- 1Veterinary Education, Research, and Outreach (VERO), Department of Veterinary Pathobiology, College of Veterinary Medicine and Biomedical Sciences, Texas A&M University, College Station, TX, United States
- 2Department of Agricultural Sciences, Paul Engler College of Agriculture and Natural Sciences, West Texas A&M University, Canyon, TX, United States
- 3Veterinary Education, Research, and Outreach (VERO), Department of Large Animal Clinical Sciences, College of Veterinary Medicine and Biomedical Sciences, Texas A&M University, Canyon, TX, United States
Bovine Respiratory Disease (BRD) represents a significant burden to the health of feedlot cattle and the profitability of the beef industry in the US. Mannheimia haemolytica is widely regarded as the primary bacterial pathogen driving acute BRD. While Mycoplasma bovis is most commonly implicated in chronic cases of BRD, this agent's potential role in acute stages of BRD is unclear. Therefore, this study aimed to evaluate potential associations between M. bovis and M. haemolytica during acute BRD in feedlot cattle. Nasal swabs (n = 1,044) were collected over time from feedlot cattle (n = 270) enrolled in an experiment assessing the effect of vaccination for Bovine Respiratory Syncytial Virus (BRSV). Swabs were analyzed for detection of M. bovis, M. haemolytica, Pasteurella multocida, Histophilus somni, and BRSV via multiplex qPCR assays. Data were analyzed using inverse conditional probability weighted (ICPW) logistic regression models to investigate potential effects of M. bovis presence on arrival (d0), day seven (d7) and day 14 (d14) post-arrival on M. haemolytica prevalence on day 28 (d28) post-arrival, adjusting for the previous history of P. multocida, H. somni, BRSV, BRD morbidity, and body weight. The potential association between time-to-BRD detection and M. bovis presence on d0, d7, and d14 post-arrival, was inferred via an ICPW time-to-event model. The presence of M. bovis in nasal swabs collected on d7 post-arrival was significantly associated with an increase in the prevalence of M. haemolytica on d28 (prevalence difference: 45%; 95% Confidence Interval: 31%, 60%; P-value < 0.001). Significant time-varying coefficients for M. bovis presence were detected at d0, d7, and d14 post-arrival in the ICPW time-to-event model (P-value < 0.001). The shortest median time-to-BRD detection was 29 days in cattle that were M. bovis positive on d0, d7, and d14 post-arrival and in those that were positive on d0 and d14 post-arrival. Under the conditions of this study, our findings suggest that M. bovis may be influencing the respiratory environment during the acute phase of BRD, increasing the abundance of M. haemolytica, which could have important impacts on the occurrence of BRD.
Introduction
Bovine respiratory disease (BRD) represents an enormous animal welfare issue and an economic burden to beef cattle production in the US (Peel, 2020). It is estimated that BRD morbidity is 16.2% in the feedlots (USDA, 2013). BRD mortality accounts for 55 and 36.3% of non-predator mortality in cattle and calves, respectively, in feedlots and backgrounding operations in the US (USDA, 2017). Additionally, BRD is the disease most frequently associated with antimicrobial drug (AMD) treatment in beef cattle (Brault et al., 2019). Common clinical signs of BRD are nasal discharge, depression, dyspnea, fever, appetite loss, and even acute mortality (Griffin et al., 2010).
Several viruses (BHV-1, BVDV, PI3V, BRSV) and bacteria (Mannheimia haemolytica, Histophilus somni, Pasteurella multocida, and Mycoplasma bovis) have been associated with BRD (Angen et al., 2009; Gershwin et al., 2015; Cirone et al., 2019). Interestingly, these bacterial agents are commonly present in the upper respiratory tract of healthy animals and could be considered to be commensals until other factors triggers the BRD pathogenesis (Confer, 2009; Bakaletz, 2017; Lion et al., 2021). Among the BRD bacterial agents, M. haemolytica is the most relevant in acute BRD, causing fibrinonecrotizing lesions in the lungs (Rice et al., 2007; Booker et al., 2008; Mason et al., 2022).
Mycoplasma bovis is a bacterium member of the family Mycoplasmataceae, characterized by a small genome, lack of cell wall, and high nutritional requirements for in vitro growth (Li et al., 2011; Parker et al., 2018; Dudek et al., 2020). Traditionally, M. bovis has most typically been linked to chronic BRD with characteristic pneumonic lesions that are often non-responsive to AMD therapy (Booker et al., 2008; Hermeyer et al., 2012a; Gershwin et al., 2015; Becker et al., 2020). Several virulence factors have been implicated in the ability of M. bovis to persist in the lungs of cattle with BRD, such as variable surface proteins (Vsp), adhesins, nucleases, H2O2 production, and biofilm formation (Burki et al., 2015; Perez-Casal, 2020). Due to the difficulties of growing M. bovis in the lab, the associated culture bias (Prakash et al., 2021) may have falsely shaped our prior understanding of the role of M. bovis in BRD pathogenesis.
Despite accepted dogma regarding M. bovis as a pathogen associated with chronic pneumonia in cattle, recent evidence has emerged linking M. bovis presence in the upper respiratory tract with acute BRD status (Timsit et al., 2018; Centeno-Martinez et al., 2022; Crosby et al., 2022). However, the influence of M. bovis on other members of the microbial community of the respiratory tract, especially other BRD agents such as M. haemolytica, remains unknown shortly after arrival and mixing with other cattle at feedlots. Therefore, this study evaluated the associations of M. bovis and M. haemolytica during the early feeding period and acute BRD occurrence in feedlot cattle. A more comprehensive understanding of the dependencies among BRD pathogens over time will help develop new non-antibiotic control strategies and subsequently reduce the burden of BRD on beef cattle production.
Materials and methods
Experimental design
Crossbred beef bull and steer calves that were considered to have a high risk for BRD based upon demographics and prior experience were purchased at an auction market and then enrolled in a randomized controlled vaccine efficacy study; these animals were also used to address this study's objectives. For that vaccine trial (Powledge et al., 2022), cattle were randomly assigned to three treatment groups: an unvaccinated negative control group, a group vaccinated intranasally with a trivalent modified-live virus (MLV) respiratory vaccine (BHV-1, BRSV, and PI3V) with parenteral BVDV type I and type II vaccine, and a group vaccinated with a parenteral pentavalent MLV respiratory vaccine (BHV-1, BRSV, PI3V, BVDV type I and type II). Treatment was applied the following morning after arrival (6:00 am CST), but that day will be equated to arrival for simplicity. The random allocation of treatment was stratified by individual body weight (BW), sex (bull or steer), and presence/absence of a pre-existing ranch tag. Additionally, stratification by the truckload (total of five truckloads) was performed. Treatment pen assignment (15 pens per treatment group) was spatially arranged to minimize unwanted MLV transmission between the vaccine treatments and the negative control group. Tildipirosin (Zuprevo, Merck Animal Health, NJ, USA) was administered as BRD metaphylaxis to all cattle as per label.
