- 1Department of Orthopedic Surgery, West China Hospital, Sichuan University, Chengdu, China
- 2West China School of Public Health, Sichuan University, Chengdu, China
- 3Boston University Henry M. Goldman School of Dental Medicine, Boston, MA, United States
- 4West China Hospital of Stomatology, Sichuan University, Chengdu, China
Bone infection results in a complex inflammatory response and bone destruction. A broad spectrum of bacterial species has been involved for jaw osteomyelitis, hematogenous osteomyelitis, vertebral osteomyelitis or diabetes mellitus, such as Staphylococcus aureus (S. aureus), coagulase-negative Staphylococcus species, and aerobic gram-negative bacilli. S. aureus is the major pathogenic bacterium for osteomyelitis, which results in a complex inflammatory response and bone destruction. Although various antibiotics have been applied for bone infection, the emergence of drug resistance and biofilm formation significantly decrease the effectiveness of those agents. In combination with gram-positive aerobes, gram-negative aerobes and anaerobes functionally equivalent pathogroups interact synergistically, developing as pathogenic biofilms and causing recurrent infections. The adhesion of biofilms to bone promotes bone destruction and protects bacteria from antimicrobial agent stress and host immune system infiltration. Moreover, bone is characterized by low permeability and reduced blood flow, further hindering the therapeutic effect for bone infections. To minimize systemic toxicity and enhance antibacterial effectiveness, therapeutic strategies targeting on biofilm and bone infection can serve as a promising modality. Herein, we focus on biofilm and bone infection eradication with targeting therapeutic strategies. We summarize recent targeting moieties on biofilm and bone infection with peptide-, nucleic acid-, bacteriophage-, CaP- and turnover homeostasis-based strategies. The antibacterial and antibiofilm mechanisms of those therapeutic strategies include increasing antibacterial agents’ accumulation by bone specific affinity, specific recognition of phage-bacteria, inhibition biofilm formation in transcription level. As chronic inflammation induced by infection can trigger osteoclast activation and inhibit osteoblast functioning, we additionally expand the potential applications of turnover homeostasis-based therapeutic strategies on biofilm or infection related immunity homeostasis for host-bacteria. Based on this review, we expect to provide useful insights of targeting therapeutic efficacy for biofilm and bone infection eradication.
Introduction
Bone infection is an inflammatory process characterized by microorganism invasion, which results in localized bone loss and destruction (Lew and Waldvogel, 2004; Chen et al., 2021). A broad spectrum of bacterial species has been isolated in cases of bone infection diseases. Although various antibiotics have been applied for bone infection, the emergence of drug resistance and biofilm formation significantly decrease the effectiveness of those agents. Biofilms are matrices comprising polysaccharides, proteins, extracellular DNA (eDNA) and host components that are encased within bacterial communities (Hall-Stoodley et al., 2004; Lei et al., 2021). The adhesion of biofilms to bone promotes bone destruction and protects bacteria from antimicrobial agent stress and host immune system infiltration (Sanchez et al., 2013). In jaw osteomyelitis and periodontitis, multiple species, including species of Actinomyces, Fusobacterium, Parvimonas, Tannerella, Porphyromonas, and Staphylococcus, are synergistically involved (Gaetti-Jardim et al., 2010; Settem et al., 2012; Chen et al., 2021). Moreover, several species have been identified in bone infections secondary to trauma, surgery or insertion of a joint prosthesis, including Staphylococcus aureus, coagulase-negative Staphylococcus species, and aerobic gram-negative bacilli (Pseudomonas species, Enterobacter species and Escherichia coli). Those particularly isolated in polymicrobial infections include anaerobes (such as Bacteroides gracilis, Propionibacterium acnes, Fusobacterium nucleatum, Prevotella buccae, and Eubacterium lentum), and those particularly isolated in traumatic dental injuries include species of Actinomyces, Candida, and Sporothrix (Lew and Waldvogel, 2004; Schmitt, 2017; Fouad, 2019). Hematogenous osteomyelitis (AHO) is usually monomicrobial. S. aureus is a predominant bacterium in AHO and can also combine with other microorganisms, including Streptococcus, Salmonella, Enterobacter cloacae, Pseudomonas aeruginosa, and Bartonella henselae (McNeil et al., 2016; Schmitt, 2017; McNeil, 2020). Microorganisms in blood are in slow flow, particularly at the vascular loops. Metaphysis near the epiphyseal plates distributed with vascular loops facilitates the deposition of microbes (Calhoun et al., 2009). Vertebral osteomyelitis is also commonly induced by the hematogenous deposition of microbes in vertebral bodies (Maamari et al., 2022). S. aureus is the most common pathogen in the setting of vertebral osteomyelitis, which is particularly assumed to be associated with S. aureus bacteremia (Berbari et al., 2015). Alternative pathogens include Escherichia coli, Mycobacterium tuberculosis, Brucella, Propionibacterium acnes, Candida, and Aspergillus, which are involved in spinal surgery, immunocompromised patients and intravenous catheters (Berbari et al., 2015). Diabetes mellitus, as a worldwide public health threat, leads to significant bone and soft-tissue ischemia, peripheral neuropathy and immunocompromise. All these pathological changes subsequently result in skin ulceration and diabetic foot infections (Jneid et al., 2017). By microbiota analysis, diabetic cutaneous tissue presents an increase in S. aureus compared with non-diabetic cutaneous tissue (Redel et al., 2013). A decrease in bacterial diversity and an increase in opportunistic pathogens have been identified in infective diabetic cutaneous tissue compared with contralateral intact skin (Gontcharova et al., 2010; Dunyach-Remy et al., 2016). In combination with gram-positive aerobes (Staphylococcus epidermidis, Streptococcus pneumoniae, Enterococcus faecalis, etc.), gram-negative aerobes (Pseudomonas aeruginosa, Enterobacteriaceae, etc.), and anaerobes (Peptostreptococcus spp., Bacteroides spp., Prevotella spp., etc.), functionally equivalent pathogroups interact synergistically, developing as pathogenic biofilms and causing recurrent diabetic foot infections (Claros et al., 2013; Lipsky et al., 2013; Jneid et al., 2017). The above etiologies of microbial distribution and pathological mechanism are mapped in Figure 1.
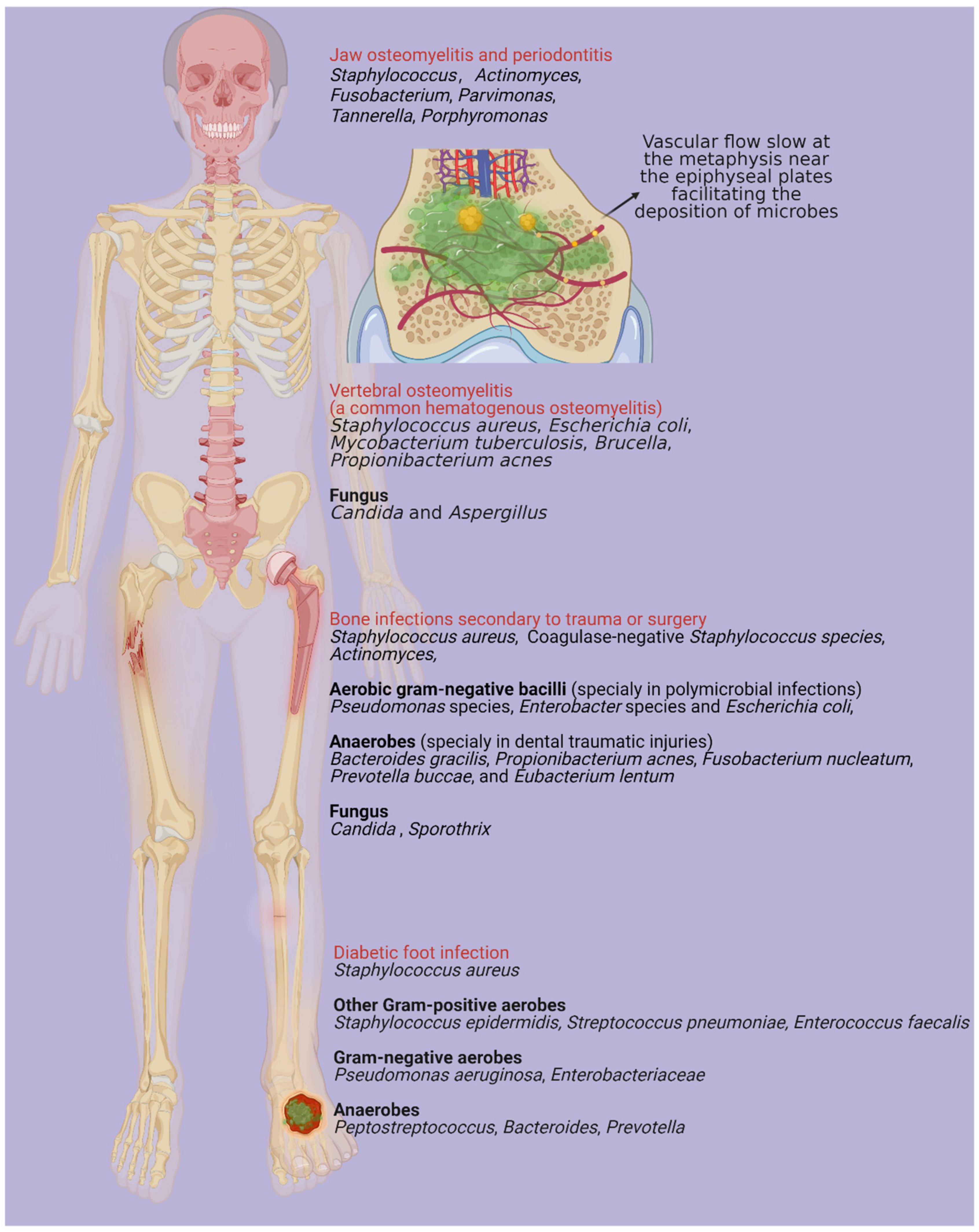
Figure 1. There are various microorganisms associated with different types of bone infections, mainly including jaw osteomyelitis, periodontitis, bone infections secondary to trauma or surgery, diabetic foot infection and hematogenous osteomyelitis (Lew and Waldvogel, 2004). Vascular flow slows at the metaphysis near the epiphyseal plates facilitating the deposition of microbes and vertebral osteomyelitis is one of most common types in hematogenous osteomyelitis (Maamari et al., 2022). Created with BioRender.com.
S. aureus is the most prevalent bacterial species that contributes to bone infections (Wright and Nair, 2010). Many mechanisms have been applied to enhance S. aureus resistance (Figure 2). Bone, as a highly mineralized tissue, is characterized by low permeability and reduced blood flow, further increasing the difficulty of treatment for bone infections (Shi et al., 2020). Notably, there are a group of clones derived from mutations in the electron transport pathway that impair the growth of bacterial cells, resulting in cells that are smaller in size. These so-called small colony variants (SCVs) particularly adapt to invading and persisting within host cells (Proctor et al., 2006; Proctor, 2019). This internalized S. aureus can survive in a dormant state, enabling bacterial escape from antimicrobials and host immune responses. Taken together, SCV cells do not respond to clinical treatment effectively and are related to latent or recurrent infections, representing a troublesome problem in the management of Staphylococcal bone disease (Proctor et al., 2006). As conservative antibiotics should penetrate through the rigid bone structure, biofilm and even host cells to maintain a local sufficient therapeutic concentration, bone infection treatment can be challenging. To improve therapeutic effects and minimize systematic toxicity, infective bone locus-targeted management with high efficacy on biofilm bacteria could serve as a promising modality (Zimmerli and Sendi, 2017). Herein, we summarize recent targeting moieties on biofilm and bone infection with peptide-, nucleic acid-, bacteriophage-, CaP- and turnover homeostasis-based strategies.
