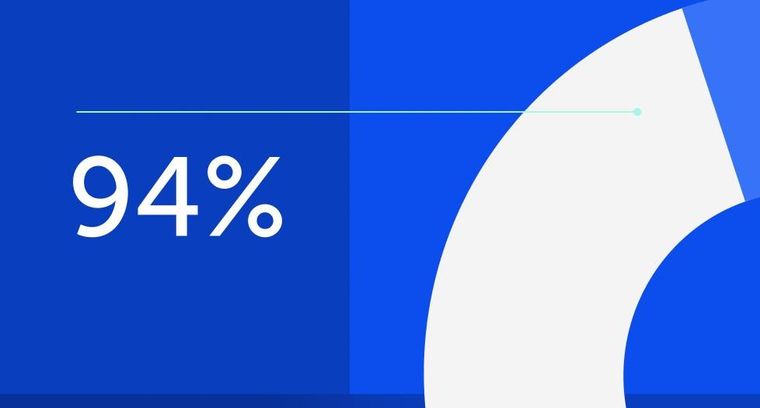
94% of researchers rate our articles as excellent or good
Learn more about the work of our research integrity team to safeguard the quality of each article we publish.
Find out more
REVIEW article
Front. Microbiol., 02 August 2022
Sec. Food Microbiology
Volume 13 - 2022 | https://doi.org/10.3389/fmicb.2022.951182
This article is part of the Research TopicGenomics and metagenomics approaches for food value chain quality, safety, and product developmentView all 11 articles
Biopreservation is a sustainable approach to improve food safety and maintain or extend food shelf life by using beneficial microorganisms or their metabolites. Over the past 20 years, omics techniques have revolutionised food microbiology including biopreservation. A range of methods including genomics, transcriptomics, proteomics, metabolomics and meta-omics derivatives have highlighted the potential of biopreservation to improve the microbial safety of various foods. This review shows how these approaches have contributed to the selection of biopreservation agents, to a better understanding of the mechanisms of action and of their efficiency and impact within the food ecosystem. It also presents the potential of combining omics with complementary approaches to take into account better the complexity of food microbiomes at multiple scales, from the cell to the community levels, and their spatial, physicochemical and microbiological heterogeneity. The latest advances in biopreservation through omics have emphasised the importance of considering food as a complex and dynamic microbiome that requires integrated engineering strategies to increase the rate of innovation production in order to meet the safety, environmental and economic challenges of the agri-food sector.
Foods of animal or plant origins are complex ecosystems, rich in nutrients, with physicochemical characteristics enabling microbial growth during processing and storage. These ecosystems are colonised by microbial communities that can include pathogenic or spoilage microorganisms but also beneficial ones. The consumption of food contaminated with pathogens is an important cause of morbidity and mortality worldwide. Every year, approximately 600 million people – 1 in 10 people – get sick from foodborne pathogens, 420,000 of whom die. Human damage caused by foodborne pathogens results in colossal economic losses amounting to USD 110 billion due to lost productivity and health expenses (World Health Organization, 2015). Spoilage organisms are responsible for colour, odour, texture, taste or packaging defects leading to inedible products. Food microbial contaminants (spoilage as well as pathogenic microorganisms) contribute to global food loss and waste. According to the Food and Agriculture Organization (FAO), food waste occurs during the retail and consumption stages while food loss occurs after harvest or slaughter until retail (FAO, 2019). The causes of food waste and loss are numerous but a significant part of food destruction linked to microbial contamination is due to non-compliance with pathogen-related regulations or spoilage. At the European Union level, 20% of total available food is lost or wasted, fruits and vegetables being the most impacted category (43.5% of the food group), ahead of meat and fish products (26.3%; Caldeira et al., 2019). Worldwide, one-third of food produced for human consumption, about 1.3 billion tonnes per year, is estimated to be lost or wasted along the food supply chain (HPLE, 2014), while about 12% of the world population suffers from hunger (Agriculture and Economic Development Analysis Division, 2013). Reducing food wastage is thus crucial not only for ethical reasons but also for economic reasons. Food loss and waste are responsible for direct costs of about USD one trillion every year, but hidden costs extend much further. Indeed, global costs (including environmental, social, and economic costs) are evaluated by the FAO to amount to USD 2.6 trillion per year (FAO, 2014).
In addition, as food waste is correlated with high greenhouse gas emissions, reducing the undesirable impact of microorganisms has become a major objective in a situation of climate emergency. In this context, microorganisms also offer potential levers of action and can represent real opportunities for resolving food safety issues (Cavicchioli et al., 2019). In the global food system, approaches based on the barrier properties of biological systems appear attractive because of their efficiency and sustainability. These approaches are grouped under various names including biosanitation, biocontrol, bioprotection, and biopreservation. In this review, we will refer to food biopreservation, which is based on the hurdle technology that consists in using microorganisms (often lactic acid bacteria) as protective cultures and/or their metabolites to optimise the microbiological quality and shelf life of food by ensuring safety or reducing food waste, as defined by Stiles (Stiles, 1996). The use of protective cultures is often considered as an alternative to chemical additives or as a replacement for certain ingredients. Therefore, biopreservation should also help to meet the strong expectations of consumers who want “healthier” and more “natural” foods, and contribute to nutritional recommendations aimed at reducing salts, sugars, and additives in foods.
The concept of biopreservation was inspired by food fermentation ancestrally used to preserve food, except that fermentation involves substantial transformation of the food matrix, which is usually not the intention when engineering biopreservation systems. Consistently, the intentional addition of microorganisms or their metabolites for specific preservation purposes was largely investigated on fermented food, i.e., dairy products (cheeses, yoghurt), bakery products, or fermented sausages. Nevertheless, biopreservation was successfully extended to non-fermented food such as seafood, raw meat and non-fermented plant products. For example, biopreservation of seafood as fresh fish fillets, smoked fish or cooked shrimps aimed at controlling spoilers or pathogens such as Listeria monocytogenes, Staphylococcus aureus, Vibrio and histamine-producing bacteria (for a recent review, see Rathod et al., 2022). Biopreservation of raw meat (lamb, pork, beef, or poultry) and processed meat (sausage, cooked ham) has also focused on the control of pathogenic and spoilage bacteria as well as extension of shelf life (Jones et al., 2010; Zagorec and Champomier-Vergès, 2017; Wang et al., 2019). Biopreservation of non-fermented plant products is essentially dedicated to fighting against spoilage microorganisms including yeasts, moulds, spore-forming bacteria (Bacillus subtilis, Bacillus licheniformis), and pathogenic bacteria such as L. monocytogenes (Leyva Salas et al., 2017).
Most species used for food biopreservation by the food industry are lactic acid bacteria belonging essentially to the genera Lactococcus, Lactobacillus lato sensu and Carnobacterium. One strain of the Gram-negative species Hafnia alvei is also available on the market for anti-Escherichia coli purposes (Callon et al., 2016; Frétin et al., 2020). These bacteria are derived from food microbiota and are therefore particularly well adapted to food matrices. Moreover, as these species have been studied for several decades, their use as protective cultures is considered safe. Latilactobacillus sakei, Latilactobacillus curvatus, Carnobacterium divergens, Carnobacterium maltaromaticum and Lactococcus lactis are the main species providing protective cultures for meat/seafood products (Leroi et al., 2015; Comi et al., 2016; Ramaroson et al., 2018; Iacumin et al., 2020), while lactobacilli or even yeast strains can be used for vegetable food biopreservation (Siedler et al., 2019; Truchado et al., 2020; Windholtz et al., 2021). Although several biopreservation technologies are already available on the market, the rate and speed of innovation in this area needs to increase considerably in order to meet global climate-related challenges. Indeed, the scientific literature dealing with biopreservation is considerably larger than the actual application of biopreservation in the food sector. Foods are complex systems because of their diversity, their various physical, chemical, and biological structures, and the numerous processes used to produce them. Moreover, food microbial community dynamics during shelf life depends on abiotic parameters, which are mostly linked to production processes or storage conditions, and biotic parameters where microbial interactions play a major role. The complexity and diversity of food microbial communities has been a major barrier to the widespread use of biopreservation. Therefore, the conception of efficient biopreservation technology requires the implementation of methodological approaches adapted to the high complexity of food ecosystems. Food microbiology has long been studied by classical culture-dependent methods. During the last decade, omics techniques including genomics, transcriptomics, proteomics, metabolomics, culturomics, and phenomics have revolutionised all areas of the life sciences, including food quality and safety assessment, because of their ability to decipher food systems as a whole (Cook and Nightingale, 2018). The application of omics provides a more realistic portrait of the complex interactions occurring in the food ecosystem (Gálvez et al., 2007), and it has significantly increased our understanding of the potential of microbiomes to increase the productivity and sustainability of food systems. These omics approaches have caused a paradigm shift from unsocial undesirable microorganisms colonising food to strongly interacting microorganisms establishing stable networks (Berg et al., 2020). Omics approaches have been applied to explore various aspects of biopreservation, such as selection and characterisation of protective cultures, investigation of the mechanisms involved in the protective effect, or the impact on food microbial communities. After a brief overview of food biopreservation, the different omics approaches used in studies dealing with biopreservation are reviewed below and analysed by illustrating to what extent they can answer questions related to the impact of biopreservation on the food microbial ecosystem.
Classical approaches to identify protective culture candidates are mainly based on the detection of inhibition zones in laboratory conditions. Recently, the use of phenomics and genomics has proved highly effective. Phenomics can be defined as the high-throughput study of phenotypes. In the case of biopreservation, the phenotype of interest is the inhibition of spoilage microorganisms or food-borne pathogens. Phenomics has been successfully used to identify strains exhibiting remarkable anti-L. monocytogenes properties by using a high-throughput liquid handling system and a genetically engineered luminescent strain of L. monocytogenes to set up mixed culture competition assays in food matrices (Riedel et al., 2007; El Kheir et al., 2018). This method was first used to select anti-Listeria candidates from a collection of strains isolated from raw milk and a collection of Lactococcus piscium. The majority of the candidates obtained did not produce an inhibitory halo following a classical agar diffusion-based method, suggesting promising inhibitory mechanisms (El Kheir et al., 2018). Lately, this high-throughput competition assay was implemented to study the inhibition phenotype of a collection of C. maltaromaticum strains under multiple varying conditions. This method resulted in the selection of robust antagonistic C. maltaromaticum strains whose anti-Listeria properties are insensitive to fluctuations, i.e., inoculation level and time lag of L. monocytogenes and candidate inoculation (Borges and Revol-Junelles, 2019). It is expected that phenomics approaches extended to other target microorganisms (Besnard et al., 2021), as well as other phenotypes related to the sensory profile or use of nutrients (Wiernasz et al., 2017; Acin-Albiac et al., 2020), may in the future enable the identification of microorganisms with high biopreservation performances.
Genomics is an interesting approach for selecting protective cultures as genome mining may point out important features that can be involved in the preservation effect (Baltz, 2019), and also prove the absence of some unwanted functions such as antibiotic resistance or biogenic amine synthesis. Genome mining involves the analysis of functional gene annotation, resulting in particular from antiSMASH (Blin et al., 2017) and BAGEL (van Heel et al., 2018), which are designed to identify clusters of genes involved in the biosynthesis of antimicrobial compounds, combined with genome comparison to find correlation between the presence/absence of genes and protective properties. As an example, the genome sequence of L. sakei 23K, a meat adapted bacterium used as a starter for sausage fermentation, but also proposed as a biopreservative agent for raw meat products (Zagorec and Champomier-Vergès, 2017), revealed its strong ability to be competitive in meat products (Chaillou et al., 2005; Eijsink and Axelsson, 2005). Indeed, genome analysis highlighted the presence in the genome of elements putatively enabling the use of alternative carbon sources, such as ribose, inosine, and adenosine. Their efficacy was subsequently proven and helps to explain the fitness in meat of L. sakei, which thereby escapes competition for energy sources (Rimaux et al., 2011). Also, the requirement for haem and iron, two components present in meat, could be assessed by genomics through the gene repertoire and further evidenced by functional genomics (Duhutrel et al., 2010; Verplaetse et al., 2020).
