- 1State Key Laboratory of Oral Diseases, National Clinical Research Center for Oral Diseases, West China Hospital of Stomatology, Sichuan University, Chengdu, China
- 2The School and Hospital of Stomatology, Tianjin Medical University, Tianjin, China
Cyclic di-adenosine monophosphate (c-di-AMP) is a second messenger which is widely used in signal transduction in bacteria and archaea. c-di-AMP plays an important role in the regulation of bacterial physiological activities, such as the cell cycle, cell wall stability, environmental stress response, and biofilm formation. Moreover, c-di-AMP produced by pathogens can be recognized by host cells for the activation of innate immune responses. It can induce type I interferon (IFN) response in a stimulator of interferon genes (STING)-dependent manner, activate the nuclear factor kappa B (NF-κB) pathway, inflammasome, and host autophagy, and promote the production and secretion of cytokines. In addition, c-di-AMP is capable of triggering a host mucosal immune response as a mucosal adjuvant. Therefore, c-di-AMP is now considered to be a new pathogen-associated molecular pattern in host immunity and has become a promising target in bacterial/viral vaccine and drug research. In this review, we discussed the crosstalk between bacteria and host immunity mediated by c-di-AMP and addressed the role of c-di-AMP as a mucosal adjuvant in boosting evoked immune responses of subunit vaccines. The potential application of c-di-AMP in immunomodulation and immunotherapy was also discussed in this review.
Introduction
Cyclic di-adenosine monophosphate (c-di-AMP) is a newly discovered signaling molecule after cyclic adenosine monophosphate (cAMP), guanosine tetraphosphate (ppGpp), and cyclic di-guanosine monophosphate (c-di-GMP). c-di-AMP was first identified in 2008 during the crystallization of a Bacillus subtilis (B. subtilis) DNA integrity scanning (DisA) protein (Witte et al., 2008). The DisA_N domain was characterized to have diadenylate cyclase (DAC) activity and could synthesize c-di-AMP using adenosine triphosphate (ATP) and adenosine diphosphate (ADP) as substrates. There are many other unknown functional domains around the DAC that presumably control the signal inputs and outputs of DisA (Witte et al., 2008). The DAC domain is widely distributed in Gram-positive and Gram-negative bacteria and archaea but not in eukaryotes. The Pfam protein database1 shows that there are 5407 proteins from 3991 species that contain the DAC domain, with a consensus sequence of 120 amino acids (Mistry et al., 2021). These species include not only most bacteria (not identified in the alpha, beta, and gamma proteobacteria) but also Euryarchaeota. This suggests an ancient origin of c-di-AMP, with critical roles in bacterial physiology that cannot be ignored.
Bacteria that lack the DAC domain, such as Escherichia coli (E. coli), cannot synthesize c-di-AMP. Whereas B. subtilis has three DAC proteins, of which, DisA is regulated by the salt pressure response sigma factor SigM, and synthesizes 50% of c-di-AMP in early sporulation (Cao et al., 2002; Jervis et al., 2007). Since the regulatory networks of the three proteins in B. subtilis are completely different, they may play different roles under different environmental stimuli (Mehne et al., 2013). DAC activity in an alkaline pH is higher than that in an acidic environment and depends on divalent metal ions (e.g., Mg2+, Mn2+, and Co2+) (Bai et al., 2014). Proteins containing the DHH/DHHA1 domain, such as YybT (c-di-AMP phosphodiesterase) in B. subtilis, CnpB (cyclic nucleotide phosphodiesterase) in Mycobacterium tuberculosis, and Pde (phosphodiesterase) in Streptococcus pneumoniae, have phosphatase or phosphodiesterase activities, that can degrade c-di-AMP into pApA or AMP (Rao et al., 2010; Bai et al., 2014; Yang et al., 2014). The level of c-di-AMP increases considerably in the knockout strain for the gene encoding hydrolyase (Corrigan et al., 2011; Gall et al., 2022).
Cyclic di-adenosine monophosphate plays an important role in the regulation of bacterial physiological activities, such as the cell cycle, cell wall stability, environmental stress response, and biofilm formation. Moreover, c-di-AMP can be recognized by host cells for the activation of innate immune responses. In addition, c-di-AMP is capable of triggering a host mucosal immune response as a mucosal adjuvant. Therefore, c-di-AMP, a new pathogen-associated molecular pattern in host immunity, has become a promising target in vaccine and drug research.
Cyclic di-adenosine monophosphate regulates physiological functions of bacteria
Cyclic di-adenosine monophosphate is involved in many essential cellular processes of bacteria, as shown in Figure 1 and Table 1. DisA scans the chromosome and pauses at sites of DNA lesions. Intracellular levels of c-di-AMP rise markedly at the onset of sporulation in a DisA-dependent manner. However, exposing sporulating cells to DNA-damaging agents can cause a decrease in the level of c-di-AMP, leading to a delay in sporulation, which can be controlled by the external supplementation of c-di-AMP. These results suggest that c-di-AMP acts as a critical secondary messenger in DNA integrity during sporulation (Boye, 2006). Both low and high levels of accumulation of c-di-AMP result in cell growth inhibition, suggesting a sensitive relationship between c-di-AMP homeostasis and cell growth (Mehne et al., 2013). Several studies revealed an essential role for c-di-AMP in peptidoglycan homeostasis and cell envelope stress response (Corrigan et al., 2011; Luo and Helmann, 2012; Wang et al., 2017). c-di-AMP fluctuates dynamically in coordination with Mg2+ and K+ levels, facilitating bacterial adaptation to osmotic stress (Zarrella et al., 2018; Quintana et al., 2019; Cereija et al., 2021; Wendel et al., 2022). Moreover, c-di-AMP could modulate bacterial acid stress, biofilm formation, and metabolism (Bowman et al., 2016; Townsley et al., 2018; Krüger et al., 2022).
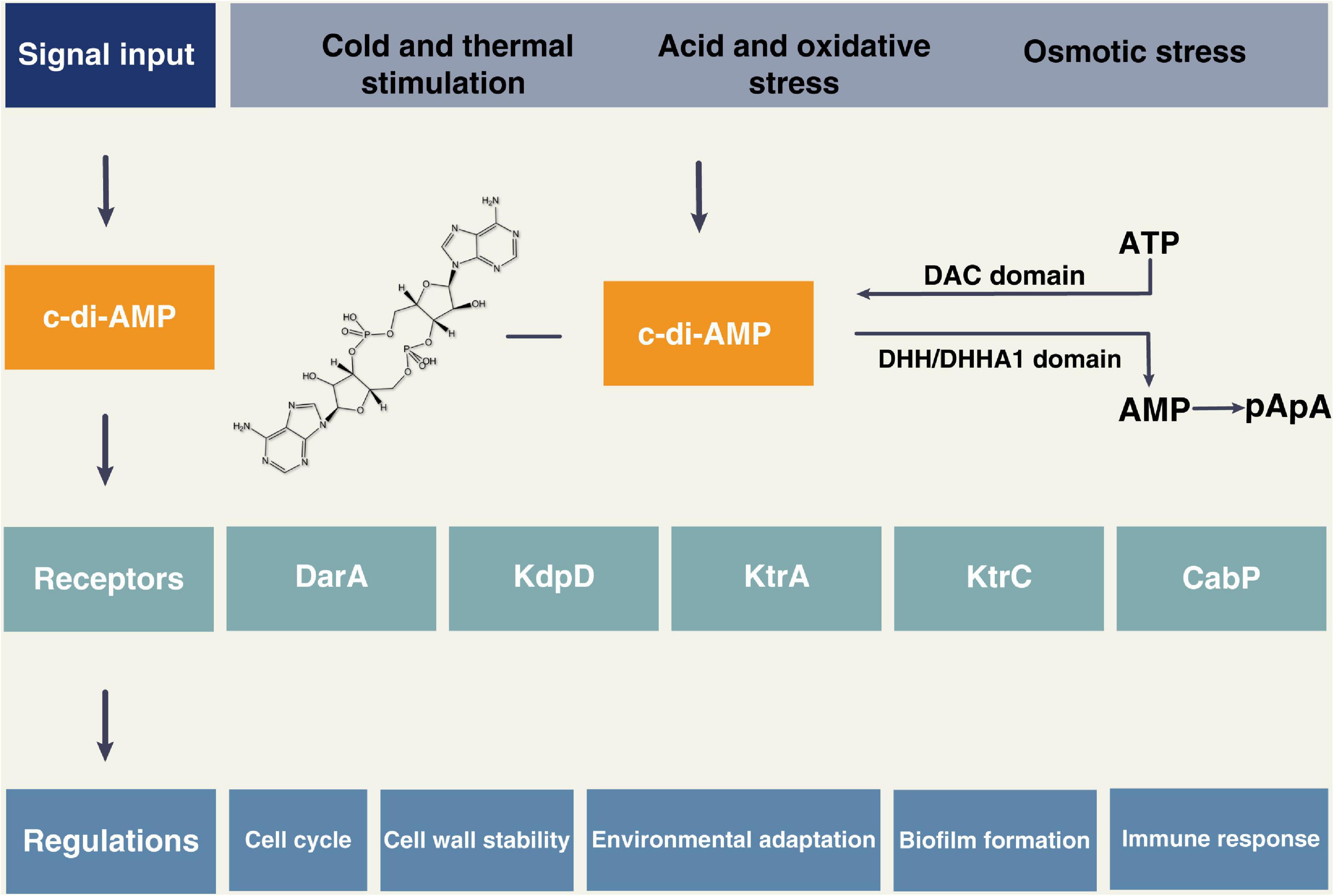
Figure 1. The cyclic di-adenosine monophosphate (c-di-AMP) signal system. c-di-AMP can be synthesized from ATP via proteins containing the DAC domain, and proteins containing DHH/DHHA1 domain can degrade c-di-AMP into AMP or pApA. The external stimuli can induce the production of c-di-AMP, which then binds to the receptors such as DarA, KdpD, KtrA, KtrC, and CabP. The c-di-AMP signaling pathway is involved in many bacterial physiological activities, such as the cell cycle, cell wall stability, environmental stress response, biofilm formation, and host immune response.