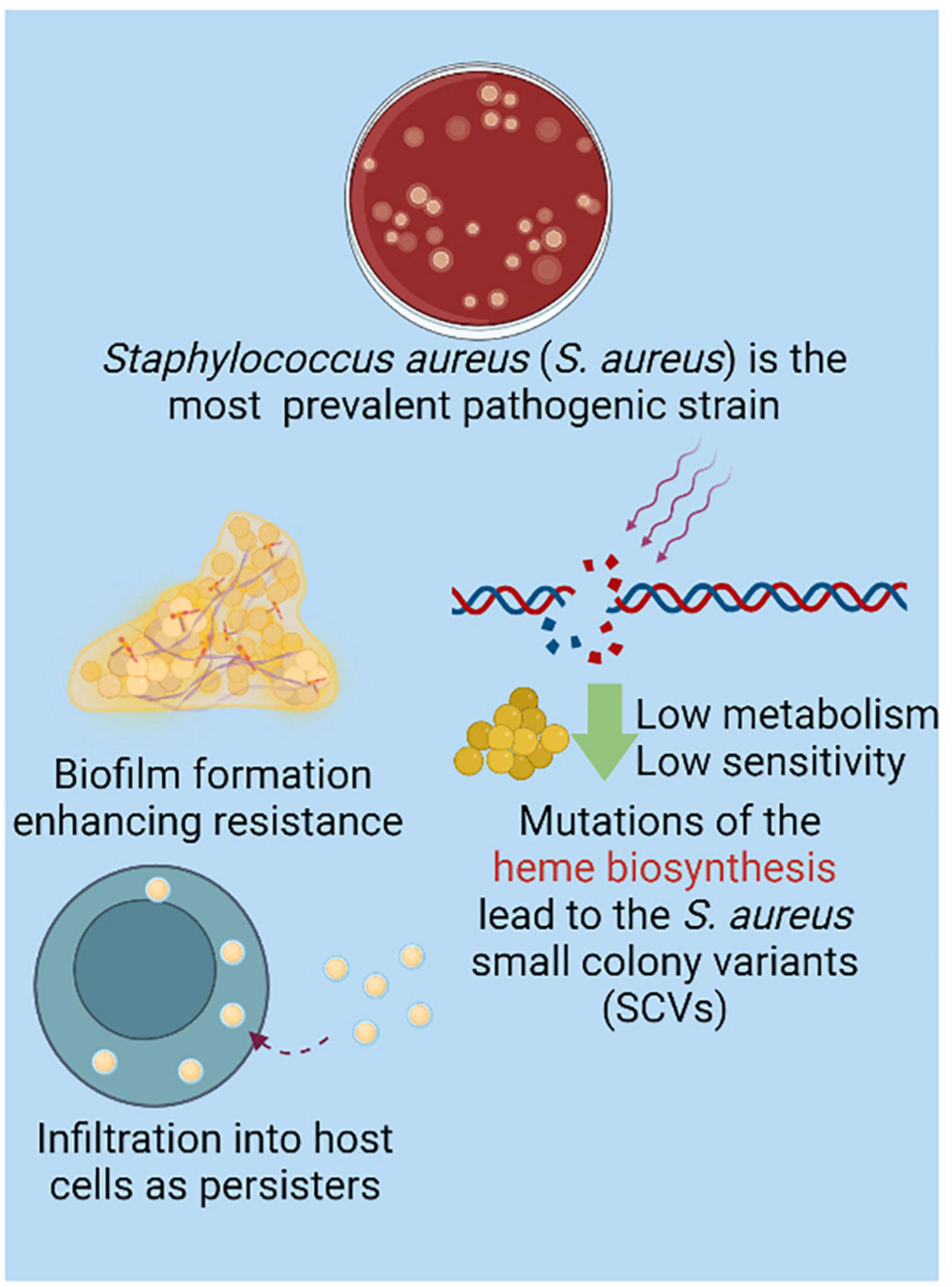
Figure 2. Although species contribute to bone infection is in diversity, Staphylococcus aureus (S. aureus) is the most prevalent isolated strain (Wright and Nair, 2010). The biofilm formation significantly enhances the resistance of strains (Sanchez et al., 2013). With mutation of heme biosynthesis, small colony variant of S. aureus (SCV) is charactered by low metabolism which resulted in low sensitivity to the conventional antibacterial strategy. Interestingly, the intracellular SCV in host cells are causing the recurrent and refractory infective locus as persisters, which further provide the resistance of S. aureus (Proctor, 2019). Created with BioRender.com.
Peptide-Based Bone-Targeted Therapeutic Strategy Negatively Regulate Biofilm Development and Maintenance
Bone is uniquely composed of the inorganic compound hydroxyapatite (HA). Therefore, HA could be a promising target for bone-targeting drug designs. Bone matrix proteins, such as osteopontin, the most abundant non-collagenous protein in bone, are observed to strongly bind to Ca2+ and the mineral surfaces of bone. Several bone proteins exhibit repetitive sequences of acidic amino acids (aspartate, Asp or glutamic acid, Glu) (Takahashi-Nishioka et al., 2008). These moieties can complement or chelate with calcium ions in the spatial HA orientation, resulting in a strong affinity to the bone (Wang et al., 2005). Thus, acidic oligopeptide moieties are considered candidates for bone-targeting carriers (Figure 3, green background). The different features in bone formation and bone resorption physical chemistry provide a series of clues for developing specific affinity agents. On the bone formation surfaces, osteoblasts are characteristically covered by lowly crystallized hydroxyapatite and amorphous calcium phosphonate. Nevertheless, the physical chemistry of bone resorption surfaces is characterized by osteoclast distribution on highly crystallized hydroxyapatite (Wang et al., 2007). Bone-targeting oligopeptides have an interesting property of selective binding to bone resorption or bone formation surfaces. Asp8 has a stronger affinity for highly crystallized hydroxyapatite than for less crystallized hydroxyapatite, which is beneficial for binding to bone resorption sites and selectively approaching osteoclasts (Liu et al., 2015). For infection treatment, acidic oligopeptides can chemically conjugate with antibiotics, which enhances the bone specificity of antibiotics and limits their systemic toxicity. Yao et al. (2021) reported that eight repeating sequences of aspartate (D-Asp8) could be used as a bone-targeting delivery system with enoxacin-loaded mesoporous silica nanoparticles (MSNs). Enoxacin, as a third-generation fluoroquinolone antibiotic, has not only a bactericidal effect but also an inhibitory effect on osteoclasts (Toro et al., 2012).
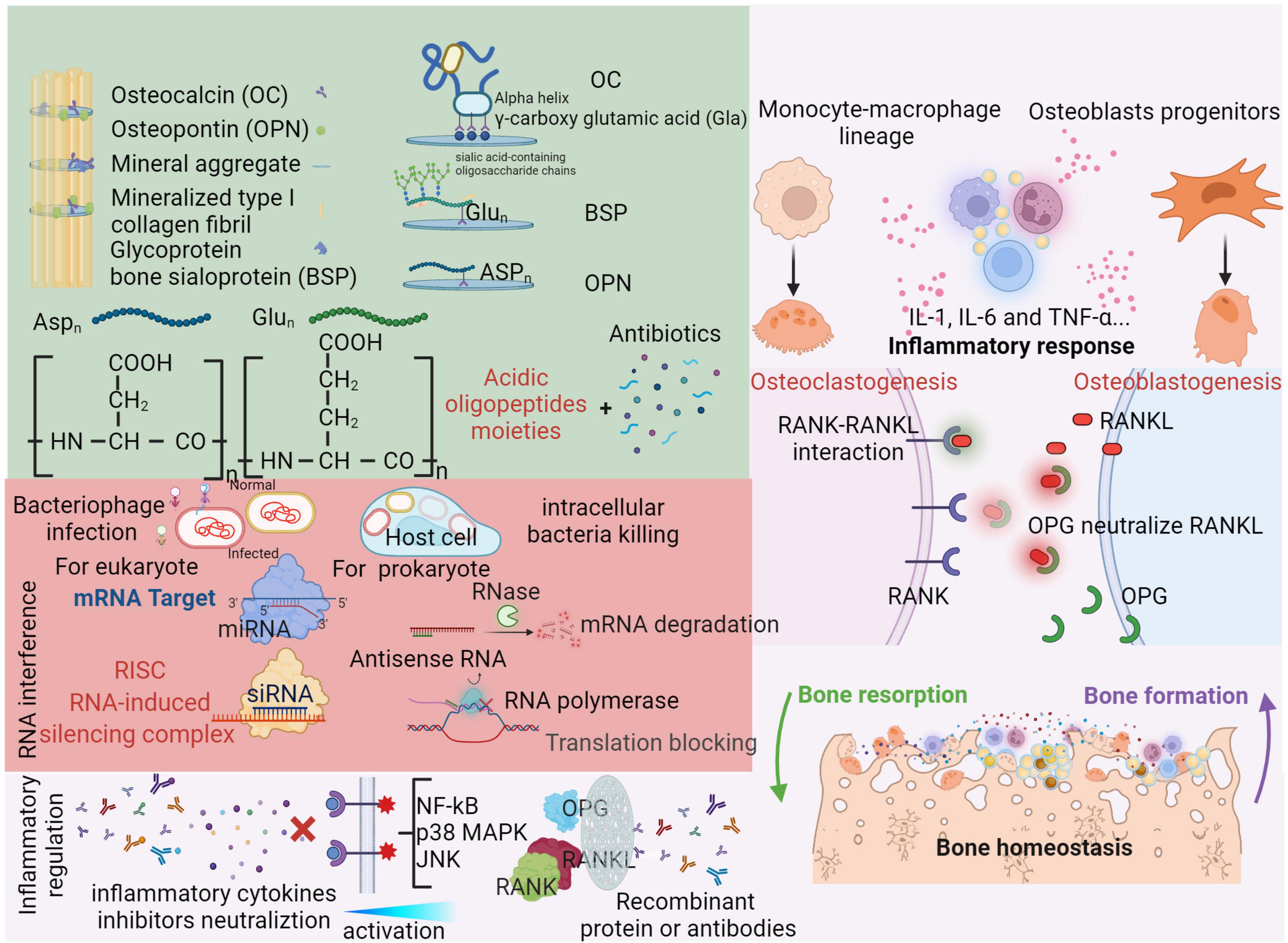
Figure 3. Organic compounds are also essential for bone matrix. Besides collagenous proteins, non-collagenous proteins (OC, BSP, and OPN) can strongly bind to Ca2+ or the mineral surfaces of bone with repetitive acidic amino acids moieties (aspartate, Aspn and glutamic, Glun) (Takahashi-Nishioka et al., 2008). Through three neighboring γ-carboxy glutamic acid (Gla) residues on the same face of the alpha helix, osteocalcin (OC) can bind with mineral aggregate (Stock, 2015). RNA interference (RNAi) is effectiveness in transcriptive level as a novel administration. For bacterial elimination, antisense RNA can recruit RNase to degrade base-paired mRNA and repel RNA polymerase to block translation (Quemener et al., 2022). Similarly, miRNA and siRNA can form RNA-induced silencing complex intraocularly for eukaryote, which are enable to modulate bone homeostasis (Jinek and Doudna, 2009). Bacteriophages can specifically and continually infect bacterial even in host cells. Therefore, a cocktail of several phages is designed as an infection targeting therapy for osteomyelitis (Chan et al., 2013). Please see above context in red background. In responses to bone infection, the inflammation induced release of various cytokines (IL-1, IL-6, and TNF-α) which can active the nuclear factor kB (NF-kB), p38 mitogen-activated protein kinase (MAPK) and c-Jun N-terminal kinase (JNK) pathways (Wright and Nair, 2010). Subsequently, the expressions of bone turnover proteins (OPG, RANKL and RANK) and cross-talk between osteoclasts and osteoblasts are alternated. RANKL can be released by osteoblasts and bind with RANK to active osteoclasts. As a result, the activity of bone resorption is significantly increased. Also, osteoblasts can release OPG which plays a role on neutralize RANKL as a soluble decoy receptor (Jin et al., 2007). Recently, many inhibitors or recombinant antibodies are developed as an inflammation targeting therapy to alleviate hyperinflammatory status and rebuilt the balance of bone homeostasis (Tang et al., 2020). Please see detail in light red background. Created with BioRender.com.
Conversely, six repetitive sequences of (AspSerSer)6 are favorable for binding to amorphous calcium phosphate and are regarded as a selective targeting moiety for bone formation surfaces (Zhang et al., 2012). To decrease the potential toxic side effects of bone-targeting delivery systems at the tissue level, such as undesirable accumulation in other endothelial cells or lymphocytes near the bone formation surface, a more targeted system at the cellular level is developed to promote bone-targeting delivery efficacy. Sun et al. (2016) identified an osteoblast-targeted peptide sequence as Ser-Asp-Ser-Ser-Asp (SDSSD). The SDSSD peptide binds with Periostin (an osteoblast-specific factor 2, OSF-2) in a ligand-receptor-specific manner, indicating its osteoblast-targeting ability. With angomirs/antagomirs, a class of endogenous non-coding microRNAs (miRNAs) can widely modulate gene expression to participate in maintaining cellular homeostasis. Induced by anti-miR-214, the levels of miR-214 in osteoblasts were significantly decreased, while bone formation was increased (Sun et al., 2016), which could be applied to stimulate inactivated osteoblasts in the infection microenvironment.