A total of 12 cattle were randomly assigned to each pen, six of which were also randomly selected for sampling (n = 270 cattle selected for sampling). Nasal swabs were collected on days 0, 7, 14, and 28 post-arrival. Nasal swabs (n = 1,044 swabs) were collected by personnel blinded to treatment allocation and stored at −20°C until submitted for analysis at the Texas Veterinary Medicine Diagnostic Laboratory (TVMDL) in Canyon, TX. Nasal swabs were used for molecular detection of BRSV, H. somni, M. haemolytica, P. multocida, and M. bovis via a multiplex qPCR with TaqMan chemistry (Applied Biosystems, MA, USA).
Using a clinical illness score (CIS) (Pillen et al., 2016), personnel blinded to treatment allocation evaluated cattle daily for BRD symptoms. Cattle with a CIS of 2 or 3 were administered florfenicol (40 mg/kg BW, Nuflor, Merck Animal Health). Enrofloxacin (11 mg/kg BW, Baytril, Bayer Animal Health, KS, USA) was administered as a second antimicrobial treatment to those animals with a CIS of 2 or 3 after 3 days of the treatment with florfenicol. Ceftiofur (6.6 mg/kg BW, Excede, Zoetis, NJ, USA) was administered in case a third antimicrobial treatment was needed (CIS of 2 or 3 after 3 days of the treatment with enrofloxacin). Cattle with a CIS of 4 were humanely euthanized. Cattle deemed chronically ill (three antimicrobial treatments combined with <1.0 lb in average daily gain since arrival or body condition scoring <3) were removed from the study.
Statistical analysis
The molecular detection of BRSV, H. somni, M. haemolytica, P. multocida, and M. bovis generated continuous variables (Cycle threshold or Ct value) and binary variables (presence/absence) based on a cutoff value (Ct value of 36) validated by the TVMDL. Any instance of no detection, i.e., below the limit of detection of the qPCR assay, was assigned the highest Ct value possible (Ct value of 40).
Mixed-effects logistic regression was used to evaluate the longitudinal associations of anti-BRSV vaccination with the prevalence of each pathogen, calculating the prevalence difference (PD) as a measure of association. Therefore, each pathogen's presence/absence variable was regressed on treatment group, time (sampling day), and their interaction, as fixed effects. Block, pen, and animal were used as nested random intercepts. Similarly, mixed-effects linear regression was used to assess the longitudinal associations of BRSV vaccination with the amount of DNA detected for each pathogen, using the Ct values as a proxy. These mixed-effects linear models used the same variables described for the mixed-effects logistic regression, using restricted maximum likelihood parameter estimation with Kenward-Roger method to calculate degrees of freedom. Additional mixed-effects linear regression models, in which M. haemolytica Ct values were regressed on either M. bovis, H. somni, P. multocida, or BRSV Ct values, adjusting for treatment and time, were also evaluated, using the model parameters previously described. Likelihood ratio tests were used to select the random effects retained in the final models (Self and Liang, 1987).
The potential effects of the presence of M. bovis on M. haemolytica prevalence and Ct values on day 28 post-arrival, mortality, and BRD morbidity (on days 14 and 28 post-arrival) were evaluated using inverse conditional probability weighted (ICPW) regression (He, 2018) to account for time-varying confounding at the pen level (Ji et al., 2020). Morbidity was abstracted from the records of antibiotic treatment due to BRD. Inverse conditional probability weighted logistic regression was used for M. haemolytica prevalence, mortality, and morbidity. Inverse conditional probability weighted linear regression was used for M. haemolytica Ct values. Inverse conditional probability weights consisted of stabilized weights that were estimated via conditional logistic regression (see Table 1 for a list of variables included in the estimation of propensity scores). Each outcome was regressed on M. bovis presence at previous time points, treatment group, and their interactions.
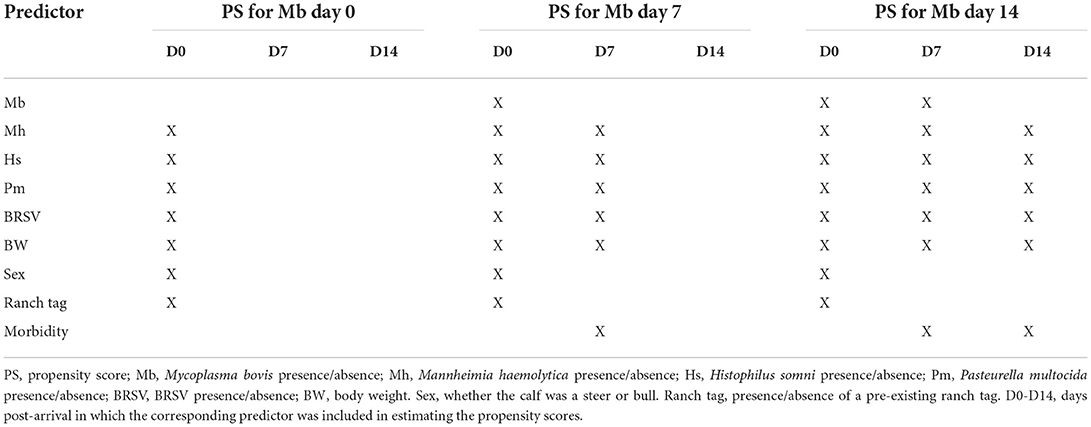
Table 1. Predictors used to estimate the propensity scores for Mycoplasma bovis prevalence at days 0, 7, and 14 post-arrival.