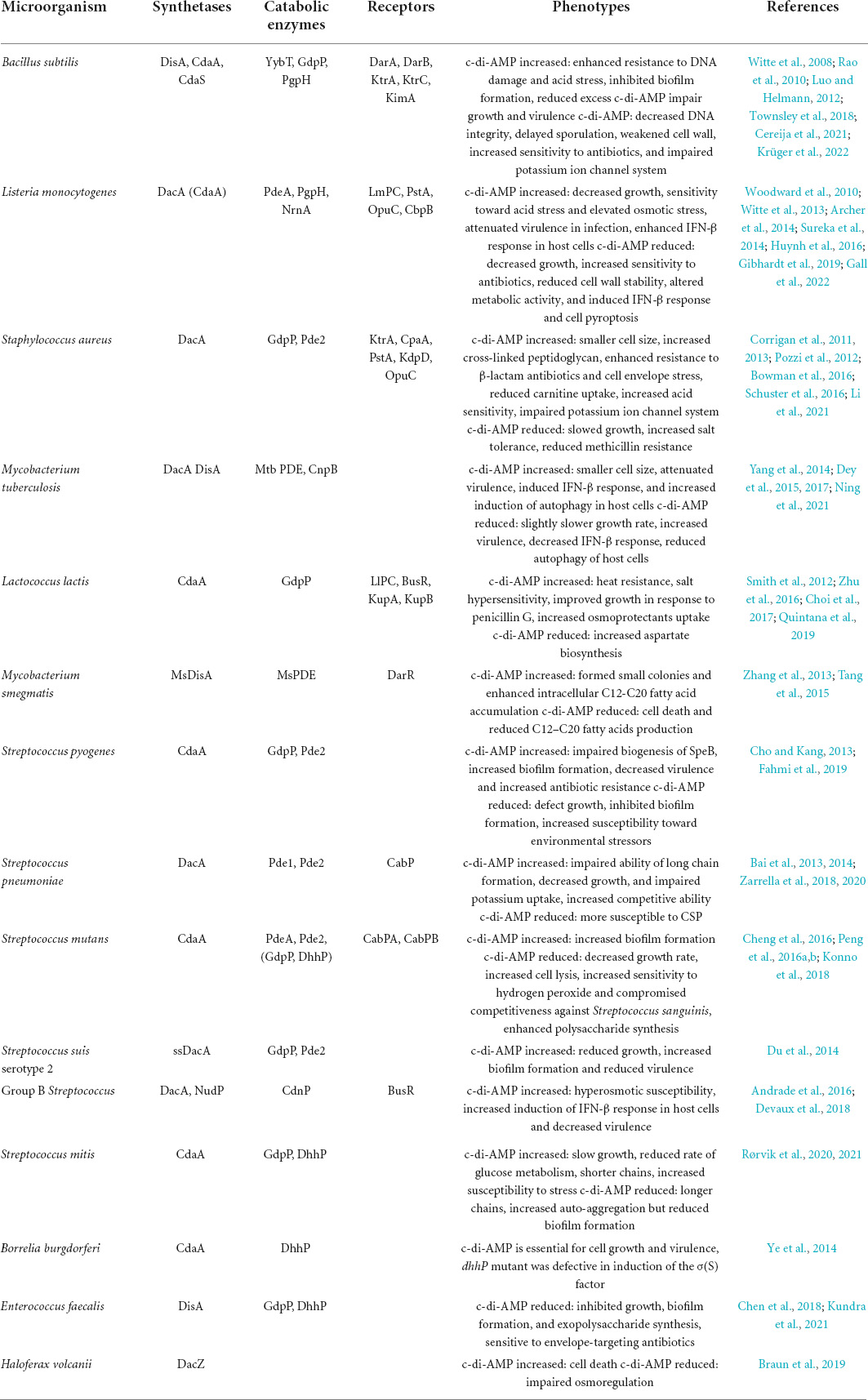
Table 1. Summary of cyclic di-adenosine monophosphate (c-di-AMP) synthetases, catabolic enzymes, and related physiological functions in bacteria and archaea.
Cheng et al. (2016) identified and characterized a DAC, CdaA (cyclic di-AMP synthase) in Streptococcus mutans (S. mutans), which is the primary etiological factor of human dental caries. Deletion of cdaA in S. mutans UA159 led to a decreased growth rate and increased cell lysis. The S. mutans cdaA mutant exhibited increased sensitivity to oxidative stress and compromised competitiveness against Streptococcus sanguinis. The global transcriptional data showed that several genomic islands, such as TnSmu1 (a conjugative transposon), TnSmu2 (a large transposon-like region), CRISPR1-Cas (clustered regularly interspaced short palindromic repeats/CRISPR-associated genes), and CRISPR2-Cas formed enriched interaction clusters in a cdaA mutant. Almost all of the upregulated genes formed a highly linked network, which indicated that a decrease in the c-di-AMP concentration in the mutant cells induced the expression of a set of genes with physiological functions (Cheng et al., 2016). Furthermore, c-di-AMP could regulate the expression of glucosyltransferases (gtfs) by interacting with CabPA (a c-di-AMP binding protein)/VicR (the response regulator in VicRK two-component signal transduction system) complex, modulating exopolysaccharide synthesis and biofilm formation of S. mutans (Peng et al., 2016a,b; Konno et al., 2018). All these studies indicate that c-di-AMP plays an important role in the regulation of bacterial physiological activities.
Cyclic di-adenosine monophosphate modulates host innate immune response
Microbial challenges to the host trigger an array of defense processes through the activation of innate and adaptive immunity. Innate immunity is the first important line of defense against microbial invasion, especially for intracellular bacterial infection, and makes a critical contribution to many inflammatory conditions. The initiation of an inflammatory response relies on the recognition of a diverse range of pathogen-associated molecular patterns (PAMPs) and damage-associated molecular patterns (DAMPs) through pattern recognition receptors (PRRs) (Kumar et al., 2013). Different classes of PRRs include the Toll-like receptors (TLRs), C-type lectins, nucleotide-binding oligomerization domain-like receptors (NLRs), DNA sensors including those absent in melanoma 2 (AIM2), and RNA-sensing retinoic acid-inducible gene I-like helicases (Schroder and Tschopp, 2010). PRRs are not only expressed on the cell membrane but are also widely distributed in the endosomal membrane, lysosomal membrane, and cytoplasm. PRRs are found on both immune and non-immune cells and respond to PAMPs and DAMPs by initiating downstream signaling cascades, including the production and secretion of signaling mediators such as cytokines. Cytokines can be either pro- or anti-inflammatory and modulate the immune response, ensuring pathogen elimination, and removal of cellular debris, while also limiting excessive tissue damage (Seoane et al., 2020).
Cyclic di-adenosine monophosphate produced by pathogens can be recognized by host cells to activate immune responses (Figure 2). c-di-AMP may be recognized by multiple sensors/receptors in eukaryotic cells at the same time after bacterial infection. Therefore, they can precisely regulate the anti-infection status of the organism and affect the growth and spread of bacteria in the host. So far, four c-di-AMP sensors/receptors or adaptors have been found in eukaryotic cells: stimulator of interferon genes (STING) (Aliakbar Tehrani et al., 2021); RNA helicase DDX41 (D-E-A-D [aspartate–glutamate–alanine–aspartate]-box polypeptide 41) (Parvatiyar et al., 2012); reductase controlling The nuclear factor kappa B (NF-kB) (RECON) (McFarland et al., 2017); and endoplasmic reticulum membrane adaptor (ERAdP) (Xia et al., 2018). Hence, c-di-AMP is now considered to be a new PAMP in innate immunity (Dey et al., 2015). It can induce IFN response in a STING-dependent manner and activate the NF-κB pathway, inflammasome, and host autophagy.
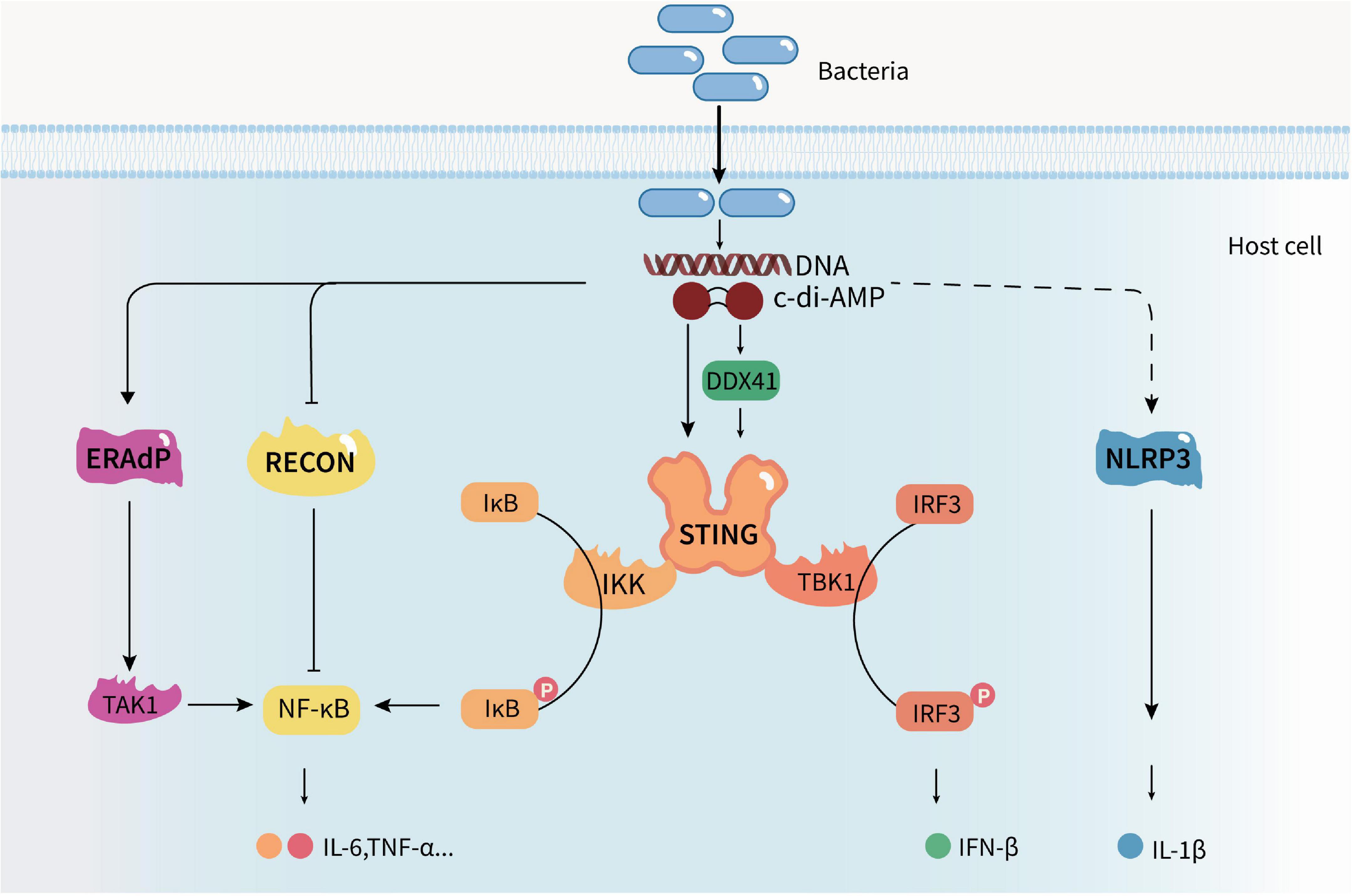
Figure 2. Cyclic di-adenosine monophosphate regulates the host’s innate immune response. c-di-AMP produced by pathogens can be recognized by host cells and activate host innate immune responses. It can induce a type I IFN response in a STING-dependent manner, trigger the nuclear factor kappa B (NF-κB) pathway by activating ERAdP or inhibiting RECON, induce NLRP3-dependent inflammasome response, and activate host cell autophagy, promoting the production and secretion of cytokines.
