- 1Department of Environmental Microbiology, UFZ-Helmholtz Centre for Environmental Research, Leipzig, Germany
- 2Bio Pilot Plant, Leibniz Institute for Natural Product Research and Infection Biology - Hans-Knöll Institute, Jena, Germany
- 3Faculty of Biological Sciences, Friedrich Schiller University Jena, Jena, Germany
Microbial electrosynthesis (MES) from CO2 provides chemicals and fuels by driving the metabolism of microorganisms with electrons from cathodes in bioelectrochemical systems. These microorganisms are usually strictly anaerobic. At the same time, the anode reaction of bioelectrochemical systems is almost exclusively water splitting through the oxygen evolution reaction (OER). This creates a dilemma for MES development and engineering. Oxygen penetration to the cathode has to be excluded to avoid toxicity and efficiency losses while assuring low resistance. We show that this dilemma derives a strong need to identify novel reactor designs when using the OER as an anode reaction or to fully replace OER with alternative oxidation reactions.
Introduction
Microbial electrochemical synthesis (MES) is the execution of microbially catalyzed electrochemical reactions to transform a substance into the desired product (Schröder et al., 2015). In simple words, MES is the generation of valuable extracellular multicarbon materials using electric energy based on combining microbial and electrochemical transformations (Zhang et al., 2013). MES can be based on anodic as well as cathodic reactions and covers the production of a variety of chemicals (refer to also Table 1) (Schröder et al., 2015). One highly interesting carbon substrate for cathodic MES is carbon dioxide (CO2).
Strategies to utilize CO2 as feedstock for the production of chemicals and fuels are of general high interest. Using CO2 shall allow creating sustainable carbon neutral (or even carbon negative) production routes and thus set the foundation for a circular biobased economy. CO2 is chemically stable; therefore, its utilization for chemical as well as (abiotic) electrochemical transformations requires large activation energy, respectively, overpotential (Gawel et al., 2022). In addition to chemical, biological synthesis from CO2 is also intensively researched, including photobiological synthesis or gas fermentation, which has been proven to yield multicarbon products from CO2 (Kondaveeti et al., 2020). These biological CO2 capturing methods possess benefits such as being based on widely available materials, ambient working conditions, being free of hazardous compounds, and being sustainable (Bajracharya et al., 2016a). In this line, MES that is using CO2 as carbon feedstock at cathodes was most deeply investigated. Hereby, electrons are supplied via a cathode to reduce carbon dioxide into products mainly by obligate anaerobic microorganisms that are capable of using the Wood-Ljungdahl CO2 fixation pathway (Nevin et al., 2011; Igarashi and Kato, 2017).
Among the most common products gained from CO2 via MES are acetate (CH3COOH) and ethanol (C2H5OH) (Table 1). Sporomusa ovata, Sporomusa silvacetica, Sporomusa sphaeroides, Clostridium ljungdahlii, Clostridium aceticum, and Moorella thermoacetica (Table 1) are among the autotrophic microorganisms that have been observed to generate acetate from CO2 through this pathway (Nevin et al., 2011). Another important group of autotrophic biocatalysts fixes CO2 via MES to methane, with the methanogens Methanobacterium palustre and Methanococcus maripaludis being most investigated (Enzmann et al., 2019). All these autotrophic biocatalysts or bioelectrocatalysts have in common that they are obligate anaerobic. Obligate anaerobes cannot sustain oxygen as they depend on low-potential flavoproteins for their respiration (Buckel and Thauer, 2018). Any exposure to oxygen can result in the biochemical and electrochemical formation of superoxide and hydrogen peroxide that are leading to oxidative stress and cell damage (Imlay, 2002). Thus, even small traces of oxygen in the MES setup can strongly hamper bioproduction. This means that most microorganisms suitable for performing MES from CO2 require (strictly) oxygen-free that is anoxic conditions to grow well and unravel their full performance.
To overcome or avoid this threat of oxygen toward the biocatalysts, many MES systems are operated with undefined mixed cultures at the biocathode (Gildemyn et al., 2015; Patil et al., 2015; Bajracharya et al., 2016b). However, a number of competitive microbial reactions happen in these MES, and therefore, the product (usually acetate) concentration profile fluctuates strongly. In a pure culture biocathode; in contrast, the production profile is more stable and reliable, indicating that no or only few competitive processes are present. In a direct comparison of pure and mixed culture MES, the highest production rate of a mixed culture biocathode was 1.35 mM/day, corresponding to 50% current efficiency, meanwhile, for a pure culture biocathode, the maximal production rate was 2.4 mM/day, corresponding to 89% current recovery between days 11 and 12 (Bajracharya et al., 2015). However, while the product spectrum was more stable for the pure culture, the overall operation was more unstable, likely due to the higher performance sensitivity of the pure culture catalyst, and this maximal performance was held only for 2 days of operation. Working with mixed cultures also greatly limits the perspectives to diversify the product spectrum since the community driving force is going toward thermodynamically stable products such as methane or acetate when methanogenesis is suppressed. The target production of diverse biochemicals or the application of molecular engineering for production pathways is not possible with mixed cultures.
