trials have also been conducted with postbiotics, i.e., bacteria-derived metabolites, in patients with inflammatory diseases. However, despite very promising data in rodent models, supplementation with individual SCFAs has had less success in human clinical trials. While consumption of sodium butyrate has been determined to be safe for humans, multiple clinical trials in patients with IBD have resulted in little to no amelioration of symptoms, nor was it effective as a therapeutic strategy in type 1 diabetes (Steinhart et al., 1996; de Groot et al., 2020; Facchin et al., 2020; Jamka et al., 2021). However, these clinical trials differed from studies in animal models in a few key ways. First of all, sodium butyrate was administered to patients with IBD by enema, whereas the previous studies in mice were conducted by butyrate feeding, and another mouse study demonstrated diametrically opposing effects on inflammation by butyrate enema depending on the dosage used (Liu et al., 2020). While the clinical trial conducted with patients with diabetes also administered butyrate orally, it is important to note that all of the previously mentioned preclinical studies demonstrated a protective effect of butyrate when administered before the inflammatory insult occurred. More studies are needed to determine whether, in humans too, butyrate could be used as an effective prophylactic against inflammatory diseases.
How -omics-based characterization and mechanistic insight could shape the future of clinical trials
Since the diversity, complexity, and responses of the gut microbiota to disease states became apparent, much controversy has revolved around whether these microbial communities were markers of disease, or whether they were one of, if not the main drivers of pathology. In recent years, numerous studies have uncovered a causal link between the GM and inflammatory diseases, with our knowledge of the biological mechanisms they employ to do this having come forward by leaps and bounds. Despite these advances, there still exist some significant gaps, both in our understanding of how these bacteria influence disease and in our ability to bridge the gap between the microbiology laboratory and the clinical world, with human trials ending in far less conclusive victories than many of their in vitro or preclinical rodent model counterparts. While it is well known that these models are oversimplified and thus can never fully replicate a human patient, some of these clinical disappointments might, at least in part, be due to unknown and unforeseen confounding factors. In a clinical setting, the biological mechanism via which these bacteria might influence inflammation is often overlooked. While this point of view is understandable in a diagnostic context, where the presence of a certain bacteria can be seen as a pathological red flag regardless of the “why,” it can also limit our ability to develop novel therapeutic strategies.
One of the biggest issues standing in the way of bridging the gap between models and humans is the relatively superficial information that is gleaned from microbiotic profiling of human patients. While laboratory studies on individual bacterial species can have the luxury of a deep characterization of the bacteria in question, down to the peculiarities of the strain and its extracellular chemistry, GM-profiling of human stool samples usually involves identifying the bacteria found at the family or at most the genus level. These methods can give a general overview of the state of the GM in human subjects but is not sufficient to identify specific bacterial markers or drivers of inflammatory diseases, especially in light of the discovery that different strains of the same species can have diametrically opposite effects on inflammation. Given the large heterogeneity uncovered by studies into the biological mechanisms of bacteria-induced inflammation, it is therefore understandable that association studies in humans lacking strain-level information can sometimes be difficult to interpret or reproduce. On the other hand, -omics-based methodologies currently available to characterize the microbiome, exposome, and immunome of patients have improved dramatically in recent years, bringing us ever closer to novel personalized medicine interventional strategies (Figure 1 and Putignani et al., 2019). It must also be noted that, though bacteria have historically been the largest focus of studies in microbiome research, the healthy GM is also composed of viruses, fungi, and archaea that have their own regulatory role in inflammation (Norman et al., 2014). While including these modulators of chronic inflammation was unfortunately beyond the scope of this review, a deeper characterization of the microbiome in this precision medicine model would also ideally not exclude these important players, to better bridge this gap between the lab and the clinic.
This in-depth characterization of the biological mechanisms by which bacteria modulate inflammation has brought to light another significant issue in current clinical trial design. In the case of FMT, donors are selected based on age, gender, and overall metabolic health, and samples are screened for pathogens, but usually little is known about the microbial composition of their GM. While this may be sufficient characterization in the treatment of C. difficile infection, it is likely not enough to adequately choose the most appropriate donor for patients with inflammatory diseases, likely contributing to the uncertain outcomes described previously. Similarly, there is also the often-overlooked variability in the production of probiotics, especially those which are anaerobic. For example, F. prausnitzii, which has gained much interest in recent years as a next-generation probiotic, is also very sensitive to oxygen, which can make the purification of enough live bacteria to make a difference when ingested very tricky (Kim et al., 2020). Variability between production methods can often lead to not only differences in viability of the bacteria but also in effector molecule production, which can be destroyed by industrial methods and are virtually never taken into consideration as quality control markers for probiotic production (Duboux et al., 2021). Given the anti-inflammatory importance of specific bacteria-derived molecules that has come to light in recent years, and which has been discussed previously in this review, a lack of standard protocols in ensuring the integrity of these molecules during probiotic production could well mask beneficial effects on inflammatory diseases conferred by these microbes. Furthermore, in the case of both FMT and probiotic use, standard protocols are not only needed in how the therapeutic agent is treated but also in the confirmation that the therapy had a measurable effect on the GM of the recipient. If the FMT did not graft, or if the probiotic dosage used was too low to ensure the survival and colonization of the bacteria in the gut, then the therapy does not have any chance of having a protective effect. In the case of probiotics, they are sometimes administered in clinical trials together with various non-digestible fiber sources, known as prebiotics, which adds yet another layer of variability between different clinical trials that can make outcomes difficult to interpret or reproduce.
Biological life is inherently variable, which is why those who study it are obligated to adapt their protocols to fit the scenario and employ different statistical methods to ensure that differences measured are significant. However, when high variability is not compensated for by large sample sizes, many potentially significant results can be masked by it. Additionally, the vast methodological variability between different clinical trials has undoubtedly contributed to the lack of reproducibility of some clinical outcomes. While a certain amount of flexibility must exist in clinical trial design to adjust the treatment to the circumstances, a certain amount of standardization could help to reduce uncertainty due to unnecessary variability in study design.
Another source of variability that is not often discussed is the impressive difference in GM composition between individuals. Though it is well known that diet has a strong effect on the gut microbiota, one could argue that a healthy microbial community is associated with a healthy diet, and thus that diet-induced changes to this ideal GM could be considered unhealthy, or dysbiotic. However, geographical location is another important factor that is often overlooked. One study demonstrated that the microbiota of Colombian adults possessed a different microbial signature than that of Americans, Europeans, and Asians, as well as a different set of microbes altered in obese Columbian individuals (Escobar et al., 2014). Consistently, a study on fecal samples from healthy United States and Spanish participants found a significant difference in GM diversity overall and of specific bacterial genera in particular between the two nationalities (Allali et al., 2015). Even within the same country, GM composition was found to be the number one predictor of whether a person came from Northern or Southern China, with geography having a far larger influence on the GM than ethnicity (Lu et al., 2021; Wang et al., 2022). While the diets of different populations in different parts of the world undoubtedly contribute to these differences, these studies still beg the question: what is a healthy GM? Could a “healthy” GM characterization conducted in the United States really be used as a model for a “healthy” European, Chinese, or Brazilian person? When we identify dysbiosis in patients with inflammatory diseases, what are we comparing them too? Given recent evidence, it is far more likely that there are many different compositions of the GM that have evolved over time and that are “healthy,” based on the climate, diet, and genetics the person finds themselves with. Greater consideration of these differences between human populations must be taken, as well as an expansion of available geographically specific data on human GM composition (Figure 1).
As with the majority of human ailments, there is no “one size fits all” answer to the question of how we can alleviate inflammation via the GM, with each method having its up- and downsides. Methods such as FMT and multispecies probiotics have the benefit of taking into account the larger biological picture, since many bacteria need to be balanced and act in concert with others in order to elicit a protective effect. However, this strength can also be considered its greatest weakness, since a lack of standard operating procedure and superficial characterization of the donor/probiotic material also introduces a variability between patients that is not always possible to overcome statistically. On the other hand, treatment with single probiotics or metabolites can help overcome these statistical challenges but not always be effective enough to act on their own. Instead of attempting to find a solution to all of these problems at once, one should instead use the mechanistic knowledge gained to best inform their clinical study design. For example, upon the discovery of the importance of B. longum 3564-derived sEPS in inflammation, any clinical trial conducted with this strain should take the integrity of its extracellular chemistry during probiotic production as a fundamental quality control parameter. With the discovery of the dual role of B. fragilis in inflammation based on a series of different effector molecules it can produce, a safer and more intriguing clinical trial could be conducted on the efficacy of PSA itself, rather than risk worsening symptoms by attempting to treat patients with an insufficiently well-characterized B. fragilis strain. Although designing a clinical trial for every single promising effector molecule is unreasonable, time-consuming, and expensive, the same can be said when FMT- or probiotic-based clinical trials are designed and conducted only to yield inconclusive results. A more well-rounded approach that takes biological mechanism into consideration could help identify key pitfalls and help to design better, more robust clinical studies.
Discussion
It is clear that, at least in some cases, the GM can modulate inflammation both in the intestine and beyond. However, even if, in some disease contexts, dysbiosis is more a marker than a driver of inflammation, there is still much to be gained by a deeper understanding of microbial markers in inflammatory diseases. For example, when does dysbiosis in the gut occur? Does it precede disease onset, or does it occur after symptoms present themselves? Can we, in short, employ our ever-expanding -omics repertoire to design longitudinal studies, with the aim of identifying early microbial markers of inflammatory diseases? By combining our mechanistic knowledge of microbially modulated inflammation with a deeper -omics-based patient GM characterization, designing more personalized therapeutic or prophylactic interventions becomes ever more within our reach (Figure 1).
Author contributions
AP and LP wrote the review. Both authors contributed to the article and approved the submitted version.
Funding
This study was funded by the Ministry of Health (Ricerca Corrente RC2021_Modale_Putignani; 202005_Genetica_Putignani).
Conflict of interest
The authors declare that the research was conducted in the absence of any commercial or financial relationships that could be construed as a potential conflict of interest.
Publisher’s note
All claims expressed in this article are solely those of the authors and do not necessarily represent those of their affiliated organizations, or those of the publisher, the editors and the reviewers. Any product that may be evaluated in this article, or claim that may be made by its manufacturer, is not guaranteed or endorsed by the publisher.
References
Ahn, S. B., Jun, D. W., Kang, B.-K., Lim, J. H., Lim, S., and Chung, M.-J. (2019). Randomized, double-blind, placebo-controlled study of a multispecies probiotic mixture in nonalcoholic fatty liver disease. Sci. Rep. 9:5688. doi: 10.1038/s41598-019-42059-3
Alang, N., and Kelly, C. R. (2015). Weight gain after fecal microbiota transplantation. Open Forum Infect. Dis. 2:ofv004. doi: 10.1093/ofid/ofv004
Allali, I., Delgado, S., Marron, P. I., Astudillo, A., Yeh, J. J., Ghazal, H., et al. (2015). Gut microbiome compositional and functional differences between tumor and non-tumor adjacent tissues from cohorts from the US and Spain. Gut Microbes 6, 161–172. doi: 10.1080/19490976.2015.1039223
Allegretti, J. R., Kassam, Z., Carrellas, M., Mullish, B. H., Marchesi, J. R., Pechlivanis, A., et al. (2019). Fecal microbiota transplantation in patients with primary sclerosing cholangitis: a pilot clinical trial. Am. J. Gastroenterol. 114, 1071–1079. doi: 10.14309/ajg.0000000000000115
Allegretti, J. R., Kassam, Z., Mullish, B. H., Chiang, A., Carrellas, M., Hurtado, J., et al. (2020). Effects of fecal microbiota transplantation with oral capsules in obese patients. Clin. Gastroenterol. Hepatol. 18, 855–863.e2. doi: 10.1016/j.cgh.2019.07.006.
