- 1Department of Agricultural Botany, Faculty of Agriculture (Saba Basha), Alexandria University, Alexandria, Egypt
- 2Department of Biological Science, Faculty of Sciences, King Abdulaziz University, Jeddah, Saudi Arabia
- 3Department of Plant Protection and Biomolecular Diagnosis, Arid Lands Cultivation Research Institute, City of Scientific Research and Technological Applications, Alexandria, Egypt
- 4Department of Stored Product Pests, Agriculture Research Center (ARC), Plant Protection Institute, Sabahia, Alexandria, Egypt
- 5Department of Agricultural Microbiology, Faculty of Agriculture, Zagazig University, Zagazig, Egypt
- 6Center for Food Analysis (NAL), Technological Development Support Laboratory (LADETEC), Federal University of Rio de Janeiro (UFRJ), Cidade Universitária, Rio de Janeiro, Brazil
This study revealed the possible effects of various levels of silver nanoparticle (AgNP) application on plant diseases and soil microbial diversity. It investigated the comparison between the application of AgNPs and two commercial nanoproducts (Zn and FeNPs) on the rhizobacterial population and Botrytis fabae. Two experiments were conducted. The first studied the influence of 13 AgNP concentration on soil bacterial diversity besides two other commercial nanoparticles, ZnNPs (2,000 ppm) and FeNPs (2,500 ppm), used for comparison and application on onion seedlings. The second experiment was designed to determine the antifungal activity of previous AgNP concentrations (150, 200, 250, 300, 400, and 500 ppm) against B. fabae, tested using commercial fungicide as control. The results obtained from both experiments revealed the positive impact of AgNPs on the microbial community, representing a decrease in both the soil microbial biomass and the growth of brown spot disease, affecting microbial community composition, including bacteria, fungi, and biological varieties. In contrast, the two commercial products displayed lower effects compared to AgNPs. This result clearly showed that the AgNPs strongly inhibited the plant pathogen B. fabae growth and development, decreasing the number of bacteria (cfu/ml) and reducing the rhizosphere. Using AgNPs as an antimicrobial agent in the agricultural domain is recommended.
Introduction
Globally, there are increasing applications of silver nanoparticles (AgNPs) based on their unique properties, which affect ecosystems. Some findings suggested population densities of microorganisms in the rhizosphere based on the physicochemical structure of the rhizosphere soil, variations in soil pH, water ability, and chemical traits of plant exudation (Mishra and Kumar, 2009). The rhizosphere is delineated as the area near a plant root, and it is inhabited by a unique population of microorganisms affected. It includes three zones, which are well-defined based on their relative proximity to the root (Hiltner, 1904). The rhizoplane can be defined as the medial zone, immediately adjacent to the root with the root epidermis and mucilage (Hartmann et al., 2008). It is the thin area of the soil or substrate directly affected by the secretions of roots and the linked soil microorganism identified as the root microbiome. It comprises several kinds of bacteria and other microorganisms, some are harmful, and others are helpful to crops (Lovern and Klaper, 2006; Hartmann et al., 2008).
The control and understanding of sizes are roughly called nanotechnology, with a size range of 1–100 nm (Klaine et al., 2008). Recently, nanoparticles (NPs) have accepted enhanced attention because of their exclusive effects. NPs are assessed for their toxic impacts on activity, abundance, and diversity of flora and fauna (Nayak et al., 2013; Zarina and Nanda, 2014; Gomaa et al., 2021; Alsalem et al., 2022). Several studies have stated that NPs involve microscopic properties of soil, such as humic acid matter and soil bacterial community (Ben-Moshe et al., 2013). Previously, its release in the ecosystem impacted microbial variety, and fungi interactions can also affect plant growth (Kumar et al., 2012; Hassan et al., 2022). Increased concentration of NPs can also impair normal microflora growth. It could also have toxic effects on various organisms (Abdelsalam et al., 2019b; Zhao et al., 2020).
Numerous NPs have already been recorded to have antimicrobial properties and directly influence microorganisms and other crop performance (Fouda et al., 2020a; Ghareeb et al., 2020; Youssef et al., 2020). Microbial toxicity has been reported for some NP products (Lovern and Klaper, 2006). “Plant growth-promoting rhizobacteria” (PGPR) are native to the plant rhizosphere and play a role as a bio-control agent for anti-plant pathogens (Khan et al., 2018). Also, PGPR raises the phytohormone production and enhances the lateral root branches. It modifies the root functions and improves plant nutrition to enhance plant physiology (Adesemoye and Egamberdieva, 2013; Abdel-Aziz et al., 2014). They play an essential role in nitrogen fixation (Yang et al., 2009; Khan et al., 2018).
Botrytis spp. is a common plant pathogen with more than 235 plants serving as hosts, including legumes, strawberries, and orchids. AgNPs are considered an inhibiting agent against microorganisms (Sondi and Salopek-Sondi, 2004; Majeed et al., 2014) and can be used as alternatives to fungicides (Kim et al., 2012) including Alternaria alternata, Botrytis spp., and Fusarium sp. in different crops. Also, the previous studies (Sondi and Salopek-Sondi, 2004; Elkobrosy et al., 2022) clarified that AgNPs could damage bacterial cells.
AgNPs are the most widely used in consumer products and medical applications. The antibacterial and antifungal properties of AgNPs may lead to a greater risk to humans and environmental health (Zhao et al., 2019; Kandil et al., 2020). NPs can accumulate in the soil due to their technological activities, involving deliberate releases by soil and water remediation (Nayak et al., 1998; Lakshmipathy and Nanda, 2013; Nayak et al., 2014). The effect of AgNPs on microorganisms can be identified through the measurement of the soil’s respiratory and enzymatic activities (Sompornpailin et al., 2014; Simonin and Richaume, 2015). The results found by Cao et al. (2016) showed that a high concentration of Fe3O4 NPs significantly decreased bacterial content in the soil. In addition, zinc oxide and CeO2 NPs influence the plate counts of Azotobacter (Chai et al., 2015).