The reactors used for MES are termed bioelectrochemical systems (BESs). A whole plethora of BES architecture is currently used when performing MES from CO2 (Table 1). Thereby, two fundamentally different types of reactors can be distinguished, namely, one-chambered and two-chambered BES. In one-chambered BES, anodic and cathodic reactions proceed in the same electrolyte solution that is the microbial medium, whereas in two-chambered reactors, cathode and anode are physically separated but ionically connected. It is commonly considered that in one-chambered reactors, compounds formed at one electrode may hamper the reaction at the other one and vice versa, whereas in two-chambered reactors, this is not the case, but resistance induced by the membrane separator may limit the overall performance (Krieg et al., 2018). For BES, very often reactor designs are used that are deduced from classical (abiotic) electrochemical cells. Furthermore, BESs are often not only inadequately described in terms of bioprocess engineering but they are also unsuitable for process development and scale-up (Rosa et al., 2019).
Bioelectrochemical systems for MES from CO2 using autotrophic microorganisms are commonly operated with the oxygen evolution reaction (OER) as counter-reaction at the anode (vide infra). Considering the strictly anaerobic nature of the discussed cathodic microbial biocatalysts, the evolved oxygen might more than likely hamper the MES performance.
The challenges of designing bioelectrochemical systems for microbial electrosynthesis create a dilemma
Bioelectrochemical systems consist of an anode where oxidations take place and a cathode where reductions take place. In the case of two-chambered BES, a membrane is placed between the electrodes. When performing MES from CO2 at the cathode, the most common anode reaction is an electrolytic splitting of water by the OER (Eq. 1):
The oxygen formed at the anode can penetrate into the cathode chamber. It can have a strong impact on the reactions in the BES, as recently illustrated for cathodic microbial electrochemical sulfate reduction (Dai et al., 2022). The presence of oxygen at the cathode is very intuitive for one-chamber BES. Yet, also in two-chamber BES, penetration of oxygen from the anode chamber cannot be completely circumvented with a separator like a cation or a proton exchange membrane (Harnisch and Schröder, 2009; Rahman et al., 2018). If oxygen from the anode as well as from the reactor environment is reaching the cathode (Figure 1), this will have several detrimental effects on the cathodic MES performance. First, and very importantly, oxygen can be reduced by abiotic or biotic cathode reactions (Eq. 2a) and thus decrease the coulombic efficiency (CE) (Dessì et al., 2021). The CE is the overall share of electrons being used for the target reaction, which in this study is the formation of products from CO2. Thus, any cathodic electron reacting directly or indirectly with oxygen is a lost electron in terms of production (Kocha et al., 2006). Second, as discussed above, oxygen is toxic for the most dominating MES biocatalysts and will reduce biocatalytic activity. Furthermore, the oxygen reduction reaction in addition to water can also yield hydrogen peroxide (Eq. 2b), which also leads to oxidative stress for the microorganisms (refer to above).
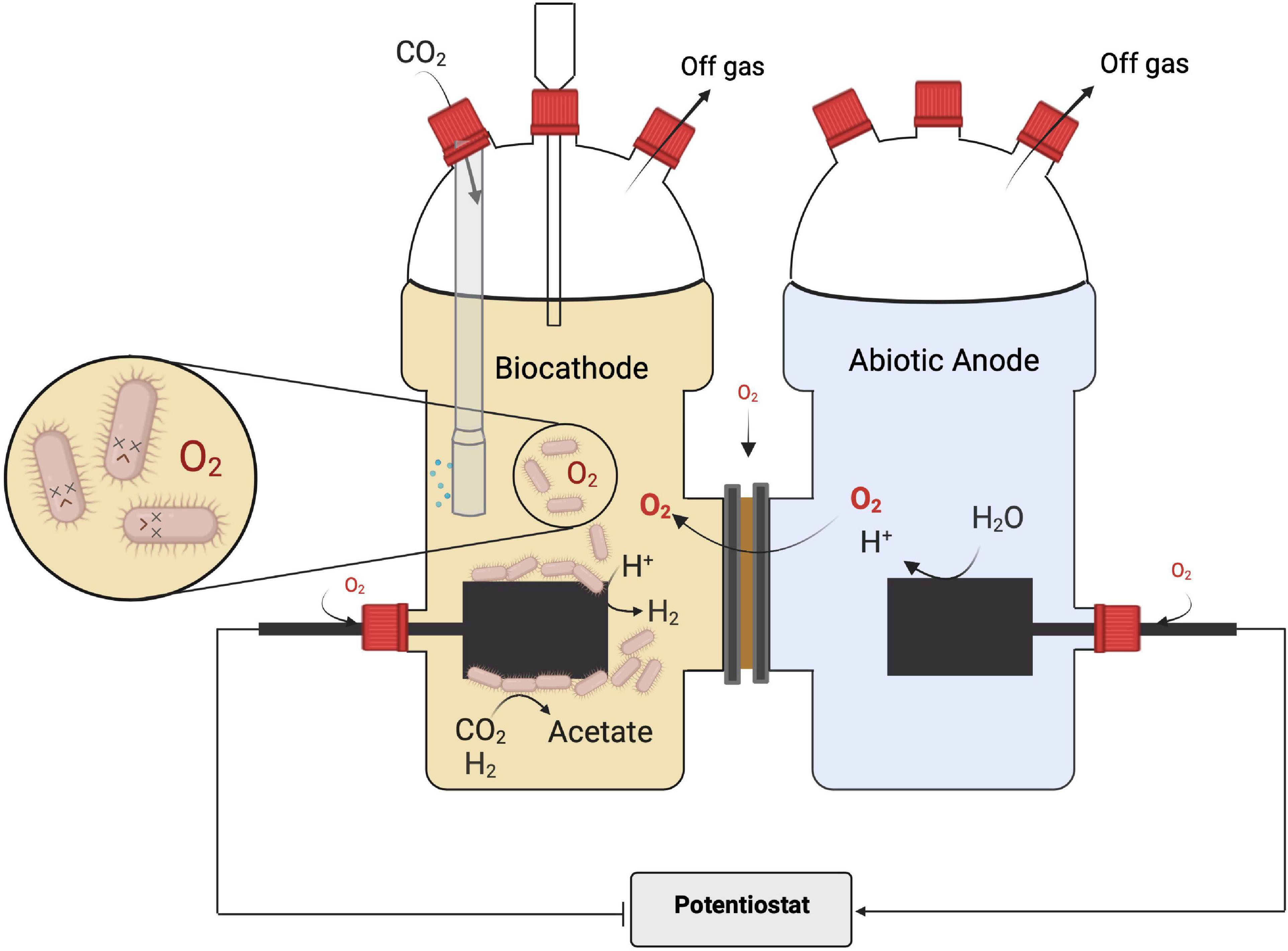
Figure 1. Microbial electrosynthesis in an H-type reactor as the archetype of a two-chambered bioelectrochemical system and often used for microbial electrosynthesis (MES) from CO2 (refer also to Table 1): the two chambers are separated by an ion-exchange membrane. As indicated, this setup provides more entry points for oxygen affecting the performance of the obligate anaerobic biocatalyst at the cathode with the main entry point being the membrane interface. Image created with BioRender.
