- 1Department of Dermatology, University Hospitals Cleveland Medical Center/Case Western Reserve University School of Medicine, Cleveland, OH, United States
- 2Department of Biomedical, Surgical and Dental Sciences, University of Milan, Milan, Italy
- 3Clinical Dermatology, IRCCS Istituto Ortopedico Galeazzi, Milan, Italy
- 4Department of Pharmaceutical and Pharmacological Sciences, PhD Degree Program in Pharmacological Sciences, University of Padua, Padua, Italy
Atopic dermatitis (AD) is associated with cutaneous dysbiosis, barrier defects, and immune dysregulation, but the interplay between these factors needs further study. Early-onset barrier dysfunction may facilitate an innate immune response to commensal organisms and, consequently, the development of allergic sensitization. We aimed to compare the cutaneous microbiome in patients with active dermatitis with and without a history of childhood flexural dermatitis (atopic dermatitis). Next-gen Ion-Torrent deep-sequencing identified AD-associated changes in the skin bacterial microbiome (“bacteriome”) and fungal microbiome (“mycobiome”) of affected skin in swabs from areas of skin affected by dermatitis. Data were analyzed for diversity, abundance, and inter-kingdom correlations. Microbial interactions were assessed in biofilms using metabolic activity (XTT) assay and scanning electron microscopy (SEM), while host-pathogen interactions were determined in cultured primary keratinocytes exposed to biofilms. Increased richness and abundance of Staphylococcus, Lactococcus, and Alternaria were found in atopics. Staphylococcus and Alternaria formed robust mixed-species biofilms (based on XTT and SEM) that were resistant to antifungals/antimicrobials. Furthermore, their biofilm supernatant was capable of influencing keratinocytes biology (pro-inflammatory cytokines and structural proteins), suggesting an additive effect on AD-associated host response. In conclusion, microbial inter-kingdom and host-microbiome interactions may play a critical role in the modulation of atopic dermatitis to a greater extent than in non-atopic adults with allergic contact dermatitis.
Introduction
Atopic dermatitis (AD) is a chronic condition of the skin with periodic cutaneous flares frequently accompanied by respiratory symptoms (atopic march) such as allergic asthma or rhinitis (Thestrup-Pedersen, 2000). AD is a multifactorial disease, in which changes in microbial flora, skin barrier defects, and dysregulation of innate and adaptive immunity have been noted (Jinnestal et al., 2014; Nedorost, 2020). Culture-based studies have previously associated AD flares with distinct populations of cutaneous bacteria (e.g., Staphylococcus aureus) and fungi (e.g., Malassezia and Candida) (Ramirez De Knott et al., 2005, 2006; Kong et al., 2012; Chandra et al., 2016) which seem to play a potentially prominent role in cutaneous sensitization (Ramirez De Knott et al., 2005, 2006).
Cutaneous contact sensitization led to durable antigen-specific Th2 responses in humans with AD (Newell et al., 2013), suggesting that the “atopic march” originates as a response to allergic contact dermatitis. In the context of this predisposition to cutaneous sensitization, commensal organisms may colonize and sensitize patients with AD (Ramirez De Knott et al., 2006). The progression of atopic dermatitis is strongly linked to defects in skin barrier function, reduced levels of antimicrobial peptides (AMPs), and an increased local inflammatory response (Werfel et al., 2016). Production of AMPs can be positively regulated by TNF-α and IL-1β, and negatively regulated by TSLP (Lee et al., 2016; Li et al., 2016).
Sequencing analyses allow the characterization of skin microbiota and have largely focused on the bacterial microbiota (bacteriome) (Kong et al., 2012; Seite et al., 2014). Although bacteria coexist with fungi in most body sites colonized by microbes, the role of the fungal microbiome (mycobiome) in AD has been investigated less thoroughly.
Previous studies have compared the bacteriome and mycobiome in healthy patients to those with AD, which we define as a history of childhood-onset flexural dermatitis. Genetically induced barrier dysfunction and the resultant irritant dermatitis generate chronic innate immune signals leading to sensitization to weakly potent allergens including proteins with Th2 skewing (Kohli and Nedorost, 2016; Nedorost, 2020). Our current study is the first to characterize the bacteriome and mycobiome profiles of affected and unaffected skin in patients with a history of childhood-onset flexural dermatitis (atopic dermatitis) compared with those without childhood flexural dermatitis but with active dermatitis, under investigation for allergic contact dermatitis (non-atopic patients). Comparison of two cohorts with dermatitis but with a different history of the time of onset helps us to understand the factors of type and duration of innate immunity and relationship with communities of cutaneous commensal organisms.
This is important because dermatitis is increasingly recognized as a genetic (e.g., AD) and environmental (e.g., allergic contact dermatitis) disease with considerable overlap between the groups. We hypothesized that patients with childhood-onset flexural dermatitis (aka “atopic dermatitis”) were more likely to be sensitized by organisms in the cutaneous microbiome that impair the already dysfunctional cutaneous barrier. The implication of this is that patients sensitized to the cutaneous microbiome are less likely to benefit from avoidable allergens in the workplace or in personal care products, more likely to benefit from immunomodulatory treatments such as monoclonal antibodies or JAK-STAT inhibitors, as well as from topical or systemic treatments that aim to improve the diversity of the microbiome, decrease pathogen concentration, and break or dissolve biofilm.
We conducted a proof-of-concept pilot study to characterize the skin bacteriome and mycobiome of atopic vs. non-atopic patients with active dermatitis. We also identified inter-kingdom correlations, determined whether bacteria and fungi interact as biofilms, and investigated whether these biofilms secrete biomolecules that influence the production of cytokines and structural proteins in primary keratinocytes.
Results
Clustering and diversity analyses
Bacterial and fungal DNA sequencing produced 1.59 and 1.77 million raw sequences, respectively. In Atopics, skin bacteriome formed clusters which exhibited partial overlap between affected and unaffected sites (Figures 1A–F), while the mycobiome exhibited complete overlap (Figures 1G–J). Among the non-atopics, this pattern in clustering was reversed, with bacteria showing complete overlap (Figures 1M–R), while the mycobiome exhibited clustering with partial overlap (Figures 1S–X). However, there was no significant difference in group homogeneities (based on the distance to the centroid) between affected and unaffected skin in both AD and non-atopic skin (P ≥ 0.11). Three principal components accounted for variability in the bacteriome at the phylum level, while in the mycobiome, 6 and 13 fungal species accounted for 99% variability in affected and unaffected samples, respectively.
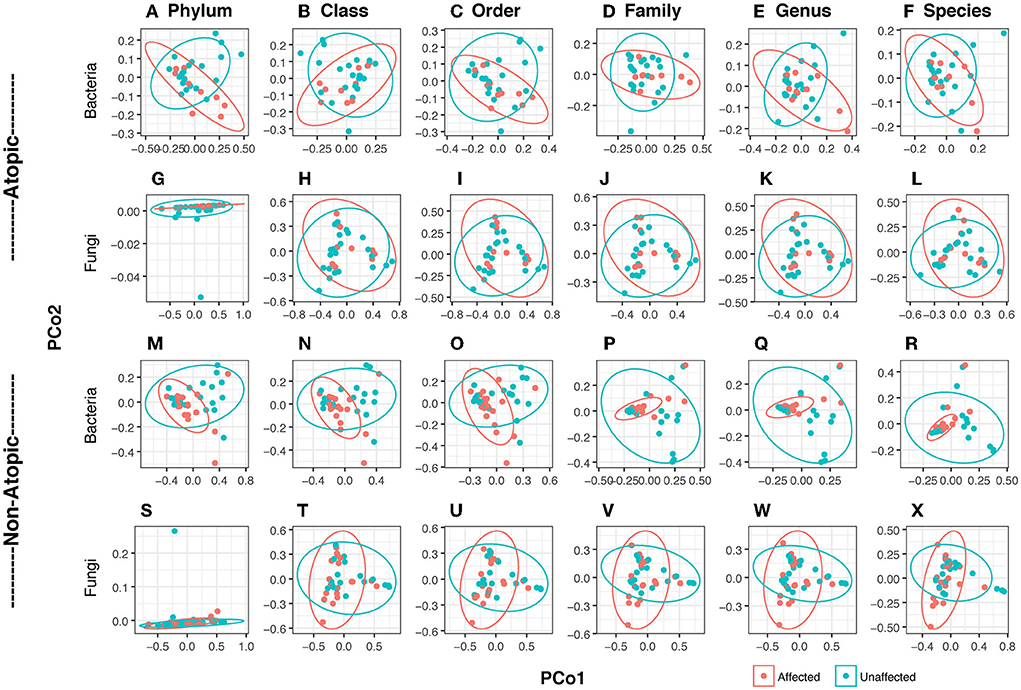
Figure 1. Principal coordinates analysis (PCoA) of bacteriome and mycobiome in skin swabs of atopic and non-atopic patients. Clustering at all taxa levels (Phylum, Class, Order, Family, Genus, and Species) is shown. Ellipses are drawn at a confidence level of 0.95. (A–L) Atopic, (M–X) non-atopic, (A–F,M–R) bacteria, (G–L,S–X) fungi. Principal coordinates analysis (PCoA) was conducted with identified reads/OTUs using classical multidimensional scaling (functions dist/Euclidean, cmdscale) to analyze the distribution of dissimilarities and hierarchical clustering in dendrograms in vegan (Oksanen et al., 2017). Homogeneity of groups and beta diversity was evaluated using adonis (which explores the differences in group means and implements a multivariate analysis of variances using distance matrices) and betadisper (which studies the differences in group homogeneities, similar to Levene's test of the equality of variances) functions in vegan.