The same results with titanium dioxide NPs were noticed. The data reported by Maliszewska (2016) proved that the AuNPs up to 33 mg kg–1 do not affect soil processes. However, the antibacterial activities in the inhibition zone were detected on some bacterial isolates treated with ZnO NPs and produced by biological methods (Venkataraju et al., 2014). AgNPs are one of the most widely used nanomaterials (Khan et al., 2018). Silver and/or silver ions have powerful effects, such as killing and inhibiting bacteria. AgNPs could reduce plant diseases and pests (Shams et al., 2013) and improve seed germination and growth (Thuesombat et al., 2014).
Therefore, this study examines the negative or positive applications of AgNPs compared with the two commercial nanoproducts (Zn and Fe NPs) on the rhizobacterial and B. fabae population.
Materials and Methods
Preparation of Applications of Silver Nanoparticles
AgNPs were received at 2,000 ppm, as per the datasheet provided by the company, and serial dilution with deionized water was conducted to prepare the following working concentrations: 5, 10, 15, 20, 25, 30, 40, 50, 60, 70, 75, 80, 100, 200, 300, 400, and 500 ppm.
Characterization of Applications of Silver Nanoparticles
First, “UV-vis. TG 0; Germany” depicted the absorbance band AgNPs. Notably, AgNPs display a great absorption peak that could be subjected to surface plasmon excitation. The particle shape and distribution were determined using a “transmission electron microscope”: “TEM; JEOLJEM-1230; Japan.” The LINS (AgNPs) stock-concentrated solutions were centrifuged at 20,000 rpm for 60 min to produce AgNPs in powder form. The obtained AgNPs powder was observed using “scanning electron microscopy”; SEM: Quanta 400, Oxford, United Kingdom.
Experiment Parts
The selected plants are collected from the in-house Alexandria University Farm allocated at the Alexandria University campus for Research and Development only. Different experiments were conducted at the Faculty of Agriculture (Saba Basha), Alexandria University, Alexandria. First, an experiment was conducted to complete the previous research on the influence of a high amount of green synthesized AgNPs on agronomic traits of onion using different concentrations of AgNPs as 5, 10, 15, 20, 25, 30, 40, 50, 60, 70, 75, 80, and 100 ppm. In addition, two commercial nanoparticle products, ZnNPs (2,000 ppm) and FeNPs (2,500 ppm), were published by the same author (Fouda et al., 2020b) to study the effects of AgNPs on the soil bacterial diversity in the rhizosphere area of onion roots, which was treated with previous AgNP concentration by collecting the soil beside the root area in size 200–250 g/sample and mixed well, and then, it was transferred to the microbiology laboratory. The soil samples were organized by suspending 10-g soil in 100-ml sterile distilled water, shaken (30 min, 200 rpm), and filtered on cellulose filters (Filtrak, Germany). The addition of silver nanoparticles (AgNPs) to environmental samples (during the plant growth stage to the soil through three adding times) was 25, 40, and 55 days.
In vitro Experiment
Composition of Nutrient Agar Media
Nutrient agar concentration was prepared by adding current components such as 0.5% peptone, 0.3% beef extract, 15% agar, and 0.5% NaCl and then dissolved in distilled water with stirring under the head to fully dissolve the components (pH = 7.4) at 25°C. The mixture was autoclaved for 15 min at 121°C.
Bacterial Count Experimental Procedure
Five (9 ml) sterilized distilled water tubes were labeled as 10–3, 10–4, 10–5, 10–6, and 10–7. The primary dilution was produced by transferring a 1-ml sample from the flask (which dilution is 10–2) to 99-ml sterilized distilled water (1/1,000 or 10–3 dilution). After the 10–3 dilution was shaken, 1 ml was transferred to the second 9-ml tube, which was represented as a 10–4 dilution of the original sample. The same steps were performed for the 10–5, 10–6, and 10–7 dilutions. Then, 1-ml sterilized tips were carefully added to each plate and incubated. Finally, all Petri plates containing 30 and 300 colonies were calculated.
The calculation of the bacterial number (CFU)/milliliter of the sample is as follows:
The decrease in microorganism number® (total and species) due to nanoparticle treatment was calculated using the equation:
where N: N is the number of microorganisms in the sample with nanoparticles (CFU/100 ml), and N0 is the number of microorganisms without nanoparticles (CFU/100 ml).
In vivo Experiment
Experimental Setup
The Effect of Tested Nanomaterial Concentration on Bacterial Community. In this part of a joint study, we spiked an agricultural soil with AgNPs with the most effective concentrations (80 and 100 ppm) as mentioned in previous in vitro experiment compared with two commercial nanoparticles: ZnNPs (2,000 ppm) and Fe NPs (2,500 ppm), and the effects of these NPs on onion (Allium cepa) combined with Pseudomonas putida as phosphate solubilizing bacteria, and whether these effects depended on the concentrations of the applied NPs.
Onion (Allium cepa) single seedlings were transferred in plastic pots (11.5-cm deep and 17 cm in diameter) filled with 2,000 g growth-autoclaved substrate (50% peat moss and 50% sand) and fertilized twice per month with 100-mL/plant mineral fertilizer. NPs were mixed with soil with four different concentrations. Onion seedlings were inoculated with PGPRs and their combinations with and without NPs. All pots were irrigated with tap water daily to keep the soil at 70% of its field capacity by weighing the pots regularly. Seedlings were collected to measure the effect of NP treatment on some vegetative characters other than shoot and root biomass. Data were subjected to the statistical analysis described in “Data confirmation and validation.”
Preparation of Bacterial Inocula. One strain of PGPR (Pseudomonas putida; ATCC17453) was obtained from the Bio-fertilization unit, Faculty of Agriculture, Ain Shams University, Cairo, Egypt. The bacteria were reproduced on Luria Bertani (LB) medium comprising (g/L) tryptone, 10; yeast extract, 5; NaCl, 5. The pH of the medium was adjusted to 7.2–7.4 using 1-N HCl or 1-N NaOH and sterilized by autoclaving at 121°C for 15 min, L.B., media were inoculated for 24 h and incubated in a shaking incubator at 30°C for 72 h. The inocula were centrifuged at 3,000 rpm for 10 min. The supernatant was removed, but the pellet was suspended in distilled water to change the optical density at 660 nm, equivalent to 106 cells per ml. Then, 30-day-old seedlings were soaked in bacterial inocula for 2 h, whereas control seedlings were soaked in autoclaved LB broth for 2 h. The seedlings were sown in pots combined with different kinds of nano-treatments. The seedlings were then allowed to grow under natural conditions. The AgNPs were donated by Fouda et al. (2020b).