Thus, the oxygen evolution in multiple ways is directly affecting the performance of MES in terms of yields and rates. At the same time, it is without question that ionic contact between the anode and cathode chamber is required for operating any BES (Harnisch and Schröder, 2009). This contact has to be established with the high ionic conductivity of the separator at low resistance to the entire BES to limit energetic losses. Yet, separator materials providing high ionic conductivity usually also show a low resistance for penetration of oxygen: It is a dilemma!
Ways out of the dilemma
It is a strong imperative to establish technical solutions for BES that shield the cathode compartment from O2 for successful MES. Different strategies that are briefly introduced below can help counteracting or resolving the above-described dilemma. In case the OER cannot be replaced as an anode reaction (refer to (b)), we proposed technical solutions to prevent or at least minimize O2 penetration to the cathode (refer to (c) to (g)) for lab-scale but also for scale-up applications.
a) Use of microbial collaboration to scavenge away oxygen
In this study, the reported dilemma with oxygen toxification mainly applies to MES with defined microbial catalysts, especially pure cultures. However, many research groups successfully operated MES with undefined mixed cultures (Jourdin et al., 2014; Jourdin et al., 2015; Jiang et al., 2020). Using mixed cultures or reactor microbiomes intrinsically resolves the oxygen toxicity dilemma by immediate consumption of oxygen through aerobic microbial community members. While these systems are operating fairly robust, they create different limitations and challenges to upscaling MES. With undefined mixed cultures, highly efficient MES processes will stay limited to very few stable end-products such as methane, while other target products such as acetate or ethanol require a very good balance of the community activities to avoid their subsequent (re-consumption and conversion to unwanted side-products such as methane). Additionally, pure culture MES processes in the future might allow for the production of new, higher-value target compounds through genetic engineering of the biocatalysts, increasing the scope and impact of this technology. This important route of development is not at all available for mixed cultures. Most importantly, however, the oxygen dilemma regarding efficiency losses by oxygen reactions with cathodic components is just as pronounced for mixed cultures than for pure cultures. Thus, all the following strategies might also be suitable and advisable to improve the efficiency of MES with mixed microbial catalysts.
b) Replacement of the anode reaction
The most effective strategy to avoid any harm of oxygen to the cathodic reaction certainly is its avoidance. An anode reaction that does not generate oxygen (Eq. 2a) or hydrogen peroxide (Eq 2b) is not affecting the cathodic microorganisms in general. On several anode materials, the OER (Eq.1) requires a remarkable overpotential. Therefore, oxidation of chemical compounds at lower overpotential in these materials seems very attractive, e.g., the oxidation of glycerol to formic acid (Eq. 3), or to glyceraldehyde (Eq. 4), or the oxidation of glucose to gluconic acid (Eq. 5). It was shown that this anode reaction can be more energetically and economically favorable than water oxidation (Jourdin and Burdyny, 2021).
