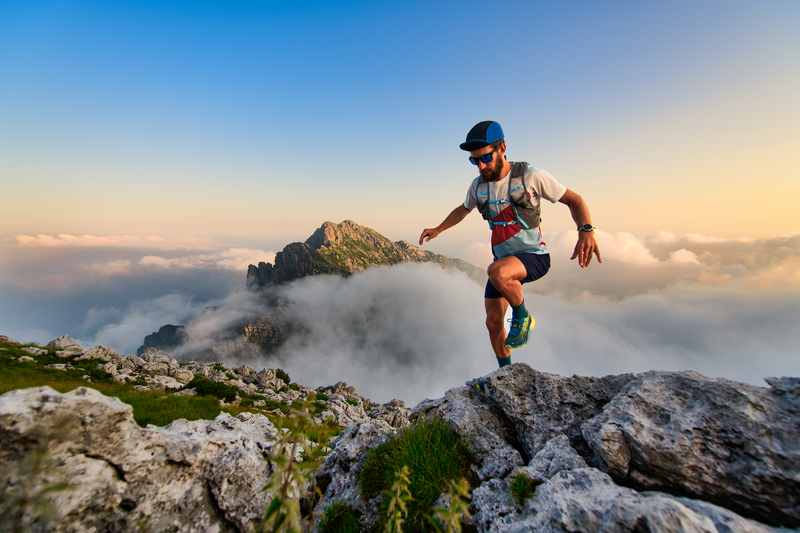
94% of researchers rate our articles as excellent or good
Learn more about the work of our research integrity team to safeguard the quality of each article we publish.
Find out more
ORIGINAL RESEARCH article
Front. Microbiol. , 13 July 2022
Sec. Microbial Physiology and Metabolism
Volume 13 - 2022 | https://doi.org/10.3389/fmicb.2022.956578
This article is part of the Research Topic Algal Photosynthesis View all 13 articles
Translocation of chloroplast-located genes to mitochondria or nucleus is considered to be a safety strategy that impedes mutation of photosynthetic genes and maintains their household function during evolution. The organelle translocation strategy is also developed in photosynthetic NDH-1 (pNDH-1) genes but its understanding is still far from complete. Here, we found that the mutation rate of the conserved pNDH-1 genes was gradually reduced but their selection pressure was maintained at a high level during evolution from cyanobacteria to angiosperm. By contrast, oxygenic photosynthesis-specific (OPS) pNDH-1 genes had an opposite trend, explaining the reason why they were transferred from the reactive oxygen species (ROS)-enriched chloroplast to the ROS-barren nucleus. Further, genome-wide sequence analysis supported the possibility that all conserved pNDH-1 genes lost in chloroplast genomes of Chlorophyceae and Pinaceae were transferred to the ROS-less mitochondrial genome as deduced from their truncated pNDH-1 gene fragments. Collectively, we propose that the organelle translocation strategy of pNDH-1 genes during evolution is necessary to maintain the function of the pNDH-1 complex as an important antioxidant mechanism for efficient photosynthesis.
During evolution of photosynthetic organisms, the rise of O2 and environmental stress inevitably results in the production of reactive oxygen species (ROS), which can trigger the mutation of genes under the low selection pressure (Ishikawa et al., 2008; Otten and Smeets, 2015). It is well known that in eukaryotic photosynthetic organisms, genes are located in the genomes of chloroplast, mitochondria, and nucleus. Considering the O2 content, the ROS concentrations of chloroplast, mitochondria, and nucleus under environmental stresses are assumed to be relatively abundant, less, and barren, respectively (Allen and Raven, 1996; Martin and Herrmann, 1998; Adams and Palmer, 2003; Foyer and Noctor, 2003; Laloi et al., 2004; Zhao et al., 2020). As a consequence, the organelle translocation is considered to be an important safety strategy that impedes mutation of photosynthetic genes and maintains their household function during evolution.
Complete sequencing of the chloroplast genomes of Marchantia polymorpha and Nicotiana tabacum unexpectedly demonstrates the presence of photosynthetic NDH-1 (pNDH-1) (hereafter referred to as pNDH-1) genes (Ohyama et al., 1986; Shinozaki et al., 1986). All complexes of pNDH-1 are involved in cyclic electron transfer around photosystem I (Bernát et al., 2011), which is an important antioxidant mechanism that balances the ATP/NADPH ratio required for the Calvin–Benson cycle and reduces the ROS production (Arnon, 1971; Kramer and Evans, 2011). They consist of conserved subunits (NdhA to NdhK) and oxygenic photosynthesis-specific (OPS) subunits (such as NdhL to NdhQ, NdhS, and NdhV) (Laughlin et al., 2019; Schuller et al., 2019, 2020; Pan et al., 2020; Zhang et al., 2020; Shen et al., 2022). It is found that these conserved genes of pNDH-1 reside in chloroplast genome (Ohyama et al., 1986; Shinozaki et al., 1986) but its OPS genes are translocated to the nucleus genome (Rumeau et al., 2005; Ishikawa et al., 2008; Shimizu et al., 2008; Suorsa et al., 2009; Yamamoto et al., 2011; Fan et al., 2015). However, little is known regarding the reason why these OPS pNDH-1 genes are translocated to the nucleus genome. In addition, genome-wide sequence analysis of pNDH-1 genes in Chlorophyceae and Pinaceae indicates that OPS pNDH-1 genes reside also in the nucleus genome, but all conserved pNDH-1 genes are lost entirely in the chloroplast and nucleus genomes (Wakasugi et al., 1994; Maul et al., 2002; Nystedt et al., 2013; Neale et al., 2014; Ranade et al., 2016; Lin et al., 2017). However, as yet, whether all these conserved pNDH-1 genes have been lost entirely or transferred to the mitochondrial genome remains a mystery.