Growth Parameters and Chlorophyll Concentration Index. After 8 weeks of onion growth, branches and leaves number were calculated. Fresh and dry weight of shoots and roots were assessed after 2 months of seedling growth. Plants were collected after 60 d of planting. Before collection, the chlorophyll concentration index was measured using a chlorophyll meter (Model: CCM-200, Hudson, NH 03051, United States).
The Effect of the Tested Applications of Silver Nanoparticle Concentration on Botrytis fabae in vitro Experiment. The antifungal activity of AgNPs was determined against B. fabae under laboratory conditions. The antifungal activity was calculated as the inhibition percentage in the growth relative to the control treatment. Potato dextrose agar (PDA) spiked with AgNPs at 26 concentrations (5, 10, 15, 20, 25, 30, 35, 40, 45, 50, 55, 60, 65, 70, 75, 80, 85, 90, 95, 100, 150, 200, 250, 300, 400, and 500 ppm) was used. In addition, Ridomil Gold was used as the negative (non) and positive control (mz 68 wg, Company: Syngenta, United States, Active ingredients: Mefenoxam + Mancozeb) and was poured into Petri dishes (9-cm diameter: 15 ml/dish). Mycelial disks of 5-mm diameter were cut from the leading edge of 7-day-old fungal cultures (B. fabae) and used for inoculation into Petri dishes containing the corresponding culture media (PDA) and the different treatments. Agar plugs of 5-mm diameter were achieved from the actively growing B. fabae inoculated into the center of plates supplemented with various treatments. The incubation time for the plates at 28°C was extended until the full growth of B. fabae (mycelium reaching the edge of the plate) in the untreated control group. Colony diameters were measured at the time of full growth in the control. Control plates were inoculated with B. fabae in a growth medium without AgNPs. The percentage of inhibition was measured compared with the control using the next formula:
where R and r are the radial growth of fungus in control and treated plates, respectively.
In vivo Experiment. The efficacy of AgNPs against B. fabae was determined under greenhouse conditions. Faba bean seedlings were grown in plastic pots randomly arranged for each treatment. Five replicates were used in this experiment. Based on the achieved data, four successful concentrations of AgNPs solution (100, 200, 300, and 400 ppm) were applied to the leaves of plants. For an inoculation pretreatment, AgNPs were sprayed on thirty-day-old plants. After that, the plants were inoculated with conidial suspensions (105 conidia/ml), and the inoculated plants were coated with plastic for 12 h. The distilled water was used as a control, and the fungicide was used as Ridomil Gold (mz 68 wg, Company: Syngenta, United States, Active ingredients: Mefenoxam + Mancozeb). Ten days after treatment, disease severity (DS) was calculated using the disease scale from 0 to 5 suggested by ICARDA (2005), where 0 means the plants without chocolate spots, one means the leaf with a few chocolate spots, two means the number of spots increased, and scattered, three means the spots combined and became larger, four means the necrotic spots reached half of the leaf, and five means that most of the leaves were necrotic and dropped. The disease severity index (DSI) percentage was calculated according to Chiang et al. (2017) as follows:
After planting, samples were taken for 45 d to estimate growth parameters as chlorophyll index, shoot and root lengths, shoot, fresh root weights, and shoot and root dry weights.
Statistical Analysis. One-way ANOVA (p < 0.05) of the in vivo experiment was conducted by Tukey’s HSD (significant difference) and at p ≤ 0.05 to detect significant interactions between factors. Randomized block design (split-plot) in three replicate nanoparticles and control, AgNPs 80 ppm, AgNPs 100 ppm, Zn NPs 2,000 ppm, and Fe NPs 2,500 ppm were the main plot, and the two treatments uninoculated and inoculated with Pseudomonas putida were randomly distributed in the sub-plot; all data are shown as mean values. The reduction in the bacterial count due to AgNPs treatment was calculated using equation (1), where “N” is the number of microorganisms in the samples with NPs. Statistically significant differences were observed among control samples (without AgNPs) and samples with AgNPs.
Results
Characterization of Silver Nanoparticles
To characterize the commercial silver nanoparticles, TEM was applied to detect the particle shape of AgNPs. It was observed at two different magnifications (Figure 1). The evaluation was conducted by diluting the original sample, LINS, with deionized water followed by ultrasonication—for 5 min at room temperature. It was depicted that the particles’ shape was small and spherical size (around 40–60 nm). The concentrated sample obtained from the company makes it feasible for industrial application in several domains.
Impact of Applications of Silver Nanoparticles on the Number of Bacteria (cfu/ml) and Reduction Percentage
In vitro Experiment
The recording results demonstrated the impact of different AgNP concentrations on total count soil bacteria isolated from soil samples compared with some commercial products, that is, ZnNPs and FeNPs. As shown in Table 1 and Figures 2, 3, the highest number of bacteria was in control (untreated) soil samples, 152.50 × 105 cfu/ml forward by the samples treated with Fe and Zn (2,500 and 2,000 ppm), and it was 41.30 × 105 and 40.00 × 105, respectively. At a concentration of 80 ppm, bacteria were susceptible to AgNPs with the highest reduction percentage of 97.37% compared with the other two commercial products, which showed 73.77 and 72.92%, respectively, for Zn and FeNPs (Table 1 and Figures 2, 3).