Cyclic di-adenosine monophosphate induces type I interferon response
The cytosolic surveillance pathway (CSP) is an important immune defense mechanism against bacterial or viral infection. The endoplasmic reticulum (ER)-associated protein STING encompasses a vital part of this immune cytosolic surveillance system. STING functions as a direct PRR of cyclic dinucleotides (CDNs) such as c-di-AMP and c-di-GMP. Originating from intracellular bacteria as an essential adaptor, STING indirectly detects cytosolic DNA through cyclic GMP-AMP (cGAMP) synthase (cGAS) (Aliakbar Tehrani et al., 2021; Guimarães et al., 2021). STING activation culminates in a robust inflammatory response associated with interferon regulatory factor 3 (IRF3) and the subsequent expression of IFN and other coregulated genes (Guimarães et al., 2021). The helicase DDX41 is also a PRR that senses both c-di-GMP and c-di-AMP, and DDX41 has a higher affinity for c-di-GMP than STING. DDX41 recognizes bacterial secondary messengers and forms a complex with STING to signal to TANK-binding kinase 1 (TBK1)-IRF3 activating a type I IFN immune response (Parvatiyar et al., 2012). Type I IFN is associated with efficient immune responses; it can activate host immune cells, such as macrophages and natural killer cells, and boost cell-autonomous defense mechanisms (Lee and Ashkar, 2018).
In Woodward et al. (2010) first identified that c-di-AMP secreted by intracellular Listeria monocytogenes triggers the host type I interferon response. The strong IFN-β-inducing ability of specific strains of L. monocytogenes is due to a genetic defect in tetR (tetracycline resistance transcriptional repressor) that results in the overexpression of mdrT (cholic acid efflux transporter) and a concomitant increase in c-di-AMP secretion (Yamamoto et al., 2012). Increased levels of c-di-AMP during intracellular infection led to the hyperactivation of the CSP. Conditional depletion of the dacA gene also caused increased IFN-β transcription and a concomitant increase in host cell pyroptosis, due to increased bacteriolysis and subsequent bacterial DNA release. Furthermore, c-di-AMP-activated STING-dependent type I IFN production inhibited cell-mediated immunity to L. monocytogenes (Archer et al., 2014). Gries et al. (2016) found that c-di-AMP released from the Staphylococcus aureus biofilm induced a macrophage type I IFN response. Moreover, a recent study demonstrated that thymidine metabolism disruption in S. aureus led to elevated c-di-AMP-mediated STING-dependent inflammation (Tang et al., 2022). These data suggest that c-di-AMP mediates the host innate immune response characterized by IFN-β release via the STING pathway.
Excess c-di-AMP secreted by a DAC-overexpressing M. tuberculosis strain could activate the interferon regulatory factor pathway with elevated levels of IFN-β in a STING-dependent manner, elicit increased macrophage autophagy and attenuate virulence in mice (Dey et al., 2015). The CnpB protein of M. tuberculosis hydrolyzes extracellular bacterial c-di-AMP and mutation of cnpB considerably enhances c-di-AMP secretion and accumulation. Infection with the ΔcnpB strain enhanced IFN-β expression in macrophages and exhibited significant inflammation attenuation. The infection resulted in less bacterial burden in the lungs and the spleen, thereby extending the survival of the mice. This indicated that the deletion of cnpB led to a reduction in the virulence associated with increased c-di-AMP levels (Yang et al., 2014; Dey et al., 2017). Group B Streptococcus also expresses an ectonucleotidase, cdnP, to mediate the hydrolysis of c-di-AMP. Inactivation of CdnP led to IFN-β overproduction, which is due to the cumulative effect of STING-dependent sensing of accumulated c-di-AMP outside the bacteria. Higher IFN-β levels in vivo increase bacterial killing by the host (Andrade et al., 2016). Pde2-deficient S. pneumoniae with elevated c-di-AMP drive aberrant innate immune responses from macrophages involving the hyperactivation of STING, excessive IFN-β expression, and rapid cytotoxicity (Wooten et al., 2020). The effect of the Streptococcus suis SntA (hemin-binding protein) on c-di-AMP is comparable with the activity of cdnP that dampened type I IFN response (Cabezas et al., 2022). These findings reveal that bacteria have developed a unique mechanism to inhibit STING activation and dampen the host cytosolic surveillance pathway. As bacteria can synthesize and degrade c-di-AMP, it suggests that c-di-AMP homeostasis plays a crucial role in governing pathogen infections.
Further studies have revealed that the endogenous ligand 2′,3′-cGAMP generated by cGAS had a higher binding affinity for STING compared with bacterial CDNs such as c-di-GMP, c-di-AMP, and 3′,3′-cGAMP (Gao et al., 2013). The extensive interactions and shape complementarity between asymmetric 2′,3′-cGAMP and the ligand-binding pocket make it the most preferred ligand for porcine STING. That geometry constraint limits the binding between symmetric 3′,3′-CDN and porcine STING (Cong et al., 2019). The ligand recognition and discrimination mechanism of porcine STING observed here expands our understanding of the activation of the CDN-STING pathway and its role in anti-pathogen defense. The 4′-thiomodified c-di-AMP analog, synthesized via the replacement of oxygen atoms with sulfur atoms at the 4′-position on the furanose ring of c-di-AMP, acted as a potent STING agonist and resulted in an enhanced immunostimulatory effect (Saito-Tarashima et al., 2021). Louie et al. (2020) explored the in vivo role of the STING pathway during bacterial pathogenesis and found that STING activation led to a reduced bacterial burden and correlated with the recruitment of monocytes to the intestines during L. monocytogenes-induced enterocolitis. This STING-mediated protective response was triggered by the c-di-AMP secretion of L. monocytogenes, while the disruption of type I IFN signaling did not recapitulate STING deficiency. These data suggest that STING signaling could provide pronounced contributions to immune responses at the initial site of infection in the mucosal barrier and that the activity of other immune sensors can compensate for STING function at later stages of infection.
Released type I IFN can bind to the IFN receptor, activating STATs (signal transducer and activator of transcription), mitogen-activated protein kinase (MAPK), and phosphatidylinositol 3-kinase (PI3K) signal pathways, regulating various physiological activities and initiating anti-pathogen responses. Mahmoud et al. (2019) demonstrated that c-di-AMP could specifically enhance the expression of additional inflammatory cytokines, namely, IL-6, CXCL2, CCL3, and CCL4, the mRNAs of which contain AU-rich elements (AREs) in the 3′ UTR that promote decay and repress translation. c-di-AMP was able to promote the phosphorylation of p38 MAPK as well as the induction of the ARE-binding protein TTP, both of which are components of a signaling pathway that modulate the expression of ARE-containing mRNAs at the post-transcriptional level. Pharmacological inhibition of p38 reduced the c-di-AMP-dependent release of induced cytokines, while TTP knockdown increased their release and mRNA stability (Mahmoud et al., 2019). These data propose that c-di-AMP can activate the p38 MAPK pathway via a post-transcriptional regulatory effect and subsequently enhance the expression of inflammatory cytokines.
Also worth noting is that c-di-AMP could significantly induce IFN-β response in dendritic cells. However, upon continuous cell stimulation, c-di-AMP induced downregulation of STING due to proteolytic degradation, leading to a decrease in IFN-β secretion (Rueckert et al., 2017). It suggests that bacteria-derived c-di-AMP can induce a host immune response, but the effect may be self-limited and discontinuous. It is indicated that the host has corresponding regulatory mechanisms to avoid an excessive c-di-AMP-induced immune response leading to immunopathologic damage.
Cyclic di-adenosine monophosphate activates nuclear factor kappa B pathway
The nuclear factor kappa B is a ubiquitously expressed transcription factor that controls the expression of important regulatory genes that are responsible for various biological processes like immune responses, inflammation, cell proliferation, and apoptosis (Zinatizadeh et al., 2020). In the immune responses, NF-κB regulates the expression of many target genes that are playing critical roles in anti-infection and anti-inflammation processes such as immune recognition receptors, cytokines, antigen-presenting proteins, adhesion molecules, and chemokines.
Nuclear factor kappa B protein is located in the cytoplasm and can be activated by various cellular stimuli. Oxidoreductase RECON was revealed to negatively regulate NF-κB and STING activation. McFarland et al. (2017) identified that RECON functions as a cytosolic sensor for bacterial cyclic dinucleotides. High-affinity c-di-AMP binding inhibits RECON enzyme activity by simultaneously blocking the substrate and cosubstrate sites, inducing NF-κB activation and reducing bacterial survival. This suggests that c-di-AMP inhibition of RECON promotes a pro-inflammatory, anti-bacterial condition via STING and NF-κB pathways. However, other research by the same group demonstrated that L. monocytogenes secretion of c-di-AMP inhibits the enzymatic activity of RECON resulting in enhanced NF-κB activation and nitric oxide production, ultimately promoting cell-to-cell spread (McFarland et al., 2018). This finding is different from the previous recognization that c-di-AMP is capable of helping the host to clear the pathogenic bacterial infection. This indicates that despite c-di-AMP stimulation, the host immune response to infection depends on the type of pathogen.
The ERAdP acts as a direct sensor for c-di-AMP. c-di-AMP is bound to the C-terminal domain of ERAdP, which in turn leads to the dimerization of ERAdP, resulting in association with and activation of the kinase TAK1. TAK1 activation consequently induces the activation of the transcription factor NF-κB to induce the production of pro-inflammatory cytokines in innate immune cells (Xia et al., 2018). Moreover, ERAdP-deficient mice were highly susceptible to L. monocytogenes infection and exhibited reduced pro-inflammatory cytokines. Double knockout of ERAdP and TAK1 resulted in heightened susceptibility to L. monocytogenes infection. These data suggest that c-di-AMP is critical for controlling bacterial infection and can activate the NF-κB pathway through ERAdP mediation (Xia et al., 2018).