Another example of genomics input is the production of antagonistic molecules by protective strains. This has long been studied through the production of bacteriocins. The genome sequence analysis of L. curvatus CRL705, a strain known to produce two bacteriocins (lactocin 705 and AL705), revealed the presence of additional genes putatively involved in bacteriocin production (sakacin P, sakacin Q, sakacin X, and sakacin T; Hebert et al., 2012). Divercin V41 is a bacteriocin involved in the protective function of C. divergens V41 a lactic acid bacterium strain whose operon sequence was reported more than two decades ago (Metivier et al., 1998). The genome analysis of this strain revealed that an additional gene was present in the divercin V41 operon (Remenant et al., 2016), the function of which was shown to be important for bacteriocin production (Back et al., 2015). Comparative genomics of Carnobacterium highlighted potential new candidate strains for biopreservation, efficient against L. monocytogenes and harbouring original bacteriocin gene equipment. For example, five different bacteriocins and a 16 kDa new one were predicted in the C. maltaromaticum SF668 and C. maltaromaticum EBP3019 genomes, respectively (Begrem et al., 2020). Combining genome analysis and peptidomics of a Companilactobacillus crustorum strain enabled, from the peptides produced during the growth of this strain, the discovery of eight novel bacteriocins and two other antimicrobials with a broad spectrum of action against Gram-positive and Gram-negative pathogens (Yi et al., 2018).
L. piscium CNCM I-4031 (EU2241) is a protective strain for seafood products that improves the sensory quality of shrimp and cold-smoked salmon (Fall et al., 2012; Leroi et al., 2015). This strain is also particularly efficient against the pathogen L. monocytogenes by reducing growth and virulence (Saraoui et al., 2018). Combined phenotyping and genome analyses evidenced that the inhibitory effect is dependent on cell-to-cell contact instead of extracellular molecules such as bacteriocins, organic acids, or hydrogen peroxide (Saraoui et al., 2016; Marché et al., 2017). This unusual mechanism still remains to be elucidated; nevertheless medium- and high-throughput screening revealed that other strains of the same species could be selected as new protective cultures, notably related to their large antimicrobial capacities (Wiernasz et al., 2017; El Kheir et al., 2018).
Genomics can reveal other unexpected features, as exemplified by the genome sequence of C. divergens V41 which contains an intriguing long genomic island (∼40 kb) encoding polyketide synthases/non-ribosomal peptide synthases (PKS/NRPS) or PKS/NRPS-like enzymes, putatively involved in the production of a secondary metabolite of unknown function (Remenant et al., 2016). Such molecules may have antimicrobial functions or be associated with oxidative stress resistance, and immunomodulatory or cytotoxicity activities. Comparative genomic analysis of Carnobacterium strains showed that this PKS/NRPS gene cluster is unique in C. divergens V41 (Begrem et al., 2020). Other PKs/NRPs antimicrobial compounds, such as milkisin produced by a Pseudomonas sp. strain, with potentially interesting properties for the biopreservation of milk products have been described (Schlusselhuber et al., 2018, 2020). Reuterin-producing Limosilactobacillus reuteri are known as bioprotective agents for dairy products (Ortiz-Rivera et al., 2017). Some rare strains harbour a PKS/NRPS genomic island encoding rtc genes involved in the synthesis, regulation, immunity, and secretion of an antimicrobial tetramic acid named reutericyclin (Gänzle et al., 2000; Lin et al., 2015).
While many published papers describe the screening and evaluation of bioprotective antifungal lactic acid bacteria strains in vitro and in situ (Delavenne et al., 2012; Leyva Salas et al., 2018), as well as the identification of metabolites involved in their antifungal activities, the application of genomics and functional genomics to the selection of antifungal microorganisms is still in its infancy. Studies providing insights into the metabolic pathways of antifungal metabolites such as phenyllactic acid (Wu et al., 2020), clearly point out that in-depth studies of antifungal lactic acid bacteria using comparative and functional genomics are needed. Such studies should help to elucidate yet uncharacterised biosynthetic pathways of known antifungal molecules and potentially reveal new ones and to establish whether other competition exclusion phenomena exist between lactic acid bacteria and spoilage fungi, with the objective to further understand their action mechanism and possibly provide helpful tools for strain selection.
Some species with bioprotective properties are also described as potential spoilage organisms, depending on the type of food or process (Brillet et al., 2004; Leisner et al., 2007; Andreevskaya et al., 2015; Leroi et al., 2015; Saraoui et al., 2016; Poirier et al., 2018). Thus comparative genomics between strains known to be responsible for spoilage or on the contrary known as protective cultures should be an interesting approach for the selection of strains of interest. In addition, as a complementary approach to phenotypic tests, genome analysis of potent protective strains can be a complementary tool for their safety assessment i.e., screening for the presence of genes related to biogenic amine production, antibiotic resistance genes, as well as their location with respect to mobile genetic elements, i.e., plasmids and bacteriophages. Such an approach was recently applied to a Lactiplantibacillus plantarum starter culture used in the manufacturing of nahm fermented pork (Chokesajjawatee et al., 2020), as well as to a potent L. plantarum protective culture (Barbosa et al., 2021).
The role of protective cultures is mainly related to their fitness (nutritional competition, ability to resist harsh conditions encountered in food), enabling them to establish and dominate at the expense of other undesirable species, as well as to their ability to produce antimicrobial molecules, such as organic acids, hydrogen peroxide or specialised metabolites as bacteriocins, biosurfactants, or lipopeptides (Ben Said et al., 2019; Bourdichon et al., 2021; Table 1). The expected impact is induction of decay or at least growth inhibition of the undesirable microorganism. Classical methods such as qRT-PCR are a priori methods that involve a prior selection of the target genes to be studied. On the contrary, omics approaches such as RNAseq can be used without a priori, opening the possibility to identify original unsuspected mechanisms. These approaches have revealed that the mechanisms involved are more complex than previously thought, and involve microbial sensing capacity, gene regulation, and the potential for combining antimicrobial compounds for microbial inactivation (Figure 1).
Figure 1. Major advances in the field of biopreservation thanks to omics approaches. The objective of biopreservation is to induce growth cessation or decay of pathogens and spoilers. Omics approaches have shown that in response to biopreservation, target microorganisms modulate the expression of genes involved in cell metabolism, stress tolerance and virulence. In addition, omics revealed that biopreservation can decrease the diversity of food microbiota. Conversely, depending on its structure, the food microbiota can either improve or reduce the effectiveness of biopreservation agents. Food microbiota can also have intrinsic protective properties and thus inhibit unwanted microorganisms.
Two recent studies have explored the mechanism of inhibition of L. monocytogenes by antimicrobial lipopeptide (P34) or the peptide nisin (encapsulated or not) by proteomic analysis after incubation in a laboratory medium (Pinilla et al., 2021; Stincone et al., 2021). The lipopeptide P34 caused the downregulation of proteins involved in manganese transport and upregulation of proteins related to iron transport in L. monocytogenes. In addition, reduction of stress tolerance proteins related to the sigma B and VirR regulons and modulation of phosphoenolpyruvate phosphotransferase systems for sugar transport were observed (Stincone et al., 2021). Exposure of L. monocytogenes to nisin induced the synthesis of proteins related to ATP-binding cassette transporter systems, transmembrane proteins, RNA-binding proteins, and diverse stress response proteins (Pinilla et al., 2021). Some of the proteins detected in the presence of free nisin were related to translocation of L. monocytogenes virulence factors, activation of the LiaR-mediated stress defence, and glycosylation of cell wall teichoic acid. The comparison of treatment by free and encapsulated nisin revealed that L. monocytogenes did not express some stress proteins when nisin was encapsulated, suggesting the production of nisin-resistance factors by exposure to encapsulated nisin. The authors suggested that liposomes allow controlled release of nisin in the medium, resulting in fewer interactions between nisin and bacteria compared with free nisin, which may impact the mechanism of action of nisin (Pinilla et al., 2021). The induction of resistance factors was also observed by exposing L. monocytogenes to sublethal doses of pediocin. Transcriptomic analysis revealed the expression modulation of the two-component system LisRK and the alternative sigma factors SigB and SigL, resulting in an increased resistance of L. monocytogenes to this bacteriocin (Laursen et al., 2015).
Recently, the ability of C. maltaromaticum, Leuconostoc gelidum, or L. piscium strains to inhibit L. monocytogenes was demonstrated in seafood (Saraoui et al., 2016, 2018; Wiernasz et al., 2017). L. piscium inhibition required cell-to-cell contact with L. monocytogenes, affecting its cell surface, and decreasing its virulence (Saraoui et al., 2018). The metabolomic fingerprints suggested that this inhibition might not involve nutritional competition and remains to be explored (Saraoui et al., 2016).
Lactococcus garvieae was shown to inhibit S. aureus growth in milk, in cheese, and in vitro, potentially through hydrogen peroxide (H2O2) production (Delbes-Paus et al., 2010). To better characterise this mechanism of inhibition in vitro, the transcriptomes of L. garvieae and S. aureus co-culture have been explored by RNA-seq and RT-qPCR (Delpech et al., 2015, 2017). L. garvieae repressed the expression of S. aureus genes involved in stress response, including oxidative stress generated by H2O2, and in cell division. It also modulated the expression of virulence-related genes (particularly agrA, hld, and enterotoxin-encoding genes; Delpech et al., 2015). For L. garvieae, a high concentration of H2O2 was not associated with higher expression of the H2O2 synthesis genes pox, sodA, and spxA1, but rather with repression of H2O2-degradation genes (trxB1, ahpC, ahpF, and gpx; Delpech et al., 2017). The interaction between L. lactis and S. aureus has also been widely studied and transcriptomic analyses were performed with microarrays and RT-qPCR. In a chemically defined medium held at a constant pH value of 6.6, the growth of the two bacteria in co-culture was not modified, but their transcriptome was modulated (Even et al., 2009; Nouaille et al., 2009). The expression of S. aureus virulence-related genes was impaired by L. lactis: the expression of genes encoding global regulators, including agr and consequently the agr-controlled enterotoxin genes and sar, was strongly reduced (Even et al., 2009). This downregulation of agr in S. aureus was associated with the reducing properties of L. lactis (Nouaille et al., 2014). L. lactis genes associated with amino acid metabolism, ion transport, oxygen response, menaquinone metabolism, cell surface, and phage expression were differentially expressed in co-culture compared to monoculture (Nouaille et al., 2009). In a complex medium such as the cheese matrix, the acidifying, proteolytic, and reducing activities of L. lactis were shown to affect carbohydrate and nitrogen metabolisms and the stress response of S. aureus (Cretenet et al., 2011). Enterotoxin gene expression was positively or negatively modulated by both L. lactis and the cheese matrix itself, depending on the enterotoxin type. Again, the agr operon was downregulated by the presence of L. lactis, in part because of a drop in pH (Cretenet et al., 2011). All these data highlight the intimate link between environment, metabolism, and virulence expression. A third binary interaction between Enterococcus faecalis and S. aureus was studied in milk and in cheese (Viçosa et al., 2018; Nogueira Viçosa et al., 2019). When co-cultured, the growth of S. aureus was decreased and the classical enterotoxins were not produced. The expression of several enterotoxins and global regulator genes (including agr) was downregulated, while the expression of genes involved in metabolism was upregulated. Finally, the interaction of S. aureus with a mixed culture of Enterococcus durans, E. faecalis and L. lactis in milk confirmed that the production of enterotoxins was reduced in mixed culture and the expression of several genes involved in virulence was inhibited (Zdenkova et al., 2016). All these studies on the interaction of S. aureus with different lactic acid bacteria converge on a very important idea that the mechanism of action of biopreservation can result in the inhibition of virulence by inhibiting the production of enterotoxins through the decreased expression of genes involved in their synthesis.