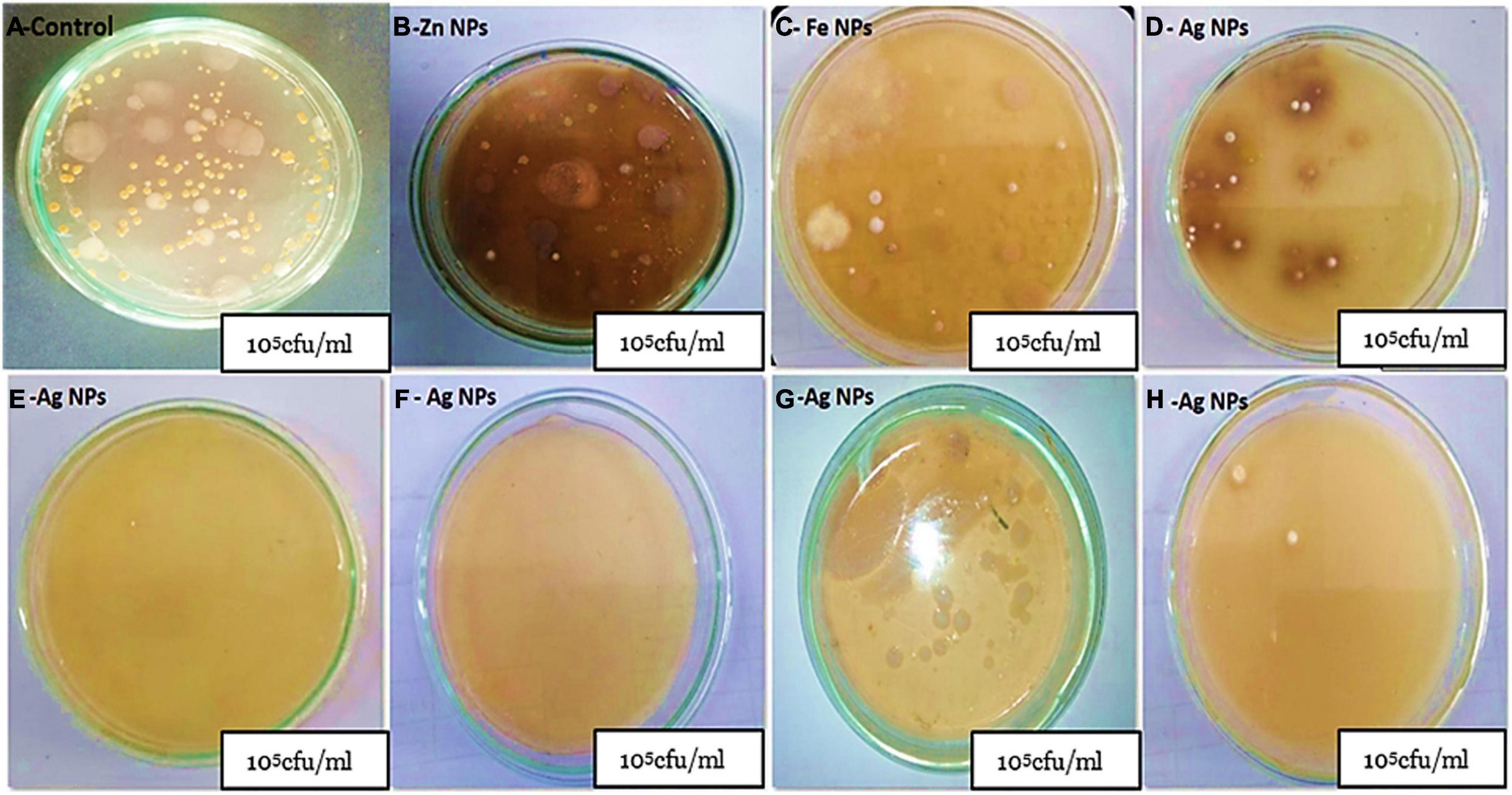
Figure 3. Calculation of the number of bacteria (cfu) per milliliter at soil samples treated with AgNPs with different concentrations compared with commercial products Zn and FeNPs. (A) Total bacteria cfu/ml as control, (B,C) total bacteria cfu/ml treated with Zn and FeNPs, respectively, (D) total bacteria cfu/ml treated with 30 ppm Ag NPs, (E,F) samples with low total count cfu/ml with 80 and 50 ppm Ag NPs, respectively, (G) total bacteria cfu/ml treated with 100 ppm AgNPs, and (H) samples treated with 5 ppm AgNPs.
However, a growth (in CFU) was seen on mostly low concentration AgNPs. Ag NPs decreased the bacterial number when using all concentrations with a percentage of almost 90%. The number of colonies formed on nutrient agar media was observed for nanoparticles at different concentrations as Zn and Fe nanoparticles. The present data showed no differences in the soil samples exposed to Zn and Fe NPs for the number of colony-forming units. Alternatively, the highest values for all AgNPs samples were 154.67 × 104 cfu/ml demonstrated under 30 ppm concentration even though the samples with five ppm concentration recorded 77.00 × 104 cfu/ml. Still, viability count is not a reliable method for evaluating the toxicity of nanoparticles on soil diversity.
Figures 2, 3 represent the different reduction percentages of microbial communities in soil treated with Ag, Zn, and FeNPs, compared with control. The microbial communities from the incubated soil sample with a combination of low concentrations of AgNPs indicated a high reduction in using two substrates (Zn and Fe) compared with the control samples. A decrease in AgNPs use was recorded from the samples treated with a low concentration of 97.0, 96, 95.08, and 94.9% with concentration values of 15, 10, 20, and 5 ppm, and between the high concentration of AgNPs, the high reduction recorded in 50 and 60 ppm, respectively, was 97.18%. The highest decrease in bacterial numbers (R = 97.37%) was observed with the soil sample treated with 80 ppm AgNPs, while the lowest was with the iron soil samples (R = 72.92%) (Table 1 and Figure 2).
In vivo Experiment
Table 2 shows significant differences in the number of onion leaves and branches between inoculated and uninoculated plants under all different levels of nanoparticles. Data in Table 2 showed that the number of leaves increased in all inoculated plants with P. putida than in uninoculated plants. The maximum increase in leaf number appeared in AgNPs control plants in the case of inoculated plants with P. putida (24.67). However, the number of branches has the highest value in inoculated plants treated with 100-ppm AgNPs (4.67).