Altmann, F., Kosma, P., O’Callaghan, A., Leahy, S., Bottacini, F., Molloy, E., et al. (2016). Genome analysis and characterisation of the exopolysaccharide produced by Bifidobacterium longum subsp. longum 35624TM. PLoS One 11:e0162983. doi: 10.1371/journal.pone.0162983
Altomare, A., del Chierico, F., Rocchi, G., Emerenziani, S., Nuglio, C., Putignani, L., et al. (2021). Association between dietary habits and fecal microbiota composition in irritable bowel syndrome patients: a pilot study. Nutrients 13:1479. doi: 10.3390/nu13051479
Arthur, J. C., Perez-Chanona, E., Mühlbauer, M., Tomkovich, S., Uronis, J. M., Fan, T.-J., et al. (2012). Intestinal inflammation targets cancer-inducing activity of the microbiota. Science 338, 120–123. doi: 10.1126/science.1224820
Bajaj, J. S., Gavis, E. A., Fagan, A., Wade, J. B., Thacker, L. R., Fuchs, M., et al. (2021). A randomized clinical trial of fecal microbiota transplant for alcohol use disorder. Hepatology 73, 1688–1700. doi: 10.1002/hep.31496
Bajaj, J. S., Kassam, Z., Fagan, A., Gavis, E. A., Liu, E., Cox, I. J., et al. (2017). Fecal microbiota transplant from a rational stool donor improves hepatic encephalopathy: a randomized clinical trial. Hepatology 66, 1727–1738. doi: 10.1002/hep.29306
Bajic, D., Niemann, A., Hillmer, A.-K., Mejias-Luque, R., Bluemel, S., Docampo, M., et al. (2020). Gut microbiota-derived propionate regulates the expression of reg3 mucosal lectins and ameliorates experimental colitis in mice. J. Crohn’s Colitis 14, 1462–1472. doi: 10.1093/ecco-jcc/jjaa065
Balish, E., and Warner, T. (2002). Enterococcus faecalis induces inflammatory bowel disease in interleukin-10 knockout mice. Am. J. Pathol. 160, 2253–2257. doi: 10.1016/S0002-9440(10)61172-8
Bartolomaeus, H., Balogh, A., Yakoub, M., Homann, S., Markó, L., Höges, S., et al. (2019). Short-Chain fatty acid propionate protects from hypertensive cardiovascular damage. Circulation 139, 1407–1421. doi: 10.1161/CIRCULATIONAHA.118.036652
Bastos, R. M. C., Simplício-Filho, A., Sávio-Silva, C., Oliveira, L. F. V., Cruz, G. N. F., Sousa, E. H., et al. (2022). Fecal microbiota transplant in a pre-clinical model of type 2 diabetes mellitus, obesity and diabetic kidney disease. Int. J. Mol. Sci. 23:3842. doi: 10.3390/ijms23073842
Behary, J., Amorim, N., Jiang, X.-T., Raposo, A., Gong, L., McGovern, E., et al. (2021). Gut microbiota impact on the peripheral immune response in non-alcoholic fatty liver disease related hepatocellular carcinoma. Nat. Commun. 12:187. doi: 10.1038/s41467-020-20422-7
Berni Canani, R., Paparo, L., Nocerino, R., di Scala, C., della Gatta, G., Maddalena, Y., et al. (2019). Gut microbiome as target for innovative strategies against food allergy. Front. Immunol. 10:191. doi: 10.3389/fimmu.2019.00191
Blandford, L. E., Johnston, E. L., Sanderson, J. D., Wade, W. G., and Lax, A. J. (2019). Promoter orientation of the immunomodulatory Bacteroides fragilis capsular polysaccharide a (PSA) is off in individuals with inflammatory bowel disease (IBD). Gut Microbes 10, 569–577. doi: 10.1080/19490976.2018.1560755
Bouwman, L. I., de Zoete, M. R., Bleumink-Pluym, N. M. C., Flavell, R. A., and van Putten, J. P. M. (2014). Inflammasome activation by Campylobacter jejuni. J. Immunol. 193, 4548–4557. doi: 10.4049/jimmunol.1400648
Bunyavanich, S., and Berin, M. C. (2019). Food allergy and the microbiome: current understandings and future directions. J. Allergy Clin. Immunol. 144, 1468–1477. doi: 10.1016/j.jaci.2019.10.019
Burgos-Portugal, J. A., Mitchell, H. M., Castaño-Rodríguez, N., and Kaakoush, N. O. (2014). The role of autophagy in the intracellular survival of Campylobacter concisus. FEBS Open Bio 4, 301–309. doi: 10.1016/j.fob.2014.03.008
Cammarota, G., Ianiro, G., Kelly, C. R., Mullish, B. H., Allegretti, J. R., Kassam, Z., et al. (2019). International consensus conference on stool banking for faecal microbiota transplantation in clinical practice. Gut 68, 2111–2121. doi: 10.1136/gutjnl-2019-319548
Cao, J., Amakye, W. K., Qi, C., Liu, X., Ma, J., and Ren, J. (2021). Bifidobacterium lactis Probio-M8 regulates gut microbiota to alleviate Alzheimer’s disease in the APP/PS1 mouse model. Eur. J. Nutr. 60, 3757–3769. doi: 10.1007/s00394-021-02543-x
Carroll, I. M., Chang, Y.-H., Park, J., Sartor, R. B., and Ringel, Y. (2010). Luminal and mucosal-associated intestinal microbiota in patients with diarrhea-predominant irritable bowel syndrome. Gut Pathogens 2:19. doi: 10.1186/1757-4749-2-19
Castaño-Rodríguez, N., Kaakoush, N. O., Lee, W. S., and Mitchell, H. M. (2017). Dual role of Helicobacter and Campylobacter species in IBD: a systematic review and meta-analysis. Gut 66, 235–249. doi: 10.1136/gutjnl-2015-310545
Chahwan, B., Kwan, S., Isik, A., van Hemert, S., Burke, C., and Roberts, L. (2019). Gut feelings: a randomised, triple-blind, placebo-controlled trial of probiotics for depressive symptoms. J. Affect. Disord. 253, 317–326. doi: 10.1016/j.jad.2019.04.097
Chen, D., Jin, D., Huang, S., Wu, J., Xu, M., Liu, T., et al. (2020a). Clostridium butyricum, a butyrate-producing probiotic, inhibits intestinal tumor development through modulating Wnt signaling and gut microbiota. Cancer Lett. 469, 456–467. doi: 10.1016/j.canlet.2019.11.019
Chen, G., Ran, X., Li, B., Li, Y., He, D., Huang, B., et al. (2018). Sodium butyrate inhibits inflammation and maintains epithelium barrier integrity in a TNBS-induced inflammatory bowel disease mice model. EBioMedicine 30, 317–325. doi: 10.1016/j.ebiom.2018.03.030
Chen, H., Ma, X., Liu, Y., Ma, L., Chen, Z., Lin, X., et al. (2019). Gut microbiota interventions with Clostridium butyricum and norfloxacin modulate immune response in experimental autoimmune encephalomyelitis mice. Front. Immunol. 10:1662. doi: 10.3389/fimmu.2019.01662
Chen, J., Pitmon, E., and Wang, K. (2017). Microbiome, inflammation and colorectal cancer. Semin. Immunol. 32, 43–53. doi: 10.1016/j.smim.2017.09.006
Chen, W., Zhang, S., Wu, J., Ye, T., Wang, S., Wang, P., et al. (2020b). Butyrate-producing bacteria and the gut-heart axis in atherosclerosis. Clin. Chim. Acta Int. J. Clin. Chem. 507, 236–241. doi: 10.1016/j.cca.2020.04.037
Cheng, Y., Ling, Z., and Li, L. (2020). The intestinal microbiota and colorectal cancer. Front. Immunol. 11:615056. doi: 10.3389/fimmu.2020.615056
Choi, E.-J., Lee, H. J., Kim, W.-J., Han, K.-I., Iwasa, M., Kobayashi, K., et al. (2019). Enterococcus faecalis EF-2001 protects DNBS-induced inflammatory bowel disease in mice model. PLoS One 14:e0210854. doi: 10.1371/journal.pone.0210854
Chung, H. K. L., Tay, A., Octavia, S., Chen, J., Liu, F., Ma, R., et al. (2016). Genome analysis of Campylobacter concisus strains from patients with inflammatory bowel disease and gastroenteritis provides new insights into pathogenicity. Sci. Rep. 6:38442. doi: 10.1038/srep38442
Ciacci-Woolwine, F., Blomfield, I. C., Richardson, S. H., and Mizel, S. B. (1998). Salmonella flagellin induces tumor necrosis factor alpha in a human promonocytic cell line. Infect. Immun. 66, 1127–1134. doi: 10.1128/IAI.66.3.1127-1134.1998
Coffey, M. J., Garg, M., Homaira, N., Jaffe, A., and Ooi, C. Y. (2020). Probiotics for people with cystic fibrosis. Cochrane Database Systematic Rev. 1:CD012949. doi: 10.1002/14651858.CD012949.pub2
Conte, M. P., Schippa, S., Zamboni, I., Penta, M., Chiarini, F., Seganti, L., et al. (2006). Gut-associated bacterial microbiota in paediatric patients with inflammatory bowel disease. Gut 55, 1760–1767. doi: 10.1136/gut.2005.078824
Costa, R. F. A., Ferrari, M. L. A., Bringer, M.-A., Darfeuille-Michaud, A., Martins, F. S., and Barnich, N. (2020). Characterization of mucosa-associated Escherichia coli strains isolated from Crohn’s disease patients in Brazil. BMC Microbiol. 20:178. doi: 10.1186/s12866-020-01856-x
Costello, S. P., Hughes, P. A., Waters, O., Bryant, R. V., Vincent, A. D., Blatchford, P., et al. (2019). Effect of fecal microbiota transplantation on 8-week remission in patients with ulcerative colitis: a randomized clinical trial. JAMA 321, 156–164. doi: 10.1001/jama.2018.20046
Craven, L., Rahman, A., Nair Parvathy, S., Beaton, M., Silverman, J., Qumosani, K., et al. (2020). Allogenic fecal microbiota transplantation in patients with nonalcoholic fatty liver disease improves abnormal small intestinal permeability: a randomized control trial. Am. J. Gastroenterol. 115, 1055–1065. doi: 10.14309/ajg.0000000000000661
Custodero, C., Mankowski, R. T., Lee, S. A., Chen, Z., Wu, S., Manini, T. M., et al. (2018). Evidence-based nutritional and pharmacological interventions targeting chronic low-grade inflammation in middle-age and older adults: a systematic review and meta-analysis. Ageing Res. Rev. 46, 42–59. doi: 10.1016/j.arr.2018.05.004
Dasgupta, S., Erturk-Hasdemir, D., Ochoa-Reparaz, J., Reinecker, H.-C., and Kasper, D. L. (2014). Plasmacytoid dendritic cells mediate anti-inflammatory responses to a gut commensal molecule via both innate and adaptive mechanisms. Cell Host Microbe 15, 413–423. doi: 10.1016/j.chom.2014.03.006
Davar, D., Dzutsev, A. K., McCulloch, J. A., Rodrigues, R. R., Chauvin, J.-M., Morrison, R. M., et al. (2021). Fecal microbiota transplant overcomes resistance to anti-PD-1 therapy in melanoma patients. Science 371, 595–602. doi: 10.1126/science.abf3363
de Groot, P., Nikolic, T., Pellegrini, S., Sordi, V., Imangaliyev, S., Rampanelli, E., et al. (2021). Faecal microbiota transplantation halts progression of human new-onset type 1 diabetes in a randomised controlled trial. Gut 70, 92–105. doi: 10.1136/gutjnl-2020-322630
