- 1Department of Molecular and Cellular Biology, University of Guelph, Guelph, ON, Canada
- 2Guelph Research and Development Centre, Agriculture and Agri-Food Canada, Guelph, ON, Canada
Mycotoxins are toxic secondary metabolites produced by certain genera of fungi including but not limited to Fusarium, Aspergillus, and Penicillium. Their persistence in agricultural commodities poses a significant food safety issue owing to their carcinogenic, teratogenic, and immunosuppressive effects. Due to their inherent stability, mycotoxin levels in contaminated food often exceed the prescribed regulatory thresholds posing a risk to both humans and livestock. Although physical and chemical methods have been applied to remove mycotoxins, these approaches may reduce the nutrient quality and organoleptic properties of food. Microbial transformation of mycotoxins is a promising alternative for mycotoxin detoxification as it is more specific and environmentally friendly compared to physical/chemical methods. Here we review the biological detoxification of the major mycotoxins with a focus on microbial enzymes.
Introduction
Mycotoxins are secondary metabolites produced by several genera of filamentous fungi and are toxic to animals at low concentrations (Stoev, 2013). These toxins producing fungi typically infect crops, including cereals, nuts, oilseeds, and fruits (Table 1; Stoev, 2013). Since many of these crops are also used to feed farm animals, meat and animal products including milk and eggs can also become contaminated by mycotoxins (Zain, 2011). Ingestion of mycotoxin contaminated food poses a health safety risk for humans and livestock, causing mycotoxicosis. This can result in death and chronic effects that may lead to cancer or developmental defects. Besides their negative impact on human and animal health, mycotoxins are an economic burden to the agriculture industry due to reduced crop productivity and value, as well as the cost of regulatory programs to ensure that these toxins are not introduced into the food chain (Zain, 2011). Indeed, many countries have implemented legislation and guidelines to limit the levels of mycotoxins found in food and feed commodities. Nevertheless, it is estimated that mycotoxins can be detected in 60%–80% of food grains and up to 20% could be above regulatory limits (Eskola et al., 2020).
Strategies to mitigate mycotoxin contamination include the use of fungi-resistant crops and the application of pesticides, fungicides, or biological control methods to limit the growth and transmission of fungal pathogens (Cleveland et al., 2003). However, mycotoxin contamination frequently occurs post-harvest as the environmental conditions for the storage of agri-food commodities, such as grains, are often conducive to the growth of molds (Neme and Mohammed, 2017). Chemicals such as ammonia and ozone can react with mycotoxins to form less toxic products, but they leave chemical residues and can have negative effects on nutritional quality (Chelkowski et al., 1981; Conte et al., 2020). Physical methods for mycotoxin removal involve the use of washing, heat, radiation, or adsorbents, such as clay, activated carbon, or microbial cells (Stoev, 2013). In particular, the use of adsorbents that can bind to mycotoxins without dissociating in the digestive tract has been applied successfully in animal feed (Vila-Donat et al., 2018). Prophylactic use of calcium montmorillonite clay has also shown some promise in human clinical trials and may be useful for individuals at high risk of developing aflatoxicoses from consumption of contaminated food (Afriyie-Gyawu et al., 2008; Wang et al., 2008; Phillips et al., 2019). Several recent reviews on the use of microbial cells as adsorbents of mycotoxins are available (Vila-Donat et al., 2018; Xu et al., 2022) and therefore this topic is not covered in this review. Due to their rapid evolution and metabolic plasticity, microorganisms have the capability to degrade a large number of organic compounds and xenobiotics. Not surprisingly, both bacteria and fungi have been isolated from soil, the digestive tract of animals, or mycotoxin-contaminated products that are capable of transforming mycotoxins (Zhu et al., 2017). The toxicity of mycotoxins is often attributed to specific functional groups in their chemical structure. Therefore, selective modification of these functional groups by microbial enzymes could reduce or eliminate the mycotoxins toxicity (Figure 1). Microorganisms and their associated enzymes are therefore potentially useful for mycotoxin detoxification in food as they are specific and capable of catalyzing the biotransformation of mycotoxins under benign environmental conditions. Here, we provide a comprehensive review of the transformation of major mycotoxins by microbial enzymes and discuss their potential application for mycotoxin detoxification in food and feed. Many of the microbial enzymes involved in mycotoxin transformation require cofactors or co-substrates and we address their significance for the successful implementation of enzymatic strategies to remove mycotoxins in food/feed.
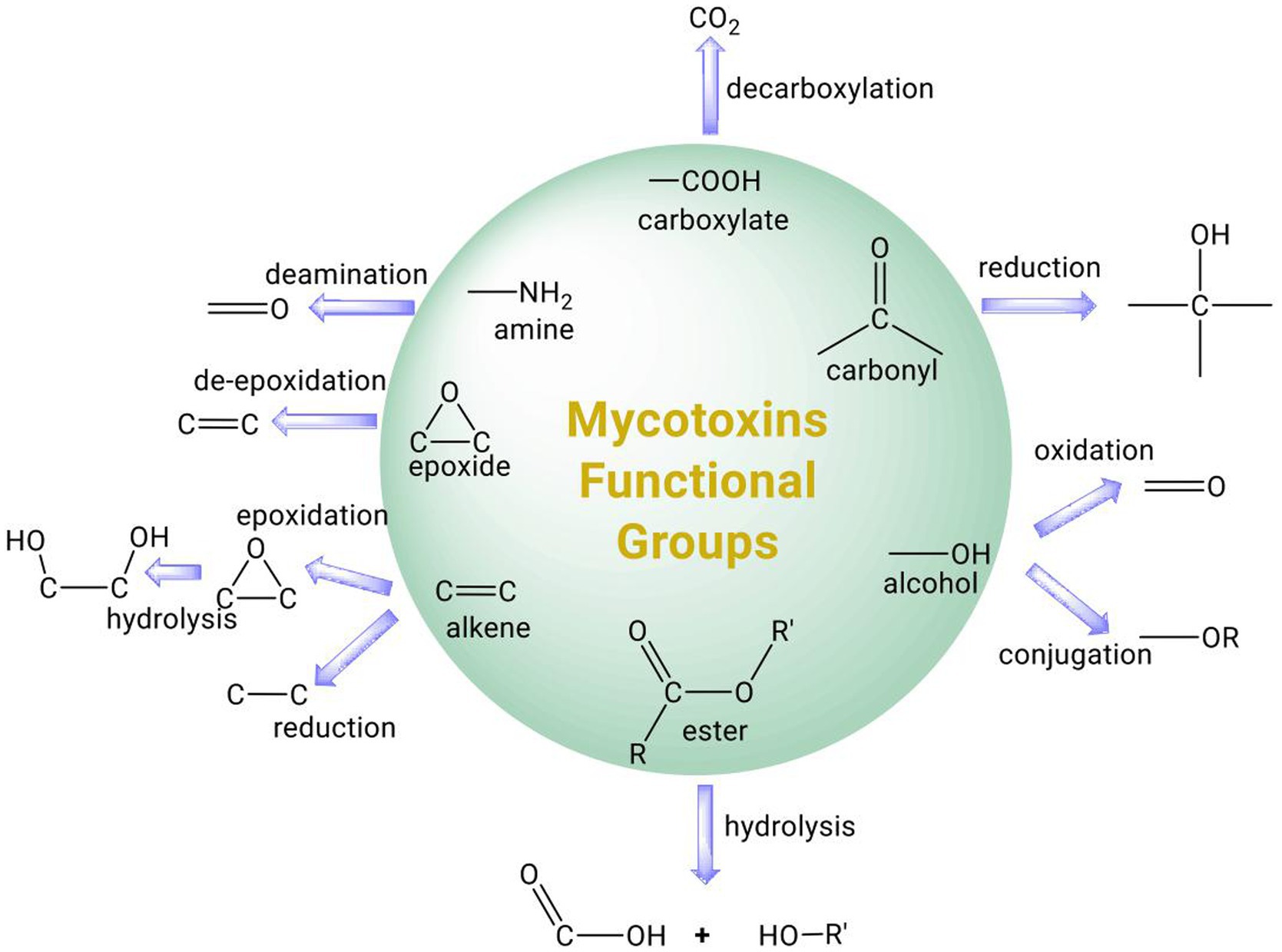
Figure 1. Summary of common functional groups of mycotoxins (circle) and their possible transformation by microbial enzymes.
Aflatoxins
Aflatoxins are difuranocoumarin mycotoxins that are produced by various species of Aspergillus that frequently associate with crops such as oilseed, corn, peanuts, etc. under warm and humid conditions (Mahato et al., 2019). Due to their fat solubility, these toxins can accumulate in animals exposed to contaminated feed, resulting in their occurrence in meat and other animal products (Pickova et al., 2021). Exposure to aflatoxins in animals can cause acute aflatoxicosis and death, while long-time exposure to low concentrations may cause cancer or lower performance and production of farm animals. The main aflatoxins of significance are aflatoxins B1, B2, G1, and G2, with aflatoxin B1 being the most toxic and the most potent liver carcinogen. AFB1 can be transformed by microsomal cytochrome P450 enzyme, inducing cellular oxidative stress and forming highly reactive AFB1-exo 8,9-epoxide that can form adducts with DNA and other cellular macromolecules, thereby contributing to its carcinogenic, teratogenic and mutagenic activity (Eaton and Gallagher, 1994; Figure 2A). In the liver, AFB1 and AFB2 are also metabolized by microsomal monooxygenases to the hydroxylated derivatives AFM1 and AFM2. AFM1 and AFM2 can however be secreted in milk (Prandini et al., 2009). Although less carcinogenic than their parent compounds when tested on rats (Cullen et al., 1987), the hydroxylated derivatives of the aflatoxins have similar toxicity as their parent compounds when tested on rats (Pong and Wogan, 1971) and ducks (Purchase, 1967).
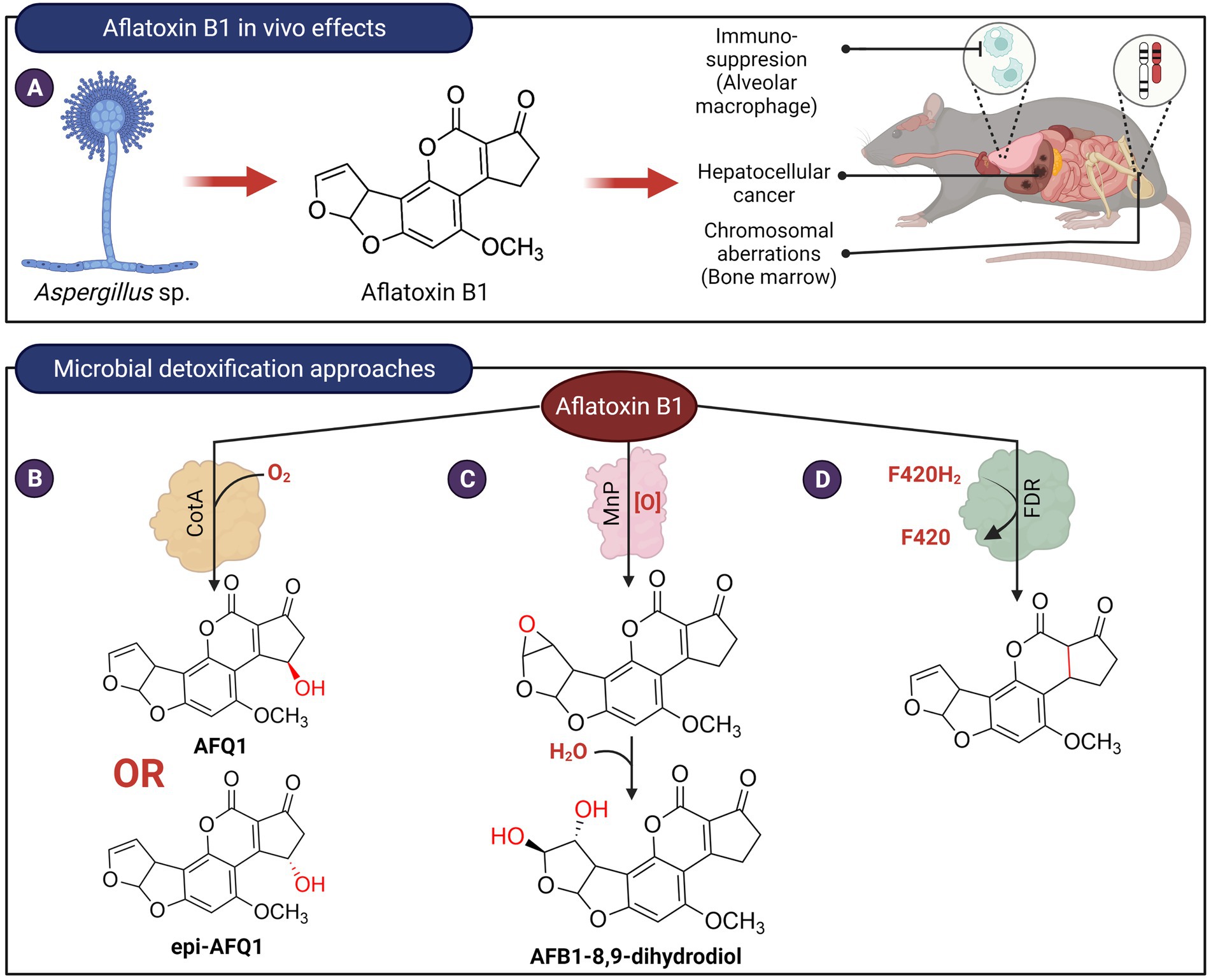
Figure 2. In vivo effects of aflatoxin B1 and microbial detoxification approaches. (A) Aflatoxin B1, produced by members of Aspergillus is carcinogenic, genotoxic, and possesses immunosuppressive properties. (B) Bacillus licheniformis ANSB821 utilizes the laccase, CotA to biotransform aflatoxin B1. (C) The manganese peroxidase MnP from the white-rot fungus Phanerochaete sordida YK-624 oxidizes AFB1 to form AFB1-8,9-dihydrodiol via an 8,9-epoxide intermediate. (D) Proposed reduction of AFB1 ring by a deazaflavin cofactor (F420) containing M. smegmatis enzyme.