Antibiotic resistance in bacteria, attributed to multiple mechanisms, imposes a serious threat to global health. Moreover, SCVs of S. aureus are capable of infiltrating different types of host cells, resulting in regional recurrent and chronic bone infections (Kahl et al., 2016). Through the adhesion of surface proteins, fibronectin-binding proteins (FnBP) and activated uptake, the SCVs of S. aureus can be located intracellularly in host cells, particularly in osteoblasts, and osteoclasts (Kavanagh et al., 2018). The internalization of SCVs with osteoclasts promotes proinflammatory cytokine (macrophage inflammatory protein, keratinocyte chemoattractant, and granulocyte macrophage colony-stimulating factor) secretion and enhances the bone resorption capacities of osteoclasts (Trouillet-Assant et al., 2015). Additionally, internalized S. aureus can induce apoptosis of osteoblasts via the TRAIL/caspase pathway, which results in imbalanced bone homeostasis (Tucker et al., 2000; Ning et al., 2011; Widaa et al., 2012). Recent studies have shown that antimicrobial peptides (AMPs) can be released from osteoblasts infected by S. aureus for self-defense as a promising and effective strategy to treat osteomyelitis, notably for intracellular S. aureus (Zhu et al., 2013; Josse et al., 2015). From this point of view, AMPs present distinct advantages over traditional antibiotics (Browne et al., 2020). AMPs are part of the innate immune system and exhibit broad-spectrum antimicrobial activity against ESKAPE (referring to Enterococcus faecium, S. aureus, Klebsiella pneumoniae, Acinetobacter baumannii, Pseudomonas aeruginosa, and Enterobacter sp.) (Costa et al., 2021).
The direct interaction on the bacterial cell membrane contributes to the main antibacterial mechanism of AMPs. Usually, AMPs are positively charged hydrophobic peptides. Through electrostatic interactions with anionic bacterial membranes, cationic antimicrobial peptides, such as AMPs, promote pore formation and alternate membrane permeability, ultimately causing bacterial cell death (Zhang and Gallo, 2016). AMPs can also translocate through the membrane to reach intracellular targets. The antibiofilm action mechanisms of AMPs were speculated to act by stimulating bacterial surface motility and reducing cell attachment (Bucki et al., 2010). Moreover, by downregulating quorum-sensing systems, AMPs negatively regulate biofilm development and maintenance (Overhage et al., 2008). The stringent stress response (SR) is highly conserved among gram-negative and gram-positive bacteria. With secondary messenger molecule (p) ppGpp medication, SR plays an important role in biofilm development. AMPs can bind to and degrade secondary messengers, acting as antibiofilm peptides (de la Fuente-Nunez et al., 2014; Pletzer and Hancock, 2016). Considering the dispersal of bacterial cells from biofilms, which may cause recurrent infection, antibiofilm AMPs can be applied synergistically with conventional antibiotics for biofilm infection (Reffuveille et al., 2014). In addition to their antibacterial and antibiofilm properties, AMPs are also involved in modulating the immune response and anticancer activity and are termed host defense peptides (HDPs) (Raheem and Straus, 2019).
In orthopedic applications, AMPs have been utilized mainly in release modes and immobilization strategies (Costa et al., 2021). Calcium phosphate cement (CPC) is regarded as a common scaffold for the augmentation of bone defects. Our previous study reported that CPC could be applied with antibiotics for osteomyelitis treatment (Wu et al., 2021a). To overcome the resistance of methicillin-resistant Staphylococcus aureus (MRSA), Stallmann et al. (2003) combined CPC with human lactoferrin 1-11 (hLF1-11) AMP. The cements showed a continuous low-level release of AMP after a 24-h burst. Low concentrations of AMPs lead to biofilm dispersal, while higher doses result in biofilm cell death. In this study, AMP acted as a replacement for antibiotics in CPC, which provided sufficient bactericidal activity for resistant infections (Stallmann et al., 2003). Considering that titanium (Ti) has been widely used for orthopedic implants, the surface of Ti can be functionalized with oxhydryl and amino groups and tether biomolecules to obtain antibacterial properties (Costa et al., 2011). In one previous study, the Ti surface was amine-functionalized by a silanization process and coated with PEG to be covalently bound with enoxacin (Nie et al., 2017). To avoid systematic cytotoxicity and elongating antibacterial effects, AMPs can also be immobilized onto biomaterial surfaces and provide antimicrobial action at the implant site (Gabriel et al., 2006). FK-16, as a fragment corresponding to residues 17–32 of human LL-37, presents broad-spectrum activity against ESKAPE pathogens (Li et al., 2006). Mishra and Wang (2017) reported that Ti was coated with applied FK-16 via silanization treatment, which illustrated a significant inhibition of bacterial inoculation. In addition to titanium, other orthopedic implant-related polymeric and metal surfaces, such as silicone, polyethylene terephthalate, silicon, and stainless steel, can also be modified with AMPs according to an immobilization approach and possess antibacterial and antibiofilm characteristics (Costa et al., 2011).
Nucleic Acid-Based Therapeutic Strategy Inhibits Biofilm Formation by Down-Regulating Bacterial Growth and Pathogenicity
RNA interference (RNAi) is an essential cellular regulation mechanism in which gene expression can be silenced by directly targeting a sequence (Lei et al., 2018). Accordingly, RNAi offers a new genetic medical approach targeting bone disease-associated pathogenic genes and could be a potential translational therapy for bone-related diseases (Novina and Sharp, 2004). To decrease adverse effects in non-skeletal tissues of systemic RNAi administration, bone-specific delivery systems for RNAi-based therapies are highly desirable. Zhang et al. (2015) developed a bone-targeting D-Asp8-HPMA [N-(2-hydroxypropyl) methacrylamide copolymer] polymeric nanoparticle equipped with siRNA for sema4D in osteoclasts. Osteoclasts can communicate with osteoblasts via semaphorins and suppress osteoblast maturation (Leah, 2011), which could be a promising preventative approach for infection-induced bone loss. An RNAi mechanism is illustrated in Figure 3 with a red background in the lower arrow.
Aptamers are a class of small (25–35 bases in length) single-stranded RNA (ssRNA) or DNA (ssDNA) nucleotide sequences. These specially arranged nucleotide sequences have the ability to fold into unique tertiary conformations. Based on these structures, aptamers can recognize and bind with specific targets, reminiscent of antibodies (Nimjee et al., 2005). By employing cell-SELEX, Liang et al. (2015) identified an osteoblast-specific aptamer termed CH6 (5’AGTCTGTTGGACCGAATCCCGTGGACGCACCC TTTGGACG-3’). With lipid nanoparticles encapsulating Plekho1 siRNA, the CH6 aptamer can be specifically taken up by osteoblasts via micropinocytosis, which silences the Plekho1 gene and promotes bone formation. Although there are subtle distinctions between mammalian cells and bacterial cells, nucleic acid-based strategies are also considered for bacterial targeting. In bacteria, ComE, a competence protein family, is available to recognize and transport DNA materials across bacterial cell membranes (Dubnau and Blokesch, 2019). The DNA can be recognized and taken up by the cells at an extremely fast rate, which offers a potential targeting approach on bacteria even without particular targeting moieties. As DNase is mainly located in the nucleus and mitochondria of mammalian cells, DNA-based vectors can remain stable in the cytoplasm (Boone and Tsang, 1997; Setyawati et al., 2014). While nucleases can be found on bacterial membranes, DNA degradation is triggered in transition or transformation processes (Okabayaski and Mizuno, 1974). Considering the distinct localization of DNase in mammalian and bacterial cells, DNA-based nanoparticle vectors such as tetrahedral framework nucleic acids (tFNAs) have the advantage of invading initially and combating intercellular bacterial cells such as the SCV of S. aureus. In a study by Setyawati et al. (2014) a DNA nanopyramid (DP) was prepared with 4 strands of oligonucleotides. Actinomycin D (AMD) was chosen as a model antimicrobial compound. By the interaction of its phenoxazone aromatic rings parallel with the guanine base of DNA, AMD is logged into the DNA framework. With the degradation of the DP structure by DNase, the released AMD could kill the infectious bacteria and then degrade the biofilms effectively.
Antisense oligonucleotides (ASOs) are short synthetic DNA or RNA molecules. These ASOs, as structural blockers that inhibit the translation of RNA, can be programmed to various sequences and specifically recognize targeting RNA by Watson–Crick base pairing. Depending on DNA/RNA heteroduplex formation, RNA cleavage is induced by RNase, and the mRNA expression level is ultimately modulated. ASOs are highly valuable as a novel strategy to treat a wide range of diseases linked to dysregulated gene expression (Quemener et al., 2022). The two-component regulatory system (TCS) commonly comprises two components termed “the sensor kinase” and “the response regulator.” TCSs are ubiquitous in bacteria but rare in mammalian cells. The membrane-bound sensor kinase mainly responds to environmental stimuli, including antibiotic stress, and induces an adaptive response with cognate response regulators, which enables bacteria to adapt to diverse environmental stresses. Therefore, TCSs have the potential to serve as targets for antimicrobial chemotherapy (Hirakawa et al., 2020). In our previous study, YycFG, also named VicRK or WalRK, was the only essential TCS contributing to bacterial pathogenicity and biofilm formation in gram-positive bacteria with a low G-C content, including S. aureus and E. faecalis (Wu et al., 2021c). Zhang et al. (2020) applied an ASO (multi-targeting the highly conserved promoter regions of gtfBCD, gbpB, and ftf, which can be modulated by VicRK TCS) to inhibit biofilm formation and S. mutans virulence with tFNAs as vectors. One of our studies indicated that antisense yycF (ASyycF) significantly inhibited antibiotic resistance and pathogenicity in S. aureus bone infection biofilms (Wu et al., 2021b). Therefore, based on the above ASO strategies and tFNA delivery systems, a TCS-targeting ASO could be developed as an efficient specific therapeutic strategy at the transcriptional level. Considering the stability of ASOs in biological fluids, many improvements have been introduced to increase resistance against nucleases. Peptide nucleic acid (PNA) molecules are the third generation of ASOs (Quemener et al., 2022). They consist of a chain of N-(2-aminoethyl) glycine units, and the nucleobases can attach to N-(2-aminoethyl) glycine units via a methyl carbonyl linker (Larsen et al., 1999). As a highly conserved FtsZ protein plays a role in bacterial cell division, Zhang et al. (2018) utilized an antisense PNA (asPNA) targeting the ftsZ gene. With tetrahedral DNA nanostructures as vectors, asPNA was successfully delivered into MRSA and effectively inhibited cell growth.
Bacteriophage-Based Bone Infection Targeted Therapeutics Strategy to Eliminate the Not Only Planktonic but Also Biofilm Bacteria
Phages are viruses that specifically infect bacterial species. By adhering to specific surface receptors of bacteria, phages insert their hereditary material into their bacterial hosts (Onsea et al., 2020). With an estimated 1031 phage types (Gibb and Hadjiargyrou, 2021), the main mechanism through which phages kill bacteria is by taking over cell metabolism and ultimately inducing bacterial lysis. For S. aureus infection, a bacteriophage (MSa, “M” is for mutant; “Sa” is for S. aureus) was identified with activity against S. aureus, including MRSA. MSa can utilize the N-acetylglucosamine of the cell wall as its receptor (Gordillo Altamirano and Barr, 2021). Interestingly, S. aureus infected with phages can be delivered inside cells such as macrophages simultaneously with the process of S. aureus infecting host cells. As the phages replicate and release from infected S. aureus, the rest of the intracellular S. aureus will be subsequently affected and killed (Capparelli et al., 2007). In some cases, phages can incorporate their genome into the host genome as a prophage and reenter the lytic cycle under environmental stressors (Doss et al., 2017). This property seems to endow phages with the ability to kill persister cells in biofilms with low metabolic states, as prophages can lyse these cells when metabolic activity is recovered (Geneviere et al., 2021). This mechanism is illustrated in Figure 3 with a red background. As the resistance of bacteria in biofilms is up to 1,000-fold that of planktonic bacteria, as biofilm formation makes it difficult for conventional antibiotics to penetrate. Based on their lytic cycle, bacteriophages self-amplify in biofilms and possess an inherent ability to degrade biofilms completely (Akanda et al., 2018).