L. curvatus, L. plantarum, and Enterococcus mundtii strains can inhibit the growth of E. coli O157:H7 when co-cultured in a meat model medium (Orihuel et al., 2018). The antagonistic effect of the most efficient E. mundtii strains against E. coli O157:H7 were characterised by a proteomic analysis (Orihuel et al., 2018). The expression of E. mundtii proteins involved in carbohydrate/amino acid metabolisms, energy production, transcription/translation, and cell division was modified in the presence of E. coli. Reciprocally, the presence of E. mundtii resulted in repression of E. coli synthesis of proteins related to metabolism and transport of amino acids and nucleotides, as well as overexpression of proteins involved in stress, energy production, and transcription (Orihuel et al., 2019). In addition, proteins associated with adhesion to extracellular matrix proteins of meat were modulated in E. coli in accordance with its decreased adhesion capacity when co-cultured with E. mundtii. E. mundtii did not influence the lytic cycle of the E. coli O157:H7 strain, indicating its potentially safe use as a bioprotective agent, since engagement in the lytic cycle results in the production of shiga toxin (Orihuel et al., 2019). The interaction of E. coli O157:H7 with ground beef microbiota was also studied (Galia et al., 2017). A beef piece was divided in two parts with the inner part considered sterile and the outer part as encompassing a natural meat microbiota. The microbial community structure was assessed by 16S rDNA amplicon sequencing, and the transcriptome of two inoculated strains (E. coli O157:H7 and E. coli O26:H11) was studied by RNAseq comparing samples of sterile and naturally contaminated meat. This study revealed that the two E. coli strains behave differently. On the one hand, an upregulation of genes involved in detoxification and stress response and a downregulation of peR, a gene negatively associated with virulence phenotype, were observed in E. coli O157:H7. On the other hand, the interaction of E. coli O26:H11 with ground beef microbiota revealed that genes involved in division, peptidoglycan synthesis, DNA repair, metal acquisition, and carbohydrate and amino acid metabolism were downregulated (Galia et al., 2017).
In a recent review of biopreservation against moulds in dairy products (Shi and Maktabdar, 2022), the authors point out newly described antifungal mechanisms. Among these is a perfect example of how omics technologies shed new light on the understanding of the protective mechanisms of antifungal lactobacilli toward dairy product spoilage fungi (Siedler et al., 2019). This work revealed that manganese scavenging by Lacticaseibacillus rhamnosus and Lacticaseibacillus paracasei antifungal strains, previously known as a defence mechanism against oxidative stress, was a main inhibitory mechanism (i.e., competitive exclusion) against many yeast and mould species involved in dairy product spoilage. Indeed, following milk fermentation and supplementation with manganese, their bioactivity was completely lost. A transcriptomic approach based on RNA-seq further showed that one of the most highly expressed gene products in these strains encoded a manganese transporter (MntH1). The role of MntH1 in manganese scavenging was confirmed in a ΔmntH1 L. paracasei strain in which no significant antifungal activity was detected, while bioactivity was restored in the ΔmntH1 mutant complemented with a plasmid containing the mntH1 gene under its own promoter.
Besides the above-mentioned discovery that competitive exclusion for manganese was an important antifungal mechanism, production of antifungal metabolites and pH decrease were believed to be the main mechanisms involved in the bioactivity of antifungal protective lactic acid bacteria. Through the use of metabolomic targeted and untargeted approaches with or without prior medium fractionation and bioactivity testing, more than 60 molecules have been thought to play a role in antifungal activity (see recent reviews by Leyva Salas et al., 2017 and Siedler et al., 2019). These metabolites include molecules produced through carbohydrate metabolism (e.g., organic acids such as lactic, acetic, formic, and succinic acids, volatile compounds such as diacetyl), proteolysis (e.g., bioactive peptides resulting from casein cleavage), amino acid metabolism (e.g., phenyllactic acid), lipolysis and free fatty acid metabolism (e.g., 3-hydroxydecanoic, caproic – i.e., hexanoic- and caprylic – i.e., octanoic – acids), but also complex compounds derived from bioconversions (e.g., benzoic acid) or peptide synthesis [e.g., cyclic dipeptides such as cyclo(L-Phe-L-Pro) and cyclo(L-Phe-trans-4-OH-L-Pro)] (Ström et al., 2002; Aunsbjerg et al., 2015; McNair et al., 2018; Leyva Salas et al., 2019; Garnier et al., 2020; Shi and Knøchel, 2021a,b). It should be underlined that with the exception of lactic and acetic acids which are produced in g/kg or g/L amounts, these molecules are produced in quite low quantities, all of which are at concentrations far from their individual minimum inhibitory concentration (MIC), thus suggesting that they act in synergy or by additive effects. In a recent study, Leyva Salas et al. (2019) used a metabolomics approach coupled with supervised multivariate analysis to investigate 56 antifungal compounds as well as volatiles. It was found that 9 key compounds including acetic acid, 5 aromatic acids, and three volatiles were associated with antifungal activity against Mucor racemosus and Penicillium commune, although their concentrations were below their respective MICs. Further investigation on Penicillium roqueforti and Mucor circinelloides revealed that several combinations presented additive (e.g., diacetyl + 3-phenylpropanoic acid, diacetyl + acetic acid) or synergistic effects (diacetyl + octanoic acid, octanoic + 3-phenyl propanoic acids), clearly reinforcing the idea that additive and synergistic effects of antifungal molecules are involved in lactic acid bacteria bioactivity. To go further, future work could include the investigation of more complex mixtures of antifungal compounds at concentrations close to those encountered in biopreserved foods. Moreover, it is not clear how antifungal molecule synthesis and competitive exclusion interact together in different fungal species with various susceptibilities to protective strains.
The primary objective of biopreservation is to limit the presence of unwanted microorganisms in food. However, the addition of biopreservatives can have an overall impact on the food microbiome. Omics approaches have helped clarify the complex interactions occurring in the food ecosystem and their impact on the organoleptic properties of foods (Figure 1).
Prior to the availability of high-throughput DNA sequencing (HTS) techniques, DNA fingerprinting techniques as PCR-denaturing gradient gel electrophoresis or PCR-temporal temperature gradient electrophoresis, targeting mainly the V3 region of 16S rDNA, were employed to determine whether bioprotective lactic acid bacteria strains were able to colonise the food matrix and dominate the microbiota (Hu et al., 2008; Saraoui et al., 2017; Zhang et al., 2018). However, discrepancies between these DNA fingerprinting approaches and cultural methods (Ercolini et al., 2010) have highlighted the need for methods of higher resolution. There are two commonly used HTS methods in microbiome research: amplicon sequencing and metagenomic sequencing (Liu et al., 2021). Amplicon sequencing is the most widely used, including in the field of biopreservation. It demonstrated that the three protective cultures L. rhamnosus LRH05, L. sakei LSK04, and C. maltaromaticum CNB06, alone or in combination, were able to colonise cheese and became dominant after storage (Bassi et al., 2020). In shrimp, the results of amplicon sequencing were consistent with the successful colonisation of the food matrix by the biopreservative strains L. plantarum and Lacticaseibacillus casei, and a reduction in the relative abundance of Shewanella, which includes the spoilage species Shewanella baltica (Li et al., 2019). In addition, in beef burgers, the analysis of predicted metagenomes revealed that nisin-activated packaging resulted in a reduction in the abundance of specific metabolic pathways related to spoilage (Ferrocino et al., 2015). Table 2 summarises the different studies using omics approaches to assess the impact of biopreservation on food ecosystems and the main findings.
Table 2. Overview of omics approaches used to assess the impact of biopreservation on food ecosystems and main findings.
HTS approaches open up the possibility of going much further than simply answering the question of the implantation success of the protective microorganisms and their effect on the targets. Amplicon sequencing can be used to estimate the impact of biopreservation on food microbiota. When batches of cold-smoked salmon were inoculated with a bioprotective strain of L. piscium, the microbiota structure differed significantly between control and biopreserved products after 3 weeks of storage (Leroi et al., 2015). The impact of biopreservation strains can be stronger, as in the case of fermented sausages where 16S rRNA-based analysis revealed a markedly lower microbial diversity of the metabolically active microbial community (Giello et al., 2018). Thus, although special attention is paid to the selection of strains with a narrow antimicrobial spectrum in biopreservation (e.g., bacteriocin-producing bacteria), biopreservation can end up with significant changes in food microbiota structure. Although this side effect can be undesirable, especially in fermented foods, in some circumstances it is possible to take advantage of this broad impact on food microbiota to target spoilage microorganisms, which can encompass a large number of phylogenetic taxa. In dairy products such as low-salt fresh cheeses, spoilage bacteria are mainly psychrotrophic Gram-negative species, including several Enterobacteriaceae and Pseudomonas spp. (Ledenbach and Marshall, 2009; Spanu et al., 2018; Bassi et al., 2020). The three lactic acid bacterial strains L. rhamnosus LRH05, L. sakei LSK04, and C. maltaromaticum CNB06, added alone or in combination, were found after 5 weeks of storage to be effective in inhibiting the Gram-negative bacteria population of fresh Primo Sale cheese inoculated with a cocktail of 10 bacterial spoilage isolates (Bassi et al., 2020). In Italian fresh filled pasta cheese, the impact of protective cultures at the community level can be used to reduce the initial microbiota associated with raw materials and to confer a competitive advantage on safer or more acceptable bacterial species such as Leuconostoc mesenteroides, at the expense of more problematic species such as Streptococcus uberis and Streptococcus parauberis (Tabanelli et al., 2020).
While, on the one hand, biopreservation can have an impact on the microbiota, on the other hand, the microbiota can also affect the efficiency of biopreservation. The protective property of a nisin-producing L. lactis strain was tested in combination with high pressure under controlled conditions of microbiota composition in reduced-nitrite diced cooked ham (Chaillou et al., 2022). Sterile diced ham cubes were inoculated with two different microbiota collected from cooked hams, together with the protective L. lactis strain prior to vacuum packaging and high-pressure treatment. During storage, the two selected cooked ham microbiota were both characterised by a microbial community enriched in high potential spoilage bacterial species (especially Proteobacteria). Comparison of the bacterial community composition after 1 month of storage revealed that the protective effect of the L. lactis/high-pressure combination is highly dependent on the ham microbiota. Indeed, when ham samples were inoculated with Pseudomonas spp. and Serratia spp. rich-microbiota, L. lactis became dominant (>90% relative abundance). However, when ham samples were instead inoculated with microbiota dominated by Psychrobacter sp. and Vibrio sp., L. lactis was not competitive and Brochothrix thermosphacta became dominant (Chaillou et al., 2022). Thus, by interacting with the protective strain, food microbiota can act on the efficiency of biopreservation. By contrast, it is also possible that the microbiota can contribute to biopreservation in concert with the protective strains by acting directly on the unwanted microorganism through its intrinsic protective properties. In uncooked pressed cheese, the protective activity of a consortium comprising three strains belonging to the species H. alvei, L. plantarum, and L. lactis was dependent on the composition of the microbiota colonising the processed raw milk. The raw milk batches associated with the lowest growth of E. coli O26:H11 were characterised by greater relative abundance of lactic acid bacteria, the three Gammaproteobacteria Acinetobacter, Serratia, and Hafnia, as well as Macrococcus. On the other hand, the highest levels of E. coli O26:H11 were observed when the milk microbiota was significantly enriched with bacteria from the genera Ramboutsia, Paeniclostridium, and Turicibacter (Frétin et al., 2020).