Enzymes that depolymerize polyaromatic lignin, such as laccases and peroxidases, have been shown to oxidize aflatoxins. These enzymes frequently require additional co-substrates or mediators that may limit their practical use for the decontamination of aflatoxins in food. These redox mediators are the true substrate of laccases, and when oxidized, they form radicals that can in turn react with aflatoxins. For example, studies showed that laccase from Pleurotus eryngii was not capable of oxidizing AFB1 in the absence of redox mediators (Loi et al., 2016, 2018). Pleurotus pulmonarius laccase can transform 23% of AFB1 but the addition of 1 mM redox mediators, 2,2′-azino-bis-3-ethylbenzothiazoline-6-sulfonic acid, or acetosyringone resulted in an increased transformation of AFB1 to about 45% (Loi et al., 2016). The involvement of redox mediators in the laccase-catalyzed reactions indicates that they are not specific to aflatoxins and may potentially react with other molecules found in food. Recently, a bacterial laccase, CotA, from Bacillus licheniformis ANSB821 was found to transform AFB1 into two 3-hydroxy epimers of AFB1, AFQ1 and epi-AFQ1, without any redox mediators (Guo et al., 2020; Figure 2B). These two epimers do not exert any toxic effects on human hepatic (L-02) cells. AFQ1 toxicity was also shown to be about 1% of AFB1 in rainbow trout (Eaton and Gallagher, 1994) and 18-times less toxic than AFB1 in chicken embryo assay (Hsieh et al., 1974). However, the mechanism for direct oxidation of a non-phenolic moiety of AFB1 by CotA remains paradoxical and requires further investigation.
Treatment of AFB1 with manganese peroxidase MnP from the white-rot fungus Phanerochaete sordida YK-624 resulted in the formation of AFB1-8,9-dihydrodiol via an 8,9-epoxide intermediate (Wang et al., 2011; Figure 2C). The mutagenicity of AFB1 following treatment by this enzyme was reduced by about 50%–70%, suggesting that the highly reactive and toxic 8,9-epoxide did not accumulate in the reaction. Like laccases, the transformation of AFB1 by MnP is thought to be indirect. It was speculated that MnP produced formate or superoxide anion radicals that in turn react with AFB1. Transformation of AFB1 is also enhanced by the presence of Tween 80 and not Tween 20 (Bao et al., 1994). The former contains unsaturated fatty acids, suggesting that AFB1 oxidation by MnP can also be mediated by lipid peroxyl radicals. A recombinant N246A variant of the dye-decolorizing peroxidase, DypB (Rh_DypB) from Rhodococcus jostii (Vignali et al., 2018) was also able to transform AFB1. LC–MS–MS results suggested that it oxidizes ring A of AFB1 to form a product likely to be AFQ1 (Loi et al., 2020). However, DypB requires hydrogen peroxide as a co-substrate and may be undesirable for use in food.
An oxidase from the mushroom Armillariella tabescens was previously reported to oxidize AFB1 (Liu et al., 2001). This enzyme is not homologous to known aflatoxin oxidases, such as laccases or peroxidases. Instead, it shares sequence and structural homology with the hydrolase, dipeptidyl dipeptidase III (Xu et al., 2017). Subsequent investigation revealed that this enzyme had peptidase activity and lacked the previously ascribed aflatoxin oxidative activity (Karačić et al., 2017).
The lactone moieties of AFB1 are thought to be important for its toxicity as cleavage of the lactone ring in AFB1 resulted in the loss of fluorescence and its mutagenicity and toxicity are reduced by 450- and 18-times, respectively (Lee et al., 1981). Eleven Bacillus species from pond mud and soil samples were able to reduce the fluorescence of AFB1 (González Pereyra et al., 2019). Three of the strains, B. subtilis RC1B, B. cereus RC1C, and B. mojavensis RC3B contained genes that are homologous to aiiA, a lactonase that can hydrolyze the lactone ring of the Gram-negative bacteria quorum sensing autoinducer, acyl-homoserine lactone. However, hydrolysis of the AFB1 lactone ring has not been demonstrated with purified AiiA. In addition, not all the Bacillus strains tested contained the aiiA gene, suggesting that other enzymes may be responsible for the observed hydrolysis of AFB1.
Lastly, nine F(420)-dependent reductases (FDR) from Mycobacterium smegmatis that utilize the deazaflavin cofactor F420H2 were found to catalyze the transformation of AFG1, AFG2, AFB1, and AFB2, with AFG1 being the best substrate for all nine enzymes (Taylor et al., 2010). The products as determined from LC–MS have an increased m/z of 2.02 indicative of the reduction of aflatoxin by 2 electron transfers from the F420H2 cofactor of the enzymes. It is suggested that the α,β unsaturated ring A of the aflatoxins was reduced by the enzyme (Figure 2D). However, the toxicity of the ring A saturated products in comparison to AFB1 has not been determined. In addition, this enzyme will require reducing agents to recycle the oxidized deazaflavin cofactor, which may not be practical for the decontamination of aflatoxins in food products.
Zearalenone
Zearalenone (ZEN) is a α,β-resorcylic acid lactone, and its toxicity is attributed to its estrogenic properties (Metzler et al., 2010; Figure 3A). Various microorganisms, including gut microbes, were able to reduce the C6 keto group of zearalenone to produce α-zearalenol (α-ZEL) and β-zearalenol (β-ZEL; Figure 3B; El-Sharkawy and Abul-Hajj, 1988; El-Sharkawy et al., 1991). While β-ZEL is less toxic than ZEN, α-ZEL binds to estrogen receptors 10–20 times stronger and exhibits >90-fold higher estrogenic activity, compared to ZEN (Metzler et al., 2010). The enzyme(s) responsible for the reduction of ZEN has not been identified and therefore it is not clear if the two activities can be separated.
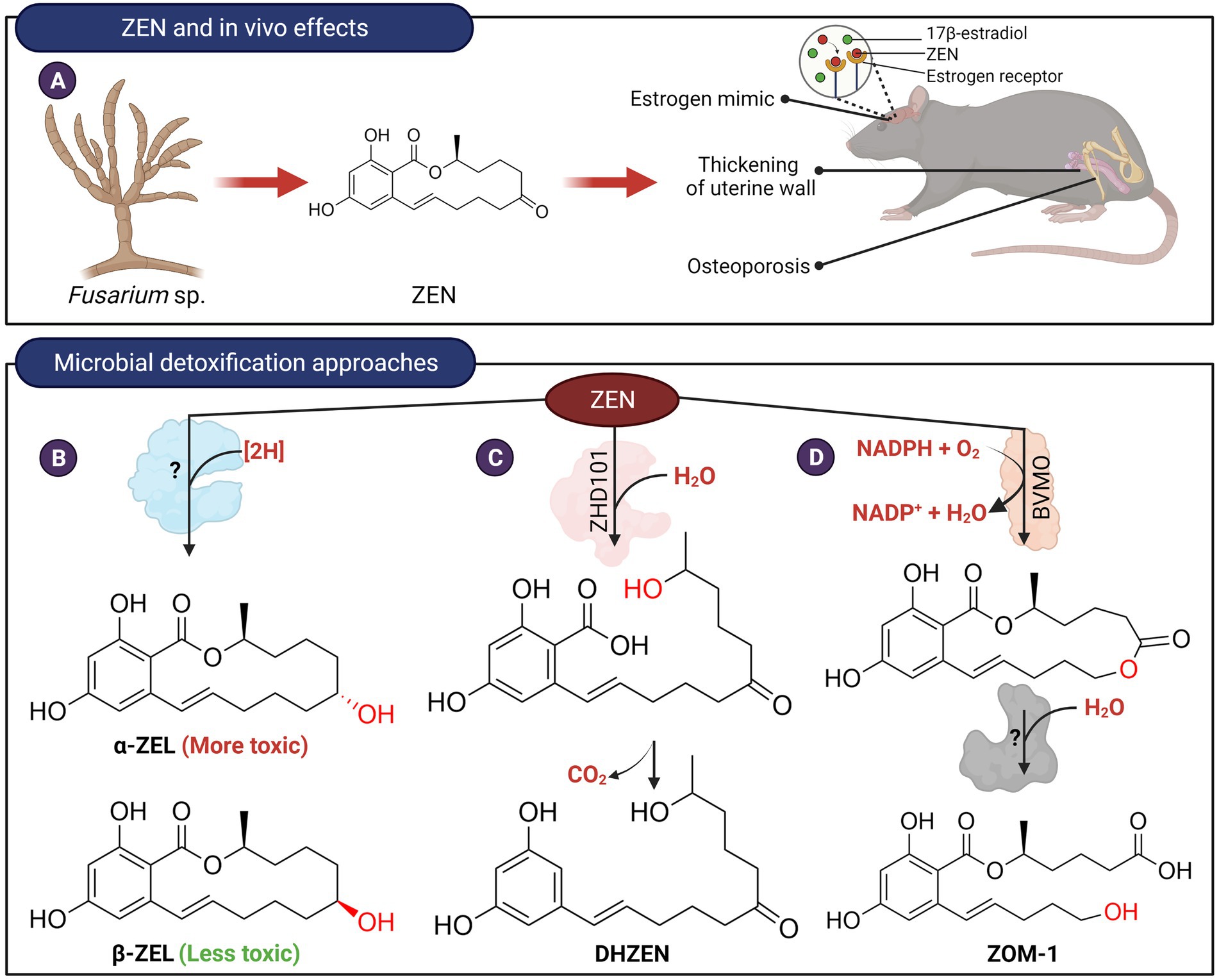
Figure 3. In vivo effects of ZEN and microbial detoxification approaches. (A) ZEN possesses estrogenic properties which in turn affect the endocrine and reproductive systems of animals. (B) Transformation of ZEN to α-ZEL or β-ZEL by microorganisms. The C7-carbonyl oxygen is reduced to an alcohol stereospecifically (red). The enzyme(s) responsible for catalyzing this reaction have not been isolated and identified. (C) ZEN can be hydrolyzed by lactone hydrolases followed by spontaneous decarboxylation to produce DHZEN. (D) Transformation of ZEN to ZOM-1 was postulated to occur in two steps. The first step could potentially be catalyzed by a Baeyer-Villiger type monooxygenase. The lactone product can then be hydrolyzed to ZOM-1.
Detoxification of ZEN can also occur through modification of the C14-hydroxyl group or hydrolysis of the lactone ring as these moieties are important for the estrogenic property of ZEN (El-Sharkawy and Abul-Hajj, 1988). For example, ZEN can be conjugated with glucoside and sulfate at the C14-hydroxyl groups by plants and various microbial species (Kamimura, 1986; El-sharkawy and Abul-hajj, 1987; El-Sharkawy et al., 1991; Plasencia and Mirocha, 1991; Jard et al., 2010; Brodehl et al., 2014; Paris et al., 2014). Although these esters are not estrogenic, they can be hydrolyzed to the parent compound by gut microbiota and are therefore not considered a viable method to reduce the toxicity of ZEN (Dall’Erta et al., 2013; Paris et al., 2014). More recently, several strains of Bacillus have been found capable of transforming ZEN to ZEN-14-phosphate (Zhu et al., 2021). Such a microbial transformation system may provide a new possibility for ZEN detoxification although the stability and toxicity reduction of ZEN-14-phosphate need to be further demonstrated in animal trials.
Clonostachys rosea (synonym: Gliocladium roseum) IFO 7063, was found to transform ZEN into a non-estrogenic product, 1-(3,5-dihydroxyphenyl)-10′-hydroxy-1′-undecen-6′-one (named DHZEN), through cleavage of the lactone ring followed by spontaneous decarboxylation (Figure 3C; Kakeya et al., 2002). The enzyme responsible for this activity, named ZHD101, was isolated and found to adopt an α/β-hydrolase fold with a typical catalytic triad of Ser-His and Asp found in many hydrolytic enzymes (Takahashi-Ando et al., 2002). Homologs of ZHD101 capable of hydrolyzing the lactone ring of ZEN were also found in other microbes, including Cladophialophora bantiana (Hui et al., 2017) and Rhinocladiella mackenzieican (Zheng et al., 2018). Another ZEN lactonase enzyme from Rhodococcus erythropolis was marketed as a feed additive by Biomin, Austria (Gruber-Dorninger et al., 2021). Recombinant Saccharomyces cerevisiae, Escherichia coli or Lactobacillus reuteri have been constructed that expressed these lactone hydrolases and found to hydrolyze ZEN to various extents (Takahashi-Ando et al., 2004; Yang et al., 2017; Liu et al., 2019). In particular, the genetically engineered L. reuteri, which expresses the lactonohydrolase, could potentially be used as a probiotic feed additive for the degradation of ZEN.
Trichosporon mycotoxinivorans, a basidiomycete yeast, was also found to cleave the lactone undecyl ring system at the ketone group at C7, leading to the formation of 5-({2,4-dihydroxy-6-[(1E)-5-hydroxypent-1-en-1-yl]benzoyl}oxy)hexanoic acid (named ZOM-1; Figure 3D; Vekiru et al., 2010). The transformation of ZEN to ZOM-1 was postulated to occur in two steps. First, a Baeyer-Villiger oxidation adds oxygen to the macrocyclic ring followed by hydrolysis of the resultant lactone. ZOM-1 was established to be non-estrogenic but the enzymes responsible for its formation from ZEN have not been identified.
Citrinin
Citrinin is a mycotoxin produced by fungi from the genus Penicillium, Aspergillus, and Monascus that mainly contaminate grains. The toxicity of citrinin is attributed mainly to its ability to induce oxidative stress (Vanacloig-Pedros et al., 2016) as well as its nephrotoxic properties (Shinohara et al., 1976; Figure 4A). A marine bacterial strain, Moraxella sp. MB1, is capable of transforming citrinin to decarboxycitrinin (Figure 4B; Devi et al., 2006). Decarboxycitrinin is not toxic when tested on mice (Jackson and Ciegler, 1978). The decarboxylating activity was observed in cell extract but not cell-free culture supernatant, suggesting that the unidentified enzyme responsible for this transformation is intracellular (Devi et al., 2006). Incidentally, decarboxycitrinin is a natural metabolite of the citrinin-producing fungus Penicillium citrinum and could also be produced by heat treatment of citrinin (Curtis et al., 1968; Jackson and Ciegler, 1978). Other microorganisms, such as Rhizobium borbori and Klebsiella pneumoniae NPUST-B11 have been reported to transform citrinin but the products have not been identified (Chen et al., 2011; Kanpiengjai et al., 2016).
