- 1School of Medicine, Ningbo University, Ningbo, China
- 2Key Laboratory of Marine Materials and Related Technologies, Zhejiang Key Laboratory of Marine Materials and Protective Technologies, Ningbo Institute of Materials Technology and Engineering, Chinese Academy of Sciences, Ningbo, China
- 3Ningbo Key Laboratory of Hearing and Balance Medicine, The Affiliated Hospital of Medical School of Ningbo University, Ningbo, China
Antimicrobial photodynamic inactivation (aPDI) is a newly emerged treatment approach that can effectively address the issue of multidrug resistance resulting from the overuse of antibiotics. Fullerenes can be used as promising photosensitizers (PSs) for aPDI due to the advantages of high triplet state yields, good photostability, wide antibacterial spectrum, and permissibility of versatile functionalization. This review introduces the photodynamic activities of fullerenes and the up-to-date understanding of the antibacterial mechanisms of fullerene-based aPDI. The most recent works on the functionalization of fullerenes and the application of fullerene derivatives as PSs for aPDI are also summarized. Finally, certain remaining challenges are emphasized to provide guidance on future research directions for achieving clinical application of fullerene-based aPDI.
Introduction
In the past few decades, the overuse of antibiotics has brought about the emergence of super bacteria with multidrug resistance, posing tremendous challenges to the treatment of bacterial infections (Prestinaci et al., 2015; Willyard, 2017; Rawson et al., 2021; Varela et al., 2021). Antimicrobial photodynamic inactivation (aPDI) is a newly emerged treatment approach that can effectively address the issues of multidrug resistance (Hu et al., 2018; Blum et al., 2020; Wang et al., 2021). Up till now, no resistance against aPDI has been reported in bacteria even after 20 successive bactericidal cycles (Giuliani et al., 2010; Hamblin, 2016, 2018; Al-Mutairi et al., 2018; Pieranski et al., 2020; Wozniak et al., 2021). aPDI starts with the injection or coating of photosensitizers (PSs) to the infected area. After the accumulation of PSs in the targeted area, irradiation with the light of specific wavelengths is applied to trigger photodynamic reactions. Under irradiation, PSs in the ground state adsorb photons and are excited into a transient singlet state, from which they enter a relatively stable triplet state by fluorescence, heat conversion, or undergoing an intersystem crossing (ISC) process (Ochsner, 1997). The two mechanisms of aPDI are as follows: (1) Type-I mechanism: PS in the triplet state produces reactive oxygen species (ROS), such as hydroxyl radicals (HO⋅), hydrogen peroxide, and superoxide anion radicals (O2⋅–) through gain or loss of electrons; and (2) Type-II mechanism: PS in the triplet state interacts with oxygen and produces singlet oxygen (1O2). ROS and 1O2 are strong oxidants that can cause disruption of the cell wall, alteration of the cell membrane structure, damage of DNA and RNA molecules, inhibition of essential enzymatic activities, denaturation of proteins, peroxidation of unsaturated lipids, leakage of intracellular substances, and disruption of the transmembrane transport system, leading to irreversible damage to the bacterial cells (Michaeli and Feitelson, 1994; Cadet et al., 2010; Allison and Moghissi, 2013; Vatansever et al., 2013; Gao et al., 2021; Rapacka-Zdonczyk et al., 2021). The Type-I mechanism does not rely on oxygen and is not limited by oxygen deprivation, while the Type-II mechanism is limited by the lack of oxygen in certain infected areas. To improve the effectiveness of aPDI treatment, the ideal PSs are required to produce high yields of ROS or 1O2 that also possess supreme bacteria-targeting abilities to minimize damage to the normal tissue.
Owing to the fact that PSs can be directly injected or coated to the infected site, aPDI will not cause damage to the entire body like antibiotics do and thus can be repetitively applied to achieve higher bactericidal efficiency while minimizing the side effects inflicted on patients (Hamblin, 2016). These advantages of aPDI have been particularly demonstrated in the treatment of dental infections, such as peri-implantitis and periodontitis (Oruba and Chomyszyn-Gajewska, 2016; Diogo et al., 2019; Tan et al., 2020, 2021; Betancourt et al., 2021; Luchian et al., 2021), and infections due to burns or damaged tissues with insufficient blood supply that are hardly available for antibiotics (Gad et al., 2004; Choudhary et al., 2009; Sperandio et al., 2010; Simonetti et al., 2021).
Among the commonly used PSs (e.g., porphyrin and its derivatives, phthalocyanine, xanthene dyes, etc.), fullerenes are promising candidates due to their high triplet state yields, good photostability, high photoactivity, and supreme ROS and 1O2 production abilities (Table 1). Fullerenes are a kind of hollow molecules composed entirely of carbon atoms with a spherical, ellipsoidal, cylindrical, or tubular shape. The condensed aromatic rings of fullerenes cause extended π-conjugation of molecular orbits (Rahmati and Mozafari, 2019; Mohammed et al., 2020). The large amounts of conjugated double bonds can effectively adsorb light in the ultraviolet (UV) and visible regions, generating ROS with high triplet yields under irradiation (Hamblin, 2018). This review introduces the photodynamic activities of fullerenes and the up-to-date understanding of the antibacterial mechanisms of fullerene-based aPDI. The most recent works on the functionalization of fullerenes and the application of fullerene derivatives as PSs for aPDI are also summarized.
Advantages of Fullerene Derivatives as Photosensitizers for Antimicrobial Photodynamic Inactivation
Fullerenes are a kind of PSs that can produce high yields of 1O2 and ROS, including O2⋅–, HO⋅, lipid hydroperoxides, and hydrogen peroxides through both Type-I and Type-II mechanisms (Yamakoshi et al., 2003; Vileno et al., 2006). Under UV or visible light irradiation, fullerenes can be excited into the transient singlet state (process A in Figure 1) and then immediately transform into the triplet state by ISC (process B in Figure 1; Guldi and Prato, 2000; Koeppe and Sariciftci, 2006). Compared with other photosensitizers, fullerenes have a higher triplet yield of nearly 100% with a longer lifetime of 50∼100 μs (Sharma et al., 2011; Huang Y.-Y. et al., 2014). Subsequent charge transfers to the surrounding biomolecules lead to the formation of ROS (Type-I mechanism as illustrated by process C in Figure 1). The remaining fullerene radicals can further transfer an electron to oxygen to form O2⋅– (process D in Figure 1). Fullerenes in the triplet state can also transfer energy to oxygen, forming 1O2 (Type-II mechanism, as illustrated by process E in Figure 1).
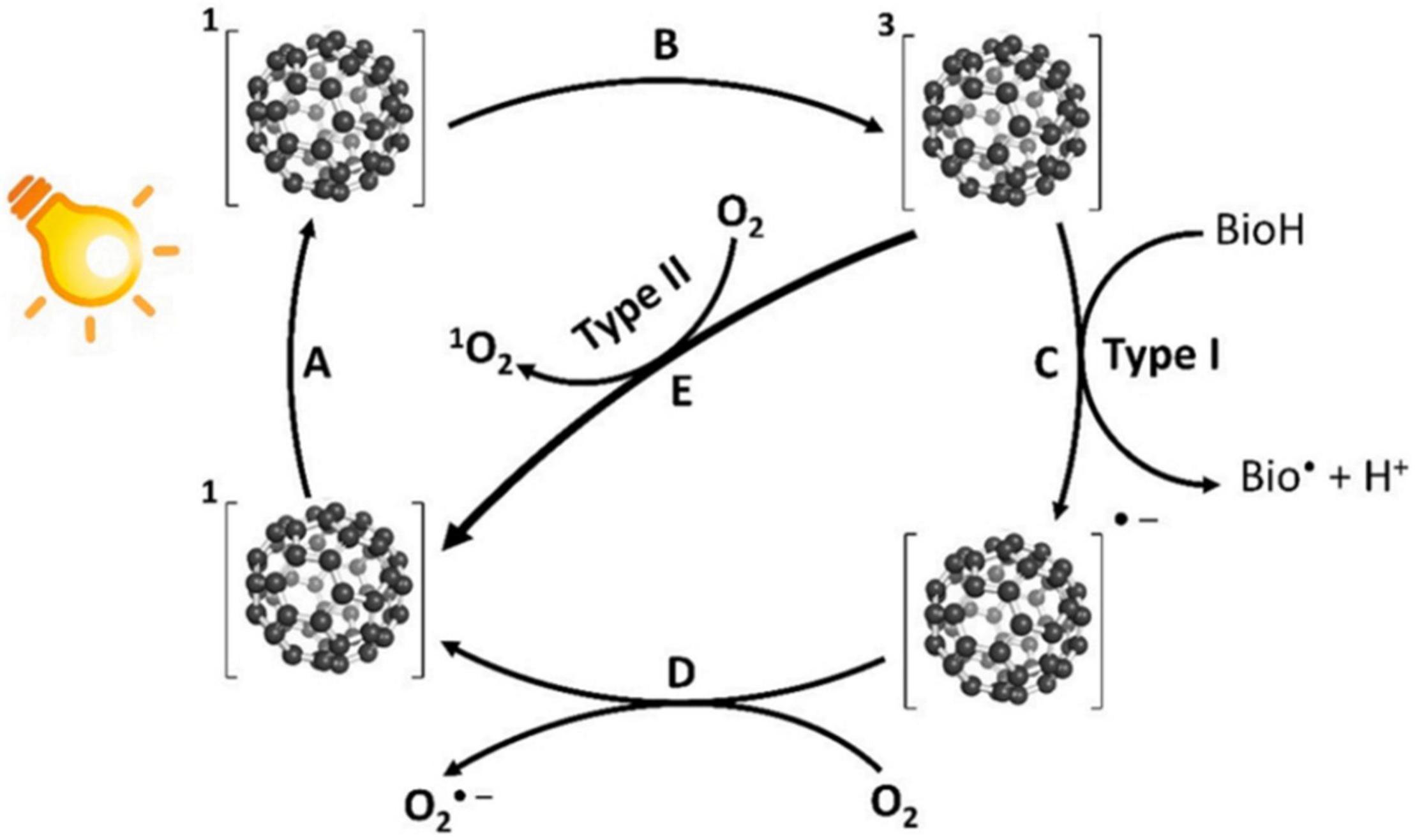
Figure 1. Schematic representation of the photodynamic activities of fullerenes (Heredia et al., 2022). A: Photoexcitation of fullerenes to the singlet state; B: Transition from the singlet state to the triplet state by ISC; C: Charge transfer to surrounding biomolecules to form ROS (Type-I mechanism); D: Fullerenes can further transfer an electron to O2 to form O2⋅–; and E: Energy transfer from the excited triplet state to O2 to form 1O2 (Type-II mechanism).
Generally speaking, aPDI is more effective against Gram-positive bacteria than against Gram-negative bacteria. The cell wall of Gram-positive bacteria is a thick but porous peptidoglycan layer, while that of Gram-negative bacteria is composed of a relatively dense outer phospholipid bilayer and a thin peptidoglycan inner layer. Owing to the differences in membrane permeability and polar head size of phospholipids, which is smaller for Gram-positive bacteria (Indeglia et al., 2018), the cell wall and cell membrane of Gram-positive bacteria are easier to be penetrated by 1O2 and ROS while Gram-negative bacteria are difficult to be killed (Bertoloni et al., 1992; Kashef and Hamblin, 2017). Compared with other PSs, fullerene can produce HO⋅ free radicals with stronger toxicity that are also capable of killing Gram-negative bacteria (Huang et al., 2012). Huang L. et al. (2014) reported that fullerene-mediated PDT could treat wound infections caused by virulent Gram-negative bacterial species.