Overall, these data were mainly produced thanks to amplicon sequencing. The major drawbacks of this approach are the biases in relative abundances resulting from PCR amplification and differences in the number of ribosomal operons between species (Edgar, 2017), the limited taxonomic resolution, mainly at the genus level even if some efforts have been made in developing specialised databases such as the DAIRYdb (Meola et al., 2019), and the lack of functional information. Amplicon sequencing targeting housekeeping genes such as gyrB is also promising for a better identification at the species or even intra-species level, with also less bias for relative abundance determination (Poirier et al., 2018). Investigating food communities by using shotgun metagenomics could help to improve accuracy, especially by gathering information at the species or even the strain level, and could give a global view of the functions involved in the process of biopreservation at the microbial community scale. Furthermore, network analysis of metagenetic and metagenomic data could be used to identify patterns (i.e., co-occurrence and mutual exclusion) in food microbial communities, biopreserved or not. Such an approach would enable hypotheses to be drawn regarding biotic interactions occurring between microorganisms, which could then be tested experimentally, and could be applied to the selection of potential candidates for biopreservation or for a deeper understanding of the impact of selected bioprotective cultures at a community level.
In addition to their impact on food microorganisms, biopreservation agents are likely to modify the properties of the food matrix and in particular its organoleptic characteristics. Metabolomics approaches allow us to go much further than sensory analyses in the study of the impact of biopreservation agents on the matrix. SPME/GC-MS showed that cooked peeled shrimp contains a reduced amount of unwanted aldehydes and alcohols associated with sensory spoilage when L. piscium is used as an inhibitor of B. thermosphacta (Fall et al., 2012). Biopreservatives can thus play a positive sensory role by inhibiting a target microorganism responsible for spoilage. However, biopreservation can also negatively impact the matrix and can be responsible for significant changes, highlighting the interest of the polyphasic omics approach to the selection of candidate strains. An exemplary study describes the use of Head Space-Solid Phase MicroExtraction/Gas Chromatography- Mass Spectrometry (HS-SPME/GC-MS) to reveal that the impact of biopreservation candidates varied dramatically depending on the strain considered, and that specific signatures could be associated with each strain. Coupled to microbial community structure investigation by amplicon sequencing, the authors were able to rationally select two strains with the highest protective effect and the lowest sensory activity in salmon gravlax (Wiernasz et al., 2020; Table 2). Nuclear Magnetic Resonance (NMR) also has great potential in the study of living organisms, owing to its non-destructive nature, i.e., it can be used for in vivo and in vitro measurements of biological processes, with no quenching of the metabolism required. NMR spectroscopy can be particularly helpful for the kinetic analysis of the metabolism of protective cultures, as in the development of in vitro NMR kinetic measurements of lactic acid bacteria (Ebrahimi et al., 2016; Table 2). Such a polyphasic approach was also used successfully in wine to assess biopreservation as an alternative to sulphites (Simonin et al., 2020). The wine metabolome being of high complexity, an ultra-high-resolution mass spectrometric method -the Fourier transform ion cyclotron resonance mass spectrometry- was used to identify and annotate more than 7,000 molecules. Clustering analysis revealed that even if the biopreservation agent has a molecular impact on the product, it is significantly lower than the winery effect, showing that biopreservation has preserved the typicality of the products, which can be of high relevance for products with protected designation of origin (Simonin et al., 2020; Table 2).
Genomic tools based on amplicon sequencing, although powerful for food microbial ecosystem description, may show some limits for biopreserved products. Bioprotective cultures inoculated at a high level are usually dominant, at least at the beginning of storage. As it is generally admitted that taxa representing less than 0.01% of the dominant ones are not detected with metagenetics, the richness of biopreserved food may be underestimated. In the gut, the use of shotgun metagenomics has revealed that the majority of species harbour multiple strains (Ellegaard and Engel, 2016). For instance, despite a low species complexity, the infant gut microbiota exhibits extensive intra-species diversity, with an average of 4.9 strains per subject (Luo et al., 2015). In food, low resolution is a limitation in estimating the impact of protective cultures on subdominant species, such as pathogens usually present at low levels (<102 CFU/g). This problem is less important for specific spoilage organisms that generally dominate at sensory rejection time. However, the interaction between bacteria can modify the sensory characteristics (Stohr et al., 2001; Joffraud et al., 2006; Silbande et al., 2018), and it may be that underestimated taxa play a role in the deterioration of food. In addition, most efforts have been focused on bacteria, and to a lesser extent on yeasts and moulds, although phages, viruses, or Archaea may also be present in food microbiota (Roh et al., 2010; Park et al., 2011).
Omics are powerful tools in analysing protective cultures and their interactions at the population level. However, most of these techniques hardly consider microbial population heterogeneity in food systems that can trigger important community functions. Diversification of cell types can originate from genetic variation, ageing, gene expression stochasticity, and environmental condition fluctuation (Bury-Moné and Sclavi, 2017). In structured food matrices such as ground meat or cheese, microorganism microenvironments are highly heterogeneous and vary over time depending upon local microbial activities (Ferrier et al., 2013; Jeanson et al., 2015). Above critical textural levels, individual cells are not able to sediment or swim inside the matrix and eventually grow as large 3D microcolonies (Darsonval et al., 2021; Saint Martin et al., 2022). Microcolony size and sphericity depend on several factors such as local rheological properties, nutrient availability, competing microbiota, and associated interference interactions (Verheyen et al., 2018). Sharp gradients of nutrients and metabolites are generated inside and around the colonies, thus expanding the functional diversification of the local populations. In other structured biosystems such as bacterial biofilms (Lenz et al., 2008; Pérez-Osorio and Franklin, 2008) or gut (Consentino et al., 2021), laser capture microdissection has been put to use in applying omics to defined spatial regions or localised subpopulations of the sample. This could be of interest in deciphering the local behaviour of protective cultures and their targets in distinct and heterogeneous regions of food matrices. The few studies that have approached population functional heterogeneity in real food matrices took advantage of fluorescence microscopy associated with strains reporting the expression of genes of interest by fluorescent proteins (Fleurot et al., 2014; Hernández-Galán et al., 2017). However, food matrices are often opaque to fluorescence microscopy and the density of the population of interest can be too low for reliable quantitative measurements (e.g., bacterial pathogens < 102 CFU/g). Synthetic microbial ecology approaches, where the complexity of the communities and the factors of influence are reduced to their minimum, but are increased in their controllability, can be used to examine interactions and ecological theories (Connell et al., 2012, 2014; Rothschild, 2016; Hynes et al., 2018). Such approaches can be combined with new creative experimental designs for the study of previously unexplored aspects of bacterial behaviour in spatially structured populations (Connell et al., 2013; Wessel et al., 2013; Bridier et al., 2017). In particular, 3D bioprinting of simplified structured matrices with patterned microbial ecosystems could help study population heterogeneities and interspecies interactions at a single-cell scale in structured matrices (Moon et al., 2016; Kyle, 2018; Gyimah et al., 2021; Krishna Kumar et al., 2021). Target microorganisms could be fluorescently tagged for their geolocalisation and the feeding of spatial models of interactions (with food components and with other microorganisms during growth in the printed matrix; Krishna Kumar et al., 2021). Recently, a transcriptome-imaging approach (par-seqFISH for parallel sequential fluorescence in situ hybridisation) was reported to capture gene expression and spatial context within microscale assemblies at a single-cell and molecule resolution and could be put to use in such synthetic ecology approaches (Dar et al., 2021). Biopreservation studies could also benefit from microfluidic approaches to assess small-scale interactions between microorganisms (Burmeister and Grünberger, 2020).
The consideration of population heterogeneity in structured food matrices is starting to be integrated into mathematical spatial modelling and predictive microbiology (Verheyen and Van Impe, 2021). Predictive microbiology is useful to quantify both the impact of biopreservation on the food matrix and to simulate the behaviour (survival, growth, inactivation) of the undesirable target microorganism (Leroy and De Vuyst, 2003; Koutsoumanis et al., 2004; Couvert et al., 2010; Habimana et al., 2011; Møller et al., 2013). Another important point to consider is that the contamination of food products with pathogens such as L. monocytogenes and E. coli O157:H7 occurs accidentally and usually at low levels, thus requiring single-cell level approaches. Individual-based modelling combined with microenvironment (pH, aw variabilities) modelling of vacuum-packed cold-smoked salmon was more effective in describing variability in the growth of a few L. monocytogenes cells than the traditional population models (Ferrier et al., 2013; Augustin et al., 2015). Such stochastic approaches need to be improved by characterising a wider range of microenvironmental factors, such as the variability of the viscosity within food matrices between liquid and solid states, as well as considering biotic factors, namely food components and food microbial communities. However, they could provide complementary information about the behaviour of unwanted microorganisms at realistic contamination levels.
Omics tools have become essential in the field of biopreservation, both for selecting innovative agents and for studying their effectiveness, their mechanism of action, and their impact on the food ecosystem. These approaches have revealed the diversity and complexity of the molecular mechanisms responsible for the protective activity of biopreservation agents. They have provided fundamental knowledge about biopreservation issues in terms of community description (taxonomy), biotic interactions, and impact on the organoleptic quality of the product. They have also shown that the food microbiota plays a major role in biopreservation by acting positively or negatively (Figure 1). It is now clear that the food microbiota must, in the future, be fully integrated into the biopreservation system engineering process to bring the field into the dimension of food microbiome engineering, so that it can play its protective role against pathogens and spoilage microorganisms as well as serve its technological purpose. Moreover, beyond the functioning of the food microbiome, this engineering process must better integrate its interconnections with other microbiomes (soil, water, plant, animal and consumer) to avoid disrupting their functioning and even to contribute to their balance. In this respect, efforts should be pursued to make more extensive use of multi-omics approaches and to combine them with other complementary approaches that take into account the heterogeneity of microorganisms at the cellular, population, and community levels, as well as the heterogeneity of the food matrix.
FB and MZ coordinated the work and consolidated the manuscript. All authors contributed to the design of the review, carried out the bibliographic data search, drafted the manuscript and approved the submitted version.
This work was financed by the RMT* Actia Florepro, a scientific and technical partnership in the field of biopreservation established and supported by the French Ministry responsible for Food, under the coordination of Actia. All authors of this review are members of the RMT Actia Florepro. *Réseau mixte technologique: Joint Technological Network.
The authors declare that the research was conducted in the absence of any commercial or financial relationships that could be construed as a potential conflict of interest.
All claims expressed in this article are solely those of the authors and do not necessarily represent those of their affiliated organizations, or those of the publisher, the editors and the reviewers. Any product that may be evaluated in this article, or claim that may be made by its manufacturer, is not guaranteed or endorsed by the publisher.
Acin-Albiac, M., Filannino, P., Gobbetti, M., and Di Cagno, R. (2020). Microbial high throughput phenomics: the potential of an irreplaceable omics. Comput. Struct. Biotechnol. J. 18, 2290–9. doi: 10.1016/j.csbj.2020.08.010
Agriculture and Economic Development Analysis Division (2013). The State of Food and Agriculture 2013: Food System for Better Nutrition. Rome: FAO.