de Groot, P. F., Nikolic, T., Imangaliyev, S., Bekkering, S., Duinkerken, G., Keij, F. M., et al. (2020). Oral butyrate does not affect innate immunity and islet autoimmunity in individuals with longstanding type 1 diabetes: a randomised controlled trial. Diabetologia 63, 597–610. doi: 10.1007/s00125-019-05073-8
DeFilipp, Z., Bloom, P. P., Torres Soto, M., Mansour, M. K., Sater, M. R. A., Huntley, M. H., et al. (2019). Drug-Resistant E. coli bacteremia transmitted by fecal microbiota transplant. New England J. Med. 381, 2043–2050. doi: 10.1056/NEJMoa1910437
Dejea, C. M., Fathi, P., Craig, J. M., Boleij, A., Taddese, R., Geis, A. L., et al. (2018). Patients with familial adenomatous polyposis harbor colonic biofilms containing tumorigenic bacteria. Science 359, 592–597. doi: 10.1126/science.aah3648
del Chierico, F., Abbatini, F., Russo, A., Quagliariello, A., Reddel, S., Capoccia, D., et al. (2018). Gut microbiota markers in obese adolescent and adult patients: age-dependent differential patterns. Front. Microbiol. 9:1210. doi: 10.3389/fmicb.2018.01210
del Chierico, F., Gnani, D., Vernocchi, P., Petrucca, A., Alisi, A., Dallapiccola, B., et al. (2014). Meta-omic platforms to assist in the understanding of NAFLD gut microbiota alterations: tools and applications. Int. J. Mol. Sci. 15, 684–711. doi: 10.3390/ijms15010684
del Chierico, F., Manco, M., Gardini, S., Guarrasi, V., Russo, A., Bianchi, M., et al. (2021). Fecal microbiota signatures of insulin resistance, inflammation, and metabolic syndrome in youth with obesity: a pilot study. Acta Diabetol. 58, 1009–1022. doi: 10.1007/s00592-020-01669-4
Demirci, M., Tokman, H. B., Uysal, H. K., Demiryas, S., Karakullukcu, A., Saribas, S., et al. (2019). Reduced Akkermansia muciniphila and Faecalibacterium prausnitzii levels in the gut microbiota of children with allergic asthma. Allergol. Immunopathol. 47, 365–371. doi: 10.1016/j.aller.2018.12.009
Den, H., Dong, X., Chen, M., and Zou, Z. (2020). Efficacy of probiotics on cognition, and biomarkers of inflammation and oxidative stress in adults with Alzheimer’s disease or mild cognitive impairment - a meta-analysis of randomized controlled trials. Aging 12, 4010–4039. doi: 10.18632/aging.102810
Deng, M., Qu, F., Chen, L., Liu, C., Zhang, M., Ren, F., et al. (2020). SCFAs alleviated steatosis and inflammation in mice with NASH induced by MCD. J. Endocrinol. 245, 425–437. doi: 10.1530/JOE-20-0018
Deshpande, N. P., Kaakoush, N. O., Wilkins, M. R., and Mitchell, H. M. (2013). Comparative genomics of Campylobacter concisus isolates reveals genetic diversity and provides insights into disease association. BMC Genomics 14:585. doi: 10.1186/1471-2164-14-585
d’Ettorre, G., Ceccarelli, G., Giustini, N., Serafino, S., Calantone, N., de Girolamo, G., et al. (2015). Probiotics reduce inflammation in antiretroviral treated, HIV-Infected individuals: results of the “Probio-HIV”. clinical trial. PLoS One 10:e0137200. doi: 10.1371/journal.pone.0137200
Dou, X., Gao, N., Yan, D., and Shan, A. (2020). Sodium butyrate alleviates mouse colitis by regulating gut microbiota dysbiosis. Animals Open Access J. MDPI 10:1154. doi: 10.3390/ani10071154
Duboux, S., van Wijchen, M., and Kleerebezem, M. (2021). The possible link between manufacturing and probiotic efficacy; a molecular point of view on bifidobacterium. Front. Microbiol. 12:812536. doi: 10.3389/fmicb.2021.812536
Eaves-Pyles, T., Murthy, K., Liaudet, L., Virág, L., Ross, G., Soriano, F. G., et al. (2001). Flagellin, a novel mediator of Salmonella-induced epithelial activation and systemic inflammation: I kappa B alpha degradation, induction of nitric oxide synthase, induction of proinflammatory mediators, and cardiovascular dysfunction. J. Immunol. 166, 1248–1260. doi: 10.4049/jimmunol.166.2.1248
Ehrlich, A. M., Pacheco, A. R., Henrick, B. M., Taft, D., Xu, G., Huda, M. N., et al. (2020). Indole-3-lactic acid associated with Bifidobacterium-dominated microbiota significantly decreases inflammation in intestinal epithelial cells. BMC Microbiol. 20:357. doi: 10.1186/s12866-020-02023-y
El-Salhy, M., Hatlebakk, J. G., Gilja, O. H., Bråthen Kristoffersen, A., and Hausken, T. (2020). Efficacy of faecal microbiota transplantation for patients with irritable bowel syndrome in a randomised, double-blind, placebo-controlled study. Gut 69, 859–867. doi: 10.1136/gutjnl-2019-319630
Escobar, J. S., Klotz, B., Valdes, B. E., and Agudelo, G. M. (2014). The gut microbiota of Colombians differs from that of Americans. Europeans and Asians. BMC Microbiol. 14:311. doi: 10.1186/s12866-014-0311-6
Facchin, S., Vitulo, N., Calgaro, M., Buda, A., Romualdi, C., Pohl, D., et al. (2020). Microbiota changes induced by microencapsulated sodium butyrate in patients with inflammatory bowel disease. Neurogastroenterol. Motility 32:e13914. doi: 10.1111/nmo.13914
Fanning, S., Hall, L. J., Cronin, M., Zomer, A., MacSharry, J., Goulding, D., et al. (2012). Bifidobacterial surface-exopolysaccharide facilitates commensal-host interaction through immune modulation and pathogen protection. Proc. Natl. Acad. Sci. U S A. 109, 2108–2113. doi: 10.1073/pnas.1115621109
Felizardo, R. J. F., de Almeida, D. C., Pereira, R. L., Watanabe, I. K. M., Doimo, N. T. S., Ribeiro, W. R., et al. (2019). Gut microbial metabolite butyrate protects against proteinuric kidney disease through epigenetic- and GPR109a-mediated mechanisms. FASEB J. 33, 11894–11908. doi: 10.1096/fj.201901080R
Fritsch, J., Garces, L., Quintero, M. A., Pignac-Kobinger, J., Santander, A. M., Fernández, I., et al. (2021). Low-Fat, high-fiber diet reduces markers of inflammation and dysbiosis and improves quality of life in patients with ulcerative colitis. Clin. Gastroenterol. Hepatol. 19, 1189–1199.e30. doi: 10.1016/j.cgh.2020.05.026
Fujita, H., Eishi, Y., Ishige, I., Saitoh, K., Takizawa, T., Arima, T., et al. (2002). Quantitative analysis of bacterial DNA from Mycobacteria spp., Bacteroides vulgatus, and Escherichia coli in tissue samples from patients with inflammatory bowel diseases. J. Gastroenterol. 37, 509–516. doi: 10.1007/s005350200079
Fyderek, K., Strus, M., Kowalska-Duplaga, K., Gosiewski, T., Wedrychowicz, A., Jedynak-Wasowicz, U., et al. (2009). Mucosal bacterial microflora and mucus layer thickness in adolescents with inflammatory bowel disease. World J. Gastroenterol. 15, 5287–5294. doi: 10.3748/wjg.15.5287
Gagnière, J., Raisch, J., Veziant, J., Barnich, N., Bonnet, R., Buc, E., et al. (2016). Gut microbiota imbalance and colorectal cancer. World J. Gastroenterol. 22, 501–518. doi: 10.3748/wjg.v22.i2.501
Galligan, J. J. (2018). Beneficial actions of microbiota-derived tryptophan metabolites. Neurogastroenterol. Motility 30:e13283. doi: 10.1111/nmo.13283
Gasaly, N., de Vos, P., and Hermoso, M. A. (2021). Impact of bacterial metabolites on gut barrier function and host immunity: a focus on bacterial metabolism and its relevance for intestinal inflammation. Front. Immunol. 12:658354. doi: 10.3389/fimmu.2021.658354
Gelpi, M., Ueland, P. M., Trøseid, M., Mocroft, A., Lebech, A.-M., Ullum, H., et al. (2020). Abdominal adipose tissue is associated with alterations in tryptophan-kynurenine metabolism and markers of systemic inflammation in people with human immunodeficiency virus. J. Infect. Dis. 221, 419–427. doi: 10.1093/infdis/jiz465
Glassner, K. L., Abraham, B. P., and Quigley, E. M. M. (2020). The microbiome and inflammatory bowel disease. J. Allergy Clin. Immunol. 145, 16–27. doi: 10.1016/j.jaci.2019.11.003
Golińska, E., Tomusiak, A., Gosiewski, T., Wiêcek, G., Machul, A., Mikołajczyk, D., et al. (2013). Virulence factors of Enterococcus strains isolated from patients with inflammatory bowel disease. World J. Gastroenterol. 19, 3562–3572. doi: 10.3748/wjg.v19.i23.3562
Gopal, A., Chidambaram, I. S., Devaraj, N., and Devaraj, H. (2017). Shigella dysenteriae infection activates proinflammatory response through β-catenin/NF-κB signaling pathway. PLoS One 12:e0174943. doi: 10.1371/journal.pone.0174943
Groeger, D., O’Mahony, L., Murphy, E. F., Bourke, J. F., Dinan, T. G., Kiely, B., et al. (2013). Bifidobacterium infantis 35624 modulates host inflammatory processes beyond the gut. Gut Microbes 4, 325–339. doi: 10.4161/gmic.25487
Groeger, D., Schiavi, E., Grant, R., Kurnik-Łucka, M., Michalovich, D., Williamson, R., et al. (2020). Intranasal Bifidobacterium longum protects against viral-induced lung inflammation and injury in a murine model of lethal influenza infection. EBioMedicine 60:102981. doi: 10.1016/j.ebiom.2020.102981
Guerry, P., Szymanski, C. M., Prendergast, M. M., Hickey, T. E., Ewing, C. P., Pattarini, D. L., et al. (2002). Phase variation of Campylobacter jejuni 81-176 lipooligosaccharide affects ganglioside mimicry and invasiveness in vitro. Infect. Immun. 70, 787–793. doi: 10.1128/IAI.70.2.787-793.2002
Guo, Z., Zhang, J., Wang, Z., Ang, K. Y., Huang, S., Hou, Q., et al. (2016). Intestinal microbiota distinguish gout patients from healthy humans. Sci. Rep. 6:20602. doi: 10.1038/srep20602
Haghikia, A., Zimmermann, F., Schumann, P., Jasina, A., Roessler, J., Schmidt, D., et al. (2022). Propionate attenuates atherosclerosis by immune-dependent regulation of intestinal cholesterol metabolism. Eur. Heart J. 43, 518–533. doi: 10.1093/eurheartj/ehab644
Hajifaraji, M., Jahanjou, F., Abbasalizadeh, F., Aghamohammadzadeh, N., Abbasi, M. M., and Dolatkhah, N. (2018). Effect of probiotic supplements in women with gestational diabetes mellitus on inflammation and oxidative stress biomarkers: a randomized clinical trial. Asia Pac. J. Clin. Nutr. 27, 581–591.