The association of M. bovis presence on days 0, 7, and 14 post-arrival with time-to-first BRD antibiotic treatment was estimated using an ICPW Cox proportional hazards model using weights accounting for time-varying confounding and censoring. The administration of the first antibiotic treatment after arrival due to BRD was considered the event, and cattle that died or reached the end of the follow-up (70 days) without experiencing the event, were deemed to be right-censored. Since violations of the proportional hazard assumption were detected for M. bovis presence on days 0, 7, and 14 post-arrival using Schoenfeld residuals (Dohoo et al., 2012), those variables were included in an ICPW Cox model with time-varying coefficients. All statistical analyses were performed in Stata 17 (StataCorp, 2021), and the significance level was set to 0.05 a priori.
Results
In this study, the vaccination groups did not differ in BW at arrival nor in the proportion of bulls and steers (Table 2). Twenty-six out of 270 cattle died before the end of the experiment (overall mortality: 9.6%). Therefore, 1,044 nasal swabs were used in the qPCR assays. The values of health parameters related to BRD at the end of the experiment are shown in Table 3. There was no significant difference in the proportion of cattle receiving antibiotics for BRD symptoms, or for retreatment, among the vaccination groups (Table 3). Likewise, the proportion of animals with chronic BRD and mortality did not significantly differ among the vaccination groups (Table 3). Of notice, we detected no significant differences between the cattle randomly selected for sampling and those not selected in the overall proportion of cattle receiving antibiotics for BRD (P-value: 0.846) or overall mortality (P-values: 0.32).

Table 2. Characteristics of the cattle after random allocation to the vaccination groups at arrival.
The prevalence of all evaluated BRD pathogens changed significantly over time (overall test P-value < 0.0001). Highly heterogeneous longitudinal trends in the prevalence of BRD pathogens were observed throughout the study (Figure 1). For instance, in the control group, M. bovis prevalence increased from arrival until day 14 post-arrival and slightly decreased on day 28 post-arrival. By contrast, M. haemolytica showed a sharp decrease on day seven post-arrival with subsequent increments on the following 2 weeks.
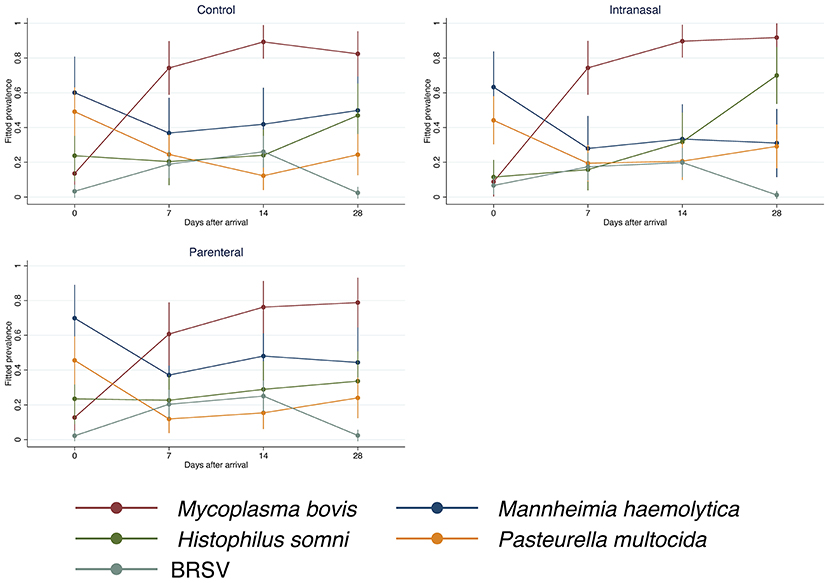
Figure 1. Prevalence of bovine respiratory disease pathogens by treatment. Control: unvaccinated negative control cattle. Intranasal: cattle vaccinated intranasally with a trivalent modified-live virus (MLV) respiratory vaccine (BHV-1, BRSV, and PI3V) with parenteral BVDV type I and type II vaccine. Parenteral: cattle vaccinated with a parenteral pentavalent MLV respiratory vaccine (BHV-1, BRSV, PI3V, BVDV type I and type II). The estimation of the prevalence values assumes that the animals share the same random effects.
On average, vaccination against BRSV significantly influenced the longitudinal trends of M. bovis and H. somni prevalence in the upper respiratory tract (overall joint test P-value = 0.0143 and 0.0001, respectively). A lower M. bovis prevalence was detected in the parenteral vaccination group when compared with the other two vaccination groups on day 7 (PD: −0.14, 95% Confidence Interval or 95% CI: −0.27 to −0.004, P-value: 0.043) and 14 post-arrival (parenteral vs. control PD: −0.13, 95% CI: −0.25 to −0.01, P-value: 0.029; parenteral vs. intranasal PD: −0.13, 95% CI: −0.25 to −0.02, P-value: 0.024). Mycoplasma bovis prevalence in the parenteral vaccination group continued to be lower than that in the intranasal vaccination group on day 28 post-arrival (PD: −0.13, 95% CI: −0.24 to −0.01, P-value: 0.027). The effect of the vaccination against BRSV on H. somni prevalence was only observed on day 28 post-arrival, in which H. somni prevalence in the upper respiratory tract of the intranasal vaccination group was significantly higher than the control (PD: 0.23, 95% CI: 0.07 to 0.39, P-value: 0.005) and parenteral vaccination group (PD: 0.36, 95% CI: 0.21 to 0.52, P-value < 0.001).
As measured by qPCR Ct values, the dynamics of M. bovis and H. somni also differed according to vaccination against BRSV (P-value = 0.0062 and < 0.0001, respectively). Significant differences were detected in M. bovis dynamics on days 7, 14, and 28 post-arrival, in which M. bovis qPCR Ct values in nasal swabs from cattle of the intranasal BRSV vaccine group were consistently lower (1.54 to 2.75 cycles, P-value < 0.05) than in cattle of the other two vaccination groups, except M. bovis qPCR Ct values of the control group on day seven and the parenteral vaccination group on day 28 post-arrival, which did not differ significantly from the corresponding M. bovis qPCR Ct values of the intranasal vaccination group. At day 28 post-arrival, H. somni qPCR Ct values were significantly lower in the intranasal vaccination group than in the other two vaccination groups (intranasal vs. control: −2.9 cycles, 95% CI: −4.23 to −1.62 cycles, P-value < 0.001; intranasal vs. parenteral: −3.52 cycles, 95% CI: −4.82 to −2.22 cycles, P-value < 0.001).