Moreover, when used in conjunction with antibiotics, fullerene-based aPDI can increase the susceptibility of multidrug-resistant bacteria to antibiotics. Wozniak et al. (2021) reported that the synergistic effect of fullerene-based aPDI and imipenem reduced the resistance of Enterococcus faecalis against imipenem. The use of antibiotics, including gentamycin, streptomycin, tigecycline, doxycycline, and daptomycin, accompanied by fullerene-based aPDI exhibited a more pronounced inhibition of multidrug-resistant Enterococcus faecium than when these antibiotics were used alone. The resensitization of multidrug-resistant bacteria to antibiotics could be attributed to the aPDI-induced increase in membrane permeability (Wozniak et al., 2021).
Other advantages of fullerenes as a PS are as follows: (1) High resistance to photobleaching. Many traditional PSs completely lose their photoactivity after relatively low total energy doses (<10 J/cm2), while fullerenes can retain photoactivity even after very high energy doses (>1,000 J/cm2) (Hamblin, 2018). (2) Abundance of double bonds on the surface that allows versatile functionalization. (3) Oxygen-independent photokilling through Type-I mechanism. These combined advantages make fullerenes supreme PSs with promising prospects of application in aPDI.
Antibacterial Mechanisms of Fullerene-Based Antimicrobial Photodynamic Inactivation
To deepen the understanding of fullerene-based aPDI, researchers have strived to explore the underlying photodynamic antibacterial mechanisms of fullerenes. Up till now, three major mechanisms have been proposed: disruption of the cell membrane, denaturation of proteins, and induction of DNA damage (Figure 2).
Fullerene-based aPDI can lead to the peroxidation of lipids, causing bacterial cell membrane disruption, cytoplasm leakage, and eventually cell death (Sayes et al., 2004). Fang et al. (2007) found that the photodynamic reactions of fullerene changed the composition of the bacterial cell membrane in a strain-dependent manner. For Gram-negative bacterium Pseudomonas putida, aPDI decreased the proportions of unsaturated fatty acids while increasing that of cyclopropane fatty acids. For Gram-positive Bacillus subtilis, aPDI increased the proportions of monounsaturated fatty acids and membrane fluidity. Grinholc et al. (2015) also reported that photodynamic reactions of fullerene compromised the structural integrity of the cell membrane of Staphylococcus aureus, indicating that the bacterial cell membrane is a major target for fullerene-based aPDI. In addition, the inherent hydrophobic structure of fullerenes can cause mechanical disruption of the cell membrane. Even at low concentrations, fullerenes can adhere to and insert into the cell membrane, leading to the change in membrane permeability and leakage of intracellular substances, eventually causing bacterial death (Zhang et al., 2021). Furthermore, fullerenes can cause protein denaturation under irradiation (Radic et al., 2014). Nakagawa et al. (2014) reported that fullerenols induced necrocytosis and apoptosis of bacteria by causing protein denaturation through oxidation of cysteine.
Fullerenes have also been reported to bind DNA molecules after entering microbial cells and change the structures and biochemical functions of DNA, inducing oxidative stress and cellular respiration interruption (Lyon and Alvarez, 2008; Nishizawa et al., 2009; An and Jin, 2011). Moreover, fullerenes are prone to adhere to DNA chains due to their high affinity to guanine, causing damage to DNA and eventually bacterial death (Zhao et al., 2005). An and Jin (2015) found that fullerenes bound to nucleic acids and disrupted cell reproduction and regeneration, leading to apoptotic responses of bacteria. Additionally, the 1O2 and O2⋅– generated by fullerene can cause photocleavage of DNA chains (Ikeda et al., 2007). Iwamoto and Yamakoshi (2006) reported DNA cleavage induced by C60–N-vinylpyrrolidone copolymer. It has also been reported that the 1O2 produced by fullerene induced DNA mutation in Salmonella strains under visible light irradiation (Sera et al., 1996).
Application of Fullerene Derivatives in Antimicrobial Photodynamic Inactivation
Despite the many advantages of fullerene as a PS, there exist certain drawbacks, limiting the application of fullerene in aPDI. First of all, the inherent hydrophobicity and poor water solubility of fullerene make the injection of fullerene into the target area virtually impossible. Fullerene nanoparticles are also prone to agglomerate in a liquid environment, adversely affecting their photoactivity (Mizuno et al., 2011). Second, the ROS and 1O2 generated by the photodynamic reactions of fullerene may also inflict damage on normal tissue. Lack of ability to target bacterial cells leads to increased cytotoxicity, limiting the clinical use of fullerene-based aPDI. Furthermore, the adsorption range of fullerene is limited to the green, blue, or UV region (Heredia et al., 2022). The applicability of fullerene-based aPDI in deep areas of the body is limited by the poor penetration depth of light of short wavelengths. Nevertheless, the abundant double bonds of fullerene allow surface grafting of various functional groups for increased water solubility, improved targeting ability, and broadened adsorption range. In recent years, research attention is being increasingly focused on the synthesis and development of functionalized fullerene derivatives for application in aPDI (Zhang et al., 2019; Pan et al., 2020; Pochkaeva et al., 2020; Heredia et al., 2022).
Cationic Fullerene Derivatives
The ideal PSs for aPDI shall selectively attack microbes without damaging mammalian cells. It was found that bacterial cell surfaces are more negatively charged compared to mammalian cell surfaces. Therefore, grafting cationic functional groups on the surface of fullerene not only improves water solubility, but also facilitates targeted attacks on bacteria by rapidly binding to the anionic residues on the bacterial cell wall (Merchat et al., 1996; Mashino et al., 2003; Hamblin, 2016). Additionally, the cellular uptake of cationic fullerene derivatives by bacteria is significantly greater than that by mammalian cells (Soncin et al., 2002), making cationic fullerene derivatives perfect PSs for aPDI. Based on the type of cationic functional groups, cationic fullerene derivatives can be classified into fullerene-pyrrolidine derivatives and fullerene-cyclopropane derivatives.
Fullerene-Pyrrolidine Derivatives
Pyrrolidination of fullerene is one of the main approaches to prepare cationic fullerene derivatives. (Huang Y.-Y. et al., 2014) grafted electroneutral dienol groups and positively charged pyrrolidinium groups on fullerene and compared the antibacterial effectiveness and spectrum of the two derivatives. It was found that the neutral dienol-functionalized fullerene exhibited only modest antibacterial activity against Gram-positive Staphylococcus aureus, while the cationic pyrrolidinium-functionalized fullerene effectively induced light-mediated killing of Gram-positive S. aureus, Gram-negative Escherichia coli and Pseudomonas aeruginosa, and fungal yeast Candida albicans by inhibiting oxygen uptake. Spesia et al. (2008) investigated the relationship between the number of cationic charges of fullerene-pyrrolidine derivatives and aPDI effectiveness against E. coli suspensions. After 30 min of irradiation, the dicationic fullerene derivative DTC602+ (Figure 3A) and the monocationic fullerene derivative DTC60+ (Figure 3B) decreased the viability of E. coli by 99.97 and 96.8%, respectively, while the non-charged fullerene derivative MAC60 showed negligible effect on E. coli. These results indicate that the effectiveness of aPDI is positively correlated to the number of charges on cationic fullerene derivatives.
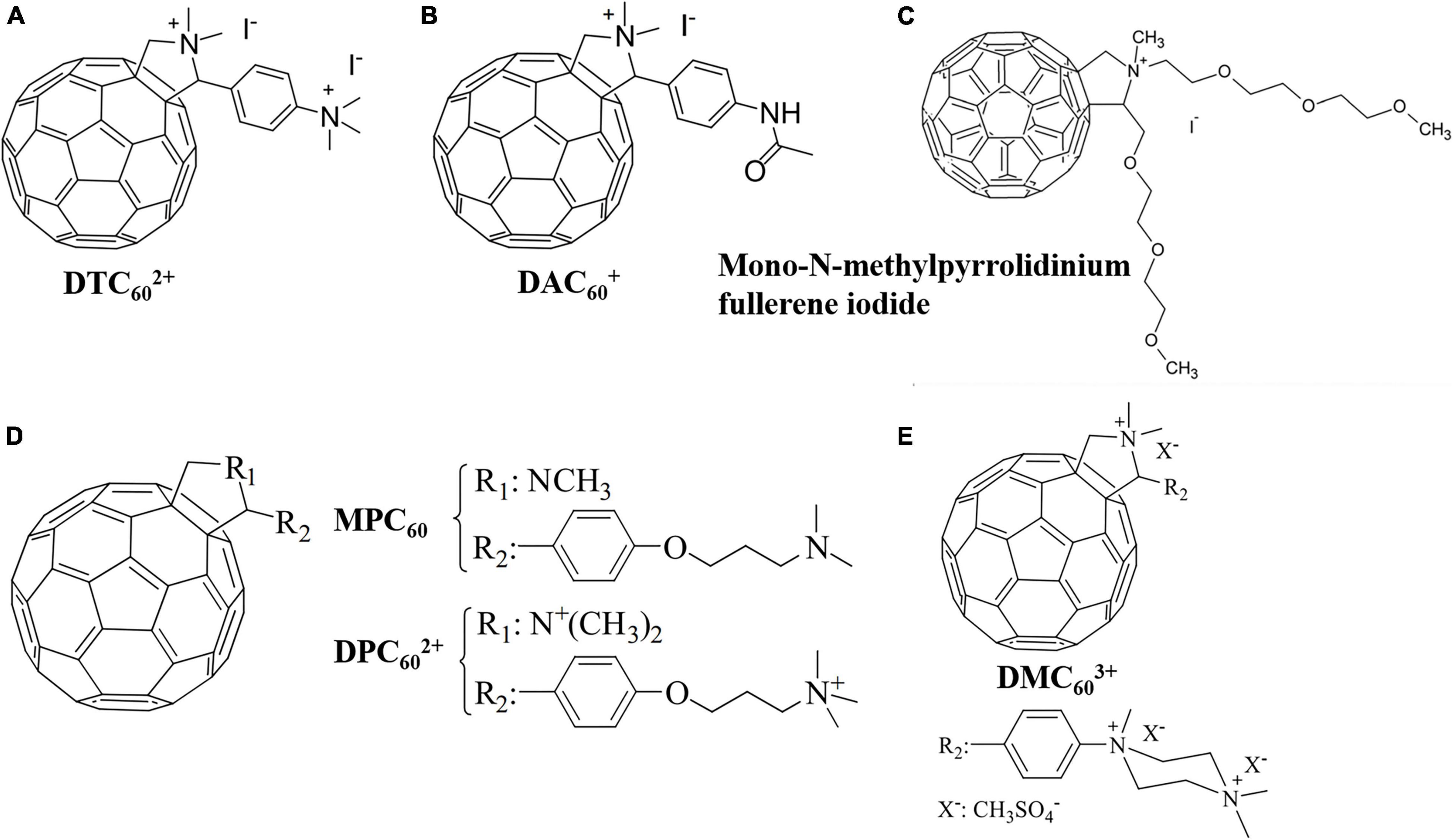
Figure 3. Molecular structures of the fullerene-pyrrolidine derivatives. (A) DTC602+. (B) DAC60+ (Spesia et al., 2008). (C) Mono-N-methylpyrrolidinium fullerene iodide (Grinholc et al., 2015). (D) MPC60 and DPC602+ (Agazzi et al., 2015). (E) DMC603+ (Agazzi et al., 2021).
Grinholc et al. (2015) prepared a mono-N-methylpyrrolidinium fullerene iodide (Figure 3C) with supreme bactericidal effect on E. coli, P. aeruginosa, S. aureus, and C. albicans under white light irradiation. The bacterial inactivation observed was attributed to cell membrane disruption induced by 1O2 and O2⋅– instead of DNA cleavage. In vivo studies showed that the as-prepared fullerene derivative was effective in treating mice infected with S. aureus (Grinholc et al., 2015). Agazzi et al. (2015) synthesized a novel fulleropyrrolidine C60 derivative (MPC60) containing an aliphatic chain with an amino group at the end. By exhaustive methylation of the amino group, a dicationic fulleropyrrolidinium (DPC602+) was further synthesized (Figure 3D). Although MPC60 generated higher yields of 1O2 than DPC602+, higher photoinactivation of S. aureus was reported for the dicationic DPC602+. After incubation with 0.5 μM fullerene and irradiation for 30 min, MPC60 and DPC602+ decreased the cell viability by 4.4 and 5.0 log, respectively. Oxygen was found to be essential for effective aPDI by DPC602+, and 1O2 was detected in S. aureus cells. After the addition of NADH (which is able to quench 1O2) into the bacterial suspension, a weakened aPDI performance was observed, indicating that DPC602+ killed S. aureus mainly through Type-II mechanism (Gsponer et al., 2016). In 2021, the same research group prepared an amphiphilic tricationic fullerene derivative DMC603+ (Figure 3E) with strong UV adsorption that effectively inhibited the growth of E. coli and S. aureus through the synergistic effect of Type-I and Type-II mechanisms (Agazzi et al., 2021).