Hall, A. B., Yassour, M., Sauk, J., Garner, A., Jiang, X., Arthur, T., et al. (2017). A novel Ruminococcus gnavus clade enriched in inflammatory bowel disease patients. Genome Med. 9:103. doi: 10.1186/s13073-017-0490-5
Hansen, R., Berry, S. H., Mukhopadhya, I., Thomson, J. M., Saunders, K. A., Nicholl, C. E., et al. (2013). The microaerophilic microbiota of de-novo paediatric inflammatory bowel disease: the BISCUIT study. PLoS One 8:e58825. doi: 10.1371/journal.pone.0058825
Hao, Z., Wang, W., Guo, R., and Liu, H. (2019). “Faecalibacterium prausnitzii (ATCC 27766) has preventive and therapeutic effects on chronic unpredictable mild stress-induced depression-like and anxiety-like behavior in rats.” Psychoneuroendocrinology 104, 132–142. doi: 10.1016/j.psyneuen.2019.02.025
Harsch, I. A., and Konturek, P. C. (2019). Adhesion ileus after fecal microbiota transplantation in long-standing radiation colitis. Case Rep. Gastrointestinal Med. 2019:2543808. doi: 10.1155/2019/2543808
Haynie, T., Gubler, S., Drees, C., Heaton, T., Mitton, T., Gleave, Q., et al. (2021). Synthesis of the pentasaccharide repeating unit from Ruminococcus gnavus and measurement of its inflammatory properties. RSC Adv. 11, 14357–14361. doi: 10.1039/d1ra01918j
He, Z., Gharaibeh, R. Z., Newsome, R. C., Pope, J. L., Dougherty, M. W., Tomkovich, S., et al. (2019). Campylobacter jejuni promotes colorectal tumorigenesis through the action of cytolethal distending toxin. Gut 68, 289–300. doi: 10.1136/gutjnl-2018-317200
Henke, M. T., Brown, E. M., Cassilly, C. D., Vlamakis, H., Xavier, R. J., and Clardy, J. (2021). Capsular polysaccharide correlates with immune response to the human gut microbe Ruminococcus gnavus. Proc. Natl. Acad. Sci. U S A. 118:e2007595118. doi: 10.1073/pnas.2007595118
Henke, M. T., Kenny, D. J., Cassilly, C. D., Vlamakis, H., Xavier, R. J., and Clardy, J. (2019). Ruminococcus gnavus, a member of the human gut microbiome associated with Crohn’s disease, produces an inflammatory polysaccharide. Proc. Natl. Acad. Sci. U S A. 116, 12672–12677. doi: 10.1073/pnas.1904099116
Hong, J., Jia, Y., Pan, S., Jia, L., Li, H., Han, Z., et al. (2016). Butyrate alleviates high fat diet-induced obesity through activation of adiponectin-mediated pathway and stimulation of mitochondrial function in the skeletal muscle of mice. Oncotarget 7, 56071–56082. doi: 10.18632/oncotarget.11267
Hu, W., Lu, W., Li, L., Zhang, H., Lee, Y.-K., Chen, W., et al. (2021). Both living and dead Faecalibacterium prausnitzii alleviate house dust mite-induced allergic asthma through the modulation of gut microbiota and short-chain fatty acid production. J. Sci. Food Agriculture 101, 5563–5573. doi: 10.1002/jsfa.11207
Huang, Y., Gao, S., Chen, J., Albrecht, E., Zhao, R., and Yang, X. (2017). Maternal butyrate supplementation induces insulin resistance associated with enhanced intramuscular fat deposition in the offspring. Oncotarget 8, 13073–13084. doi: 10.18632/oncotarget.14375
Iglesias-Vázquez, L., van Ginkel Riba, G., Arija, V., and Canals, J. (2020). Composition of gut microbiota in children with autism spectrum disorder: a systematic review and meta-analysis. Nutrients 12:792. doi: 10.3390/nu12030792
Ikram, J., Alamgeer-Muhammad Irfan, H., Akram, M., Hussain Asim, M., Hadal Alotaibi, N., Saad Alharbi, K., et al. (2021). Ethyl-acetate extract of tara mira (Eruca sativa) alleviates the inflammation and rheumatoid arthritis in rats. Pakistan J. Pharmaceutical Sci. 34(5 Suppl.ary), 1897–1902.
Ishikawa, H., Akedo, I., Umesaki, Y., Tanaka, R., Imaoka, A., and Otani, T. (2003). Randomized controlled trial of the effect of bifidobacteria-fermented milk on ulcerative colitis. J. Am. Coll. Nutr. 22, 56–63. doi: 10.1080/07315724.2003.10719276
Jamka, M., Kokot, M., Kaczmarek, N., Bermagambetova, S., Nowak, J. K., and Walkowiak, J. (2021). The effect of sodium butyrate enemas compared with placebo on disease activity, endoscopic scores, and histological and inflammatory parameters in inflammatory bowel diseases: a systematic review of randomised controlled trials. Complementary Med. Res. 28, 344–356. doi: 10.1159/000512952
Jones, S. E., Paynich, M. L., Kearns, D. B., and Knight, K. L. (2014). Protection from intestinal inflammation by bacterial exopolysaccharides. J. Immunol. 192, 4813–4820. doi: 10.4049/jimmunol.1303369
Joossens, M., Huys, G., Cnockaert, M., de Preter, V., Verbeke, K., Rutgeerts, P., et al. (2011). Dysbiosis of the faecal microbiota in patients with Crohn’s disease and their unaffected relatives. Gut 60, 631–637. doi: 10.1136/gut.2010.223263
Kaakoush, N. O., Deshpande, N. P., Wilkins, M. R., Tan, C. G., Burgos-Portugal, J. A., Raftery, M. J., et al. (2011). The pathogenic potential of Campylobacter concisus strains associated with chronic intestinal diseases. PLoS One 6:e29045. doi: 10.1371/journal.pone.0029045
Kanauchi, O., Suga, T., Tochihara, M., Hibi, T., Naganuma, M., Homma, T., et al. (2002). Treatment of ulcerative colitis by feeding with germinated barley foodstuff: first report of a multicenter open control trial. J. Gastroenterol. 37, (Suppl. 1), 67–72. doi: 10.1007/BF03326417
Kang, D.-W., Adams, J. B., Gregory, A. C., Borody, T., Chittick, L., Fasano, A., et al. (2017). Microbiota transfer therapy alters gut ecosystem and improves gastrointestinal and autism symptoms: an open-label study. Microbiome 5:10. doi: 10.1186/s40168-016-0225-7
Kao, D., Roach, B., Silva, M., Beck, P., Rioux, K., Kaplan, G. G., et al. (2017). Effect of oral capsule- vs colonoscopy-delivered fecal microbiota transplantation on recurrent clostridium difficile infection: a randomized clinical trial. JAMA 318, 1985–1993. doi: 10.1001/jama.2017.17077
Kerckhoffs, A. P. M., Samsom, M., van der Rest, M. E., de Vogel, J., Knol, J., Ben-Amor, K., et al. (2009). Lower Bifidobacteria counts in both duodenal mucosa-associated and fecal microbiota in irritable bowel syndrome patients. World J. Gastroenterol. 15, 2887–2892. doi: 10.3748/wjg.15.2887
Kim, C.-S., Cha, L., Sim, M., Jung, S., Chun, W. Y., Baik, H. W., et al. (2021). Probiotic supplementation improves cognitive function and mood with changes in gut microbiota in community-dwelling older adults: a randomized, double-blind, placebo-controlled, multicenter trial. J. Gerontol. A Biol. Sci. Med. Sci. 76, 32–40. doi: 10.1093/gerona/glaa090
Kim, H., Jeong, Y., Kang, S., You, H. J., and Ji, G. E. (2020). Co-Culture with Bifidobacterium catenulatum improves the growth, gut colonization, and butyrate production of Faecalibacterium prausnitzii: in vitro and in vivo studies. Microorganisms 8:788. doi: 10.3390/microorganisms8050788
Kishikawa, T., Maeda, Y., Nii, T., Motooka, D., Matsumoto, Y., Matsushita, M., et al. (2020). Metagenome-wide association study of gut microbiome revealed novel aetiology of rheumatoid arthritis in the Japanese population. Ann. Rheum. Dis. 79, 103–111. doi: 10.1136/annrheumdis-2019-215743
Kong, X.-J., Liu, J., Liu, K., Koh, M., Sherman, H., Liu, S., et al. (2021). Probiotic and oxytocin combination therapy in patients with autism spectrum disorder: a randomized. double-blinded, placebo-controlled pilot trial. Nutrients 13:1552. doi: 10.3390/nu13051552
Konieczna, P., Groeger, D., Ziegler, M., Frei, R., Ferstl, R., Shanahan, F., et al. (2012). Bifidobacterium infantis 35624 administration induces Foxp3 T regulatory cells in human peripheral blood: potential role for myeloid and plasmacytoid dendritic cells. Gut 61, 354–366. doi: 10.1136/gutjnl-2011-300936
Konig, M. F. (2020). The microbiome in autoimmune rheumatic disease. best practice & research. Clin. Rheumatol. 34:101473. doi: 10.1016/j.berh.2019.101473
Koopman, M., and el Aidy, S. (2017). Depressed gut? the microbiota-diet-inflammation trialogue in depression. Curr. Opin. Psychiatry 30, 369–377. doi: 10.1097/YCO.0000000000000350
Kostic, A. D., Chun, E., Robertson, L., Glickman, J. N., Gallini, C. A., Michaud, M., et al. (2013). Fusobacterium nucleatum potentiates intestinal tumorigenesis and modulates the tumor-immune microenvironment. Cell Host Microbe 14, 207–215. doi: 10.1016/j.chom.2013.07.007
Kostic, A. D., Xavier, R. J., and Gevers, D. (2014). The microbiome in inflammatory bowel disease: current status and the future ahead. Gastroenterology 146, 1489–1499. doi: 10.1053/j.gastro.2014.02.009
Kowalska-Duplaga, K., Gosiewski, T., Kapusta, P., Sroka-Oleksiak, A., Wêdrychowicz, A., Pieczarkowski, S., et al. (2019). Differences in the intestinal microbiome of healthy children and patients with newly diagnosed Crohn’s disease. Sci. Rep. 9:18880. doi: 10.1038/s41598-019-55290-9
Krüger, J. F., Hillesheim, E., Pereira, A. C. S. N., Camargo, C. Q., and Rabito, E. I. (2021). Probiotics for dementia: a systematic review and meta-analysis of randomized controlled trials. Nutr. Rev. 79, 160–170. doi: 10.1093/nutrit/nuaa037
Kulkarni, P., Devkumar, P., and Chattopadhyay, I. (2021). Could dysbiosis of inflammatory and anti-inflammatory gut bacteria have an implications in the development of type 2 diabetes? a pilot investigation. BMC Res. Notes 14:52. doi: 10.1186/s13104-021-05466-2
Kunde, S., Pham, A., Bonczyk, S., Crumb, T., Duba, M., Conrad, H. J., et al. (2013). Safety, tolerability, and clinical response after fecal transplantation in children and young adults with ulcerative colitis. J. Pediatr. Gastroenterol. Nutr. 56, 597–601. doi: 10.1097/MPG.0b013e318292fa0d
Lai, H.-H., Chiu, C.-H., Kong, M.-S., Chang, C.-J., and Chen, C.-C. (2019). Probiotic Lactobacillus casei: effective for managing childhood diarrhea by altering gut microbiota and attenuating fecal inflammatory markers. Nutrients 11:1150. doi: 10.3390/nu11051150
Lapiere, A., Geiger, M., Robert, V., Demarquay, C., Auger, S., Chadi, S., et al. (2020). Prophylactic Faecalibacterium prausnitzii treatment prevents the acute breakdown of colonic epithelial barrier in a preclinical model of pelvic radiation disease. Gut Microbes 12, 1–15. doi: 10.1080/19490976.2020.1812867