Cyclic di-adenosine monophosphate activates inflammasome
The inflammasome is an essential component of the natural immune system (Lamkanfi and Dixit, 2014). The inflammasome is a family of multi-protein complexes comprising a cytosolic PRR, an adaptor protein called ASC (apoptosis-associated speck-like protein containing a caspase recruitment domain), and the protease caspase-1 (formed when the scaffolding PRR senses or binds to its activating stimuli). Following activation in inflammasomes, caspase-1 cleaves the inactive cytokine precursors pro-interleukin (IL)-1β and pro-IL-18, which subsequently leads to the secretion of their active forms (Liston and Masters, 2017). In addition, caspase-1 cleaves gasdermin D, which forms pores in the plasma membrane and, in many settings, can induce a rapid form of cell death called pyroptosis (Shi et al., 2015). Although several inflammasomes have been described (NLRP1b, NLRP3, NLRC4, and AIM2), the best-characterized inflammasome is formed by the NLR family pyrin domain-containing 3 (NLRP3) (Mangan et al., 2018). NLRP3 is mainly distributed in the cytosol and intracellular membrane of macrophages and neutrophils and is closely related to viruses and intracellular bacterial infection.
In previous studies, it was found that physiological levels of both c-di-AMP and c-di-GMP could induce the expression of caspase-1 and stimulate a robust secretion of IL-1β through the NLRP3 inflammasome (Abdul-Sater et al., 2013). However, this process was independent of STING. The response to c-di-GMP is dependent on the mobilization of potassium and calcium ions and is not associated with significant changes in mitochondrial potential or the generation of mitochondrial reactive oxygen species. It suggests that cyclic dinucleotides activate the NLRP3 inflammasome through a unique pathway that could have evolved to detect pervasive bacterial PAMPs associated with intracellular infections (Abdul-Sater et al., 2013). A recent study demonstrated that a combination adjuvant composed of c-di-AMP and a plant-derived nanoparticle significantly elicited an enhanced immune response to intradermal-injected vaccines in mice and pigs. The combination of adjuvant-induced robust activation of both NF-κB and IFN regulatory factor signaling pathways and the NLRP3 inflammasome, with increased expression of costimulatory molecules and secretion of TNF and IL-1β (Hernandez-Franco et al., 2021). Therefore, c-di-AMP activates an inflammasome that facilitates host anti-infection.
Cyclic di-adenosine monophosphate activates host autophagy
Autophagy is a ubiquitous and fundamental eukaryotic pathway that has multiple effects on immunity. Autophagy contributes to the direct elimination of intracellular microbes, inflammation control, antigen presentation, lymphocyte homeostasis, and the secretion of immune mediators (Deretic et al., 2013). The microtubule-associated protein 1 (MAP1) light chain 3 (LC3) on the autophagosome membrane and isolation membranes is a reliable marker for the molecule of autophagosomes (Yoshimori, 2004).
Cyclic dinucleotides have been reported as potent autophagy inducers. Dey et al. (2015) first found that the expression of autophagosome membrane-specific markers (LC3-I and LC3-II) increased significantly in macrophages infected with recombinant M. tuberculosis overexpressing c-di-AMP synthetase. It is indicated that c-di-AMP activated autophagy, which resulted in the attenuation of the intracellular growth of bacteria in infected cells. Moretti et al. (2017) demonstrated that c-di-AMP in live Gram-positive bacteria served as a vita-PAMP, a specific class of PAMP that denotes microbial viability and elicits a commensurate innate response. c-di-AMP produced by bacteria was detected by the innate sensor STING, which then quickly mobilized interdependent pre-formed cell-autonomous responses, namely, ER stress, MTOR inactivation, and reticulophagy. ER stress induced macroautophagy/autophagy sequestered stressed ER membranes, resolved ER stress, and prevented apoptosis in response to live bacteria. This vita-PAMP-induced ER-phagy additionally orchestrates a type I IFN response by localizing ER-resident STING to autophagosomes (Moretti et al., 2017; Moretti and Blander, 2018). These findings identified stress-mediated ER-phagy as a cell-autonomous response mobilized by STING-dependent sensing of a specific vita-PAMP and elucidated how innate receptors engage multilayered homeostatic mechanisms to promote immunity and survival after infection. Ning et al. (2019) found that c-di-AMP evidently initiated autophagy with the elevated expression of genes associated with autophagy in macrophages. However, recombinant BCG vaccine formulated with c-di-AMP does not affect the level of LC3 (Ning et al., 2021). Another study revealed that the recombinant vaccine blocks autophagic flux by inhibiting p62 degradation, the level of which is negatively correlated with autophagy (Shen et al., 2022).
Autophagy is a precise regulatory process involving multiple genes. Factors such as nutrition deficiency and infection can induce autophagy, and the lack of inhibition of the autophagy signal is one of the important reasons for the immune escape of intracellular bacteria (Alharbi et al., 2021). Therefore, the activation of autophagy by increasing the level of c-di-AMP may assist the host in resisting infection by pathogenic microbes.
Cyclic di-adenosine monophosphate triggers mucosal immune response as a mucosal adjuvant
The mucosal immune system is an immune response network composed of mucosal epithelium and secretion, mucosa-related lymphoid tissue, immunocompetent cells, and microflora that are widely distributed in the respiratory tract, gastrointestinal tract, urogenital tract, and their associated exocrine glands. Mucosal immunity is an immune response in local mucosal tissue induced by pathogens, food antigens, allergens, and other stimuli (Mettelman et al., 2022).
New effective adjuvants are necessary to boost and modulate evoked immune responses of subunit vaccines. Ebensen et al. (2011) found that c-di-AMP exerted strong adjuvant properties when delivered by the mucosal route. c-di-AMP is capable of stimulating macrophages as well as dendritic cells. Intranasal delivery of β-galactosidase adjuvanted with c-di-AMP to mice elicited significantly higher serum antigen-specific IgG and IgA titers compared with those in controls. The cellular immune responses were found to be strong with a balanced Th1/Th2/Th17 response pattern (Ebensen et al., 2011). After subcutaneous vaccination, recombinant vaccine adjuvanted with the STING-agonist c-di-AMP induced a significantly stronger Th1, IL-17, and IFNγ-producing cell response, outperforming other formulations, such as poly(I:C)/CpG and Tc52 (titanium salan complex) (Matos et al., 2017; Volckmar et al., 2019). Overexpressing DisA, the DAC of M. tuberculosis, in recombinant BCG increased IFN-β, IL-1, IL-2, IL-6, and IL-10 secretion, increased the expression of H3K4me3, an epigenetic marker of innate immune memory, and decreased bacterial burdens in the lungs and the spleen (Ning et al., 2019; Dey et al., 2020). The combination of the lipopeptide (Mycoplasma fermentans-derived)-based nanocarrier systems used for efficient antigen delivery across the mucosal barrier with c-di-AMP, allowed antigen dose reduction while maintaining vaccine efficacy against a respiratory challenge with a heterologous Streptococcus pyogenes strain (Schulze et al., 2017a). Nasal vaccination with BtaF (bacterial trimeric autotransporter adhesin) + c-di-AMP protects against intragastric challenge with Brucella suis by inducing local and systemic antibody responses with increased IL-2, IL-5, IL-17, and IFN-γ secretion, central memory CD4+ T cells and strong Th1 responses (Muñoz González et al., 2019). Oral administration of a genetically engineered Lactococcus lactis strain that simultaneously synthesizes the adjuvant c-di-AMP as well as a heterologous antigen is able to elicit a specific immune response, representing a new design strategy to develop a mucosal vaccine prototype (Quintana et al., 2018).
Vaccines adjuvanted with c-di-AMP induce strong humoral and cellular immune responses and protect against virus challenges. Recombinant hepatitis C virus vaccines adjuvanted with c-di-AMP evoked comparable neutralizing antibodies but a more robust cellular immune response, as revealed by strongly activated CD4+ T cells, compared with adjuvants using aluminum hydroxide/monophosphoryl lipid A (Alum/monophos./MPLA) and MF59 (an oil-in-water emulsion-based adjuvant) (Landi et al., 2017). c-di-AMP served as an adjuvant and provided efficient protection against influenza H5N1/H1N1 via the intranasal route in mice (Ebensen et al., 2017; Schulze et al., 2017b). In addition, c-di-AMP can also be combined with other adjuvants like alum, to produce a marked humoral and cellular immune response (Ebensen et al., 2019).
Furthermore, STING-agonist c-di-AMP coated on microneedles induced significantly higher IgG2a antibodies in serum as well as a higher level of Th1 cytokines (IFN-γ and IL-2) than that in a control group, suggesting that c-di-AMP could fulfill the role of adjuvants for microneedle-based skin allergen-specific immunotherapy (Shakya et al., 2018). To sum up, c-di-AMP promoted (i) effective local and systemic humoral immune responses, including protective hemagglutination inhibition titers; (ii) effective cellular immune responses, including multifunctional T-cell activity; (iii) induction of long-lasting immunity; and (iv) protection against bacterial or viral challenge (Ebensen et al., 2017; Schulze et al., 2017b). Hence, c-di-AMP, which has effective adjuvant properties, is a promising immunomodulator for the development of mucosal vaccines.
Conclusion and outlook
As a microbial second messenger, c-di-AMP plays an important role in the regulation of bacterial physiological activities such as the cell cycle, cell wall stability, environmental stress response, and biofilm formation. Moreover, c-di-AMP produced by pathogens can be recognized by host cells and activate host innate immune responses. It can induce a type I IFN response in a STING-dependent manner, trigger the NF-κB pathway by activating ERAdP or inhibiting RECON, induce an NLRP3-dependent inflammasome response, and activate host cell autophagy, promoting the production and secretion of signaling mediators such as cytokines, thus modulating the host defense against infection. In addition, c-di-AMP is able to trigger a host mucosal immune response as a mucosal adjuvant. It can promote effective humoral and cellular immune responses, facilitating the induction of long-lasting immunity and protection against bacterial or viral challenges.