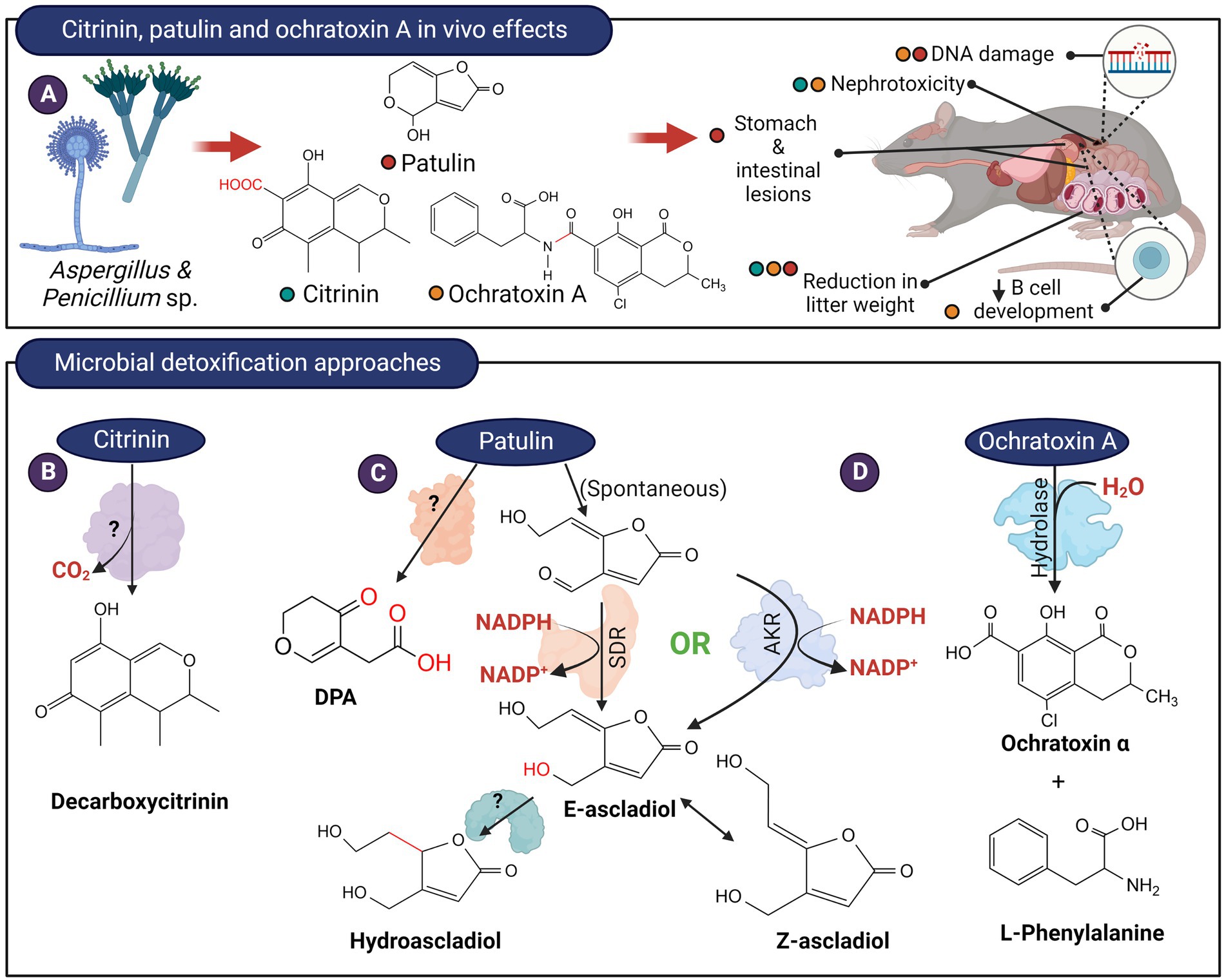
Figure 4. In vivo effects of citrinin, patulin, ochratoxin and microbial detoxification approaches. (A) These mycotoxins are produced by members of Aspergillus and Penicillium and typically, citrinin and ochratoxin possess nephrotoxic effects while patulin exerts serious gastrointestinal effects. (B) Decarboxylation of citrinin to decarboxycitrinin. (C) The hemiacetal ring of patulin likely exist in equilibrium with the ring-open form. The aldehyde in the ring-opened form of patulin can be reduced by enzymes from the short-chain dehydrogenase (SDR) or aldo-keto reductase (AKR) family to (E)-ascladiol. The E-ascladiol can be converted to the (Z)-isomer catalyzed by cellular sulfhydryl compounds, such as cysteine. Ascladiol can potentially be reduced to hydroascladiol by some microorganisms, such as Lactobacillus plantanum. Other basidiomycete yeasts can also transform patulin to DPA, although the formation of E-ascladiol appears to be more ubiquitous among various yeast and bacterial species. (D) The amide bond of ochratoxin A can be hydrolyzed to from L-phenylalanine and ochratoxin α.
Patulin
Patulin is a bicyclic polyketide produced primarily by Penicillium expansum but can also be produced by other species of Penicillium as well as fungi belonging to the Aspergillus and Byssochlamys genus (Wright, 2015). The electrophilic α,β-unsaturated lactone present in patulin is reactive toward electron-rich sulfhydryl groups such as cysteine and glutathione, thereby affecting protein functions and increasing oxidative stress (Fliege and Metzler, 2000). The genotoxicity of patulin can also be attributed to the α,β-unsaturated lactone that induces double-stranded breaks in DNA strands (Pfenning et al., 2016). Short-term rat feeding studies also indicated that patulin directly affects the gastrointestinal tract as well as the kidneys (Speijers et al., 1988; Figure 4A). Patulin is largely found in apples and apple-based products but it can also contaminate other fruit products such as juices and jams as well as grain products and cheese (Pattono et al., 2013; Wright, 2015).
When incubated with patulin, certain microorganisms including Saccharomyces cerevisiae (Moss and Long, 2002), Kodameae ohmeri (Dong et al., 2015), Gluconobacter oxydans (Ricelli et al., 2007), and Sporobolomyces accumulate E-and/or Z-ascladiol (Ianiri et al., 2013). The reaction likely proceeds by the opening of the hemiacetal ring, followed by the reduction of the aldehyde group to alcohol (Figure 4C). It is thought that the Z-isomer of ascladiol is produced from E-ascladiol and was catalyzed by cellular sulfhydryl compounds such as glutathione and cysteine (Sekiguchi et al., 1983). An enzyme from the short-chain dehydrogenase (SDR) family has been isolated from Candida guilliermondii that catalyzed the transformation of patulin to E-ascladiol in an NADPH-dependent manner (Chen et al., 2017; Xing et al., 2021). The rate of patulin transformation by this enzyme appeared to be low as the enzyme at 150 μg/ml concentration took 72 h to fully transform 40 μg/ml of patulin. Enzymes related to the SDR family that can reduce patulin to E-ascladiol also appeared to be present in Gluconobacter oxydans (Ricelli et al., 2007; Lyagin and Efremenko, 2019). Chan et al., (2022) isolated 4 enzymes in G. oxydans that are capable of reducing patulin to E-ascladiol in an NADPH-dependent manner. Two of the enzymes (GOX0525 and GOX1899), belonging to the SDR family, was purified from recombinant E. coli and showed superior activity compared to the enzyme from C. guilliermondii. Recently, an aldo-keto reductase, originally isolated from Devosia mutans for the detoxification of the trichothecene, deoxynivalenol (DON; Carere et al., 2018b), was also found to be capable of reducing patulin to E-ascladiol (Abraham et al., 2022; Figure 4C). The catalytic efficiency of this enzyme, DepB, is 80-and 4-times lower compared to the SDRs GOX0525 and GOX1899 from G. oxydans. DepB can however utilize the less expensive NADH as a coenzyme, albeit less efficiently than NADPH.
Patulin can also be transformed to hydroascladiol (Figure 4C) by Lactobacillus plantanum (Hawar et al., 2013). Presumably, the ascladiol produced can be further reduced to hydroascladiol by certain microorganisms. Toxicity studies on hydroascladiol have not been reported.
In a transcriptomic study of Pichia caribbica, several genes, including PcCRG1, have been noted to be upregulated when incubated with patulin (Wang et al., 2019). PcCRG1 codes for a methyltransferase that is dependent on the presence of S-adenosylmethione (S-Met) to transform patulin in vitro (Wang et al., 2019). Due to the necessity of S-Met, a methylated patulin was suggested to be the product of the reaction catalyzed by the enzyme (Wang et al., 2019). A knockout mutant of the PcCRG1 gene had significantly reduced patulin transformation activity. Conversely, overexpression of the gene resulted in increased transformation activity (Wang et al., 2019). Interestingly, P. caribbica have been found to transform patulin into ascladiol in vivo, and not into a methylated patulin as expected from a PcCRG1 catalyzed reaction (Wang et al., 2019). This suggests that other enzymes are present in the fungus that can reduce patulin into ascladiol, but to date, an enzyme with this activity has not been isolated from the bacteria.
An orotate phophoribosyltransferase enzyme was isolated from Rhodotorula mucilaginosa and its patulin degrading activity was evaluated in apple juice (Tang et al., 2019). The degradation product from this enzyme has yet to be determined but it has been hypothesized to be a phosphoribosyl modified patulin, a metabolite of an unknown toxicity profile (Lyagin and Efremenko, 2019). However, an in vivo study on R. mucilaginosa has shown that ascladiol is the main byproduct of patulin degradation that suggests the activity of a reductase than a transferase (Yang et al., 2021).
Desoxypatulinic acid (DPA), is a less toxic metabolite of patulin that is produced by Rhodosporidium kratochvilovae (Castoria et al., 2011), Rhodosporidium paludigenum (Zhu et al., 2015), and Sporobolomyces roseus strain IAM 13481 (Ianiri et al., 2013). DPA appeared to be a metabolite of the hydrolyzed 5-membered lactone ring of patulin. The enzyme(s) responsible for transforming patulin to DPA has not been identified (Figure 4C).
Ochratoxins
Ochratoxins, originally named from the producing strain Aspergillus ochraceus, are isocoumarin mycotoxin derivatives. They are also produced by species of Aspergillus and Penicillium and exist in three isoforms, ochratoxin A, B, and C. Of the 3 isoforms, ochratoxin A is the most potent, having nephrotoxic, immunosuppressive, teratogenic, and carcinogenic properties (Figure 4A).
The amide bond of ochratoxin A can be hydrolyzed to form phenylalanine and ochratoxin α, both of which are non-toxic (Figure 4D). The first enzyme discovered that can catalyze this reaction was bovine carboxypeptidase A, an enzyme with specificity toward peptides containing a terminal aromatic amino acid (Pitout, 1969). Subsequently, Saccharomyces cerevisiae carboxypeptidase Y was found to hydrolyze ochratoxin, albeit at a much lower rate compared to bovine carboxypeptidase A (Abrunhosa et al., 2010). Commercially available hydrolase preparations were then screened and one preparation from Aspergillus niger, marketed as Amano A lipase, was able to hydrolyze ochratoxin (Stander et al., 2000). Subsequent studies revealed that Amano A contained a mixture of enzymes, and the active enzyme against ochratoxin A is an amidase belonging to the amidohydrolase family of enzymes (Dobritzsch et al., 2014).
Fumonisins
Fumonisins are aliphatic diesters that inhibit the enzyme ceramide synthase, thereby preventing sphingolipid biosynthesis. Currently, 28 fumonisin analogs have been identified differing in the substituents on the main chain (Alberts et al., 2016). Fumonisin B1 (FB1) is the most prevalent and toxic fumonisin. It is hepatoxic, nephrotoxic, and is a possible carcinogen (Figure 5A). The two tricarballylic acid moieties are essential for toxicity and they can be removed by hydrolysis of the ester bonds catalyzed by carboxylesterases present in bacteria and fungi. Of note is the carboxylesterase from the fumonisin-degrading bacterium Sphingopyxis macrogoltabida (Heinl et al., 2011) that has been commercialized as a feed additive by the company Biomin (Austria; Figure 5B). Although hydrolyzed FB1 (HFB1) is not toxic, the C2 amino group can be acylated by fatty acids in vivo to generate a ceramide synthase inhibitor that is 10-times more toxic than FB1 in mammalian HT-29 cells (Seiferlein et al., 2007). To prevent the acylation of the hydrolyzed FB1, the C2-amino group can be further deaminated. The bacterium, Sphingopyxis sp. MTA144, utilizes a pyridoxal phosphate-dependent amino transferase, with pyruvate as an amino acceptor to catalyze the deamination of HFB1 to produce 2-keto-HFB1 (Heinl et al., 2011; Figure 5C). The yeast, Exophiala spinifera, was also able to deaminate HFB1, and the enzyme that catalyzed the reaction was speculated to be an amine oxidase (Blackwell et al., 1999; Figure 5D). While amine oxidase requires oxygen for activity, it does not require a keto acid as a co-substrate, unlike aminotransferases.