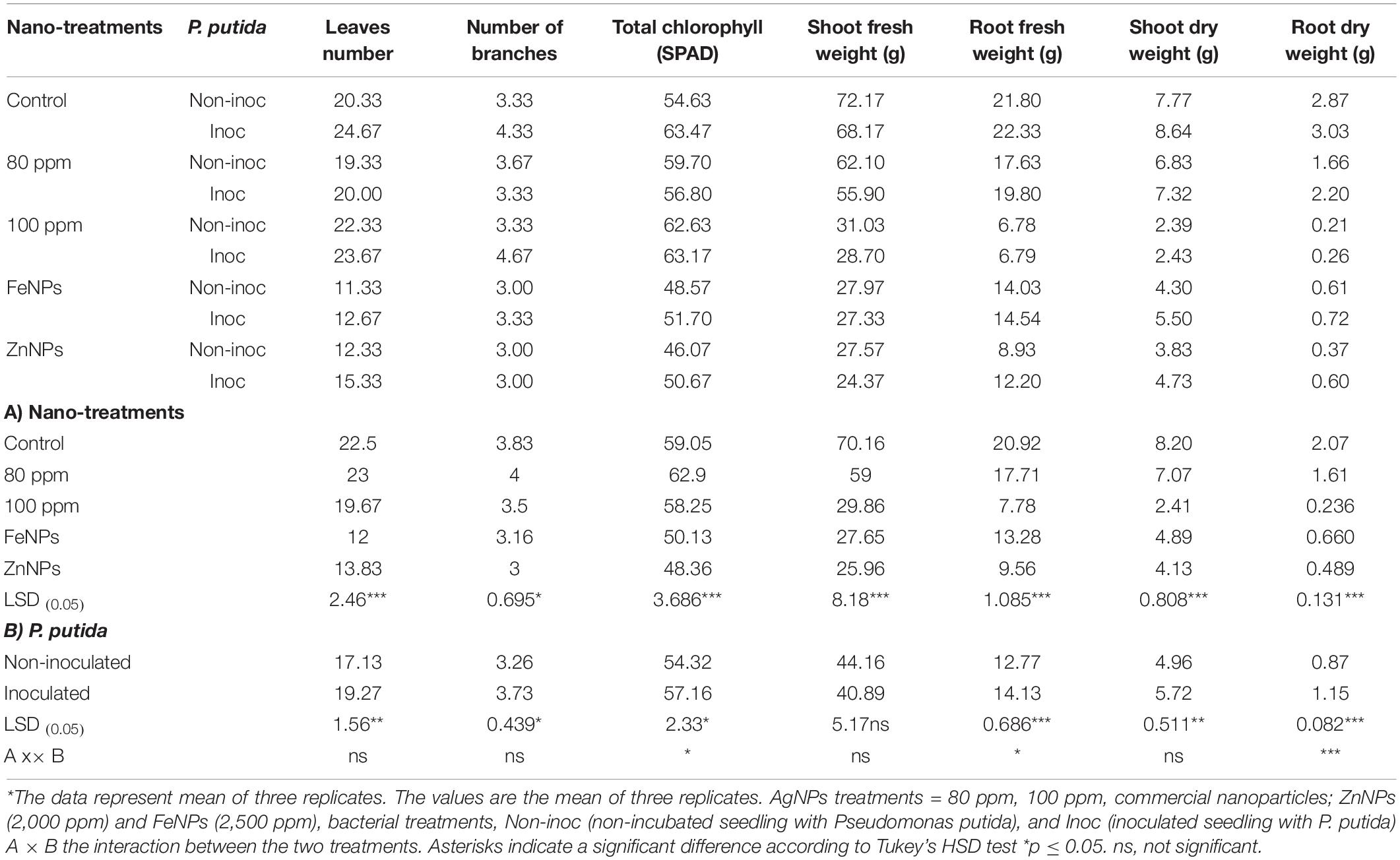
Table 2. Effects of PGPR and different NPs on leaves number, number of branches, total chlorophyll (SPAD), shoot, and root parameters after 8 weeks of seeds germination in onion plants.
The greenhouse experiment confirmed the data, which indicated that the nanoparticles decreased the number of leaves and branches. The highest decrease was recorded in plants treated with Fe NPs (2,500 ppm) concentration in the case of non-inoculated plants with P. putida were 11.33 and 3, formed for the numbers of leaf and branch, respectively. Moreover, Table 2 could conclude that the interaction between the nanoparticles treatments and bacterial inoculation had no significant impact. The total chlorophyll content was increased compared to control (non-treated plants with NPs) and compared with inoculated and uninoculated with Pseudomonas putida (Table 2). The maximum total chlorophyll content was 63.47. A similar response was observed in 100-ppm Ag NPs concentration. By contrast, the two commercial nanoparticles, Zn NPs (2,000 ppm) and FeNPs (2,500 ppm), recorded the lowest chlorophyll content (46.07 and 48.57), respectively (Table 2). The interaction between inoculation and nanoparticles with different concentrations had a significant difference. In addition, there is a variate impact between the four nanoparticles’ concentration and control.
As revealed in Table 2, there remained a significant difference in both shoot and fresh root weight of onion leaves after 8 weeks of applications for all treatments. The fresh weight in control untreated with any kind of nanoparticle was 70.16 and 20.92 g/plant for shoot and root, respectively, while it was 59 and 17.71 g/plant at the lowest concentrations of AgNPs 80 ppm. However, the highest concentrations (100 ppm) decreased the value of shoot and fresh root weights after application. Furthermore, the application of Zn and FeNPs with concentrations (2,000-ppm Zn and 2,500-ppm Fe) had the lowest fresh weight in the case of plants inoculated or uninoculated with growth-promoting rhizobacteria (PGPR) Pseudomonas putida.
However, the interaction between bacterial inoculation and nanoparticle treatment recorded a significant difference in root fresh weight; also, no significant differences in shoot fresh shoot weight were observed. Overall, it can observe that there was a direct relationship among the high concentration of nanoparticles, even AgNPs or the commercial Zn and Fe NPs. However, the fresh shoot weight showed non-significant differences between bacterial inoculation and the interaction between all treatments. On the contrary, plants treated with different types and concentrations of AgNPs had a significant impact.