Here, we calculated and analyzed the mutation frequency and selection pressure, explaining the reason why these OPS pNDH-1 genes were transferred from chloroplast to the nucleus. Further, we found the presence of conserved pNDH-1 gene fragments in mitochondrial genomes of Chlorophyceae and Pinaceae, implying that these conserved pNDH-1 genes lost in chloroplast genomes of Chlorophyceae and Pinaceae were transferred to their mitochondrial genomes. Collectively, our data provide new insights into the organelle translocation of pNDH-1 genes during evolution from cyanobacteria to angiosperm.
Phylogenetic tree was constructed based on rbcL gene of Methanogen, Cyanobacteria, Chlorophyceae, Bryophyta, Pinaceae, Monocots, and Dicots. The gene sequences of rbcL from different species were obtained from the National Center for Biotechnology Information (NCBI1). The names of the selected species and their GenBank accession numbers are listed in Supplementary Table 1. Sequence alignments were performed using MUSCLE (Edgar, 2004). The aligned dataset was analyzed in Data Analysis in Molecular Biology and Evolution (DAMBE) version 7 (Xia, 2018), and was converted into MEGA format. Unrooted phylogenetic trees were created using MEGA version 7 (Kumar et al., 2016) and maximum likelihood method (Felsenstein, 1981) with the bootstrap support of 1,000 replicates. Creating the phylogenetic tree, the parameters used were: complete deletion of gaps/missing data, distance model set to applying the nucleotide kimura-2-parameter, homogeneous pattern among lineages and uniform rates among sites and using the maximum composite likelihood model. The FigTree (v1.3.12) was used for the unrooted phylogenetic tree visualization.
The values of synonymous substitution rate (dS) and non-synonymous substitution rate (dN) were calculated using DNAsp6 software (Rozas et al., 2017). We first removed the terminators of each sequence and then used MUSCLE for sequence alignment, and the alignments of all these genes of pNDH-1 and rNDH-1 were converted into a codon alignment using TranslatorX (Abascal et al., 2010). The ambiguously aligned regions were excluded using trimAl v1.2 (Capella-Gutiérrez et al., 2009) and the results were exported as a Fasta file. Having opened the exported file with DNAsp6, we set the genomic state and chromosomal location, assigned the coding regions, and calculated the dS and dN values. The average values were calculated using SigmaPlot 14.0.
Calculation of amino acid conservative substitution (Kc) was carried out with the aid of a pipeline SAMEM v.0.83.3 The SAMEM package (Gunbin et al., 2011) has a major path for the gene evolution analysis. We divided these 20 amino acids into two groups according to their physicochemical properties, such as volume (RQEHILKMFWYV ANDCGPST), which are related to protein function. Amino acid substitutions within groups are called conservative substitutions (Hanada et al., 2007). The general step and method of calculating Kc are as follows: translation of nucleic acid sequence into amino acid sequence by Transeq, multiple alignment of amino acid sequences by the Mafft 6.717 algorithm (Katoh and Toh, 2008) using the BLOSUM 62 matrix (Henikoff and Henikoff, 1992), and models of amino acid substitutions were calculated based on multiple alignment using the Modelestimator algorithm (Arvestad, 2006), phylogenetic trees were calculated on the basis of the replacement model using FastTree 2.1.1 (Price et al., 2010), ancestral gene sequences are reconstructed based on gapless alignments of codons using FASTML (Pupko et al., 2002), calculation of Kc using Zhang’s (2000) method (the HON-NEW program). For each of 531 properties (Kawashima et al., 2008), amino acids are divided into classes by k-means clustering using R.
Homology search was performed by comparing amino acid sequences with sequences in local nucleotide databases (TBLASTN). Eleven Arabidopsis thaliana pNDH-1 gene sequences were used as templates for TBLASTN searches of Chlorophyceae and Pinaceae mitochondrial genomes. The TBLASTN expectation value threshold E was altered to 10 to allow for a less stringent alignment search.
The data underlying this article are available within the NCBI GenBank database,4 and all GenBank accession numbers are listed in Supplementary Table 1. The alignments analyzed in this study are available in the article’s online Supplementary Figures 2–4.