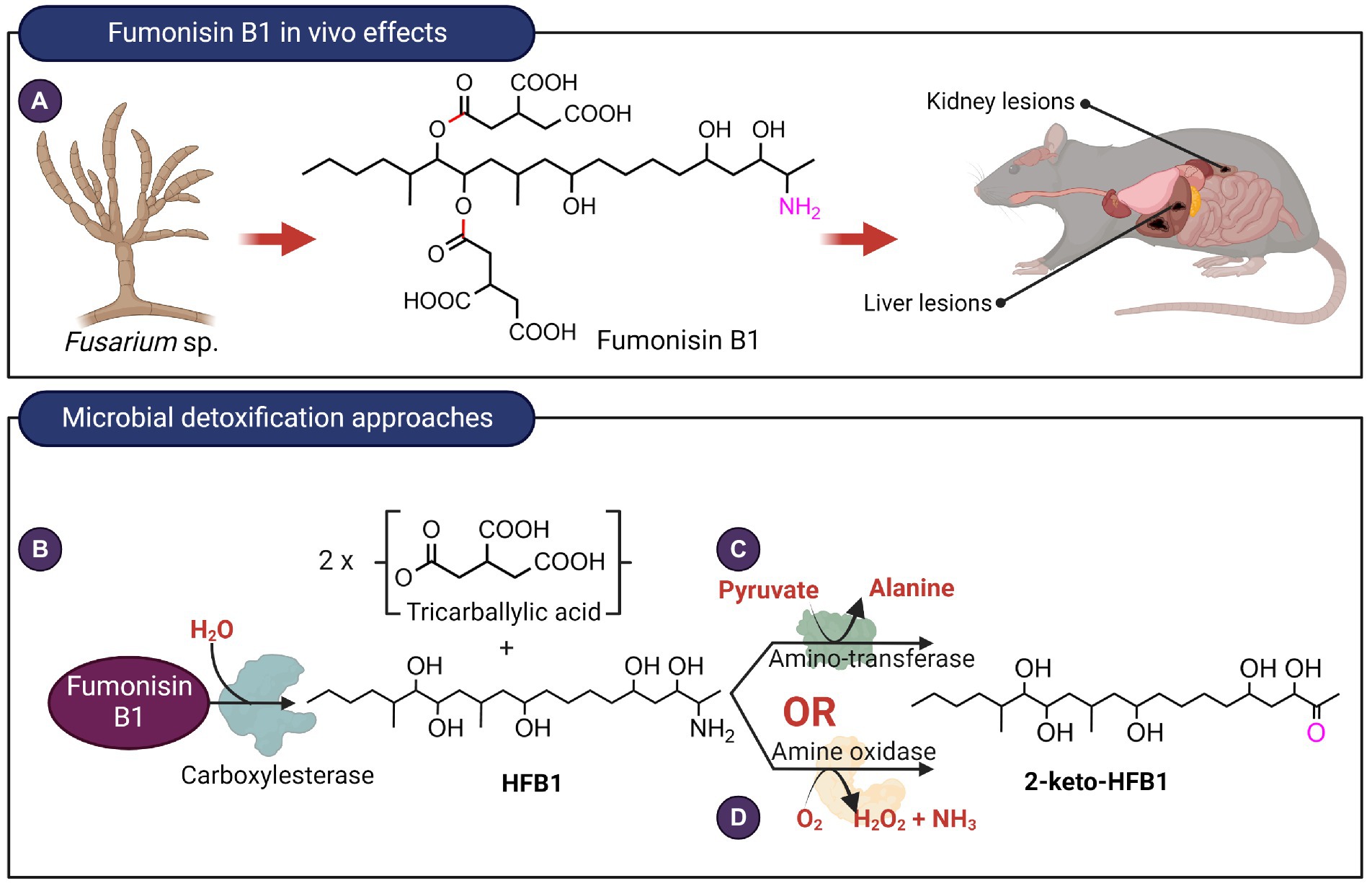
Figure 5. In vivo effects of fumonisin B1 and microbial detoxification approaches. (A) Fumonisin B1, typically produced by Fusarium species exert toxic effects on the liver as well as kidneys. (B) Hydrolysis of fumonisin B1 catalyzed by carboxyesterases target the ester linkages highlighted in red. Deamination reaction catalyzed by (C) aminotransferase or (D) an amine oxidase with the targeted C2 amino group highlighted in fuschia. The aminotransferase and amine oxidase from Sphingopyxis sp. MTA144 and Exophiala spinifera utilize hydrolyzed fumonisin B1 as substrate.
Interestingly, certain fumonisin-producing Aspergillus niger accumulated deaminated fumonisin B1 in culture supernatant. The enzyme responsible for this reaction is later identified to be a monoamine oxidase and was found to also deaminate fumonisin derivatives, FB2 and FB3, with a preference for FB2 (Garnham et al., 2020). Although this enzyme-catalyzed a similar deamination reaction as the amine oxidase from E. spinifera discussed earlier, the two enzymes differed in two aspects. Firstly, A. niger monoamine oxidase deaminates fumonisin while the E. spinifera enzyme only deaminates HFB1. Secondly, A niger monoamine oxidase utilized FADH2 as a cofactor while amine oxidase typically requires topaquinone as a cofactor (Klinman, 2003).
Trichothecenes
Trichothecenes are tetracyclic sesquiterpenoid secondary metabolites primarily produced by members of the Fusarium, Myrothecium, Stachybotrys, and Trichoderma genera (Desjardins et al., 1993; Wilkins et al., 2003). The structural backbone is a fused tricyclic ring system comprising a cyclohexene ring (A-ring), a tetrahydropyran ring (B-ring), and a cyclopentyl ring (C-ring). An epoxide ring is attached to the tetrahydropyran ring and overall, this core structure is referred to as the 12,13-epoxytrichothec-9-ene (EPT) nucleus. Various substituent groups decorating the EPT nucleus are key to modulating the toxicity of trichothecenes and based on these substitution patterns, trichothecenes can be sub-classified into four groups designated Type A–D. Type A trichothecenes are distinguished from Type B which have a ketone group present on C8 of the EPT nucleus. Type C possesses an epoxide ring at C8 while Type D trichothecenes possess a di-or tri-ester linkage spanning the C4 and C15 regions (Shank et al., 2011; Figure 6).
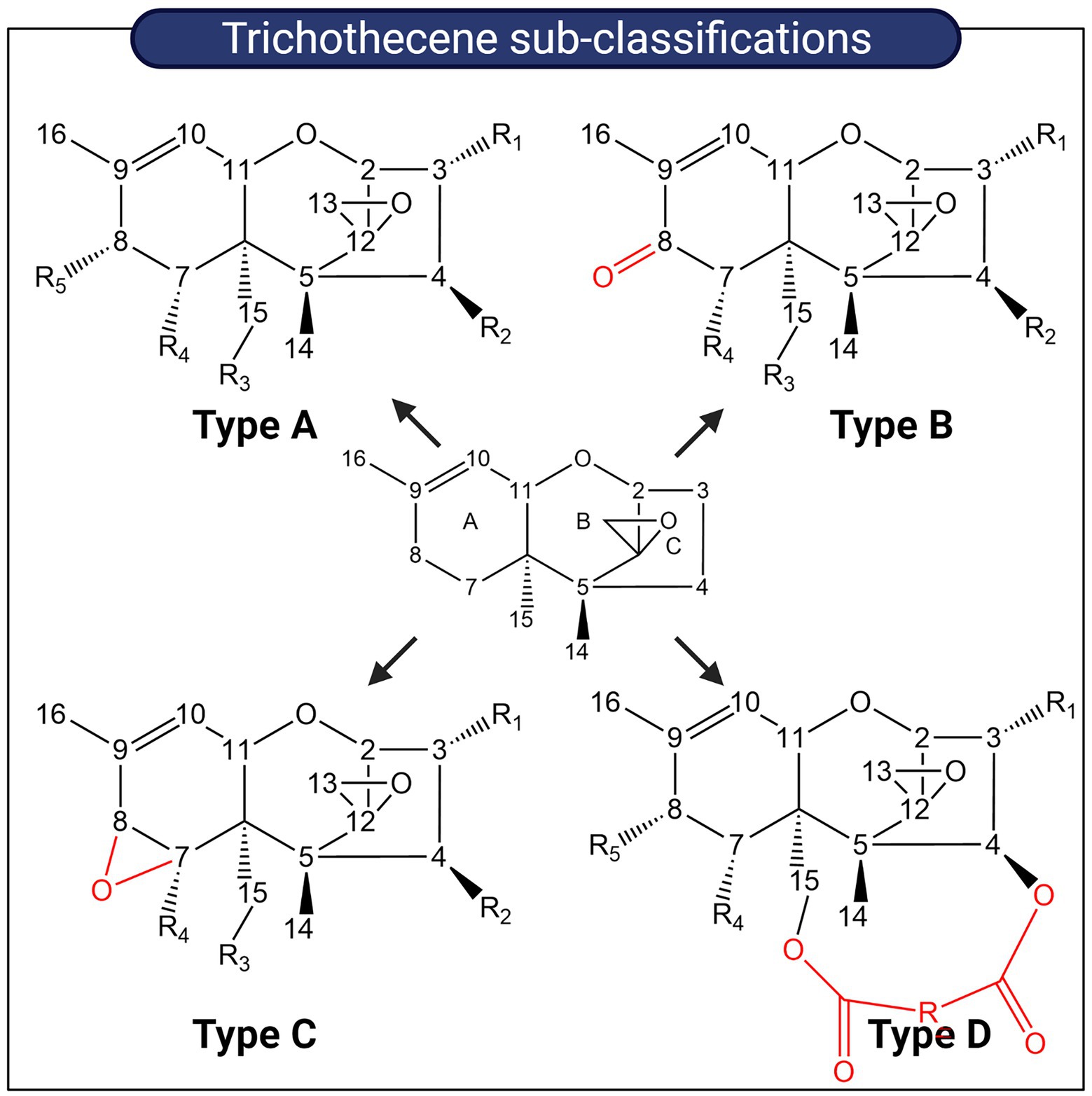
Figure 6. Sub-classification of trichothecenes. Trichothecenes can be sub-classified into four groups based on substitution patterns around the EPT nucleus. Key functional groups which distinguish each group from one another are highlighted in red.
Trichothecenes are small molecular inhibitors of protein synthesis and trigger ribotoxic stress by binding to the peptidyl transferase site of the large 60S subunit of eukaryotic ribosomes (Garreau De Loubresse et al., 2014). These interactions are mediated by the 12,13-epoxide ring, and for Type A trichothecenes such as T-2 toxin and Type B trichothecenes such as DON, the C3-hydroxyl is another contributing factor (Wang et al., 2021). Type A (T-2, HT-2, neosolaniol, diacetoxyscirpenol (DAS), monoacetoxyscirpenol (MAS), verrucarol (VER), scirpentriol (SCP)) and Type B trichothecenes (Deoxynivalenol (DON), nivalenol (NIV) and their acetylated derivatives) are the most prevalent chemotypes contaminating cereal grain crops (Foroud and Eudes, 2009). Ingestion of contaminated grain triggers gastrointestinal ailments in livestock and symptoms include diarrhea, emesis, feed refusal, and subsequent weight loss (Eriksen and Pettersson, 2004; Figures 7A, 8A). Limited studies exist for the detoxification of type C and D trichothecenes; instead, more research has been devoted to type A and B trichothecenes due to their significant agroeconomic impact. Microbial biotransformation of trichothecenes can occur through a variety of mechanisms including de-epoxidation, oxidation, epimerization, hydrolysis, acetylation, hydroxylation, and glycosylation.
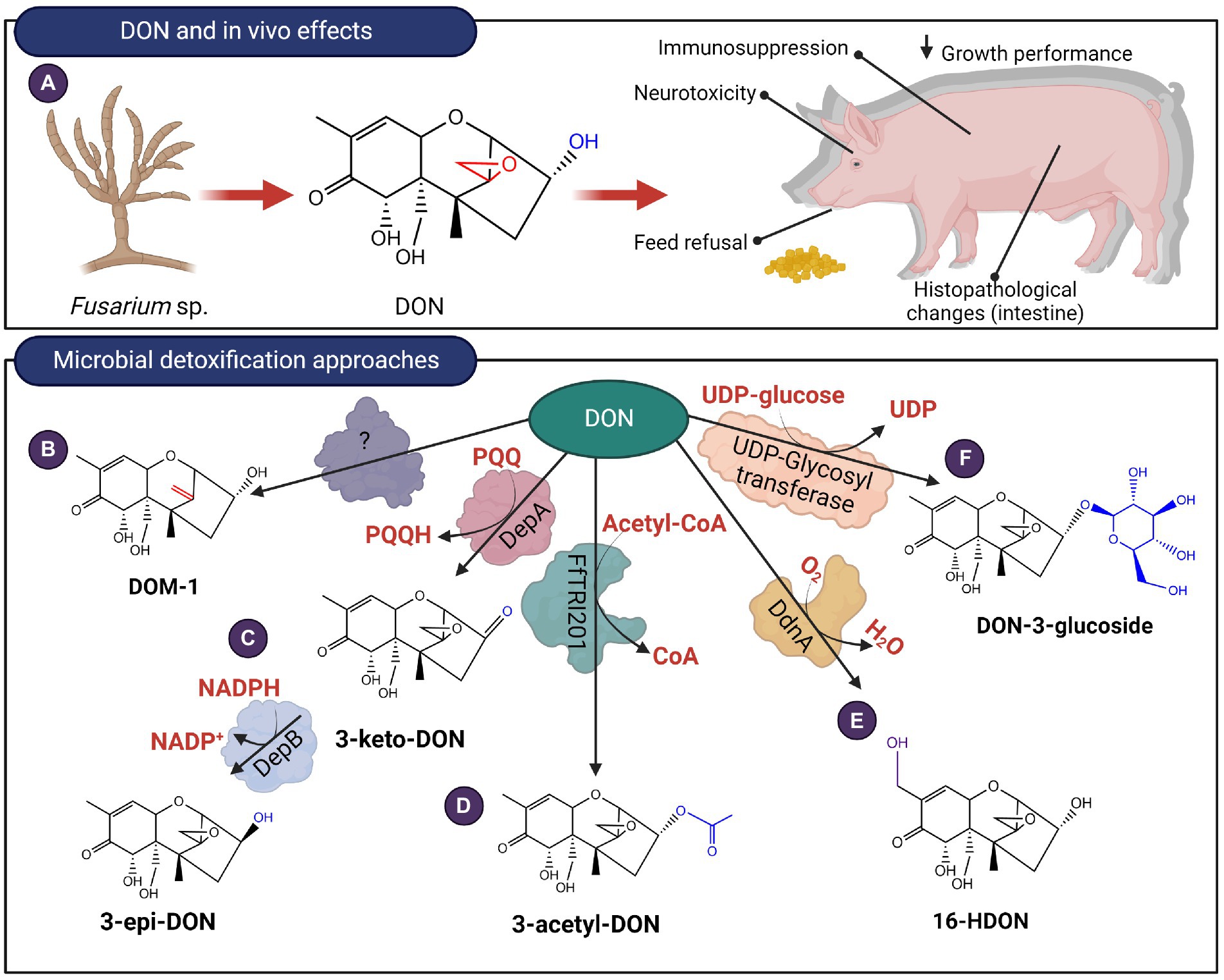
Figure 7. In vivo effects of Type B trichothecenes like DON and microbial detoxification approaches. (A) DON affects the gastrointestinal system and at a molecular level, the mycotoxin can bind to ribosomes triggering ribotoxic stress. (B–F) DON can be transformed by de-epoxidation, oxidation, epimerization, acetylation, hydroxylation and glycosylation.