Andreevskaya, M., Johansson, P., Laine, P., Smolander, O.-P., Sonck, M., Rahkila, R., et al. (2015). Genome sequence and transcriptome analysis of meat-spoilage-associated Lactic acid bacterium Lactococcus piscium MKFS47. Appl. Environ. Microbiol. 81, 3800–11. doi: 10.1128/AEM.00320-15
Augustin, J.-C., Ferrier, R., Hezard, B., Lintz, A., and Stahl, V. (2015). Comparison of individual-based modeling and population approaches for prediction of foodborne pathogens growth. Food Microbiol. 45, 205–15. doi: 10.1016/j.fm.2014.04.006
Aunsbjerg, S. D., Honoré, A. H., Marcussen, J., Ebrahimi, P., Vogensen, F. K., Benfeldt, C., et al. (2015). Contribution of volatiles to the antifungal effect of Lactobacillus paracasei in defined medium and yogurt. Int. J. Food Microbiol. 194, 46–53. doi: 10.1016/j.ijfoodmicro.2014.11.007
Back, A., Borges, F., Mangavel, C., Paris, C., Rondags, E., Kapel, R., et al. (2015). Recombinant pediocin in Lactococcus lactis: increased production by propeptide fusion and improved potency by co-production with PedC. Microb. Biotechnol. 9, 466–77. doi: 10.1111/1751-7915.12285
Baltz, R. H. (2019). Natural product drug discovery in the genomic era: realities, conjectures, misconceptions, and opportunities. J. Ind. Microbiol. Biotechnol. 46, 281–99. doi: 10.1007/s10295-018-2115-4
Barbosa, J., Albano, H., Silva, B., Almeida, M. H., Nogueira, T., and Teixeira, P. (2021). Characterization of a Lactiplantibacillus plantarum R23 isolated from Arugula by whole-genome sequencing and its bacteriocin production ability. IJERPH 18:5515. doi: 10.3390/ijerph18115515
Bassi, D., Gazzola, S., Sattin, E., Dal Bello, F., Simionati, B., and Cocconcelli, P. S. (2020). Lactic acid bacteria adjunct cultures exert a mitigation effect against spoilage microbiota in fresh cheese. Microorganisms 8:E1199. doi: 10.3390/microorganisms8081199
Begrem, S., Ivaniuk, F., Gigout-Chevalier, F., Kolypczuk, L., Bonnetot, S., Leroi, F., et al. (2020). New insight into antimicrobial compounds from food and marine-sourced Carnobacterium species through phenotype and genome analyses. Microorganisms 8:1093. doi: 10.3390/microorganisms8071093
Ben Said, L., Gaudreau, H., Dallaire, L., Tessier, M., and Fliss, I. (2019). Bioprotective culture: a new generation of food additives for the preservation of food quality and safety. Ind. Biotechnol. 15, 138–47. doi: 10.1089/ind.2019.29175.lbs
Berg, G., Rybakova, D., Fischer, D., Cernava, T., Vergès, M.-C. C., Charles, T., et al. (2020). Microbiome definition re-visited: old concepts and new challenges. Microbiome 8:103. doi: 10.1186/s40168-020-00875-0
Besnard, A., Desmasures, N., Voisin-Anastasie, A., Gréau, L., Lelièvre, V., Bré, J.-M., et al. (2021). Aerococcus sp. a promising genus as a source of anti-Salmonella bioprotective agents for the dairy industry revealed by a miniaturised screening method. Int. Dairy J. 116:104949. doi: 10.1016/j.idairyj.2020.104949
Blin, K., Wolf, T., Chevrette, M. G., Lu, X., Schwalen, C. J., Kautsar, S. A., et al. (2017). antiSMASH 4.0-improvements in chemistry prediction and gene cluster boundary identification. Nucleic Acids Res. 45, W36–41. doi: 10.1093/nar/gkx319
Borges, F., and Revol-Junelles, A.-M. (2019). Nouvelles Souches de Carnobacterium Maltaromaticum et Leurs Utilisations. French Patent No FR1911895. Courbevoie: Institut National de la Propriété Industrielle.
Bourdichon, F., Arias, E., Bückle, A., Bello, F. D., Dubois, A., et al. (2021). The forgotten role of food cultures. FEMS Microbiol. Lett. 368:fnab085. doi: 10.1093/femsle/fnab085
Bridier, A., Piard, J.-C., Pandin, C., Labarthe, S., Dubois-Brissonnet, F., and Briandet, R. (2017). Spatial Organization Plasticity as an adaptive driver of surface microbial communities. Front. Microbiol. 8:1364. doi: 10.3389/fmicb.2017.01364
Brillet, A., Pilet, M.-F., Prévost, H., Bouttefroy, A., and Leroi, F. (2004). Biodiversity of Listeria monocytogenes sensitivity to bacteriocin-producing Carnobacterium strains and application in sterile cold-smoked salmon. J. Appl. Microbiol. 97, 1029–37. doi: 10.1111/j.1365-2672.2004.02383.x
Burmeister, A., and Grünberger, A. (2020). Microfluidic cultivation and analysis tools for interaction studies of microbial co-cultures. Curr. Opin. Biotechnol. 62, 106–15. doi: 10.1016/j.copbio.2019.09.001
Bury-Moné, S., and Sclavi, B. (2017). Stochasticity of gene expression as a motor of epigenetics in bacteria: from individual to collective behaviors. Res. Microbiol. 168, 503–14. doi: 10.1016/j.resmic.2017.03.009
Caldeira, C., De Laurentiis, V., Corrado, S., van Holsteijn, F., and Sala, S. (2019). Quantification of food waste per product group along the food supply chain in the European Union: a mass flow analysis. Resour. Conserv. Recycl. 149, 479–88. doi: 10.1016/j.resconrec.2019.06.011
Callon, C., Arliguie, C., and Montel, M.-C. (2016). Control of Shigatoxin-producing Escherichia coli in cheese by dairy bacterial strains. Food Microbiol. 53, 63–70. doi: 10.1016/j.fm.2015.08.009
Cavicchioli, R., Ripple, W. J., Timmis, K. N., Azam, F., Bakken, L. R., Baylis, M., et al. (2019). Scientists’ warning to humanity: microorganisms and climate change. Nat. Rev. Microbiol. 17, 569–86. doi: 10.1038/s41579-019-0222-5
Chaillou, S., Champomier-Vergès, M.-C., Cornet, M., Crutz-Le Coq, A.-M., Dudez, A.-M., Martin, V., et al. (2005). The complete genome sequence of the meat-borne lactic acid bacterium Lactobacillus sakei 23K. Nat. Biotechnol. 23, 1527–33. doi: 10.1038/nbt1160
Chaillou, S., Ramaroson, M., Coeuret, G., Rossero, A., Anthoine, V., Champomier-Vergès, M., et al. (2022). Combination of high pressure treatment at 500 MPa and biopreservation with a Lactococcus lactis strain for lowering the bacterial growth during storage of diced cooked ham with reduced nitrite salt. Microorganisms 10:456. doi: 10.3390/microorganisms10020456
Chokesajjawatee, N., Santiyanont, P., Chantarasakha, K., Kocharin, K., Thammarongtham, C., Lertampaiporn, S., et al. (2020). Safety assessment of a Nham starter culture Lactobacillus plantarum BCC9546 via whole-genome analysis. Sci. Rep. 10:10241. doi: 10.1038/s41598-020-66857-2
Comi, G., Andyanto, D., Manzano, M., and Iacumin, L. (2016). Lactococcus lactis and Lactobacillus sakei as bio-protective culture to eliminate Leuconostoc mesenteroides spoilage and improve the shelf life and sensorial characteristics of commercial cooked bacon. Food Microbiol. 58, 16–22. doi: 10.1016/j.fm.2016.03.001
Connell, J. L., Kim, J., Shear, J. B., Bard, A. J., and Whiteley, M. (2014). Real-time monitoring of quorum sensing in 3D-printed bacterial aggregates using scanning electrochemical microscopy. Proc. Natl. Acad. Sci. U S A. 111, 18255–60. doi: 10.1073/pnas.1421211111
Connell, J. L., Ritschdorff, E. T., Whiteley, M., and Shear, J. B. (2013). 3D printing of microscopic bacterial communities. Proc. Natl. Acad. Sci. U S A. 110, 18380–5. doi: 10.1073/pnas.1309729110
Connell, J. L., Whiteley, M., and Shear, J. B. (2012). Sociomicrobiology in engineered landscapes. Nat. Chem. Biol. 8, 10–3. doi: 10.1038/nchembio.749
Consentino, L., Rejasse, A., Crapart, N., Bevilacqua, C., and Nielsen-LeRoux, C. (2021). Laser capture microdissection to study Bacillus cereus iron homeostasis gene expression during Galleria mellonella in vivo gut colonization. Virulence 12, 2104–21. doi: 10.1080/21505594.2021.1959790
Cook, P. W., and Nightingale, K. K. (2018). Use of omics methods for the advancement of food quality and food safety. Anim. Front. 8, 33–41. doi: 10.1093/af/vfy024
Couvert, O., Pinon, A., Bergis, H., Bourdichon, F., Carlin, F., Cornu, M., et al. (2010). Validation of a stochastic modelling approach for Listeria monocytogenes growth in refrigerated foods. Int. J. Food Microbiol. 144, 236–42. doi: 10.1016/j.ijfoodmicro.2010.09.024
Cretenet, M., Nouaille, S., Thouin, J., Rault, L., Stenz, L., François, P., et al. (2011). Staphylococcus aureus virulence and metabolism are dramatically affected by Lactococcus lactis in cheese matrix: S. aureus interaction with L. lactis in cheese matrix. Environ. Microbiol. Rep. 3, 340–51. doi: 10.1111/j.1758-2229.2010.00230.x
Dar, D., Dar, N., Cai, L., and Newman, D. K. (2021). Spatial transcriptomics of planktonic and sessile bacterial populations at single-cell resolution. Science 373:eabi4882. doi: 10.1126/science.abi4882
Darsonval, M., Grégoire, M., Deschamps, J., and Briandet, R. (2021). “Confocal laser microscopy analysis of Listeria monocytogenes biofilms and spatially organized communities,” in Listeria monocytogenes. Methods Mol Biol, eds E. M. Fox, H. Bierne, and B. Stessl (New York, NY: Springer), 123–36. doi: 10.1007/978-1-0716-0982-8_10
Delavenne, E., Déniel, F., Barbier, G., and Le Blay, G. (2012). Biodiversity of antifungal lactic acid bacteria isolated from raw milk samples from cow, ewe and goat over one-year period. Int. J. Food Microbiol. 155, 185–90. doi: 10.1016/j.ijfoodmicro.2012.02.003
Delbes-Paus, C., Dorchies, G., Chaabna, Z., Callon, C., and Montel, M.-C. (2010). Contribution of hydrogen peroxide to the inhibition of Staphylococcus aureus by Lactococcus garvieae in interaction with raw milk microbial community. Food Microbiol. 27, 924–32. doi: 10.1016/j.fm.2010.05.031
Delpech, P., Bornes, S., Alaterre, E., Bonnet, M., Gagne, G., Montel, M.-C., et al. (2015). Staphylococcus aureus transcriptomic response to inhibition by H2O2-producing Lactococcus garvieae. Food Microbiol. 51, 163–70. doi: 10.1016/j.fm.2015.05.014
Delpech, P., Rifa, E., Ball, G., Nidelet, S., Dubois, E., Gagne, G., et al. (2017). New insights into the anti-pathogenic potential of Lactococcus garvieae against Staphylococcus aureus based on RNA sequencing Profiling. Front. Microbiol. 8:359. doi: 10.3389/fmicb.2017.00359
Duhutrel, P., Bordat, C., Wu, T.-D., Zagorec, M., Guerquin-Kern, J.-L., and Champomier-Vergès, M.-C. (2010). Iron sources used by the nonpathogenic lactic acid bacterium Lactobacillus sakei as revealed by electron energy loss spectroscopy and secondary-ion mass spectrometry. Appl. Environ. Microbiol. 76, 560–5. doi: 10.1128/AEM.02205-09
Ebrahimi, P., Larsen, F. H., Jensen, H. M., Vogensen, F. K., and Engelsen, S. B. (2016). Real-time metabolomic analysis of lactic acid bacteria as monitored by in vitro NMR and chemometrics. Metabolomics 12, 1–17. doi: 10.1007/s11306-016-0996-7
Edgar, R. C. (2017). UNBIAS: an attempt to correct abundance bias in 16S sequencing, with limited success. bioRxiv 2017:124149. doi: 10.1101/124149
Eijsink, V. G. H., and Axelsson, L. (2005). Bacterial lessons in sausage making. Nat. Biotechnol. 23, 1494–5. doi: 10.1038/nbt1205-1494
El Kheir, S. M., Cherrat, L., Awussi, A. A., Ramia, N. E., Taha, S., Rahman, A., et al. (2018). High-throughput identification of candidate strains for biopreservation by using bioluminescent Listeria monocytogenes. Front. Microbiol. 9:1883. doi: 10.3389/fmicb.2018.01883
Ellegaard, K. M., and Engel, P. (2016). Beyond 16S rRNA community profiling: intra-species diversity in the gut microbiota. Front. Microbiol. 7:1475. doi: 10.3389/fmicb.2016.01475
Elsser-Gravesen, D., and Elsser-Gravesen, A. (2013). “Biopreservatives,” in Biotechnology of Food and Feed Additives Advances in Biochemical Engineering/Biotechnology, eds H. Zorn and P. Czermak (Berlin: Springer), 29–49. doi: 10.1007/10_2013_234
Ercolini, D., Ferrocino, I., La Storia, A., Mauriello, G., Gigli, S., Masi, P., et al. (2010). Development of spoilage microbiota in beef stored in nisin activated packaging. Food Microbiol. 27, 137–43. doi: 10.1016/j.fm.2009.09.006
Even, S., Charlier, C., Nouaille, S., Ben Zakour, N. L., Cretenet, M., Cousin, F. J., et al. (2009). Staphylococcus aureus virulence expression is impaired by Lactococcus lactis in mixed cultures. Appl. Environ. Microbiol. 75, 4459–72. doi: 10.1128/AEM.02388-08
Fall, P. A., Pilet, M. F., Leduc, F., Cardinal, M., Duflos, G., Guérin, C., et al. (2012). Sensory and physicochemical evolution of tropical cooked peeled shrimp inoculated by Brochothrix thermosphacta and Lactococcus piscium CNCM I-4031 during storage at 8°C. Int. J. Food Microbiol. 152, 82–90. doi: 10.1016/j.ijfoodmicro.2011.07.015
FAO (2019). State of Food and Agriculture 2019. Moving Forward on Food Loss and Waste Reduction. Rome: FAO.