Genome-wide sequence analysis suggests that pNDH-1 originates from a group 4 membrane-bound [NiFe] hydrogenase (Böhm et al., 1990; Peltier et al., 2016) and evolves from archaea (gray tree branches in Figure 1) to prokaryote (blue tree branches in Figure 1) and to eukaryotic photosynthetic organisms (green tree branches in Figure 1). In eukaryotic photosynthetic organisms except Chlorophyceae, Pinaceae and Orchidaceae, conserved pNDH-1 genes reside in chloroplast genome, whereas OPS pNDH-1 genes are transferred to the nucleus genome (Rumeau et al., 2005; Ishikawa et al., 2008; Shimizu et al., 2008; Suorsa et al., 2009; Yamamoto et al., 2011; Fan et al., 2015). In addition, in eukaryotic Chlorophyceae and Pinaceae (red species name in Figure 1), OPS pNDH-1 genes reside also in the nucleus genome, but all conserved pNDH-1 genes are lost entirely in their chloroplast and nucleus genomes (Wakasugi et al., 1994; Maul et al., 2002; Lin et al., 2017). The below investigations will try to explain the reason why these OPS pNDH-1 genes in eukaryotic photosynthetic organisms except Chlorophyceae, Pinaceae, and Orchidaceae were transferred from chloroplast genome to the nucleus genome and unravel the mystery whether conserved pNDH-1 genes of Chlorophyceae and Pinaceae have been lost entirely or transferred to the mitochondrial genome.
Figure 1. Phylogenetic tree marks these important events of photosynthetic NDH-1 (pNDH-1) during evolution from archaea to eukaryote. Phylogenetic tree was constructed based on marker gene rbcL. The pNDH-1 originates from a group 4 membrane-bound [NiFe] hydrogenase and evolves from archaea (gray tree branches) to prokaryote (blue tree branches) and to eukaryotic photosynthetic organisms (green tree branches). In chloroplast, the species with pNDH-1 are represented by black letters, and the species without pNDH-1 are represented by red letters.
During the evolution process from cyanobacteria to dicots, we found that the mutation rate of conserved pNDH-1 genes was gradually decreased, as deduced from the results of dS (Figures 2A–F). This was supported by the data of Kc (Supplementary Figure 1). As a consequence, the mutation rate of conserved pNDH-1 genes has a trend of gradual decrease during evolution.
Figure 2. The trend of mutation rate of pNDH-1 genes during evolution from cyanobacteria to dicots. The mutation rate is deduced from the values of synonymous substitution rate (dS). The Scatter plot shows the respective dS values of conserved genes and oxygenic photosynthesis-specific (OPS) genes of pNDH-1 in methanogen (A), cyanobacteria (B), bryophyta (C), monocots (D), and dicots (E). The pink dots represent conserved genes and the green triangles represent OPS genes. Box plot shows the average dS values of conserved genes and OPS genes of pNDH-1 in methanogen, cyanobacteria, bryophyta, monocots, and dicots (F). The box represents the values between the quartiles and the black lines inside the box represent the median value.
To fully understand the trend of conserved pNDH-1 genes during evolution, we calculated the ratio of dN to dS. Our data indicated that all dN/dS ratios of conserved pNDH-1 genes were less than 1 (Figures 3A–F), indicating that the evolutional rate of all these conserved pNDH-1 genes was relatively slow and under the purifying selection (Endo et al., 1997; Messier and Stewart, 1997). Further, under the purifying selection, the magnitude of dN/dS values could reflect the selection pressure of these conserved pNDH-1 genes (Berg and Kurland, 2000). If the dN/dS ratio was more close to 0, the selection pressure was higher, whereas if the ratio was more close to 1, the selection pressure was lower. We found that the selection pressure of conserved pNDH-1 genes was increased during evolution from cyanobacteria to dicots, as deduced from the results of dN/dS values (Figures 3A–F). Collectively, we propose that conserved pNDH-1 genes have an evolutionary trend that the mutation rate was gradually decreased but the selection pressure was maintained at a relatively high level.
Figure 3. The trend of selection pressure of pNDH-1 genes during evolution from cyanobacteria to dicots. The selection pressure is deduced from the values of non-synonymous substitution rate (dN)/dS. Scatter plot shows the respective dN/dS values of conserved genes and OPS genes of pNDH-1 in methanogen (A), cyanobacteria (B), bryophyta (C), monocots (D), and dicots (E). The pink dots represent conserved genes and the green triangles represent OPS genes. Box plot shows the average dN/dS values of conserved genes and OPS genes of pNDH-1 in methanogen, cyanobacteria, bryophyta, monocots, and dicots (F). The box represents the values between the quartiles and the black lines inside the box represent the median value.
Unexpectedly, the mutation rate of OPS pNDH-1 genes was increased and kept at a high level during evolution from cyanobacteria to dicots, as deduced from the dS values (Figures 2A–F). Meanwhile, the selection pressure of OPS pNDH-1 genes was deceased during evolution from cyanobacteria to dicots, as deduced from the data of dN/dS values (Figures 3A–F). Taking all these results together, we can clearly find that OPS and conserved pNDH-1 genes have a distinctly different trend of their mutation rate and selection pressure during evolution.
To unravel the mystery whether these conserved pNDH-1 genes have been lost entirely or transferred to the mitochondrial genome, we conducted the sequence searches in the mitochondrial genomes of Chlorophyceae and Pinaceae using pNDH-1 sequences of Arabidopsis thaliana as templates. Our results revealed that the fragments of conserved pNDH-1 genes lost in the chloroplast genomes of Chlorophyceae and Pinaceae were found to be in their mitochondrial genomes (Figure 4 and Supplementary Figures 2–4). Collectively, we propose that these conserved pNDH-1 genes lost in the chloroplast genomes of Chlorophyceae and Pinaceae were transferred to their mitochondrial genomes.