Alternatively, chemical electron donors such as ferrocyanide (Yang et al., 2021) or anaerobic microbial oxidations could replace OER at the anode (Xiang et al., 2017). However, for the first, sustainable and complete recycling of the generated ferricyanide is currently not established and would lead to high economic and ecological costs. The latter approach comes with the intrinsic limitation that in this case also the anode potential needs to be carefully controlled to avoid toxic redox stress to the anodic microbial biofilm. As another option, Dinh et al. (2022) used the anodic oxidation of sulfide to sulfate as a counter-reaction for their cathodic electromethanogenesis.
c) Purging inert gas in the cathode chamber
Purging inert gas such as nitrogen (N2) or CO2/N2 mixtures to the cathode chamber can be an option for small-scale laboratory experiments to assure anoxic conditions like it is used for other obligate anaerobic bioprocesses (Valdez-Vazquez et al., 2005). A continuous low stream of the CO2 feed supply might not be sufficient for oxygen removal, but higher gas fluxes come with some challenges. Already at the laboratory scale, we have to care about mechanical stress to microorganisms and in the case of pure N2, about removing the carbon source CO2 with the inert gas stream by outgassing. As water evaporation in the cathode has to be avoided, humidifying the gas is required at the lab scale (Wiegmann et al., 2018). Yet, purging high-volume streams of inert gas in the cathode chamber is no solution at technical scale and beyond. Apart from the environmental footprint that comes with using gaseous N2, the operational expenditures (opex) would be significant.
d) Adapting the bioelectrochemical systems design
By changing reactor design, the share of O2 that is instantaneously removed from the anode solution can be decisively influenced. For example, a straightforward approach is the placement of the electrode. For instance, placing the anode on top of the cathode and in close vicinity to the water/gas interface will increase the mass transfer of O2 to the gas phase (Giddings et al., 2015). Thus, a lower amount of oxygen will penetrate into the anode chamber and thus less O2 is likely to penetrate the cathode. Thereby, it is important to keep a close distance between the cathode and anode. In the mentioned study, the electrode spacing was not changed. In any case, fluid dynamics and redox potential modeling approaches are recommended to obtain estimates on the impact of configurational changes on reactor performance (Rosa et al., 2019).
e) Operating bioelectrochemical systems with oxygen scavengers
Using oxygen scavengers, i.e., materials that chemically bind or physically fix O2, seems highly promising but may come at higher costs for chemicals and at an additional overpotential. For instance, metal-organic frameworks (MOFs) could become of interest (DeCoste et al., 2014). Yet, the application of MOFs in aqueous solutions is challenging (Kavoosi et al., 2018), but tailoring the material may allow creating MOFs with specific properties needed for a certain MES. Thereby, a combination of improving the BES design and using a scavenger, for instance being placed close to the membrane, seems most promising. This means that the majority of oxygen would directly leave the anode solution, e.g., as bubbles, and only the O2 molecules close to the membrane would need to be scavenged away before penetrating to the cathode. For sustainability reasons, scavenger materials have to be recyclable for use in multiple MES operations.
f) Using tailored membranes
As mentioned earlier, the membrane has to provide high ionic conductivity while minimizing O2 penetration. Therefore, tailoring membrane materials is required. Today, mainly polymer materials that are optimized for application in abiotic chemical reactors are used (Koók et al., 2019). In addition to these, ceramic membranes are promising, albeit they face the same dilemma of requiring to be repellant to oxygen at low ionic resistance. Apart from very few uses for fundamental research, due to the high resistance, the application of salt bridges for MES is not an option. Albeit having some application relevance, the use of advanced bipolar membranes currently does not seem to be a technical solution for scale-up, as well. Using bipolar membranes comes with increased opex and a loss of system efficiency, as the cell potential has to provide sufficient driving force for water splitting at the membrane (Harnisch and Schröder, 2009). Yet, using bipolar membranes can also come with a case-specific benefit of lowering the potential needed for the cathode reaction by a pH gradient across the membrane. This can partly outbalance the need for an external driving force for water splitting, which is, however, highly case-specific (Pärnamäe et al., 2021).
g) Microbial strain adaptation to oxygen
A stepwise adaptive laboratory evolution (ALE) strategy can be used to increase the tolerance of anaerobic acetogens. Shi et al. (2021) developed two Sporomusa ovata strains that are adapted to 5% oxygen by a stepwise ALE. The adapted S. ovata strains showed better performance in autotrophic conditions in the presence of 5% oxygen where the OD reached 0.17 and 0.19 ± 0.02 for the adapted strains compared with the wild-type strain, which was not able to grow at all at this oxygen concentration. In addition, the adapted strains were 1.5-fold more active for acetate production in BES, which emphasizes the importance of solving the oxygen dilemma to overcome limitations.
As illustrated in this prospective study, there are several ways out of the oxygen dilemma for strict anaerobic MES. We are convinced that there is no silver bullet to solve this, and since there is no “one-fits-all” solution and MES concepts, target scales and economic boundaries will play great roles in choosing a certain route to mitigate the problem. All the above-mentioned strategies (Use of microbial collaboration to scavenge away oxygen) to (Microbial strain adaptation to oxygen) and even further options have to be considered, integrated, and investigated for finding solutions to enhance the function of pure culture MES. Independent of what the technical approaches are specifically, they need to be environmentally sustainable, biocompatible, feasible for scale-up, and economically sound. Intuitively, the option to avoid the problem by choosing alternative anodic reactions seems to be most appealing; however, if scalability or rate limitations greatly lack behind the anodic performance of OER, all the other options might be more feasible in the end. Therefore, only a very targeted and integrated solution will help to pave the way for defined MES from CO2 from the lab bench to biotechnological plants.