Ferrier, R., Hezard, B., Lintz, A., Stahl, V., and Augustin, J.-C. (2013). Combining individual-based modeling and food microenvironment descriptions to predict the growth of Listeria monocytogenes on smear soft cheese. Appl. Environ. Microbiol. 2013:13. doi: 10.1128/AEM.01311-13
Ferrocino, I., Greppi, A., Storia, A. L., Rantsiou, K., Ercolini, D., and Cocolin, L. (2015). Impact of nisin-activated packaging on microbiota of beef burgers during storage. Appl. Environ. Microbiol. 2015:15. doi: 10.1128/AEM.03093-15
Finnegan, M., Linley, E., Denyer, S. P., McDonnell, G., Simons, C., and Maillard, J. Y. (2010). Mode of action of hydrogen peroxide and other oxidizing agents: differences between liquid and gas forms. J. Antimicrob. Chemother. 65, 2108–15. doi: 10.1093/jac/dkq308
Fleurot, I., Aigle, M., Fleurot, R., Darrigo, C., Hennekinne, J.-A., Gruss, A., et al. (2014). Following pathogen development and gene expression in a food ecosystem: the case of a Staphylococcus aureus isolate in cheese. Appl. Environ. Microbiol. 80, 5106–15. doi: 10.1128/AEM.01042-14
Frétin, M., Chassard, C., Delbès, C., Lavigne, R., Rifa, E., Theil, S., et al. (2020). Robustness and efficacy of an inhibitory consortium against E. coli O26:H11 in raw milk cheeses. Food Control 115:107282. doi: 10.1016/j.foodcont.2020.107282
Galia, W., Leriche, F., Cruveiller, S., Garnier, C., Navratil, V., Dubost, A., et al. (2017). Strand-specific transcriptomes of Enterohemorrhagic Escherichia coli in response to interactions with ground beef microbiota: interactions between microorganisms in raw meat. BMC Genom. 18:574. doi: 10.1186/s12864-017-3957-2
Gálvez, A., López, R. L., and Ben Omar, N. (2007). Bacteriocin-based strategies for food biopreservation. Int. J. Food Microbiol. 120, 51–70. doi: 10.1016/j.ijfoodmicro.2007.06.001
Garnier, L., Penland, M., Thierry, A., Maillard, M.-B., Jardin, J., Coton, M., et al. (2020). Antifungal activity of fermented dairy ingredients: identification of antifungal compounds. Int. J. Food Microbiol. 322:108574. doi: 10.1016/j.ijfoodmicro.2020.108574
Giello, M., La Storia, A., De Filippis, F., Ercolini, D., and Villani, F. (2018). Impact of Lactobacillus curvatus 54M16 on microbiota composition and growth of Listeria monocytogenes in fermented sausages. Food Microbiol. 72, 1–15. doi: 10.1016/j.fm.2017.11.003
Guillier, L., Stahl, V., Hezard, B., Notz, E., and Briandet, R. (2008). Modelling the competitive growth between Listeria monocytogenes and biofilm microflora of smear cheese wooden shelves. Int. J. Food Microbiol. 128, 51–7. doi: 10.1016/j.ijfoodmicro.2008.06.028
Gyimah, N., Scheler, O., Rang, T., and Pardy, T. (2021). Can 3D printing bring droplet microfluidics to every lab?—A systematic review. Micromachines 12:339. doi: 10.3390/mi12030339
Habimana, O., Guillier, L., Kulakauskas, S., and Briandet, R. (2011). Spatial competition with Lactococcus lactis in mixed-species continuous-flow biofilms inhibits Listeria monocytogenes growth. Biofouling 27, 1065–72. doi: 10.1080/08927014.2011.626124
Hebert, E. M., Saavedra, L., Taranto, M. P., Mozzi, F., Magni, C., Nader, M. E. F., et al. (2012). Genome sequence of the bacteriocin-producing Lactobacillus curvatus strain CRL705. J. Bacteriol. 194, 538–9. doi: 10.1128/JB.06416-11
Hernández-Galán, L., Cattenoz, T., Le Feunteun, S., Canette, A., Briandet, R., Le-Guin, S., et al. (2017). Effect of dairy matrices on the survival of Streptococcus thermophilus, Brevibacterium aurantiacum and Hafnia alvei during digestion. Food Res. Int. 100, 477–88. doi: 10.1016/j.foodres.2017.07.044
Hibbing, M. E., Fuqua, C., Parsek, M. R., and Peterson, S. B. (2010). Bacterial competition: surviving and thriving in the microbial jungle. Nat. Rev. Microbiol. 8, 15–25. doi: 10.1038/nrmicro2259
Gänzle, M. G., Höltzel, A., Walter, J., Jung, G., and Hammes, W. P. (2000). Characterization of reutericyclin produced by Lactobacillus reuteri LTH2584. Appl. Environ. Microbiol. 66, 4325–33. doi: 10.1128/AEM.66.10.4325-4333.2000
HPLE (2014). “Food losses and waste in the context of sustainable food systems,” in A report by the high level panel of experts on food security and nutrition. HLPE Report 8, (Rome: FAO).
Hu, P., Xu, X. L., Zhou, G. H., Han, Y. Q., Xu, B. C., and Liu, J. C. (2008). Study of the Lactobacillus sakei protective effect towards spoilage bacteria in vacuum packed cooked ham analyzed by PCR-DGGE. Meat Sci. 80, 462–9. doi: 10.1016/j.meatsci.2008.01.011
Hynes, W. F., Chacón, J., Segrè, D., Marx, C. J., Cady, N. C., and Harcombe, W. R. (2018). Bioprinting microbial communities to examine interspecies interactions in time and space. Biomed. Phys. Eng. Express 4:55010. doi: 10.1088/2057-1976/aad544
Iacumin, L., Cappellari, G., Colautti, A., and Comi, G. (2020). Listeria monocytogenes survey in cubed cooked ham packaged in modified atmosphere and bioprotective effect of selected lactic acid bacteria. Microorganisms 8:898. doi: 10.3390/microorganisms8060898
Jameson, J. E. (1962). A discussion of the dynamics of salmonella enrichment. J. Hyg. 60, 193–207. doi: 10.1017/s0022172400039462
Jeanson, S., Floury, J., Gagnaire, V., Lortal, S., and Thierry, A. (2015). Bacterial colonies in solid media and foods: a review on their growth and interactions with the micro-environment. Front. Microbiol. 6:1284. doi: 10.3389/fmicb.2015.01284
Joffraud, J.-J., Cardinal, M., Cornet, J., Léon, S., Gigout, F., et al. (2006). Effect of bacterial interactions on the spoilage of cold-smoked salmon. Int. J. Food Microbiol. 112, 51–61. doi: 10.1016/j.ijfoodmicro.2006.05.014
Jones, R. J., Wiklund, E., Zagorec, M., and Tagg, J. R. (2010). Evaluation of stored lamb bio-preserved using a three-strain cocktail of Lactobacillus sakei. Meat Sci. 86, 955–9. doi: 10.1016/j.meatsci.2010.07.023
Koutsoumanis, K. P., Kendall, P. A., and Sofos, J. N. (2004). A comparative study on growth limits of Listeria monocytogenes as affected by temperature, pH and aw when grown in suspension or on a solid surface. Food Microbiol. 21, 415–22. doi: 10.1016/j.fm.2003.11.003
Krishna Kumar, R., Meiller-Legrand, T. A., Alcinesio, A., Gonzalez, D., Mavridou, D. A. I., Meacock, O. J., et al. (2021). Droplet printing reveals the importance of micron-scale structure for bacterial ecology. Nat. Commun. 12:857. doi: 10.1038/s41467-021-20996-w
Kyle, S. (2018). 3D printing of bacteria: the next frontier in bofabrication. Trends Biotechnol. 36, 340–1. doi: 10.1016/j.tibtech.2018.01.010
Laursen, M. F., Bahl, M. I., Licht, T. R., Gram, L., and Knudsen, G. M. (2015). A single exposure to a sublethal pediocin concentration initiates a resistance-associated temporal cell envelope and general stress response in Listeria monocytogenes. Environ. Microbiol. 17, 1134–51. doi: 10.1111/1462-2920.12534
Ledenbach, L. H., and Marshall, R. T. (2009). “Microbiological spoilage of dairy products,” in Compendium of the Microbiological Spoilage of Foods and Beverages, eds W. H. Sperber and M. P. Doyle (New York, NY: Springer), 41–67. doi: 10.1007/978-1-4419-0826-1_2
Leisner, J. J., Laursen, B. G., Prevost, H., Drider, D., and Dalgaard, P. (2007). Carnobacterium: positive and negative effects in the environment and in foods. FEMS Microbiol. Rev. 31, 592–613. doi: 10.1111/j.1574-6976.2007.00080.x
Lenz, A. P., Williamson, K. S., Pitts, B., Stewart, P. S., and Franklin, M. J. (2008). Localized gene expression in Pseudomonas aeruginosa biofilms. Appl. Environ. Microbiol. 74, 4463–71. doi: 10.1128/AEM.00710-08
Leroi, F., Cornet, J., Chevalier, F., Cardinal, M., Coeuret, G., Chaillou, S., et al. (2015). Selection of bioprotective cultures for preventing cold-smoked salmon spoilage. Int. J. Food Microbiol. 213, 79–87. doi: 10.1016/j.ijfoodmicro.2015.05.005
Leroy, F., and De Vuyst, L. (2003). A combined model to predict the functionality of the bacteriocin-producing Lactobacillus sakei strain CTC 494. Appl. Environ. Microbiol. 69, 1093–9. doi: 10.1128/AEM.69.2.1093-1099.2003
Leyva Salas, M., Mounier, J., Maillard, M.-B., Valence, F., Coton, E., and Thierry, A. (2019). Identification and quantification of natural compounds produced by antifungal bioprotective cultures in dairy products. Food Chem. 301:125260. doi: 10.1016/j.foodchem.2019.125260
Leyva Salas, M., Mounier, J., Valence, F., Coton, M., Thierry, A., and Coton, E. (2017). Antifungal microbial agents for food biopreservation-A Review. Microorganisms 5:5030037. doi: 10.3390/microorganisms5030037
Leyva Salas, M., Thierry, A., Lemaître, M., Garric, G., Harel-Oger, M., Chatel, M., et al. (2018). Antifungal activity of lactic acid bacteria combinations in dairy mimicking models and their potential as bioprotective cultures in pilot scale applications. Front. Microbiol. 9:1787. doi: 10.3389/fmicb.2018.01787
Li, J., Yang, X., Shi, G., Chang, J., Liu, Z., and Zeng, M. (2019). Cooperation of lactic acid bacteria regulated by the AI-2/LuxS system involve in the biopreservation of refrigerated shrimp. Food Res. Int. 120, 679–87. doi: 10.1016/j.foodres.2018.11.025