Apart from the number of cationic charges, the position of charges may also affect the aPDI effectiveness of fullerene-pyrrolidine derivatives. Mizuno et al. (2011) found that distributing cationic charges around the fullerene cage can minimize the tendency of fullerene to aggregate and enhance their aPDI effectiveness. Palacios et al. (2021) prepared four fullerene-pyrrolidine derivatives and found that compounds with the highest dipole moments exhibited a stronger inactivation effect against methicillin-resistant E. coli and S. aureus.
Fullerene-Cyclopropane Derivatives
Cyclopropanation is another common approach to prepare cationic fullerene derivatives. Fullerene-cyclopropane derivatives can effectively target Gram-negative bacteria due to their rich abundance of quaternary ammonium cations. When irradiated with UV (wavelength 320∼420 nm) or white light, fullerene-cyclopropane derivatives can generate both 1O2 and ROS (predominantly HO⋅) with bactericidal potencies against multiple drug-resistant bacterial species. Yin et al. (2015) synthesized three fullerene-cyclopropane derivatives as PSs for aPDI, C60[>M(C3N6+C3)2]-(I–)10 (LC14), C60[>CPAF-(MN6+C3)2]-(I–)10 (LC15), and C60[>M(C3N6+C3)2][>M(C3N6C3)2]-(I–)10 (LC16), and their molecular structures are shown in Figure 4. Zhang et al. (2015) investigated the ROS-generating ability of these three fullerene derivatives and their inhibition effectiveness against methicillin-resistant S. aureus, E. coli, and C. albicans. LC15 showed the best broad-spectrum antibacterial properties, followed by LC16 and LC14 in sequence. Owing to the lack of electron-donating groups, LC14 could only produce 1O2 via a Type-II mechanism that is only effective against Gram-positive bacteria. Nevertheless, 1O2 cannot effectively kill Gram-negative bacteria due to the difficulty in penetrating their cell wall. LC15, which produces HO⋅, was more effective against Gram-negative bacteria. Wang et al. (2013) prepared a water-soluble decacationic fullerene derivative C60[>M(C3N6+C3)2]-(I–)10 capable of producing both 1O2 and HO⋅ under UV and white light irradiation. The 10 quaternary ammonium cations per C60 provided the compound with excellent bactericidal ability against pathogenic Gram-positive and Gram-negative bacteria. Wang et al. (2012) compared the bactericidal effect of decacationic C60 and C70 derivatives C60[>M(C3N6+C3)2] and C70[>M(C3N6+C3)2] (LC17). Compared with C60[>M(C3N6+C3)2], C70[>M(C3N6+C3)2] was more effective against Gram-negative bacteria due to its ability to produce HO⋅ via the Type-I mechanism. Based on LC17, Sperandio et al. (2013) added a deca-tertiary ethyleneamino-chain as an electron source and prepared C70[>M(C3N6+C3)2][M(C3N6C3)2] (LC18). Huang L. et al. (2014) reported excellent effectiveness of LC18 in treating mice with third-degree burns infected by Gram-negative bacteria. The 10 tertiary amine groups of LC18 (with each tertiary amine group providing one electron for the C70 cage) enable the production of HO⋅ under UV light irradiation. Sperandio et al. (2013) further replaced the C70 of LC17 and LC18 with C84O2 and prepared C84O2[>M(C3N6+C3)2] (LC19) and C84O2[>M(C3N6+C3)2][M(C3N6C3)2] (LC20). LC19 was found to exhibit stronger phototoxicity when irradiated with light of longer wavelengths (615∼645 nm), while LC20 exhibited stronger phototoxicity at short wavelengths (350–420 nm).
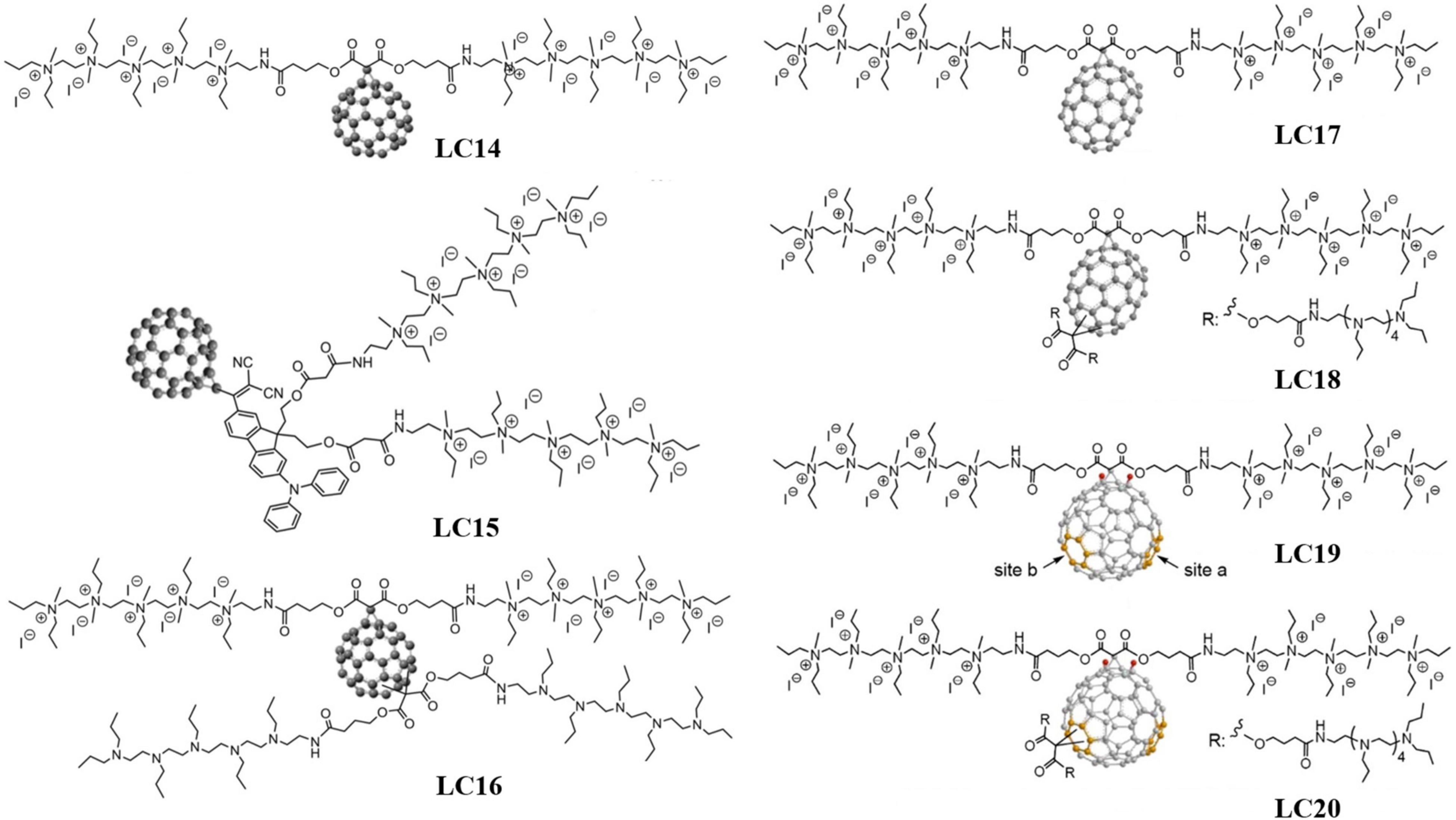
Figure 4. Molecular structures of the fullerene-cyclopropane derivatives, LC14–LC20 (Sperandio et al., 2013; Yin et al., 2015).
Fullerenols
Fullerenols are a family of polyhydroxylated fullerene that can be used as PSs for aPDI and are prepared by chemical functionalization of fullerene using hydrogen peroxide. Pickering and Wiesner (2005) first proved the ability of fullerenols to simultaneously produce 1O2 and O2⋅– under UV or polychromatic light irradiation. Fullerenols were found to exhibit 2∼3 times greater light adsorption in the wavelength range of 300∼400 nm than in the visible range, accompanied by an order of magnitude greater ROS generation rate. Compared with C60 nanoparticles, fullerenols possess stronger abilities to bind bacterial DNA and induce DNA mutations (An and Jin, 2011). Aoshima et al. (2009) reported that fullerenols C60(OH)12, C60(OH)36.8H2O, and C60(OH)44.8H2O significantly inhibited the growth of C. albicans, Malassezia furfur, and Propionibacterium acnes. Furthermore, C60(OH)44.8H2O was found to inhibit the growth of E. coli, S. aureus, Staphylococcus epidermidis, and Bacillus sp. Brunet et al. (2009) found that the photodynamic activities of fullerenols were dependent on the solution in which they were suspended. When suspended in ultrapure water, fullerenols generated abundant 1O2, while when suspended in minimal Davis microbial growth medium, fullerenols generated both 1O2 and O2⋅–. However, when suspended in tetrahydrofuran, fullerenols exhibited no photoactivity. Kuo et al. (2020) reported massive production of ROS and complete elimination of methicillin-resistant S. aureus by water-soluble fullerenol C60(OH)30 under two-photon excitation in the near-infrared region (760 nm).
Fullerenols can also be used in conjunction with other antibacterial agents for enhanced aPDI performances. Krishna found that the adsorption of polyhydroxyl fullerene on the surface of TiO2 nanoparticles increased the photocatalytic performance of TiO2 nanoparticles by 70% through scavenging of photo-generated electrons. The combination of fullerene and TiO2 achieved a 2-fold higher killing rate against E. coli due to increased yields of HO⋅ (Krishna et al., 2008). Bai et al. (2012) synthesized a nanocomposite of polyhydroxy fullerene and TiO2 that can effectively kill microbes, such as Aspergillus niger, under visible light irradiation. The spore inactivation rate of this nanocomposite was 3∼4 times greater than that of pure TiO2. Wan et al. (2021) used fullerenols in conjunction with ultra-small copper nanoparticles to achieve synergistic antibacterial and antioxidative effects. The addition of fullerenols reduced the usage of copper nanoparticles, alleviating the copper-induced environmental harm. Ansari et al. (2022) loaded the surface of fullerenols with an antibacterial drug sulfasalazine and found that the addition of fullerenols decreased the minimum inhibitory concentration (MIC) of sulfasalazine against Bacillus cereus, Proteus mirabilis, and Salmonella typhimurium, but not against E. coli or S. aureus.