Data availability statement
The original contributions presented in the study are included in the article/supplementary material, further inquiries can be directed to the corresponding author/s.
Author contributions
MR and FH developed the concept and supervised MA and SA. MA and SA performed literature research, complied the table, designed the figure and wrote sections of the manuscript. FH wrote the first full draft of the manuscript. All authors contributed to manuscript revision, read, and approved the submitted version.
Funding
This study was supported by the Helmholtz Association in the frame of the Integration Platform “Tapping nature’s potential for sustainable production and a healthy environment” at the UFZ. The authors gratefully acknowledge funding from the Deutsche Forschungsgemeinschaft (priority program SPP2240, project no. 445388719).
Conflict of interest
The authors declare that the research was conducted in the absence of any commercial or financial relationships that could be construed as a potential conflict of interest.
Publisher’s note
All claims expressed in this article are solely those of the authors and do not necessarily represent those of their affiliated organizations, or those of the publisher, the editors and the reviewers. Any product that may be evaluated in this article, or claim that may be made by its manufacturer, is not guaranteed or endorsed by the publisher.
References
Annie Modestra, J., and Venkata Mohan, S. (2019). Capacitive biocathodes driving electrotrophy towards enhanced CO2 reduction for microbial electrosynthesis of fatty acids. Bioresour. Technol. 294:122181. doi: 10.1016/j.biortech.2019.122181
Aryal, N., Halder, A., Tremblay, P.-L., Chi, Q., and Zhang, T. (2016). Enhanced microbial electrosynthesis with three-dimensional graphene functionalized cathodes fabricated via solvothermal synthesis. Electrochim. Acta 217, 117–122. doi: 10.1016/j.electacta.2016.09.063
Aryal, N., Halder, A., Zhang, M., Whelan, P. R., Tremblay, P.-L., Chi, Q., et al. (2017). Freestanding and flexible graphene papers as bioelectrochemical cathode for selective and efficient CO2 conversion. Sci. Rep. 7:9107. doi: 10.1038/s41598-017-09841-7
Aryal, N., Tremblay, P.-L., Xu, M., Daugaard, A. E., and Zhang, T. (2018). Highly conductive poly(3,4-ethylenedioxythiophene) polystyrene sulfonate polymer coated cathode for the microbial electrosynthesis of acetate from carbon dioxide. Front. Energy Res. 6:72. doi: 10.3389/fenrg.2018.00072
Bajracharya, S., ter Heijne, A., Dominguez Benetton, X., Vanbroekhoven, K., Buisman, C. J. N., Strik, D. P. B. T. B., et al. (2015). Carbon dioxide reduction by mixed and pure cultures in microbial electrosynthesis using an assembly of graphite felt and stainless steel as a cathode. Bioresour. Technol. 195, 14–24. doi: 10.1016/j.biortech.2015.05.081
Bajracharya, S., Sharma, M., Mohanakrishna, G., Dominguez Benneton, X., Strik, D. P. B. T. B., Sarma, P. M., et al. (2016a). An overview on emerging bioelectrochemical systems (BESs): Technology for sustainable electricity, waste remediation, resource recovery, chemical production and beyond. Renew. Energy 98, 153–170. doi: 10.1016/j.renene.2016.03.002
Bajracharya, S., Vanbroekhoven, K., Buisman, C. J. N., Pant, D., and Strik, D. P. B. T. B. (2016b). Application of gas diffusion biocathode in microbial electrosynthesis from carbon dioxide. Environ. Sci. Pollut. Res. 23, 22292–22308. doi: 10.1007/s11356-016-7196-x
Bajracharya, S., Yuliasni, R., Vanbroekhoven, K., Buisman, C. J. N., Strik, D. P. B. T. B., and Pant, D. (2017). Long-term operation of microbial electrosynthesis cell reducing CO2 to multi-carbon chemicals with a mixed culture avoiding methanogenesis. Bioelectrochemistry 113, 26–34. doi: 10.1016/j.bioelechem.2016.09.001
Batlle-Vilanova, P., Ganigué, R., Ramió-Pujol, S., Bañeras, L., Jiménez, G., Hidalgo, M., et al. (2017). Microbial electrosynthesis of butyrate from carbon dioxide: Production and extraction. Bioelectrochemistry 117, 57–64. doi: 10.1016/j.bioelechem.2017.06.004
Batlle-Vilanova, P., Puig, S., Gonzalez-Olmos, R., Balaguer, M. D., and Colprim, J. (2016). Continuous acetate production through microbial electrosynthesis from CO 2 with microbial mixed culture. J. Chem. Technol. Biotechnol. 91, 921–927. doi: 10.1002/jctb.4657