Leong, K. S. W., Jayasinghe, T. N., Wilson, B. C., Derraik, J. G. B., Albert, B. B., Chiavaroli, V., et al. (2020). Effects of fecal microbiome transfer in adolescents with obesity: the gut bugs randomized controlled trial. JAMA Network Open 3:e2030415. doi: 10.1001/jamanetworkopen.2020.30415
Lewis, S. B., Prior, A., Ellis, S. J., Cook, V., Chan, S. S. M., Gelson, W., et al. (2016). Flagellin induces β-Defensin 2 in human colonic ex vivo infection with enterohemorrhagic Escherichia coli. Front. Cell. Infect. Microbiol. 6:68. doi: 10.3389/fcimb.2016.00068
Lin, C.-D., Lai, C.-K., Lin, Y.-H., Hsieh, J.-T., Sing, Y.-T., Chang, Y.-C., et al. (2011). Cholesterol depletion reduces entry of Campylobacter jejuni cytolethal distending toxin and attenuates intoxication of host cells. Infect. Immun. 79, 3563–3575. doi: 10.1128/IAI.05175-11
Lippert, E., Karrasch, T., Sun, X., Allard, B., Herfarth, H. H., Threadgill, D., et al. (2009). Gnotobiotic IL-10; NF-kappaB mice develop rapid and severe colitis following Campylobacter jejuni infection. PLoS One 4:e7413. doi: 10.1371/journal.pone.0007413
Liu, A., Ma, T., Xu, N., Jin, H., Zhao, F., Kwok, L.-Y., et al. (2021). Adjunctive probiotics alleviates asthmatic symptoms via modulating the gut microbiome and serum metabolome. Microbiol. Spectrum 9:e0085921. doi: 10.1128/Spectrum.00859-21
Liu, H.-N., Wu, H., Chen, Y.-Z., Chen, Y.-J., Shen, X.-Z., and Liu, T.-T. (2017). Altered molecular signature of intestinal microbiota in irritable bowel syndrome patients compared with healthy controls: a systematic review and meta-analysis. Digestive Liver Dis. 49, 331–337. doi: 10.1016/j.dld.2017.01.142
Liu, J., Zhu, H., Li, B., Lee, C., Alganabi, M., Zheng, S., et al. (2020). Beneficial effects of butyrate in intestinal injury. J. Pediatr. Surg. 55, 1088–1093. doi: 10.1016/j.jpedsurg.2020.02.036
lo Presti, A., Zorzi, F., del Chierico, F., Altomare, A., Cocca, S., Avola, A., et al. (2019). Fecal and mucosal microbiota profiling in irritable bowel syndrome and inflammatory bowel disease. Front. Microbiol. 10:1655. doi: 10.3389/fmicb.2019.01655
Lopetuso, L. R., Quagliariello, A., Schiavoni, M., Petito, V., Russo, A., Reddel, S., et al. (2020). Towards a disease-associated common trait of gut microbiota dysbiosis: the pivotal role of Akkermansia muciniphila. Digestive Liver Dis. 52, 1002–1010. doi: 10.1016/j.dld.2020.05.020
Louwen, R., Heikema, A., van Belkum, A., Ott, A., Gilbert, M., Ang, W., et al. (2008). The sialylated lipooligosaccharide outer core in Campylobacter jejuni is an important determinant for epithelial cell invasion. Infect. Immun. 76, 4431–4438. doi: 10.1128/IAI.00321-08
Lu, J., Zhang, L., Zhai, Q., Zhao, J., Zhang, H., Lee, Y.-K., et al. (2021). Chinese gut microbiota and its associations with staple food type, ethnicity, and urbanization. NPJ Biofilms Microbiomes 7:71. doi: 10.1038/s41522-021-00245-0
Lucas, C., Barnich, N., and Nguyen, H. T. T. (2017). Microbiota, inflammation and colorectal cancer. Int. J. Mol. Sci. 18:1310. doi: 10.3390/ijms18061310
Lv, B.-M., Quan, Y., and Zhang, H.-Y. (2021). Causal inference in microbiome medicine: principles and applications. Trends Microbiol. 29, 736–746. doi: 10.1016/j.tim.2021.03.015
Lyons, A., O’Mahony, D., O’Brien, F., MacSharry, J., Sheil, B., Ceddia, M., et al. (2010). Bacterial strain-specific induction of Foxp3+ T regulatory cells is protective in murine allergy models. Clin. Exp. Allergy? 40, 811–819. doi: 10.1111/j.1365-2222.2009.03437.x
Maeda, Y., Kurakawa, T., Umemoto, E., Motooka, D., Ito, Y., Gotoh, K., et al. (2016). Dysbiosis contributes to arthritis development via activation of autoreactive t cells in the intestine. Arthritis Rheumatol. 68, 2646–2661. doi: 10.1002/art.39783
Mahendran, V., Liu, F., Riordan, S. M., Grimm, M. C., Tanaka, M. M., and Zhang, L. (2016). Examination of the effects of Campylobacter concisus zonula occludens toxin on intestinal epithelial cells and macrophages. Gut Pathogens 8:18. doi: 10.1186/s13099-016-0101-9
Mahendran, V., Riordan, S. M., Grimm, M. C., Tran, T. A. T., Major, J., Kaakoush, N. O., et al. (2011). Prevalence of Campylobacter species in adult Crohn’s disease and the preferential colonization sites of Campylobacter species in the human intestine. PLoS One 6:e25417. doi: 10.1371/journal.pone.0025417
Man, S. M., Kaakoush, N. O., Leach, S. T., Nahidi, L., Lu, H. K., Norman, J., et al. (2010). Host attachment, invasion, and stimulation of proinflammatory cytokines by Campylobacter concisus and other non-Campylobacter jejuni Campylobacter species. J. Infect. Dis. 202, 1855–1865. doi: 10.1086/657316
Manco, M., Putignani, L., and Bottazzo, G. F. (2010). Gut microbiota, lipopolysaccharides, and innate immunity in the pathogenesis of obesity and cardiovascular risk. Endocr. Rev. 31, 817–844. doi: 10.1210/er.2009-0030
Mandaliya, D. K., Patel, S., and Seshadri, S. (2021). The combinatorial effect of acetate and propionate on high-fat diet induced diabetic inflammation or metaflammation and t cell polarization. Inflammation 44, 68–79. doi: 10.1007/s10753-020-01309-7
Manichanh, C., Rigottier-Gois, L., Bonnaud, E., Gloux, K., Pelletier, E., Frangeul, L., et al. (2006). Reduced diversity of faecal microbiota in Crohn’s disease revealed by a metagenomic approach. Gut 55, 205–211. doi: 10.1136/gut.2005.073817
Martin, H. M., Campbell, B. J., Hart, C. A., Mpofu, C., Nayar, M., Singh, R., et al. (2004). Enhanced Escherichia coli adherence and invasion in Crohn’s disease and colon cancer. Gastroenterology 127, 80–93. doi: 10.1053/j.gastro.2004.03.054
Martín, R., Chain, F., Miquel, S., Lu, J., Gratadoux, J.-J., Sokol, H., et al. (2014). The commensal bacterium Faecalibacterium prausnitzii is protective in DNBS-induced chronic moderate and severe colitis models. Inflamm. Bowel Dis. 20, 417–430. doi: 10.1097/01.MIB.0000440815.76627.64
Martín, R., Miquel, S., Benevides, L., Bridonneau, C., Robert, V., Hudault, S., et al. (2017). Functional characterization of novel Faecalibacterium prausnitzii strains isolated from healthy volunteers: a step forward in the use of F. prausnitzii as a next-generation probiotic. Front. Microbiol. 8:1226. doi: 10.3389/fmicb.2017.01226
Maslowski, K. M., Vieira, A. T., Ng, A., Kranich, J., Sierro, F., Yu, D., et al. (2009). Regulation of inflammatory responses by gut microbiota and chemoattractant receptor GPR43. Nature 461, 1282–1286. doi: 10.1038/nature08530
Matsubara, V. H., Bandara, H. M. H. N., Ishikawa, K. H., Mayer, M. P. A., and Samaranayake, L. P. (2016). The role of probiotic bacteria in managing periodontal disease: a systematic review. Expert Rev. Anti-Infective Therapy 14, 643–655. doi: 10.1080/14787210.2016.1194198
Mazmanian, S. K., Liu, C. H., Tzianabos, A. O., and Kasper, D. L. (2005). An immunomodulatory molecule of symbiotic bacteria directs maturation of the host immune system. Cell 122, 107–118. doi: 10.1016/j.cell.2005.05.007
Mazmanian, S. K., Round, J. L., and Kasper, D. L. (2008). A microbial symbiosis factor prevents intestinal inflammatory disease. Nature 453, 620–625. doi: 10.1038/nature07008
Mennini, M., Reddel, S., del Chierico, F., Gardini, S., Quagliariello, A., Vernocchi, P., et al. (2021). Gut microbiota profile in children with IgE-Mediated cow’s milk allergy and cow’s milk sensitization and probiotic intestinal persistence evaluation. Int. J. Mol. Sci. 22:1649. doi: 10.3390/ijms22041649
Miao, E. A., Mao, D. P., Yudkovsky, N., Bonneau, R., Lorang, C. G., Warren, S. E., et al. (2010). Innate immune detection of the type III secretion apparatus through the NLRC4 inflammasome. Proc. Natl. Acad. Sci. U S A. 107, 3076–3080. doi: 10.1073/pnas.0913087107
Mills, R. H., Dulai, P. S., Vázquez-Baeza, Y., Sauceda, C., Daniel, N., Gerner, R. R., et al. (2022). Multi-omics analyses of the ulcerative colitis gut microbiome link Bacteroides vulgatus proteases with disease severity. Nat. Microbiol. 7, 262–276. doi: 10.1038/s41564-021-01050-3
Mirsepasi-Lauridsen, H. C., Vallance, B. A., Krogfelt, K. A., and Petersen, A. M. (2019). Escherichia coli pathobionts associated with inflammatory bowel disease. Clin. Microbiol. Rev. 32:e0060-18. doi: 10.1128/CMR.00060-18
Moayyedi, P., Surette, M. G., Kim, P. T., Libertucci, J., Wolfe, M., Onischi, C., et al. (2015). Fecal microbiota transplantation induces remission in patients with active ulcerative colitis in a randomized controlled trial. Gastroenterology 149, 102–109.e6. doi: 10.1053/j.gastro.2015.04.001.
Montanari, C., Parolisi, S., Borghi, E., Putignani, L., Bassanini, G., Zuvadelli, J., et al. (2021). Dysbiosis, host metabolism, and non-communicable diseases: trialogue in the inborn errors of metabolism. Front. Physiol. 12:716520. doi: 10.3389/fphys.2021.716520
Mukhopadhya, I., Thomson, J. M., Hansen, R., Berry, S. H., El-Omar, E. M., and Hold, G. L. (2011). Detection of Campylobacter concisus and other Campylobacter species in colonic biopsies from adults with ulcerative colitis. PLoS One 6:e21490. doi: 10.1371/journal.pone.0021490
Myers, B., Brownstone, N., Reddy, V., Chan, S., Thibodeaux, Q., Truong, A., et al. (2019). The gut microbiome in psoriasis and psoriatic arthritis. best practice & research. Clin. Rheumatol. 33:101494. doi: 10.1016/j.berh.2020.101494
Naito, M., Frirdich, E., Fields, J. A., Pryjma, M., Li, J., Cameron, A., et al. (2010). Effects of sequential Campylobacter jejuni 81-176 lipooligosaccharide core truncations on biofilm formation, stress survival, and pathogenesis. J. Bacteriol. 192, 2182–2192. doi: 10.1128/JB.01222-09
Nielsen, H. L., Dalager-Pedersen, M., and Nielsen, H. (2019). Risk of inflammatory bowel disease after Campylobacter jejuni and Campylobacter concisus infection: a population-based cohort study. Scand. J. Gastroenterol. 54, 265–272. doi: 10.1080/00365521.2019.1578406
Nikolaus, S., Schulte, B., Al-Massad, N., Thieme, F., Schulte, D. M., Bethge, J., et al. (2017). Increased tryptophan metabolism is associated with activity of inflammatory bowel diseases. Gastroenterology 153, 1504–1516.e2. doi: 10.1053/j.gastro.2017.08.028.