Figure 4. Comparison of Chlorophyceae and Pinaceae mitochondrial genomes with Arabidopsis thaliana chloroplast pNDH-1 genes. The arrows indicate coding regions. The arrowheads indicate the direction of the genes.
Consistent with the conserved pNDH-1 genes, the mutation rate of respiratory NDH-1 (rNDH-1) genes was gradually decreased but their selection pressure was maintained at a relatively high level during evolution from methanogen to dicots (pink in Figures 5A,B). However, the mutation rate and selection pressure of rNDH-1 genes in Chlorophyceae and Pinaceae did not follow the trend of rNDH-1 during evolution from methanogen to dicots (red in Figures 5A,B). It is reasonable to infer that the transfer of chloroplast pNDH-1 genes of Chlorophyceae and Pinaceae to their mitochondrial genomes results in mitochondrial DNA rearrangement, thereby increasing the mutation rate of rNDH-1 genes and relatively decreasing their selection pressure. This supports the conclusion that these conserved pNDH-1 genes lost in chloroplast genomes of Chlorophyceae and Pinaceae were transferred to their mitochondrial genomes.
Figure 5. The trends of mutation rate and selection pressure of rNDH-1 genes during evolution from chlorophyceae to dicots. The mutation rate and selection pressure are deduced from the values of dS and dN/dS, respectively. Boxplots show the average dS (A) and dN/dS (B) values of rNDH-1 in methanogen, Chlorophyceae, bryophyta, Pinaceae, monocots, and dicots. The box represents the values between the quartiles and the black lines inside the box represent the median value.
During the evolution from prokaryotic cyanobacteria to eukaryotic angiosperm, organelle translocation is considered to be an important safety strategy that impedes mutation of photosynthetic genes and maintain their household function (Baldauf and Palmer, 1990; Gantt et al., 1991; Martin et al., 1998; Adams and Palmer, 2003; Rokka et al., 2005). Such organelle translocation is also found to be a universal safety strategy, for example, the mitochondrial genome contains between 1 and 10% of chloroplast sequences in different seed plants (Stern and Lonsdale, 1982; Joyce and Gray, 1989; Wang et al., 2007, 2012). In eukaryotic photosynthetic organisms except Chlorophyceae, Pinaceae, and Orchidaceae, OPS pNDH-1 genes are found to transfer from chloroplast genome to the nucleus genome during evolution (Rumeau et al., 2005; Ishikawa et al., 2008; Shimizu et al., 2008; Suorsa et al., 2009; Yamamoto et al., 2011; Fan et al., 2015), although conserved pNDH-1 genes still reside in chloroplast genome (Ohyama et al., 1986; Shinozaki et al., 1986).
The results of this study found that two distinctly different strategies have been developed by conserved and OPS pNDH-1 genes to impede their mutations and maintain their functions (Figures 2, 3 and Supplementary Figure 1). Conserved pNDH-1 genes develop a safety strategy via decreasing their mutation rate and increasing their selection pressure, while OPS pNDH-1 genes develop another safety strategy via transferring them from the chloroplast genome to a relatively safe nucleus genome (Figures 2, 3 and Supplementary Figure 1). It is worthy of note that according to Mahler’s ratchet effect (Muller, 1964), gene recombination is lacked in chloroplast because of no sexual reproduction. When a gene is successfully transferred from chloroplast to nuclear, in other words, from asexual to sexual, gene recombination is restored and provides a chance to get rid of the fate crisis of gene mutation, reinforcing the conclusion that the nucleus is much safer than the chloroplast. Collectively, during evolution of photosynthetic organisms, these two distinctly different strategies jointly maintain the function of pNDH-1 as an important antioxidant mechanism for efficient photosynthesis through impeding mutation of its conserved and OPS genes.
Consistent with the previously reported Orchidaceae (Lin et al., 2015, 2017), these conserved pNDH-1 genes lost in Chlorophyceae and Pinaceae were transferred from chloroplast genome to the mitochondrial genome as deduced from their common gene fragments (Figure 4 and Supplementary Figures 2–4) and the abnormal mutation rate and selection pressure of rNDH-1 genes (Figure 5). It has been reported that Chlorophyceae green algae frequently meet with various environmental challenges, such as fluctuations in nutrient, light availability, and temperature, in their natural habitat (Varshney et al., 2015). Consistent with this situation, the chloroplast DNA and mitochondrial DNA of Chlorophyceae underwent substantial changes in their architecture (such as gene losses and genome expansion in the case of mitochondrial DNA) during evolution (Turmel et al., 2002; Wodniok et al., 2011). Under this background, it is reasonable to infer that these conserved pNDH-1 genes of Chlorophyceae are lost in chloroplast and are transferred to the mitochondrial genome.
Compared with the land plants, Bryophyta and Pteridophyte, the trees in Pinaceae grow quite high (Graham et al., 1995), implying that they have an efficient photosynthesis and produce more O2. Consistent with the hypothesis, Pinaceae underwent an O2-rise phase (Savard et al., 1994; Berner, 2006) and may produce abundant ROS in chloroplast but less ROS in mitochondria under environmental stresses (Foyer and Noctor, 2003; Laloi et al., 2004; Zhao et al., 2020). As a consequence, it appears plausible that pNDH-1 genes lost in Pinaceae are transferred from O2-enriched chloroplast to the O2-less mitochondria as oxygen-consuming organelle.