Mycoplasma bovis and M. haemolytica dynamics were positively associated in the upper respiratory tract of feedlot cattle. An increase in the qPCR Ct value of one cycle for M. bovis was associated, on average, with an increase in 0.08 cycles in the qPCR Ct value of M. haemolytica (95% CI: 0.02, 0.13) after adjusting for vaccination group and days post-arrival. Mycoplasma bovis was the only pathogen with a statistically significant association with M. haemolytica dynamics (Table 4).
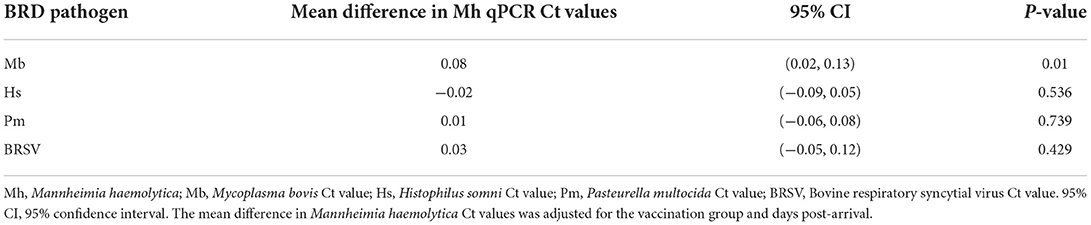
Table 4. Association of Mannheimia haemolytica qPCR Ct values with those from Mycoplasma bovis, Histophilus somni, Pasteurella multocida, and BRSV.
The presence of M. bovis in the upper respiratory tract of cattle on day seven post-arrival to the feedlot was associated with a significant increase in M. haemolytica prevalence on day 28 post-arrival, a significant decrease in M. haemolytica qPCR Ct values on day 28 post-arrival, and a significant increase in mortality, after adjusting for time-varying confounding (Table 5). Conversely, BRD morbidity on day 28 post-arrival was eight percentage points lower in cattle with M. bovis than cattle without M. bovis on arrival (Table 5). Additionally, M. haemolytica qPCR Ct values on day 28 post-arrival were on average higher in cattle with M. bovis than cattle without M. bovis on arrival (Table 5).
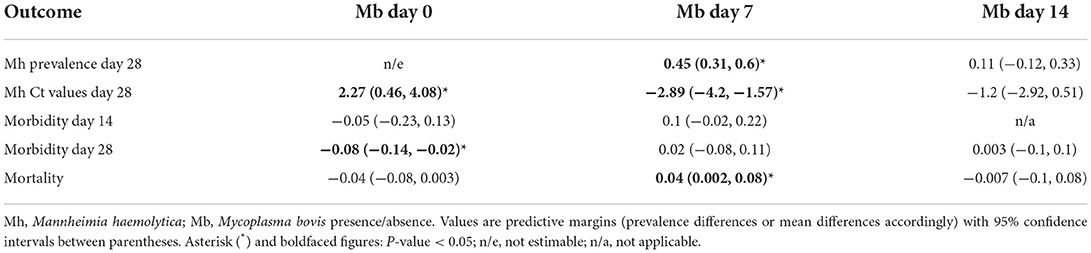
Table 5. Association of Mannheimia haemolytica prevalence and Ct values on day 28, mortality, and morbidity (on day 14 and 28) with the presence of Mycoplasma bovis on previous time points.
During the study, 149 cattle received antibiotic treatment against BRD (the event in the survival analysis). Five cattle were right-censored because they died before receiving any antibiotic treatment against BRD. Additionally, 116 cattle had not experienced the event by the end of the experiment, and therefore, they were also right-censored. The data from 26 cattle could not be used in the survival analysis because the inverse conditional probability weights could not be computed.
A highly heterogeneous association was observed between M. bovis presence and the time-to-first BRD antibiotic treatment. Significant time-varying coefficients for M. bovis presence were detected in all evaluated time points in the ICPW Cox model (P < 0.001). The shortest median time-to-first BRD antibiotic treatment was 29 days in cattle M. bovis positive on days 0, 7, 14 post-arrival and those positive on days 0 and 14. A median time-to-first BRD antibiotic treatment of 51 days was estimated for cattle M. bovis positive only on arrival. In the rest of the categories, the median time-to-first BRD antibiotic treatment was greater than 70 days (Figure 2).
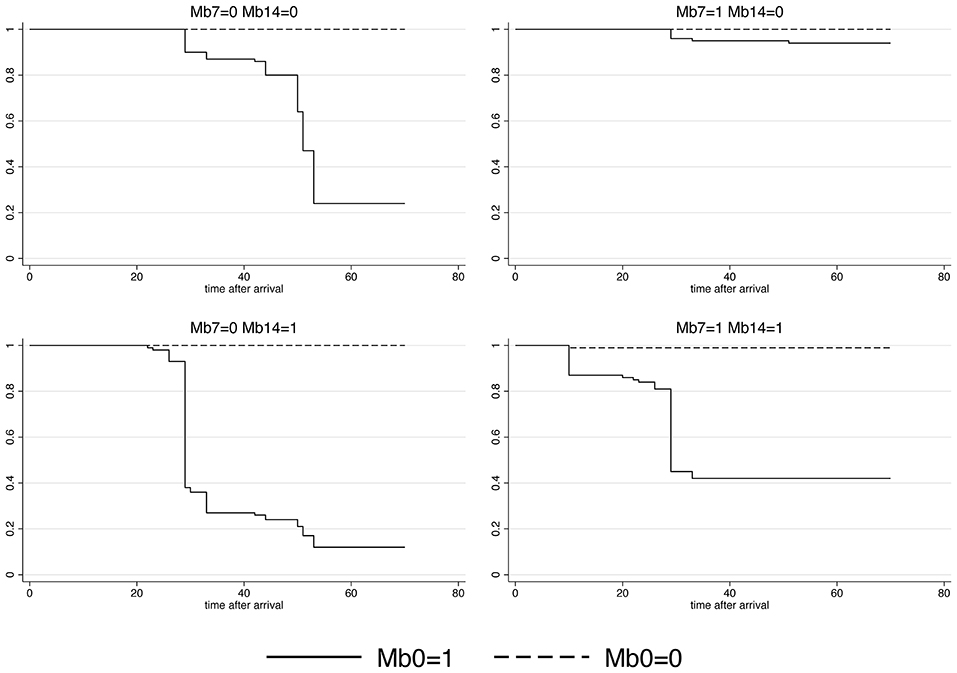
Figure 2. Survival curves for Mycoplasma bovis presence in the upper respiratory tract of cattle on days 0, 7, and 14 post-arrival to the feedlot. The event was the first antibiotic treatment against bovine respiratory disease. Mb0, M. bovis presence on arrival; Mb7, M. bovis presence on day seven post-arrival; Mb14, M. bovis presence on day 14 after arrival. The values 1 and 0 represent the presence and absence of M. bovis, respectively.