The results obtained from Table 2 showed that the impact of AgNPs can be partially affected by shoot and dry root weights under the high concentration, and it is completed by decreasing the value from 100 to 80 ppm. Further increase in AgNPs decreased the plant biomass related to the strong antibacterial activity. By contrast, control plants observed the highest biomass growth. The maximum shoot dry weight was observed in plants inoculated with P. putida in case of plants untreated with any NPs. However, 100-ppm AgNPs give the highest value compared to other commercial nanoparticles (Zn NPs and FeNPs). The root dry weight had the same results, and the highest concentration of AgNPs decreased bacterial growth, followed by a decrease in the onion plant biomass. Data in Table 2 recorded that a significant change was observed in the shoot and root dry weight of onion plants within treatments of nanoparticles and treatments of the bacterial inoculation. Moreover, the interaction between both factors had the same result.
Impact of Applications of Silver Nanoparticles on Botrytis fabae
Impact of Applications of Silver Nanoparticles on Growth Inhibition Percentages of Botrytis fabae
The radial growth technique is used to check the potential of AgNPs for suppressing the development of B. fabae compared with chemical fungicide (Ridomil Gold, Syngenta, United States) and control during this study. The AgNPs with different concentrations potentially suppressed B. fabae development compared with the control group. The same previous concentrations from 5 to 100 ppm were used during this study.
The radial growth (cm) of B. fabae decreased with the increase in AgNP concentration, and the results were divided into some groups, starting from a range from 7 to 8 cm including the concentrations of 5, 10.15, 20, 25, and 30 ppm; the second group was from 6 to 7 cm and included the 35, 40, 45, 50, and 55 ppm concentration; the third group was from 5 to 6 cm and counting the AgNP concentration of 60, 65, 70, 75, and 80 ppm; the fourth group from 2 to 4 cm and includes 85, 90, 95, and 100 ppm; the fifth group from 1 to 2 cm and includes 150, 200, 250, 300, 400, and 500 ppm comparing with control, which showed 90 mm (9 cm) for the full Petri dish as found in Figure 4. In addition, the data for commercial fungicide showed 1.07 cm.
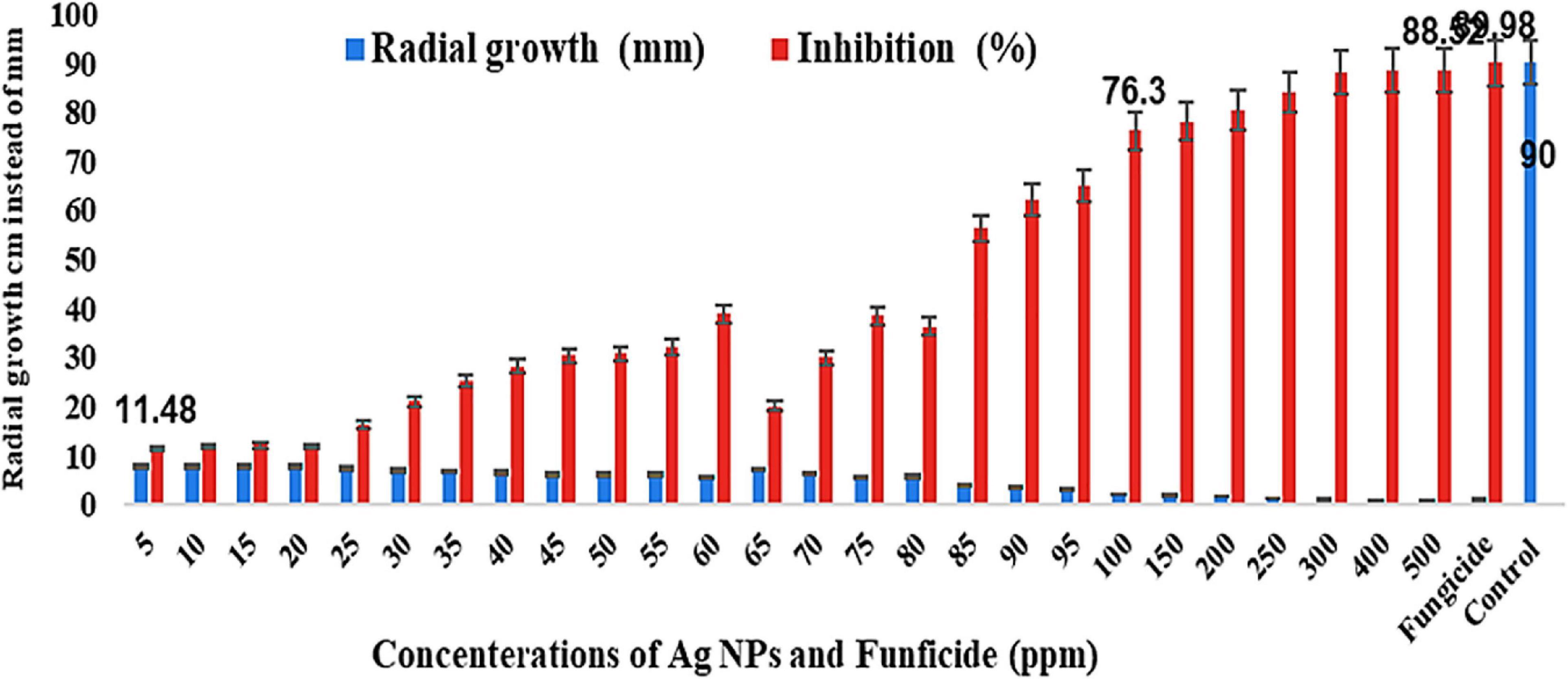
Figure 4. Effect of different AgNP concentrations on growth inhibition percentages of B. fabae under laboratory conditions compared with fungicide and control.