Recent evidence has shown successful bacteriophage therapy for bone and joint infections (Onsea et al., 2021). Considering the limited host range of particular bacteriophages, cocktail therapy involving more than one phage delivered simultaneously has been developed (Chan et al., 2013). When two or more phages in the cocktail attack the same bacterium, the combination may produce a better killing effect than any single phage to eliminate biofilm (Schmerer et al., 2014). In an osteomyelitis rabbit model, Kishor et al. (2016) treated MRSA infections with a cocktail of seven phages, which were selected based on their virulence. The results indicated that the phage cocktail is sufficient for treating osteomyelitis, and the combination treatment demonstrated a very good profile against infection synergistically. Yilmaz et al. (2013) combined local injection of bacteriophage Sb-1 and intraperitoneal teicoplanin administration for an MRSA implant-related infection rat model. This combination not only eradicated planktonic bacteria but also destroyed the biofilm. When phages are used to treat polymicrobial infections, multiple phages are typically applied simultaneously as a cocktail. In one previous case, a multiphage application (ᶲAbKT21phi3 and ᶲKpKT21phi1) and antibiotics (meropenem and colistin) successfully treated a patient with a trauma-related left tibial infection with XDR (extensively drug-resistant) Acinetobacter baumannii and MDR (multidrug-resistant) Klebsiella pneumoniae and avoided the serious complication of leg amputation (Nir-Paz et al., 2019).
Encapsulation of phage into a carrier is applied to control the release of phages in a musculoskeletal infection locally. Wroe et al. (2020) developed a four-arm poly (ethylene glycol)-4-maleimide (PEG-4MAL) macromer-based hydrogel that is capable of encapsulating Pseudomonas aeruginosa bacteriophage. In a murine local bone infection model, the antibacterial effect of the bacteriophage can remain for at least 7 days with this hydrogel (Wroe et al., 2020). Hydroxyapatite and beta-tricalcium phosphate (β-TCP) are materials commonly used in bone repair. In another study, one kind of Escherichia coli phage was applied to load with HA or β-TCP. This in vitro study demonstrated that those phages could be sustainedly released and were sufficient to eliminate the bacteria and to degrade the biofilms (Meurice et al., 2012). However, phages have varying degrees of antigenicity, although phages are not pathogens for eukaryotic cells. Notably, several mechanisms are involved in bacterial resistance to phages. Through genetic modification, genetically engineered bacteriophages can be less immunogenic and adaptive to resistance (Ly-Chatain, 2014; Gibb and Hadjiargyrou, 2021). Phage therapy was regarded as a promising strategy for persistent and resistant bacterial infections. Recent reports have described the clinical use of bacteriophages (Gelman et al., 2021). AB-SA01 (AmpliPhi Biosciences) is a manufacturing practice (GMP)-quality phage preparation involving obligately lytic tailed double-stranded DNA phages. Previously, thirteen S. aureus infected patients including septic shock, osteomyelitis, and infective endocarditis in Australia received the intravenous administrations of AB-SA01 as adjunctive bacteriophage therapies. There were eight patients (62%) presented clinically improved without adverse reactions even after 90 days observation, indicating the safety of bacteriophage therapy in severe Staphylococcus aureus infections (Petrovic Fabijan et al., 2020).
CaP-Based Bone-Targeting Therapeutic Strategy Can Be Designed to Carry Antibiotics for Bone Infection Control
CaP-based bone-targeting therapies, including tetracycline, hydroxyapatite and bisphosphonates, have a high affinity for skeletal tissues and increase the topical concentration (Figure 4). Tetracycline (TC) and its derivatives are an important class of broad-spectrum antibiotics (Shi et al., 2020; Rusu and Buta, 2021). The tetracyclic naphthacene carboxamide ring is a basic structure. Various functional groups are modified in tetracycline to create drug derivatives. TC has received particular attention in relation to skeletal tissues due to its interactions with hydroxyapatite (HA) [Ca10(PO4)6(OH)2] of the bone matrix by van der Waals interactions, hydrogen bonding and good metal-complexing abilities chelated with Ca2+ (Wang et al., 2010, 2015). Previous studies showed the anti-inflammatory and osteoblast-stimulating effects of TC, which could regulate the infective immune microenvironment and inhibit bone destruction (Reichert et al., 2012; Gomes et al., 2017). However, considering the inherent toxicity and side effects, such as nausea, hypoglycemia, tooth discoloration and increased risk of mortality, in serious infection applications, tigecycline is rarely recommended as a monotherapy in osteomyelitis (Sanchez et al., 2004; Moenster et al., 2013).
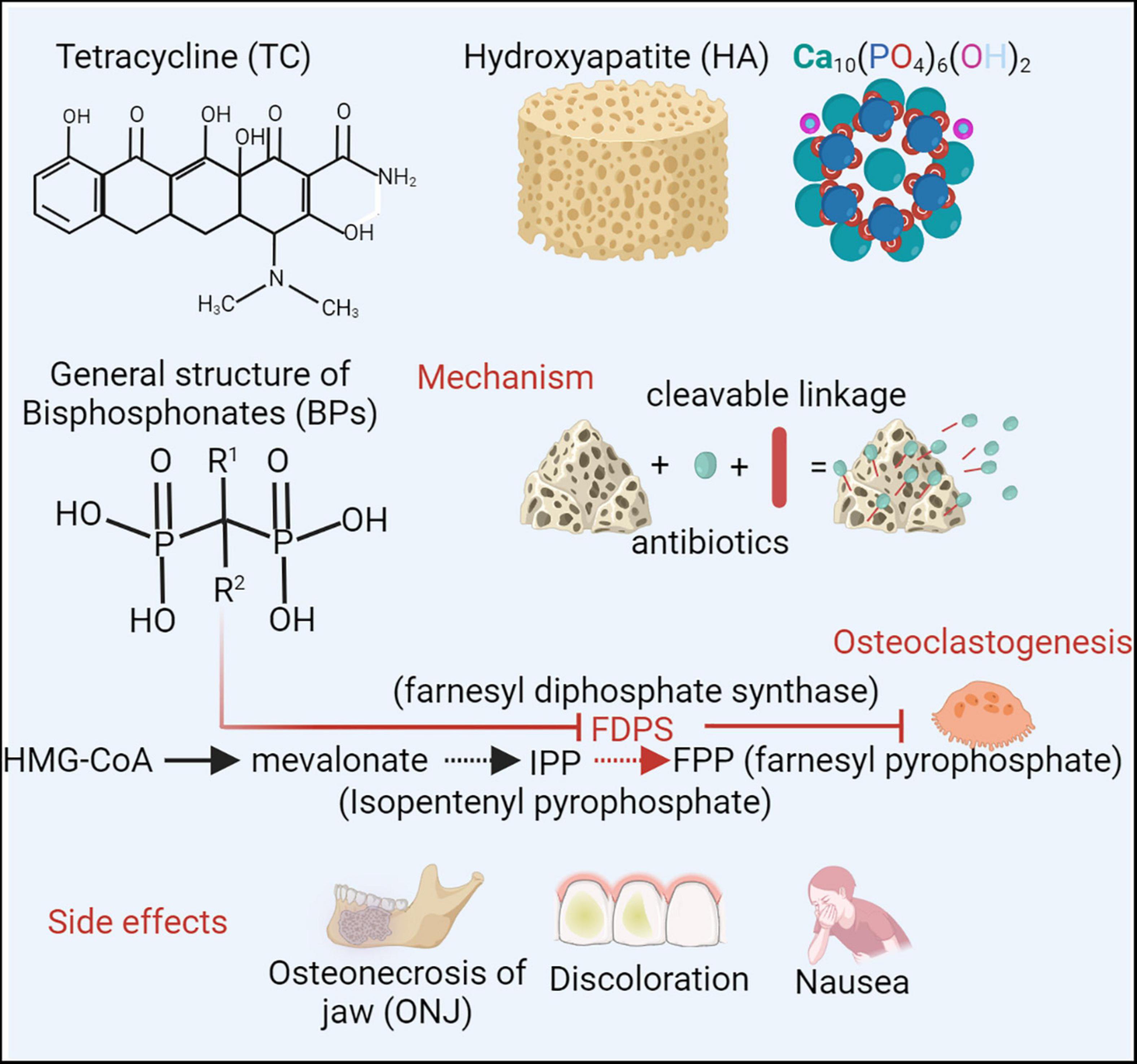
Figure 4. To improve therapeutic efficacy and minimize systematic toxicity, the bone targeting strategies have been applied (Shi et al., 2020). Tetracycline (TC) and its derivative are an important class of broad-spectrum antibiotics. Considering interactions with bone matrix of hydroxyapatite (HA) [Ca10(PO4)6(OH)2] and chelating with Ca2+, TC arouse attractions in skeletal tissues. However, side effects, such as nausea, hypoglycemia, dental discoloration, restrict TC applications as a monotherapy in osteomyelitis (Rusu and Buta, 2021). Bisphosphonates (BPs), (HO)2P(O)CR1R2P(O)(OH)2, can also interact with calcium and acquire the specificity of bone affinity. By blocking enzyme farnesyl diphosphate synthase (FDPS), BPs can inhibit FPP (farnesyl pyrophosphate) production and osteoclastogenesis, which subsequently prevents bone loss (Bjelic and Finsgar, 2022). Due to high accumulation in jawbone matrix, BP probably induce a serious complication of osteonecrosis of the jaws (ONJ) (Cheong et al., 2014). Combined with CaP based bone targeting materials, different antimicrobial agents can be conjugated for local administration. Created with BioRender.com.
Local antimicrobial administration provides a high antibiotic concentration and reduces systemic adverse effects. This infection treatment pattern is available to eliminate biofilm persisting bacteria, such as SCVs (Huiras et al., 2012). Conventional polymethylmethacrylate (PMMA) is often utilized for the delivery of antibiotics, such as gentamicin and vancomycin, in the form of beads, which can be embedded into the surroundings of musculoskeletal infections (Cyphert et al., 2021). Due to the recurrence of S. aureus SCVs following local application of gentamicin in osteomyelitis, gentamicin is not recommended as a single-application treatment (Miller et al., 1978; von Eiff et al., 1997; Wright and Nair, 2010). Considering the release performance and low degradability of PMMA, a more biocompatible and bioactive calcium phosphate, hydroxyapatite (HA), has been developed as an essential element for bone formation and replacement (Lotsari et al., 2018). HA can be designed as a matrix for drug delivery, such as vancomycin in osteomyelitis (Joosten et al., 2005; Abdul Halim et al., 2021). It consists of positively charged Ca2+ and negatively charged PO43– ions, which have the ability to attract drugs as nanocarriers (Abdul Halim et al., 2021). Nanoscale particles, as a delivery system with good permeability, are intended to transport the drug to the target location in the body in a controlled manner, which reduces toxicity and side effects and relieves patients by lowering the dose (Vega-Vasquez et al., 2020). Ciprofloxacin is a broad-spectrum antimicrobial for pathogens such as S. aureus in long bone osteomyelitis and P. aeruginosa or Aggregatibacter spp. in jawbone osteomyelitis (Reeves et al., 2013; Kim et al., 2014). Additionally, HA can be designed as hydroxyapatite nanocomposites (HANs) to carry antibiotics such as ciprofloxacin for bone infection (Mahdavinia et al., 2019).
Bisphosphonates (BPs) are a group of compounds with the general structure (HO)2P(O)CR1R2P(O)(OH)2. The bone-specific affinity of BPs is mainly attributed to two phosphonate groups (P-C-P), which can interact with calcium to form bi- and tridentate ligands (Sun et al., 2021). BPs can also chemically link to drugs as drug-releasing conjugates. By cleavable carbamate linkage, drugs can be designed with BPs and can be released in sites under an acidic and enzymatic environment (e.g., inflammation or infection) (Ossipov, 2015). Local infection and inflammation induce accelerated bone turnover and accumulation of BPs (Sedghizadeh et al., 2017). Thus, BPs, as a bone-targeting delivery for infective treatment, are preferably targeted at skeletal sites of active metabolism, such as infection and inflammation regions. Chronic inflammation induced by infection can trigger osteoclast activation and inhibit osteoblast functioning. BPs can decrease osteoclast activation and enhance osteoblast functioning, subsequently reducing chronic inflammation. By blocking the enzyme farnesyl diphosphate synthase (FPP), BPs can inhibit osteoclastogenesis and intervene in osteoclast survival (Bjelic and Finsgar, 2022). Alendronate, a BP, has been shown to promote osteoblast activity in osteogenesis (Petrovic et al., 2020). Although BPs are regarded as a bone homeostasis regulator for bone infection therapy, there is a serious complication of osteonecrosis of the jaw (ONJ) during BP treatment, which is related to the high accumulation of BP in the jawbone matrix (Cheong et al., 2014).