Discussion
This research showed that M. bovis and M. haemolytica are associated during the acute stages of BRD in feedlot cattle. This finding broadens our understanding of BRD pathogenesis and opens new avenues for the development of control strategies that do not rely in AMD. We observed that M. bovis prevalence increased in the upper respiratory tract of cattle 1 week after arrival to the feedlot, which was associated with a substantial increase in M. haemolytica prevalence 2 weeks later. Cattle consistently harboring M. bovis in the upper respiratory tract during the first 2 weeks at the feedlot were at a greater risk of receiving antibiotics as treatment for BRD. Additionally, we detected an effect of BRSV vaccination on M. bovis and H. somni dynamics in the upper respiratory tract, which demonstrates that interventions targeting one agent could result in unintended consequences in the respiratory microbiome.
Mycoplasma bovis is considered a commensal of the upper respiratory tract of cattle and has been documented to rapidly colonize cattle once they begin commingling in feedlots (Allen et al., 1991, 1992; Caswell and Archambault, 2008; Castillo-Alcala et al., 2012). We also observed a rapid spread of M. bovis among cattle after arrival at the feedlot. Since Mycoplasma are bacteria with an obligate parasitic lifestyle (Razin et al., 1998), M. bovis transmission in cattle should require close direct or indirect contact (Hermeyer et al., 2012b; Kanci et al., 2017; Haapala et al., 2018; Gille et al., 2020; Timonen et al., 2020). There is evidence that M. bovis can remain viable in materials commonly present in cattle production settings (Pfützner, 1984; Justice-Allen et al., 2010). However, the actual M. bovis persistence in the feedlot environment is unknown.
Mannheimia haemolytica is an upper respiratory tract commensal widely present in cattle (Rice et al., 2007; Confer, 2009; Klima et al., 2014). The observed decrease in M. haemolytica prevalence 1 week after arrival at the feedlot is very interesting. It may reflect changes in the location of the bacteria within the respiratory tract shortly after stressful events such as transportation to the feedlot (Grey and Thomson, 1971; Timsit et al., 2013; Caswell, 2014; Mosier, 2014; Boukahil and Czuprynski, 2015). Another possibility is the translocation of M. haemolytica to intracellular endosomes in bovine airway epithelial cells during the early stages of the infection (Cozens et al., 2019), which would reduce its detection in nasal swabs.
Traditionally, M. bovis has been associated with chronic stages of BRD. Furthermore, previous studies have documented that M. haemolytica-associated pneumonia during acute BRD predisposes cattle to subsequent infection by M. bovis in the lungs during chronic BRD, exhibiting caseonecrotic lesions (Adegboye et al., 1995; Rodriguez et al., 1996; Gagea et al., 2006a,b). However, our findings suggest that M. bovis presence may be a predisposing factor for an expansion of M. haemolytica in the upper respiratory tract of feedlot cattle within 1 month after arrival, in agreement with the findings of Gourlay and Houghton (1985), who documented that M. bovis increased the disease severity and extension of lesions in conventionally reared and gnotobiotic calves when inoculated before M. haemolytica. By contrast, experimental evidence indicates that M. bovis does not affect the pulmonary clearance of M. haemolytica (Lopez et al., 1982).
Harboring M. bovis in the upper respiratory tract during the first 2 weeks after arrival at the feedlot seems to be a risk factor for acute BRD. The observed increased hazard (and therefore risk) of receiving antibiotic therapy due to BRD symptoms was independent of the other BRD pathogens, suggesting that M. bovis may be participating in the pathogenesis of acute BRD, either directly or indirectly via changes in the respiratory microbiome. Timsit et al. (2018) observed that M. bovis, M. haemolytica, and P. multocida were enriched in the nasopharynx and trachea of cattle with bronchopneumonia.
We recognize that the scope of this work is not without limitations. When estimating associations between variables that change over time, time-varying confounding (Hernan and Robins, 2020) is a significant hurdle regardless of randomization at baseline. In this study, confounding due to variables at the pen level was accounted for entirely using conditional logistic regression models (Ji et al., 2020) to estimate the propensity scores for the presence of M. bovis during the first 2 weeks after arrival at the feedlot. Additionally, we incorporated within-pen variables to account for further confounding. However, bias due to confounding may still be present to a small degree (residual confounding) (Weiss and Koepsell, 2014). The Ct values without a standard curve or internal control to achieve absolute or relative quantification, respectively, include some degree of measurement error and therefore should be interpreted as an approximation. However, since the same amount of DNA per sample was used in the qPCR assays and lab personnel was blinded to treatment allocation, we consider that the measurement error in the Ct values is most likely non-differential. The antemortem diagnosis of BRD remains a clinical challenge. Therefore, non-differential misclassification of BRD could reduce the magnitude of the associations of interest (Rothman et al., 2008). Future research could expand upon the findings of this study by evaluating BRD dynamics in commercial feedlots, employing molecular techniques to track M. bovis and M. haemolytica at the strain level, and incorporating a more comprehensive approach to the diagnosis of BRD, adding thoracic ultrasound evaluations (Jourquin et al., 2022) for instance.
We are only beginning to grasp the subtleties of the interdependence among the bacterial and viral pathogens involved in BRD. Under the conditions of this study, we showed evidence suggesting that M. bovis could have a significant role during the acute phase of BRD, potentially favoring an imbalance in M. haemolytica abundance in the upper respiratory tract. Therefore, a reassessment of the relevance of M. bovis as an agent of BRD in cattle is warranted.
Data availability statement
The raw data supporting the conclusions of this article will be made available by the authors, without undue reservation.
Ethics statement
The animal study was reviewed and approved by West Texas A&M University Institutional Animal Care and Use Committee (IACUC # 2020.10.002).