The richness of affected skin was significantly lower than that of unaffected skin at the Class level (P = 0.03) and a trend at the phylum level (P = 0.054). Shannon diversity index (SDI) among patients with AD was similar between the affected and unaffected areas. There were no significant differences in diversity between affected and unaffected areas in non-atopics (Supplementary Figure 1).
Core bacteriome and mycobiome
The core bacteriome (comprising taxa detected with a frequency of at least 20%, at an abundance >1%) comprised four unique Genera (Gemella, Veillonella, Fusobacterium, Leptotrichia) in unaffected samples and one (Leuconostoc) in affected AD samples, while the core mycobiome comprised two and five phyla in AD and non-atopic subjects, respectively (Figure 2). Interestingly, affected AD samples contained higher numbers of unique fungal genera (23), compared to unaffected samples (0), while in non-atopic samples, unaffected samples had a larger number of unique genera (19) compared to affected samples (2). Similar patterns were observed for other taxa levels (Figure 2).
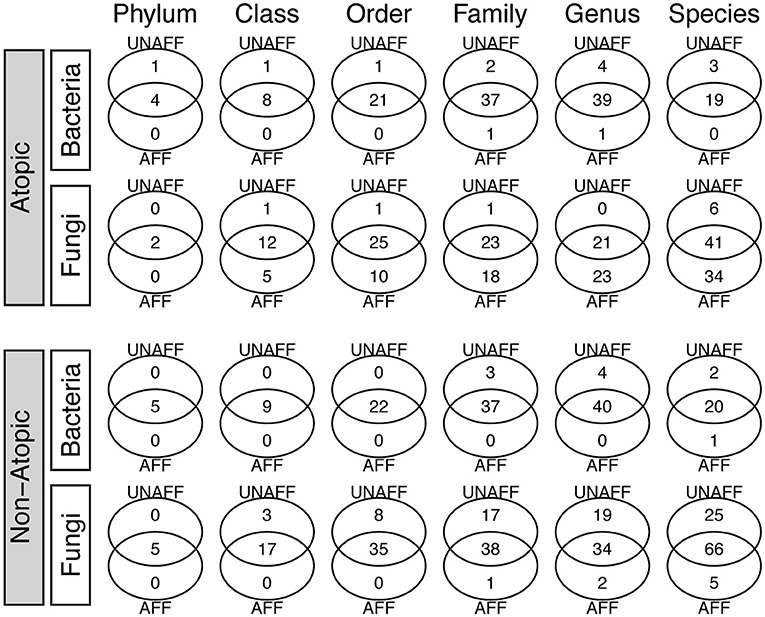
Figure 2. Core bacteriome and mycobiome in affected (AFF) and unaffected (UNAFF) samples of atopic and non-atopic patients. Numbers indicate the actual count of members identified in each taxon (Phylum through Species). The core biome comprised taxa with a frequency of at least 20% (and relative abundance ≥1%).
Abundance profile of bacteriome and mycobiome
Analysis of the abundance distribution of different bacterial and fungal phyla in AD and non-atopic subjects revealed three bacterial (Actinobacteria, Firmicutes, and Proteobacteria) and two fungal (Ascomycota and Basidiomycota) phyla dominating the bacteriome and mycobiome, respectively (Figure 3).
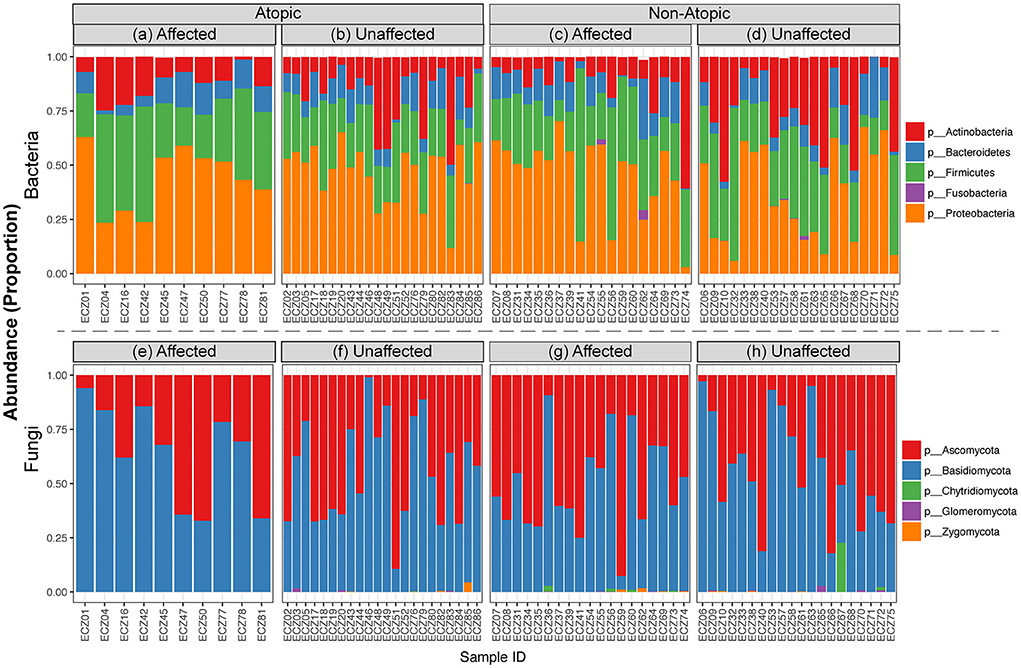
Figure 3. Relative abundance of bacterial and fungal taxa in affected and unaffected skin in atopic and non-atopic dermatitis. Stacked bar charts showing the distribution of (a–d) bacterial and (e–h) fungal phyla across affected and unaffected samples collected from atopic and non-atopic patients.
Among samples from AD subjects, abundance levels of bacteria were significantly different at Family, Genus, and Species levels between affected and unaffected skin in both AD and non-atopic subject samples (P ≤ 0.049 and =0.013, respectively) (Figure 4).
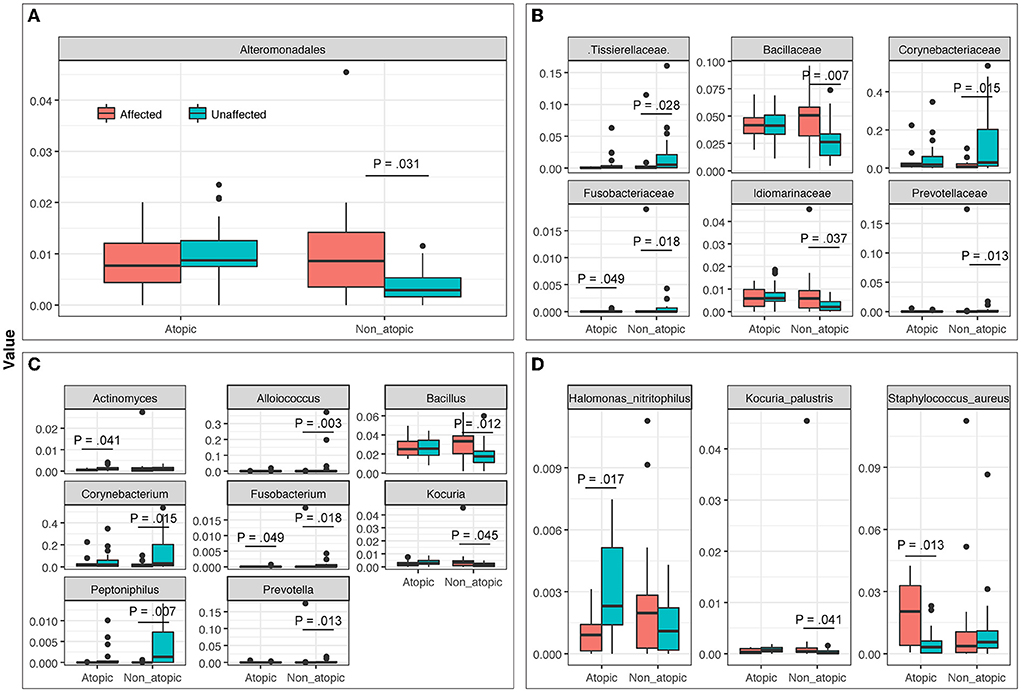
Figure 4. The abundance of bacterial taxa at (A) Order, (B) Family, (C) Genus, and (D) Species levels in affected and unaffected skin of atopic and non-atopic patients. Taxa that exhibited statistically significant differences (P < 0.05) are shown.
Furthermore, several known allergenic or pathogenic fungal genera showed noticeable trends in patients with AD, where levels of Alternaria, Scleroderma, and Fusarium were increased in the affected skin, compared to unaffected skin (Supplementary Figure 2).