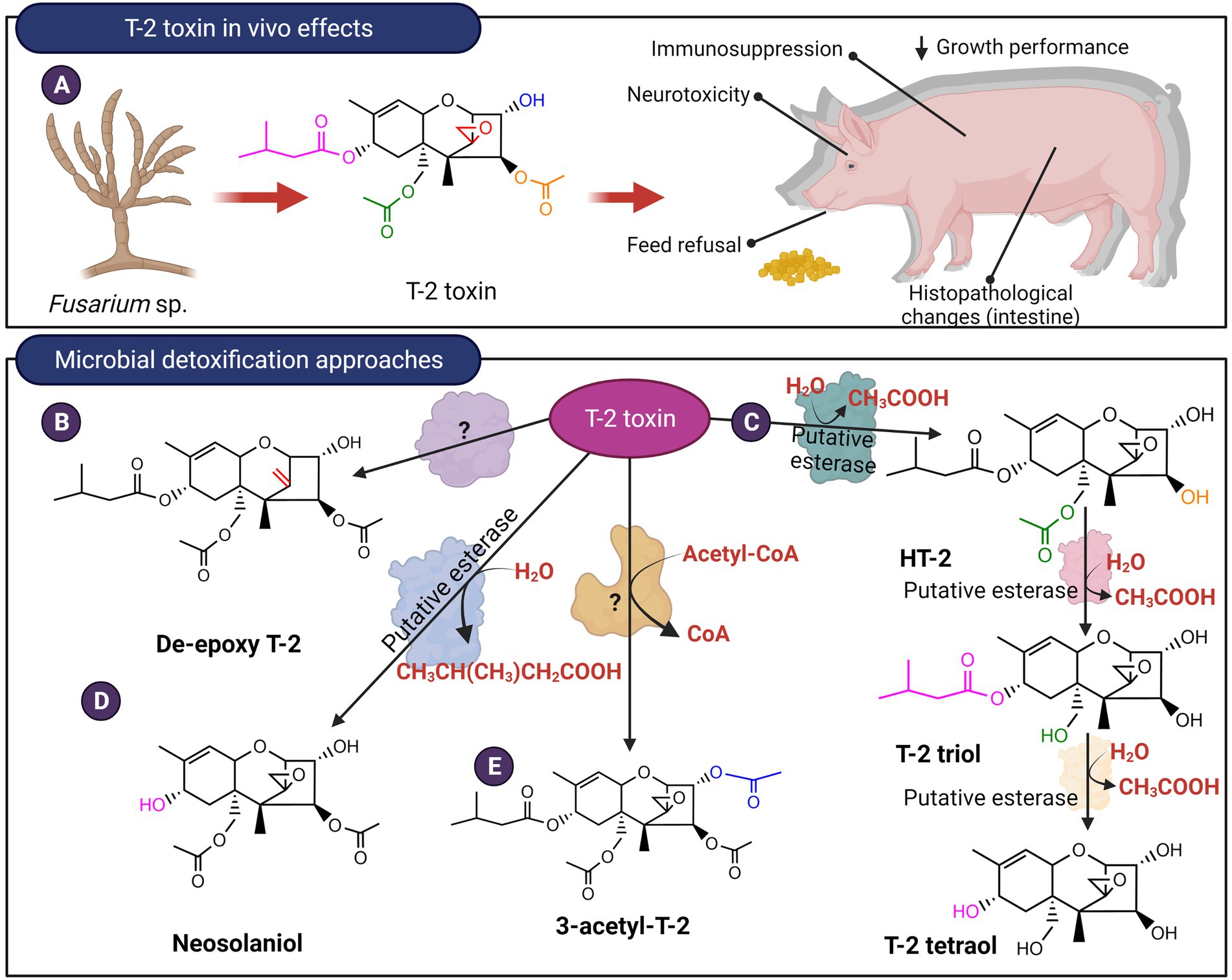
Figure 8. In vivo effects of Type A trichothecenes like T-2 toxin and microbial detoxification approaches. (A) T-2, like DON, causes negative gastrointestinal effects and ribotoxic stress. (B–E) The mycotoxin can be detoxified by de-epoxidation, hydrolysis of the ester linkages or acetylation.
De-epoxidation of trichothecenes has been primarily reported for anaerobic bacteria present in the gastrointestinal tract of ruminants and poultry or from soil environments. Eubacterium BBSH 797 commercialized as Biomin BBSH® 797 is a gram-positive, strict anaerobe isolated from ruminal fluid. This strain is proposed to conduct reductive de-epoxidation of the C12,13 epoxide ring of Type A and Type B trichothecenes such as T-2 and DON (Fuchs et al., 2000, 2002; Figures 7B, 8B). Since then, other microbial strains have been identified including Bacillus sp. LS100 (Yu et al., 2010), a consortium isolated from agricultural soil (Islam et al., 2012), a microbial consortium termed DX100 (Ahad et al., 2017), Eggerthella sp. DII-9 (Gao et al., 2018), Desulfitobacterium sp. strain PGC-3-9 (He et al., 2020), and Slackia sp. D-G6 (Gao et al., 2020). The reduced toxicity of de-epoxy derivatives of T-2 and DAS was demonstrated using a brine shrimp toxicity bioassay that showed higher LC50 values (Swanson et al., 1987). Likewise, cytotoxicity assays of de-epoxy derivatives of DON (named DOM-1) and NIV showed IC50 values that were 52 and 51 times higher than the parent mycotoxins, respectively (Eriksen and Pettersson, 2004). To date, the isolation of enzymes responsible for the reductive de-epoxidation of trichothecenes has remained elusive. Comparative genomics of Slackia sp. with closely related strains that showed no DON de-epoxidation identified 13 possible gene clusters that may be responsible for de-epoxidase activity (Gao et al., 2020). Five of these clusters were recombinantly expressed but no in vitro trichothecene de-epoxidation activity was observed. The recombinant strain used was not indicated and it is unclear if the lack of activity is due to poor heterologous expression of the genes.
The C3 OH of DON can be epimerized from the R configuration to the S configuration (Hassan et al., 2017). The resulting diastereomer, 3-epi-DON, interacts less strongly with ribosomes with an IC50 357 times higher than DON (He et al., 2015; Wang et al., 2021). In vivo studies were also conducted for pigs fed diets spiked with either DON, de-epoxy DON (DOM-1), or 3-epi-DON. Treatments containing either DOM-1 or 3-epi-DON did not show significant morphological changes in the intestine, nor did they elicit a strong immunological response when compared with DON (Bracarense et al., 2020). Certain strains belonging to Nocardiodes (Ikunaga et al., 2011) Sphingomonas (He et al., 2017), and Devosia (He et al., 2015; Hassan et al., 2017) were shown to aerobically detoxify DON in this manner. In Devosia mutans 17-2-E-8, the enzymes responsible for epimerization were identified and recombinantly expressed in E. coli. Oxidation is first conducted by DepA (for DON Epimerization), a Type I pyrroloquinoline quinone-dependent alcohol dehydrogenase (PQQ-ADH; Carere et al., 2018a). The subsequent step involves DepB, an aldo-keto reductase that stereo-specifically reduces 3-keto-DON to 3-epi-DON in an NADPH-dependent manner (Carere et al., 2018b; Figure 7C). Homologs of DepB have been identified in Sphingomonas sp. S3-4 (He et al., 2017), Devosia sp. strain D6-9 (He et al., 2020) and Rhizobium leguminosarum (Abraham et al., 2022). Unlike DepB, its homolog from Sphingomonas sp. S3-4 oxidizes DON, while a second, unidentified enzyme is believed to stereo-specifically reduce 3-keto-DON to 3-epi-DON, however, this enzyme has not been isolated to date. While epimerization remains a promising approach for enzymatic detoxification of trichothecenes such as DON, its feasibility is limited due to the requirement for cofactors PQQ and NADPH. Interestingly, DepBRleg can reduce 3-keto-DON to 3-epi-DON with NADH, albeit with a catalytic efficiency 40-fold lower than with NADPH (Abraham et al., 2022).
Certain bacteria can oxidize DON to 3-keto-DON but were not capable of transforming 3-keto-DON to 3-epi-DON. These bacteria include strain E3-39 from the Agrobacterium-Rhizobium group of bacteria (Shima et al., 1997), a mixed culture termed D107 (Völkl et al., 2004) and Pelagibacterium halotolerans ANSP101 (Zhang et al., 2020). The enzymes responsible for transforming DON to 3-keto-DON in these bacteria have not been identified. MTT bioassays showed that 3-keto-DON has an IC50 value of at least 3 times higher compared with DON but is still significantly more toxic than 3-epi-DON (He et al., 2015).
Curtobacterium sp. strain 114–2 was reported to utilize T-2 toxin as a carbon and energy source (Ueno et al., 1983). The strain hydrolyzed the ester linkage at the C4 position to produce HT-2 toxin which is in turn hydrolyzed at the C15 ester linkage to produce T-2 triol. This intermediate is finally degraded to unknown metabolic products (Figure 8C). In vivo toxicology studies showed that T-2 triol possesses an LD50 23 and 13 times higher in comparison with T-2 and HT-2 toxins, respectively (Ueno et al., 1983). This hydrolysis was attributed to putative esterases and activity was confirmed for both culture supernatants as well as whole cells, however, the extracellular enzymes proved to be unstable. Curtobacterium sp. strain 114-2 was also capable of transforming DAS to produce scirpentriol by targeting the ester linkages at the C4 and the C15 positions. In contrast, in the ruminal anaerobe Butyrivibrio fibrisolvens, only the C4 ester linkage can be hydrolyzed producing 15-acetoxyscirpenol (Matsushima et al., 1996). Other microbial consortiums from soil and freshwater can further hydrolyze T-2 triol to produce T-2 tetraol (Figure 8C), as well as transform T-2 into neosolaniol (Beeton and Bull, 1989). In terms of toxicity, T-2 tetraol is about 20-fold less toxic than T-2 toxin when administered to mice (Yoshizawa et al., 1984). Similarly, yeast species from the Trichomonascus clade, namely Blastobotrys capitulata, Blastobotrys mokoenaii, and Blastobotrys malaysiensis, converted T-2 toxin into neosolaniol by removal of the C8 isovaleryl group (Figure 8D). Certain species within this clade were also able to modify the C3 OH of T-2 toxin and neosolaniol to form an acetylated derivative (McCormick et al., 2012; Figure 8E). To date, specific enzymes that hydrolyze the ester linkages or modify the C3 OH of trichothecenes have not been isolated from these yeast species.
Acetylated precursors of trichothecenes are formed during the final stages of their synthesis as a self-protection mechanism for the trichothecene-producing fungi (Kimura et al., 1998). Acetylation of DON to mitigate levels in distillers dried grains with solubles (DDGS) has been demonstrated by recombinantly expressing these trichothecene 3-O-acetyltransferases in Saccharomyces cerevisiae strain RW2802 (Khatibi et al., 2011). Among the acetyltransferases examined, FfTRI201, an ortholog of TRI101 from F. graminearum showed the highest conversion of DON to 3-acetyl-DON (3-ADON; Figure 7D). The 3-O-acetylated derivatives showed 100-fold higher IC50 values relative to the parent mycotoxins when administered to rabbit reticulocytes (Kimura et al., 1998).
Hydroxylation of DON at the C16 methyl group had been demonstrated for a Sphingomonas strain KSM1 isolated from a lake in Japan (Ito et al., 2013; Figure 7E). The hydroxylation reaction was determined to involve a cytochrome P450 oxidase (ddnA), an NADH-dependent ferredoxin reductase KdR and ferredoxin Kdx (Ito et al., 2013). When all three proteins were heterologously expressed in E.coli Rosetta 2 (DE3), they oxidized DON to 3α,7α,15,16-tetrahydroxy-12,13-epoxytrichothec-9-en-8-one or 16-HDON (Ito et al., 2013). Phytotoxicity assays showed that 16-HDON had a 10-fold reduced toxicity in comparison to DON.
DON glycosylation at the C3 OH position is a phase II detoxification mechanism typically employed by plants. The enzyme, a UDP-glucosyltransferase, transfers glucose from the substrate, UDP-glucose to an alcohol group, producing DON-3-glucoside (D3G; Poppenberger et al., 2003; Figure 7F). Recent evidence suggests that fungi such as Trichoderma sp. (Tian et al., 2016) and Clonostachys rosea (Demissie et al., 2020) also adopt a similar strategy to detoxify DON as well as its acetylated derivative, 15-acetyl-deoxynivalenol (15-ADON). Plate confrontation assays with either Trichoderma strains or C. rosea against F. graminearum, resulted in the accumulation of D3G and 15-acetyl-DON-3-glucoside, respectively. It is postulated that either an extracellular protein is involved, or a glycosyl-transferase, which affixes a glucose moiety to DON or 15-ADON. These metabolites are subsequently exported to the media via transporter proteins. The growth rates of S. cerevisiae and Chlamydomonas reinhardtii supplemented with D3G revealed no significant effects, demonstrating the attenuated toxicity of the glycosylated derivative (Suzuki and Iwahashi, 2015).
Practical aspects and future prospects
A wide variety of microbial enzymes are capable of transforming mycotoxins to less toxic compounds (Table 2). Mycotoxins, such as ZEN, contain hydroxyl substituents that can be conjugated via ester linkages to sugars or sulfate (Kamimura, 1986; Plasencia and Mirocha, 1991; Paris et al., 2014). However, conjugated mycotoxins are labile and could be hydrolyzed to the parent mycotoxins in the gut by acid/alkaline or intestinal microbiota. Therefore, enzymes that form mycotoxin conjugates are generally not reliable as detoxification strategies.
Other enzymes such as oxidases, reductases, and amino transferases require cofactors or co-substrates, such as NADH or hydrogen peroxide, for activity. This adds additional costs, introduces chemical residues, and complicates the scale-up of the mycotoxins detoxification process. In the biotechnology industry, a secondary enzyme such as formate dehydrogenase has been explored for regenerating NADH from NAD+ that will provide a reduced cofactor for reductase/dehydrogenase reactions (Hummel and Gröger, 2014). Formate will simultaneously be converted to carbon dioxide gas that is easily removed in the reaction. The application of this coenzyme recycling strategy for the enzymatic detoxification of mycotoxins is worth exploring. Alternatively, the use of whole microorganisms instead of purified enzymes could circumvent the need to supplement coenzymes or co-substrates for biotransformation as they can be generated in situ within the microbial cells. Furthermore, increasing the intracellular pool of coenzymes such as NADPH may be achieved through metabolic engineering approaches as described in other reviews (Lee et al., 2013).
However, precautions must be taken to ensure that these microorganisms are not pathogenic and do not have secondary activities that significantly alter the composition of the food product. Novel microbes destined for use in the food industry may modulate the gut microbiota, hence, these candidates must be screened for virulence, antibiotic resistance profiles as well as their propensity for the production of antimicrobial compounds (Brodmann et al., 2017).
The use of whole microorganisms for mycotoxin detoxification is particularly beneficial if these microorganisms are already in use in the food production process, such as in fermentation. For example, Saccharomyces cerevisiae has been demonstrated to degrade patulin during fermentation to ascladiol (Moss and Long, 2002). As a result, the final processed product of cheeses and alcoholic ciders was observed to not experience significant patulin contamination, although the raw materials were initially contaminated with the mycotoxin.