Based on the aforementioned analysis, we propose that translocation of pNDH-1 genes from chloroplast genome to the nucleus genome or mitochondrial genome is important to maintain the architecture and household function of pNDH-1 during evolution. As a consequence, the function of pNDH-1 as an important antioxidant mechanism can reduce ROS production necessary for the survival of eukaryotic photosynthetic organisms in aerobic environment.
The raw data supporting the conclusions of this article will be made available by the authors, without undue reservation.
WM conceived and designed the project. JY and JZ performed the bioinformatics analysis. JY, ZR, LW, and WM interpreted the data and wrote the manuscript. All authors read and approved the final manuscript.
This work was supported by the National Natural Science Foundation of China (grant nos. 32170257, 32070381, and 31770259), and Shanghai Science and Technology Committee (grant nos. 22010503500 and 20ZR1441300).
The authors declare that the research was conducted in the absence of any commercial or financial relationships that could be construed as a potential conflict of interest.
All claims expressed in this article are solely those of the authors and do not necessarily represent those of their affiliated organizations, or those of the publisher, the editors and the reviewers. Any product that may be evaluated in this article, or claim that may be made by its manufacturer, is not guaranteed or endorsed by the publisher.
The Supplementary Material for this article can be found online at: https://www.frontiersin.org/articles/10.3389/fmicb.2022.956578/full#supplementary-material
dN, non-synonymous substitution rate; dS, synonymous substitution rate; Kc, amino acid conservative substitution; OPS, oxygenic photosynthesis-specific; pNDH-1, photosynthetic NDH-1; rNDH-1, respiratory NDH-1; ROS, reactive oxygen species.
Abascal, F., Zardoya, R., and Telford, M. J. (2010). TranslatorX: multiple alignment of nucleotide sequences guided by amino acid translations. Nucleic Acids Res. 38, W7–W13. doi: 10.1093/nar/gkq291
Adams, K. L., and Palmer, J. D. (2003). Evolution of mitochondrial gene content: gene loss and transfer to the nucleus. Mol. Phylogenet. Evol. 29, 380–395. doi: 10.1016/s1055-7903(03)00194-5
Allen, J. F., and Raven, J. A. (1996). Free-radical-induced mutation vs redox regulation: costs and benefits of genes in organelles. J. Mol. Evol. 42, 482–492. doi: 10.1007/BF02352278
Arnon, D. I. (1971). The light reactions of photosynthesis. Proc. Natl. Acad. Sci. U.S.A. 68, 2883–2892. doi: 10.1073/pnas.68.11.2883
Arvestad, L. (2006). Efficient methods for estimating amino acid replacement rates. J. Mol. Evol. 62, 663–673. doi: 10.1007/s00239-004-0113-9
Baldauf, S. L., and Palmer, J. D. (1990). Evolutionary transfer of the chloroplast tufA gene to the nucleus. Nature 344, 262–265. doi: 10.1038/344262a0
Berg, O. G., and Kurland, C. G. (2000). Why mitochondrial genes are most often found in nuclei. Mol. Biol. Evol. 17, 951–961. doi: 10.1093/oxfordjournals.molbev.a026376
Bernát, G., Appel, J., Ogawa, T., and Rögner, M. (2011). Distinct roles of multiple NDH-1 complexes in the cyanobacterial electron transport network as revealed by kinetic analysis of P700+ reduction in various ndh-deficient mutants of Synechocystis sp. strain PCC6803. J. Bacteriol. 193, 292–295. doi: 10.1128/JB.00984-10
Berner, R. A. (2006). GEOCARBSULF: a combined model for Phanerozoic atmospheric O2 and CO2. Geochim. Cosmochim. Acta 70, 5653–5664. doi: 10.1016/j.gca.2005.11.032
Böhm, R., Sauter, M., and Böck, A. (1990). Nucleotide sequence and expression of an operon in Escherichia coli coding for formate hydrogen lyase components. Mol. Microbiol. 4, 231–243. doi: 10.1111/j.1365-2958.1990.tb00590.x
Capella-Gutiérrez, S., Silla-Martínez, J. M., and Gabaldón, T. (2009). trimAl: a tool for automated alignment trimming in large-scale phylogenetic analyses. Bioinformatics 25, 1972–1973. doi: 10.1093/bioinformatics/btp348
Edgar, R. C. (2004). MUSCLE: multiple sequence alignment with high accuracy and high throughput. Nucleic Acids Res. 32, 1792–1797. doi: 10.1093/nar/gkh340
Endo, T., Imanishi, T., Gojobori, T., and Inoko, H. (1997). Evolutionary significance of intra-genome duplications on human chromosomes. Gene 205, 19–27. doi: 10.1016/S0378-1119(97)00478-2