Author contributions
RV-C, SP, TM, PM, and JR: conceptualization, investigation, methodology, and writing-review and editing. RV-C and JR: funding acquisition and visualization. RV-C: formal analysis and writing-original draft preparation. PM and JR: supervision. All authors have read and agreed to the published version of the manuscript.
Funding
This research was partially funded with RV-C start-up funds provided by Texas A&M University.
Acknowledgments
Authors would like to acknowledge the students and staff working at WTAMU research feedlot for their help in the execution of the field trial.
Conflict of interest
The authors declare that the research was conducted in the absence of any commercial or financial relationships that could be construed as a potential conflict of interest.
Publisher's note
All claims expressed in this article are solely those of the authors and do not necessarily represent those of their affiliated organizations, or those of the publisher, the editors and the reviewers. Any product that may be evaluated in this article, or claim that may be made by its manufacturer, is not guaranteed or endorsed by the publisher.
References
Adegboye, D. S., Halbur, P. G., Cavanaugh, D. L., Werdin, R. E., Chase, C. C. L., Miskimins, D. W., et al. (1995). Immunohistochemical and pathological study of Mycoplasma bovis-associated lung abscesses in calves. J. Vet. Diagn. Invest. 7, 333–337. doi: 10.1177/104063879500700306
Allen, J. W., Viel, L., Bateman, K. G., and Rosendal, S. (1992). Changes in the bacterial flora of the upper and lower respiratory tracts and bronchoalveolar lavage differential cell counts in feedlot calves treated for respiratory diseases. Can. J. Vet. Res. 56, 177–183.
Allen, J. W., Viel, L., Bateman, K. G., Rosendal, S., Shewen, P. E., and Physick-Sheard, P. (1991). The microbial flora of the respiratory tract in feedlot calves: associations between nasopharyngeal and bronchoalveolar lavage cultures. Can. J. Vet. Res. 55, 341–346.
Angen, O., Thomsen, J., Larsen, L. E., Larsen, J., Kokotovic, B., Heegaard, P. M., et al. (2009). Respiratory disease in calves: microbiological investigations on trans-tracheally aspirated bronchoalveolar fluid and acute phase protein response. Vet. Microbiol. 137, 165–171. doi: 10.1016/j.vetmic.2008.12.024
Bakaletz, L. O. (2017). Viral-bacterial co-infections in the respiratory tract. Curr. Opin. Microbiol. 35, 30–35. doi: 10.1016/j.mib.2016.11.003
Becker, C. A. M., Ambroset, C., Huleux, A., Vialatte, A., Colin, A., Tricot, A., et al. (2020). Monitoring Mycoplasma bovis diversity and antimicrobial susceptibility in calf feedlots undergoing a respiratory disease outbreak. Pathogens 9, 593. doi: 10.3390/pathogens9070593
Booker, C. W., Abutarbush, S. M., Morley, P. S., Jim, G. K., Pittman, T. J., Schunicht, O. C., et al. (2008). Microbiological and histopathological findings in cases of fatal bovine respiratory disease of feedlot cattle in western Canada. Can. Vet. J. 49, 473–481.
Boukahil, I., and Czuprynski, C. J. (2015). Characterization of Mannheimia haemolytica biofilm formation in vitro. Vet. Microbiol. 175, 114–122. doi: 10.1016/j.vetmic.2014.11.012
Brault, S. A., Hannon, S. J., Gow, S. P., Warr, B. N., Withell, J., Song, J., et al. (2019). Antimicrobial use on 36 beef feedlots in Western Canada: 2008–2012. Front. Vet. Sci. 6, 329. doi: 10.3389/fvets.2019.00329
Burki, S., Frey, J., and Pilo, P. (2015). Virulence, persistence and dissemination of Mycoplasma bovis. Vet. Microbiol. 179, 15–22. doi: 10.1016/j.vetmic.2015.02.024
Castillo-Alcala, F., Bateman, K. G., Cai, H. Y., Schott, C. R., Parker, L., Clark, M., et al. (2012). Prevalence and genotype of Mycoplasma bovis in beef cattle after arrival at a feedlot. Am. J. Vet. Res. 73, 1932–1943. doi: 10.2460/ajvr.73.12.1932
Caswell, J. L. (2014). Failure of respiratory defenses in the pathogenesis of bacterial pneumonia of cattle. Vet. Pathol. 51, 393–409.doi: 10.1177/0300985813502821
Caswell, J. L., and Archambault, M. (2008). Mycoplasma bovis pneumonia in cattle. Anim. Health Res. Rev. 8, 161–186. doi: 10.1017/S1466252307001351
Centeno-Martinez, R. E., Glidden, N., Mohan, S., Davidson, J. L., Fernandez-Juricic, E., Boerman, J. P., et al. (2022). Identification of bovine respiratory disease through the nasal microbiome. Anim. Microbiome 4, 15. doi: 10.1186/s42523-022-00167-y
Cirone, F., Padalino, B., Tullio, D., Capozza, P., Lo Surdo, M., Lanave, G., et al. (2019). Prevalence of pathogens related to bovine respiratory disease before and after transportation in beef steers: preliminary results. Animals 9, 06. doi: 10.3390/ani9121093
Confer, A. W. (2009). Update on bacterial pathogenesis in BRD. Anim. Health Res. Rev. 10, 145–148. doi: 10.1017/S1466252309990193
Cozens, D., Sutherland, E., Lauder, M., Taylor, G., Berry, C., and Davies, R. (2019). Pathogenic Mannheimia haemolytica invades differentiated bovine airways epithelial cells. Infect. Immun. 87, e00078–00019. doi: 10.1128/IAI.00078-19
Crosby, W. B., Pinnell, L. J., Richeson, J. T., Wolfe, C., Loy, J. D., Gow, S. P., et al. (2022). Comparison of sampling and diagnostic techniques for recovery of Mannheimia haemolytica and respiratory microbiome characterization from feedlot cattle. Res. Square. doi: 10.21203/rs.3.rs-1054768/v1
Dohoo, I., Martin, W., and Stryhn, H. (2012). Methods in Epidemiologic Research. Charlottetown, Prince Edward Island, Canada: VER.