Bian, B., Alqahtani, M. F., Katuri, K. P., Liu, D., Bajracharya, S., Lai, Z., et al. (2018). Porous nickel hollow fiber cathodes coated with CNTs for efficient microbial electrosynthesis of acetate from CO 2 using Sporomusa ovata. J. Mater. Chem. A 6, 17201–17211. doi: 10.1039/C8TA05322G
Buckel, W., and Thauer, R. K. (2018). Flavin-based electron bifurcation, ferredoxin, flavodoxin, and anaerobic respiration with protons (Ech) or NAD+ (Rnf) as electron acceptors: a historical review. Front. Microbiol. 9:401. doi: 10.3389/fmicb.2018.00401
Chen, L., Tremblay, P.-L., Mohanty, S., Xu, K., and Zhang, T. (2016). Electrosynthesis of acetate from CO 2 by a highly structured biofilm assembled with reduced graphene oxide–tetraethylene pentamine. J. Mater. Chem. 4, 8395–8401. doi: 10.1039/C6TA02036D
Cui, M., Nie, H., Zhang, T., Lovley, D., and Russell, T. P. (2017). Three-dimensional hierarchical metal oxide–carbon electrode materials for highly efficient microbial electrosynthesis. Sustain. Energy Fuels 1, 1171–1176. doi: 10.1039/C7SE00073A
Dai, S., Korth, B., Schwab, L., Aulenta, F., Vogt, C., and Harnisch, F. (2022). Deciphering the fate of sulfate in one- and two-chamber bioelectrochemical systems. Electrochim. Acta 408:139942. doi: 10.1016/j.electacta.2022.139942
DeCoste, J. B., Weston, M. H., Fuller, P. E., Tovar, T. M., Peterson, G. W., LeVan, M. D., et al. (2014). Metal–Organic Frameworks for Oxygen Storage. Angew. Chemie Int. Ed. 53, 14092–14095. doi: 10.1002/anie.201408464
Dessì, P., Rovira-Alsina, L., Sánchez, C., Dinesh, G. K., Tong, W., Chatterjee, P., et al. (2021). Microbial electrosynthesis: towards sustainable biorefineries for production of green chemicals from CO2 emissions. Biotechnol. Adv. 46:107675. doi: 10.1016/j.biotechadv.2020.107675
Dinh, H. T. T., Kambara, H., Matsushita, S., Aoi, Y., Kindaichi, T., Ozaki, N., et al. (2022). Biological methane production coupled with sulfur oxidation in a microbial electrosynthesis system without organic substrates. J. Environ. Sci. 116, 68–78. doi: 10.1016/j.jes.2021.07.027
Enzmann, F., Mayer, F., Stöckl, M., Mangold, K.-M., Hommel, R., and Holtmann, D. (2019). Transferring bioelectrochemical processes from H-cells to a scalable bubble column reactor. Chem. Eng. Sci. 193, 133–143. doi: 10.1016/j.ces.2018.08.056
Gawel, A., Jaster, T., Siegmund, D., Holzmann, J., Lohmann, H., Klemm, E., et al. (2022). Electrochemical CO2 reduction - The macroscopic world of electrode design, reactor concepts & economic aspects. iScience 25:104011. doi: 10.1016/j.isci.2022.104011
Giddings, C. G. S., Nevin, K. P., Woodward, T., Lovley, D. R., and Butler, C. S. (2015). Simplifying microbial electrosynthesis reactor design. Front. Microbiol. 6:468. doi: 10.3389/fmicb.2015.00468
Gildemyn, S., Verbeeck, K., Slabbinck, R., Andersen, S. J., Prévoteau, A., and Rabaey, K. (2015). Integrated production, extraction, and concentration of acetic acid from CO 2 through microbial electrosynthesis. Environ. Sci. Technol. Lett. 2, 325–328. doi: 10.1021/acs.estlett.5b00212
Harnisch, F., and Schröder, U. (2009). Selectivity versus mobility: separation of anode and cathode in microbial bioelectrochemical systems. ChemSusChem 2, 921–926. doi: 10.1002/cssc.200900111
Igarashi, K., and Kato, S. (2017). Extracellular electron transfer in acetogenic bacteria and its application for conversion of carbon dioxide into organic compounds. Appl. Microbiol. Biotechnol. 101, 6301–6307. doi: 10.1007/s00253-017-8421-3
Imlay, J. A. (2002). How oxygen damages microbes: oxygen tolerance and obligate anaerobiosis. Adv. Microb. Physiol. 2002, 111–153. doi: 10.1016/S0065-2911(02)46003-1
Jabeen, G., and Farooq, R. (2016). Bio-electrochemical synthesis of commodity chemicals by autotrophic acetogens utilizing CO2 for environmental remediation. J. Biosci. 41, 367–380. doi: 10.1007/s12038-016-9625-x
Jiang, Y., Chu, N., Qian, D.-K., and Jianxiong Zeng, R. (2020). Microbial electrochemical stimulation of caproate production from ethanol and carbon dioxide. Bioresour. Technol. 295:122266. doi: 10.1016/j.biortech.2019.122266
Jourdin, L., and Burdyny, T. (2021). Microbial electrosynthesis: where do we go from here? Trends Biotechnol. 39, 359–369. doi: 10.1016/j.tibtech.2020.10.014