It is very important to gain more knowledge about the regulation of c-di-AMP production and degradation, and the role of c-di-AMP in bacterial physiological activities, environmental stress adaptation, and virulence. Also worth noting is that the role of c-di-AMP in archaea remains unclear, making it a valuable target for further study. Research on the effects of c-di-AMP on microbial physiology will lay a foundation for further studies regarding the immunomodulation activity of c-di-AMP. The roles of c-di-AMP-mediated bacteria and host interaction in the occurrence and development of diseases are yet unclear. In previous investigations, researchers found that c-di-AMP could regulate the gingival epithelial cytokine response and affect critical processes of gingival fibroblasts, playing a substantial role in the pathogenesis of the periodontal disease (Elmanfi et al., 2018, 2020; Onyedibe et al., 2021). More studies are needed to explore the regulatory effect of bacterial c-di-AMP on the immune microenvironment and the underlying mechanisms. Understanding the stimulation of the immune response by c-di-AMP may enable new approaches to achieve multilevel control of complex homeostatic processes in diseases and develop novel immunotherapies. In addition to acting as a mucosal adjuvant, c-di-AMP has gained attention in cancer immunotherapy. Vasiyani et al. (2021) demonstrated that c-di-AMP-activated and modulated the STING pathway to induce mitochondrial-mediated apoptosis in estrogen-receptor negative breast cancer cells. The combinatorial use of TLR9- and STING-agonist c-di-AMP induced innate and adaptive IFN-γ and exerted robust anti-tumor activities (Temizoz et al., 2022). Re-engineered BCG overexpressing c-di-AMP augmented trained immunity and exhibited improved efficacy against bladder cancer (Singh et al., 2022). In addition, small molecules have been designed to modulate the cyclic dinucleotide-cGAS-STING axis (Sintim et al., 2019), and inhibitors targeting M. tuberculosis cyclic dinucleotide phosphodiesterase have been identified in the regulation of the innate immune system (Karanja et al., 2021).
Also worth noting is that both c-di-AMP and c-di-GMP are critical bacterial second messengers regulating bacterial physiological activities and host immune responses. c-di-GMP is synthesized from two guanosine triphosphate (GTP) molecules by diguanylate cyclases (DGCs) and is degraded into pGpG by phosphodiesterases. While c-di-AMP is widely distributed in Gram-positive bacteria, c-di-GMP and its metabolizing enzymes have been identified in many Gram-negative bacteria such as Pseudomonas aeruginosa, Caulobacter crescentus, and Escherichia coli, and a few Gram-positive bacteria such as B. subtilis and L. monocytogenes (Ross et al., 1987; Kalia et al., 2013). c-di-GMP mainly regulates the bacterial motility-to-sessility transition, biofilm formation, and virulence factor production (Randall et al., 2022). Both c-di-AMP and c-di-GMP can trigger type I interferon response. However, c-di-GMP has a higher affinity for DDX41 compared with that STING (Burdette et al., 2011; Parvatiyar et al., 2012; Li et al., 2019). Intranasal c-di-GMP administration did not cause strong inflammatory responses or lung injury but enhanced type II (IFN-γ)/III IFN (IFN-λ) production and Th1, Th2, and Th17 responses with the production of polarizing cytokines, namely, IL-6, IL-12, IL-13, IL-23, and TGF-β in mice (Yan et al., 2009; Blaauboer et al., 2015). Moreover, c-di-AMP and c-di-GMP activate the NLRP3 inflammasome through a unique pathway (Abdul-Sater et al., 2013). Therefore, cyclic nucleotide signaling molecules act in a highly specific manner forming a multilayered signaling scaffold. More studies are needed to elucidate the function of cyclic nucleotides, such as c-di-AMP and c-di-GMP, in bacterial physiology as well as in the host immune system. The associations among these cyclic nucleotides also need further exploration and may provide novel targets for preventing infections.
In conclusion, c-di-AMP plays a major role in host immune responses and has become a promising target in vaccine adjuvants and immunotherapy.
Author contributions
XC and JN drafted the manuscript. XX and XZ edited and added valuable insights to the manuscript. All authors approved the final manuscript and agreed to be accountable for all aspects of the work.
Funding
This study was supported by the National Natural Science Foundation (82101002), the Postdoctoral Fund of Sichuan University (2021SCU12113), and the Research Funding from West China Hospital of Stomatology Sichuan University (RCDWJS2021-16).
Conflict of interest
The authors declare that the research was conducted in the absence of any commercial or financial relationships that could be construed as a potential conflict of interest.
The reviewer JL declared a shared affiliation with the author XC to the handling editor at the time of review.
Publisher’s note
All claims expressed in this article are solely those of the authors and do not necessarily represent those of their affiliated organizations, or those of the publisher, the editors and the reviewers. Any product that may be evaluated in this article, or claim that may be made by its manufacturer, is not guaranteed or endorsed by the publisher.
Abbreviations
c-di-AMP, cyclic di-adenosine monophosphate; cAMP, cyclic adenosine monophosphate; c-di-GMP, cyclic di-guanosine monophosphate; cGAMP, cyclic GMP-AMP; ppGpp, guanosine tetraphosphate; DAC, diadenylate cyclase; PAMPs, pathogen-associated molecular patterns; DAMPs, damage-associated molecular patterns; PRRs, pattern recognition receptors; STING, stimulator of interferon genes; DDX41, D-E-A-D [aspartate–glutamate–alanine–aspartate]-box polypeptide 41; RECON, reductase controlling NF-κB; ERAdP, endoplasmic reticulum membrane adaptor; CSP, cytosolic surveillance pathway; CDNs, cyclic dinucleotides; IFN, type I interferon; MAPK, mitogen-activated protein kinase; NF-κB, nuclear factor kappa B; NLRP3, nucleotide oligomerization domain-like receptor family pyrin domain-containing 3.
Footnotes
References
Abdul-Sater, A. A., Tattoli, I., Jin, L., Grajkowski, A., Levi, A., Koller, B. H., et al. (2013). Cyclic-di-GMP and cyclic-di-AMP activate the NLRP3 inflammasome. EMBO Rep. 14, 900–906. doi: 10.1038/embor.2013.132
Alharbi, Y. M., Bima, A. I., and Elsamanoudy, A. Z. (2021). An overview of the perspective of cellular autophagy: mechanism, regulation, and the role of autophagy dysregulation in the pathogenesis of diseases. J. Microsc. Ultrastruct. 9, 47–54. doi: 10.4103/JMAU.JMAU_33_20
Aliakbar Tehrani, Z., Rulíšek, L., and Èernı, J. (2021). Molecular dynamics simulations provide structural insight into binding of cyclic dinucleotides to human STING protein. J. Biomol. Struct. Dyn. [Epub ahead of print]. doi: 10.1080/07391102.2021.1942213
Andrade, W. A., Firon, A., Schmidt, T., Hornung, V., Fitzgerald, K. A., Kurt-Jones, E. A., et al. (2016). Group B Streptococcus degrades cyclic-di-AMP to modulate STING-dependent type I interferon production. Cell Host Microbe 20, 49–59. doi: 10.1016/j.chom.2016.06.003
Archer, K. A., Durack, J., and Portnoy, D. A. (2014). STING-dependent type I IFN production inhibits cell-mediated immunity to Listeria monocytogenes. PLoS Pathog. 10:e1003861. doi: 10.1371/journal.ppat.1003861
Bai, Y., Yang, J., Eisele, L. E., Underwood, A. J., Koestler, B. J., Waters, C. M., et al. (2013). Two DHH subfamily 1 proteins in Streptococcus pneumoniae possess cyclic di-AMP phosphodiesterase activity and affect bacterial growth and virulence. J. Bacteriol. 195, 5123–5132. doi: 10.1128/JB.00769-13
Bai, Y., Yang, J., Zarrella, T. M., Zhang, Y., Metzger, D. W., and Bai, G. (2014). Cyclic di-AMP impairs potassium uptake mediated by a cyclic di-AMP binding protein in Streptococcus pneumoniae. J. Bacteriol. 196, 614–623. doi: 10.1128/JB.01041-13
Blaauboer, S. M., Mansouri, S., Tucker, H. R., Wang, H. L., Gabrielle, V. D., and Jin, L. (2015). The mucosal adjuvant cyclic di-GMP enhances antigen uptake and selectively activates pinocytosis-efficient cells in vivo. eLife 4:e06670. doi: 10.7554/eLife.06670
Bowman, L., Zeden, M. S., Schuster, C. F., Kaever, V., and Gründling, A. (2016). New insights into the cyclic di-adenosine monophosphate (c-di-AMP) degradation pathway and the requirement of the cyclic dinucleotide for acid stress resistance in Staphylococcus aureus. J. Biol. Chem. 291, 26970–26986. doi: 10.1074/jbc.M116.747709
Boye, E. (2006). DisA, a busy bee that monitors chromosome integrity. Cell 125, 641–643. doi: 10.1016/j.cell.2006.05.004
Braun, F., Thomalla, L., van der Does, C., Quax, T., Allers, T., Kaever, V., et al. (2019). Cyclic nucleotides in archaea: cyclic di-AMP in the archaeon Haloferax volcanii and its putative role. MicrobiologyOpen 8:e00829. doi: 10.1002/mbo3.829
Burdette, D. L., Monroe, K. M., Sotelo-Troha, K., Iwig, J. S., Eckert, B., Hyodo, M., et al. (2011). STING is a direct innate immune sensor of cyclic di-GMP. Nature 478, 515–518. doi: 10.1038/nature10429