Dudek, K., Nicholas, R. A. J., Szacawa, E., and Bednarek, D. (2020). Mycoplasma bovis infections-occurrence, diagnosis and control. Pathogens 9, 640. doi: 10.3390/pathogens9080640
Gagea, M. I., Bateman, K. G., Shanahan, R. A., van Dreumel, T., McEwen, B. J., Carman, S., et al. (2006a). Naturally occurring Mycoplasma bovis-associated pneumonia and polyarthritis in feedlot beef calves. J. Vet. Diagn. Invest. 18, 29–40. doi: 10.1177/104063870601800105
Gagea, M. I., Bateman, K. G., van Dreumel, T., McEwen, B. J., Carman, S., Archambault, M., et al. (2006b). Diseases and pathogens associated with mortality in Ontario beef feedlots. J. Vet. Diagn. Invest. 18, 18–28. doi: 10.1177/104063870601800104
Gershwin, L. J., Van Eenennaam, A. L., Anderson, M. L., McEligot, H. A., Shao, M. X., Toaff-Rosenstein, R., et al. (2015). Single pathogen challenge with agents of the bovine respiratory disease complex. PLoS ONE 10, e0142479. doi: 10.1371/journal.pone.0142479
Gille, L., Evrard, J., Callens, J., Supre, K., Gregoire, F., Boyen, F., et al. (2020). The presence of Mycoplasma bovis in colostrum. Vet. Res. 51, 54. doi: 10.1186/s13567-020-00778-w
Gourlay, R. N., and Houghton, S. B. (1985). Experimental pneumonia in conventionally reared and gnotobiotic calves by dual infection with Mycoplasma bovis and Pasteurella haemolytica. Res. Vet. Sci. 38, 377–382. doi: 10.1016/S0034-5288(18)31813-7
Grey, C. T., and Thomson, R. G. (1971). Pasteurella haemolytica in the tracheal air of calves. Can. J. Comp. Med. 35, 121–128.
Griffin, D., Chengappa, M. M., Kuszak, J., and McVey, D. S. (2010). Bacterial pathogens of the bovine respiratory disease complex. Vet. Clin. North Am. Food Anim. Pract. 26, 381–394. doi: 10.1016/j.cvfa.2010.04.004
Haapala, V., Pohjanvirta, T., Vahanikkila, N., Halkilahti, J., Simonen, H., Pelkonen, S., et al. (2018). Semen as a source of Mycoplasma bovis mastitis in dairy herds. Vet. Microbiol. 216, 60–66. doi: 10.1016/j.vetmic.2018.02.005
He, Z. (2018). Inverse conditional probability weighting with clustered data in causal inference. Arxiv, preprint arXiv:1808.01647.
Hermeyer, K., Buchenau, I., Thomasmeyer, A., Baum, B., Spergser, J., Rosengarten, R., et al. (2012a). Chronic pneumonia in calves after experimental infection with Mycoplasma bovis strain 1067: characterization of lung pathology, persistence of variable surface protein antigens and local immune response. Acta Vet. Scand. 54, 9. doi: 10.1186/1751-0147-54-9
Hermeyer, K., Peters, M., Brugmann, M., Jacobsen, B., and Hewicker-Trautwein, M. (2012b). Demonstration of Mycoplasma bovis by immunohistochemistry and in situ hybridization in an aborted bovine fetus and neonatal calf. J. Vet. Diagn. Invest. 24, 364–369. doi: 10.1177/1040638711435145
Ji, J., Wang, C., He, Z., Hay, K. E., Barnes, T. S., and O'Connor, A. M. (2020). Comparing the estimates of effect obtained from statistical causal inference methods: an example using bovine respiratory disease in feedlot cattle. PLoS ONE 15, e0233960. doi: 10.1371/journal.pone.0233960
Jourquin, S., Bokma, J., De Cremer, L., van Leenen, K., Vereecke, N., and Pardon, B. (2022). Randomized field trial comparing the efficacy of florfenicol and oxytetracycline in a natural outbreak of calf pneumonia using lung reaeration as a cure criterion. J. Vet. Intern. Med. 36, 820–8. doi: 10.1111/jvim.16348
Justice-Allen, A., Trujillo, J., Corbett, R., Harding, R., Goodell, G., and Wilson, D. (2010). Survival and replication of Mycoplasma species in recycled bedding sand and association with mastitis on dairy farms in Utah. J. Dairy Sci. 93, 192–202. doi: 10.3168/jds.2009-2474
Kanci, A., Wawegama, N. K., Marenda, M. S., Mansell, P. D., Browning, G. F., and Markham, P. F. (2017). Reproduction of respiratory mycoplasmosis in calves by exposure to an aerosolised culture of Mycoplasma bovis. Vet. Microbiol. 210, 167–173. doi: 10.1016/j.vetmic.2017.09.013
Klima, C. L., Zaheer, R., Cook, S. R., Booker, C. W., Hendrick, S., Alexander, T. W., et al. (2014). Pathogens of bovine respiratory disease in North American feedlots conferring multidrug resistance via integrative conjugative elements. J. Clin. Microbiol. 52, 438–448. doi: 10.1128/JCM.02485-13
Li, Y., Zheng, H., Liu, Y., Jiang, Y., Xin, J., Chen, W., et al. (2011). The complete genome sequence of Mycoplasma bovis strain Hubei-1. PLoS ONE 6, e20999. doi: 10.1371/journal.pone.0020999
Lion, A., Secula, A., Rancon, C., Boulesteix, O., Pinard, A., Deslis, A., et al. (2021). Enhanced pathogenesis caused by influenza D virus and Mycoplasma bovis coinfection in calves: a disease severity linked with overexpression of IFN-g as a key player of the enhanced innate immune response in lungs. Microbiol. Spectr. 9, e01690–01621. doi: 10.1128/spectrum.01690-21
Lopez, A., Maxie, M. G., Savan, M., Ruhnke, H. L., Thomson, R. G., Barnum, D. A., et al. (1982). The pulmonary clearance of Pasteurella haemolytica in calves infected with bovine virus diarrhea or Mycoplasma bovis. Can. J. Comp. Med. 46, 302–306.