Fan, X., Zhang, J., Li, W., and Peng, L. (2015). The NdhV subunit is required to stabilize the chloroplast NADH dehydrogenase-like complex in Arabidopsis. Plant J. 82, 221–231. doi: 10.1111/tpj.12807
Felsenstein, J. (1981). Evolutionary trees from DNA sequences: a maximum likelihood approach. J. Mol. Evol. 17, 368–376. doi: 10.1007/bf01734359
Foyer, C. H., and Noctor, G. (2003). Redox sensing and signalling associated with reactive oxygen in chloroplasts, peroxisomes and mitochondria. Physiol. Plant 119, 355–364. doi: 10.1034/j.1399-3054.2003.00223.x
Gantt, J. S., Baldauf, S. L., Calie, P. J., Weeden, N. F., and Palmer, J. D. (1991). Transfer of rpl22 to the nucleus greatly preceded its loss from the chloroplast and involved the gain of an intron. EMBO J. 10, 3073–3078. doi: 10.1002/j.1460-2075.1991.tb07859.x
Graham, J. B., Aguilar, N. M., Dudley, R., and Gans, C. (1995). Implications of the late Palaeozoic oxygen pulse for physiology and evolution. Nature 375, 117–120. doi: 10.1038/375117a0
Gunbin, K. V., Suslov, V. V., Genaev, M. A., and Afonnikov, D. A. (2011). Computer system for analysis of molecular evolution modes (SAMEM): analysis of molecular evolution modes at deep inner branches of the phylogenetic tree. In Silico Biol. 11, 109–123. doi: 10.3233/ISB-2012-0446
Hanada, K., Shiu, S. H., and Li, W. H. (2007). The nonsynonymous/synonymous substitution rate ratio versus the radical/conservative replacement rate ratio in the evolution of mammalian genes. Mol. Biol. Evol. 24, 2235–2241. doi: 10.1093/molbev/msm152
Henikoff, S., and Henikoff, J. G. (1992). Amino acid substitution matrices from protein blocks. Proc. Natl. Acad. Sci. U.S.A. 89, 10915–10919. doi: 10.1073/pnas.89.22.10915
Ishikawa, K., Takenaga, K., Akimoto, M., Koshikawa, N., Yamaguchi, A., Imanishi, H., et al. (2008). ROS-generating mitochondrial DNA mutations can regulate tumor cell metastasis. Science 320, 661–664. doi: 10.1126/science.1156906
Joyce, P. B., and Gray, M. W. (1989). Chloroplast-like transfer RNA genes expressed in wheat mitochondria. Nucleic Acids Res. 17, 5461–5476. doi: 10.1093/nar/17.14.5461
Katoh, K., and Toh, H. (2008). Recent developments in the MAFFT multiple sequence alignment program. Brief. Bioinform. 9, 286–298. doi: 10.1093/bib/bbn013
Kawashima, S., Pokarowski, P., Pokarowska, M., Kolinski, A., Katayama, T., and Kanehisa, M. (2008). AAindex: amino acid index database, progress report 2008. Nucleic Acids Res. 36, D202–D205. doi: 10.1093/nar/gkm998
Kramer, D. M., and Evans, J. R. (2011). The importance of energy balance in improving photosynthetic productivity. Plant Physiol. 155, 70–78. doi: 10.1104/pp.110.166652
Kumar, S., Stecher, G., and Tamura, K. (2016). MEGA7: molecular evolutionary genetics analysis version 7.0 for bigger datasets. Mol. Biol. Evol. 33, 1870–1874. doi: 10.1093/molbev/msw054
Laloi, C., Apel, K., and Danon, A. (2004). Reactive oxygen signalling: the latest news. Curr. Opin. Plant Biol. 7, 323–328. doi: 10.1016/j.pbi.2004.03.005
Laughlin, T. G., Bayne, A. N., Trempe, J. F., Savage, D. F., and Davies, K. M. (2019). Structure of the complex I-like molecule NDH of oxygenic photosynthesis. Nature 566, 411–414. doi: 10.1038/s41586-019-0921-0
Lin, C. S., Chen, J. J. W., Chiu, C. C., Hsiao, H. C. W., Yang, C. J., Jin, X. H., et al. (2017). Concomitant loss of NDH complex-related genes within chloroplast and nuclear genomes in some orchids. Plant J. 90, 994–1006. doi: 10.1111/tpj.13525
Lin, C. S., Chen, J. J. W., Huang, Y. T., Chan, M. T., Daniell, H., Chang, W. J., et al. (2015). The location and translocation of ndh genes of chloroplast origin in the Orchidaceae family. Sci. Rep. 5, 1–10. doi: 10.1038/srep09040
Martin, W., and Herrmann, R. G. (1998). Gene transfer from organelles to the nucleus: how much, what happens, and why? Plant Physiol. 118, 9–17. doi: 10.1104/pp.118.1.9
Martin, W., Stoebe, B., Goremykin, V., Hansmann, S., Hasegawa, M., and Kowallik, K. V. (1998). Gene transfer to the nucleus and the evolution of chloroplasts. Nature 393, 162–165. doi: 10.1038/30234
Maul, J. E., Lilly, J. W., Cui, L., dePamphilis, C. W., Miller, W., Harris, E. H., et al. (2002). The Chlamydomonas reinhardtii plastid chromosome: islands of genes in a sea of repeats. Plant Cell 14, 2659–2679. doi: 10.1105/tpc.006155
Messier, W., and Stewart, C. B. (1997). Episodic adaptive evolution of primate lysozymes. Nature 385, 151–154. doi: 10.1038/385151a0