Several mycotoxins contain lactone rings, amide, or ester linkages that can be hydrolyzed by specific enzymes. The hydrolytic reaction requires an aqueous environment but no co-substrates or coenzymes are necessary. The simplicity of the hydrolytic reaction likely contributed to the successful commercialization of purified hydrolases against fumonisin and ZEN applied to feed additives (Heinl et al., 2011; Gruber-Dorninger et al., 2021).
Advances in mass spectrometry techniques to interrogate the proteome coupled with genome sequencing and analysis should continue to accelerate the discovery of microbial enzymes with mycotoxin transformation activity in the future (Chen et al., 2017; Sandlin et al., 2022). This will provide a robust selection of enzymes that could be used for mycotoxin detoxification.
Author contributions
NA and SS were involved in the development of the topic and initial drafts. EC and TZ edited and added additional information to the draft. TZ conceived and coordinated the study. All authors contributed to the article and approved the submitted version.
Funding
This study was supported by Agriculture and Agri-Food Canada (AAFC J-002250) and Grain Farmers of Ontario through the Industry-led Research and Development Project (J-002433).
Acknowledgments
Special thanks to the Guelph Research and Development Centre and Agriculture and Agri-Food Canada for providing the financial support and allocated resources for this project. ChemDraw and BioRender were utilized in the creation of these figures.
Conflict of interest
The authors declare that the research was conducted in the absence of any commercial or financial relationships that could be construed as a potential conflict of interest.
Publisher’s note
All claims expressed in this article are solely those of the authors and do not necessarily represent those of their affiliated organizations, or those of the publisher, the editors and the reviewers. Any product that may be evaluated in this article, or claim that may be made by its manufacturer, is not guaranteed or endorsed by the publisher.
References
Abraham, N., Schroeter, K. L., Zhu, Y., Chan, J., Evans, N., Kimber, M. S., et al. (2022). Structure–function characterization of an aldo–keto reductase involved in detoxification of the mycotoxin, deoxynivalenol. Sci. Rep. 12, 14737–14713. doi: 10.1038/s41598-022-19040-8
Abrunhosa, L., Paterson, R. R. M., and Venâncio, A. (2010). Biodegradation of ochratoxin a for food and feed decontamination. Toxins 2, 1078–1099. doi: 10.3390/toxins2051078
Afriyie-Gyawu, E., Wang, Z., Ankrah, N. A., Xu, L., Johnson, N. M., Tang, L., et al. (2008). NovaSil clay does not affect the concentrations of vitamins a and E and nutrient minerals in serum samples from Ghanaians at high risk for aflatoxicosis. Food Addit. Contam. Part Chem. Anal. Control. Expo. Risk Assess. 25, 872–884. doi: 10.1080/02652030701854758
Ahad, R., Zhou, T., Lepp, D., and Pauls, K. P. (2017). Microbial detoxification of eleven food and feed contaminating trichothecene mycotoxins. BMC Biotechnol. 17, 1–11. doi: 10.1186/s12896-017-0352-7
Alberts, J. F., van Zyl, W. H., and Gelderblom, W. C. A. (2016). Biologically based methods for control of fumonisin-producing fusarium species and reduction of the fumonisins. Front. Microbiol. 7:548. doi: 10.3389/fmicb.2016.00548
Bao, W., Fukushima, Y., Jensen, K. A., Moen, M. A., and Hammel, K. E. (1994). Oxidative degradation of non-phenolic lignin during lipid peroxidation by fungal manganese peroxidase. FEBS Lett. 354, 297–300. doi: 10.1016/0014-5793(94)01146-X
Beeton, S., and Bull, A. T. (1989). Biotransformation and detoxification of T-2 toxin by soil and freshwater bacteria. Appl. Environ. Microbiol. 55, 190–197. doi: 10.1128/aem.55.1.190-197.1989
Blackwell, B. A., Gilliam, J. T., Savard, M. E., Miller, J. D., and Duvick, J. P. (1999). Oxidative deamination of hydrolyzed fumonisin B1 (AP1) by cultures of Exophiala spinifera. Nat. Toxins 7, 31–38. doi: 10.1002/(sici)1522-7189(199902)7:1<31::aid-nt36>3.0.co;2-w
Bracarense, A. P. F. L., Pierron, A., Pinton, P., Gerez, J. R., Schatzmayr, G., Moll, W. D., et al. (2020). Reduced toxicity of 3-epi-deoxynivalenol and de-epoxy-deoxynivalenol through deoxynivalenol bacterial biotransformation: in vivo analysis in piglets. Food Chem. Toxicol. 140:111241. doi: 10.1016/J.FCT.2020.111241
Braun, M. S., and Wink, M. (2018). Exposure, occurrence, and chemistry of Fumonisins and their cryptic derivatives. Compr. Rev. Food Sci. Food Saf. 17, 769–791. doi: 10.1111/1541-4337.12334
Brodehl, A., Möller, A., Kunte, H. J., Koch, M., and Maul, R. (2014). Biotransformation of the mycotoxin zearalenone by fungi of the genera Rhizopus and aspergillus. FEMS Microbiol. Lett. 359, 124–130. doi: 10.1111/1574-6968.12586
Brodmann, T., Endo, A., Gueimonde, M., Vinderola, G., Kneifel, W., de Vos, W. M., et al. (2017). Safety of novel microbes for human consumption: practical examples of assessment in the European Union. Front. Microbiol. 8, 1–15. doi: 10.3389/fmicb.2017.01725
Carere, J., Hassan, Y. I., Lepp, D., and Zhou, T. (2018a). The enzymatic detoxification of the mycotoxin deoxynivalenol: identification of DepA from the DON epimerization pathway. J. Microbial. Biotechnol. 11, 1106–1111. doi: 10.1111/1751-7915.12874
Carere, J., Hassan, Y. I., Lepp, D., and Zhou, T. (2018b). The identification of DepB: an enzyme responsible for the final detoxification step in the deoxynivalenol epimerization pathway in devosia mutans 17-2-E-8. Front. Microbiol. 9, 1–9. doi: 10.3389/fmicb.2018.01573
Castoria, R., Mannina, L., Durán-Patrón, R., Maffei, F., Sobolev, A. P., De Felice, D. V., et al. (2011). Conversion of the mycotoxin patulin to the less toxic desoxypatulinic acid by the biocontrol yeast Rhodosporidium kratochvilovae strain LS11. J. Agric. Food Chem. 59, 11571–11578. doi: 10.1021/jf203098v
Chan, E. T. S., Zhu, Y., Li, X. Z., Zhou, T., and Seah, S. Y. K. (2022). Characterization of Two Dehydrogenases from Gluconobacter oxydans Involved in the Transformation of Patulin to Ascladiol. Toxins (Basel) 14:423. doi: 10.3390/toxins14070423
Chelkowski, J., Goliński, P., Godlewska, B., Radomyska, W., Szebiotko, K., and Wiewiórowska, M. (1981). Mycotoxins in cereal grain. Part IV. Inactivation of ochratoxin a and other mycotoxins during ammoniation. Food Nahrung 25, 631–637. doi: 10.1002/food.19810250705
Chen, Y., Peng, H., Wang, X., Li, B., Long, M., and Tian, S. (2017). Biodegradation mechanisms of Patulin in Candida guilliermondii: an iTRAQ-based proteomic analysis. Toxins 9:48. doi: 10.3390/toxins9020048
Chen, Y. H., Sheu, S. C., Mau, J. L., and Hsieh, P. C. (2011). Isolation and characterization of a strain of Klebsiella pneumoniae with citrinin-degrading activity. World J. Microbiol. Biotechnol. 27, 487–493. doi: 10.1007/s11274-010-0478-4
Cleveland, T. E., Dowd, P. F., Desjardins, A. E., Bhatnagar, D., and Cotty, P. J. (2003). United States Department of Agriculture-Agricultural Research Service research on pre-harvest prevention of mycotoxins and mycotoxigenic fungi in US crops. Pest Manag. Sci. 59, 629–642. doi: 10.1002/ps.724
Conte, G., Fontanelli, M., Galli, F., Cotrozzi, L., Pagni, L., and Pellegrini, E. (2020). Mycotoxins in feed and food and the role of ozone in their detoxification and degradation: an update. Toxins 12:486. doi: 10.3390/toxins12080486
Cullen, J. M., Ruebner, B. H., Hsieh, L. S., Hyde, D. M., and Hsieh, D. P. (1987). Carcinogenicity of dietary aflatoxin M1 in male Fischer rats compared to aflatoxin B1. Cancer Res. 47, 1913–1917.
Curtis, R. F., Hassall, C. H., and Nazar, M. (1968). The biosynthesis of phenols. Part XV. Some metabolites of Penicillium citrinum related to citrinin. J. Chem. Soc. C Org. 1, 85–93. doi: 10.1039/j39680000085
Dall’Erta, A., Cirlini, M., Dall’Asta, M., Del Rio, D., Galaverna, G., and Dall’Asta, C. (2013). Masked mycotoxins are efficiently hydrolyzed by human colonic microbiota releasing their aglycones. Chem. Res. Toxicol. 26, 305–312. doi: 10.1021/tx300438c
Demissie, Z. A., Witte, T., Robinson, K. A., Sproule, A., Foote, S. J., Johnston, A., et al. (2020). Transcriptomic and Exometabolomic profiling reveals antagonistic and defensive modes of Clonostachys rosea action against fusarium graminearum. Mol. Plant Microbe Interact. 33, 842–858. doi: 10.1094/MPMI-11-19-0310-R
Desjardins, A. E., Hohn, T. M., and McCormick, S. P. (1993). Trichothecene biosynthesis in fusarium species: chemistry, genetics, and significance. Microbiol. Rev. 57, 595–604. doi: 10.1128/mmbr.57.3.595-604.1993
Devi, P., Naik, C. G., and Rodrigues, C. (2006). Biotransformation of citrinin to decarboxycitrinin using an organic solvent-tolerant marine bacterium, Moraxella sp. MB1. Marine Biotechnol. 8, 129–138. doi: 10.1007/s10126-005-5021-5
Dobritzsch, D., Wang, H., Schneider, G., and Yu, S. (2014). Structural and functional characterization of ochratoxinase, a novel mycotoxin-degrading enzyme. Biochem. J. 462, 441–452. doi: 10.1042/BJ20140382
Dong, X., Jiang, W., Li, C., Ma, N., Xu, Y., and Meng, X. (2015). Patulin biodegradation by marine yeast Kodameae ohmeri. Food Addit. Contam. - Part A 32, 352–360. doi: 10.1080/19440049.2015.1007090
Duarte, S. C., Pena, A., and Lino, C. M. (2010). A review on ochratoxin a occurrence and effects of processing of cereal and cereal derived food products. Food Microbiol. 27, 187–198. doi: 10.1016/j.fm.2009.11.016
Eaton, D. L., and Gallagher, E. P. (1994). Mechanisms of aflatoxin carcinogenesis. Annu. Rev. Pharmacol. Toxicol. 34, 135–172. doi: 10.1146/annurev.pa.34.040194.001031
El-sharkawy, S., and Abul-hajj, Y. (1987). Microbial transformation of Zearalenone, I. formation of Zearalenone-4-o-β-glucoside. J. Nat. Prod. 50, 520–521. doi: 10.1021/np50051a038
El-Sharkawy, S. H., and Abul-Hajj, Y. J. (1988). Microbial transformation of Zearalenone. 2. Reduction, hydroxylation, and methylation products. J. Org. Chem. 53, 515–519. doi: 10.1021/jo00238a008
El-Sharkawy, S. H., Selim, M. I., Afifi, M. S., and Halaweish, F. T. (1991). Microbial transformation of zearalenone to a zearalenone sulfate. Appl. Environ. Microbiol. 57, 549–552. doi: 10.1128/aem.57.2.549-552.1991
Eriksen, G. S., and Pettersson, H. (2004). Toxicological evaluation of trichothecenes in animal feed. Anim. Feed Sci. Technol. 114, 205–239. doi: 10.1016/j.anifeedsci.2003.08.008
Eskola, M., Kos, G., Elliott, C. T., Hajšlová, J., Mayar, S., and Krska, R. (2020). Worldwide contamination of food-crops with mycotoxins: validity of the widely cited ‘FAO estimate’ of 25%. Crit. Rev. Food Sci. Nutr. 60, 2773–2789. doi: 10.1080/10408398.2019.1658570
Fliege, R., and Metzler, M. (2000). Electrophilic properties of patulin. Adduct structures and reaction pathways with 4-bromothiophenol and other model nucleophiles. Chem. Res. Toxicol. 13, 363–372. doi: 10.1021/tx9901478
Foroud, N. A., and Eudes, F. (2009). Trichothecenes in cereal grains. Int. J. Mol. Sci. 10, 147–173. doi: 10.3390/ijms10010147
Fuchs, E., Binder, E. M., Heidler, D., and Krska, R. (2000). Characterisation of metabolites after the microbial degradation of A-and B-trichothecenes by BBSH. Mycotoxin Res. 16, 66–69. doi: 10.1007/bf02942984
Fuchs, E., Binder, E. M., Heidler, D., and Krska, R. (2002). Structural characterization of metabolites after the microbial degradation of type a trichothecenes by the bacterial strain BBSH 797. Food Addit. Contam. 19, 379–386. doi: 10.1080/02652030110091154
Gao, X., Mu, P., Wen, J., Sun, Y., Chen, Q., and Deng, Y. (2018). Detoxification of trichothecene mycotoxins by a novel bacterium, Eggerthella sp. DII-9. Food Chem. Toxicol. 112, 310–319. doi: 10.1016/j.fct.2017.12.066
Gao, X., Mu, P., Zhu, X., Chen, X., Tang, S., Wu, Y., et al. (2020). Dual function of a novel bacterium, Slackia sp. D-G6: detoxifying deoxynivalenol and producing the natural estrogen analogue, Equol. Toxins 12:12. doi: 10.3390/toxins12020085