Mason, C., Errington, J., Foster, G., Thacker, J., Grace, O., and Baxter-Smith, K. (2022). Mannheimia haemolytica serovars associated with respiratory disease in cattle in Great Britain. BMC Vet. Res. 18, 5. doi: 10.1186/s12917-021-03121-3
Mosier, D. (2014). Review of BRD pathogenesis: the old and the new. Anim. Health Res. Rev. 15, 166–168. doi: 10.1017/S1466252314000176
Parker, A. M., Sheehy, P. A., Hazelton, M. S., Bosward, K. L., and House, J. K. (2018). A review of mycoplasma diagnostics in cattle. J. Vet. Intern. Med. 32, 1241–1252. doi: 10.1111/jvim.15135
Peel, D. S. (2020). The effect of market forces on bovine respiratory disease. Vet. Clin. North Am. Food Anim. Pract. 36, 497–508. doi: 10.1016/j.cvfa.2020.03.008
Perez-Casal, J. (2020). Pathogenesis and Virulence of Mycoplasma bovis. Vet. Clin. North Am. Food Anim. Pract. 36, 269–278. doi: 10.1016/j.cvfa.2020.02.002
Pfützner, H. (1984). Tenazität von Mycoplasma bovis. Zentralblatt für Bakteriologie Mikrobiologie und Hygiene. Ser. A Med. Microbiol. Infect. Dis. Virol. Parasitol. 258, 38–41. doi: 10.1016/S0176-6724(84)80006-1
Pillen, J. L., Pinedo, P. J., Ives, S. E., Covey, T. L., Naikare, H. K., and Richeson, J. T. (2016). Alteration of activity variables relative to clinical diagnosis of bovine respiratory disease in newly received feedlot cattle. Bovine Practition. 50, 1–8. doi: 10.21423/bovine-vol50no1p1-8
Powledge, S. A., McAtee, T. B., Woolums, A. R., Falkner, T. R., Groves, J. T., Thoresen, M., et al. (2022). Clinical and microbiological effects in high-risk beef calves administered intranasal or parenteral modified-live virus vaccines. J. Anim. Sci. (In Press).
Prakash, O., Parmar, M., Vaijanapurkar, M., Rale, V., and Shouche, Y. S. (2021). Recent trend, biases and limitations of cultivation-based diversity studies of microbes. FEMS Microbiol. Lett. 368, fnab118. doi: 10.1093/femsle/fnab118
Razin, S., Yogev, D., and Naot, Y. (1998). Molecular biology and pathogenicity of Mycoplasmas. Microbiol. Mol. Biol. Rev. 62, 1094–1156. doi: 10.1128/MMBR.62.4.1094-1156.1998
Rice, J. A., Carrasco-Medina, L., Hodgins, D. C., and Shewen, P. E. (2007). Mannheimia haemolytica and bovine respiratory disease. Anim. Health Res. Rev. 8, 117–128. doi: 10.1017/S1466252307001375
Rodriguez, F., Bryson, D. G., Ball, H. J., and Forster, F. (1996). Pathological and immunohistochemical studies of natural and experimental Mycoplasma bovis pneumonia in calves. J. Comp. Pathol. 115, 151–162. doi: 10.1016/S0021-9975(96)80037-5
Rothman, K. J., Greenland, S., and Lash, T. L. (2008). “Validity in Epidemiologic Studies,” in Modern Epidemiology, eds. K. J. Rothman, S. Greenland and T. L. Lash. 3rd ed (Philadelphia, PA: Lippincott Williams and Wilkins), 128–147. Available online at: https://shop.lww.com/Modern-Epidemiology/p/9781451193282
Self, S. G., and Liang, K.-Y. (1987). Asymptotic properties of maximum likelihood estimators and likelihood ratio tests under nonstandard conditions. J. Am. Stat. Assoc. 82, 605–610. doi: 10.1080/01621459.1987.10478472
Timonen, A. A. E., Autio, T., Pohjanvirta, T., Hakkinen, L., Katholm, J., Petersen, A., et al. (2020). Dynamics of the within-herd prevalence of Mycoplasma bovis intramammary infection in endemically infected dairy herds. Vet. Microbiol. 242, 108608. doi: 10.1016/j.vetmic.2020.108608
Timsit, E., Christensen, H., Bareille, N., Seegers, H., Bisgaard, M., and Assie, S. (2013). Transmission dynamics of Mannheimia haemolytica in newly-received beef bulls at fattening operations. Vet. Microbiol. 161, 295–304. doi: 10.1016/j.vetmic.2012.07.044
Timsit, E., Workentine, M., van der Meer, F., and Alexander, T. (2018). Distinct bacterial metacommunities inhabit the upper and lower respiratory tracts of healthy feedlot cattle and those diagnosed with bronchopneumonia. Vet. Microbiol. 221, 105–113. doi: 10.1016/j.vetmic.2018.06.007
USDA (2013). “Feedlot 2011 Part IV: health and health management on U.S. feedlots with a capacity of 1000 or more head”, in USDA-APHIS-VS-CEAH-NAHMS. Available online at: https://www.aphis.usda.gov/animal_health/nahms/feedlot/downloads/feedlot2011/Feed11_dr_PartIV_1.pdf
USDA (2017). “Death loss in U.S. cattle and calves due to predator and nonpredator causes, 2015”, in USDA-APHIS-VS-CEAH-NAHMS. Available online at: https://www.aphis.usda.gov/animal_health/nahms/general/downloads/cattle_calves_deathloss_2015.pdf
Keywords: Mycoplasma bovis, acute BRD, beef cattle, IPW models, Mannheimia haemolytica
Citation: Valeris-Chacin R, Powledge S, McAtee T, Morley PS and Richeson J (2022) Mycoplasma bovis is associated with Mannheimia haemolytica during acute bovine respiratory disease in feedlot cattle. Front. Microbiol. 13:946792. doi: 10.3389/fmicb.2022.946792
Received: 18 May 2022; Accepted: 11 July 2022;
Published: 01 August 2022.
Edited by:
Stefan Schild, University of Graz, AustriaReviewed by:
Anthony W. Confer, Oklahoma State University, United StatesBrent Credille, University of Georgia, United States
John A. Ellis, University of Saskatchewan, Canada
Jodi L. McGill, Iowa State University, United States
Copyright © 2022 Valeris-Chacin, Powledge, McAtee, Morley and Richeson. This is an open-access article distributed under the terms of the Creative Commons Attribution License (CC BY). The use, distribution or reproduction in other forums is permitted, provided the original author(s) and the copyright owner(s) are credited and that the original publication in this journal is cited, in accordance with accepted academic practice. No use, distribution or reproduction is permitted which does not comply with these terms.
*Correspondence: Robert Valeris-Chacin, cnZhbGVyaXNjaGFjaW5AY3ZtLnRhbXUuZWR1