Turnover Homeostasis-Based Bone-Targeted Therapeutic Strategies on Biofilm Infection Related Immunity Homeostasis for Host-Bacteria
In addition to bacterial invasion, bone infections are accompanied by complicated immune responses with various immune cell infiltrations that release inflammatory cytokines locally and systemically (Wright and Nair, 2010). Immune cells, such as essential neutrophils and monocytes, are involved in innate or adaptive immunity against intruding pathogens and result in a severe cytokine storm, such as interleukin (IL)-1, IL-6 and TNF-α release, to produce a hyperinflammatory status. The net inflammatory reaction in the infection condition is shown in Figure 3 with a light red background. Inflammasome activation greatly contributes to bone resorption by upregulating osteoclast activity (Roper et al., 2020; Jin et al., 2021). Osteoclasts are derived from the monocyte-macrophage lineage in bone marrow and mediate bone resorption. Therefore, the combination treatment of antibiotics and immunomodulatory therapy limits the immune response and related bone destruction, which is proposed for future strategies against bone infections (Kwiecinski et al., 2013; Ali et al., 2015a,b; Jin et al., 2021). Tumor necrosis factor (TNF)–α, as a central mediator of inflammation and immune regulation, has a detrimental effect in cases of systematic S. aureus infections (Riegels-Nielsen et al., 1989). Fei et al. (2011) combined the TNF-α inhibitor Enbrel and antibiotics in S. aureus arthritis mouse models. This novel strategy significantly reduced the extent of bone damage. Additionally, the severity of staphylococcal enterotoxin shock syndrome in S. aureus sepsis was restricted to a low level, which may be associated with restoration of the hemostatic balance and downregulation of high-mobility group protein B1.
TNF-α inhibitors exhibit not only anti-inflammatory but also inhibitory functions in osteoclast formation. Peptidoglycan (PGN) and lipoteichoic acids (LTAs) are the major structures of the S. aureus cell wall (Brown et al., 2013). These structures can be recognized by the host immune system and strongly induce the release of proinflammatory cytokines, including TNF-α and IL-6. The receptor activator of nuclear factor-κB ligand (RANKL), a member of the tumor necrosis factor (TNF) superfamily, is found on the surface of osteoblasts. RANKL is active as both a trimeric transmembrane protein and a soluble monomer after it is cleaved from the cell surface by the metalloprotease-disintegrin TNF-α converting enzyme (TACE) (Kanzaki et al., 2016; Saint-Pastou Terrier and Gasque, 2017). When RANKL binds with receptor activator of nuclear factor kappa-B (RANK) on the surfaces of osteoclasts, osteoclasts can be activated, resulting in bone matrix absorption (Jin et al., 2021). In RANKL-induced osteoclastogenesis, TNF-α, produced by osteoclast progenitors, plays a mediating role (Zou et al., 2001). Hence, the combination therapy of antibiotics and anti-TNF reduces bone destruction of S. aureus sepsis in mice. Other inflammatory cytokine inhibitors, such as IL-1 and IL-6R antagonists, also inhibit the activation of osteoclasts and reduce inflammatory reactivity in response to pathogen-associated molecules (Tang et al., 2020). The immunomodulatory therapy is also playing an essential role in immunocompromised patients, such as patients with diabetes. In diabetic patients, the monocytes recruitment is significantly inhibited and pro-inflammatory macrophages are prevented from turning into its anti-inflammatory phenotype, which induces a prolonged inflammatory phase and leads to the chronic wound healing. The concentration of pro-inflammatory cytokines, such as tumor necrosis factor α (TNF-α) and interleukin-1b (IL-1b), are increased in diabetic wounds. Therefore, the maintenance of balanced inflammatory response is essential for the wound healing process (Rong et al., 2022). Sesamol (SM) is a natural organic compound and derived from sesame oil, which has properties including antioxidant, anti-inflammatory and antihyperglycemic. In one previous study, SM is Loaded with poly (lactic-co-glycolic acid), also named PLGA, and significantly accelerated wound process in rat diabetic foot ulcers by reduction of inflammatory mediators’ expression such as TNF-α (Gourishetti et al., 2020).
Persistent cells within biofilms can produce many virulence factors, such as S. aureus protein A (SpA), which is primarily anchored to the cell wall. SpA can adjust the host immunity system mainly based on the mechanism of binding with the Fc domain of antibodies. Additionally, SpA can interact with osteoblasts, preventing the expression of osteogenic markers that are essential for bone formation and mediating cell death (Claro et al., 2013). SpA can induce osteoblasts to release soluble RANKL for osteoclast migration, which promotes osteoclastogenesis and osteoclastic activity in bone resorption (Widaa et al., 2012). Accordingly, the use of the RANKL inhibitor can be a bone-targeting therapy to inhibit excessive differentiation of osteoclasts stimulated by infection-induced inflammation and limit bone destruction. Denosumab is a fully human monoclonal IgG2 antibody that inhibits bone remodeling by blocking the RANKL–RANK interaction (Kostenuik et al., 2009). Recently, denosumab has been successfully applied to suppress inflammatory joint destruction (Takeuchi et al., 2019). Compared with bisphosphonates, which are preferentially distributed in the gaps of bone resorption in trabecular bone, denosumab is more effective and distributed widely in both cortical and cancellous bone (Baron et al., 2011). However, RANKL is also expressed on immune cells (T and B cells), which can potentially inhibit immunity via RANKL and increase the infection rates (Ferrari-Lacraz and Ferrari, 2011).
In bone remodeling, osteoblasts can physiologically produce osteoprotegerin (OPG) to neutralize RANKL as a soluble decoy receptor and inhibit osteoclast formation and bone resorption activities (Jin et al., 2007). In S. aureus infection, PGN promotes osteoblast secretion of RANKL and reduces OPG production by activating the Toll-like receptor-2 pathway (Chen et al., 2014). An imbalance of these factors with an increase in the RANKL/OPG ratio is associated with increased osteoclastogenesis and results in osteolytic pathologies. In contrast to RANKL inhibitors, OPG also prevents tumor necrosis factor-related apoptosis-induced ligand (TRAIL), which plays an important role in immunosurveillance (Vitovski et al., 2007). Therefore, blockade of RANKL by administration of OPG had few effects on inflammation. Verdrengh et al. (2010) applied a RANKL-targeting treatment, OPG-Fc, in a septic arthritis mouse model. In combination with cloxacillin, the activity of osteoclasts was significantly decreased, and infection-triggered osteoporosis was limited (Verdrengh et al., 2010). Therefore, OPG may be an osteoclast-targeting agent for antibiotics.
Taken together, these targeting strategies have various potential clinical applications for biofilm and bone infections. Bone infections involving S. aureus and other bacterial infection is difficult to treat due to the poor penetration of antibiotics into bones and infective biofilm. The targeting antibacterial agents have been designed as an interesting strategy for infection treatment to improve the local concentrations. According to constituents of bone, CaP-based and peptide-based strategies are two main bone targeting moieties, which can be localized to enhance concentration and reduce the systemic effects. For nucleic acid-based and bacteriophage-based strategy, both moieties can be applied systemically or topically and target on specific microorganism, which facilitates the elimination of biofilm. Host immune system also plays a pivotal role in infection. Moderate inflammatory reaction can promote the infection clearance and accelerate healing process. The application of immune modulators such as TNF-α inhibitors helps to maintain the immunity homeostasis even in immunocompromised patients and promotes the infection healing.
Conclusion
S. aureus is the major pathogenic bacterium for osteomyelitis, which results in a complex inflammatory response and bone destruction. Although various antibiotics have been applied widely for bone infection, the emergence of resistance and biofilm formation significantly decreases the effectiveness of those agents. To increase the local concentration and decrease unintended systemic toxicity, bone-targeting therapeutic strategies have been developed. Additionally, many peptides with repetitive acidic amino acid moieties favorably bind to the bone. By chemical conjunction with antibiotics, bone-targeting composites can release antibiotics at high local concentrations to kill bacteria effectively. Intracellular S. aureus, such as SCVs, particularly contributes to recurrent persisting infections. Phages can specifically infect bacterial pathogens in infected regions. Moreover, phages can infect intercellular S. aureus and eliminate those persister strains. CaP-based materials present a high affinity for the bone matrix via chelation with Ca2 + on bone. In addition to bacterial killing, imbalanced homeostasis of bone turnover is related to bone destruction. Pathogen molecule-induced cytokines can be regulated by specific antagonists and inhibit the activity of osteoclasts in bone resorption. As a supplementary strategy, immunomodulatory therapy can significantly improve antimicrobial strategies as a new approach to bone infection treatment.
Author Contributions
SW, BW, LL, and HZ: conceptualization (equal), data curation (equal), formal analysis (equal), validation (equal), writing—original draft (equal), and writing—review and editing (equal). SD and YL: conceptualization (supporting), formal analysis (supporting), funding acquisition (lead), and writing—review and editing (equal). All authors contributed to the article and approved the submitted version.
Funding
This work was supported by the National Natural Science Foundation of China (Grant No. 82170948), Sichuan Provincial Natural Science Foundation of China (Grant Nos. 2021YJ0455 and 2022YFS0161) and Post-Doctor Research Project, West China Hospital, Sichuan University (Grant No. 2020HXBH134).
Conflict of Interest
The authors declare that the research was conducted in the absence of any commercial or financial relationships that could be construed as a potential conflict of interest.
Publisher’s Note
All claims expressed in this article are solely those of the authors and do not necessarily represent those of their affiliated organizations, or those of the publisher, the editors and the reviewers. Any product that may be evaluated in this article, or claim that may be made by its manufacturer, is not guaranteed or endorsed by the publisher.