Cabezas, A., Costas, M. J., Canales, J., Pinto, R. M., Rodrigues, J. R., Ribeiro, J. M., et al. (2022). Enzyme characterization of pro-virulent SntA, a cell wall-anchored protein of Streptococcus suis, with phosphodiesterase activity on cyclic-di-AMP at a level suited to limit the innate immune system. Front. Microbiol. 13:843068. doi: 10.3389/fmicb.2022.843068
Cao, M., Kobel, P. A., Morshedi, M. M., Wu, M. F., Paddon, C., and Helmann, J. D. (2002). Defining the Bacillus subtilis sigma(W) regulon: a comparative analysis of promoter consensus search, run-off transcription/macroarray analysis (ROMA), and transcriptional profiling approaches. J. Mol. Biol. 316, 443–457. doi: 10.1006/jmbi.2001.5372
Cereija, T. B., Guerra, J., Jorge, J., and Morais-Cabral, J. H. (2021). c-di-AMP, a likely master regulator of bacterial K+ homeostasis machinery, activates a K+ exporter. Proc. Natl. Acad. Sci. U.S.A. 118:e2020653118. doi: 10.1073/pnas.2020653118
Chen, L., Li, X., Zhou, X., Zeng, J., Ren, Z., Lei, L., et al. (2018). Inhibition of Enterococcus faecalis growth and biofilm formation by molecule targeting cyclic di-AMP synthetase activity. J. Endod. 44, 1381.e2–1388.e2. doi: 10.1016/j.joen.2018.05.008
Cheng, X., Zheng, X., Zhou, X., Zeng, J., Ren, Z., Xu, X., et al. (2016). Regulation of oxidative response and extracellular polysaccharide synthesis by a diadenylate cyclase in Streptococcus mutans. Environ. Microbiol. 18, 904–922. doi: 10.1111/1462-2920.13123
Cho, K. H., and Kang, S. O. (2013). Streptococcus pyogenes c-di-AMP phosphodiesterase, GdpP, influences SpeB processing and virulence. PLoS One 8:e69425. doi: 10.1371/journal.pone.0069425
Choi, P. H., Vu, T., Pham, H. T., Woodward, J. J., Turner, M. S., and Tong, L. (2017). Structural and functional studies of pyruvate carboxylase regulation by cyclic di-AMP in lactic acid bacteria. Proc. Natl. Acad. Sci. U.S.A. 114, E7226–E7235. doi: 10.1073/pnas.1704756114
Cong, X., Yuan, Z., Du, Y., Wu, B., Lu, D., Wu, X., et al. (2019). Crystal structures of porcine STINGCBD-CDN complexes reveal the mechanism of ligand recognition and discrimination of STING proteins. J. Biol. Chem. 294, 11420–11432. doi: 10.1074/jbc.RA119.007367
Corrigan, R. M., Abbott, J. C., Burhenne, H., Kaever, V., and Gründling, A. (2011). c-di-AMP is a new second messenger in Staphylococcus aureus with a role in controlling cell size and envelope stress. PLoS Pathog. 7:e1002217. doi: 10.1371/journal.ppat.1002217
Corrigan, R. M., Campeotto, I., Jeganathan, T., Roelofs, K. G., Lee, V. T., and Gründling, A. (2013). Systematic identification of conserved bacterial c-di-AMP receptor proteins. Proc. Natl. Acad. Sci. U.S.A. 110, 9084–9089. doi: 10.1073/pnas.1300595110
Deretic, V., Saitoh, T., and Akira, S. (2013). Autophagy in infection, inflammation and immunity. Nat. Rev. Immunol. 13, 722–737. doi: 10.1038/nri3532
Devaux, L., Sleiman, D., Mazzuoli, M. V., Gominet, M., Lanotte, P., Trieu-Cuot, P., et al. (2018). Cyclic di-AMP regulation of osmotic homeostasis is essential in Group B Streptococcus. PLoS Genet. 14:e1007342. doi: 10.1371/journal.pgen.1007342
Dey, B., Dey, R. J., Cheung, L. S., Pokkali, S., Guo, H., Lee, J. H., et al. (2015). A bacterial cyclic dinucleotide activates the cytosolic surveillance pathway and mediates innate resistance to tuberculosis. Nat. Med. 21, 401–406. doi: 10.1038/nm.3813
Dey, R. J., Dey, B., Singh, A. K., Praharaj, M., and Bishai, W. (2020). Bacillus Calmette-Guérin overexpressing an endogenous stimulator of interferon genes agonist provides enhanced protection against pulmonary tuberculosis. J. Infect. Dis. 221, 1048–1056. doi: 10.1093/infdis/jiz116
Dey, R. J., Dey, B., Zheng, Y., Cheung, L. S., Zhou, J., Sayre, D., et al. (2017). Inhibition of innate immune cytosolic surveillance by an M. tuberculosis phosphodiesterase. Nat. Chem. Biol. 13, 210–217. doi: 10.1038/nchembio.2254
Du, B., Ji, W., An, H., Shi, Y., Huang, Q., Cheng, Y., et al. (2014). Functional analysis of c-di-AMP phosphodiesterase, GdpP, in Streptococcus suis serotype 2. Microbiol. Res. 169, 749–758. doi: 10.1016/j.micres.2014.01.002
Ebensen, T., Debarry, J., Pedersen, G. K., Blazejewska, P., Weissmann, S., and Schulze, K. (2017). Mucosal administration of cycle-di-nucleotide-adjuvanted virosomes efficiently induces protection against influenza H5N1 in mice. Front. Immunol. 8:1223. doi: 10.3389/fimmu.2017.01223
Ebensen, T., Delandre, S., Prochnow, B., Guzmán, C. A., and Schulze, K. (2019). The combination vaccine adjuvant system alum/c-di-AMP results in quantitative and qualitative enhanced immune responses post immunization. Front. Cell. Infect. Microbiol. 9:31. doi: 10.3389/fcimb.2019.00031
Ebensen, T., Libanova, R., Schulze, K., Yevsa, T., Morr, M., and Guzmán, C. A. (2011). Bis-(3’,5’)-cyclic dimeric adenosine monophosphate: strong Th1/Th2/Th17 promoting mucosal adjuvant. Vaccine 29, 5210–5220. doi: 10.1016/j.vaccine.2011.05.026
Elmanfi, S., Sintim, H. O., Zhou, J., Gürsoy, M., Könönen, E., and Gürsoy, U. K. (2020). Activation of gingival fibroblasts by bacterial cyclic dinucleotides and lipopolysaccharide. Pathogens 9:792. doi: 10.3390/pathogens9100792
Elmanfi, S., Zhou, J., Sintim, H. O., Könönen, E., Gürsoy, M., and Gürsoy, U. K. (2018). Regulation of gingival epithelial cytokine response by bacterial cyclic dinucleotides. J. Oral Microbiol. 11:1538927. doi: 10.1080/20002297.2018.1538927
Fahmi, T., Faozia, S., Port, G. C., and Cho, K. H. (2019). The second messenger c-di-AMP regulates diverse cellular pathways involved in stress response, biofilm formation, cell wall homeostasis, SpeB expression, and virulence in Streptococcus pyogenes. Infect. Immun. 87:e00147-19. doi: 10.1128/IAI.00147-19
Gall, A. R., Hsueh, B. Y., Siletti, C., Waters, C. M., and Huynh, T. N. (2022). NrnA is a linear dinucleotide phosphodiesterase with limited function in cyclic dinucleotide metabolism in Listeria monocytogenes. J. Bacteriol. 204:e0020621. doi: 10.1128/JB.00206-21
Gao, P., Ascano, M., Zillinger, T., Wang, W., Dai, P., Serganov, A. A., et al. (2013). Structure-function analysis of STING activation by c[G(2’,5’)pA(3’,5’)p] and targeting by antiviral DMXAA. Cell 154, 748–762. doi: 10.1016/j.cell.2013.07.023
Gibhardt, J., Hoffmann, G., Turdiev, A., Wang, M., Lee, V. T., and Commichau, F. M. (2019). c-di-AMP assists osmoadaptation by regulating the Listeria monocytogenes potassium transporters KimA and KtrCD. J. Biol. Chem. 294, 16020–16033. doi: 10.1074/jbc.RA119.010046
Gries, C. M., Bruger, E. L., Moormeier, D. E., Scherr, T. D., Waters, C. M., and Kielian, T. (2016). Cyclic di-AMP released from Staphylococcus aureus biofilm induces a macrophage type I interferon response. Infect. Immun. 84, 3564–3574. doi: 10.1128/IAI.00447-16
Guimarães, E. S., Marinho, F. V., de Queiroz, N., Antunes, M. M., and Oliveira, S. C. (2021). Impact of STING inflammatory signaling during intracellular bacterial infections. Cells 11:74. doi: 10.3390/cells11010074
Hernandez-Franco, J. F., Mosley, Y. C., Franco, J., Ragland, D., Yao, Y., and HogenEsch, H. (2021). Effective and safe stimulation of humoral and cell-mediated immunity by intradermal immunization with a cyclic dinucleotide/nanoparticle combination adjuvant. J. Immunol. 206, 700–711. doi: 10.4049/jimmunol.2000703
Huynh, T. N., Choi, P. H., Sureka, K., Ledvina, H. E., Campillo, J., Tong, L., et al. (2016). Cyclic di-AMP targets the cystathionine beta-synthase domain of the osmolyte transporter OpuC. Mol. Microbiol. 102, 233–243. doi: 10.1111/mmi.13456
Jervis, A. J., Thackray, P. D., Houston, C. W., Horsburgh, M. J., and Moir, A. (2007). SigM-responsive genes of Bacillus subtilis and their promoters. J. Bacteriol. 189, 4534–4538. doi: 10.1128/JB.00130-07
Kalia, D., Merey, G., Nakayama, S., Zheng, Y., Zhou, J., Luo, Y., et al. (2013). Nucleotide, c-di-GMP, c-di-AMP, cGMP, cAMP, (p)ppGpp signaling in bacteria and implications in pathogenesis. Chem. Soc. Rev. 42, 305–341. doi: 10.1039/c2cs35206k
Karanja, C. W., Yeboah, K. S., and Sintim, H. O. (2021). Identification of a Mycobacterium tuberculosis cyclic dinucleotide phosphodiesterase inhibitor. ACS Infect. Dis. 7, 309–317. doi: 10.1021/acsinfecdis.0c00444
Konno, H., Yoshida, Y., Nagano, K., Takebe, J., and Hasegawa, Y. (2018). Biological and biochemical roles of two distinct cyclic dimeric adenosine 3’,5’-monophosphate- associated phosphodiesterases in Streptococcus mutans. Front. Microbiol. 9:2347. doi: 10.3389/fmicb.2018.02347
Krüger, L., Herzberg, C., Wicke, D., Scholz, P., Schmitt, K., Turdiev, A., et al. (2022). Sustained control of pyruvate carboxylase by the essential second messenger cyclic di-AMP in Bacillus subtilis. mBio 13:e0360221. doi: 10.1128/mbio.03602-21
Kumar, S., Ingle, H., Prasad, D. V., and Kumar, H. (2013). Recognition of bacterial infection by innate immune sensors. Crit. Rev. Microbiol. 39, 229–246. doi: 10.3109/1040841X.2012.706249
Kundra, S., Lam, L. N., Kajfasz, J. K., Casella, L. G., Andersen, M. J., Abranches, J., et al. (2021). c-di-AMP is essential for the virulence of Enterococcus faecalis. Infect. Immun. 89:e0036521. doi: 10.1128/IAI.00365-21