Alternatively, the inhibition (%) increased from the lowest to highest concentrations and started from 11.48% (5 ppm) to 76.30% (100 ppm) as found in Figures 4, 5 and jumped from 78.15% (150 ppm) to 88.52% (500 ppm) (Figures 6, 7), besides these values of the chemical fungicide showed 89.98% (Figure 7) compared with control. Nevertheless, the greatest growth inhibition percentage of B. fabae was reached at the greatest concentration (500 ppm). The growth inhibition percentage of B. fabae treated with AgNPs ranged from 11.48 to 88.52, while fungicide was the most effective treatment against the fungus with 93.7% (Figures 4–7). AgNPs activity on B. fabae increased with increasing concentration. The results also showed no difference between 400 and 500 ppm on suppressing the effect on the radial growth of the fungus.
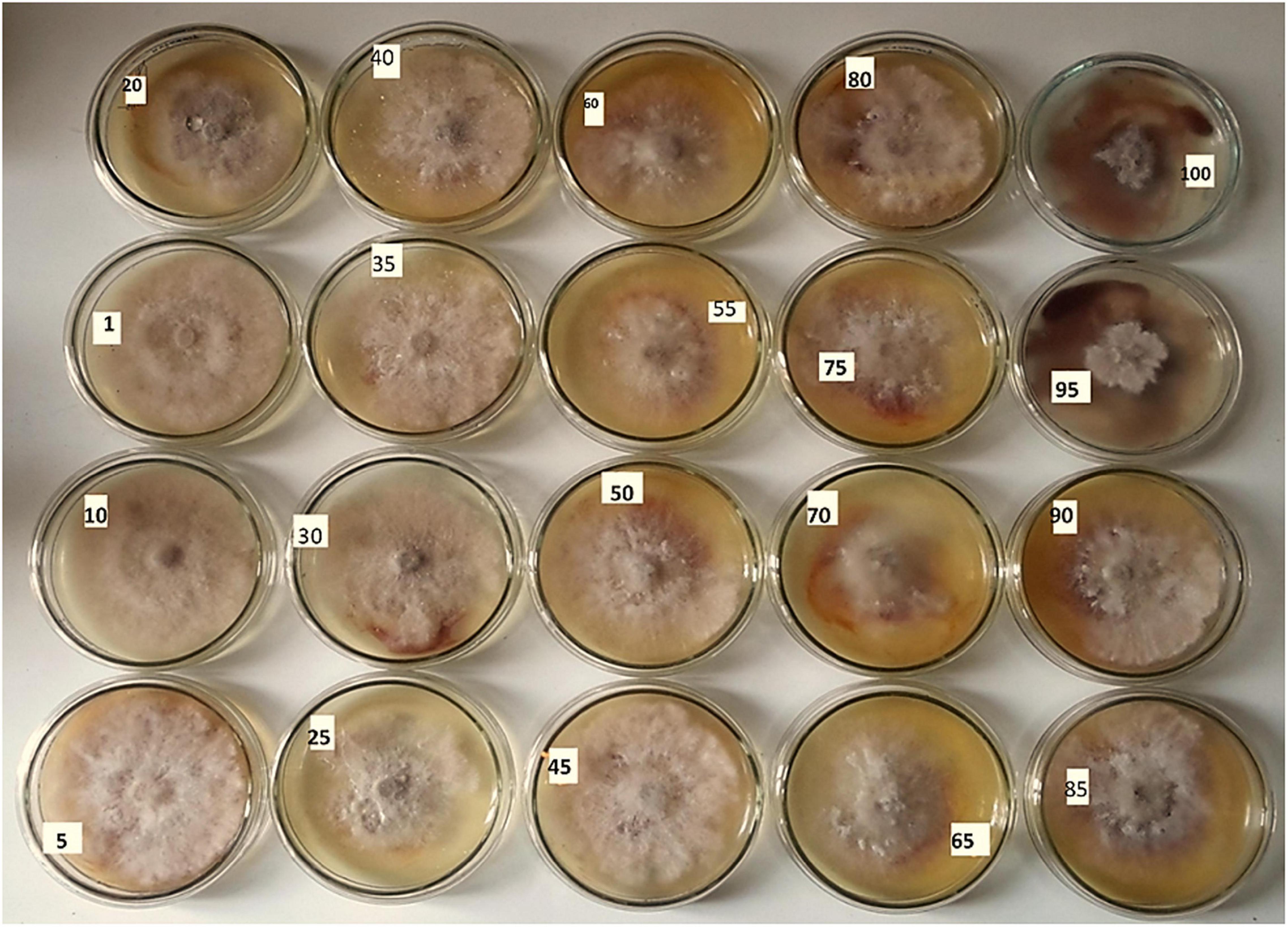
Figure 5. Antifungal activity of the AgNPs against Botrytis fabae of the concentrations of 5, 10, 15, 20, 25, 30, 35, 40, 45, 50, 55, 60, 65, 70, 75, 80, 85, 90, 95, and 100 ppm.