Fullerene Derivatives With Light-Harvesting Antenna
Despite the supreme 1O2 and ROS-generating ability, the applicability of fullerenes as a PS for aPDI in deep areas of the body is limited due to their short adsorption wavelengths (usually green, blue, or UV light which is hard to penetrate deep into the body) (Agazzi et al., 2015; Sobotta et al., 2019). Thus, covalently grafting light-harvesting agents, such as chromophores, on the surface of fullerene to broaden its adsorption spectrum is a desirable strategy to improve the aPDI effectiveness of fullerene in deep areas of the body. 4,4-Difluoro-4-bora-3a,4a-diaza-s-indacene (BODIPY) has the advantages of good photostability, low toxicity, high adsorption, and fluorescence emission in the visible range, and thus can be covalently grafted on the surface of fullerene as a “light-harvesting antenna” to increase the generation of excited triplet state and produce 1O2 through the Type-II mechanism (Wei et al., 2017; Durantini et al., 2018; Agazzi et al., 2019a,b). Agazzi et al. (2019b) grafted dimethylaminophenyl-BODIPY (aBDP) on the surface of N-methylfulleropyrrolidine (MC60) to synthesize a novel C60-BODIPY dyad (BDP-C60) (Figure 5A). The aBDP acted as a “light-harvesting antenna” and singlet energy or electron donor, while C60 served as singlet energy or electron acceptor to produce triplet state C60. Grafting of BODIPY red-shifted the adsorption peak from 415 to 515 nm, even longer than that of pure BODIPY (502 nm). Additionally, the 1O2 yield was found to be correlated with solvent polarity. In the toluene solution with strong polarity, 1O2 yield was high; while in the dimethylformamide solution with weak polarity, 1O2 yield was relatively low. The presence of BODIPY also increased the ROS yield of fullerene. The dyad exhibited excellent aPDI effectiveness at physiological pH, decreasing the viability of S. aureus by 99.97% and E. coli by 99.98% within 15 and 30 min of green light irradiation, respectively. Covalently binding a bis(difluoroboron)-1,2-bis((1H-pyrrol-2-yl)methylene)hydrazine (BOPHY) fluorophore unit to N-methylfulleropyrrolidine, Lopez et al. (2021) synthesized a BOPHY-C60 (BP-C60) dyad (Figure 5B) in which the BOPHY “antenna” not only improved light adsorption in the visible range, but also enhanced the photodynamic performance of the fullerene moiety. Under irradiation, the dyad effectively inactivated S. aureus by generating ROS through both Type-I and Type-II mechanisms in the presence of oxygen. However, this dyad did not exhibit a significant aPDI effect against E. coli. Sarikaya et al. (2019) synthesized a distryl-BODIPY-cyclotriphosphazene-fullerene triad (Figure 5C) with light adsorption and emission in the near-IR range, potentially extending the application of fullerene in aPDI. Agazzi et al. (2020) prepared a diketopyrrolopyrrole-C60 conjugate in which diketopyrrolopyrrole dyes acted as a light-harvesting antenna. The conjugate exhibited a high level of ROS generation and bactericidal effect against S. aureus under green light irradiation.
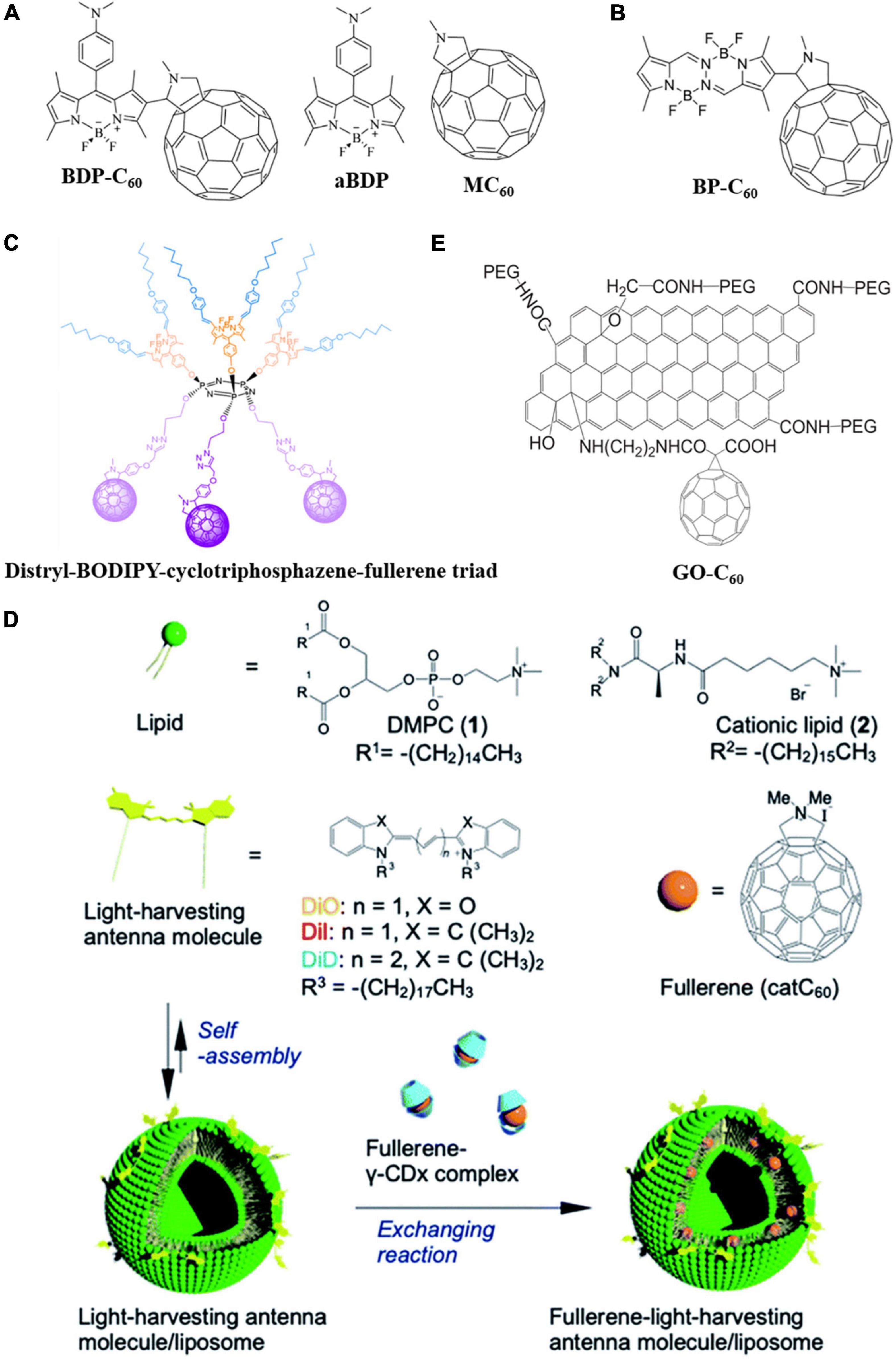
Figure 5. Molecular structures of the fullerene derivatives with a light-harvesting antenna. (A) BDP-C60, aBDP, and MC60 (Agazzi et al., 2019b). (B) BP-C60 (Lopez et al., 2021). (C) Distryl-BODIPY-cyclotriphosphazene-fullerene triad (Sarikaya et al., 2019). (D) Schematic diagram of LMIcatC60–light-harvesting antenna molecules (Kawasaki et al., 2020). (E) GO-C60 (Li et al., 2017).
Kawasaki et al. (2020) combined fullerene with light-harvesting agents using liposomes as a platform. Light-harvesting antenna molecules [DPMC and dyes, such as 1,1′-dioctadecyl-3,3,3′,3′-tetramethylindocarbocyanine perchlorate (DiI), λmax = 549 nm, 1,1′-dioctadecyl-3,3,3′,3′-tetramethylindodicarbocyanine (DiD), λmax = 644 nm, and 3,3′-dioctadecyloxacarbocyanine perchlorate (DiO), λmax = 484 nm] were first grafted on liposome surfaces, followed by the self-assembly of fullerene-cyclodextrin derivatives into liposomes to form LMIcatC60–light-harvesting antenna molecules (Figure 5D). Photoactivation of the antenna molecules efficiently transferred energy to fullerenes, eliminating bacteria without causing harm to erythrocytes. Gotfredsen et al. (2017) prepared a class of thieno-fused subporphyrazines with broad adsorption spectra and red-shifted adsorption limits as light-harvesting agents. These molecules are easy to co-crystallize with C60 and thus could be used as antennas to improve the aPDI effectiveness of fullerene. Li et al. (2017) covalently grafted graphene oxide (GO) as a light-harvesting antenna on fullerene to produce a PS for aPDI (Figure 5E). Taking advantage of GO’s strong adsorption in the near-IR range, the adsorption spectrum of GO-C60 was significantly broadened to enable photodynamic treatment in deep areas of the body.
Fullerene Derivatives Modified With Amino Acids and Peptides
Fullerene derivatives can be modified with amino acids and peptides through nucleophilic addition reactions (Yang et al., 2014; Pochkaeva et al., 2020). Kornev et al. (2012) prepared pentakisamino derivatives of C60 using C60Cl6 as a precursor with strong antibacterial activities against E. coli and B. subtilis. Antibacterial peptides are a family of novel antibacterial agents that kill bacteria by inducing cell membrane rupture and cellular content leakage via electrostatic interactions with the cell membrane (Raheem and Straus, 2019; Yan et al., 2021). Nevertheless, the bactericidal performance of antibacterial peptides against Gram-negative bacteria is limited by the relatively dense outer cell membrane. Conjugation of fullerene and antibacterial peptides can greatly overcome this limitation. Generally, there are two common strategies to combine fullerene with peptides: (1) Covalently grafting pre-formed peptides on fullerene; and (2) Introducing the fullerene fragment as a special amino acid into the peptide sequence (Pochkaeva et al., 2020). Using the first approach, Pellarini et al. (2001) prepared a C60-functionalized amino acid by condensing a fullerene derivative containing a free amino group with N-Fmoc-L-glutamic acidr-tert-butyl ester (Figure 6A). The unmodified peptides showed no antibacterial activity against E. coli or S. aureus. Conjugation of fullerene significantly enhanced the antibacterial performance, with a MIC of 8 and 64 μM against S. aureus and E. coli, respectively. Pantarotto et al. (2002) adopted the second strategy and introduced a fulleropyrrolidino-glutamic acid residue (Fgu) into the peptide sequence (H-Gly-(Nle)2-Gln-Orn-Nle-Gly-(Orn)2-Nle-(Orn)2-Nle-Gly-(Orn)2-Nle-Gly-Tyr-NH2) using solid-phase synthesis and investigated the influence of the position of Fgu on aPDI performance (Figure 6B). Compared with other cationic antimicrobial peptides, the Fgu-functionalized peptides exhibited significantly higher antibacterial activities against S. aureus. The introduction of Fgu at the N terminus of the peptide achieved better antibacterial effectiveness than in the middle of the peptide. Zhang et al. (2018) fabricated a hybrid hydrogel containing C60 pyrrolidine tris-acid (C60-PTC) and amphiphilic small peptides (Fmoc-FF) (Figure 6C). The hybrid hydrogel effectively inhibited S. aureus growth by generating ROS and promoted wound healing.