Jourdin, L., Freguia, S., Donose, B. C., Chen, J., Wallace, G. G., Keller, J., et al. (2014). A novel carbon nanotube modified scaffold as an efficient biocathode material for improved microbial electrosynthesis. J. Mater. Chem. A 2, 13093–13102. doi: 10.1039/C4TA03101F
Jourdin, L., Freguia, S., Flexer, V., and Keller, J. (2016). Bringing high-rate, co 2 -based microbial electrosynthesis closer to practical implementation through improved electrode design and operating conditions. Environ. Sci. Technol. 50, 1982–1989. doi: 10.1021/acs.est.5b04431
Jourdin, L., Grieger, T., Monetti, J., Flexer, V., Freguia, S., Lu, Y., et al. (2015). High acetic acid production rate obtained by microbial electrosynthesis from carbon dioxide. Environ. Sci. Technol. 49, 13566–13574. doi: 10.1021/acs.est.5b03821
Jourdin, L., Raes, S. M. T., Buisman, C. J. N., and Strik, D. P. B. T. B. (2018). Critical biofilm growth throughout unmodified carbon felts allows continuous bioelectrochemical chain elongation from co2 up to caproate at high current density. Front. Energy Res. 6:7. doi: 10.3389/fenrg.2018.00007
Jourdin, L., Winkelhorst, M., Rawls, B., Buisman, C. J. N., and Strik, D. P. B. T. B. (2019). Enhanced selectivity to butyrate and caproate above acetate in continuous bioelectrochemical chain elongation from CO2: Steering with CO2 loading rate and hydraulic retention time. Bioresour. Technol. Reports 7:100284. doi: 10.1016/j.biteb.2019.100284
Kavoosi, N., Savchenko, T., Senkovska, I., Maliuta, M., Bon, V., Eychmüller, A., et al. (2018). Selective pore opening and gating of the pillared layer metal-organic framework DUT-8(Ni) upon liquid phase multi-component adsorption. Microporous Mesoporous Mater. 271, 169–174. doi: 10.1016/j.micromeso.2018.05.024
Kocha, S. S., Deliang Yang, J., and Yi, J. S. (2006). Characterization of gas crossover and its implications in PEM fuel cells. AIChE J. 52, 1916–1925. doi: 10.1002/aic.10780
Kondaveeti, S., Abu-Reesh, I. M., Mohanakrishna, G., Bulut, M., and Pant, D. (2020). Advanced routes of biological and bio-electrocatalytic carbon dioxide (CO2) mitigation toward carbon neutrality. Front. Energy Res. 8, 1–24. doi: 10.3389/fenrg.2020.00094
Koók, L., Bakonyi, P., Harnisch, F., Kretzschmar, J., Chae, K.-J., Zhen, G., et al. (2019). Biofouling of membranes in microbial electrochemical technologies: causes, characterization methods and mitigation strategies. Bioresour. Technol. 279, 327–338. doi: 10.1016/j.biortech.2019.02.001
Kracke, F., Wong, A. B., Maegaard, K., Deutzmann, J. S., Hubert, M. A., Hahn, C., et al. (2019). Robust and biocompatible catalysts for efficient hydrogen-driven microbial electrosynthesis. Commun. Chem. 2:45. doi: 10.1038/s42004-019-0145-0
Krieg, T., Madjarov, J., Rosa, L. F. M., Enzmann, F., Harnisch, F., Holtmann, D., et al. (2018). Reactors for Microbial Electrobiotechnology. Adv. Biochem. Eng. Biotechnol. 167, 231–271. doi: 10.1007/10_2017_40
LaBelle, E. V., and May, H. D. (2017). Energy efficiency and productivity enhancement of microbial electrosynthesis of acetate. Front. Microbiol. 8:756. doi: 10.3389/fmicb.2017.00756
Marshall, C. W., Ross, D. E., Fichot, E. B., Norman, R. S., and May, H. D. (2013). Long-term operation of microbial electrosynthesis systems improves acetate production by autotrophic microbiomes. Environ. Sci. Technol. 47, 6023–6029. doi: 10.1021/es400341b
Nevin, K. P., Hensley, S. A., Franks, A. E., Summers, Z. M., Ou, J., Woodard, T. L., et al. (2011). Electrosynthesis of organic compounds from carbon dioxide is catalyzed by a diversity of acetogenic microorganisms. Appl. Environ. Microbiol. 77, 2882–2886. doi: 10.1128/AEM.02642-10
Nevin, K. P., Woodard, T. L., Franks, A. E., Summers, Z. M., and Lovley, D. R. (2010). Microbial electrosynthesis: feeding microbes electricity to convert carbon dioxide and water to multicarbon extracellular organic compounds. MBio 1:10. doi: 10.1128/mBio.00103-10
Nie, H., Zhang, T., Cui, M., Lu, H., Lovley, D. R., and Russell, T. P. (2013). Improved cathode for high efficient microbial-catalyzed reduction in microbial electrosynthesis cells. Phys. Chem. Chem. Phys. 15:14290. doi: 10.1039/c3cp52697f
Pärnamäe, R., Mareev, S., Nikonenko, V., Melnikov, S., Sheldeshov, N., Zabolotskii, V., et al. (2021). Bipolar membranes: a review on principles, latest developments, and applications. J. Memb. Sci. 617:118538. doi: 10.1016/j.memsci.2020.118538