Genus-level abundance comparison
The most abundant bacterial genera in Atopics were Staphylococcus and Halomonas in affected and unaffected skin, respectively (Figure 5). In Non-Atopic patients, Halomonas and Corynebacterium were the most abundant bacterial genera in affected and unaffected skin, respectively (Figure 5). A comparison of mean proportions revealed that 24 and 27 genera were significantly different in the Atopic and Non-Atopic groups, respectively. These genera included Staphylococcus and Lactococcus, which were detected at significantly elevated levels in affected skin (P = 0.030 and 0.025, respectively), while the abundance of Bacteroides was significantly decreased in affected skin (P = 0.049), compared to unaffected skin in patients with Atopic dermatitis (Supplementary Table 3). In contrast, in the patients with Non-Atopic dermatitis, an abundance of Bacillus and Brevibacillus was significantly increased while that of Corynebacterium was significantly decreased in affected vs. unaffected skin (P ≤ 0.018, Table 1). The abundance of Fusobacterium was significantly decreased in affected skin in both atopic and non-atopic groups, compared to unaffected skin (P = 0.049 and 0.018, respectively).
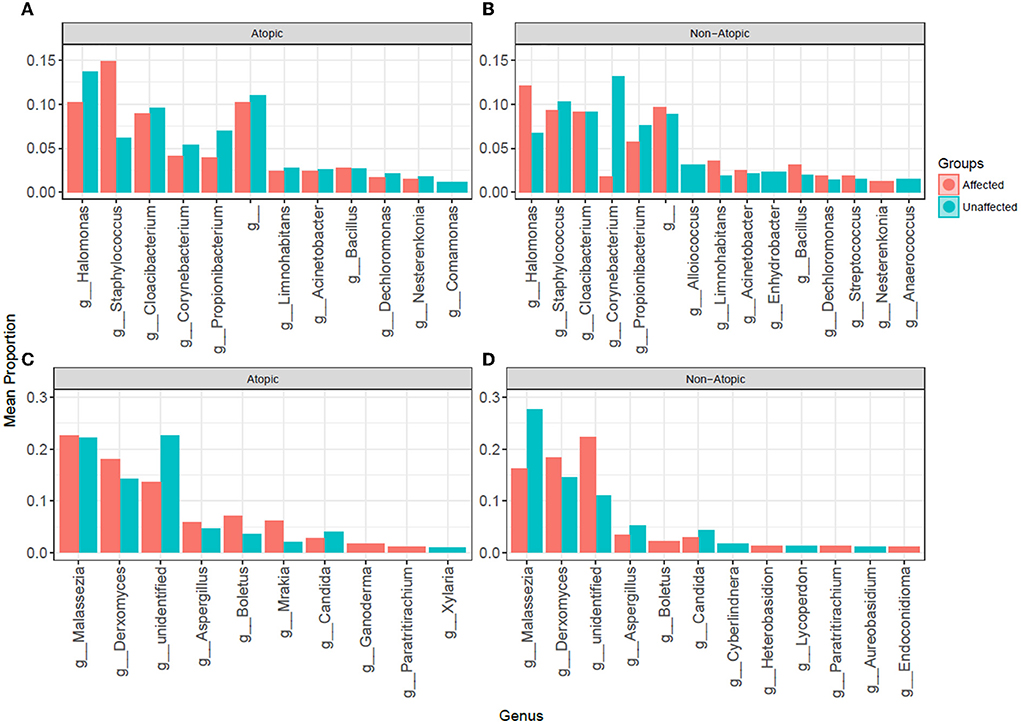
Figure 5. The abundance of (A,B) bacterial and (C,D) fungal genera in affected and unaffected skin of patients with atopic and non-atopic dermatitis.
The mycobiome also exhibited specific significant differences between affected and unaffected skin in AD and non-atopic subject samples (P ≤ 0.048). A statistically significant increase in the abundance of Candida albicans was observed in affected samples from AD skin (P = 0.046), which was also seen in non-atopic samples, however, the abundance in non-atopic samples did not reach significance (Figure 6).
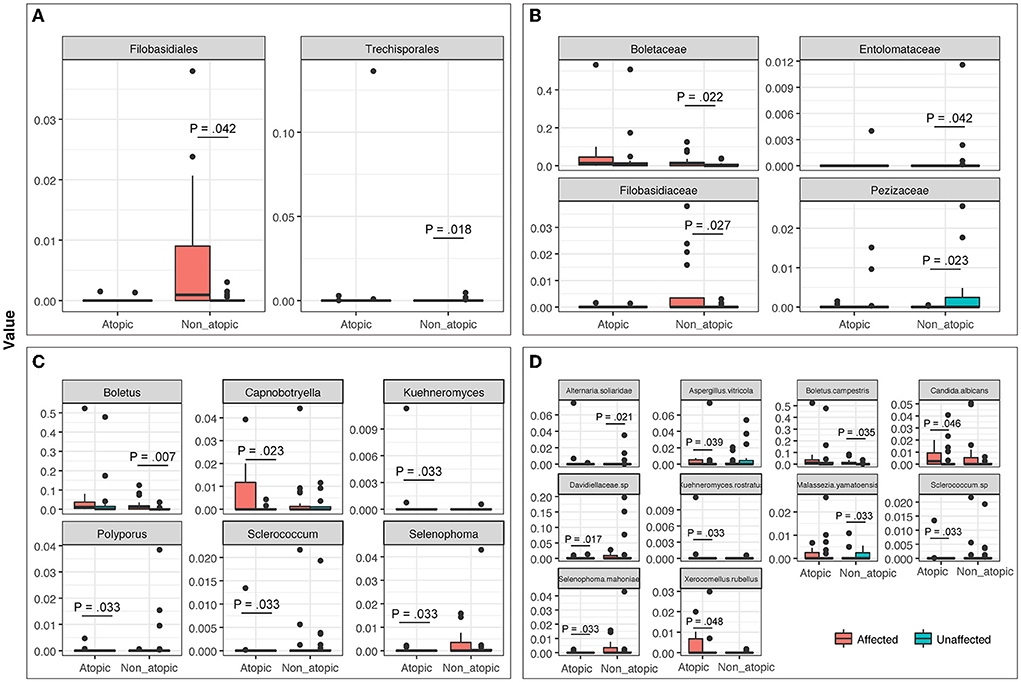
Figure 6. The abundance of fungal taxa at (A) Order, (B) Family, (C) Genus, and (D) Species levels in affected and unaffected skin of atopic and non-atopic patients. Taxa that exhibited statistically significant differences (P < 0.05) are shown.
Mycobiome analysis also revealed that Malassezia was the most abundant fungal genus in the affected and unaffected skin of atopics and the unaffected skin of non-atopics (Figure 4). There was no significant difference in the abundance of fungal genera between affected and unaffected skin in both patient groups. However, several known allergenic or pathogenic fungal genera showed a noticeable trend in Atopics, where levels of Alternaria, Scleroderma, and Fusarium tended to increase in the affected skin, while that of Trichosporon tended to decrease, compared to unaffected skin (Supplementary Figure 2).
Fold changes in genus abundance between affected and unaffected skin
Next, we determined the fold change in the abundance of bacterial and fungal genera between affected and unaffected skin in the two subject groups.
Staphylococcus abundance was increased by 2.4-fold in affected skin compared to unaffected areas in patients with AD (14.8 vs. 6.1%, respectively, P = 0.030), but not in non-atopic controls (9.3 vs. 10.3%, respectively).
In the mycobiome, the highest fold change was observed for Alternaria, which was elevated by 137-fold in affected skin compared to unaffected skin of patients with AD (0.97 vs. 0.007%, respectively, P = 0.18), but decreased by 15-fold in non-atopic subjects (0.03 vs. 0.45%) (Supplementary Figure 2).
Staphylococcus and Alternaria form robust, drug-resistant, mixed-species biofilms
Our results showed that in patients with AD, Staphylococcus abundance was significantly elevated in affected skin. Additionally, the fold change in abundance between affected and unaffected AD skin was the highest for Alternaria, an allergenic fungus previously associated with asthma, a key manifestation of adult AD and the “atopic march” (Knutsen et al., 2012; Celakovska et al., 2015; Fukutomi and Taniguchi, 2015). Allergic sensitization through the skin is facilitated by a high concentration of antigen per unit area of inflamed skin. Therefore, we determined whether these two genera interact with each other to form biofilms. Alternaria formed robust biofilms as determined by scanning electron microscopy (SEM) and metabolic activity assay. SEM analyses showed that Alternaria alternata and Staphylococcus aureus were able to form robust biofilms when grown singly (Supplementary Figures 3A,B). However, in the presence of Staphylococcus, Alternaria biofilms were increased (Supplementary Figures 3C–E). Notably, mixed biofilms comprised bacterial cells closely aggregating on the fungal hyphae and distinctly enmeshed into the extracellular matrix (Supplementary Figures 3C,D). Fungal biofilm formation increased in the presence of bacterial cells, in a dose-dependent manner (Supplementary Figure 3E). A significant increase in biofilm formation was observed in the presence of 103 and 105 S. aureus cells, compared to Alternata biofilms formed in the absence of S. aureus (P ≤ 0.00015, for biofilms formed in presence of both bacterial densities, compared to biofilms formed with no bacteria).
Biofilms constituents that influence cytokine production in keratinocytes
Next, we determined whether mixed-species biofilms formed by S. aureus and A. alternata secrete factors that influence the expression of host variables associated with AD including thymic stromal lymphopoietin (TSLP) (known to be highly expressed in the skin of patients with AD and believed to activate downstream immune signals) (Komine, 2009) and pro-inflammatory cytokines (IL-18, IL-23, TNF-α) in keratinocytes (Werfel et al., 2016) (Figure 7).