References
Abdul Halim, N. A., Hussein, M. Z., and Kandar, M. K. (2021). Nanomaterials-upconverted hydroxyapatite for bone tissue engineering and a platform for drug delivery. Int. J. Nanomedicine 16, 6477–6496. doi: 10.2147/IJN.S298936
Akanda, Z. Z., Taha, M., and Abdelbary, H. (2018). Current review-the rise of bacteriophage as a unique therapeutic platform in treating peri-prosthetic joint infections. J. Orthop. Res. 36, 1051–1060. doi: 10.1002/jor.23755
Ali, A., Welin, A., Schwarze, J. C., Svensson, M. N., Na, M., Jarneborn, A., et al. (2015a). CTLA4 immunoglobulin but not anti-tumor necrosis factor therapy promotes Staphylococcal septic arthritis in mice. J. Infect. Dis. 212, 1308–1316. doi: 10.1093/infdis/jiv212
Ali, A., Zhu, X., Kwiecinski, J., Gjertsson, I., Lindholm, C., Iwakura, Y., et al. (2015b). Antibiotic-killed Staphylococcus aureus induces destructive arthritis in mice. Arthritis Rheumatol. 67, 107–116. doi: 10.1002/art.38902
Baron, R., Ferrari, S., and Russell, R. G. (2011). Denosumab and bisphosphonates: different mechanisms of action and effects. Bone 48, 677–692. doi: 10.1016/j.bone.2010.11.020
Berbari, E. F., Kanj, S. S., Kowalski, T. J., Darouiche, R. O., Widmer, A. F., Schmitt, S. K., et al. (2015). 2015 Infectious Diseases Society of America (IDSA) clinical practice guidelines for the diagnosis and treatment of native vertebral osteomyelitis in adults. Clin. Infect. Dis. 61, e26–e46. doi: 10.1093/cid/civ633
Bjelic, D., and Finsgar, M. (2022). Bioactive coatings with anti-osteoclast therapeutic agents for bone implants: enhanced compliance and prolonged implant life. Pharmacol. Res. 176:106060. doi: 10.1016/j.phrs.2022.106060
Boone, D. L., and Tsang, B. K. (1997). Identification and localization of deoxyribonuclease I in the rat ovary. Biol. Reprod. 57, 813–821. doi: 10.1095/biolreprod57.4.813
Brown, S., Santa Maria, J. P. Jr., and Walker, S. (2013). Wall teichoic acids of gram-positive bacteria. Annu. Rev. Microbiol. 67, 313–336. doi: 10.1146/annurev-micro-092412-155620
Browne, K., Chakraborty, S., Chen, R., Willcox, M. D., Black, D. S., Walsh, W. R., et al. (2020). A new era of antibiotics: the clinical potential of antimicrobial peptides. Int. J. Mol. Sci. 21:7047. doi: 10.3390/ijms21197047
Bucki, R., Leszczynska, K., Namiot, A., and Sokolowski, W. (2010). Cathelicidin LL-37: a multitask antimicrobial peptide. Arch. Immunol. Ther. Exp. 58, 15–25. doi: 10.1007/s00005-009-0057-2
Calhoun, J. H., Manring, M. M., and Shirtliff, M. (2009). Osteomyelitis of the long bones. Semin. Plast. Surg. 23, 59–72. doi: 10.1055/s-0029-1214158
Capparelli, R., Parlato, M., Borriello, G., Salvatore, P., and Iannelli, D. (2007). Experimental phage therapy against Staphylococcus aureus in mice. Antimicrob. Agents Chemother. 51, 2765–2773. doi: 10.1128/AAC.01513-06
Chan, B. K., Abedon, S. T., and Loc-Carrillo, C. (2013). Phage cocktails and the future of phage therapy. Future Microbiol. 8, 769–783. doi: 10.2217/fmb.13.47
Chen, J., Xiong, A., Ma, Y., Qin, C., and Ho, C. L. (2021). Impact of the host-microbiome on osteomyelitis pathogenesis. Front. Mol. Biosci. 8:702484. doi: 10.3389/fmolb.2021.702484
Chen, Q., Hou, T., Luo, F., Wu, X., Xie, Z., and Xu, J. (2014). Involvement of toll-like receptor 2 and pro-apoptotic signaling pathways in bone remodeling in osteomyelitis. Cell. Physiol. Biochem. 34, 1890–1900. doi: 10.1159/000366387
Cheong, S., Sun, S., Kang, B., Bezouglaia, O., Elashoff, D., McKenna, C. E., et al. (2014). Bisphosphonate uptake in areas of tooth extraction or periapical disease. J. Oral Maxillofac. Surg. 72, 2461–2468. doi: 10.1016/j.joms.2014.07.004
Claro, T., Widaa, A., McDonnell, C., Foster, T. J., O’Brien, F. J., and Kerrigan, S. W. (2013). Staphylococcus aureus protein A binding to osteoblast tumour necrosis factor receptor 1 results in activation of nuclear factor kappa B and release of interleukin-6 in bone infection. Microbiology 159, 147–154. doi: 10.1099/mic.0.063016-0
Claros, M., Citron, D. M., Goldstein, E. J., Merriam, C. V., and Tyrrell, K. L. (2013). Differences in distribution and antimicrobial susceptibility of anaerobes isolated from complicated intra-abdominal infections versus diabetic foot infections. Diagn. Microbiol. Infect. Dis. 76, 546–548. doi: 10.1016/j.diagmicrobio.2013.04.025
Costa, B., Martinez-de-Tejada, G., Gomes, P. A. C., Martins, M. C. L., and Costa, F. (2021). Antimicrobial peptides in the battle against orthopedic implant-related infections: a review. Pharmaceutics 13:1918. doi: 10.3390/pharmaceutics13111918
Costa, F. I, Carvalho, F., Montelaro, R. C., Gomes, P., and Martins, M. C. (2011). Covalent immobilization of antimicrobial peptides (AMPs) onto biomaterial surfaces. Acta Biomater. 7, 1431–1440. doi: 10.1016/j.actbio.2010.11.005
Cyphert, E. L., Zhang, N., Marques, D. W., Learn, G. D., Zhang, F., and von Recum, H. A. (2021). Poly(methyl methacrylate) bone cement composite can be refilled with antibiotics after implantation in femur or soft tissue. J. Funct. Biomater. 12:8. doi: 10.3390/jfb12010008
de la Fuente-Nunez, C., Reffuveille, F., Haney, E. F., Straus, S. K., and Hancock, R. E. (2014). Broad-spectrum anti-biofilm peptide that targets a cellular stress response. PLoS Pathog. 10:e1004152. doi: 10.1371/journal.ppat.1004152
Doss, J., Culbertson, K., Hahn, D., Camacho, J., and Barekzi, N. (2017). A review of phage therapy against bacterial pathogens of aquatic and terrestrial organisms. Viruses 9:50. doi: 10.3390/v9030050
Dubnau, D., and Blokesch, M. (2019). Mechanisms of DNA uptake by naturally competent bacteria. Annu. Rev. Genet. 53, 217–237. doi: 10.1146/annurev-genet-112618-043641
Dunyach-Remy, C., Ngba Essebe, C., Sotto, A., and Lavigne, J. P. (2016). Staphylococcus aureus toxins and diabetic foot ulcers: role in pathogenesis and interest in diagnosis. Toxins 8:209. doi: 10.3390/toxins8070209
Fei, Y., Wang, W., Kwiecinski, J., Josefsson, E., Pullerits, R. I, Jonsson, M., et al. (2011). The combination of a tumor necrosis factor inhibitor and antibiotic alleviates Staphylococcal arthritis and sepsis in mice. J. Infect. Dis. 204, 348–357. doi: 10.1093/infdis/jir266
Ferrari-Lacraz, S., and Ferrari, S. (2011). Do RANKL inhibitors (Denosumab) affect inflammation and immunity? Osteoporos. Int. 22, 435–446. doi: 10.1007/s00198-010-1326-y
Fouad, A. F. (2019). Microbiological aspects of traumatic injuries. J. Endod. 45, S39–S48. doi: 10.1016/j.joen.2019.05.011
Gabriel, M., Nazmi, K., Veerman, E. C., Nieuw Amerongen, A. V., and Zentner, A. (2006). Preparation of LL-37-grafted titanium surfaces with bactericidal activity. Bioconjug. Chem. 17, 548–550. doi: 10.1021/bc050091v
Gaetti-Jardim, E. Jr., Fardin, A. C., Gaetti-Jardim, E. C., de Castro, A. L., Schweitzer, C. M., and vila-Campos, M. J. A. (2010). Microbiota associated with chronic osteomyelitis of the jaws. Braz. J. Microbiol. 41, 1056–1064. doi: 10.1590/S1517-838220100004000025
Gelman, D., Yerushalmy, O., Alkalay-Oren, S., Rakov, C., Ben-Porat, S., Khalifa, L., et al. (2021). Clinical phage microbiology: a suggested framework and recommendations for the in-vitro matching steps of phage therapy. Lancet Microbe 2, e555–e563. doi: 10.1016/S2666-5247(21)00127-0
Geneviere, J., McCallin, S., Huttner, A., Pham, T. T., and Suva, D. (2021). A systematic review of phage therapy applied to bone and joint infections: an analysis of success rates, treatment modalities and safety. Efort Open Rev. 6, 1148–1156. doi: 10.1302/2058-5241.6.210073
Gibb, B. P., and Hadjiargyrou, M. (2021). Bacteriophage therapy for bone and joint infections. Bone Joint J. 103-B, 234–244. doi: 10.1302/0301-620X.103B2.BJJ-2020-0452.R2
Gomes, K. D. N., Alves, A. P. N. N., Dutra, P. G. P., and Viana, G. S. B. (2017). Doxycycline induces bone repair and changes in Wnt signalling. Int. J. Oral Sci. 9, 158–166. doi: 10.1038/ijos.2017.28
Gontcharova, V., Youn, E., Sun, Y., Wolcott, R. D., and Dowd, S. E. (2010). A comparison of bacterial composition in diabetic ulcers and contralateral intact skin. Open Microbiol. J. 4, 8–19. doi: 10.2174/1874285801004010008
Gordillo Altamirano, F. L., and Barr, J. J. (2021). Unlocking the next generation of phage therapy: the key is in the receptors. Curr. Opin. Biotechnol. 68, 115–123. doi: 10.1016/j.copbio.2020.10.002
Gourishetti, K., Keni, R., Nayak, P. G., Jitta, S. R., Bhaskaran, N. A., Kumar, L., et al. (2020). Sesamol-loaded PLGA nanosuspension for accelerating wound healing in diabetic foot ulcer in rats. Int. J. Nanomedicine 15, 9265–9282. doi: 10.2147/IJN.S268941
Hall-Stoodley, L., Costerton, J. W., and Stoodley, P. (2004). Bacterial biofilms: from the natural environment to infectious diseases. Nat. Rev. Microbiol. 2, 95–108. doi: 10.1038/nrmicro821
Hirakawa, H., Kurushima, J., Hashimoto, Y., and Tomita, H. (2020). Progress overview of bacterial two-component regulatory systems as potential targets for antimicrobial chemotherapy. Antibiotics 9:635. doi: 10.3390/antibiotics9100635
Huiras, P., Logan, J. K., Papadopoulos, S., and Whitney, D. (2012). Local antimicrobial administration for prophylaxis of surgical site infections. Pharmacotherapy 32, 1006–1019. doi: 10.1002/phar.1135
Jin, Q., Cirelli, J. A., Park, C. H., Sugai, J. V., Taba, M. Jr., Kostenuik, P. J., et al. (2007). RANKL inhibition through osteoprotegerin blocks bone loss in experimental periodontitis. J. Periodontol. 78, 1300–1308. doi: 10.1902/jop.2007.070073
Jin, T., Mohammad, M., Pullerits, R., and Ali, A. (2021). Bacteria and host interplay in Staphylococcus aureus septic arthritis and sepsis. Pathogens 10:158. doi: 10.3390/pathogens10020158
Jinek, M., and Doudna, J. A. (2009). A three-dimensional view of the molecular machinery of RNA interference. Nature 457, 405–412. doi: 10.1038/nature07755
Jneid, J., Lavigne, J. P., La Scola, B., and Cassir, N. (2017). The diabetic foot microbiota: a review. Hum. Microbiome J. 5-6, 1–6. doi: 10.1016/j.humic.2017.09.002
Joosten, U., Joist, A., Gosheger, G., Liljenqvist, U., Brandt, B., and von Eiff, C. (2005). Effectiveness of hydroxyapatite-vancomycin bone cement in the treatment of Staphylococcus aureus induced chronic osteomyelitis. Biomaterials 26, 5251–5258. doi: 10.1016/j.biomaterials.2005.01.001
Josse, J., Velard, F., and Gangloff, S. C. (2015). Staphylococcus aureus vs. osteoblast: relationship and consequences in osteomyelitis. Front. Cell. Infect. Microbiol. 5:85. doi: 10.3389/fcimb.2015.00085
Kahl, B. C., Becker, K., and Loffler, B. (2016). Clinical significance and pathogenesis of Staphylococcal small colony variants in persistent infections. Clin. Microbiol. Rev. 29, 401–427. doi: 10.1128/CMR.00069-15
Kanzaki, H., Makihira, S., Suzuki, M., Ishii, T., Movila, A., Hirschfeld, J., et al. (2016). Soluble RANKL cleaved from activated lymphocytes by TNF-alpha-converting enzyme contributes to osteoclastogenesis in periodontitis. J. Immunol. 197, 3871–3883. doi: 10.4049/jimmunol.1601114