Garnham, C. P., Butler, S. G., Telmer, P. G., Black, F. E., Renaud, J. B., and Sumarah, M. W. (2020). Identification and characterization of an aspergillus Niger amine oxidase that detoxifies intact Fumonisins. J. Agric. Food Chem. 68, 13779–13790. doi: 10.1021/acs.jafc.0c04504
Garreau De Loubresse, N., Prokhorova, I., Holtkamp, W., Rodnina, M. V., Yusupova, G., and Yusupov, M. (2014). Structural basis for the inhibition of the eukaryotic ribosome. Nature 513, 517–522. doi: 10.1038/nature13737
González Pereyra, M. L., Martínez, M. P., and Cavaglieri, L. R. (2019). Presence of aiiA homologue genes encoding for N-acyl homoserine lactone-degrading enzyme in aflatoxin B 1-decontaminating bacillus strains with potential use as feed additives. Food Chem. Toxicol. 124, 316–323. doi: 10.1016/j.fct.2018.12.016
Gruber-Dorninger, C., Faas, J., Doupovec, B., Aleschko, M., Stoiber, C., Höbartner-Gußl, A., et al. (2021). Metabolism of Zearalenone in the rumen of dairy cows with and without application of a Zearalenone-degrading enzyme. Toxins 13, 1–18. doi: 10.3390/toxins13020084
Guo, Y., Qin, X., Tang, Y., Ma, Q., Zhang, J., and Zhao, L. (2020). CotA laccase, a novel aflatoxin oxidase from bacillus licheniformis, transforms aflatoxin B1 to aflatoxin Q1 and epi-aflatoxin Q1. Food Chem. 325:126877. doi: 10.1016/j.foodchem.2020.126877
Hassan, Y. I., He, J. W., Perilla, N., Tang, K. J., Karlovsky, P., and Zhou, T. (2017). The enzymatic epimerization of deoxynivalenol by Devosia mutans proceeds through the formation of 3-keto-DON intermediate. Sci. Rep. 7, 6929–6911. doi: 10.1038/s41598-017-07319-0
Hawar, S., Vevers, W., Karieb, S., Ali, B. K., Billington, R., and Beal, J. (2013). Biotransformation of patulin to hydroascladiol by lactobacillus plantarum. Food Control 34, 502–508. doi: 10.1016/j.foodcont.2013.05.023
He, J. W., Bondy, G. S., Zhou, T., Caldwell, D., Boland, G. J., and Scott, P. M. (2015). Toxicology of 3-epi-deoxynivalenol, a deoxynivalenol-transformation product by Devosia mutans 17-2-E-8. Food Chem. Toxicol. 84, 250–259. doi: 10.1016/j.fct.2015.09.003
He, W. J., Shi, M. M., Yang, P., Huang, T., Zhao, Y., Wu, A. B., et al. (2020). A quinone-dependent dehydrogenase and two NADPH-dependent aldo/keto reductases detoxify deoxynivalenol in wheat via epimerization in a Devosia strain. Food Chem. 321:126703. doi: 10.1016/j.foodchem.2020.126703
He, W. J., Zhang, L., Yi, S. Y., Tang, X. L., Yuan, Q. S., Guo, M. W., et al. (2017). An aldo-keto reductase is responsible for fusarium toxindegrading activity in a soil Sphingomonas strain. Sci. Rep. 7, 1–13. doi: 10.1038/s41598-017-08799-w
Heinl, S., Hartinger, D., Thamhesl, M., Schatzmayr, G., Moll, W. D., and Grabherr, R. (2011). An aminotransferase from bacterium ATCC 55552 deaminates hydrolyzed fumonisin B1. Biodegradation 22, 25–30. doi: 10.1007/s10532-010-9371-y
Hsieh, D. P. H., Salhab, A. S., Wong, J. J., and Yang, S. L. (1974). Toxicity of aflatoxin Q1 as evaluated with the chicken embryo and bacterial auxotrophs. Toxicol. Appl. Pharmacol. 30, 237–242. doi: 10.1016/0041-008X(74)90095-7
Hui, R., Hu, X., Liu, W., Liu, W., Zheng, Y., Chen, Y., et al. (2017). Characterization and crystal structure of a novel zearalenone hydrolase from Cladophialophora bantiana. Acta Crystallogr. Sect. Struct. Biol. Commun. 73, 515–519. doi: 10.1107/S2053230X17011840
Hummel, W., and Gröger, H. (2014). Strategies for regeneration of nicotinamide coenzymes emphasizing self-sufficient closed-loop recycling systems. J. Biotechnol. 191, 22–31. doi: 10.1016/j.jbiotec.2014.07.449
Ianiri, G., Idnurm, A., Wright, S. A. I., Durán-Patrón, R., Mannina, L., Ferracane, R., et al. (2013). Searching for genes responsible for Patulin degradation in a biocontrol yeast provides insight into the basis for resistance to this. Appl. Environ. Microbiol. 79, 3101–3115. doi: 10.1128/AEM.03851-12
Ikunaga, Y., Sato, I., Grond, S., Numaziri, N., Yoshida, S., Yamaya, H., et al. (2011). Nocardioides sp. strain WSN05-2, isolated from a wheat field, degrades deoxynivalenol, producing the novel intermediate 3-epi-deoxynivalenol. Appl. Microbiol. Biotechnol. 89, 419–427. doi: 10.1007/s00253-010-2857-z
Islam, R., Zhou, T., Young, J. C., Goodwin, P. H., and Pauls, K. P. (2012). Aerobic and anaerobic de-epoxydation of mycotoxin deoxynivalenol by bacteria originating from agricultural soil. World J. Microbiol. Biotechnol. 28, 7–13. doi: 10.1007/s11274-011-0785-4
Ito, M., Sato, I., Ishizaka, M., Yoshida, S. I., Koitabashi, M., Yoshida, S., et al. (2013). Bacterial cytochrome P450 system catabolizing the fusarium toxin deoxynivalenol. Appl. Environ. Microbiol. 79, 1619–1628. doi: 10.1128/AEM.03227-12
Jackson, L. K., and Ciegler, A. (1978). Production and analysis of citrinin in corn. Appl. Environ. Microbiol. 36, 408–411. doi: 10.1128/aem.36.3.408-411.1978
Jard, G., Liboz, T., Mathieu, F., Guyonvarc’H, A., André, F., Delaforge, M., et al. (2010). Transformation of zearalenone to zearalenone-sulfate by aspergillus spp. World Mycotoxin J. 3, 183–191. doi: 10.3920/WMJ2009.1184
Ji, F., He, D., Olaniran, A. O., Mokoena, M. P., Xu, J., and Shi, J. (2019). Occurrence, toxicity, production and detection of fusarium mycotoxin: a review. Food Prod. Process. Nutr. 1, 1–14. doi: 10.1186/s43014-019-0007-2
Kakeya, H., Takahashi-Ando, N., Kimura, M., Onose, R., Yamaguchi, I., and Osada, H. (2002). Biotransformation of the mycotoxin, Zearalenone, to a non-estrogenic compound by a fungal strain of Clonostachys sp. Biosci. Biotechnol. Biochem. 66, 2723–2726. doi: 10.1271/bbb.66.2723
Kamimura, H. (1986). Conversion of zearalenone to zearalenone glycoside by Rhyzopus sp. Appl. Environ. Microbiol. 52, 515–519. doi: 10.1128/aem.52.3.515-519.1986
Kanpiengjai, A., Mahawan, R., Lumyong, S., and Khanongnuch, C. (2016). A soil bacterium rhizobium borbori and its potential for citrinin-degrading application. Ann. Microbiol. 66, 807–816. doi: 10.1007/s13213-015-1167-1
Karačić, Z., Ban, Ž., and Macheroux, P. (2017). A novel member of the dipeptidyl peptidase III family from Armillariella tabescens. Curr. Top. Pept. Protein Res. 18, 41–48.
Khatibi, P. A., Newmister, S. A., Rayment, I., McCormick, S. P., Alexander, N. J., and Schmale, D. G. (2011). Bioprospecting for trichothecene 3-o-acetyltransferases in the fungal genus fusarium yields functional enzymes with different abilities to modify the mycotoxin deoxynivalenol. Appl. Environ. Microbiol. 77, 1162–1170. doi: 10.1128/AEM.01738-10
Kimura, M., Kaneko, I., Komiyama, M., Takatsuki, A., Koshino, H., Yoneyama, K., et al. (1998). Trichothecene 3-O-acetyltransferase protects both the producing organism and transformed yeast from related mycotoxins. Cloning and characterization of Tri101. J. Biol. Chem. 273, 1654–1661. doi: 10.1074/jbc.273.3.1654
Klinman, J. P. (2003). The multi-functional topa-quinone copper amine oxidases. Biochim. Biophys. Acta Proteins Proteomics 1647, 131–137. doi: 10.1016/S1570-9639(03)00077-3
Lee, L. S., Dunn, J. J., DeLucca, A. J., and Ciegler, A. (1981). Role of lactone ring of aflatoxin B1 in toxicity and mutagenicity. Experientia 37, 16–17. doi: 10.1007/BF01965543
Lee, W. H., Kim, M. D., Jin, Y. S., and Seo, J. H. (2013). Engineering of NADPH regenerators in Escherichia coli for enhanced biotransformation. Appl. Microbiol. Biotechnol. 97, 2761–2772. doi: 10.1007/s00253-013-4750-z
Liu, F., Malaphan, W., Xing, F., and Yu, B. (2019). Biodetoxification of fungal mycotoxins zearalenone by engineered probiotic bacterium lactobacillus reuteri with surface-displayed lactonohydrolase. Appl. Microbiol. Biotechnol. 103, 8813–8824. doi: 10.1007/s00253-019-10153-1
Liu, D. L., Yao, D. S., Liang, Y. Q., Zhou, T. H., Song, Y. P., Zhao, L., et al. (2001). Production, purification, and characterization of an intracellular aflatoxin-detoxifizyme from Armillariella tabescens (E-20). Food Chem. Toxicol. 39, 461–466. doi: 10.1016/S0278-6915(00)00161-7
Loi, M., Fanelli, F., Cimmarusti, M. T., Mirabelli, V., Haidukowski, M., Logrieco, A. F., et al. (2018). In vitro single and combined mycotoxins degradation by Ery4 laccase from Pleurotus eryngii and redox mediators. Food Control 90, 401–406. doi: 10.1016/j.foodcont.2018.02.032
Loi, M., Fanelli, F., Zucca, P., Liuzzi, V. C., Quintieri, L., Cimmarusti, M. T., et al. (2016). Aflatoxin B1 and M1 degradation by Lac2 from Pleurotus pulmonarius and redox mediators. Toxins 8, 1–16. doi: 10.3390/toxins8090245
Loi, M., Renaud, J. B., Rosini, E., Pollegioni, L., Vignali, E., Haidukowski, M., et al. (2020). Enzymatic transformation of aflatoxin B1 by Rh_DypB peroxidase and characterization of the reaction products. Chemosphere 250, 126296–126297. doi: 10.1016/j.chemosphere.2020.126296
Lyagin, I., and Efremenko, E. (2019). Enzymes for detoxification of various mycotoxins: origins and mechanisms of catalytic action. Molecules 24, 1–39. doi: 10.3390/molecules24132362
Mahato, D. K., Lee, K. E., Kamle, M., Devi, S., Dewangan, K. N., Kumar, P., et al. (2019). Aflatoxins in food and feed: an overview on prevalence, detection and control strategies. Front. Microbiol. 10, 1–10. doi: 10.3389/fmicb.2019.02266
Matsushima, T., Okamoto, E., Miyagawa, E., Matsui, Y., Shimizu, H., and Asano, K. (1996). Deacetylation of diacetoxyscirpenol to 15-acetoxyscirpenol by rumen bacteria. J. Gen. Appl. Microbiol. 42, 225–234. doi: 10.2323/jgam.42.225
McCormick, S. P., Price, N. P. J., and Kurtzman, C. P. (2012). Glucosylation and other biotransformations of T-2 toxin by yeasts of the trichomonascus clade. Appl. Environ. Microbiol. 78, 8694–8702. doi: 10.1128/AEM.02391-12
Metzler, M., Pfeiffer, E., and Hildebrand, A. A. (2010). Zearalenone and its metabolites as endocrine disrupting chemicals. World Mycotoxin J. 3, 385–401. doi: 10.3920/WMJ2010.1244
Moss, M. O., and Long, M. T. (2002). Fate of patulin in the presence of the yeast Saccharomyces cerevisiae. Food Addit. Contam. 19, 387–399. doi: 10.1080/02652030110091163
Neme, K., and Mohammed, A. (2017). Mycotoxin occurrence in grains and the role of postharvest management as a mitigation strategies: A review. Food Control 78, 412–425. doi: 10.1016/j.foodcont.2017.03.012
Paris, M. P. K., Schweiger, W., Hametner, C., Stückler, R., Muehlbauer, G. J., Varga, E., et al. (2014). Zearalenone-16-o-glucoside: a new masked mycotoxin. J. Agric. Food Chem. 62, 1181–1189. doi: 10.1021/jf405627d
Pattono, D., Grosso, A., Stocco, P. P., Pazzi, M., and Zeppa, G. (2013). Survey of the presence of patulin and ochratoxin a in traditional semi-hard cheeses. Food Control 33, 54–57. doi: 10.1016/j.foodcont.2013.02.019
Pfenning, C., Esch, H. L., Fliege, R., and Lehmann, L. (2016). The mycotoxin patulin reacts with DNA bases with and without previous conjugation to GSH: implication for related α,β-unsaturated carbonyl compounds? Arch. Toxicol. 90, 433–448. doi: 10.1007/s00204-014-1443-z
Phillips, T. D., Wang, M., Elmore, S. E., Hearon, S., and Wang, J.-S. (2019). NovaSil clay for the protection of humans and animals from aflatoxins and other contaminants. Clays Clay Miner. 67, 99–110. doi: 10.1007/s42860-019-0008-x
Pickova, D., Ostry, V., Toman, J., and Malir, F. (2021). Aflatoxins: history, significant milestones, recent data on their toxicity and ways to mitigation. Toxins 13, 1–23. doi: 10.3390/toxins13060399
Pitout, M. J. (1969). The hydrolysis of ochratoxin a by some proteolytic enzymes. Biochem. Pharmacol. 18, 485–491. doi: 10.1016/0006-2952(69)90224-X
Plasencia, J., and Mirocha, C. J. (1991). Isolation and characterization of zearalenone sulfate produced by fusarium spp. Appl. Environ. Microbiol. 57, 146–150. doi: 10.1128/aem.57.1.146-150.1991
Pong, R. S., and Wogan, G. N. (1971). Toxicity and biochemical and fine structural effects of synthetic aflatoxins M 1 and B 1 in rat liver. J. Natl. Cancer Inst. 47, 585–592.