Patil, S. A., Arends, J. B. A., Vanwonterghem, I., van Meerbergen, J., Guo, K., Tyson, G. W., et al. (2015). Selective enrichment establishes a stable performing community for microbial electrosynthesis of acetate from CO 2. Environ. Sci. Technol. 49, 8833–8843. doi: 10.1021/es506149d
Rahman, A., Borhan, M. S., and Rahman, S. (2018). Evaluation of microbial fuel cell (MFC) for bioelectricity generation and pollutants removal from sugar beet processing wastewater (SBPW). Water Sci. Technol. 77, 387–397. doi: 10.2166/wst.2017.549
Rosa, L. F. M., Hunger, S., Zschernitz, T., Strehlitz, B., and Harnisch, F. (2019). Integrating electrochemistry into bioreactors: effect of the upgrade kit on mass transfer, mixing time and sterilizability. Front. Energy Res. 7:1–11. doi: 10.3389/fenrg.2019.00098
Roy, M., Yadav, R., Chiranjeevi, P., and Patil, S. A. (2021). Direct utilization of industrial carbon dioxide with low impurities for acetate production via microbial electrosynthesis. Bioresour. Technol. 320:124289. doi: 10.1016/j.biortech.2020.124289
Schröder, U., Harnisch, F., and Angenent, L. T. (2015). Microbial electrochemistry and technology: terminology and classification. Energy Environ. Sci. 8, 513–519. doi: 10.1039/c4ee03359k
Shi, X.-C., Tremblay, P.-L., Wan, L., and Zhang, T. (2021). Improved robustness of microbial electrosynthesis by adaptation of a strict anaerobic microbial catalyst to molecular oxygen. Sci. Total Environ. 754:142440. doi: 10.1016/j.scitotenv.2020.142440
Valdez-Vazquez, I., Sparling, R., Risbey, D., Rinderknecht-Seijas, N., and Poggi-Varaldo, H. M. (2005). Hydrogen generation via anaerobic fermentation of paper mill wastes. Bioresour. Technol. 96, 1907–1913. doi: 10.1016/j.biortech.2005.01.036
Vassilev, I., Hernandez, P. A., Batlle-Vilanova, P., Freguia, S., Krömer, J. O., Keller, J., et al. (2018). Microbial electrosynthesis of isobutyric, butyric, caproic acids, and corresponding alcohols from carbon dioxide. ACS Sustain. Chem. Eng. 6, 8485–8493. doi: 10.1021/acssuschemeng.8b00739
Wang, G., Huang, Q., Song, T., and Xie, J. (2020). Enhancing microbial electrosynthesis of acetate and butyrate from co 2 reduction involving engineered clostridium ljungdahlii with a nickel-phosphide-modified electrode. Energy Fuels 34, 8666–8675. doi: 10.1021/acs.energyfuels.0c01710
Wiegmann, V., Martinez, C. B., and Baganz, F. (2018). A simple method to determine evaporation and compensate for liquid losses in small-scale cell culture systems. Biotechnol. Lett. 40, 1029–1036. doi: 10.1007/s10529-018-2556-x
Xiang, Y., Liu, G., Zhang, R., Lu, Y., and Luo, H. (2017). High-efficient acetate production from carbon dioxide using a bioanode microbial electrosynthesis system with bipolar membrane. Bioresour. Technol. 233, 227–235. doi: 10.1016/j.biortech.2017.02.104
Yang, H.-Y., Hou, N.-N., Wang, Y.-X., Liu, J., He, C.-S., Wang, Y.-R., et al. (2021). Mixed-culture biocathodes for acetate productiaon from CO2 reduction in the microbial electrosynthesis: impact of temperature. Sci. Total Environ. 790:148128. doi: 10.1016/j.scitotenv.2021.148128
Keywords: carbon dioxide valorization, microbial electrosynthesis, microbial electron uptake, extracellular electron transfer, oxygen stress
Citation: Abdollahi M, Al Sbei S, Rosenbaum MA and Harnisch F (2022) The oxygen dilemma: The challenge of the anode reaction for microbial electrosynthesis from CO2. Front. Microbiol. 13:947550. doi: 10.3389/fmicb.2022.947550
Received: 18 May 2022; Accepted: 06 July 2022;
Published: 03 August 2022.
Edited by:
Catarina M. Paquete, Universidade Nova de Lisboa, PortugalReviewed by:
Albert Guisasola, Universitat Autònoma de Barcelona, SpainLudovic Jourdin, Delft University of Technology, Netherlands
Copyright © 2022 Abdollahi, Al Sbei, Rosenbaum and Harnisch. This is an open-access article distributed under the terms of the Creative Commons Attribution License (CC BY). The use, distribution or reproduction in other forums is permitted, provided the original author(s) and the copyright owner(s) are credited and that the original publication in this journal is cited, in accordance with accepted academic practice. No use, distribution or reproduction is permitted which does not comply with these terms.
*Correspondence: Falk Harnisch, ZmFsay5oYXJuaXNjaEB1ZnouZGU=
†These authors have contributed equally to this work