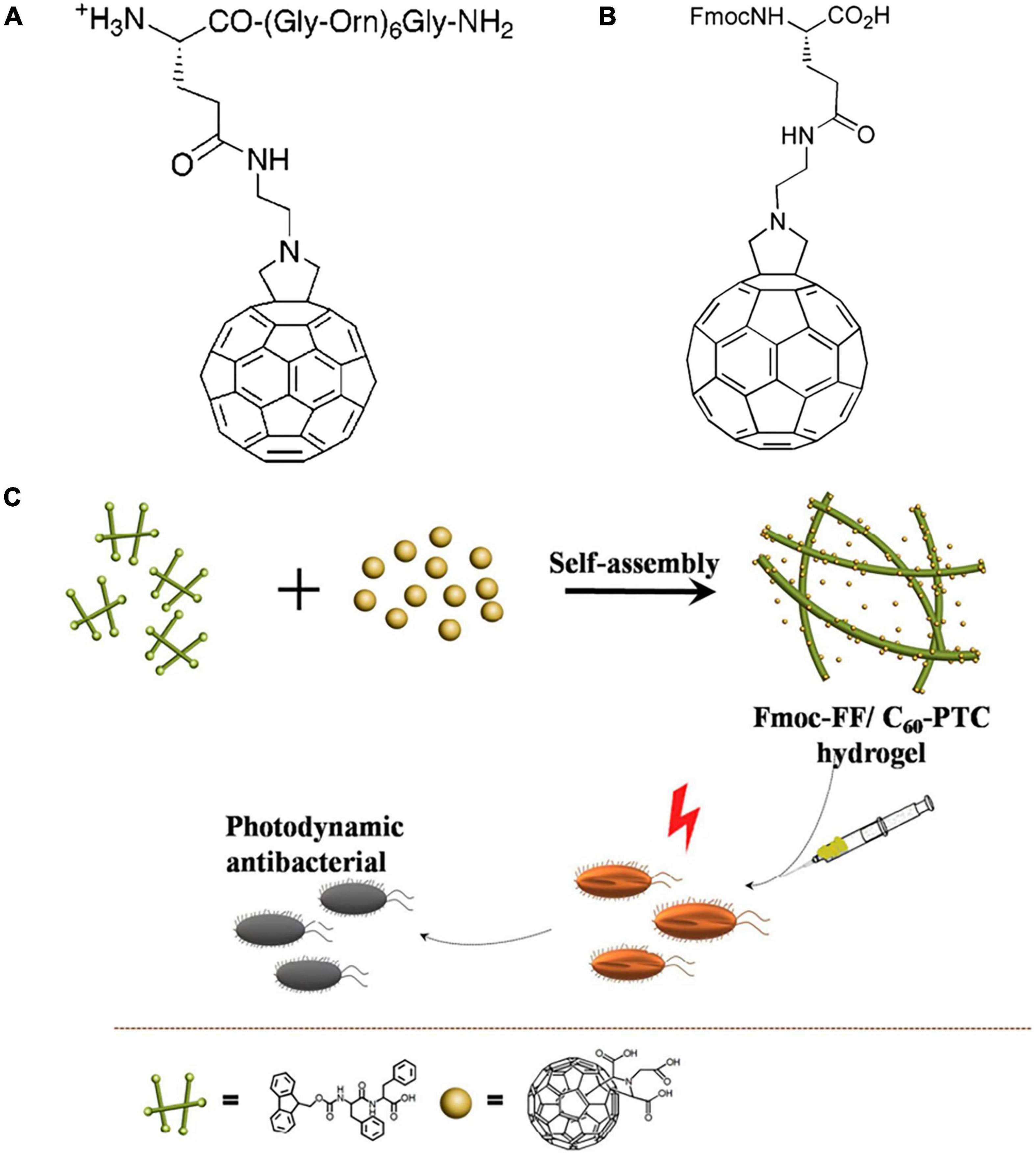
Figure 6. (A) The molecular structure of C60-functionalized amino acid with N-Fmoc-L-glutamic acidr-tert-butyl ester (Pellarini et al., 2001). (B) The molecular structure of Fgu (Pantarotto et al., 2002). (C) Schematic diagram of Fmoc-FF/C60-PTC hydrogel (Zhang et al., 2018).
Fullerene as Additives to Other Materials
Fullerenes can also be used as additives to other materials for optimized antibacterial performance. Gudkov et al. (2021) added fullerene as an additive to borosiloxane polymers and observed noticeable ROS production and bacterial inhibition under visible light irradiation. The ROS yield of the composite increased with the increase of the amount of fullerene added. A reliable indicator of oxidative damage to DNA by ROS is the levels of long-lived active forms of proteins (LRPS) and 8-oxoguanine (8-OH-Gua; Matsuda et al., 2011). It was observed that the composite induced a significant increase in LRPS and 8-OH-Gua levels, indicating that the ROS produced by fullerene caused oxidative damage to bacterial DNA and cell death. Liu et al. (2022) introduced a self-assembled fullerene derivative [6,6]-phenyl-C71-butyric acid methyl ester (PC71BM) to the surface of g-C3N4, a polymer-like organic photocatalytic semiconductor (Figure 7A) to prepare heterostructures for water purification. The presence of PC71BM extended the adsorption range of g-C3N4 from 450 to 650 nm and generated abundant ROS under irradiation. The heterostructures achieved a bactericidal rate of over 99.7% against S. aureus and E. coli, and effectively inactivated their biofilms after prolonged irradiation (Figure 7B).
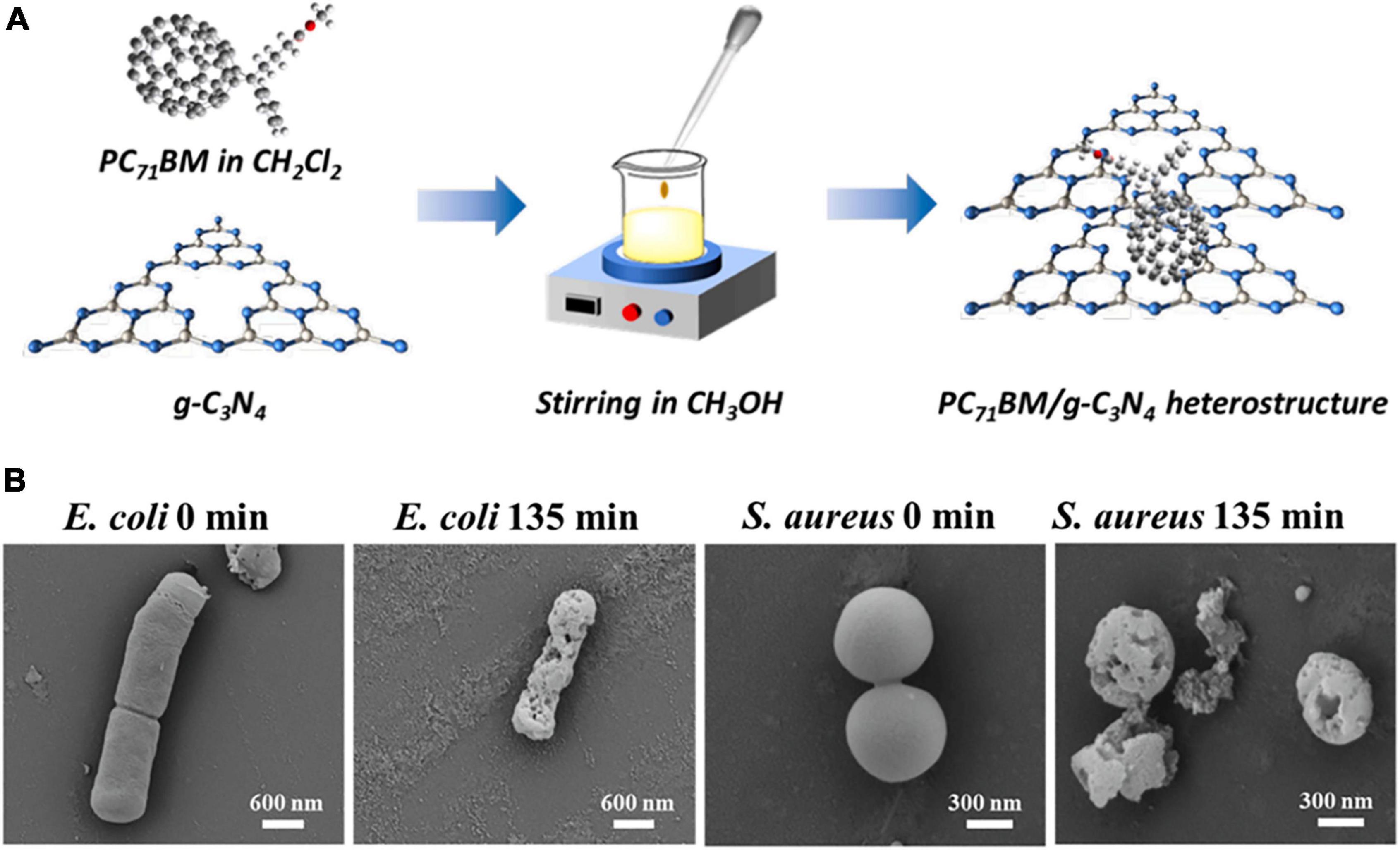
Figure 7. (A) Schematic diagram of the fabrication of PC71BM/g-C3N4 photocatalysts. (B) SEM images of E. coli and S. aureus before and after irradiation for 135 min with PC71BM/g-C3N4 (Liu et al., 2022).
Conclusion and Perspectives
Overuse of antibiotics has brought about the issue of multidrug resistance of bacteria. In the past decades, various novel antibacterial strategies have been proposed as potential alternatives to antibiotics (Alavi and Rai, 2021; Fazeli-Nasab et al., 2021; Khodadadi et al., 2021; Ahmadi, 2022; Alavi et al., 2022a,b). As a newly emerged antibacterial strategy, aPDI can effectively address the issue of drug resistance and thus has promising prospects of application in treating bacterial infections. Fullerene can be used as a PS for aPDI for its high 1O2 and ROS yields and bactericidal activities against both Gram-positive and Gram-negative bacteria. This review summarizes the up-to-date studies on the application of fullerene derivatives in aPDI, including fullerene-pyrrolidine derivatives, fullerene-cyclopropane derivatives, fullerenols, fullerene derivatives with light-harvesting antenna, fullerene derivatives modified with amino acids and peptides, and fullerenes as additives to other materials.
Grafting cationic functional groups on the surface of fullerenes is an effective strategy to improve their ability to target negatively charged bacterial cells. Nevertheless, it is worth noting that surface modification may affect the photophysical and photochemical properties of fullerene, resulting in decreased triplet state yield and ROS generation. Moreover, although fullerene-cyclopropane derivatives have a greater number of cationic charges, they are also of higher molecular weights and thus are more difficult to penetrate into bacteria. Therefore, it is desirable to find a balance between the number of cationic charges and molecular weight for optimized bactericidal efficacy.
The adsorption wavelengths of fullerenes are limited to the green, blue, and UV regions, limiting their use in deep areas of the body. Although grafting light-harvesting antennas is a potent strategy to broaden the adsorption range of fullerenes, application of this strategy in aPDI is still rare and remains to be explored. Conjugation of fullerenes can also increase the susceptibility of bacteria to antibacterial peptides. However, the binding site of fullerenes has a considerable influence on the bactericidal efficacy of antibacterial peptides, which is worthwhile to be extensively investigated. Additionally, fullerenes also possess ROS clearance ability in the dark, which is beneficial to the proliferation of normal cells and tissue growth. This advantage makes fullerenes promising candidates as additives to wound dressings and dental implants with both antibacterial and wound healing promotion abilities.
Furthermore, the shape, structure, and performance of fullerene derivatives are closely related to preparation methods. Distinct antibacterial performances have been reported for the same fullerene derivatives prepared with different methods, and for those commercially manufactured and prepared in the laboratory (Indeglia et al., 2018). Therefore, it is needed to further investigate the relationships between preparation methods, molecular structures, and antibacterial performance to optimize and standardize the preparation of fullerene derivatives. Finally, more attention needs to be paid to the histotoxicity resulting from the accumulation of fullerene nanoparticles in organs, such as the liver and the spleen (Shipkowski et al., 2019). To address this issue, the interactions between fullerene nanoparticles and serum proteins need to be disclosed, and the influence of such interactions on their in vivo destiny needs to be investigated.
Author Contributions
WH, GS, JM, and LS: writing – original draft preparation. WH and SW: writing – review and editing. XZ, WH, and YZ: funding acquisition. XZ and YZ: resources. All authors contributed to the article and approved the submitted version.
Funding
This work was supported by the Natural Science Foundation of Zhejiang Province (grant # LQ21C100001) and project of the Key Laboratory of Marine Materials and Related Technologies, CAS and Zhejiang Key Laboratory of Marine Materials and Protective Technologies (grant # 2021K09).
Conflict of Interest
The authors declare that the research was conducted in the absence of any commercial or financial relationships that could be construed as a potential conflict of interest.
Publisher’s Note
All claims expressed in this article are solely those of the authors and do not necessarily represent those of their affiliated organizations, or those of the publisher, the editors and the reviewers. Any product that may be evaluated in this article, or claim that may be made by its manufacturer, is not guaranteed or endorsed by the publisher.