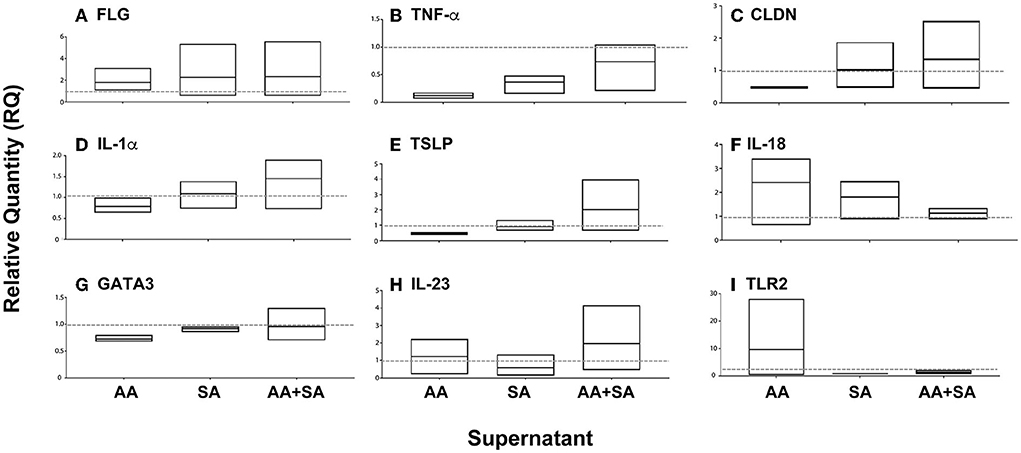
Figure 7. Effect of supernatants of single or mixed-species biofilms on the expression of cytokines and structural proteins in cultured keratinocytes. Biofilms were formed with Alternaria alternata (AA), Staphylococcus aureus (SA), or both (AA+SA), and their supernatants were added to cultured keratinocytes. Real-time PCR was used to evaluate the expression of specific cytokines and structural proteins. Average fold change (Min to Max, n = 3) with the mean represented in the floating boxes are shown compared to the normalized value for controls (dashed line at Fold Change 1).
Supernatants isolated from biofilms formed by A. alternata induced a nearly ten-fold increase in mRNA expression of Toll-Like Receptor 2 (TLR2) levels (pattern recognition receptors that are involved in the immune response against different pathogenic microorganisms), with minimal increase in IL-23 and IL-18 mRNA expression levels. Furthermore, exposure to this supernatant also reduced the expression levels of Claudin-1 (CLDN1), which encodes the claudin responsible for the paracellular barrier at tight junctions in the epidermis, and its reduction was reported to play a major role in AD (Tokumasu et al., 2016). Additionally, a reduction in GATA-binding protein 3 (GATA3) (transcription factor for Th2 differentiation and expression of IL-4, IL-13, and IL-5 by T lymphocytes) was also noted.
On the other hand, supernatants from S. aureus biofilms reduced the expression of TLR2, IL-18, and IL-23.
Interestingly, supernatant from mixed species (AA + SA) biofilms increased the expression of TSLP, CLDN1, and IL1α, while reducing the expression of TLR2.
These results suggested an additive effect of A. alternata and S. aureus on the production of AD-associated cytokines and structural proteins by keratinocytes.
Discussion
Studies over the past two decades have shown that alteration in the skin microbiome is one of the contributors to both AD pathogenesis (initializing and maintenance), AD flares, and AD severity (Faergemann, 2002; Sugita et al., 2010). Analysis of the skin microbiome in patients with AD demonstrated reduced diversity of the bacterial community and an increase in the abundance of S. aureus in the affected skin (Kong et al., 2012; Seite et al., 2014). Interestingly, this alteration was also reported to be associated with increasing disease severity, especially during disease flares (Kong et al., 2012). Unfortunately, most studies ignore the fungal community inhabiting our skin, although differences have been reported between patients with skin disorders and healthy individuals (Zhang et al., 2011; Jo et al., 2017; Han et al., 2018). Recent studies have shown evidence of the interaction between bacterial and fungal communities and how they can affect each other in health and disease (Peleg et al., 2010; Diaz et al., 2014; Deveau et al., 2018; Kruger et al., 2019). Thus, studies should not focus solely on bacterial populations but should also include the fungal community, known as the mycobiome.
Our data showed that the abundance of Staphylococcus was significantly increased in affected compared to unaffected skin, similar to the observations of other studies (Leyden et al., 1974; Aly et al., 1977; Bibel et al., 1977; Gloor et al., 1982; Higaki et al., 1999). This observation was in agreement with a meta-analysis, that included 81 studies, conducted by Totte et al. (2016) investigating the prevalence of S. aureus skin colonization in patients with AD. Furthermore, these data may explain the higher risk of AD-affected skin being impetiginized by S. aureus. Seventy percent of the patients included in the analysis carried S. aureus on the affected skin and 39% on the unaffected skin (Totte et al., 2016). In healthy individuals, S. aureus skin colonization was reported less frequently (Goh et al., 1997). S. aureus has been hypothesized to function as a superantigen in AD (Travers et al., 2010; Brauweiler et al., 2013, 2014; Giai et al., 2013; Nakamura et al., 2013; Travers, 2014; Williams and Gallo, 2015). S. aureus superantigens bind to major histocompatibility complex class II on keratinocytes causing increased production of pro-inflammatory cytokines including TNF-α and IL-1β secondary to non-specific activation of up to 20% of naïve T cells in the skin (Kim et al., 2006).
We analyzed the mycobiome composition in patients with AD compared to patients with adult-onset allergic contact dermatitis. Interestingly, the fungal genus Alternaria, a known fungus that has been linked to different allergic disorders including AD and asthma, exhibited the highest fold-increase in affected skin compared to unaffected skin exclusively in patients with a history of childhood-onset flexural dermatitis (patients with AD). Alternaria has also been associated with AD in other studies (Hedayati et al., 2009; Zhang et al., 2011; Han et al., 2018). Moreover, 16 A. alternata allergens have been linked to allergenic asthma and increased severity of asthma symptoms (Kustrzeba-Wojcicka et al., 2014; Sharpe et al., 2015; Gabriel et al., 2016).
Thus, we hypothesized that some fungal antigens may play a role in skin sensitization and subsequently share in the development of allergy. This concept is supported by the data provided by Strid et al. (2005) which showed that epicutaneous exposure of mice to peanut protein resulted in a potent Th2-type immunity. Moreover, high levels of IL-4 and serum IgE were also detected. Interestingly, an oral challenge with the same proteins induced a further stronger immune response.
In a study by Walker et al. (2018), Alternaria extracts were used for the induction of food allergy in neonatal mice heterozygous for the filaggrin mutation. Mice challenged with food allergens following skin sensitization with Alternaria extracts suffered from anaphylaxis compared to controls. Furthermore, skin barrier mutation was necessary for the induction of food allergy in mice's offspring. Filaggrin deficiency and sensitization with Alternaria enhance further sensitization to food, and potentially, to aeroallergens.
Buelow et al. (2021) have identified two pathways by which Alternaria can cause food allergic reactions using the same mouse model; an IL-33 pathway and a pathway involving oncostatin M (OSM) and amphiregulin (Areg), all of which have been reported to play a role in AD (Peng et al., 2018; Walker et al., 2018; Hashimoto et al., 2019; Scheffschick et al., 2019; Rojahn et al., 2020; Buelow et al., 2021). In the Buelow study, exposure to Alternaria increased the expression of IL-33, OSM, and Areg genes. In addition, these genes were expressed by some keratinocytes which were found in larger numbers in mice with mutations affecting the skin barrier compared to wild-type mice. In addition, the three genes were expressed by a variety of cells including cluster 6 keratinocytes which were found in larger numbers in mice with mutated skin barriers compared to the WT (Buelow et al., 2021). Moreover, increased levels of Areg in keratin-deficient keratinocytes were found to induce the production of TSLP through epidermal growth factor receptor signaling (Scheffschick et al., 2019).
Our data also showed that the supernatant from Alternaria or S. aureus biofilms alone slightly increased the TSLP message levels. On the other hand, supernatant from mixed species biofilms significantly increased the TSLP expression. This may suggest a synergistic interaction between the two species which supports our electron microscope and XTT assay observations.
Moreover, Alternaria biofilm supernatant was found to reduce expression of CLDN1 (a major component of epidermal tight junctions), which has been reported to be significantly reduced in affected AD skin compared to healthy controls, together with significant decreases in tight junction barrier of the skin in a dose-dependent manner (Bergmann et al., 2020). Additionally, in a CLDN1 knock-down (KD) in vitro 3D model of a reconstructed human epidermis that expressed different levels of CLDN1, intercellular lipid lamellae length was significantly reduced after CLDN1 KD at day 8, with a significant downregulation of filaggrin mRNA (a protein produced by keratinocytes which have been linked to several allergic diseases including AD) (Nomura et al., 2008; Thyssen and Kezic, 2014; Bergmann et al., 2020). Interestingly, downregulation of CLDN1 has also been reported to induce the production of pro-inflammatory cytokines as well as promote the inflammatory effects of non-pathogenic Staphylococci (Tokumasu et al., 2016; Bergmann et al., 2020). Despite our small cohort of patients, the observed trends mark points of departure for further exploration of the role of inter-kingdom interactions in the pathophysiology of AD.