Kavanagh, N., Ryan, E. J., Widaa, A., Sexton, G., Fennell, J., O’Rourke, S., et al. (2018). Staphylococcal osteomyelitis: disease progression, treatment challenges, and future directions. Clin. Microbiol. Rev. 31:e00084-17. doi: 10.1128/CMR.00084-17
Kim, B. N., Kim, E. S., and Oh, M. D. (2014). Oral antibiotic treatment of staphylococcal bone and joint infections in adults. J. Antimicrob. Chemother. 69, 309–322. doi: 10.1093/jac/dkt374
Kishor, C., Mishra, R. R., Saraf, S. K., Kumar, M., Srivastav, A. K., and Nath, G. (2016). Phage therapy of staphylococcal chronic osteomyelitis in experimental animal model. Indian J. Med. Res. 143, 87–94. doi: 10.4103/0971-5916.178615
Kostenuik, P. J., Nguyen, H. Q., McCabe, J., Warmington, K. S., Kurahara, C., Sun, N., et al. (2009). Denosumab, a fully human monoclonal antibody to RANKL, inhibits bone resorption and increases BMD in knock-in mice that express chimeric (murine/human) RANKL. J. Bone Miner. Res. 24, 182–195. doi: 10.1359/jbmr.081112
Kwiecinski, J., Rhost, S., Lofbom, L., Blomqvist, M., Mansson, J. E., Cardell, S. L., et al. (2013). Sulfatide attenuates experimental Staphylococcus aureus sepsis through a CD1d-dependent pathway. Infect. Immun. 81, 1114–1120. doi: 10.1128/IAI.01334-12
Larsen, H. J., Bentin, T., and Nielsen, P. E. (1999). Antisense properties of peptide nucleic acid. Biochim. Biophys. Acta 1489, 159–166. doi: 10.1016/s0167-4781(99)00145-1
Leah, E. (2011). Bone: finding that osteoclasts repel osteoblast activity through Sema4D reveals novel target for bone-boosting therapies. Nat. Rev. Rheumatol. 7:681. doi: 10.1038/nrrheum.2011.175
Lei, L., Stipp, R. N., Chen, T., Wu, S. Z., Hu, T., and Duncan, M. J. (2018). Activity of Streptococcus mutans VicR is modulated by antisense RNA. J. Dent. Res. 97, 1477–1484. doi: 10.1177/0022034518781765
Lei, L., Zeng, J., Wang, L., Gong, T., Zheng, X., Qiu, W., et al. (2021). Quantitative acetylome analysis reveals involvement of glucosyltransferase acetylation in Streptococcus mutans biofilm formation. Environ. Microbiol. Rep. 13, 86–97. doi: 10.1111/1758-2229.12907
Lew, D. P., and Waldvogel, F. A. (2004). Osteomyelitis. Lancet 364, 369–379. doi: 10.1016/S0140-6736(04)16727-5
Li, X., Li, Y., Han, H., Miller, D. W., and Wang, G. (2006). Solution structures of human LL-37 fragments and NMR-based identification of a minimal membrane-targeting antimicrobial and anticancer region. J. Am. Chem. Soc. 128, 5776–5785. doi: 10.1021/ja0584875
Liang, C., Guo, B., Wu, H., Shao, N., Li, D., Liu, J., et al. (2015). Aptamer-functionalized lipid nanoparticles targeting osteoblasts as a novel RNA interference-based bone anabolic strategy. Nat. Med. 21, 288–294. doi: 10.1038/nm.3791
Lipsky, B. A., Richard, J. L., and Lavigne, J. P. (2013). Diabetic foot ulcer microbiome: one small step for molecular microbiology … One giant leap for understanding diabetic foot ulcers? Diabetes 62, 679–681. doi: 10.2337/db12-1325
Liu, J., Dang, L., Li, D., Liang, C., He, X., Wu, H., et al. (2015). A delivery system specifically approaching bone resorption surfaces to facilitate therapeutic modulation of microRNAs in osteoclasts. Biomaterials 52, 148–160. doi: 10.1016/j.biomaterials.2015.02.007
Lotsari, A., Rajasekharan, A. K., Halvarsson, M., and Andersson, M. (2018). Transformation of amorphous calcium phosphate to bone-like apatite. Nat. Commun. 9:4170. doi: 10.1038/s41467-018-06570-x
Ly-Chatain, M. H. (2014). The factors affecting effectiveness of treatment in phages therapy. Front. Microbiol. 5:51. doi: 10.3389/fmicb.2014.00051
Maamari, J., Tande, A. J., Diehn, F., Tai, D. B. G., and Berbari, E. F. (2022). Diagnosis of vertebral osteomyelitis. J. Bone Jt. Infect. 7, 23–32. doi: 10.5194/jbji-7-23-2022
Mahdavinia, G. R., Karimi, M. H., Soltaniniya, M., and Massoumi, B. (2019). In vitro evaluation of sustained ciprofloxacin release from kappa-carrageenan-crosslinked chitosan/hydroxyapatite hydrogel nanocomposites. Int. J. Biol. Macromol. 126, 443–453. doi: 10.1016/j.ijbiomac.2018.12.240
McNeil, J. C. (2020). Acute hematogenous osteomyelitis in children: clinical presentation and management. Infect. Drug Resist. 13, 4459–4473. doi: 10.2147/IDR.S257517
McNeil, J. C., Forbes, A. R., Vallejo, J. G., Flores, A. R., Hulten, K. G., Mason, E. O., et al. (2016). Role of operative or interventional radiology-guided cultures for osteomyelitis. Pediatrics 137:e20154616. doi: 10.1542/peds.2015-4616
Meurice, E., Rguiti, E., Brutel, A., Hornez, J. C., Leriche, A., Descamps, M., et al. (2012). New antibacterial microporous CaP materials loaded with phages for prophylactic treatment in bone surgery. J. Mater. Sci. Mater. Med. 23, 2445–2452. doi: 10.1007/s10856-012-4711-6
Miller, M. H., Wexler, M. A., and Steigbigel, N. H. (1978). Single and combination antibiotic therapy of Staphylococcus aureus experimental endocarditis: emergence of gentamicin-resistant mutants. Antimicrob. Agents Chemother. 14, 336–343. doi: 10.1128/AAC.14.3.336
Mishra, B., and Wang, G. (2017). Titanium surfaces immobilized with the major antimicrobial fragment FK-16 of human cathelicidin LL-37 are potent against multiple antibiotic-resistant bacteria. Biofouling 33, 544–555. doi: 10.1080/08927014.2017.1332186
Moenster, R. P., Linneman, T. W., Call, W. B., Kay, C. L., McEvoy, T. A., and Sanders, J. L. (2013). The potential role of newer gram-positive antibiotics in the setting of osteomyelitis of adults. J. Clin. Pharm. Ther. 38, 89–96. doi: 10.1111/jcpt.12030
Nie, B., Long, T., Ao, H., Zhou, J., Tang, T., and Yue, B. (2017). Covalent immobilization of enoxacin onto titanium implant surfaces for inhibiting multiple bacterial species infection and in vivo methicillin-resistant Staphylococcus aureus infection prophylaxis. Antimicrob. Agents Chemother. 61:e01766-16. doi: 10.1128/AAC.01766-16
Nimjee, S. M., Rusconi, C. P., and Sullenger, B. A. (2005). Aptamers: an emerging class of therapeutics. Annu. Rev. Med. 56, 555–583. doi: 10.1146/annurev.med.56.062904.144915
Ning, R. D., Zhang, X. L., Li, Q. T., and Guo, X. K. (2011). The effect of Staphylococcus aureus on apoptosis of cultured human osteoblasts. Orthop. Surg. 3, 199–204. doi: 10.1111/j.1757-7861.2011.00146.x
Nir-Paz, R., Gelman, D., Khouri, A., Sisson, B. M., Fackler, J., Alkalay-Oren, S., et al. (2019). Successful treatment of antibiotic-resistant, poly-microbial bone infection with bacteriophages and antibiotics combination. Clin. Infect. Dis. 69, 2015–2018. doi: 10.1093/cid/ciz222
Novina, C. D., and Sharp, P. A. (2004). The RNAi revolution. Nature 430, 161–164. doi: 10.1038/430161a
Okabayaski, K., and Mizuno, D. (1974). Surface-bound nuclease of Staphylococcus aureus: localization of the enzyme. J. Bacteriol. 117, 215–221. doi: 10.1128/jb.117.1.215-221.1974
Onsea, J., Post, V., Buchholz, T., Schwegler, H., Zeiter, S., Wagemans, J., et al. (2021). Bacteriophage therapy for the prevention and treatment of fracture-related infection caused by Staphylococcus aureus: a preclinical study. Microbiol. Spectr. 9:e0173621. doi: 10.1128/spectrum.01736-21
Onsea, J., Wagemans, J., Pirnay, J. P., Di Luca, M., Gonzalez-Moreno, M., Lavigne, R., et al. (2020). Bacteriophage therapy as a treatment strategy for orthopaedic-device-related infections: where do we stand? Eur. Cell Mater. 39, 193–210. doi: 10.22203/eCM.v039a13
Ossipov, D. A. (2015). Bisphosphonate-modified biomaterials for drug delivery and bone tissue engineering. Expert Opin. Drug Deliv. 12, 1443–1458. doi: 10.1517/17425247.2015.1021679
Overhage, J., Campisano, A., Bains, M., Torfs, E. C., Rehm, B. H., and Hancock, R. E. (2008). Human host defense peptide LL-37 prevents bacterial biofilm formation. Infect. Immun. 76, 4176–4182. doi: 10.1128/IAI.00318-08
Petrovic, Z., Saric, A., Despotovic, I., Katic, J., Peter, R., Petravic, M., et al. (2020). A new insight into coating’s formation mechanism between TiO2 and alendronate on titanium dental implant. Materials 13:3220. doi: 10.3390/ma13143220
Petrovic Fabijan, A., Lin, R. C. Y., Ho, J., Maddocks, S., Ben Zakour, N. L., Iredell, J. R., et al. (2020). Safety of bacteriophage therapy in severe Staphylococcus aureus infection. Nat. Microbiol. 5, 465–472. doi: 10.1038/s41564-019-0634-z
Pletzer, D., and Hancock, R. E. (2016). Antibiofilm peptides: potential as broad-spectrum agents. J. Bacteriol. 198, 2572–2578. doi: 10.1128/JB.00017-16
Proctor, R. (2019). Respiration and small colony variants of Staphylococcus aureus. Microbiol. Spectr. 7, 549–561.
Proctor, R. A., von Eiff, C., Kahl, B. C., Becker, K., McNamara, P., Herrmann, M., et al. (2006). Small colony variants: a pathogenic form of bacteria that facilitates persistent and recurrent infections. Nat. Rev. Microbiol. 4, 295–305. doi: 10.1038/nrmicro1384
Quemener, A. M., Centomo, M. L., Sax, S. L., and Panella, R. (2022). Small drugs, huge impact: the extraordinary impact of antisense oligonucleotides in research and drug development. Molecules 27:536. doi: 10.3390/molecules27020536
Raheem, N., and Straus, S. K. (2019). Mechanisms of action for antimicrobial peptides with antibacterial and antibiofilm functions. Front. Microbiol. 10:2866. doi: 10.3389/fmicb.2019.02866
Redel, H., Gao, Z., Li, H., Alekseyenko, A. V., Zhou, Y., Perez-Perez, G. I., et al. (2013). Quantitation and composition of cutaneous microbiota in diabetic and nondiabetic men. J. Infect. Dis. 207, 1105–1114. doi: 10.1093/infdis/jit005
Reeves, B. D., Young, M., Grieco, P. A., and Suci, P. (2013). Aggregatibacter actinomycetemcomitans biofilm killing by a targeted ciprofloxacin prodrug. Biofouling 29, 1005–1014. doi: 10.1080/08927014.2013.823541
Reffuveille, F., de la Fuente-Nunez, C., Mansour, S., and Hancock, R. E. (2014). A broad-spectrum antibiofilm peptide enhances antibiotic action against bacterial biofilms. Antimicrob. Agents Chemother. 58, 5363–5371. doi: 10.1128/AAC.03163-14
Reichert, J. C., Cipitria, A., Epari, D. R., Saifzadeh, S., Krishnakanth, P., Berner, A., et al. (2012). A tissue engineering solution for segmental defect regeneration in load-bearing long bones. Sci. Transl. Med. 4:141ra93. doi: 10.1126/scitranslmed.3003720
Riegels-Nielsen, P., Frimodt-Moller, N., Sorensen, M., and Jensen, J. S. (1989). Antibiotic treatment insufficient for established septic arthritis. Staphylococcus aureus experiments in rabbits. Acta Orthop. Scand. 60, 113–115. doi: 10.3109/17453678909150107
Rong, Y., Yang, H., Xu, H., Li, S., Wang, P., Wang, Z., et al. (2022). Bioinformatic analysis reveals hub immune-related genes of diabetic foot ulcers. Front. Surg. 9:878965. doi: 10.3389/fsurg.2022.878965
Roper, P. M., Shao, C., and Veis, D. J. (2020). Multitasking by the OC lineage during bone infection: bone resorption, immune modulation, and microbial niche. Cells 9:2157. doi: 10.3390/cells9102157