References
Agazzi, M. L., Almodovar, V. A. S., Gsponer, N. S., Bertolotti, S., Tome, A. C., and Durantini, E. N. (2020). Diketopyrrolopyrrole-fullerene C60 architectures as highly efficient heavy atom-free photosensitizers: synthesis, photophysical properties and photodynamic activity. Org. Biomol. Chem. 18, 1449–1461. doi: 10.1039/c9ob02487e
Agazzi, M. L., Belen Ballatore, M., Durantini, A. M., Durantini, E. N., and Tome, A. C. (2019a). BODIPYs in antitumoral and antimicrobial photodynamic therapy: an integrating review. J. Photoch. Photobio. C 40, 21–48. doi: 10.1016/j.jphotochemrev.2019.04.001
Agazzi, M. L., Durantini, J. E., Gsponer, N. S., Durantini, A. M., Bertolotti, S. G., and Durantini, E. N. (2019b). Light-harvesting antenna and proton-activated photodynamic effect of a novel BODIPY-fullerene C60 dyad as potential antimicrobial agent. Chemphyschem 20, 1110–1125. doi: 10.1002/cphc.201900181
Agazzi, M. L., Durantini, J. E., Quiroga, E. D., Alvarez, M. G., and Durantini, E. N. (2021). A novel tricationic fullerene C60 as broad-spectrum antimicrobial photosensitizer: mechanisms of action and potentiation with potassium iodide. Photoch. Photobio. Sci. 20, 327–341. doi: 10.1007/s43630-021-00021-1
Agazzi, M. L., Spesia, M. B., Gsponer, N. S., Milanesio, M. E., and Durantini, E. N. (2015). Synthesis, spectroscopic properties and photodynamic activity of a fulleropyrrolidine bearing a basic amino group and its dicationic analog against Staphylococcus aureus. J. Photoch. Photobio. A 310, 171–179. doi: 10.1016/j.jphotochem.2015.05.022
Ahmadi, S. (2022). Antibacterial and antifungal activities of medicinal plant species and endophytes. Cell. Mol. Biomed. Rep. 2, 109–115. doi: 10.55705/cmbr.2022.340532.1042
Alavi, M., Hamblin, M. R., Martinez, F., Kennedy, J. F., and Khan, H. (2022a). Synergistic combinations of metal, metal oxide, or metalloid nanoparticles plus antibiotics against resistant and non-resistant bacteria. Micro. Nano Bio. Aspects 1, 1–9.
Alavi, M., Hamblin, M. R., Mozafari, M. R., de Menezes, I., and Coutinho, H. (2022b). Surface modification of SiO2 nanoparticles for bacterial decontaminations of blood products. Cell. Mol. Biomed. Rep. 2, 87–98. doi: 10.55705/cmbr.2022.338888.1039
Alavi, M., and Rai, M. (2021). Antisense RNA, the modified CRISPR-Cas9, and metal/metal oxide nanoparticles to inactivate pathogenic bacteria. Cell. Mol. Biomed. Rep. 1, 52–59. doi: 10.55705/CMBR.2021.142436.1014
Allison, R. R., and Moghissi, K. (2013). Photodynamic therapy (PDT): PDT mechanisms. Clin. Endosc. 46, 24–29. doi: 10.5946/ce.2013.46.1.24
Al-Mutairi, R., Tovmasyan, A., Batinic-Haberle, I., and Benov, L. (2018). Sublethal photodynamic treatment does not lead to development of resistance. Front. Microbiol. 9:1699. doi: 10.3389/fmicb.2018.01699
An, H., and Jin, B. (2011). DNA exposure to buckminsterfullerene (C60): toward DNA stability, reactivity, and replication. Environ. Sci. Technol. 45, 6608–6616. doi: 10.1021/es2012319
An, H., and Jin, B. (2015). Fullerenols and fullerene alter cell growth and metabolisms of Escherichia coli. J. Biomed. Nanotechnol. 11, 1261–1268. doi: 10.1166/jbn.2015.2070
Ansari, M. J., Soltani, A., Ramezanitaghartapeh, M., Singla, P., Aghaei, M., Fadafan, H. K., et al. (2022). Improved antibacterial activity of sulfasalazine loaded fullerene derivative: computational and experimental studies. J. Mol. Liq. 348:118083. doi: 10.1016/j.molliq.2021.118083
Aoshima, H., Kokubo, K., Shirakawa, S., Ito, M., Yamana, S., and Oshima, T. (2009). Antimicrobial activity of fullerenes and their hydroxylated derivatives. Biocontrol. Sci. 14, 69–72. doi: 10.4265/bio.14.69
Armstrong, D. W., Yu, J., Cole, H. D., McFarland, S. A., and Nafie, J. (2021). Chiral resolution and absolute configuration determination of new metal-based photodynamic therapy antitumor agents. J. Pharmaceut. Biomed. 204:114233. doi: 10.1016/j.jpba.2021.114233
Bai, W., Krishna, V., Wang, J., Moudgil, B., and Koopman, B. (2012). Enhancement of nano titanium dioxide photocatalysis in transparent coatings by polyhydroxy fullerene. Appl. Catal. B-Environ. 125, 128–135. doi: 10.1016/j.apcatb.2012.05.026
Bertoloni, G., Rossi, F., Valduga, G., Jori, G., Ali, H., and van Lier, J. E. (1992). Photosensitizing activity of water- and lipid-soluble phthalocyanines on prokaryotic and eukaryotic microbial cells. Microbios 71, 33–46.
Betancourt, P., Brocal, N., Sans-Serramitjana, E., and Zaror, C. (2021). Functionalized nanoparticles activated by photodynamic therapy as an antimicrobial strategy in endodontics: a scoping review. Antibiotics-Basel 10:1064. doi: 10.3390/antibiotics10091064
Blum, N. T., Zhang, Y., Qu, J., Lin, J., and Huang, P. (2020). Recent advances in self-exciting photodynamic therapy. Front. Bioeng. Biotechnol. 8:594491. doi: 10.3389/fbioe.2020.594491
Brunet, L., Lyon, D. Y., Hotze, E. M., Alvarez, P. J. J., and Wiesner, M. R. (2009). Comparative photoactivity and antibacterial properties of c-60 fullerenes and titanium dioxide nanoparticles. Environ. Sci. Technol. 43, 4355–4360. doi: 10.1021/es803093t
Cadet, J., Douki, T., and Ravanat, J.-L. (2010). Oxidatively generated base damage to cellular DNA. Free Radical Bio. Med. 49, 9–21. doi: 10.1016/j.freeradbiomed.2010.03.025
Chen, X., Pradhan, T., Wang, F., Kim, J. S., and Yoon, J. (2012). Fluorescent chemosensors based on spiroring-opening of xanthenes and related derivatives. Chem. Rev. 112, 1910–1956. doi: 10.1021/cr200201z
Choudhary, S., Nouri, K., and Elsaie, M. L. (2009). Photodynamic therapy in dermatology: a review. Laser Med. Sci. 24, 971–980. doi: 10.1007/s10103-009-0716-x
Diogo, P., Faustino, M. A. F., Neves, M. G., Palma, P. J., Baptista, I. P., Goncalves, T., et al. (2019). An insight into advanced approaches for photosensitizer optimization in endodontics-a critical review. J. Funct. Biomater. 10:44. doi: 10.3390/jfb10040044
Durantini, A. M., Heredia, D. A., Durantini, J. E., and Durantini, E. N. (2018). BODIPYs to the rescue: potential applications in photodynamic inactivation. Eur. J. Med. Chem. 144, 651–661. doi: 10.1016/j.ejmech.2017.12.068
Fang, J. S., Lyon, D. Y., Wiesner, M. R., Dong, J. P., and Alvarez, P. J. J. (2007). Effect of a fullerene water suspension on bacterial phospholipids and membrane phase behavior. Environ. Sci. Technol. 41, 2636–2642. doi: 10.1021/es062181w
Fazeli-Nasab, B., Solouki, M., and Sobhanizadeh, A. (2021). Green synthesis of silver nanoparticles using an ephedra sinica herb extract with antibacterial properties. JMB 10, 30–47.
Gad, F., Zahra, T., Francis, K. P., Hasan, T., and Hamblin, M. R. (2004). Targeted photodynamic therapy of established soft-tissue infections in mice. Photoch. Photobio. Sci. 3, 451–458. doi: 10.1039/b311901g
Galstyan, A. (2021). Turning photons into drugs: phthalocyanine-based photosensitizers as efficient photoantimicrobials. Chemistry 27, 1903–1920. doi: 10.1002/chem.202002703
Gao, Y., Tong, H., Li, J., Li, J., Huang, D., Shi, J., et al. (2021). Mitochondria-targeted nanomedicine for enhanced efficacy of cancer therapy. Front. Bioeng. Biotechnol. 9:720508. doi: 10.3389/fbioe.2021.720508
Giuliani, F., Martinelli, M., Cocchi, A., Arbia, D., Fantetti, L., and Roncucci, G. (2010). In vitro resistance selection studies of RLP068/Cl, a new Zn(II) phthalocyanine suitable for antimicrobial photodynamic therapy. Antimicrob. Agents 54, 637–642. doi: 10.1128/aac.00603-09
Gomes, A., Neves, M., and Cavaleiro, J. A. S. (2018). Cancer, photodynamic therapy and porphyrin-type derivatives. An. Acad. Bras. Cienc. 90, 993–1026. doi: 10.1590/0001-3765201820170811
Gotfredsen, H., Storm, F. E., Munoz, A. V., Santella, M., Kadziola, A., Hammerich, O., et al. (2017). Thieno-fused subporphyrazines: a new class of light harvesters. Chemistry 23, 16194–16198. doi: 10.1002/chem.201704551
Grinholc, M., Nakonieczna, J., Fila, G., Taraszkiewicz, A., Kawiak, A., Szewczyk, G., et al. (2015). Antimicrobial photodynamic therapy with fulleropyrrolidine: photoinactivation mechanism of Staphylococcus aureus, in vitro and in vivo studies. Appl. Microbiol. Biot. 99, 4031–4043. doi: 10.1007/s00253-015-6539-8
Gsponer, N. S., Agazzi, M. L., Spesia, M. B., and Durantini, E. N. (2016). Approaches to unravel pathways of reactive oxygen species in the photoinactivation of bacteria induced by a dicationic fulleropyrrolidinium derivative. Methods 109, 167–174. doi: 10.1016/j.ymeth.2016.05.019