Poppenberger, B., Berthiller, F., Lucyshyn, D., Sieberer, T., Schuhmacher, R., Krska, R., et al. (2003). Detoxification of the fusarium mycotoxin Deoxynivalenol by a UDP-glucosyltransferase from Arabidopsis thaliana. J. Biol. Chem. 278, 47905–47914. doi: 10.1074/jbc.M307552200
Prandini, A., Tansini, G., Sigolo, S., Filippi, L., Laporta, M., and Piva, G. (2009). On the occurrence of aflatoxin M1 in milk and dairy products. Food Chem. Toxicol. 47, 984–991. doi: 10.1016/j.fct.2007.10.005
Purchase, I. F. H. (1967). Acute toxicity of aflatoxins M1 and M2 in one-day-old ducklings. Food Cosmet. Toxicol. 5, 339–342. doi: 10.1016/s0015-6264(67)83060-8
Ricelli, A., Baruzzi, F., Solfrizzo, M., Morea, M., and Fanizzi, F. P. (2007). Biotransformation of Patulin by Gluconobacter oxydans. Appl. Environ. Microbiol. 73, 785–792. doi: 10.1128/AEM.02032-06
Saleh, I., and Goktepe, I. (2019). The characteristics, occurrence, and toxicological effects of patulin. Food Chem. Toxicol. 129, 301–311. doi: 10.1016/j.fct.2019.04.036
Sandlin, N., Kish, D. R., Kim, J., Zaccaria, M., and Momeni, B. (2022). Current and emerging tools of computational biology to improve the detoxification of mycotoxins. Appl. Environ. Microbiol. 88:e0210221. doi: 10.1128/aem.02102-21
Seiferlein, M., Humpf, H. U., Voss, K. A., Sullards, M. C., Allegood, J. C., Wang, E., et al. (2007). Hydrolyzed fumonisins HFB1 and HFB2 are acylated in vitro and in vivo by ceramide synthase to form cytotoxic N-acyl-metabolites. Mol. Nutr. Food Res. 51, 1120–1130. doi: 10.1002/mnfr.200700118
Sekiguchi, J., Shimamoto, T., Yamada, Y., and Gaucher, G. M. (1983). Patulin biosynthesis: enzymatic and nonenzymatic transformations of the mycotoxin (E)-ascladiol. Appl. Environ. Microbiol. 45, 1939–1942. doi: 10.1128/aem.45.6.1939-1942.1983
Shank, R. A., Foroud, N. A., Hazendonk, P., Eudes, F., and Blackwell, B. A. (2011). Current and future experimental strategies for structural analysis of trichothecene mycotoxins-a prospectus. Toxins 3, 1518–1553. doi: 10.3390/toxins3121518
Shima, J., Takase, S., Takahashi, Y., Iwai, Y., Fujimoto, H., Yamazaki, M., et al. (1997). Novel detoxification of the trichothecene mycotoxin deoxynivalenol by a soil bacterium isolated by enrichment culture. Appl. Environ. Microbiol. 63, 3825–3830. doi: 10.1128/aem.63.10.3825-3830.1997
Shinohara, Y., Arai, M., Hirao, K., Sugihara, S., and Nakanishi, K. (1976). Combination effect of citrinin and other chemicals on rat kidney tumorigenesis. Gaan 67, 147–155.
Silva, L. J. G., Pereira, A. M. P. T., Pena, A., and Lino, C. M. (2021). Citrinin in foods and supplements: a review of occurrence and analytical methodologies. Foods 10:14. doi: 10.3390/foods10010014
Speijers, G. J., Franken, M. A., and van Leeuwen, F. X. (1988). Subacute toxicity study of patulin in the rat: effects on the kidney and the gastro-intestinal tract. Food Chem. Toxicol. 26, 23–30. doi: 10.1016/0278-6915(88)90037-3
Stander, M. A., Bornscheuer, U. T., Henke, E., and Steyn, P. S. (2000). Screening of commercial hydrolases for the degradation of Ochratoxin a. J. Agric. Food Chem. 48, 5736–5739. doi: 10.1021/jf000413j
Stoev, S. D. (2013). Food safety and increasing Hazard of mycotoxin occurrence in foods and feeds. Crit. Rev. Food Sci. Nutr. 53, 887–901. doi: 10.1080/10408398.2011.571800
Suzuki, T., and Iwahashi, Y. (2015). Low toxicity of Deoxynivalenol-3-glucoside in microbial cells. Toxins 7, 187–200. doi: 10.3390/toxins7010187
Swanson, S. P., Rood, H. D., Behrens, J. C., and Sanders, P. E. (1987). Preparation and characterization of the deepoxy trichothecenes: Deepoxy HT-2, deepoxy T-2 triol, deepoxy T-2 tetraol, deepoxy 15-monoacetoxyscirpenol, and deepoxy scirpentriol. Appl. Environ. Microbiol. 53, 2821–2826. doi: 10.1128/aem.53.12.2821-2826.1987
Tang, H., Li, X., Zhang, F., Meng, X., and Liu, B. (2019). Biodegradation of the mycotoxin patulin in apple juice by Orotate phosphoribosyltransferase from Rhodotorula mucilaginosa. Food Control 100, 158–164. doi: 10.1016/j.foodcont.2019.01.020
Takahashi-Ando, N., Kimura, M., Kakeya, H., Osada, H., and Yamaguchi, I. (2002). A novel lactonohydrolase responsible for the detoxification of zearalenone’ enzyme purification and gene cloning. Biochem. J. 365, 1–6. doi: 10.1042/BJ20020450
Takahashi-Ando, N., Ohsato, S., Shibata, T., Hamamoto, H., Yamaguchi, I., and Kimura, M. (2004). Metabolism of zearalenone by genetically modified organisms expressing the detoxification gene from Clonostachys rosea. Appl. Environ. Microbiol. 70, 3239–3245. doi: 10.1128/AEM.70.6.3239-3245.2004
Taylor, M. C., Jackson, C. J., Tattersall, D. B., French, N., Peat, T. S., Newman, J., et al. (2010). Identification and characterization of two families of F420H2-dependent reductases from mycobacteria that catalyse aflatoxin degradation. Mol. Microbiol. 78, 561–575. doi: 10.1111/j.1365-2958.2010.07356.x
Tian, Y., Tan, Y., Liu, N., Yan, Z., Liao, Y., Chen, J., et al. (2016). Detoxification of Deoxynivalenol via glycosylation represents novel insights on antagonistic activities of Trichoderma when confronted with fusarium graminearum. Toxins 8:335. doi: 10.3390/toxins8110335
Ueno, Y., Nakayama, K., Ishii, K., Tashiro, F., Minoda, Y., Omori, T., et al. (1983). Metabolism of T-2 toxin in Curtobacterium sp. strain 114-2. Appl. Environ. Microbiol. 46, 120–127. doi: 10.1128/aem.46.1.120-127.1983
Vanacloig-Pedros, E., Proft, M., and Pascual-Ahuir, A. (2016). Different toxicity mechanisms for citrinin and ochratoxin a revealed by transcriptomic analysis in yeast. Toxins 8:273. doi: 10.3390/toxins8100273
Vekiru, E., Hametner, C., Mitterbauer, R., Rechthaler, J., Adam, G., Schatzmayr, G., et al. (2010). Cleavage of zearalenone by Trichosporon mycotoxinivorans to a novel nonestrogenic metabolite7. Appl. Environ. Microbiol. 76, 2353–2359. doi: 10.1128/AEM.01438-09
Vignali, E., Tonin, F., Pollegioni, L., and Rosini, E. (2018). Characterization and use of a bacterial lignin peroxidase with an improved manganese-oxidative activity. Appl. Microbiol. Biotechnol. 102, 10579–10588. doi: 10.1007/s00253-018-9409-3
Vila-Donat, P., Marín, S., Sanchis, V., and Ramos, A. J. (2018). A review of the mycotoxin adsorbing agents, with an emphasis on their multi-binding capacity, for animal feed decontamination. Food Chem. Toxicol. 114, 246–259. doi: 10.1016/j.fct.2018.02.044
Völkl, A., Vogler, B., Schollenberger, M., and Karlovsky, P. (2004). Microbial detoxification of mycotoxin deoxynivalenol. J. Basic Microbiol. 44, 147–156. doi: 10.1002/jobm.200310353
Wang, P., Afriyie-gyawu, E., Tang, Y., Johnson, N. M., Xu, L., Tang, L., et al. (2008). NovaSil clay intervention in Ghanaians at high risk for aflatoxicosis: II. Reduction in biomarkers of aflatoxin exposure in blood and urine. Food Addit. Contam. Part a Chem. Anal. Control. Expo. Risk Assess. 25, 622–634. doi: 10.1080/02652030701598694
Wang, J., Ogata, M., Hirai, H., and Kawagishi, H. (2011). Detoxification of aflatoxin B1 by manganese peroxidase from the white-rot fungus Phanerochaete sordida YK-624. FEMS Microbiol. Lett. 314, 164–169. doi: 10.1111/j.1574-6968.2010.02158.x
Wang, K., Zheng, X., Yang, Q., Zhang, H., Apaliya, M. T., Dhanasekaran, S., et al. (2019). S-Adenosylmethionine-Dependent Methyltransferase Helps Pichia caribbica Degrade Patulin. J. Agric. Food Chem. 67, 11758–11768. doi: 10.1021/acs.jafc.9b05144
Wang, W., Zhu, Y., Abraham, N., Li, X. Z., Kimber, M., and Zhou, T. (2021). The ribosome-binding mode of trichothecene mycotoxins rationalizes their structure—activity relationships. Int. J. Mol. Sci. 22, 1–17. doi: 10.3390/ijms22041604
Wilkins, K., Nielsen, K. F., and Din, S. U. (2003). Patterns of volatile metabolites and nonvolatile trichothecenes produced by isolates of Stachybotrys, fusarium, Trichoderma, Trichothecium and Memnoniella. Environ. Sci. Pollut. Res. 10, 162–166. doi: 10.1065/espr2002.05.118
Wright, S. A. I. (2015). Patulin in food. Curr. Opin. Food Sci. 5, 105–109. doi: 10.1016/j.cofs.2015.10.003
Xing, M., Chen, Y., Li, B., and Tian, S. (2021). Characterization of a short-chain dehydrogenase/reductase and its function in patulin biodegradation in apple juice. Food Chem. 348:129046. doi: 10.1016/j.foodchem.2021.129046
Xu, H., Wang, L., Sun, J., Wang, L., Guo, H., Ye, Y., et al. (2022). Microbial detoxification of mycotoxins in food and feed. Crit. Rev. Food Sci. Nutr. 62, 4951–4969. doi: 10.1080/10408398.2021.1879730
Xu, T., Xie, C., Yao, D., Zhou, C. Z., and Liu, J. (2017). Crystal structures of aflatoxin-oxidase from Armillariella tabescens reveal a dual activity enzyme. Biochem. Biophys. Res. Commun. 494, 621–625. doi: 10.1016/j.bbrc.2017.10.077
Yang, W. C., Hsu, T. C., Cheng, K. C., and Liu, J. R. (2017). Expression of the Clonostachys rosea lactonohydrolase gene by lactobacillus reuteri to increase its zearalenone-removing ability. Microb. Cell Fact. 16, 69–11. doi: 10.1186/s12934-017-0687-8
Yang, Q., Pang, B., Solairaj, D., Hu, W., Legrand, N. N. G., Ma, J., et al. (2021). Effect of Rhodotorula mucilaginosa on patulin degradation and toxicity of degradation products. Food Addit. Contam. Part a Chem. Anal. Control. Expo. Risk Assess. 38, 1427–1439. doi: 10.1080/19440049.2021.1923821
Yoshizawa, T., Sakamoto, T., and Okamoto, K. (1984). In vitro formation of 3′-hydroxy T-2 and 3′-hydroxy HT-2 toxins from T-2 toxin by liver homogenates from mice and monkeys. Appl. Environ. Microbiol. 47, 130–134. doi: 10.1128/aem.47.1.130-134.1984
Yu, H., Zhou, T., Gong, J., Young, C., Su, X., Li, X. Z., et al. (2010). Isolation of deoxynivalenol-transforming bacteria from the chicken intestines using the approach of PCR-DGGE guided microbial selection. BMC Microbiol. 10:182. doi: 10.1186/1471-2180-10-182
Zain, M. E. (2011). Impact of mycotoxins on humans and animals. J. Saudi Chem. Soc. 15, 129–144. doi: 10.1016/j.jscs.2010.06.006
Zhang, Z., Nie, D., Fan, K., Yang, J., Guo, W., Meng, J., et al. (2020). A systematic review of plant-conjugated masked mycotoxins: occurrence, toxicology, and metabolism. Crit. Rev. Food Sci. Nutr. 60, 1523–1537. doi: 10.1080/10408398.2019.1578944
Zheng, Y., Liu, W., Chen, C. C., Hu, X., Liu, W., Ko, T. P., et al. (2018). Crystal structure of a Mycoestrogen-detoxifying lactonase from Rhinocladiella mackenziei: molecular insight into ZHD substrate selectivity. ACS Catal. 8, 4294–4298. doi: 10.1021/acscatal.8b00464
Zhu, Y., Drouin, P., Lepp, D., Li, X. Z., Zhu, H., Castex, M., et al. (2021). A novel microbial zearalenone transformation through phosphorylation. Toxins 13:294. doi: 10.3390/toxins13050294
Zhu, R., Feussner, K., Wu, T., Yan, F., Karlovsky, P., and Zheng, X. (2015). Detoxification of mycotoxin patulin by the yeast Rhodosporidium paludigenum. Food Chem. 179, 1–5. doi: 10.1016/j.foodchem.2015.01.066
Keywords: Mycotoxins, aflatoxins, ochratoxin, citrinin, zearaleneone, patulin, deoxynivalenol, T2
Citation: Abraham N, Chan ETS, Zhou T and Seah SYK (2022) Microbial detoxification of mycotoxins in food. Front. Microbiol. 13:957148. doi: 10.3389/fmicb.2022.957148
Edited by:
Vittorio Capozzi, Institute of Sciences of Food Production (CNR), ItalyReviewed by:
Michele Christine Loewen, National Research Council, CanadaCarlos Augusto Fernandes Oliveira, University of São Paulo, Brazil
Copyright © 2022 Abraham, Chan, Zhou and Seah. This is an open-access article distributed under the terms of the Creative Commons Attribution License (CC BY). The use, distribution or reproduction in other forums is permitted, provided the original author(s) and the copyright owner(s) are credited and that the original publication in this journal is cited, in accordance with accepted academic practice. No use, distribution or reproduction is permitted which does not comply with these terms.
*Correspondence: Stephen Y. K. Seah, c3NlYWhAdW9ndWVscGguY2E=