Nishida, A., Imaeda, H., Ohno, M., Inatomi, O., Bamba, S., Sugimoto, M., et al. (2017). Efficacy and safety of single fecal microbiota transplantation for Japanese patients with mild to moderately active ulcerative colitis. J. Gastroenterol. 52, 476–482. doi: 10.1007/s00535-016-1271-4
Nobili, V., Mosca, A., Alterio, T., Cardile, S., and Putignani, L. (2019). “Fighting fatty liver diseases with nutritional interventions, probiotics, symbiotics, and fecal microbiota transplantation (FMT) BT,” in Probiotics and Child Gastrointestinal Health: Advances in Microbiology, Infectious Diseases and Public Health, eds Guandalini F. Indrio (Berlin: Springer International Publishing), 85–100. doi: 10.1007/5584_2018_318
Nobili, V., Putignani, L., Mosca, A., del Chierico, F., Vernocchi, P., Alisi, A., et al. (2018). Bifidobacteria and lactobacilli in the gut microbiome of children with non-alcoholic fatty liver disease: which strains act as health players? Arch. Med. Sci. AMS 14, 81–87. doi: 10.5114/aoms.2016.62150
Nogal, A., Valdes, A. M., and Menni, C. (2021). The role of short-chain fatty acids in the interplay between gut microbiota and diet in cardio-metabolic health. Gut Microbes 13, 1–24. doi: 10.1080/19490976.2021.1897212
Norman, J. M., Handley, S. A., and Virgin, H. W. (2014). Kingdom-agnostic metagenomics and the importance of complete characterization of enteric microbial communities. Gastroenterology 146, 1459–1469. doi: 10.1053/j.gastro.2014.02.001
Ochoa-Repáraz, J., Mielcarz, D. W., Wang, Y., Begum-Haque, S., Dasgupta, S., Kasper, D. L., et al. (2010). A polysaccharide from the human commensal Bacteroides fragilis protects against CNS demyelinating disease. Mucosal Immunol. 3, 487–495. doi: 10.1038/mi.2010.29
O’Hara, A. M., O’Regan, P., Fanning, A., O’Mahony, C., Macsharry, J., Lyons, A., et al. (2006). Functional modulation of human intestinal epithelial cell responses by Bifidobacterium infantis and Lactobacillus salivarius. Immunology 118, 202–215. doi: 10.1111/j.1365-2567.2006.02358.x
Ohkusa, T., Yoshida, T., Sato, N., Watanabe, S., Tajiri, H., and Okayasu, I. (2009). Commensal bacteria can enter colonic epithelial cells and induce proinflammatory cytokine secretion: a possible pathogenic mechanism of ulcerative colitis. J. Med. Microbiol. 58(Pt 5), 535–545. doi: 10.1099/jmm.0.005801-0
Olejniczak-Staruch, I., Ciąyńska, M., Sobolewska-Sztychny, D., Narbutt, J., Skibińska, M., and Lesiak, A. (2021). Alterations of the skin and gut microbiome in psoriasis and psoriatic arthritis. Int. J. Mol. Sci. 22:3998. doi: 10.3390/ijms22083998
O’Mahony, C., Scully, P., O’Mahony, D., Murphy, S., O’Brien, F., Lyons, A., et al. (2008). Commensal-induced regulatory T cells mediate protection against pathogen-stimulated NF-kappaB activation. PLoS Pathogens 4:e1000112. doi: 10.1371/journal.ppat.1000112
Palmela, C., Chevarin, C., Xu, Z., Torres, J., Sevrin, G., Hirten, R., et al. (2018). Adherent-invasive Escherichia coli in inflammatory bowel disease. Gut 67, 574–587. doi: 10.1136/gutjnl-2017-314903
Pan, L.-L., Niu, W., Fang, X., Liang, W., Li, H., Chen, W., et al. (2019a). Clostridium butyricum strains suppress experimental acute pancreatitis by maintaining intestinal homeostasis. Mol. Nutrition Food Res. 63:e1801419. doi: 10.1002/mnfr.201801419
Pan, X., Fang, X., Wang, F., Li, H., Niu, W., Liang, W., et al. (2019b). Butyrate ameliorates caerulein-induced acute pancreatitis and associated intestinal injury by tissue-specific mechanisms. Br. J. Pharmacol. 176, 4446–4461. doi: 10.1111/bph.14806
Paramsothy, S., Kamm, M. A., Kaakoush, N. O., Walsh, A. J., van den Bogaerde, J., Samuel, D., et al. (2017). Multidonor intensive faecal microbiota transplantation for active ulcerative colitis: a randomised placebo-controlled trial. Lancet 389, 1218–1228. doi: 10.1016/S0140-6736(17)30182-4
Parkes, G. C., Rayment, N. B., Hudspith, B. N., Petrovska, L., Lomer, M. C., Brostoff, J., et al. (2012). Distinct microbial populations exist in the mucosa-associated microbiota of sub-groups of irritable bowel syndrome. Neurogastroenterol. Motility 24, 31–39. doi: 10.1111/j.1365-2982.2011.01803.x
Pascal, V., Pozuelo, M., Borruel, N., Casellas, F., Campos, D., Santiago, A., et al. (2017). A microbial signature for Crohn’s disease. Gut 66, 813–822. doi: 10.1136/gutjnl-2016-313235
Peirce, J. M., and Alviña, K. (2019). The role of inflammation and the gut microbiome in depression and anxiety. J. Neurosci. Res. 97, 1223–1241. doi: 10.1002/jnr.24476
Petra, A. I., Panagiotidou, S., Hatziagelaki, E., Stewart, J. M., Conti, P., and Theoharides, T. C. (2015). Gut-Microbiota-Brain axis and its effect on neuropsychiatric disorders with suspected immune dysregulation. Clin. Ther. 37, 984–995. doi: 10.1016/j.clinthera.2015.04.002
Pinto-Sanchez, M. I., Hall, G. B., Ghajar, K., Nardelli, A., Bolino, C., Lau, J. T., et al. (2017). Probiotic Bifidobacterium longum NCC3001 reduces depression scores and alters brain activity: a pilot study in patients with irritable bowel syndrome. Gastroenterology 153, 448–459.e8. doi: 10.1053/j.gastro.2017.05.003.
Png, C. W., Lindén, S. K., Gilshenan, K. S., Zoetendal, E. G., McSweeney, C. S., Sly, L. I., et al. (2010). Mucolytic bacteria with increased prevalence in IBD mucosa augment in vitro utilization of mucin by other bacteria. Am. J. Gastroenterol. 105, 2420–2428. doi: 10.1038/ajg.2010.281
Ponziani, F. R., Bhoori, S., Castelli, C., Putignani, L., Rivoltini, L., del Chierico, F., et al. (2019). Hepatocellular carcinoma is associated with gut microbiota profile and inflammation in nonalcoholic fatty liver disease. Hepatology 69, 107–120. doi: 10.1002/hep.30036
Ponziani, F. R., de Luca, A., Picca, A., Marzetti, E., Petito, V., del Chierico, F., et al. (2022). Gut dysbiosis and fecal calprotectin predict response to immune checkpoint inhibitors in patients with hepatocellular carcinoma. Hepatol. Commun. 6, 1492–1501. doi: 10.1002/hep4.1905
Putignani, L., del Chierico, F., Vernocchi, P., Cicala, M., Cucchiara, S., and Dallapiccola, B. (2016). Gut microbiota dysbiosis as risk and premorbid factors of IBD and IBS along the childhood-adulthood transition. Inflamm. Bowel Dis. 22, 487–504. doi: 10.1097/MIB.0000000000000602
Putignani, L., Gasbarrini, A., and Dallapiccola, B. (2019). Potential of multiomics technology in precision medicine. Curr. Opin. Gastroenterol. 35, 491–498. doi: 10.1097/MOG.0000000000000589
Putignani, L., Oliva, S., Isoldi, S., del Chierico, F., Carissimi, C., Laudadio, I., et al. (2021). Fecal and mucosal microbiota profiling in pediatric inflammatory bowel diseases. Eur. J. Gastroenterol. Hepatol. 33, 1376–1386. doi: 10.1097/MEG.0000000000002050
Qiu, J., Liu, R., Ma, Y., Li, Y., Chen, Z., He, H., et al. (2020). Lipopolysaccharide-Induced depression-like behaviors is ameliorated by sodium butyrate via inhibiting neuroinflammation and oxido-nitrosative stress. Pharmacology 105, 550–560. doi: 10.1159/000505132
Qiu, X., Zhang, M., Yang, X., Hong, N., and Yu, C. (2013). Faecalibacterium prausnitzii upregulates regulatory T cells and anti-inflammatory cytokines in treating TNBS-induced colitis. J. Crohn’s Colitis 7, e558–e568. doi: 10.1016/j.crohns.2013.04.002
Quagliariello, A., del Chierico, F., Reddel, S., Russo, A., Onetti Muda, A., D’Argenio, P., et al. (2020). Fecal microbiota transplant in two ulcerative colitis pediatric cases: gut microbiota and clinical course correlations. Microorganisms 8:1486. doi: 10.3390/microorganisms8101486
Quagliariello, A., del Chierico, F., Russo, A., Reddel, S., Conte, G., Lopetuso, L. R., et al. (2018). Gut microbiota profiling and gut-brain crosstalk in children affected by pediatric acute-onset neuropsychiatric syndrome and pediatric autoimmune neuropsychiatric disorders associated with streptococcal infections. Front. Microbiol. 9:675. doi: 10.3389/fmicb.2018.00675
Quan, Y., Song, K., Zhang, Y., Zhu, C., Shen, Z., Wu, S., et al. (2018). Roseburia intestinalis-derived flagellin is a negative regulator of intestinal inflammation. Biochem. Biophys. Res. Commun. 501, 791–799. doi: 10.1016/j.bbrc.2018.05.075
Ramakrishna, C., Kujawski, M., Chu, H., Li, L., Mazmanian, S. K., and Cantin, E. M. (2019). Bacteroides fragilis polysaccharide a induces IL-10 secreting B and T cells that prevent viral encephalitis. Nat. Commun. 10:2153. doi: 10.1038/s41467-019-09884-6
Ramos, A. P., Leonhard, S. E., Halstead, S. K., Cuba, M. A., Castañeda, C. C., Dioses, J. A., et al. (2021). Guillain-Barré syndrome outbreak in peru 2019 associated with Campylobacter jejuni Infection. Neurol. Neuroimmunol. Neuroinflamm. 8:e952. doi: 10.1212/NXI.0000000000000952
Redman, M. G., Ward, E. J., and Phillips, R. S. (2014). The efficacy and safety of probiotics in people with cancer: a systematic review. Ann. Oncol. 25, 1919–1929. doi: 10.1093/annonc/mdu106
Rhee, S. H., Im, E., Riegler, M., Kokkotou, E., O’brien, M., and Pothoulakis, C. (2005). Pathophysiological role of Toll-like receptor 5 engagement by bacterial flagellin in colonic inflammation. Proc. Natl. Acad. Sci. U S A. 102, 13610–13615. doi: 10.1073/pnas.0502174102