Gudkov, S. V., Simakin, A. V., Sarimov, R. M., Kurilov, A. D., and Chausov, D. N. (2021). Novel biocompatible with animal cells composite material based on organosilicon polymers and fullerenes with light-induced bacteriostatic properties. Nanomaterials 11:2804. doi: 10.3390/nano11112804
Guldi, D. M., and Prato, M. (2000). Excited-state properties of C60 fullerene derivatives. Accounts Chem. Res. 33, 695–703. doi: 10.1021/ar990144m
Hamblin, M. R. (2016). Antimicrobial photodynamic inactivation: a bright new technique to kill resistant microbes. Curr. Opin. Microbiol. 33, 67–73. doi: 10.1016/j.mib.2016.06.008
Hamblin, M. R. (2018). Fullerenes as photosensitizers in photodynamic therapy: pros and cons. Photoch. Photobio. Sci. 17, 1515–1533. doi: 10.1039/c8pp00195b
Heredia, D. A., Durantini, A. M., Durantini, J. E., and Durantini, E. N. (2022). Fullerene C60 derivatives as antimicrobial photodynamic agents. J. Photoch. Photobio. C 51:100471. doi: 10.1016/j.jphotochemrev.2021.100471
Hu, X., Huang, Y. Y., Wang, Y., Wang, X., and Hamblin, M. R. (2018). Antimicrobial photodynamic therapy to control clinically relevant biofilm infections. Front. Microbiol. 9:1299. doi: 10.3389/fmicb.2018.01299
Huang, L., Wang, M., Dai, T., Sperandio, F. F., Huang, Y.-Y., Xuan, Y., et al. (2014). Antimicrobial photodynamic therapy with decacationic monoadducts and bisadducts of [70] fullerene: in vitro and in vivo studies. Nanomedicine 9, 253–266. doi: 10.2217/nnm.13.22
Huang, L., Xuan, Y., Koide, Y., Zhiyentayev, T., Tanaka, M., and Hamblin, M. R. (2012). Type I and type II mechanisms of antimicrobial photodynamic therapy: an in vitro study on gram-negative and gram-positive bacteria. Laser Surg. Med. 44, 490–499. doi: 10.1002/lsm.22045
Huang, Y.-Y., Sharma, S. K., Yin, R., Agrawal, T., Chiang, L. Y., and Hamblin, M. R. (2014). Functionalized fullerenes in photodynamic therapy. J. Biomed. Nanotechnol. 10, 1918–1936. doi: 10.1166/jbn.2014.1963
Ikeda, A., Doi, Y., Hashizume, M., Kikuchi, J., and Konishi, T. (2007). An extremely effective DNA photocleavage utilizing functionalized liposomes with a fullerene-enriched lipid bilayer. J. Am. Chem. Soc. 129, 4140–4141. doi: 10.1021/ja070243s
Indeglia, P. A., Georgieva, A. T., Krishna, V. B., Martyniuk, C. J., and Bonzongo, J. C. J. (2018). Toxicity of functionalized fullerene and fullerene synthesis chemicals. Chemosphere 207, 1–9. doi: 10.1016/j.chemosphere.2018.05.023
Inoue, K. (2017). 5-aminolevulinic acid-mediated photodynamic therapy for bladder cancer. Int. J. Urol. 24, 97–101. doi: 10.1111/iju.13291
Iwamoto, Y., and Yamakoshi, Y. (2006). A highly water-soluble C60-NVP copolymer: a potential material for photodynamic therapy. Chem. Commun. 46, 4805–4807. doi: 10.1039/b614305a
Kashef, N., and Hamblin, M. R. (2017). Can microbial cells develop resistance to oxidative stress in antimicrobial photodynamic inactivation? Drug Resis. Update 31, 31–42. doi: 10.1016/j.drup.2017.07.003
Kawasaki, R., Antoku, D., Ohdake, R., Sugikawa, K., and Ikeda, A. (2020). Bacterial elimination via the photodynamic activity of a fullerene/light-harvesting antenna molecule assembled system integrated into liposome membranes. Nanoscale Adv. 2, 4395–4399. doi: 10.1039/d0na00132e
Khodadadi, S., Mahdinezhad, N., Fazeli-Nasab, B., Heidari, M. J., Fakheri, B., and Miri, A. (2021). Investigating the possibility of green synthesis of silver nanoparticles using vaccinium arctostaphlyos extract and evaluating its antibacterial properties. Biomed. Res. Int. 30, 5572252. doi: 10.1155/2021/5572252
Koeppe, R., and Sariciftci, N. S. (2006). Photoinduced charge and energy transfer involving fullerene derivatives. Photoch. Photobio. Sci. 5, 1122–1131. doi: 10.1039/b612933c
Koren, H., Schenk, G. M., Jindra, R. H., Alth, G., Ebermann, R., Kubin, A., et al. (1996). Hypericin in phototherapy. J. Photochem. Photobiol. B 36, 113–119. doi: 10.1016/s1011-1344(96)07357-5
Kornev, A. B., Khakina, E. A., Troyanov, S. I., Kushch, A. A., Peregudov, A., Vasilchenko, A., et al. (2012). Facile preparation of amine and amino acid adducts of [60]fullerene using chlorofullerene C60Cl6 as a precursor. Chem. Commun. 48, 5461–5463. doi: 10.1039/c2cc00071g
Krishna, V., Yanes, D., Imaram, W., Angerhofer, A., Koopman, B., and Moudgil, B. (2008). Mechanism of enhanced photocatalysis with polyhydroxy fullerenes. Appl. Catal. B-Environ. 79, 376–381. doi: 10.1016/j.apcatb.2007.10.020
Kuo, W.-S., Chang, C.-Y., Liu, J.-C., Chen, J.-H., So, E. C., and Wu, P.-C. (2020). Two-photon photoexcited photodynamic therapy with water-soluble fullerenol serving as the highly effective two-photon photosensitizer against multidrug-resistant bacteria. Int. J. Nanomed. 15, 6813–6825. doi: 10.2147/ijn.S236897
Li, Q., Hong, L., Li, H. G., and Liu, C. G. (2017). Graphene oxide-fullerene C60 (Go-C60) hybrid for photodynamic and photothermal therapy triggered by near-infrared light. Biosens. Bioelectron. 89, 477–482. doi: 10.1016/j.bios.2016.03.072
Liu, X. J., Ji, Y. J., Du, Y. H., Jing, X., Zhao, Y., Dou, K. K., et al. (2022). A “green” all-organic heterostructure functionalized by self-assembled fullerene small molecule with enhanced photocatalytic activity. Appl. Surf. Sci. 585:152738. doi: 10.1016/j.apsusc.2022.152738
Lopez, E. J. G., Sarotti, A. M., Martinez, S. R., Macor, L. P., Durantini, J. E., Renfige, M., et al. (2021). BOPHY-fullerene C60 dyad as a photosensitizer for antimicrobial photodynamic therapy. Chemistry 28:e202103884. doi: 10.1002/chem.202103884
Luchian, I., Goriuc, A., Martu, M. A., and Covasa, M. (2021). Clindamycin as an alternative option in optimizing periodontal therapy. Antibiotics Basel 10:814. doi: 10.3390/antibiotics10070814
Lyon, D. Y., and Alvarez, P. J. J. (2008). Fullerene water suspension (nC60) exerts antibacterial effects via ros-independent protein oxidation. Environ. Sci. Technol. 42, 8127–8132. doi: 10.1021/es801869m
Mashino, T., Usui, N., Okuda, K., Hirota, T., and Mochizuki, M. (2003). Respiratory chain inhibition by fullerene derivatives: hydrogen peroxide production caused by fullerene derivatives and a respiratory chain system. Bioorgan. Med. Chem. 11, 1433–1438. doi: 10.1016/s0968-0896(02)00610-7
Matsuda, S., Matsui, S., Shimizu, Y., and Matsuda, T. (2011). Genotoxicity of colloidal fullerene C60. Environ. Sci. Technol. 45, 4133–4138. doi: 10.1021/es1036942
Merchat, M., Spikes, J. D., Bertoloni, G., and Jori, G. (1996). Studies on the mechanism of bacteria photosensitization by meso-substituted cationic porphyrins. J. Photoch. Photobio. B 35, 149–157. doi: 10.1016/s1011-1344(96)07321-6
Michaeli, A., and Feitelson, J. (1994). Reactivity of singlet oxygen toward amino acids and peptides. Photochem. photobiol. 59, 284–289. doi: 10.1111/j.1751-1097.1994.tb05035.x
Mizuno, K., Zhiyentayev, T., Huang, L., Khalil, S., Nasim, F., Tegos, G. P., et al. (2011). Antimicrobial photodynamic therapy with functionalized fullerenes: quantitative structure-activity relationships. J. Nanomed. Nanotechnol. 2, 1–9. doi: 10.4172/2157-7439.1000109
Mohammed, H., Kumar, A., Bekyarova, E., Al-Hadeethi, Y., Zhang, X., Chen, M., et al. (2020). Antimicrobial mechanisms and effectiveness of graphene and graphene-functionalized biomaterials. a scope review. Front. Bioeng. Biotechnol. 8:465. doi: 10.3389/fbioe.2020.00465
Nakagawa, Y., Suzuki, T., Nakajima, K., Inomata, A., Ogata, A., and Nakae, D. (2014). Effects of N-acetyl-L-cysteine on target sites of hydroxylated fullerene-induced cytotoxicity in isolated rat hepatocytes. Arch. Toxicol. 88, 115–126. doi: 10.1007/s00204-013-1096-3
Nishizawa, C., Hashimoto, N., Yokoo, S., Funakoshi-Tago, M., Kasahara, T., Takahashi, K., et al. (2009). Pyrrolidinium-type fullerene derivative-induced apoptosis by the generation of reactive oxygen species in HL-60 cells. Free Rad. Res. 43, 1240–1247. doi: 10.3109/10715760903273849
Ochsner, M. (1997). Photophysical and photobiological processes in the photodynamic therapy of tumours. J. Photoch. Photobio. B 39, 1–18. doi: 10.1016/s1011-1344(96)07428-3
Oruba, Z., and Chomyszyn-Gajewska, M. (2016). Application of photodynamic therapy in dentistry - literature review. Przegl. Lek. 73, 857–861.