Lin, X. B., Lohans, C. T., Duar, R., Zheng, J., Vederas, J. C., Walter, J., et al. (2015). Genetic determinants of reutericyclin biosynthesis in Lactobacillus reuteri. Appl. Environ. Microbiol. 2015:14. doi: 10.1128/AEM.03691-14
Liu, Y.-X., Qin, Y., Chen, T., Lu, M., Qian, X., Guo, X., et al. (2021). A practical guide to amplicon and metagenomic analysis of microbiome data. Protein Cell 12, 315–30. doi: 10.1007/s13238-020-00724-8
Luo, C., Knight, R., Siljander, H., Knip, M., Xavier, R. J., and Gevers, D. (2015). ConStrains identifies microbial strains in metagenomic datasets. Nat. Biotechnol. 33, 1045–52. doi: 10.1038/nbt.3319
Marché, L., Saraoui, T., Remenant, B., Zagorec, M., Prévost, H., Delbarre-Ladrat, C., et al. (2017). Complete genome sequence of Lactococcus piscium CNCM I-4031, a bioprotective strain for seafood products. Genome Announc. 5:16. doi: 10.1128/genomeA.01510-16
Martinez, R. C. R., Alvarenga, V. O., ávaro-Trindade, C. S., Sant’Ana, A., and de, S. (2016). Assessment of the inhibitory effect of free and encapsulated commercial nisin (Nisaplin®), tested alone and in combination, on Listeria monocytogenes and Bacillus cereus in refrigerated milk. LWT - Food Sci. Technol. 68, 67–75. doi: 10.1016/j.lwt.2015.12.027
McNair, L. K. F., Siedler, S., Vinther, J. M. O., Hansen, A. M., Neves, A. R., Garrigues, C., et al. (2018). Identification and characterization of a new antifungal peptide in fermented milk product containing bioprotective Lactobacillus cultures. FEMS Yeast Res. 18:foy094. doi: 10.1093/femsyr/foy094
Meola, M., Rifa, E., Shani, N., Delbès, C., Berthoud, H., and Chassard, C. (2019). DAIRYdb: a manually curated reference database for improved taxonomy annotation of 16S rRNA gene sequences from dairy products. BMC Genom. 20:560. doi: 10.1186/s12864-019-5914-8
Metivier, A., Pilet, M.-F., Dousset, X., Sorokine, O., Anglade, P., Zagorec, M., et al. (1998). Divercin V41, a new bacteriocin with two disulphide bonds produced by Carnobacterium divergens V41: primary structure and genomic organization. Microbiology 144, 2837–44. doi: 10.1099/00221287-144-10-2837
Møller, C. O. A., Ilg, Y., Aabo, S., Christensen, B. B., Dalgaard, P., and Hansen, T. B. (2013). Effect of natural microbiota on growth of Salmonella spp. in fresh pork – A predictive microbiology approach. Food Microbiol. 34, 284–95. doi: 10.1016/j.fm.2012.10.010
Moon, S., Fritz, I. L., Singer, Z. S., and Danino, T. (2016). Spatial control of bacteria using screen printing. 3D Print Addit. Manuf 3, 194–203. doi: 10.1089/3dp.2016.0040
Nogueira Viçosa, G., Vieira Botelho, C., Botta, C., Bertolino, M., Fernandes de Carvalho, A., Nero, L. A., et al. (2019). Impact of co-cultivation with Enterococcus faecalis over growth, enterotoxin production and gene expression of Staphylococcus aureus in broth and fresh cheeses. Int. J. Food Microbiol. 308:108291. doi: 10.1016/j.ijfoodmicro.2019.108291
Nouaille, S., Even, S., Charlier, C., Le Loir, Y., Cocaign-Bousquet, M., and Loubière, P. (2009). Transcriptomic response of Lactococcus lactis in mixed culture with Staphylococcus aureus. Appl. Environ. Microbiol. 75, 4473–82. doi: 10.1128/AEM.02653-08
Nouaille, S., Rault, L., Jeanson, S., Loubière, P., Le Loir, Y., and Even, S. (2014). Contribution of Lactococcus lactis reducing properties to the downregulation of a major virulence regulator in Staphylococcus aureus, the agr system. Appl. Environ. Microbiol. 80, 7028–35. doi: 10.1128/AEM.02287-14
Orihuel, A., Terán, L., Renaut, J., Planchon, S., Valacco, M. P., Masias, E., et al. (2019). Physiological and proteomic response of Escherichia coli O157:H7 to a bioprotective lactic acid bacterium in a meat environment. Food Res. Int. 125:108622. doi: 10.1016/j.foodres.2019.108622
Orihuel, A., Terán, L., Renaut, J., Vignolo, G. M., De Almeida, A. M., Saavedra, M. L., et al. (2018). Differential proteomic analysis of lactic acid bacteria-Escherichia coli O157:H7 interaction and its contribution to bioprotection strategies in Meat. Front. Microbiol. 9:1083. doi: 10.3389/fmicb.2018.01083
Ortiz-Rivera, Y., Sánchez-Vega, R., Gutiérrez-Méndez, N., León-Félix, J., Acosta-Muñiz, C., and Sepulveda, D. R. (2017). Production of reuterin in a fermented milk product by Lactobacillus reuteri: inhibition of pathogens, spoilage microorganisms, and lactic acid bacteria. J. Dairy Sci. 100, 4258–68. doi: 10.3168/jds.2016-11534
Park, E. J., Kim, K. H., Abell, G. C. J., Kim, M. S., Roh, S. W., and Bae, J. W. (2011). Metagenomic analysis of the viral communities in fermented foods. Appl. Environ. Microbiol. 77, 1284–91. doi: 10.1128/AEM.01859-10
Pérez-Osorio, A. C., and Franklin, M. J. (2008). Isolation of RNA and DNA from biofilm samples obtained by laser capture microdissection microscopy. CSH Protoc. 2008:rot5065. doi: 10.1101/pdb.prot5065
Pinilla, C. M. B., Stincone, P., and Brandelli, A. (2021). Proteomic analysis reveals differential responses of Listeria monocytogenes to free and nanoencapsulated nisin. Int. J. Food Microbiol. 346:109170. doi: 10.1016/j.ijfoodmicro.2021.109170
Poirier, S., Coeuret, G., Champomier-Vergès, M.-C., and Chaillou, S. (2018). Draft genome sequences of nine strains of Brochothrix thermosphacta, Carnobacterium divergens, Lactobacillus algidus, Lactobacillus fuchuensis, Lactococcus piscium, Leuconostoc gelidum subsp. gasicomitatum, Pseudomonas lundensis, and Weissella viridescens, a collection of psychrotrophic species involved in meat and seafood spoilage. Genome Announc. 6, e479–418. doi: 10.1128/genomeA.00479-18
Ramaroson, M., Guillou, S., Rossero, A., Rezé, S., Anthoine, V., Moriceau, N., et al. (2018). Selection procedure of bioprotective cultures for their combined use with High Pressure Processing to control spore-forming bacteria in cooked ham. Int. J. Food Microbiol. 276, 28–38. doi: 10.1016/j.ijfoodmicro.2018.04.010
Rathod, N. B., Nirmal, N. P., Pagarkar, A., Özogul, F., and Rocha, J. M. (2022). Antimicrobial impacts of microbial metabolites on the preservation of fish and fishery products: a review with current knowledge. Microorganisms 10:773. doi: 10.3390/microorganisms10040773
Remenant, B., Borges, F., Cailliez-Grimal, C., Revol-Junelles, A.-M., Marché, L., Lajus, A., et al. (2016). Draft genome sequence of Carnobacterium divergens V41, a bacteriocin-producing strain. Genome Announc. 4, e1109–16. doi: 10.1128/genomeA.01109-16
Riedel, C. U., Monk, I. R., Casey, P. G., Morrissey, D. O., Sullivan, G. C., Tangney, M., et al. (2007). Improved luciferase tagging system for Listeria monocytogenes allows real-time monitoring in vivo and in vitro. Appl. Environ. Microbiol. 73, 3091–4. doi: 10.1128/AEM.02940-06
Rimaux, T., Vrancken, G., Vuylsteke, B., De Vuyst, L., and Leroy, F. (2011). The pentose moiety of adenosine and inosine is an important energy source for the fermented-meat starter culture Lactobacillus sakei CTC 494. Appl. Environ. Microbiol. 77, 6539–50. doi: 10.1128/AEM.00498-11
Roh, S. W., Kim, K. H., Nam, Y. D., et al. (2010). Investigation of archaeal and bacterial diversity in fermented seafood using barcoded pyrosequencing. ISME J. 4, 1–16. doi: 10.1038/ismej.2009.83
Rothschild, L. J. (2016). Synthetic biology meets bioprinting: enabling technologies for humans on Mars (and Earth). Biochem. Soc. Trans. 44, 1158–64. doi: 10.1042/BST20160067
Saint Martin, C., Darsonval, M., Grégoire, M., Caccia, N., Midoux, L., Berland, S., et al. (2022). Spatial organisation of Listeria monocytogenes and Escherichia coli O157:H7 cultivated in gel matrices. Food Microbiol. 103:103965. doi: 10.1016/j.fm.2021.103965
Saraoui, T., Cornet, J., Guillouet, E., Pilet, M. F., Chevalier, F., Joffraud, J.-J., et al. (2017). Improving simultaneously the quality and safety of cooked and peeled shrimp using a cocktail of bioprotective lactic acid bacteria. Int. J. Food Microbiol. 241, 69–77. doi: 10.1016/j.ijfoodmicro.2016.09.024
Saraoui, T., Fall, P. A., Leroi, F., Antignac, J.-P., Chéreau, S., and Pilet, M. F. (2016). Inhibition mechanism of Listeria monocytogenes by a bioprotective bacteria Lactococcus piscium CNCM I-4031. Food Microbiol. 53, 70–8. doi: 10.1016/j.fm.2015.01.002
Saraoui, T., Leroi, F., Chevalier, F., Cappelier, J.-M., Passerini, D., and Pilet, M.-F. (2018). Bioprotective Effect of Lactococcus piscium CNCM I-4031 against Listeria monocytogenes growth and virulence. Front. Microbiol. 9:1564. doi: 10.3389/fmicb.2018.01564
Schlusselhuber, M., Godard, J., Sebban, M., Bernay, B., Garon, D., Seguin, V., et al. (2018). Characterization of milkisin, a novel lipopeptide with antimicrobial properties produced by Pseudomonas sp. UCMA 17988 isolated from bovine raw milk. Front. Microbiol. 9:1030. doi: 10.3389/fmicb.2018.01030
Schlusselhuber, M., Godard, J., Sebban, M., Bernay, B., Garon, D., Seguin, V., et al. (2020). Corrigendum: characterization of milkisin, a novel lipopeptide with antimicrobial properties produced by Pseudomonas sp. UCMA 17988 isolated from bovine raw milk. Front. Microbiol. 11:1323. doi: 10.3389/fmicb.2020.01323
Shi, C., and Knøchel, S. (2021a). Inhibitory effects of binary combinations of microbial metabolites on the growth of tolerant Penicillium roqueforti and Mucor circinelloides. LWT 149:112039. doi: 10.1016/j.lwt.2021.112039