Rusu, A., and Buta, E. L. (2021). The development of third-generation tetracycline antibiotics and new perspectives. Pharmaceutics 13:2085. doi: 10.3390/pharmaceutics13122085
Saint-Pastou Terrier, C., and Gasque, P. (2017). Bone responses in health and infectious diseases: a focus on osteoblasts. J. Infect. 75, 281–292. doi: 10.1016/j.jinf.2017.07.007
Sanchez, A. R., Rogers, R. S. III, and Sheridan, P. J. (2004). Tetracycline and other tetracycline-derivative staining of the teeth and oral cavity. Int. J. Dermatol. 43, 709–715. doi: 10.1111/j.1365-4632.2004.02108.x
Sanchez, C. J. Jr., Ward, C. L., Romano, D. R., Hurtgen, B. J., Hardy, S. K., Woodbury, R. L., et al. (2013). Staphylococcus aureus biofilms decrease osteoblast viability, inhibits osteogenic differentiation, and increases bone resorption in vitro. BMC Musculoskelet. Disord. 14:187. doi: 10.1186/1471-2474-14-187
Schmerer, M. I, Molineux, J., and Bull, J. J. (2014). Synergy as a rationale for phage therapy using phage cocktails. PeerJ 2:e590. doi: 10.7717/peerj.590
Schmitt, S. K. (2017). Osteomyelitis. Infect. Dis. Clin. North Am. 31, 325–338. doi: 10.1016/j.idc.2017.01.010
Sedghizadeh, P. P., Sun, S., Junka, A. F., Richard, E., Sadrerafi, K., Mahabady, S., et al. (2017). Design, synthesis, and antimicrobial evaluation of a novel bone-targeting bisphosphonate-ciprofloxacin conjugate for the treatment of osteomyelitis biofilms. J. Med. Chem. 60, 2326–2343. doi: 10.1021/acs.jmedchem.6b01615
Settem, R. P., El-Hassan, A. T., Honma, K., Stafford, G. P., and Sharma, A. (2012). Fusobacterium nucleatum and Tannerella forsythia induce synergistic alveolar bone loss in a mouse periodontitis model. Infect. Immun. 80, 2436–2443. doi: 10.1128/IAI.06276-11
Setyawati, M. I., Kutty, R. V., Tay, C. Y., Yuan, X., Xie, J., and Leong, D. T. (2014). Novel theranostic DNA nanoscaffolds for the simultaneous detection and killing of Escherichia coli and Staphylococcus aureus. ACS Appl. Mater. Interfaces 6, 21822–21831. doi: 10.1021/am502591c
Shi, C., Wu, T., He, Y., Zhang, Y., and Fu, D. (2020). Recent advances in bone-targeted therapy. Pharmacol. Ther. 207:107473. doi: 10.1016/j.pharmthera.2020.107473
Stallmann, H. P., Faber, C., Slotema, E. T., Lyaruu, D. M., Bronckers, A. L., Amerongen, A. V., et al. (2003). Continuous-release or burst-release of the antimicrobial peptide human lactoferrin 1-11 (hLF1-11) from calcium phosphate bone substitutes. J. Antimicrob. Chemother. 52, 853–855. doi: 10.1093/jac/dkg443
Stock, S. R. (2015). The mineral-collagen interface in bone. Calcif. Tissue Int. 97, 262–280. doi: 10.1007/s00223-015-9984-6
Sun, S., Tao, J., Sedghizadeh, P. P., Cherian, P., Junka, A. F., Sodagar, E., et al. (2021). Bisphosphonates for delivering drugs to bone. Br. J. Pharmacol. 178, 2008–2025. doi: 10.1111/bph.15251
Sun, Y., Ye, X., Cai, M., Liu, X., Xiao, J., Zhang, C., et al. (2016). Osteoblast-targeting-peptide modified nanoparticle for siRNA/microRNA delivery. ACS Nano 10, 5759–5768. doi: 10.1021/acsnano.5b07828
Takahashi-Nishioka, T., Yokogawa, K., Tomatsu, S., Nomura, M., Kobayashi, S., and Miyamoto, K. (2008). Targeted drug delivery to bone: pharmacokinetic and pharmacological properties of acidic oligopeptide-tagged drugs. Curr. Drug Discov. Technol. 5, 39–48. doi: 10.2174/157016308783769405
Takeuchi, T., Tanaka, Y., Soen, S., Yamanaka, H., Yoneda, T., Tanaka, S., et al. (2019). Effects of the anti-RANKL antibody denosumab on joint structural damage in patients with rheumatoid arthritis treated with conventional synthetic disease-modifying antirheumatic drugs (DESIRABLE study): a randomised, double-blind, placebo-controlled phase 3 trial. Ann. Rheum. Dis. 78, 899–907. doi: 10.1136/annrheumdis-2018-214827
Tang, R. H., Yang, J., and Fei, J. (2020). New perspectives on traumatic bone infections. Chin. J. Traumatol. 23, 314–318. doi: 10.1016/j.cjtee.2020.05.009
Toro, E. J., Zuo, J., Ostrov, D. A., Catalfamo, D., Bradaschia-Correa, V., Arana-Chavez, V., et al. (2012). Enoxacin directly inhibits osteoclastogenesis without inducing apoptosis. J. Biol. Chem. 287, 17894–17904. doi: 10.1074/jbc.M111.280511
Trouillet-Assant, S., Gallet, M., Nauroy, P., Rasigade, J. P., Flammier, S., Parroche, P., et al. (2015). Dual impact of live Staphylococcus aureus on the osteoclast lineage, leading to increased bone resorption. J. Infect. Dis. 211, 571–581. doi: 10.1093/infdis/jiu386
Tucker, K. A., Reilly, S. S., Leslie, C. S., and Hudson, M. C. (2000). Intracellular Staphylococcus aureus induces apoptosis in mouse osteoblasts. FEMS Microbiol. Lett. 186, 151–156. doi: 10.1111/j.1574-6968.2000.tb09096.x
Vega-Vasquez, P., Mosier, N. S., and Irudayaraj, J. (2020). Nanoscale drug delivery systems: from medicine to agriculture. Front. Bioeng. Biotechnol. 8:79. doi: 10.3389/fbioe.2020.00079
Verdrengh, M., Bokarewa, M., Ohlsson, C., Stolina, M., and Tarkowski, A. (2010). RANKL-targeted therapy inhibits bone resorption in experimental Staphylococcus aureus-induced arthritis. Bone 46, 752–758. doi: 10.1016/j.bone.2009.10.028
Vitovski, S., Phillips, J. S., Sayers, J., and Croucher, P. I. (2007). Investigating the interaction between osteoprotegerin and receptor activator of NF-kappaB or tumor necrosis factor-related apoptosis-inducing ligand: evidence for a pivotal role for osteoprotegerin in regulating two distinct pathways. J. Biol. Chem. 282, 31601–31609. doi: 10.1074/jbc.M706078200
von Eiff, C., Bettin, D., Proctor, R. A., Rolauffs, B., Lindner, N., Winkelmann, W., et al. (1997). Recovery of small colony variants of Staphylococcus aureus following gentamicin bead placement for osteomyelitis. Clin. Infect. Dis. 25, 1250–1251. doi: 10.1086/516962
Wang, D., Miller, S. C., Kopeckova, P., and Kopecek, J. (2005). Bone-targeting macromolecular therapeutics. Adv. Drug Deliv. Rev. 57, 1049–1076. doi: 10.1016/j.addr.2004.12.011
Wang, D., Miller, S. C., Shlyakhtenko, L. S., Portillo, A. M., Liu, X. M., Papangkorn, K., et al. (2007). Osteotropic Peptide that differentiates functional domains of the skeleton. Bioconjug. Chem. 18, 1375–1378. doi: 10.1021/bc7002132
Wang, H., Liu, J., Tao, S., Chai, G., Wang, J., Hu, F. Q., et al. (2015). Tetracycline-grafted PLGA nanoparticles as bone-targeting drug delivery system. Int. J. Nanomedicine 10, 5671–5685. doi: 10.2147/IJN.S88798
Wang, J., Hu, J., and Zhang, S. (2010). Studies on the sorption of tetracycline onto clays and marine sediment from seawater. J. Colloid Interface Sci. 349, 578–582. doi: 10.1016/j.jcis.2010.04.081
Widaa, A., Claro, T., Foster, T. J., O’Brien, F. J., and Kerrigan, S. W. (2012). Staphylococcus aureus protein A plays a critical role in mediating bone destruction and bone loss in osteomyelitis. PLoS One 7:e40586. doi: 10.1371/journal.pone.0040586
Wright, J. A., and Nair, S. P. (2010). Interaction of Staphylococci with bone. Int. J. Med. Microbiol. 300, 193–204. doi: 10.1016/j.ijmm.2009.10.003
Wroe, J. A., Johnson, C. T., and Garcia, A. J. (2020). Bacteriophage delivering hydrogels reduce biofilm formation in vitro and infection in vivo. J. Biomed. Mater. Res. A 108, 39–49. doi: 10.1002/jbm.a.36790
Wu, S., Lei, L., Bao, C., Liu, J., Weir, M. D., Ren, K., et al. (2021a). An injectable and antibacterial calcium phosphate scaffold inhibiting Staphylococcus aureus and supporting stem cells for bone regeneration. Mater. Sci. Eng. C Mater. Biol. Appl. 120:111688. doi: 10.1016/j.msec.2020.111688
Wu, S., Zhang, J., Peng, Q., Liu, Y., Lei, L., and Zhang, H. (2021c). The role of Staphylococcus aureus YycFG in gene regulation, biofilm organization and drug resistance. Antibiotics 10:1555. doi: 10.3390/antibiotics10121555
Wu, S., Liu, Y., Lei, L., and Zhang, H. (2021b). An antisense yycF RNA modulates biofilm organization of methicillin-resistant Staphylococcus aureus and pathogenicity in a rat model of osteomyelitis. Antibiotics 10:603.
Yao, C., Zhu, M., Han, X., Xu, Q., Dai, M., Nie, T., et al. (2021). A bone-targeting enoxacin delivery system to eradicate Staphylococcus aureus-related implantation infections and bone loss. Front. Bioeng. Biotechnol. 9:749910. doi: 10.3389/fbioe.2021.749910
Yilmaz, C., Colak, M., Yilmaz, B. C., Ersoz, G., Kutateladze, M., and Gozlugol, M. (2013). Bacteriophage therapy in implant-related infections: an experimental study. J. Bone Joint Surg. Am. 95, 117–125. doi: 10.2106/JBJS.K.01135
Zhang, G., Guo, B., Wu, H., Tang, T., Zhang, B. T., Zheng, L., et al. (2012). A delivery system targeting bone formation surfaces to facilitate RNAi-based anabolic therapy. Nat. Med. 18, 307–314. doi: 10.1038/nm.2617
Zhang, L. J., and Gallo, R. L. (2016). Antimicrobial peptides. Curr. Biol. 26, R14–R19. doi: 10.1016/j.cub.2015.11.017
Zhang, Y., Ma, W., Zhu, Y., Shi, S., Li, Q., Mao, C., et al. (2018). Inhibiting methicillin-resistant Staphylococcus aureus by tetrahedral DNA nanostructure-enabled antisense peptide nucleic acid delivery. Nano Lett. 18, 5652–5659. doi: 10.1021/acs.nanolett.8b02166
Zhang, Y., Wei, L., Miron, R. J., Shi, B., and Bian, Z. (2015). Anabolic bone formation via a site-specific bone-targeting delivery system by interfering with semaphorin 4D expression. J. Bone Miner. Res. 30, 286–296. doi: 10.1002/jbmr.2322
Zhang, Y., Xie, X., Ma, W., Zhan, Y., Mao, C., Shao, X., et al. (2020). Multi-targeted antisense oligonucleotide delivery by a framework nucleic acid for inhibiting biofilm formation and virulence. Nanomicro Lett. 12:74. doi: 10.1007/s40820-020-0409-3
Zhu, C., Qin, H., Cheng, T., Tan, H. L., Guo, Y. Y., Shi, S. F., et al. (2013). ’Staphylococcus aureus supernatant induces the release of mouse beta-defensin-14 from osteoblasts via the p38 MAPK and NF-kappaB pathways. Int. J. Mol. Med. 31, 1484–1494. doi: 10.3892/ijmm.2013.1346
Zimmerli, W., and Sendi, P. (2017). Orthopaedic biofilm infections. APMIS 125, 353–364. doi: 10.1111/apm.12687
Keywords: biofilm, bone infections, bone-targeted therapy, immunity homeostasis, microorganisms, Staphylococcus aureus
Citation: Wu S, Wu B, Liu Y, Deng S, Lei L and Zhang H (2022) Mini Review Therapeutic Strategies Targeting for Biofilm and Bone Infections. Front. Microbiol. 13:936285. doi: 10.3389/fmicb.2022.936285
Received: 05 May 2022; Accepted: 25 May 2022;
Published: 14 June 2022.
Edited by:
Ying Shi, University of Tübingen, GermanyReviewed by:
Xiaofen Liu, Fudan University, ChinaCopyright © 2022 Wu, Wu, Liu, Deng, Lei and Zhang. This is an open-access article distributed under the terms of the Creative Commons Attribution License (CC BY). The use, distribution or reproduction in other forums is permitted, provided the original author(s) and the copyright owner(s) are credited and that the original publication in this journal is cited, in accordance with accepted academic practice. No use, distribution or reproduction is permitted which does not comply with these terms.
*Correspondence: Lei Lei, bGVpbGVpQHNjdS5lZHUuY24=; Hui Zhang, emhhbmdodWkxNDM3QHdjaHNjdS5jbg==
†These authors share first authorship