Muller, H. J. (1964). The relation of recombination to mutational advance. Mutat. Res. 1, 2–9. doi: 10.1016/0027-5107(64)90047-8
Neale, D. B., Wegrzyn, J. L., Stevens, K. A., Zimin, A. V., Puiu, D., Crepeau, M. W., et al. (2014). Decoding the massive genome of loblolly pine using haploid DNA and novel assembly strategies. Genome Biol. 15:R59. doi: 10.1186/gb-2014-15-3-r59
Nystedt, B., Street, N. R., Wetterbom, A., Zuccolo, A., Lin, Y. C., Scofield, D. G., et al. (2013). The Norway spruce genome sequence and conifer genome evolution. Nature 497, 579–584. doi: 10.1038/nature12211
Ohyama, K., Fukuzawa, H., Kohchi, T., Shirai, H., Sano, T., Sano, S., et al. (1986). Chloroplast gene organization deduced from complete sequence of liverwort Marchantia polymorpha chloroplast DNA. Nature 322, 572–574. doi: 10.1038/322572a0
Otten, A. B., and Smeets, H. J. (2015). Evolutionary defined role of the mitochondrial DNA in fertility, disease and ageing. Hum. Reprod. Update 21, 671–689. doi: 10.1093/humupd/dmv024
Pan, X., Cao, D., Xie, F., Xu, F., Su, X., Mi, H., et al. (2020). Structural basis for electron transport mechanism of complex I-like photosynthetic NAD(P)H dehydrogenase. Nat. Commun. 11:610. doi: 10.1038/s41467-020-14456-0
Peltier, G., Aro, E. M., and Shikanai, T. (2016). NDH-1 and NDH-2 plastoquinone reductases in oxygenic photosynthesis. Annu. Rev. Plant Biol. 67, 55–80. doi: 10.1146/annurev-arplant-043014-114752
Price, M. N., Dehal, P. S., and Arkin, A. P. (2010). FastTree 2–approximately maximum-likelihood trees for large alignments. PLoS One 5:e9490. doi: 10.1371/journal.pone.0009490
Pupko, T., Pe’er, I., Hasegawa, M., Graur, D., and Friedman, N. (2002). A branch-and-bound algorithm for the inference of ancestral amino-acid sequences when the replacement rate varies among sites: application to the evolution of five gene families. Bioinformatics 18, 1116–1123. doi: 10.1093/bioinformatics/18.8.1116
Ranade, S. S., Garcia-Gil, M. R., and Rossello, J. A. (2016). Non-functional plastid ndh gene fragments are present in the nuclear genome of Norway spruce (Picea abies L. Karsch): insights from in silico analysis of nuclear and organellar genomes. Mol. Genet. Genomics 291, 935–941. doi: 10.1007/s00438-015-1159-7
Rokka, A., Suorsa, M., Saleem, A., Battchikova, N., and Aro, E. M. (2005). Synthesis and assembly of thylakoid protein complexes: multiple assembly steps of photosystem II. Biochem. J. 388, 159–168. doi: 10.1042/BJ20042098
Rozas, J., Ferrer-Mata, A., Sanchez-DelBarrio, J. C., Guirao-Rico, S., Librado, P., Ramos-Onsins, S. E., et al. (2017). DnaSP 6: DNA sequence polymorphism analysis of large data sets. Mol. Biol. Evol. 34, 3299–3302. doi: 10.1093/molbev/msx248
Rumeau, D., Becuwe-Linka, N., Beyly, A., Louwagie, M., Garin, J., and Peltier, G. (2005). New subunits NDH-M, -N, and -O, encoded by nuclear genes, are essential for plastid NDH complex functioning in higher plants. Plant Cell 17, 219–232. doi: 10.1105/tpc.104.028282
Savard, L., Li, P., Strauss, S. H., Chase, M. W., Michaud, M., and Bousquet, J. (1994). Chloroplast and nuclear gene sequences indicate late Pennsylvanian time for the last common ancestor of extant seed plants. Proc. Natl. Acad. Sci. U.S.A. 91, 5163–5167. doi: 10.1073/pnas.91.11.5163
Schuller, J. M., Birrell, J. A., Tanaka, H., Konuma, T., Wulfhorst, H., Cox, N., et al. (2019). Structural adaptations of photosynthetic complex I enable ferredoxin-dependent electron transfer. Science 363, 257–260. doi: 10.1126/science.aau3613
Schuller, J. M., Saura, P., Thiemann, J., Schuller, S. K., Gamiz-Hernandez, A. P., Kurisu, G., et al. (2020). Redox-coupled proton pumping drives carbon concentration in the photosynthetic complex I. Nat. Commun. 11:494. doi: 10.1038/s41467-020-14347-4
Shen, L., Tang, K., Wang, W., Wang, C., Mao, J., Zhou, T., et al. (2022). Architecture of the chloroplast PSI–NDH supercomplex in Hordeum vulgare. Nature 601, 649–654. doi: 10.1038/s41586-021-04277-6
Shimizu, H., Peng, L., Myouga, F., Motohashi, R., Shinozaki, K., and Shikanai, T. (2008). CRR23/NdhL is a subunit of the chloroplast NAD(P)H dehydrogenase complex in Arabidopsis. Plant Cell Physiol. 49, 835–842. doi: 10.1093/pcp/pcn058