Shi, C., and Knøchel, S. (2021b). Susceptibility of dairy associated molds towards microbial metabolites with focus on the response to diacetyl. Food Control 121:107573. doi: 10.1016/j.foodcont.2020.107573
Shi, C., and Maktabdar, M. (2022). Lactic acid bacteria as biopreservation against spoilage molds in dairy products – A review. Front. Microbiol. 12:819684. doi: 10.3389/fmicb.2021.819684
Siedler, S., Balti, R., and Neves, A. R. (2019). Bioprotective mechanisms of lactic acid bacteria against fungal spoilage of food. Curr. Opin. Biotechnol. 56, 138–46. doi: 10.1016/j.copbio.2018.11.015
Silbande, A., Cornet, J., Cardinal, M., Chevalier, F., Rochefort, K., Smith-Ravin, J., et al. (2018). Characterization of the spoilage potential of pure and mixed cultures of bacterial species isolated from tropical yellowfin tuna (Thunnus albacares). J. Appl. Microbiol. 124, 559–71. doi: 10.1111/jam.13663
Simonin, S., Roullier-Gall, C., Ballester, J., Schmitt-Kopplin, P., Quintanilla-Casas, B., Vichi, S., et al. (2020). Bio-protection as an alternative to sulphites: impact on chemical and microbial characteristics of red wines. Front. Microbiol. 11:1308. doi: 10.3389/fmicb.2020.01308
Spanu, C., Piras, F., Mocci, A. M., Nieddu, G., De Santis, E. P. L., and Scarano, C. (2018). Use of Carnobacterium spp protective culture in MAP packed Ricotta fresca cheese to control Pseudomonas spp. Food Microbiol. 74, 50–6. doi: 10.1016/j.fm.2018.02.020
Stiles, M. E. (1996). Biopreservation by lactic acid bacteria. Antonie van Leeuwenhoek 70, 331–45. doi: 10.1007/BF00395940
Stincone, P., Comerlato, C. B., and Brandelli, A. (2021). Proteomic analysis of Listeria monocytogenes exposed to free and nanostructured antimicrobial lipopeptides. Mol. Omics 17, 426–37. doi: 10.1039/D0MO00178C
Stohr, V., Joffraud, J. J., Cardinal, M., and Leroi, F. (2001). Spoilage potential and sensory profile associated with bacteria isolated from cold-smoked salmon. Food Res. Int. 34, 797–806. doi: 10.1016/S0963-9969(01)00101-6
Ström, K., Sjögren, J., Broberg, A., and Schnürer, J. (2002). Lactobacillus plantarum MiLAB 393 produces the antifungal cyclic dipeptides cyclo(L -Phe- L -Pro) and cyclo(L -Phe- trans -4-OH- L -Pro) and 3-phenyllactic acid. Appl. Environ. Microbiol. 68, 4322–7. doi: 10.1128/AEM.68.9.4322-4327.2002
Tabanelli, G., Barbieri, F., Campedelli, I., Venturini, M. C., Gardini, F., and Montanari, C. (2020). Effects of bioprotective cultures on the microbial community during storage of Italian fresh filled pasta. Food Control 115:107304. doi: 10.1016/j.foodcont.2020.107304
Truchado, P., Elsser-Gravesen, A., Gil, M. I., and Allende, A. (2020). Post-process treatments are effective strategies to reduce Listeria monocytogenes on the surface of leafy greens: a pilot study. Int. J. Food Microbiol. 313:108390. doi: 10.1016/j.ijfoodmicro.2019.108390
van Heel, A. J., de Jong, A., Song, C., Viel, J. H., Kok, J., and Kuipers, O. P. (2018). BAGEL4: a user-friendly web server to thoroughly mine RiPPs and bacteriocins. Nucleic Acids Res. 46, W278–81. doi: 10.1093/nar/gky383
Verheyen, D., and Van Impe, J. F. M. (2021). The inclusion of the food microstructural influence in predictive microbiology: state-of-the-art. Foods 10:2119. doi: 10.3390/foods10092119
Verheyen, D., érez-Rodríguez, F., Baka, M., Skåra, T., and Van Impe, J. F. (2018). Effect of food microstructure on growth dynamics of Listeria monocytogenes in fish-based model systems. Int. J. Food Microbiol. 283, 7–13. doi: 10.1016/j.ijfoodmicro.2018.05.032
Verplaetse, E., André-Leroux, G., Duhutrel, P., Coeuret, G., Chaillou, S., Nielsen-Leroux, C., et al. (2020). Heme uptake in Lactobacillus sakei evidenced by a new energy coupling factor (ECF)-like transport system. Appl. Environ. Microbiol. 2020:19. doi: 10.1128/AEM.02847-19
Viçosa, G. N., Botta, C., Ferrocino, I., Bertolino, M., Ventura, M., Nero, L. A., et al. (2018). Staphylococcus aureus undergoes major transcriptional reorganization during growth with Enterococcus faecalis in milk. Food Microbiol. 73, 17–28. doi: 10.1016/j.fm.2018.01.007
Wang, X., Wang, S., and Zhao, H. (2019). Unravelling microbial community diversity and succession of Chinese Sichuan sausages during spontaneous fermentation by high-throughput sequencing. J. Food Sci. Technol. 56, 3254–63. doi: 10.1007/s13197-019-03781-y
Warnecke, T., and Gill, R. T. (2005). Organic acid toxicity, tolerance, and production in Escherichia coli biorefining applications. Microb. Cell Fact 4:25. doi: 10.1186/1475-2859-4-25
Wessel, A. K., Hmelo, L., Parsek, M. R., and Whiteley, M. (2013). Going local: technologies for exploring bacterial microenvironments. Nat. Rev. Microbiol. 11, 337–48. doi: 10.1038/nrmicro3010
Wiernasz, N., Cornet, J., Cardinal, M., Pilet, M.-F., Passerini, D., and Leroi, F. (2017). Lactic acid bacteria selection for biopreservation as a part of hurdle technology approach applied on seafood. Front. Mar. Sci. 4:119. doi: 10.3389/fmars.2017.00119
Wiernasz, N., Leroi, F., Chevalier, F., Cornet, J., Cardinal, M., Rohloff, J., et al. (2020). Salmon Gravlax biopreservation with lactic acid bacteria: a polyphasic approach to assessing the impact on organoleptic properties, microbial ecosystem and volatilome composition. Front. Microbiol. 10:3103. doi: 10.3389/fmicb.2019.03103
Windholtz, S., Redon, P., Lacampagne, S., Farris, L., Lytra, G., Cameleyre, M., et al. (2021). Non-Saccharomyces yeasts as bioprotection in the composition of red wine and in the reduction of sulfur dioxide. LWT 149:111781. doi: 10.1016/j.lwt.2021.111781
World Health Organization (2015). WHO Estimates of the Global Burden of Foodborne Diseases: Foodborne Disease Burden Epidemiology Reference Group 2007-2015. Geneva: World Health Organization.
Wu, W., Deng, G., Liu, C., Gong, X., Ma, G., Yuan, Q., et al. (2020). Optimization and multiomic basis of phenyllactic acid overproduction by Lactobacillus plantarum. J. Agric. Food Chem. 68, 1741–9. doi: 10.1021/acs.jafc.9b07136
Yi, L., Luo, L., and Lü, X. (2018). Efficient exploitation of multiple novel bacteriocins by combination of complete genome and peptidome. Front. Microbiol. 9:1567. doi: 10.3389/fmicb.2018.01567
Zagorec, M., and Champomier-Vergès, M.-C. (2017). Lactobacillus sakei: a starter for sausage fermentation, a protective culture for meat products. Microorganisms 5:56. doi: 10.3390/microorganisms5030056
Zdenkova, K., Alibayov, B., Karamonova, L., Purkrtova, S., Karpiskova, R., and Demnerova, K. (2016). Transcriptomic and metabolic responses of Staphylococcus aureus in mixed culture with Lactobacillus plantarum, Streptococcus thermophilus and Enterococcus durans in milk. J. Ind. Microbiol. Biotechnol 43, 1237–47. doi: 10.1007/s10295-016-1794-y
Zhang, Y., Zhu, L., Dong, P., Liang, R., Mao, Y., Qiu, S., et al. (2018). Bio-protective potential of lactic acid bacteria: effect of Lactobacillus sakei and Lactobacillus curvatus on changes of the microbial community in vacuum-packaged chilled beef. Asian Austral. J. Anim. Sci. 31, 585–94. doi: 10.5713/ajas.17.0540
Keywords: shelf life, food, safety, pathogen, spoilage, microbiome, fermentation, biopreservation
Citation: Borges F, Briandet R, Callon C, Champomier-Vergès M-C, Christieans S, Chuzeville S, Denis C, Desmasures N, Desmonts M-H, Feurer C, Leroi F, Leroy S, Mounier J, Passerini D, Pilet M-F, Schlusselhuber M, Stahl V, Strub C, Talon R and Zagorec M (2022) Contribution of omics to biopreservation: Toward food microbiome engineering. Front. Microbiol. 13:951182. doi: 10.3389/fmicb.2022.951182
Received: 23 May 2022; Accepted: 14 July 2022;
Published: 02 August 2022.
Edited by:
Jerome Combrisson, Mars (United States), United StatesReviewed by:
Minakshi Prasad, Lala Lajpat Rai University of Veterinary and Animal Sciences, IndiaCopyright © 2022 Borges, Briandet, Callon, Champomier-Vergès, Christieans, Chuzeville, Denis, Desmasures, Desmonts, Feurer, Leroi, Leroy, Mounier, Passerini, Pilet, Schlusselhuber, Stahl, Strub, Talon and Zagorec. This is an open-access article distributed under the terms of the Creative Commons Attribution License (CC BY). The use, distribution or reproduction in other forums is permitted, provided the original author(s) and the copyright owner(s) are credited and that the original publication in this journal is cited, in accordance with accepted academic practice. No use, distribution or reproduction is permitted which does not comply with these terms.
*Correspondence: Frédéric Borges, ZnJlZGVyaWMuYm9yZ2VzQHVuaXYtbG9ycmFpbmUuZnI=
Disclaimer: All claims expressed in this article are solely those of the authors and do not necessarily represent those of their affiliated organizations, or those of the publisher, the editors and the reviewers. Any product that may be evaluated in this article or claim that may be made by its manufacturer is not guaranteed or endorsed by the publisher.
Research integrity at Frontiers
Learn more about the work of our research integrity team to safeguard the quality of each article we publish.