Ristori, M. V., Mortera, S. L., Marzano, V., Guerrera, S., Vernocchi, P., Ianiro, G., et al. (2020). Proteomics and metabolomics approaches towards a functional insight onto AUTISM spectrum disorders: phenotype stratification and biomarker discovery. Int. J. Mol. Sci. 21:6274. doi: 10.3390/ijms21176274
Ristori, M. V., Quagliariello, A., Reddel, S., Ianiro, G., Vicari, S., Gasbarrini, A., et al. (2019). Autism, gastrointestinal symptoms and modulation of gut microbiota by nutritional interventions. Nutrients 11:2812. doi: 10.3390/nu11112812
Romijn, A. R., Rucklidge, J. J., Kuijer, R. G., and Frampton, C. (2017). A double-blind, randomized, placebo-controlled trial of Lactobacillus helveticus and Bifidobacterium longum for the symptoms of depression. Aust. N. Z. J. Psychiatry 51, 810–821. doi: 10.1177/0004867416686694
Rossen, N. G., Fuentes, S., van der Spek, M. J., Tijssen, J. G., Hartman, J. H. A., Duflou, A., et al. (2015). Findings from a randomized controlled trial of fecal transplantation for patients with ulcerative colitis. Gastroenterology 149, 110-118.e4. doi: 10.1053/j.gastro.2015.03.045
Rossi, O., Khan, M. T., Schwarzer, M., Hudcovic, T., Srutkova, D., Duncan, S. H., et al. (2015). Faecalibacterium prausnitzii strain HTF-F and its extracellular polymeric matrix attenuate clinical parameters in DSS-Induced colitis. PLoS One 10:e0123013. doi: 10.1371/journal.pone.0123013
Rothhammer, V., Mascanfroni, I. D., Bunse, L., Takenaka, M. C., Kenison, J. E., Mayo, L., et al. (2016). Type I interferons and microbial metabolites of tryptophan modulate astrocyte activity and central nervous system inflammation via the aryl hydrocarbon receptor. Nat. Med. 22, 586–597. doi: 10.1038/nm.4106
Round, J. L., and Mazmanian, S. K. (2010). Inducible Foxp3+ regulatory T-cell development by a commensal bacterium of the intestinal microbiota. Proc. Natl. Acad. Sci. U S A. 107, 12204–12209. doi: 10.1073/pnas.0909122107
Rutsch, A., Kantsjö, J. B., and Ronchi, F. (2020). The gut-brain axis: how microbiota and host inflammasome influence brain physiology and pathology. Front. Immunol. 11:604179. doi: 10.3389/fimmu.2020.604179
Saez-Lara, M. J., Gomez-Llorente, C., Plaza-Diaz, J., and Gil, A. (2015). The role of probiotic lactic acid bacteria and bifidobacteria in the prevention and treatment of inflammatory bowel disease and other related diseases: a systematic review of randomized human clinical trials. BioMed. Res. Int. 2015:505878. doi: 10.1155/2015/505878
Sáez-Lara, M. J., Robles-Sanchez, C., Ruiz-Ojeda, F. J., Plaza-Diaz, J., and Gil, A. (2016). Effects of probiotics and synbiotics on obesity, insulin resistance syndrome, type 2 diabetes and non-alcoholic fatty liver disease: a review of human clinical trials. Int. J. Mol. Sci. 17:928. doi: 10.3390/ijms17060928
Sanna, S., van Zuydam, N. R., Mahajan, A., Kurilshikov, A., Vich Vila, A., Võsa, U., et al. (2019). Causal relationships among the gut microbiome, short-chain fatty acids and metabolic diseases. Nat. Genet. 51, 600–605. doi: 10.1038/s41588-019-0350-x
Saus, E., Iraola-Guzmán, S., Willis, J. R., Brunet-Vega, A., and Gabaldón, T. (2019). Microbiome and colorectal cancer: roles in carcinogenesis and clinical potential. Mol. Aspects Med. 69, 93–106. doi: 10.1016/j.mam.2019.05.001
Scher, J. U., Littman, D. R., and Abramson, S. B. (2016). Microbiome in inflammatory arthritis and human rheumatic diseases. Arthritis Rheumatol. 68, 35–45. doi: 10.1002/art.39259
Schiavi, E., Gleinser, M., Molloy, E., Groeger, D., Frei, R., Ferstl, R., et al. (2016). The surface-associated exopolysaccharide of Bifidobacterium longum 35624 plays an essential role in dampening host proinflammatory responses and repressing local TH17 responses. Appl. Environ. Microbiol. 82, 7185–7196. doi: 10.1128/AEM.02238-16
Schiavi, E., Plattner, S., Rodriguez-Perez, N., Barcik, W., Frei, R., Ferstl, R., et al. (2018). Exopolysaccharide from Bifidobacterium longum subsp. longum 35624TM modulates murine allergic airway responses. Beneficial Microbes 9, 761–773. doi: 10.3920/BM2017.0180
Schippa, S., Iebba, V., Totino, V., Santangelo, F., Lepanto, M., Alessandri, C., et al. (2012). A potential role of Escherichia coli pathobionts in the pathogenesis of pediatric inflammatory bowel disease. Can. J. Microbiol. 58, 426–432. doi: 10.1139/w2012-007
Schneider, K. M., Mohs, A., Gui, W., Galvez, E. J. C., Candels, L. S., Hoenicke, L., et al. (2022). Imbalanced gut microbiota fuels hepatocellular carcinoma development by shaping the hepatic inflammatory microenvironment. Nat. Commun. 13:3964. doi: 10.1038/s41467-022-31312-5
Scully, P., Macsharry, J., O’Mahony, D., Lyons, A., O’Brien, F., Murphy, S., et al. (2013). Bifidobacterium infantis suppression of Peyer’s patch MIP-1α and MIP-1β secretion during Salmonella infection correlates with increased local CD4+CD25+ T cell numbers. Cell Immunol. 281, 134–140. doi: 10.1016/j.cellimm.2013.03.008
Sears, C. L., Islam, S., Saha, A., Arjumand, M., Alam, N. H., Faruque, A. S. G., et al. (2008). Association of enterotoxigenic Bacteroides fragilis infection with inflammatory diarrhea. Clin. Infect. Dis. 47, 797–803. doi: 10.1086/591130
Shaler, C. R., Elhenawy, W., and Coombes, B. K. (2019). The unique lifestyle of crohn’s disease-associated adherent-invasive Escherichia coli. J. Mol. Biol. 431, 2970–2981. doi: 10.1016/j.jmb.2019.04.023
Shen, Y., Giardino Torchia, M. L., Lawson, G. W., Karp, C. L., Ashwell, J. D., and Mazmanian, S. K. (2012). Outer membrane vesicles of a human commensal mediate immune regulation and disease protection. Cell Host Microbe 12, 509–520. doi: 10.1016/j.chom.2012.08.004
Shiba, T., Aiba, Y., Ishikawa, H., Ushiyama, A., Takagi, A., Mine, T., et al. (2003). The suppressive effect of bifidobacteria on Bacteroides vulgatus, a putative pathogenic microbe in inflammatory bowel disease. Microbiol. Immunol. 47, 371–378. doi: 10.1111/j.1348-0421.2003.tb03368.x
Shirvani-Rad, S., Tabatabaei-Malazy, O., Mohseni, S., Hasani-Ranjbar, S., Soroush, A.-R., Hoseini-Tavassol, Z., et al. (2021). Probiotics as a complementary therapy for management of obesity: a systematic review. Evidence-Based Complementary Alternative Med. ECAM 2021:6688450. doi: 10.1155/2021/6688450
Shu, S.-A., Yuen, A. W. T., Woo, E., Chu, K.-H., Kwan, H.-S., Yang, G.-X., et al. (2019). Microbiota and food allergy. Clin. Rev. Allergy Immunol. 57, 83–97. doi: 10.1007/s12016-018-8723-y
Sibartie, S., O’Hara, A. M., Ryan, J., Fanning, A., O’Mahony, J., O’Neill, S., et al. (2009). Modulation of pathogen-induced CCL20 secretion from HT-29 human intestinal epithelial cells by commensal bacteria. BMC Immunol. 10:54. doi: 10.1186/1471-2172-10-54
Sofi, M. H., Johnson, B. M., Gudi, R. R., Jolly, A., Gaudreau, M.-C., and Vasu, C. (2019). Polysaccharide A-Dependent opposing effects of mucosal and systemic exposures to human gut commensal Bacteroides fragilis in Type 1 diabetes. Diabetes Metab. Res. Rev. 68, 1975–1989. doi: 10.2337/db19-0211
Sokol, H., Landman, C., Seksik, P., Berard, L., Montil, M., Nion-Larmurier, I., et al. (2020). Fecal microbiota transplantation to maintain remission in Crohn’s disease: a pilot randomized controlled study. Microbiome 8:12. doi: 10.1186/s40168-020-0792-5
Sokol, H., Pigneur, B., Watterlot, L., Lakhdari, O., Bermúdez-Humarán, L. G., Gratadoux, J.-J., et al. (2008). Faecalibacterium prausnitzii is an anti-inflammatory commensal bacterium identified by gut microbiota analysis of Crohn disease patients. Proc. Natl. Acad. Sci. U S A. 105, 16731–16736. doi: 10.1073/pnas.0804812105
Sokol, H., Seksik, P., Furet, J. P., Firmesse, O., Nion-Larmurier, I., Beaugerie, L., et al. (2009). Low counts of Faecalibacterium prausnitzii in colitis microbiota. Inflamm. Bowel Dis. 15, 1183–1189. doi: 10.1002/ibd.20903
Steck, N., Hoffmann, M., Sava, I. G., Kim, S. C., Hahne, H., Tonkonogy, S. L., et al. (2011). Enterococcus faecalis metalloprotease compromises epithelial barrier and contributes to intestinal inflammation. Gastroenterology 141, 959–971. doi: 10.1053/j.gastro.2011.05.035
Steinhart, A. H., Hiruki, T., Brzezinski, A., and Baker, J. P. (1996). Treatment of left-sided ulcerative colitis with butyrate enemas: a controlled trial. Alimentary Pharmacol. Therapeut. 10, 729–736. doi: 10.1046/j.1365-2036.1996.d01-509.x
Stoeva, M. K., Garcia-So, J., Justice, N., Myers, J., Tyagi, S., Nemchek, M., et al. (2021). Butyrate-producing human gut symbiont. Clostridium butyricum, and its role in health and disease. Gut Microbes 13, 1–28. doi: 10.1080/19490976.2021.1907272
Strati, F., Cavalieri, D., Albanese, D., de Felice, C., Donati, C., Hayek, J., et al. (2017). New evidences on the altered gut microbiota in autism spectrum disorders. Microbiome 5:24. doi: 10.1186/s40168-017-0242-1
Sun, X., Threadgill, D., and Jobin, C. (2012). Campylobacter jejuni induces colitis through activation of mammalian target of rapamycin signaling. Gastroenterology 142, 86–95.e5. doi: 10.1053/j.gastro.2011.09.042.