Shinozaki, K., Ohme, M., Tanaka, M., Wakasugi, T., Hayashida, N., Matsubayashi, T., et al. (1986). The complete nucleotide sequence of the tobacco chloroplast genome: its gene organization and expression. EMBO J. 5, 2043–2049. doi: 10.1002/j.1460-2075.1986.tb04464.x
Stern, D. B., and Lonsdale, D. M. (1982). Mitochondrial and chloroplast genomes of maize have a 12-kilobase DNA sequence in common. Nature 299, 698–702. doi: 10.1038/299698a0
Suorsa, M., Sirpio, S., and Aro, E. M. (2009). Towards characterization of the chloroplast NAD(P)H dehydrogenase complex. Mol. Plant 2, 1127–1140. doi: 10.1093/mp/ssp052
Turmel, M., Otis, C., and Lemieux, C. (2002). The chloroplast and mitochondrial genome sequences of the charophyte Chaetosphaeridium globosum: insights into the timing of the events that restructured organelle DNAs within the green algal lineage that led to land plants. Proc. Natl. Acad. Sci. U.S.A. 99, 11275–11280. doi: 10.1073/pnas.162203299
Varshney, P., Mikulic, P., Vonshak, A., Beardall, J., and Wangikar, P. P. (2015). Extremophilic micro-algae and their potential contribution in biotechnology. Bioresour. Technol. 184, 363–372. doi: 10.1016/j.biortech.2014.11.040
Wakasugi, T., Tsudzuki, J., Ito, S., Nakashima, K., Tsudzuki, T., and Sugiura, M. (1994). Loss of all ndh genes as determined by sequencing the entire chloroplast genome of the black pine Pinus thunbergii. Proc. Natl. Acad. Sci. U.S.A. 91, 9794–9798. doi: 10.1073/pnas.91.21.9794
Wang, D., Rousseau-Gueutin, M., and Timmis, J. N. (2012). Plastid sequences contribute to some plant mitochondrial genes. Mol. Biol. Evol. 29, 1707–1711. doi: 10.1093/molbev/mss016
Wang, D., Wu, Y. W., Shih, A. C. C., Wu, C. S., Wang, Y. N., and Chaw, S. M. (2007). Transfer of chloroplast genomic DNA to mitochondrial genome occurred at least 300 MYA. Mol. Biol. Evol. 24, 2040–2048. doi: 10.1093/molbev/msm133
Wodniok, S., Brinkmann, H., Glöckner, G., Heidel, A. J., Philippe, H., Melkonian, M., et al. (2011). Origin of land plants: do conjugating green algae hold the key? BMC Evol. Biol. 11:104. doi: 10.1186/1471-2148-11-104
Xia, X. (2018). DAMBE7: new and improved tools for data analysis in molecular biology and evolution. Mol. Biol. Evol. 35, 1550–1552. doi: 10.1093/molbev/msy073
Yamamoto, H., Peng, L., Fukao, Y., and Shikanai, T. (2011). An Src homology 3 domain-like fold protein forms a ferredoxin binding site for the chloroplast NADH dehydrogenase-like complex in Arabidopsis. Plant Cell 23, 1480–1493. doi: 10.1105/tpc.110.080291
Zhang, C., Shuai, J., Ran, Z., Zhao, J., Wu, Z., Liao, R., et al. (2020). Structural insights into NDH-1 mediated cyclic electron transfer. Nat. Commun. 11:888. doi: 10.1038/s41467-020-14732-z
Zhang, J. (2000). Rates of conservative and radical nonsynonymous nucleotide substitutions in mammalian nuclear genes. J. Mol. Evol. 50, 56–68. doi: 10.1007/s002399910007
Keywords: organelle translocation, photosynthetic NDH-1, mitochondrial NDH-1, evolutionary events, plant evolution
Citation: Yu J, Ran Z, Zhang J, Wei L and Ma W (2022) Genome-Wide Insights Into the Organelle Translocation of Photosynthetic NDH-1 Genes During Evolution. Front. Microbiol. 13:956578. doi: 10.3389/fmicb.2022.956578
Received: 30 May 2022; Accepted: 20 June 2022;
Published: 13 July 2022.
Edited by:
Hui Wu, East China University of Science and Technology, ChinaReviewed by:
Guangye Han, Institute of Botany (CAS), ChinaCopyright © 2022 Yu, Ran, Zhang, Wei and Ma. This is an open-access article distributed under the terms of the Creative Commons Attribution License (CC BY). The use, distribution or reproduction in other forums is permitted, provided the original author(s) and the copyright owner(s) are credited and that the original publication in this journal is cited, in accordance with accepted academic practice. No use, distribution or reproduction is permitted which does not comply with these terms.
*Correspondence: Lanzhen Wei, d2VpbHpAc2hudS5lZHUuY24=; Weimin Ma, d21hQHNobnUuZWR1LmNu
Disclaimer: All claims expressed in this article are solely those of the authors and do not necessarily represent those of their affiliated organizations, or those of the publisher, the editors and the reviewers. Any product that may be evaluated in this article or claim that may be made by its manufacturer is not guaranteed or endorsed by the publisher.
Research integrity at Frontiers
Learn more about the work of our research integrity team to safeguard the quality of each article we publish.