The existence of inter-kingdom interactions between Alternaria and Staphylococcus was demonstrated by biofilm studies (Figure 7). The presence of biofilms likely explains the difficulty in eradicating S. aureus in patients with AD. The presence of bacteria-fungi mixed species biofilms may provide a protective niche to both, thereby affecting skin function and resulting in skin barrier disruption, pH imbalance, and sustained inflammation.
The strongest predictors of cutaneous sensitization are inflamed skin and a high concentration of antigen(s), and biofilms increase the concentration of organisms per unit area (Fluhr et al., 2008). All our dermatitis subjects had inflamed skin but only those with a history of childhood flexural dermatitis had a high abundance of Alternaria species. This may increase the risk of sensitization to Alternaria in the affected skin of patients with AD (Hernandez-Ramirez et al., 2021). Thus, increased concentrations of cutaneous Staphylococcus and Alternaria biofilm matrix may provide an opportunity for immune sensitization and subsequent Alternaria-triggered asthma (Saunders et al., 2012; Jinnestal et al., 2014). Such interactions in AD may exacerbate skin barrier defects and inflammatory lesions, and protect pathogens (in biofilms) from antimicrobial agents. Importantly, the mechanisms behind these interactions are not completely understood, and thus, further studies are warranted.
Our findings, taken in the context of prior literature, suggest that biofilms of Staphylococcus and Alternaria may bidirectionally worsen the skin barrier and promote allergic sensitization. A recent clinical trial has reported that the use of emollients did not show a significant impact in reducing the risk of AD in high-risk infants compared to controls (Chalmers et al., 2020). Furthermore, the use of emollients was associated with an increased risk of infection and increased incidence of food allergy in infants with increased frequency of application suggested to be caused through transcutaneous sensitization (Chalmers et al., 2020; Perkin et al., 2021). Therefore, emollients may not be beneficial for patients with dermatitis complicated by the presence of biofilms, and this has clinical implications for patient counseling. Biofilm is challenging to identify from a clinical point of view but maybe hypothesized from the fast recurrence of cutaneous infections and antibiotic lack of response. Thus, emollients may not be beneficial for patients with AD complicated by the presence of biofilms, although ceramide predominate moisturizers warrant further study (Elias, 2018).
Another potential treatment target in managing AD is to modulate the skin microbiome, first, to prevent the Staphylococcus/Alternaria interaction and, second, to increase the abundance of beneficial microbiota that has been reported to play a significant role in regulating skin barrier formation and repair via aryl hydrocarbon receptor in keratinocytes (Uberoi et al., 2021).
Going forward, a deeper understanding of the role of Staph/Alternaria biofilms in both dermatitis and downstream development of fungal-triggered asthma may lead to the development of interventions to disrupt these biofilms. Avoidance of occlusive emollients should be studied as a strategy to discourage biofilm formation. Patch testing for delayed type sensitization to Staph and Alternaria would also be useful to expedite treatment with a biological drug, as avoidance of Staph and Alternaria as contact allergens is not feasible. Currently, patch testing to an extended screening series with the addition of all contact allergens obtained from exposure history is needed to determine if avoidance therapy for contact dermatitis will suffice to cure dermatitis.
The generalizability of our findings is limited by a small sample size, which enhanced the interpersonal variability recorded in microbiome studies and is one of the primary reasons for non-significance in small sample size studies. Another caveat is the fact that all subjects were recruited from a referral-based specialty clinic introducing potential selection bias (patients whose dermatitis was more refractory to entry-level treatments and required referral to tertiary care). The small number of subjects prevented us from addressing the role of potential confounding factors including gender, skin sampling location, race, age, and use of topical corticosteroids or prior response to systemic or topical antibiotic treatments. Furthermore, although sequencing-based analysis is a more sensitive detector of S. aureus than culturing techniques, we cannot provide a threshold at which the relative abundance of S. aureus would translate to a positive clinical culture, which makes these data difficult to compare to prior studies performed using culturing techniques (Byrnes et al., 2010).
Despite these caveats, our study represents a novel resource in the investigation into the interactions between skin bacteriome and mycobiome in AD which may help to personalize therapy for the diverse cohort of patients with dermatitis.
Materials and methods
Ethical approval
This study was approved by the Institutional Review Board of University Hospitals Cleveland Medical Center (UHCMC) (IRB# # 05-95-03).
Study design
Thirteen study participants (age ≥ 18 years) were recruited from the UHCMC outpatient dermatology clinic by a physician for signs of barrier disruption (Supplementary Figure 4). Patients were divided into two cohorts based on dermatologic history: patients with childhood-onset, flexural dermatitis constituted atopic dermatitis (AD) group, and patients with adult-onset dermatitis with relevant positive patch test (non-Atopic, allergic contact dermatitis control group). The use of non-atopic controls as the comparator group allowed us to control for the microbial changes associated with broken, inflamed skin and identify microbiome changes uniquely associated with genetic differences evident in childhood, thus providing a more stringent control than could a cohort of healthy individuals with intact epidermis.
Table 1 shows the subject demographics: the mean age was 52.5 years with a range of 31–68 years. There were four women and nine men. The subjects with a history of childhood flexural dermatitis were slightly younger (mean age 47.3 years), compared to 56.0 years for the subjects without a history of childhood flexural dermatitis.
Each patient was evaluated by physician inspection for visible barrier disruption in the six eligible sites for swabbing: neck, anterior or posterior axillary line, axillary vault, volar wrist, antecubital fossa, and retro-auricular fold. We collected swabs from four to six of these potential sites per patient, depending on that patient's disease distribution, with the goal of collecting an equal number of affected and unaffected swabs per patient. Prior to swabbing, we categorized each site as either affected, if there was evidence of severe barrier disruption indicated by vesicles, papules, excoriations, or lichenification, or unaffected if the skin barrier was visibly intact.
Samples were collected using dry, sterile, cotton-tipped swabs, and rolled gently over the surface of the area of interest. Skin swabs were placed in tubes containing 500 μl glass beads (Sigma-Aldrich) and 1 ml ASL™ lysis buffer (Qiagen DNA Extraction Kit). Samples were stored at −80°C until processing. Additional details of the study design are provided in Supplementary methods. A total of 29 swabs were collected from affected skin and 29 from unaffected skin.
Microbiome analyses
Fungal and bacterial genomic DNA was isolated from the collected swabs using QiaAmp DNA Mini Kit (Qiagen) following the manufacturer's instructions, and their microbiome profile was determined using the Internally Transcribed Spacer 1 (ITS1) and 16S rDNA regions for fungi and bacteria, respectively, as described previously by our group (Mukherjee et al., 2014, 2017; Chandra et al., 2016). The Greengenes V13_8 database was used for the taxonomic identification of the 16S RNA and the UNITE (7.2) database was used for the taxonomic identification of the ITS. In brief, the ITS1 region was amplified using ITS1F (CTTGGTCATTTAGAGGAAGTAA) and ITS 2 (GCTGCGTTCTTCATCGATGC) primers, and the V4 region of the 16S rRNA gene was amplified using 16S-515F: GTGCCAGCMGCCGCGGTAA and 16s-806R: GGACTACHVGGGTWTCTAAT primers. PCR reactions were carried out on 100 ng template DNA, in a 50 μl (final volume) reaction mixture. PCR products were sheared for 20 min using Ion Shear Plus Fragment Library Kit (Life Technologies, NY, USA). Amplicon library was generated with sheared PCR products using Ion Plus Fragment Library kits (<350 bp) according to the manufacturer's instructions, barcoded with Ion Xpress™ Barcode Adapter, and ligated with adaptors.
Sequencing, classification, and analysis
The adapted barcoded libraries were equalized using the Ion Library Equalizer kit to a final concentration of 100 pM, pooled and diluted to 26 pM, and attached to the surface of Ion Sphere particles (ISPs) using an Ion PGM Template OT2 200bp kit v2 (Life Technologies, USA) according to the manufacturer's instructions, via emulsion PCR. The quality of ISPs' templates was checked using the Ion Sphere™ Quality Control Kit (Part no. 4468656) with the Qubit 2.0 device. Sequencing of the pooled libraries was carried out on the Ion Torrent Personal Genome Machine (PGM) system using the Ion Sequencing 200 kit v2 (all from Life Technologies) for 150 cycles (600 flows), with a 318-chip following the manufacturer's instructions. De-multiplexing and classification were performed using the Qiime 1.6 platform. The resulting sequence data were trimmed, reads with Phred score <Q25 and <100 bp were removed (Caporaso et al., 2010), and de novo operational taxonomic units (OTUs) were clustered using the Uclust algorithm (90% similarity threshold to represent the phyla, 94% similarity threshold to present the genus, and 97% sequence similarity representing the species) (Edgar, 2010). Classification at the species level was referenced using the UNITE 5.8s database for fungi and Greengenes V13_8 using the blastn function for bacteria. The sequences were assigned to the best BLAST hit with a minimum of 98.5% sequence homology (Altschul et al., 1990; Kõljalg et al., 2013). Principal coordinates analysis (PCoA), alpha/beta diversity, correlation analyses, and non-parametric analyses (since equal variance could not be assumed due to the small sample size) were conducted using the statistical programming language R and relevant packages (vegan, PMCMR, and corrplot) (Morgan and Huttenhower, 2012; Pohlert, 2014; Wei and Simko, 2016; Oksanen et al., 2017). Taxa with a frequency of at least 20% (and relative abundance >1%) were considered to comprise the core biome. P < 0.05 were considered significant.