Sun, Y.-Y., Li, M., Li, Y.-Y., Li, L.-X., Zhai, W.-Z., Wang, P., et al. (2018). The effect of Clostridium butyricum on symptoms and fecal microbiota in diarrhea-dominant irritable bowel syndrome: a randomized, double-blind, placebo-controlled trial. Sci. Rep. 8:2964. doi: 10.1038/s41598-018-21241-z
Underwood, A. P., Kaakoush, N. O., Sodhi, N., Merif, J., Seah Lee, W., Riordan, S. M., et al. (2016). Campylobacter concisus pathotypes are present at significant levels in patients with gastroenteritis. J. Med. Microbiol. 65, 219–226. doi: 10.1099/jmm.0.000216
van Spreeuwel, J. P., Duursma, G. C., Meijer, C. J., Bax, R., Rosekrans, P. C., and Lindeman, J. (1985). Campylobacter colitis: histological immunohistochemical and ultrastructural findings. Gut 26, 945–951. doi: 10.1136/gut.26.9.945
Vernocchi, P., del Chierico, F., Fiocchi, A. G., el Hachem, M., Dallapiccola, B., Rossi, P., et al. (2015). Understanding probiotics’ role in allergic children: the clue of gut microbiota profiling. Curr. Opin. Allergy Clin. Immunol. 15, 495–503. doi: 10.1097/ACI.0000000000000203
Vernocchi, P., del Chierico, F., Quagliariello, A., Ercolini, D., Lucidi, V., and Putignani, L. (2017). A metagenomic and in silico functional prediction of gut microbiota profiles may concur in discovering new cystic fibrosis patient-targeted probiotics. Nutrients 9:1342. doi: 10.3390/nu9121342
Vernocchi, P., del Chierico, F., Russo, A., Majo, F., Rossitto, M., Valerio, M., et al. (2018). Gut microbiota signatures in cystic fibrosis: loss of host CFTR function drives the microbiota enterophenotype. PLoS One 13:e0208171. doi: 10.1371/journal.pone.0208171
Vernocchi, P., Gili, T., Conte, F., del Chierico, F., Conta, G., Miccheli, A., et al. (2020). Network analysis of gut microbiome and metabolome to discover microbiota-linked biomarkers in patients affected by non-small cell lung cancer. Int. J. Mol. Sci. 21:8730. doi: 10.3390/ijms21228730
Virtue, A. T., McCright, S. J., Wright, J. M., Jimenez, M. T., Mowel, W. K., Kotzin, J. J., et al. (2019). The gut microbiota regulates white adipose tissue inflammation and obesity via a family of microRNAs. Sci. Translational Med. 11:eaav1892. doi: 10.1126/scitranslmed.aav1892
Vlachou, E., Ntikoudi, A., Govina, O., Lavdaniti, M., Kotsalas, N., Tsartsalis, A., et al. (2020). Effects of probiotics on diabetic nephropathy: a systematic review. Curr. Clin. Pharmacol. 15, 234–242. doi: 10.2174/1574884715666200303112753
Vogt, N. M., Kerby, R. L., Dill-McFarland, K. A., Harding, S. J., Merluzzi, A. P., Johnson, S. C., et al. (2017). Gut microbiome alterations in Alzheimer’s disease. Sci. Rep. 7:13537. doi: 10.1038/s41598-017-13601-y
Vujkovic-Cvijin, I., Sklar, J., Jiang, L., Natarajan, L., Knight, R., and Belkaid, Y. (2020). Host variables confound gut microbiota studies of human disease. Nature 587, 448–454. doi: 10.1038/s41586-020-2881-9
Walczuk, U., Sobieszczańska, B., Turniak, M., Rzeszutko, M., Duda-Madej, A., and Iwańczak, B. (2019). The prevalence of mucosa-associated diffusely adherent Escherichia coli in children with inflammatory bowel disease. Adv. Clin. Exp. Med. 28, 899–905. doi: 10.17219/acem/94149
Wallimann, A., Hildebrand, M., Groeger, D., Stanic, B., Akdis, C. A., Zeiter, S., et al. (2021). An exopolysaccharide produced by Bifidobacterium longum 35624§inhibits osteoclast formation via a TLR2-Dependent mechanism. Calcif. Tissue Int. 108, 654–666. doi: 10.1007/s00223-020-00790-4
Wang, H., Gou, W., Su, C., Du, W., Zhang, J., Miao, Z., et al. (2022). Association of gut microbiota with glycaemic traits and incident type 2 diabetes, and modulation by habitual diet: a population-based longitudinal cohort study in Chinese adults. Diabetologia 65, 1145–1156. doi: 10.1007/s00125-022-05687-5
Wang, X., Ye, C., Xun, T., Mo, L., Tong, Y., Ni, W., et al. (2021). Bacteroides fragilis polysaccharide a ameliorates abnormal voriconazole metabolism accompanied with the inhibition of TLR4/NF-κB pathway. Front. Pharmacol. 12:663325. doi: 10.3389/fphar.2021.663325
Warren, R. L., Freeman, D. J., Pleasance, S., Watson, P., Moore, R. A., Cochrane, K., et al. (2013). Co-occurrence of anaerobic bacteria in colorectal carcinomas. Microbiome 1:16. doi: 10.1186/2049-2618-1-16
Wu, N., Yang, X., Zhang, R., Li, J., Xiao, X., Hu, Y., et al. (2013). Dysbiosis signature of fecal microbiota in colorectal cancer patients. Microb. Ecol. 66, 462–470. doi: 10.1007/s00248-013-0245-9
Wu, S., Rhee, K.-J., Albesiano, E., Rabizadeh, S., Wu, X., Yen, H.-R., et al. (2009). A human colonic commensal promotes colon tumorigenesis via activation of T helper type 17 T cell responses. Nat. Med. 15, 1016–1022. doi: 10.1038/nm.2015
Wu, X., Pan, S., Luo, W., Shen, Z., Meng, X., Xiao, M., et al. (2020). Roseburia intestinalis-derived flagellin ameliorates colitis by targeting miR-223-3p-mediated activation of NLRP3 inflammasome and pyroptosis. Mol. Med. Rep. 22, 2695–2704. doi: 10.3892/mmr.2020.11351
Xiao, J., Katsumata, N., Bernier, F., Ohno, K., Yamauchi, Y., Odamaki, T., et al. (2020). Probiotic Bifidobacterium breve in improving cognitive functions of older adults with suspected mild cognitive impairment: a randomized, double-blind, placebo-controlled trial. J. Alzheimer’s Dis. JAD 77, 139–147. doi: 10.3233/JAD-200488
Xie, L., Saimaier, K., Wang, C., Yang, J., Han, M., Lv, J., et al. (2021). Methyl acetate arrests Th1 in peripheral immune system and alleviates CNS inflammation in EAE. Int. Immunopharmacol. 101(Pt B):108291. doi: 10.1016/j.intimp.2021.108291
Xu, H., Ma, C., Zhao, F., Chen, P., Liu, Y., Sun, Z., et al. (2021). Adjunctive treatment with probiotics partially alleviates symptoms and reduces inflammation in patients with irritable bowel syndrome. Eur. J. Nutr. 60, 2553–2565. doi: 10.1007/s00394-020-02437-4
Yamashiro, K., Tanaka, R., Urabe, T., Ueno, Y., Yamashiro, Y., Nomoto, K., et al. (2017). Gut dysbiosis is associated with metabolism and systemic inflammation in patients with ischemic stroke. PLoS One 12:e0171521. doi: 10.1371/journal.pone.0171521
Yang, D., Meng, X.-Y., Wang, Y., Zhang, J., Zhao, Y., Zheng, Z.-C., et al. (2022). Effects of probiotics on gastric cancer-related inflammation: a systematic review and meta-analysis. J. Food Biochem. 46:e14034. doi: 10.1111/jfbc.14034
Yanine, N., Araya, I., Brignardello-Petersen, R., Carrasco-Labra, A., González, A., Preciado, A., et al. (2013). Effects of probiotics in periodontal diseases: a systematic review. Clin. Oral Investig. 17, 1627–1634. doi: 10.1007/s00784-013-0990-7
Yap, C. X., Henders, A. K., Alvares, G. A., Wood, D. L. A., Krause, L., Tyson, G. W., et al. (2021). Autism-related dietary preferences mediate autism-gut microbiome associations. Cell 184, 5916–5931.e17. doi: 10.1016/j.cell.2021.10.015
Yde Aagaard, M. E., Frahm Kirk, K., Linde Nielsen, H., Harder Tarpgaard, I., Bach Hansen, J., and Nielsen, H. (2020). Campylobacter concisus is prevalent in the gastrointestinal tract of patients with microscopic colitis. Scand. J. Gastroenterol. 55, 924–930. doi: 10.1080/00365521.2020.1792976
Yu, E. W., Gao, L., Stastka, P., Cheney, M. C., Mahabamunuge, J., Torres Soto, M., et al. (2020). Fecal microbiota transplantation for the improvement of metabolism in obesity: the FMT-TRIM double-blind placebo-controlled pilot trial. PLoS Med. 17:e1003051. doi: 10.1371/journal.pmed.1003051
Yuan, F., Ni, H., Asche, C. V., Kim, M., Walayat, S., and Ren, J. (2017). Efficacy of Bifidobacterium infantis 35624 in patients with irritable bowel syndrome: a meta-analysis. Curr. Med. Res. Opin. 33, 1191–1197. doi: 10.1080/03007995.2017.1292230
Zackular, J. P., Baxter, N. T., Iverson, K. D., Sadler, W. D., Petrosino, J. F., Chen, G. Y., et al. (2013). The gut microbiome modulates colon tumorigenesis. mBio 4:e00692-13. doi: 10.1128/mBio.00692-13
Zaharuddin, L., Mokhtar, N. M., Muhammad Nawawi, K. N., and Raja Ali, R. A. (2019). A randomized double-blind placebo-controlled trial of probiotics in post-surgical colorectal cancer. BMC Gastroenterol. 19:131. doi: 10.1186/s12876-019-1047-4
Zaiss, M. M., Joyce, Wu, H.-J., Mauro, D., Schett, G., and Ciccia, F. (2021). The gut-joint axis in rheumatoid arthritis. Nat. Rev. Rheumatol. 17, 224–237. doi: 10.1038/s41584-021-00585-3
Zelante, T., Iannitti, R. G., Cunha, C., de Luca, A., Giovannini, G., Pieraccini, G., et al. (2013). Tryptophan catabolites from microbiota engage aryl hydrocarbon receptor and balance mucosal reactivity via interleukin-22. Immunity 39, 372–385. doi: 10.1016/j.immuni.2013.08.003
Zhang, C., Yin, A., Li, H., Wang, R., Wu, G., Shen, J., et al. (2015). Dietary modulation of gut microbiota contributes to alleviation of both genetic and simple obesity in children. EBioMedicine 2, 968–984. doi: 10.1016/j.ebiom.2015.07.007
Zhang, L., Man, S. M., Day, A. S., Leach, S. T., Lemberg, D. A., Dutt, S., et al. (2009). Detection and isolation of Campylobacter species other than C. jejuni from children with Crohn’s disease. J. Clin. Microbiol. 47, 453–455. doi: 10.1128/JCM.01949-08
Zhao, Q., Yang, W.-R., Wang, X.-H., Li, G.-Q., Xu, L.-Q., Cui, X., et al. (2019). Clostridium butyricum alleviates intestinal low-grade inflammation in TNBS-induced irritable bowel syndrome in mice by regulating functional status of lamina propria dendritic cells. World J. Gastroenterol. 25, 5469–5482. doi: 10.3748/wjg.v25.i36.5469
Zheng, H. J., Guo, J., Wang, Q., Wang, L., Wang, Y., Zhang, F., et al. (2021). Probiotics, prebiotics, and synbiotics for the improvement of metabolic profiles in patients with chronic kidney disease: a systematic review and meta-analysis of randomized controlled trials. Crit. Rev. Food Sci. Nutr. 61, 577–598. doi: 10.1080/10408398.2020.1740645
Keywords: inflammation, gut microbiota, fecal microbiota transplant, therapy, probiotics, biological mechanisms, inflammatory diseases
Citation: Piazzesi A and Putignani L (2022) Extremely small and incredibly close: Gut microbes as modulators of inflammation and targets for therapeutic intervention. Front. Microbiol. 13:958346. doi: 10.3389/fmicb.2022.958346
Received: 31 May 2022; Accepted: 25 July 2022;
Published: 22 August 2022.
Edited by:
Deborah Traversi, University of Turin, ItalyReviewed by:
Silvia Turroni, University of Bologna, ItalyFrancesca Romana Ponziani, Internal Medicine, Gastroenterology, Hepatology Fondazione Policlinico Universitario Agostino Gemelli IRCCS, Italy
Copyright © 2022 Piazzesi and Putignani. This is an open-access article distributed under the terms of the Creative Commons Attribution License (CC BY). The use, distribution or reproduction in other forums is permitted, provided the original author(s) and the copyright owner(s) are credited and that the original publication in this journal is cited, in accordance with accepted academic practice. No use, distribution or reproduction is permitted which does not comply with these terms.
*Correspondence: Lorenza Putignani, bG9yZW56YS5wdXRpZ25hbmlAb3BiZy5uZXQ=