Lamkanfi, M., and Dixit, V. M. (2014). Mechanisms and functions of inflammasomes. Cell 157, 1013–1022. doi: 10.1016/j.cell.2014.04.007
Landi, A., Law, J., Hockman, D., Logan, M., Crawford, K., Chen, C., et al. (2017). Superior immunogenicity of HCV envelope glycoproteins when adjuvanted with cyclic-di-AMP, a STING activator or archaeosomes. Vaccine 35, 6949–6956. doi: 10.1016/j.vaccine.2017.10.072
Lee, A. J., and Ashkar, A. A. (2018). The dual nature of type I and type II interferons. Front. Immunol. 9:2061. doi: 10.3389/fimmu.2018.02061
Li, L., Li, Y., Zhu, F., Cheung, A. L., Wang, G., Bai, G., et al. (2021). New mechanistic insights into purine biosynthesis with second messenger c-di-AMP in relation to biofilm-related persistent methicillin-resistant Staphylococcus aureus infections. mBio 12:e0208121. doi: 10.1128/mBio.02081-21
Li, Z., Zhang, X., Cheng, L., Xu, X., Zhou, X., Wu, H., et al. (2019). Signal transduction ofsStreptococci by cyclic dinucleotide second messengers. Curr. Issues Mol. Biol. 32, 87–122. doi: 10.21775/cimb.032.087
Liston, A., and Masters, S. L. (2017). Homeostasis-altering molecular processes as mechanisms of inflammasome activation. Nat. Rev. Immunol. 17, 208–214. doi: 10.1038/nri.2016.151
Louie, A., Bhandula, V., and Portnoy, D. A. (2020). Secretion of c-di-AMP by Listeria monocytogenes leads to a STING-dependent antibacterial response during enterocolitis. Infect. Immun. 88, e407–e420. doi: 10.1128/IAI.00407-20
Luo, Y., and Helmann, J. D. (2012). Analysis of the role of Bacillus subtilis σ(M) in β-lactam resistance reveals an essential role for c-di-AMP in peptidoglycan homeostasis. Mol. Microbiol. 83, 623–639. doi: 10.1111/j.1365-2958.2011.07953.x
Mahmoud, L., Abdulkarim, A. S., Kutbi, S., Moghrabi, W., Altwijri, S., Khabar, K., et al. (2019). Post-transcriptional inflammatory response to intracellular bacterial c-di-AMP. Front. Immunol. 10:3050. doi: 10.3389/fimmu.2019.03050
Mangan, M., Olhava, E. J., Roush, W. R., Seidel, H. M., Glick, G. D., and Latz, E. (2018). Targeting the NLRP3 inflammasome in inflammatory diseases. Nat. Rev. Drug Discov. 17:688. doi: 10.1038/nrd.2018.149
Matos, M. N., Cazorla, S. I., Schulze, K., Ebensen, T., Guzmán, C. A., and Malchiodi, E. L. (2017). Immunization with Tc52 or its amino terminal domain adjuvanted with c-di-AMP induces Th17+Th1 specific immune responses and confers protection against Trypanosoma cruzi. PLoS Negl. Trop. Dis. 11:e0005300. doi: 10.1371/journal.pntd.0005300
McFarland, A. P., Burke, T. P., Carletti, A. A., Glover, R. C., Tabakh, H., Welch, M. D., et al. (2018). RECON-dependent inflammation in hepatocytes enhances Listeria monocytogenes cell-to-cell spread. mBio 9:e00526-18. doi: 10.1128/mBio.00526-18
McFarland, A. P., Luo, S., Ahmed-Qadri, F., Zuck, M., Thayer, E. F., Goo, Y. A., et al. (2017). Sensing of bacterial cyclic dinucleotides by the oxidoreductase RECON promotes NF-κB activation and shapes a proinflammatory antibacterial state. Immunity 46, 433–445. doi: 10.1016/j.immuni.2017.02.014
Mehne, F. M., Gunka, K., Eilers, H., Herzberg, C., Kaever, V., and Stülke, J. (2013). Cyclic di-AMP homeostasis in bacillus subtilis: both lack and high level accumulation of the nucleotide are detrimental for cell growth. J. Biol. Chem. 288, 2004–2017. doi: 10.1074/jbc.M112.395491
Mettelman, R. C., Allen, E. K., and Thomas, P. G. (2022). Mucosal immune responses to infection and vaccination in the respiratory tract. Immunity 55, 749–780. doi: 10.1016/j.immuni.2022.04.013
Mistry, J., Chuguransky, S., Williams, L., Qureshi, M., Salazar, G. A., Sonnhammer, E., et al. (2021). Pfam: The protein families database in 2021. Nucleic. Acids. Res. 49, D412–D419. doi: 10.1093/nar/gkaa913
Moretti, J., and Blander, J. M. (2018). Detection of a vita-PAMP STINGs cells into reticulophagy. Autophagy 14, 1102–1104. doi: 10.1080/15548627.2018.1441471
Moretti, J., Roy, S., Bozec, D., Martinez, J., Chapman, J. R., Ueberheide, B., et al. (2017). STING senses microbial viability to orchestrate stress-mediated autophagy of the endoplasmic reticulum. Cell 171, 809.e13–823.e13. doi: 10.1016/j.cell.2017.09.034
Muñoz González, F., Sycz, G., Alonso Paiva, I. M., Linke, D., Zorreguieta, A., Baldi, P. C., et al. (2019). The BtaF adhesin is necessary for full virulence during respiratory infection by Brucella suis and is a novel immunogen for nasal vaccination against Brucella infection. Front. Immunol. 10:1775. doi: 10.3389/fimmu.2019.01775
Ning, H., Wang, L., Zhou, J., Lu, Y., Kang, J., Ding, T., et al. (2019). Recombinant BCG with bacterial signaling molecule cyclic di-AMP as endogenous adjuvant induces elevated immune responses after Mycobacterium tuberculosis infection. Front. Immunol. 10:1519. doi: 10.3389/fimmu.2019.01519
Ning, H., Zhang, W., Kang, J., Ding, T., Liang, X., and Lu, Y. (2021). Subunit vaccine ESAT-6:c-di-AMP delivered by intranasal route elicits immune responses and protects against Mycobacterium tuberculosis infection. Front. Cell. Infect. Microbiol. 11:647220. doi: 10.3389/fcimb.2021.647220
Onyedibe, K. I., Elmanfi, S., Aryal, U. K., Könönen, E., Gürsoy, U. K., and Sintim, H. O. (2021). Global proteomics of fibroblast cells treated with bacterial cyclic dinucleotides, c-di-GMP and c-di-AMP. J. Oral Microbiol. 14:2003617. doi: 10.1080/20002297.2021.2003617
Parvatiyar, K., Zhang, Z., Teles, R. M., Ouyang, S., Jiang, Y., Iyer, S. S., et al. (2012). The helicase DDX41 recognizes the bacterial secondary messengers cyclic di-GMP and cyclic di-AMP to activate a type I interferon immune response. Nat. Immunol. 13, 1155–1161. doi: 10.1038/ni.2460
Peng, X., Zhang, Y., Bai, G., Zhou, X., and Wu, H. (2016a). Cyclic di-AMP mediates biofilm formation. Mol. Microbiol. 99, 945–959. doi: 10.1111/mmi.13277
Peng, X., Michalek, S., and Wu, H. (2016b). Effects of diadenylate cyclase deficiency on synthesis of extracellular polysaccharide matrix of Streptococcus mutans revisit. Environ. Microbiol. 18, 3612–3619. doi: 10.1111/1462-2920.13440
Pozzi, C., Waters, E. M., Rudkin, J. K., Schaeffer, C. R., Lohan, A. J., Tong, P., et al. (2012). Methicillin resistance alters the biofilm phenotype and attenuates virulence in Staphylococcus aureus device-associated infections. PLoS Pathog. 8:e1002626. doi: 10.1371/journal.ppat.1002626
Quintana, I., Espariz, M., Villar, S. R., González, F. B., Pacini, M. F., Cabrera, G., et al. (2018). Genetic engineering of Lactococcus lactis co-producing antigen and the mucosal adjuvant 3’ 5’- cyclic di adenosine monophosphate (c-di-AMP) as a design strategy to develop a mucosal vaccine prototype. Front. Microbiol. 9:2100. doi: 10.3389/fmicb.2018.02100
Quintana, I. M., Gibhardt, J., Turdiev, A., Hammer, E., Commichau, F. M., Lee, V. T., et al. (2019). The KupA and KupB proteins of Lactococcus lactis IL1403 are novel c-di-AMP receptor proteins responsible for potassium uptake. J. Bacteriol. 201:e00028-19. doi: 10.1128/JB.00028-19
Randall, T. E., Eckartt, K., Kakumanu, S., Price-Whelan, A., Dietrich, L., and Harrison, J. J. (2022). Sensory perception in bacterial cyclic diguanylate signal transduction. J. Bacteriol. 204:e0043321. doi: 10.1128/JB.00433-21
Rao, F., See, R. Y., Zhang, D., Toh, D. C., Ji, Q., and Liang, Z. X. (2010). YybT is a signaling protein that contains a cyclic dinucleotide phosphodiesterase domain and a GGDEF domain with ATPase activity. J. Biol. Chem. 285, 473–482. doi: 10.1074/jbc.M109.040238
Rørvik, G. H., Liskiewicz, K. A., Kryuchkov, F., Naemi, A. O., Aasheim, H. C., Petersen, F. C., et al. (2020). Cyclic di-adenosine monophosphate regulates metabolism and growth in the oral commensal Streptococcus mitis. Microorganisms 8:1269. doi: 10.3390/microorganisms8091269
Rørvik, G. H., Naemi, A. O., Edvardsen, P., and Simm, R. (2021). The c-di-AMP signaling system influences stress tolerance and biofilm formation of Streptococcus mitis. MicrobiologyOpen 10:e1203. doi: 10.1002/mbo3.1203
Ross, P., Weinhouse, H., Aloni, Y., Michaeli, D., Weinberger-Ohana, P., Mayer, R., et al. (1987). Regulation of cellulose synthesis in Acetobacter xylinum by cyclic diguanylic acid. Nature 325, 279–281. doi: 10.1038/325279a0
Rueckert, C., Rand, U., Roy, U., Kasmapour, B., Strowig, T., and Guzmán, C. A. (2017). Cyclic dinucleotides modulate induced type I IFN responses in innate immune cells by degradation of STING. FASEB J. 31, 3107–3115. doi: 10.1096/fj.201601093R
Saito-Tarashima, N., Kinoshita, M., Igata, Y., Kashiwabara, Y., and Minakawa, N. (2021). Replacement of oxygen with sulfur on the furanose ring of cyclic dinucleotides enhances the immunostimulatory effect via STING activation. RSC Med. Chem. 12, 1519–1524. doi: 10.1039/d1md00114k
Schroder, K., and Tschopp, J. (2010). The inflammasomes. Cell 140, 821–832. doi: 10.1016/j.cell.2010.01.040
Schulze, K., Ebensen, T., Chandrudu, S., Skwarczynski, M., Toth, I., Olive, C., et al. (2017a). Bivalent mucosal peptide vaccines administered using the LCP carrier system stimulate protective immune responses against Streptococcus pyogenes infection. Nanomedicine 13, 2463–2474. doi: 10.1016/j.nano.2017.08.015