Biofilm assessment
Single- or mixed-species biofilms were formed and quantitated as described previously (Chandra et al., 2008). The optical density of biofilms was calculated as described above using the XTT assay for metabolic activity (additional details are provided in Supplementary methods).
Real-time PCR assay
Primary keratinocytes were obtained from discarded neonatal foreskins (using an IRB-exempt protocol), grown to confluence, and exposed to 10% conditioned media obtained from cultured Staphylococcus aureus (SA) only, SA + Alternaria, Alternaria only, or media alone. Total RNA was extracted and analyzed for expression of targeted cytokines and regulatory genes using specific primers and the Superscript III reverse transcription kit (Life Technologies). Relative quantity (RQ) was plotted for each host variable and compared between the three groups using ANOVA and Tukey's post-hoc tests using GraphPad Prism (ver. 7.0). Additional details are provided in Supplementary methods.
Bioinformatics and statistical analyses
The statistical programming language R and related packages were used for diversity and correlation analyses, and Kruskal–Wallis (non-parametric) analysis of variance using abundance data (Morgan and Huttenhower, 2012). Principal coordinates analysis (PCoA) was conducted with identified reads/OTUs using classical multidimensional scaling (functions dist/Euclidean, cmdscale) to analyze the distribution of dissimilarities and hierarchical clustering in dendrograms in the R package vegan. The homogeneity of groups and beta diversity were evaluated using adonis (which explores the differences in group means and implements a multivariate analysis of variances using distance matrices) and betadisper (which studies the differences in group homogeneities, similar to Levene's test of the equality of variances) functions in vegan. Diversity was analyzed using the Shannon diversity index (characterizes species diversity) and abundance-based richness estimates (observed, bias-corrected Chao, and ACE) were calculated using vegan (Oksanen et al., 2017). Taxa with a frequency of at least 20% (and relative abundance >1%) were considered to comprise the core biome in our study. Intra- and inter-kingdom correlations were determined using the corrplot package in R. Proportions of specific bacteria and fungi in tested samples were compared using non-parametric tests (PMCMR package). Due to the small sample size, equal variance across data could not be assumed and data were analyzed using non-parametric tests followed by Dunn's test (Bonferroni post-hoc correction), as applicable. Spearman Rank correlation analyses were conducted to identify inter- and intra-kingdom associations and microbial interactions with AD-related host variables. P < 0.05 were considered significant.
Data availability statement
The original contributions presented in the study are included in the article/Supplementary material, further inquiries can be directed to the corresponding author/s.
Ethics statement
The studies involving human participants were reviewed and approved by Institutional Review Board of University Hospitals Cleveland Medical Center IRB# 05-95-03. The patients/participants provided their written informed consent to participate in this study.
Author contributions
Methodology and project conduction: MH. Conceptualization and project administration: PM. Writing—original draft: MH and AG. Writing—review and editing: MG, TM, SN, and GD. All authors contributed to the article and approved the submitted version.
Funding
Funding support is acknowledged from the National Eczema Association and Steris Foundation (to PM) and from the NIH (R01DE024228 to MG and PM), and the NIH-funded Skin Diseases Research Center (NIAMS P30 AR039750). Funders had no role in in study design; the collection, analysis and interpretation of data, in the writing of the report, or in the decision to submit the article for publication.
Conflict of interest
The authors declare that the research was conducted in the absence of any commercial or financial relationships that could be construed as a potential conflict of interest.
Publisher's note
All claims expressed in this article are solely those of the authors and do not necessarily represent those of their affiliated organizations, or those of the publisher, the editors and the reviewers. Any product that may be evaluated in this article, or claim that may be made by its manufacturer, is not guaranteed or endorsed by the publisher.
Supplementary material
The Supplementary Material for this article can be found online at: https://www.frontiersin.org/articles/10.3389/fmicb.2022.944365/full#supplementary-material
Supplementary Figure 1. Richness and diversity (Shannon index) of bacterial and fungal taxa in atopic and non-atopic patients. Diversity was analyzed using the Shannon diversity index (characterizes species diversity) and abundance-based richness estimates (observed, bias-corrected Chao, and ACE) were calculated using vegan. (A–D) Richness, (E–H) Shannon diversity index.
Supplementary Figure 2. The proportion of selected fungal genera in atopic and non-atopic skin.
Supplementary Figure 3. Effect of Staphylococcus on Alternaria biofilms. Scanning electron microscopy images of (A) Alternaria (AA), mag × 3,500, (B) Staphylococcus (SA), mag × 3,500, (C) Alternaria + Staphylococcus (AA + SA), mag × 3,500, (D) Alternaria + Staphylococcus (AA + SA), mag × 10,000. (E) Biofilm formation of AA (measured by absorbance of metabolic activity) in presence of increasing densities of SA cells. EPS, extracellular polysaccharide matrix.
Supplementary Figure 4. Graphic illustration of the study design.
References
Altschul, S. F., Gish, W., Miller, W., Myers, E. W., and Lipman, D. J. (1990). Basic local alignment search tool. J. Mol. Biol. 215, 403–410. doi: 10.1016/S0022-2836(05)80360-2
Aly, R., Maibach, H. I., and Shinefield, H. R. (1977). Microbial flora of atopic dermatitis. Arch. Dermatol. 113, 780–782. doi: 10.1001/archderm.1977.01640060076008
Bergmann, S., von Buenau, B., Vidal, Y. S. S., Haftek, M., Wladykowski, E., Houdek, P., et al. (2020). Claudin-1 decrease impacts epidermal barrier function in atopic dermatitis lesions dose-dependently. Sci. Rep. 10, 2024. doi: 10.1038/s41598-020-58718-9
Bibel, D. J., Greenberg, J. H., and Cook, J. L. (1977). Staphylococcus aureus and the microbial ecology of atopic dermatitis. Can. J. Microbiol. 23, 1062–1068. doi: 10.1139/m77-159
Brauweiler, A. M., Bin, L., Kim, B. E., Oyoshi, M. K., Geha, R. S., Goleva, E., et al. (2013). Filaggrin-dependent secretion of sphingomyelinase protects against staphylococcal alpha-toxin-induced keratinocyte death. J Allergy Clin Immunol 131, 421–427.e1–2. doi: 10.1016/j.jaci.2012.10.030
Brauweiler, A. M., Goleva, E., and Leung, D. Y. M. (2014). Th2 cytokines increase Staphylococcus aureus alpha toxin-induced keratinocyte death through the signal transducer and activator of transcription 6 (STAT6). J. Invest. Dermatol. 134, 2114–2121. doi: 10.1038/jid.2014.43
Buelow, L. M., Hoji, A., Tat, K., Schroeder-Carter, L. M., Carroll, D. J., Cook-Mills, J. M., et al. (2021). Mechanisms for Alternaria alternata function in the skin during induction of peanut allergy in neonatal mice with skin barrier. Mutations. 2, e677019. doi: 10.3389/falgy.2021.677019
Byrnes, M. C., Adegboyega, T., Riggle, A., Chipman, J., Beilman, G., Reicks, P., et al. (2010). Nasal swabs collected routinely to screen for colonization by methicillin-resistant Staphylococcus aureus in intensive care units are a sensitive screening test for the organism in clinical cultures. Surg Infect (Larchmt) 11, 511–515. doi: 10.1089/sur.2010.033
Caporaso, J. G., Kuczynski, J., Stombaugh, J., Bittinger, K., Bushman, F. D., Costello, E. K., et al. (2010). QIIME allows analysis of high-throughput community sequencing data. Nat. Methods 7, 335–336. doi: 10.1038/nmeth.f.303