Palacios, Y. B., Durantini, J. E., Heredia, D. A., Martinez, S. R., Gonzalez de la Torre, L., and Durantini, A. M. (2021). Tuning the polarity of fullerene C60 derivatives for enhanced photodynamic inactivation(dagger). Photoch. Photobio. 97, 1431–1444. doi: 10.1111/php.13465
Pan, Y., Liu, X., Zhang, W., Liu, Z., Zeng, G., Shao, B., et al. (2020). Advances in photocatalysis based on fullerene C60 and its derivatives: properties, mechanism, synthesis, and applications. Appl. Catal. B Environ. 265:118579. doi: 10.1016/j.apcatb.2019.118579
Pantarotto, D., Bianco, A., Pellarini, F., Tossi, A., Giangaspero, A., Zelezetsky, I., et al. (2002). Solid-phase synthesis of fullerene-peptides. J. Am. Chem. Soc. 124, 12543–12549. doi: 10.1021/ja027603q
Pellarini, F., Pantarotto, D., Da Ros, T., Giangaspero, A., Tossi, D., and Prato, N. (2001). A novel [60]fullerene amino acid for use in solid-phase peptide synthesis. Org. Lett. 3, 1845–1848. doi: 10.1021/ol015934m
Pickering, K. D., and Wiesner, M. R. (2005). Fullerol-sensitized production of reactive oxygen species in aqueous solution. Environ. Sci. Technol. 39, 1359–1365. doi: 10.1021/es048940x
Pieranski, M., Sitkiewicz, I., and Grinholc, M. (2020). Increased photoinactivation stress tolerance of Streptococcus agalactiae upon consecutive sublethal phototreatments. Free Radical Bio. Med. 160, 657–669. doi: 10.1016/j.freeradbiomed.2020.09.003
Pobłocki, K., Drzeżdżon, J., Kostrzewa, T., and Jacewicz, D. (2021). Coordination complexes as a new generation photosensitizer for photodynamic anticancer therapy. Int. J. Mol. Sci. 22:8052. doi: 10.3390/ijms22158052
Pochkaeva, E. I., Podolsky, N. E., Zakusilo, D. N., Petrov, A. V., Charykov, N. A., Vlasov, T. D., et al. (2020). Fullerene derivatives with amino acids, peptides and proteins: from synthesis to biomedical application. Prog. Solid State Ch. 57:100255. doi: 10.1016/j.progsolidstchem.2019.100255
Prestinaci, F., Pezzotti, P., and Pantosti, A. (2015). Antimicrobial resistance: a global multifaceted phenomenon. Pathog. Glob. Health 109, 309–318. doi: 10.1179/2047773215y.0000000030
Radic, S., Nedumpully-Govindan, P., Chen, R., Salonen, E., Brown, J. M., Ke, P. C., et al. (2014). Effect of fullerenol surface chemistry on nanoparticle binding-induced protein misfolding. Nanoscale 6, 8340–8349. doi: 10.1039/c4nr01544d
Raheem, N., and Straus, S. K. (2019). Mechanisms of action for antimicrobial peptides with antibacterial and antibiofilm functions. Front. Microbiol. 10:2866. doi: 10.3389/fmicb.2019.02866
Rahmati, M., and Mozafari, M. (2019). Biological response to carbon-family nanomaterials: interactions at the nano-bio interface. Front. Bioeng. Biotechnol. 7:4. doi: 10.3389/fbioe.2019.00004
Rajendran, M. (2016). Quinones as photosensitizer for photodynamic therapy: ros generation, mechanism and detection methods. Photodiagnosis Photodyn. Ther. 13, 175–187. doi: 10.1016/j.pdpdt.2015.07.177
Rapacka-Zdonczyk, A., Wozniak, A., Michalska, K., Pieranski, M., Ogonowska, P., Grinholc, M., et al. (2021). Factors determining the susceptibility of bacteria to antibacterial photodynamic inactivation. Front. Med. Lausanne 8:642609. doi: 10.3389/fmed.2021.642609
Rawson, T. M., Wilson, R. C., O’Hare, D., Herrero, P., Kambugu, A., Lamorde, M., et al. (2021). Optimizing antimicrobial use: challenges, advances and opportunities. Nat. Rev. Microbiol. 19, 747–758. doi: 10.1038/s41579-021-00578-9
Sarikaya, S. Y., Yesilot, S., Kilic, A., and Okutan, E. (2019). NIR BODIPY-cyclotriphosphazene-fullerene assemblies: photophyisical properties and photosensitized generation of singlet oxygen. Dyes Pigments 162, 734–740. doi: 10.1016/j.dyepig.2018.11.011
Sayes, C. M., Fortner, J. D., Guo, W., Lyon, D., Boyd, A. M., Ausman, K. D., et al. (2004). The differential cytotoxicity of water-soluble fullerenes. Nano Lett. 4, 1881–1887. doi: 10.1021/nl0489586
Sera, N., Tokiwa, H., and Miyata, N. (1996). Mutagenicity of the fullerene C60-generated singlet oxygen dependent formation of lipid peroxides. Carcinogenesis 17, 2163–2169. doi: 10.1093/carcin/17.10.2163
Sharma, S. K., Chiang, L. Y., and Hamblin, M. R. (2011). Photodynamic therapy with fullerenes in vivo: reality or a dream? Nanomedicine 6, 1813–1825. doi: 10.2217/nnm.11.144
Shipkowski, K. A., Sanders, J. M., McDonald, J. D., Walker, N. J., and Waidyanatha, S. (2019). Disposition of fullerene C60 in rats following intratracheal or intravenous administration. Xenobiotica 49, 1078–1085. doi: 10.1080/00498254.2018.1528646
Simonetti, O., Rizzetto, G., Radi, G., Molinelli, E., Cirioni, O., Giacometti, A., et al. (2021). New perspectives on old and new therapies of Staphylococcal skin infections: the role of biofilm targeting in wound healing. Antibiotics Basel 10:1377. doi: 10.3390/antibiotics10111377
Sobotta, L., Skupin-Mrugalska, P., Piskorz, J., and Mielcarek, J. (2019). Non-porphyrinoid photosensitizers mediated photodynamic inactivation against bacteria. Dyes Pigments 163, 337–355. doi: 10.1016/j.dyepig.2018.12.014
Soncin, M., Fabris, C., Busetti, A., Dei, D., Nistri, D., Roncucci, G., et al. (2002). Approaches to selectivity in the Zn(II)-phthalocyanine-photosensitized inactivation of wild-type and antibiotic-resistant Staphylococcus aureus. Photoch. Photobio. Sci. 1, 815–819. doi: 10.1039/b206554a
Sperandio, F. F., Sharma, S. K., Wang, M., Jeon, S., Huang, Y. Y., Dai, T. H., et al. (2013). Photoinduced electron-transfer mechanisms for radical-enhanced photodynamic therapy mediated by water-soluble decacationic C70 and C84O2 fullerene derivatives. Nanomed Nanotechnol 9, 570–579. doi: 10.1016/j.nano.2012.09.005
Sperandio, F. F., Simões, A., Aranha, A. C., Corrêa, L., and Orsini Machado de Sousa, S. C. (2010). Photodynamic therapy mediated by methylene blue dye in wound healing. Photomed. Laser Surg. 28, 581–587. doi: 10.1089/pHO.2009.2601
Spesia, M. B., Milanesio, A. E., and Durantini, E. N. (2008). Synthesis, properties and photodynamic inactivation of Escherichia coli by novel cationic fullerene C60 derivatives. Eur. J. Med. Chem. 43, 853–861. doi: 10.1016/j.ejmech.2007.06.014
Tan, O. L., Safii, S. H., and Razali, M. (2020). Commercial local pharmacotherapeutics and adjunctive agents for nonsurgical treatment of periodontitis: a contemporary review of clinical efficacies and challenges. Antib. Basel 9:11. doi: 10.3390/antibiotics9010011
Tan, O. L., Safii, S. H., and Razali, M. (2021). Clinical efficacy of repeated applications of local drug delivery and adjunctive agents in nonsurgical periodontal therapy: a systematic review. Antib. Basel 10:1178. doi: 10.3390/antibiotics10101178
Varela, M. F., Stephen, J., Lekshmi, M., Ojha, M., Wenzel, N., Sanford, L. M., et al. (2021). Bacterial resistance to antimicrobial agents. Antib. Basel 10:59. doi: 10.3390/antibiotics10050593
Vatansever, F., de Melo, W. C. M. A., Avci, P., Vecchio, D., Sadasivam, M., Gupta, A., et al. (2013). Antimicrobial strategies centered around reactive oxygen species - bactericidal antibiotics, photodynamic therapy, and beyond. FEMS Microbiol. Rev. 37, 955–989. doi: 10.1111/1574-6976.12026
Vileno, B., Marcoux, P. R., Lekka, M., Sienkiewicz, A., Feher, T., and Forro, L. (2006). Spectroscopic and photophysical properties of a highly derivatized C60 fullerol. Adv. Funct. Mater. 16, 120–128. doi: 10.1002/adfm.200500425
Wan, J., Ma, J. X., Zhang, Y. Y., Xia, Y. X., Hong, L., and Yang, C. (2021). Improved antioxidative performance of a water-soluble copper nanoparticle@fullerenol composite formed via photochemical reduction. New J. Chem. 45, 17660–17666. doi: 10.1039/d1nj03132e
Wang, M., Huang, L., Sharma, S. K., Jeon, S., Thota, S., Sperandio, F. F., et al. (2012). Synthesis and photodynamic effect of new highly photostable decacationically armed [60]- and [70]fullerene decaiodide monoadducts to target pathogenic bacteria and cancer cells. J. Med. Chem. 55, 4274–4285. doi: 10.1021/jm3000664
Wang, M., Maragani, S., Huang, L., Jeon, S., Canteenwala, T., Hamblin, M. R., et al. (2013). Synthesis of decacationic [60]fullerene decaiodides giving photoinduced production of superoxide radicals and effective pdt-mediation on antimicrobial photoinactivation. Eur. J. Med. Chem. 63, 170–184. doi: 10.1016/j.ejmech.2013.01.052
Wang, Z., Xu, F. J., and Yu, B. (2021). Smart polymeric delivery system for antitumor and antimicrobial photodynamic therapy. Front. Bioeng. Biotechnol. 9:783354. doi: 10.3389/fbioe.2021.783354
Wei, Y., Zhou, M., Zhou, Q., Zhou, X., Liu, S., Zhang, S., et al. (2017). Triplet-triplet annihilation upconversion kinetics of C60-BODIPY dyads as organic triplet photosensitizers. Phys. Chem. Chem. Phys. 19, 22049–22060. doi: 10.1039/c7cp03840b
Wozniak, A., Kruszewska, B., Pieranski, M. K., Rychlowski, M., and Grinholc, M. (2021). Antimicrobial photodynamic inactivation affects the antibiotic susceptibility of Enterococcus spp. clinical isolates in biofilm and planktonic cultures. Biomolecules 11:693. doi: 10.3390/biom11050693
Yamakoshi, Y., Umezawa, N., Ryu, A., Arakane, K., Miyata, N., Goda, Y., et al. (2003). Active oxygen species generated from photoexcited fullerene (C60) as potential medicines: O2-* versus 1O2. J. Am. Chem. Soc. 125, 12803–12809. doi: 10.1021/ja0355574
Yan, Y., Li, Y., Zhang, Z., Wang, X., Niu, Y., Zhang, S., et al. (2021). Advances of peptides for antibacterial applications. Colloid. Surface. B 202:111682. doi: 10.1016/j.colsurfb.2021.111682
Yang, X., Ebrahimi, A., Li, J., and Cui, Q. (2014). Fullerene-biomolecule conjugates and their biomedicinal applications. Int. J. Nanomed. 9, 77–92. doi: 10.2147/ijn.S52829
Yin, R., and Hamblin, M. R. (2015). Antimicrobial photosensitizers: drug discovery under the spotlight. Curr. Med. Chem. 22, 2159–2185. doi: 10.2174/0929867322666150319120134
Yin, R., Wang, M., Huang, Y.-Y., Landi, G., Vecchio, D., Chiang, L. Y., et al. (2015). Antimicrobial photodynamic inactivation with decacationic functionalized fullerenes: oxygen-independent photokilling in presence of azide and new mechanistic insights. Free Rad. Bio. Med. 79, 14–27. doi: 10.1016/j.freeradbiomed.2014.10.514
Zhang, C., Ge, Y., Lu, X., Chen, Z., Liu, J., Zhang, M., et al. (2021). Membrane perturbation of fullerene and graphene oxide distinguished by pore-forming peptide melittin. Carbon 180, 67–76. doi: 10.1016/j.carbon.2021.04.081
Zhang, M., Wu, Q., Lin, T., Guo, L., Ge, Y., Zeng, R., et al. (2020). Hematoporphyrin monomethyl ether photodynamic therapy for the treatment of facial port-wine stains resistant to pulsed dye laser. Photodiagnosis Photodyn. Ther. 31:101820. doi: 10.1016/j.pdpdt.2020.101820
Zhang, X., Cong, H., Yu, B., and Chen, Q. (2019). Recent advances of water-soluble fullerene derivatives in biomedical applications. Mini Rev. Org. Chem. 16, 92–99. doi: 10.2174/1570193x15666180712114405
Zhang, Y., Dai, T., Wang, M., Vecchio, D., Chiang, L. Y., and Hamblin, M. R. (2015). Potentiation of antimicrobial photodynamic inactivation mediated by a cationic fullerene by added iodide: in vitro and in vivo studies. Nanomedicine 10, 603–614. doi: 10.2217/nnm.14.131
Zhang, Y. K., Zhang, H., Zou, Q. L., Xing, R. R., Jiao, T. F., and Yan, X. H. (2018). An injectable dipeptide-fullerene supramolecular hydrogel for photodynamic antibacterial therapy. J. Mater. Chem. B 6, 7335–7342. doi: 10.1039/c8tb01487f
Keywords: antimicrobial photodynamic inactivation, photosensitizers, fullerene derivatives, antibacterial, multidrug resistance, functionalization
Citation: Hou W, Shi G, Wu S, Mo J, Shen L, Zhang X and Zhu Y (2022) Application of Fullerenes as Photosensitizers for Antimicrobial Photodynamic Inactivation: A Review. Front. Microbiol. 13:957698. doi: 10.3389/fmicb.2022.957698
Received: 31 May 2022; Accepted: 15 June 2022;
Published: 14 July 2022.
Edited by:
Danial Kahrizi, Razi University, IranCopyright © 2022 Hou, Shi, Wu, Mo, Shen, Zhang and Zhu. This is an open-access article distributed under the terms of the Creative Commons Attribution License (CC BY). The use, distribution or reproduction in other forums is permitted, provided the original author(s) and the copyright owner(s) are credited and that the original publication in this journal is cited, in accordance with accepted academic practice. No use, distribution or reproduction is permitted which does not comply with these terms.
*Correspondence: Yabin Zhu, emh1eWFiaW5AbmJ1LmVkdS5jbg==; Xiuqiang Zhang, emhhbmd4aXVxaWFuZzc1QDE2My5jb20=