Schulze, K., Ebensen, T., Babiuk, L. A., Gerdts, V., and Guzman, C. A. (2017b). Intranasal vaccination with an adjuvanted polyphosphazenes nanoparticle-based vaccine formulation stimulates protective immune responses in mice. Nanomedicine 13, 2169–2178. doi: 10.1016/j.nano.2017.05.012
Schuster, C. F., Bellows, L. E., Tosi, T., Campeotto, I., Corrigan, R. M., Freemont, P., et al. (2016). The second messenger c-di-AMP inhibits the osmolyte uptake system OpuC in Staphylococcus aureus. Sci. Signal. 9:ra81. doi: 10.1126/scisignal.aaf7279
Seoane, P. I., Lee, B., Hoyle, C., Yu, S., Lopez-Castejon, G., Lowe, M., et al. (2020). The NLRP3-inflammasome as a sensor of organelle dysfunction. J. Cell. Biol. 219:e202006194. doi: 10.1083/jcb.202006194
Shakya, A. K., Lee, C. H., Uddin, M. J., and Gill, H. S. (2018). Assessment of Th1/Th2 bias of STING agonists coated on microneedles for possible use in skin allergenimmunotherapy. Mol. Pharm. 15, 5437–5443. doi: 10.1021/acs.molpharmaceut.8b00768
Shen, X., Tang, C., Wei, C., Zhu, Y., and Xu, R. (2022). Melatonin induces autophagy in amyotrophic lateral sclerosis mice via upregulation of SIRT1. Mol. Neurobiol. 59, 4747–4760. doi: 10.1007/s12035-022-02875-7
Shi, J., Zhao, Y., Wang, K., Shi, X., Wang, Y., Huang, H., et al. (2015). Cleavage of GSDMD by inflammatory caspases determines pyroptotic cell death. Nature 526, 660–665. doi: 10.1038/nature15514
Singh, A. K., Praharaj, M., Lombardo, K. A., Yoshida, T., Matoso, A., Baras, A. S., et al. (2022). Re-engineered BCG overexpressing cyclic di-AMP augments trained immunity and exhibits improved efficacy against bladder cancer. Nat. Commun. 13:878. doi: 10.1038/s41467-022-28509-z
Sintim, H. O., Mikek, C. G., Wang, M., and Sooreshjani, M. A. (2019). Interrupting cyclic dinucleotide-cGAS-STING axis with small molecules. Med. Chem. Commun. 10, 1999–2023. doi: 10.1039/c8md00555a
Smith, W. M., Pham, T. H., Lei, L., Dou, J., Soomro, A. H., Beatson, S. A., et al. (2012). Heat resistance and salt hypersensitivity in Lactococcus lactis due to spontaneous mutation of llmg_1816 (gdpP) induced by high-temperature growth. Appl. Environ. Microbiol. 78, 7753–7759. doi: 10.1128/AEM.02316-12
Sureka, K., Choi, P. H., Precit, M., Delince, M., Pensinger, D. A., Huynh, T. N., et al. (2014). The cyclic dinucleotide c-di-AMP is an allosteric regulator of metabolic enzyme function. Cell 158, 1389–1401. doi: 10.1016/j.cell.2014.07.046
Tang, Q., Luo, Y., Zheng, C., Yin, K., Ali, M. K., Li, X., et al. (2015). Functional analysis of a c-di-AMP-specific phosphodiesterase MsPDE from Mycobacterium smegmatis. Int. J. Biol. Sci. 11, 813–824. doi: 10.7150/ijbs.11797
Tang, Q., Precit, M. R., Thomason, M. K., Blanc, S. F., Ahmed-Qadri, F., McFarland, A. P., et al. (2022). Thymidine starvation promotes c-di-AMP-dependent inflammation during pathogenic bacterial infection. Cell Host Microbe 30, 961.e6–974.e6. doi: 10.1016/j.chom.2022.03.028
Temizoz, B., Hioki, K., Kobari, S., Jounai, N., Kusakabe, T., Lee, M., et al. (2022). Anti-tumor immunity by transcriptional synergy between TLR9 and STING activation. Int. Immunol. 34, 353–364. doi: 10.1093/intimm/dxac012
Townsley, L., Yannarell, S. M., Huynh, T. N., Woodward, J. J., and Shank, E. A. (2018). Cyclic di-AMP acts as an extracellular signal that impacts Bacillus subtilis biofilm formation and plant attachment. mBio 9:e00341-18. doi: 10.1128/mBio.00341-18
Vasiyani, H., Shinde, A., Roy, M., Mane, M., Singh, K., Singh, J., et al. (2021). The analog of cGAMP, c-di-AMP, activates STING mediated cell death pathway in estrogen-receptor negative breast cancer cells. Apoptosis 26, 293–306. doi: 10.1007/s10495-021-01669-x
Volckmar, J., Knop, L., Stegemann-Koniszewski, S., Schulze, K., Ebensen, T., Guzmán, C. A., et al. (2019). The STING activator c-di-AMP exerts superior adjuvant properties than the formulation poly(I:C)/CpG after subcutaneous vaccination with soluble protein antigen or DEC-205-mediated antigen targeting to dendritic cells. Vaccine 37, 4963–4974. doi: 10.1016/j.vaccine.2019.07.019
Wang, X., Davlieva, M., Reyes, J., Panesso, D., Arias, C. A., and Shamoo, Y. (2017). A novel phosphodiesterase of the GdpP family modulates cyclic di-AMP levels in response to cell membrane stress in daptomycin-resistant Enterococci. Antimicrob. Agents Chemother. 61:e01422-16. doi: 10.1128/AAC.01422-16
Wendel, B. M., Pi, H., Krüger, L., Herzberg, C., Stülke, J., and Helmann, J. D. (2022). A central role for magnesium homeostasis during adaptation to osmotic stress. mBio 13:e0009222. doi: 10.1128/mbio.00092-22
Witte, C. E., Whiteley, A. T., Burke, T. P., Sauer, J. D., Portnoy, D. A., and Woodward, J. J. (2013). Cyclic di-AMP is critical for Listeria monocytogenes growth, cell wall homeostasis, and establishment of infection. mBio 4:e00282-13. doi: 10.1128/mBio.00282-13
Witte, G., Hartung, S., Büttner, K., and Hopfner, K. P. (2008). Structural biochemistry of a bacterial checkpoint protein reveals diadenylate cyclase activity regulated by DNA recombination intermediates. Mol. Cell. 30, 167–178. doi: 10.1016/j.molcel.2008.02.020
Woodward, J. J., Iavarone, A. T., and Portnoy, D. A. (2010). c-di-AMP secreted by intracellular Listeria monocytogenes activates a host type I interferon response. Science 328, 1703–1705. doi: 10.1126/science.1189801
Wooten, A. K., Shenoy, A. T., Arafa, E. I., Akiyama, H., Martin, I., Jones, M. R., et al. (2020). Unique roles for Streptococcus pneumoniae phosphodiesterase 2 in cyclic di-AMP catabolism and macrophage responses. Front. Immunol. 11:554. doi: 10.3389/fimmu.2020.00554
Xia, P., Wang, S., Xiong, Z., Zhu, X., Ye, B., Du, Y., et al. (2018). The ER membrane adaptor ERAdP senses the bacterial second messenger c-di-AMP and initiates anti-bacterial immunity. Nat. Immunol. 19, 141–150. doi: 10.1038/s41590-017-0014-x
Yamamoto, T., Hara, H., Tsuchiya, K., Sakai, S., Fang, R., Matsuura, M., et al. (2012). Listeria monocytogenes strain-specific impairment of the TetR regulator underlies the drastic increase in cyclic di-AMP secretion and beta interferon-inducing ability. Infect. Immun. 80, 2323–2332. doi: 10.1128/IAI.06162-11
Yan, H., KuoLee, R., Tram, K., Qiu, H., Zhang, J., Patel, G. B., et al. (2009). 3’,5’-cyclic diguanylic acid elicits mucosal immunity against bacterial infection. Biochem. Biophys. Res. Commun. 387, 581–584. doi: 10.1016/j.bbrc.2009.07.061
Yang, J., Bai, Y., Zhang, Y., Gabrielle, V. D., Jin, L., and Bai, G. (2014). Deletion of the cyclic di-AMP phosphodiesterase gene (cnpB) in Mycobacterium tuberculosis leads to reduced virulence in a mouse model of infection. Mol. Microbiol. 93, 65–79. doi: 10.1111/mmi.12641
Ye, M., Zhang, J. J., Fang, X., Lawlis, G. B., Troxell, B., Zhou, Y., et al. (2014). DhhP, a cyclic di-AMP phosphodiesterase of Borrelia burgdorferi, is essential for cell growth and virulence. Infect. Immun. 82, 1840–1849. doi: 10.1128/IAI.00030-14
Yoshimori, T. (2004). Autophagy: a regulated bulk degradation process inside cells. Biochem. Biophys. Res. Commun. 313, 453–458. doi: 10.1016/j.bbrc.2003.07.023
Zarrella, T. M., Metzger, D. W., and Bai, G. (2018). Stress suppressor screening leads to detection of regulation of cyclic di-AMP homeostasis by a Trk family effector protein in Streptococcus pneumoniae. J. Bacteriol. 200:e00045-18. doi: 10.1128/JB.00045-18
Zarrella, T. M., Yang, J., Metzger, D. W., and Bai, G. (2020). Bacterial second messenger cyclic di-AMP modulates the competence state in Streptococcus pneumoniae. J. Bacteriol. 202:e00691-19. doi: 10.1128/JB.00691-19
Zhang, L., Li, W., and He, Z. G. (2013). DarR, a TetR-like transcriptional factor, is a cyclic di-AMP-responsive repressor in Mycobacterium smegmatis. J. Biol. Chem. 288, 3085–3096. doi: 10.1074/jbc.M112.428110
Zhu, Y., Pham, T. H., Nhiep, T. H., Vu, N. M., Marcellin, E., Chakrabortti, A., et al. (2016). Cyclic-di-AMP synthesis by the diadenylate cyclase CdaA is modulated by the peptidoglycan biosynthesis enzyme GlmM in Lactococcus lactis. Mol. Microbiol. 99, 1015–1027. doi: 10.1111/mmi.13281
Keywords: c-di-AMP, innate immune response, STING agonist, NF-κB pathway, inflammasome, autophagy, mucosal adjuvant, immunomodulation
Citation: Cheng X, Ning J, Xu X and Zhou X (2022) The role of bacterial cyclic di-adenosine monophosphate in the host immune response. Front. Microbiol. 13:958133. doi: 10.3389/fmicb.2022.958133
Received: 31 May 2022; Accepted: 04 August 2022;
Published: 29 August 2022.
Edited by:
Chengrong Xie, Xiamen University, ChinaReviewed by:
Eric Lee Bruger, University of Idaho, United StatesSiegfried Weiss, Helmholtz Association of German Research Centers (HZ), Germany
Jiyao Li, Sichuan University, China
Copyright © 2022 Cheng, Ning, Xu and Zhou. This is an open-access article distributed under the terms of the Creative Commons Attribution License (CC BY). The use, distribution or reproduction in other forums is permitted, provided the original author(s) and the copyright owner(s) are credited and that the original publication in this journal is cited, in accordance with accepted academic practice. No use, distribution or reproduction is permitted which does not comply with these terms.
*Correspondence: Xuedong Zhou, emhvdXhkQHNjdS5lZHUuY24=