Celakovska, J., Ettlerova, K., Ettler, K., Vaneckova, J., and Bukac, J. (2015). Sensitization to aeroallergens in atopic dermatitis patients: association with concomitant allergic diseases. J. Eur. Acad. Dermatol. Venereol. 29, 1500–1505. doi: 10.1111/jdv.12891
Chalmers, J. R., Haines, R. H., Bradshaw, L. E., Montgomery, A. A., Thomas, K. S., Brown, S. J., et al. (2020). Daily emollient during infancy for prevention of eczema: the BEEP randomised controlled trial. Lancet 395, 962–972. doi: 10.1016/S0140-6736(19)32984-8
Chandra, J., Mukherjee, P. K., and Ghannoum, M. A. (2008). In vitro growth and analysis of Candida biofilms. Nat. Protoc. 3, 1909–1924. doi: 10.1038/nprot.2008.192
Chandra, J., Retuerto, M., Mukherjee, P. K., and Ghannoum, M. (2016). The fungal biome of the oral cavity. Methods Mol. Biol. 1356, 107–135. doi: 10.1007/978-1-4939-3052-4_9
Deveau, A., Bonito, G., Uehling, J., Paoletti, M., Becker, M., Bindschedler, S., et al. (2018). Bacterial-fungal interactions: ecology, mechanisms and challenges. FEMS Microbiol. Rev. 42, 335–352. doi: 10.1093/femsre/fuy008
Diaz, P. I., Strausbaugh, L. D., and Dongari-Bagtzoglou, A. (2014). Fungal-bacterial interactions and their relevance to oral health: linking the clinic and the bench. Front. Cell. Infect. Microbiol. 4, 101. doi: 10.3389/fcimb.2014.00101
Edgar, R. C. (2010). Search and clustering orders of magnitude faster than BLAST. Bioinformatics 26, 2460–2461. doi: 10.1093/bioinformatics/btq461
Elias, P. M. (2018). Primary role of barrier dysfunction in the pathogenesis of atopic dermatitis. Exp. Dermatol. 27, 847–851. doi: 10.1111/exd.13693
Faergemann, J. (2002). Atopic dermatitis and fungi. Clin. Microbiol. Rev. 15, 545–563. doi: 10.1128/CMR.15.4.545-563.2002
Fluhr, J. W., Darlenski, R., Angelova-Fischer, I., Tsankov, N., and Basketter, D. (2008). Skin irritation and sensitization: mechanisms and new approaches for risk assessment. 1. Skin irritation. Skin Pharmacol. Physiol. 21, 124–135. doi: 10.1159/000131077
Fukutomi, Y., and Taniguchi, M. (2015). Sensitization to fungal allergens: resolved and unresolved issues. Allergol. Int. 64, 321–331. doi: 10.1016/j.alit.2015.05.007
Gabriel, M. F., Postigo, I., Tomaz, C. T., and Martinez, J. (2016). Alternaria alternata allergens: markers of exposure, phylogeny and risk of fungi-induced respiratory allergy. Environ Int. 89–90, 71–80. doi: 10.1016/j.envint.2016.01.003
Giai, C., Gonzalez, C., Ledo, C., Garofalo, A., Genaro, M. S. D. i., et al. (2013). Shedding of tumor necrosis factor receptor 1 induced by protein A decreases tumor necrosis factor alpha availability and inflammation during systemic Staphylococcus aureus infection. Infect. Immun. 81, 4200–4207. doi: 10.1128/IAI.00593-13
Gloor, M., Peters, G., and Stoika, D. (1982). On the resident aerobic bacterial skin flora in unaffected skin of patients with atopic dermatitis and in healthy controls. Dermatologica 164, 258–265. doi: 10.1159/000250099
Goh, C. L., Wong, J. S., and Giam, Y. C. (1997). Skin colonization of Staphylococcus aureus in atopic dermatitis patients seen at the National Skin Centre, Singapore. Int. J. Dermatol. 36, 653–657. doi: 10.1046/j.1365-4362.1997.00290.x
Han, S. H., Cheon, H. I., Hur, M. S., Kim, M. J., Jung, W. H., Lee, Y. W., et al. (2018). Analysis of the skin mycobiome in adult patients with atopic dermatitis. Exp. Dermatol. 27, 366–373. doi: 10.1111/exd.13500
Hashimoto, T., Satoh, T., Karasuyama, H., and Yokozeki, H. (2019). Amphiregulin from basophils amplifies basophil-mediated chronic skin inflammation. J. Invest. Dermatol. 139, 1834–1837.e2. doi: 10.1016/j.jid.2019.02.023
Hedayati, M. T., Arabzadehmoghadam, A., and Hajheydari, Z. (2009). Specific IgE against Alternaria alternata in atopic dermatitis and asthma patients. Eur. Rev. Med. Pharmacol. Sci. 13, 187–191.
Hernandez-Ramirez, G., Barber, D., Tome-Amat, J., Garrido-Arandia, M., and Diaz-Perales, A. (2021). Alternaria as an inducer of allergic sensitization. J. Fungi 7:838. doi: 10.3390/jof7100838
Higaki, S., Morohashi, M., Yamagishi, T., and Hasegawa, Y. (1999). Comparative study of staphylococci from the skin of atopic dermatitis patients and from healthy subjects. Int. J. Dermatol. 38, 265–269. doi: 10.1046/j.1365-4362.1999.00686.x
Jinnestal, C. L., Belfrage, E., Back, O., Schmidtchen, A., and Sonesson, A. (2014). Skin barrier impairment correlates with cutaneous Staphylococcus aureus colonization and sensitization to skin-associated microbial antigens in adult patients with atopic dermatitis. Int. J. Dermatol. 53, 27–33. doi: 10.1111/ijd.12198
Jo, J. H., Kennedy, E. A., and Kong, H. H. (2017). Topographical and physiological differences of the skin mycobiome in health and disease. Virulence 8, 324–333. doi: 10.1080/21505594.2016.1249093
Kim, K. H., Han, J. H., Chung, J. H., Cho, K. H., and Eun, H. C. (2006). Role of staphylococcal superantigen in atopic dermatitis: influence on keratinocytes. J. Korean Med. Sci. 21, 315–323. doi: 10.3346/jkms.2006.21.2.315
Knutsen, A. P., Bush, R. K., Demain, J. G., Denning, D. W., Dixit, A., Fairs, A., et al. (2012). Fungi and allergic lower respiratory tract diseases. J. Allergy Clin. Immunol. 129, 280–291, quiz 292–293. doi: 10.1016/j.jaci.2011.12.970
Kohli, N., and Nedorost, S. (2016). Inflamed skin predisposes to sensitization to less potent allergens. J. Am. Acad. Dermatol. 75, 312–317.e1. doi: 10.1016/j.jaad.2016.03.010
Kõljalg, U., Nilsson, R. H., Abarenkov, K., Tedersoo, L., Taylor, A. F., Bahram, M., et al. (2013). Towards a unified paradigm for sequence-based identification of fungi. Mol. Ecol. 22, 5271–5277. doi: 10.1111/mec.12481
Komine, M. (2009). Analysis of the mechanism for the development of allergic skin inflammation and the application for its treatment:keratinocytes in atopic dermatitis—their pathogenic involvement. J. Pharmacol. Sci. 110, 260–264. doi: 10.1254/jphs.09R06FM
Kong, H. H., Oh, J., Deming, C., Conlan, S., Grice, E. A., Beatson, M. A., et al. (2012). Temporal shifts in the skin microbiome associated with disease flares and treatment in children with atopic dermatitis. Genome Res. 22, 850–859. doi: 10.1101/gr.131029.111
Kruger, W., Vielreicher, S., Kapitan, M., Jacobsen, I. D., and Niemiec, M. J. (2019). Fungal-bacterial interactions in health and disease. Pathogens 8, 70. doi: 10.3390/pathogens8020070
Kustrzeba-Wojcicka, I., Siwak, E., Terlecki, G., Wolanczyk-Medrala, A., and Medrala, W. (2014). Alternaria alternata and its allergens: a comprehensive review. Clin. Rev. Allergy Immunol. 47, 354–365. doi: 10.1007/s12016-014-8447-6
Lee, H., Ryu, W. I., Kim, H. J., Bae, H. C., Ryu, H. J., Shin, J. J., et al. (2016). TSLP down-regulates S100A7 and ss-defensin 2 via the JAK2/STAT3-dependent mechanism. J. Invest. Dermatol. 136, 2427–2435. doi: 10.1016/j.jid.2016.07.027
Leyden, J. J., Marples, R. R., and Kligman, A. M. (1974). Staphylococcus aureus in the lesions of atopic dermatitis. Br. J. Dermatol. 90, 525–530. doi: 10.1111/j.1365-2133.1974.tb06447.x
Li, J., Leyva-Castillo, J. M., Hener, P., Eisenmann, A., Zaafouri, S., Jonca, N., et al. (2016). Counterregulation between thymic stromal lymphopoietin- and IL-23-driven immune axes shapes skin inflammation in mice with epidermal barrier defects. J. Allergy Clin. Immunol. 138, 150–161.e13. doi: 10.1016/j.jaci.2016.01.013
Morgan, X. C., and Huttenhower, C. (2012). Chapter 12: Human microbiome analysis. PLoS Comput. Biol. 8, e1002808. doi: 10.1371/journal.pcbi.1002808
Mukherjee, P. K., Chandra, J., Retuerto, M., Arters, K. A., Consolo, M. C., Bajaksouzian, S., et al. (2017). Effect of alcohol-based hand rub on hand microbiome and hand skin health in hospitalized adult stem cell transplant patients: a pilot study. J. Am. Acad. Dermatol. 17, 32745–32747. doi: 10.1016/j.jaad.2017.11.046
Mukherjee, P. K., Chandra, J., Retuerto, M., Sikaroodi, M., Brown, R. E., Jurevic, R. J., et al. (2014). Oral mycobiome analysis of HIV-infected patients: identification of pichia as an antagonist of opportunistic fungi. PLoS Pathog. 10, e1003996. doi: 10.1371/journal.ppat.1003996
Nakamura, Y., Oscherwitz, J., Cease, K. B., Chan, S. M., Munoz-Planillo, R., Hasegawa, M., et al. (2013). Staphylococcus delta-toxin induces allergic skin disease by activating mast cells. Nature 503, 397–401. doi: 10.1038/nature12655
Nedorost, S. T. (2020). Allergic contact sensitization in healthy skin differs from sensitization in chronic dermatitis: atopic, occupational wet work, and stasis dermatitis. Dermatol. Clin. 38, 301–308. doi: 10.1016/j.det.2020.02.006
Newell, L., Polak, M. E., Perera, J., Owen, C., Boyd, P., Pickard, C., et al. (2013). Sensitization via healthy skin programs Th2 responses in individuals with atopic dermatitis. J. Invest. Dermatol. 133, 2372–2380. doi: 10.1038/jid.2013.148
Nomura, T., Akiyama, M., Sandilands, A., Nemoto-Hasebe, I., Sakai, K., Nagasaki, A., et al. (2008). Specific filaggrin mutations cause ichthyosis vulgaris and are significantly associated with atopic dermatitis in Japan. J. Invest. Dermatol. 128, 1436–1441. doi: 10.1038/sj.jid.5701205
Oksanen, J., Blanchet, F. G., Friendly, M., Kindt, R., Legendre, P., McGlinn, D., et al. (2017). vegan: Community Ecology Package. R Package version 2, 321–326. Available online at: https://cran.r-project.org/web/packages/vegan/vegan.pdf
Peleg, A. Y., Hogan, D. A., and Mylonakis, E. (2010). Medically important bacterial-fungal interactions. Nat. Rev. Microbiol. 8, 340–349. doi: 10.1038/nrmicro2313
Peng, G., Mu, Z., Cui, L., Liu, P., Wang, Y., Wu, W., et al. (2018). Anti-IL-33 antibody has a therapeutic effect in an atopic dermatitis murine model induced by 2, 4-dinitrochlorobenzene. Inflammation 41, 154–163. doi: 10.1007/s10753-017-0673-7
Perkin, M. R., Logan, K., Marrs, T., Radulovic, S., Craven, J., Boyle, R. J., et al. (2021). Association of frequent moisturizer use in early infancy with the development of food allergy. J. Allergy Clin. Immunol. 147, 967–976.e1. doi: 10.1016/j.jaci.2020.10.044
Ramirez De Knott, H. M., McCormick, T. S., Do, S. O., Goodman, W., Ghannoum, M. A., Cooper, K. D., et al. (2005). Cutaneous hypersensitivity to Candida albicans in idiopathic vulvodynia. Contact Derm. 53, 214–218. doi: 10.1111/j.0105-1873.2005.00685.x
Ramirez De Knott, H. M., Mccormick, T. S., Kalka, K., Skandamis, G., Ghannoum, M. A., Schluchter, M., et al. (2006). Cutaneous hypersensitivity to Malassezia sympodialis and dust mite in adult atopic dermatitis with a textile pattern. Contact Derm. 54, 92–99. doi: 10.1111/j.0105-1873.2006.00774.x
Rojahn, T. B., Vorstandlechner, V., Krausgruber, T., Bauer, W. M., Alkon, N., Bangert, C., et al. (2020). Single-cell transcriptomics combined with interstitial fluid proteomics defines cell type-specific immune regulation in atopic dermatitis. J. Allergy Clin. Immunol. 146, 1056–1069. doi: 10.1016/j.jaci.2020.03.041
Saunders, C. W., Scheynius, A., and Heitman, J. (2012). Malassezia fungi are specialized to live on skin and associated with dandruff, eczema, and other skin diseases. PLoS Pathog. 8, e1002701. doi: 10.1371/journal.ppat.1002701
Scheffschick, A., Kiritsi, D., and Magin, T. M. (2019). Keratin defects trigger the itch-inducing cytokine thymic stromal lymphopoietin through amphiregulin-epidermal growth factor receptor signaling. J. Allergy Clin. Immunol.144, 1719–1722.e3. doi: 10.1016/j.jaci.2019.07.041
Seite, S., Flores, G. E., Henley, J. B., Martin, R., Zelenkova, H., Aguilar, L., et al. (2014). Microbiome of affected and unaffected skin of patients with atopic dermatitis before and after emollient treatment. J. Drugs Dermatol. 13, 1365–1372. Available online at: https://jddonline.com/articles/microbiome-of-affected-and-unaffected-skin-of-patients-with-atopic-dermatitis-before-and-after-emoll-S1545961614P1365X/
Sharpe, R. A., Thornton, C. R., Tyrrell, J., Nikolaou, V., and Osborne, N. J. (2015). Variable risk of atopic disease due to indoor fungal exposure in NHANES 2005–2006. Clin. Exp. Allergy 45, 1566–1578. doi: 10.1111/cea.12549
Strid, J., Hourihane, J., Kimber, I., Callard, R., and Strobel, S. (2005). Epicutaneous exposure to peanut protein prevents oral tolerance and enhances allergic sensitization. Clin. Exp. Allergy 35, 757–766. doi: 10.1111/j.1365-2222.2005.02260.x
Sugita, T., Boekhout, T., Velegraki, A., Guillot, J., Hadina, S., Cabañes, F. J., et al. (2010). “Epidemiology of malassezia-related skin diseases,” in Malassezia and the Skin: Science and Clinical Practice, eds T. Boekhout, P. Mayser, E. Guého-Kellermann, A. Velegraki (Berlin, Heidelberg: Springer), 65–119. doi: 10.1007/978-3-642-03616-3_3
Thestrup-Pedersen, K. (2000). Clinical aspects of atopic dermatitis. Clin. Exp. Dermatol. 25, 535–543. doi: 10.1046/j.1365-2230.2000.00696.x
Thyssen, J. P., and Kezic, S. (2014). Causes of epidermal filaggrin reduction and their role in the pathogenesis of atopic dermatitis. J. Allergy Clin. Immunol. 134, 792–799. doi: 10.1016/j.jaci.2014.06.014
Tokumasu, R., Yamaga, K., Yamazaki, Y., Murota, H., Suzuki, K., Tamura, A., et al. (2016). Dose-dependent role of claudin-1 in vivo in orchestrating features of atopic dermatitis. Proc. Natl. Acad. Sci. USA. 113, E4061–E4068. doi: 10.1073/pnas.1525474113
Totte, J. E., van der Feltz, W. T., Hennekam, M., van Belkum, A., van Zuuren, E. J., Pasmans, S. G., et al. (2016). Prevalence and odds of Staphylococcus aureus carriage in atopic dermatitis: a systematic review and meta-analysis. Br. J. Dermatol. 175, 687–695. doi: 10.1111/bjd.14566
Travers, J. B. (2014). Toxic interaction between Th2 cytokines and Staphylococcus aureus in atopic dermatitis. J. Invest. Dermatol. 134, 2069–2071. doi: 10.1038/jid.2014.122
Travers, J. B., Kozman, A., Mousdicas, N., Saha, C., Landis, M., Al-Hassani, M., et al. (2010). Infected atopic dermatitis lesions contain pharmacologic amounts of lipoteichoic acid. J. Allergy Clin. Immunol. 125, 146–152.e1–2. doi: 10.1016/j.jaci.2009.09.052
Uberoi, A., Bartow-McKenney, C., Zheng, Q., Flowers, L., Campbell, A., Knight, S. A., et al. (2021). Commensal microbiota regulates skin barrier function and repair via signaling through the aryl hydrocarbon receptor. Cell Host Microbe 29, 1235–1248.e8. doi: 10.1016/j.chom.2021.05.011
Walker, M. T., Green, J. E., Ferrie, R. P., Queener, A. M., Kaplan, M. H., Cook-Mills, J. M., et al. (2018). Mechanism for initiation of food allergy: dependence on skin barrier mutations and environmental allergen costimulation. J. Allergy Clin. Immunol. 141, 1711–1725.e9. doi: 10.1016/j.jaci.2018.02.003
Wei, T., and Simko, V. (2016). corrplot: Visualization of a Correlation Matrix. R package version 0.77.
Werfel, T., Allam, J. P., Biedermann, T., Eyerich, K., Gilles, S., Guttman-Yassky, E., et al. (2016). Cellular and molecular immunologic mechanisms in patients with atopic dermatitis. J. Allergy Clin. Immunol. 138, 336–349. doi: 10.1016/j.jaci.2016.06.010
Williams, M. R., and Gallo, R. L. (2015). The role of the skin microbiome in atopic dermatitis. Curr. Allergy Asthma Rep. 15, 65. doi: 10.1007/s11882-015-0567-4
Keywords: atopic dermatitis, microbiome, cutaneous dysbiosis, Alternaria alternata, biofilm
Citation: Hammond M, Gamal A, Mukherjee PK, Damiani G, McCormick TS, Ghannoum MA and Nedorost S (2022) Cutaneous dysbiosis may amplify barrier dysfunction in patients with atopic dermatitis. Front. Microbiol. 13:944365. doi: 10.3389/fmicb.2022.944365
Received: 15 May 2022; Accepted: 13 October 2022;
Published: 14 November 2022.
Edited by:
Arun K. Bhunia, Purdue University, United StatesReviewed by:
Regina Fölster-Holst, University Medical Center Schleswig-Holstein, GermanyHelena Vidaurri De La Cruz, General Hospital of Mexico, Mexico
Copyright © 2022 Hammond, Gamal, Mukherjee, Damiani, McCormick, Ghannoum and Nedorost. This is an open-access article distributed under the terms of the Creative Commons Attribution License (CC BY). The use, distribution or reproduction in other forums is permitted, provided the original author(s) and the copyright owner(s) are credited and that the original publication in this journal is cited, in accordance with accepted academic practice. No use, distribution or reproduction is permitted which does not comply with these terms.
*Correspondence: Margaret Hammond, bWliMTlAY2FzZS5lZHU=