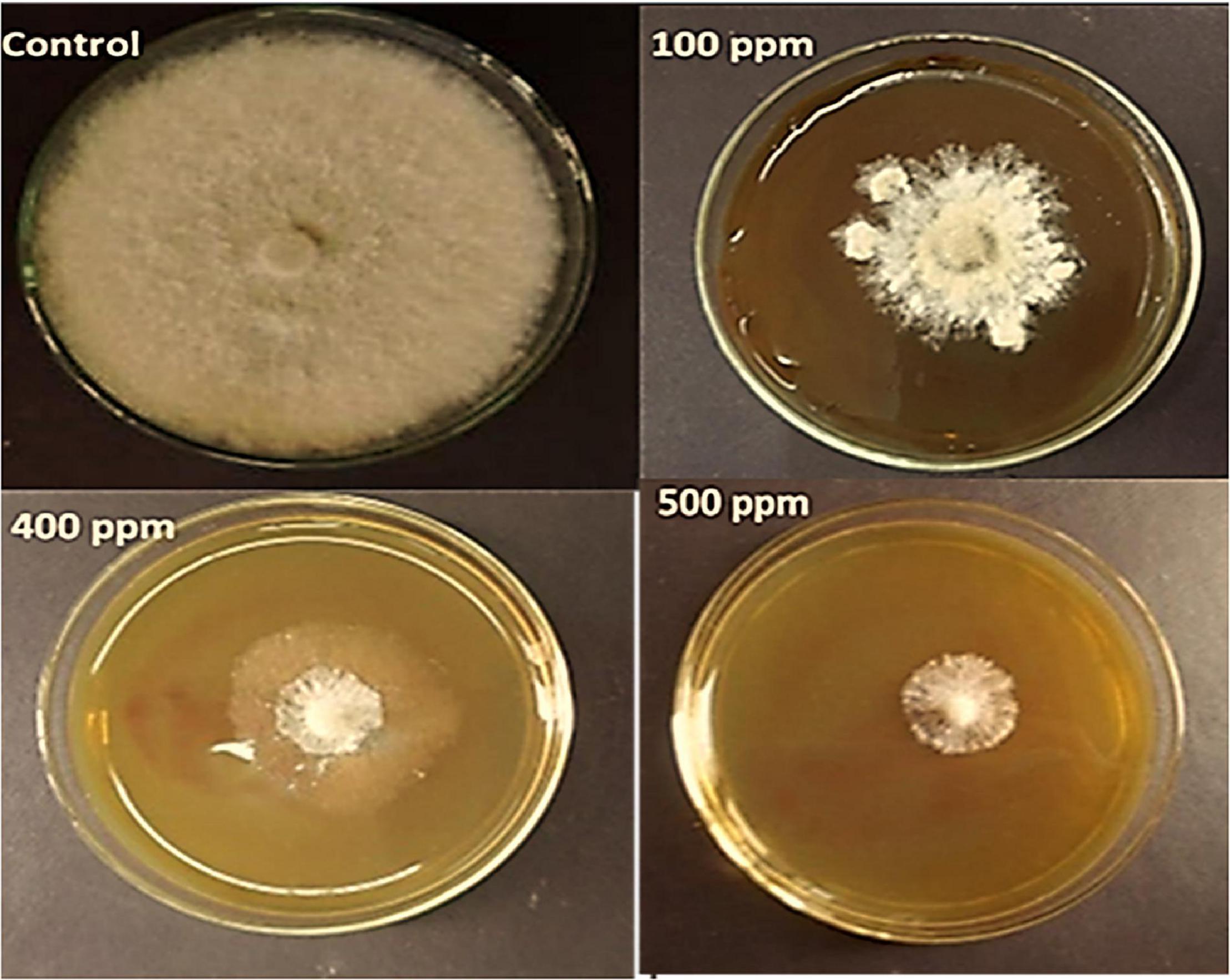
Figure 6. Antifungal activity of the AgNPs against B. fabae of the concentrations of control, 100, 400, and 500 ppm, respectively.
Disease Severity Index
Data in Table 3 show that AgNP spraying of faba bean plants with the four evaluated AgNP concentrations (100, 200, 300, and 400 ppm) had an effective role against leaf infection with B. fabae. The DS, expressed as the percentage, was significantly reduced by the AgNPs 400 ppm to 9.33%, which is insignificantly different from the fungicide treatment (8.0%) with disease reduction (89.39 and 90.91%, respectively). However, the DSI% value for the non-treated control reached 88.0%. All tested AgNP concentrations effectively reduced the DSI% values on infected faba bean plants, where the efficiency of the concentration (100, 200, and 300 ppm) was 29.33, 22.67, and 14.67, with disease reduction of 66.67, 74.24, and 83.33%, respectively. Thus, the obtained results showed that all applied concentrations of AgNPs reduced the disease severity index (DSI) caused by B. fabae compared with infected control plants.
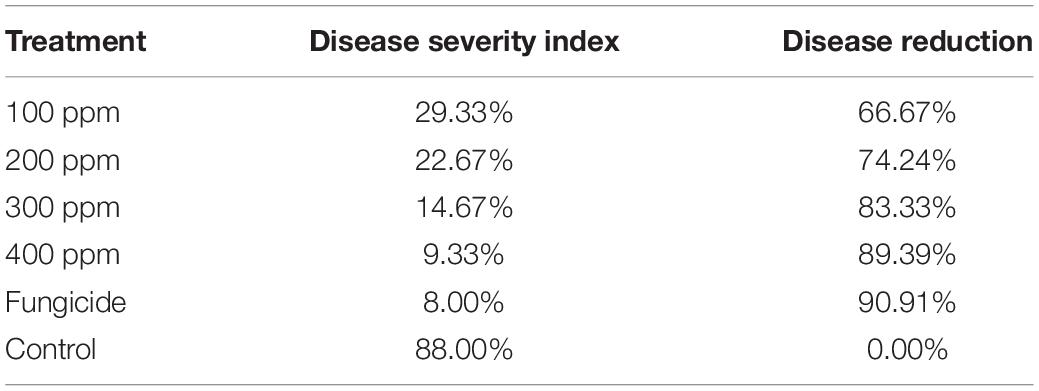
Table 3. Effect of different AgNP concentrations on disease severity and disease reduction in brown spot of faba bean under greenhouse conditions.
Impact of Applications of Silver Nanoparticles on Growth Parameters of Faba Bean
Data presented in Table 4 show that the chlorophyll content increased gradually with an increase in AgNP concentration from 100 to 400 ppm compared with fungicide and non-treated control. Compared to infected control, all tested concentrations significantly increased the chlorophyll content in faba bean leaves infected with B. fabae. The highest chlorophyll value was obtained from 400 ppm (53.76) followed by 300, 200, and 100 ppm) (49.60, 48.08, and 45.66, respectively), while the fungicide treatment was 46.06 compared with the infected control (29.60). In contrast, AgNPs increased the shoot and root lengths compared with fungicide and infected control. The highest value was obtained from 400 ppm for shoot length (35.34 cm) and root length (9.90 cm) compared with the infected control, 18.68 and 6.88 cm for shoot and root, respectively.
Data showed that spraying faba bean plants with AgNPs significantly affected fresh shoot and root weight (Table 4). The highest shoot and fresh root weights were 27.02 and 4.18 g, respectively, observed under 400 ppm AgNPs, superior over infected control, which was recorded at 19.89 and 3.22 g for shoot and root, respectively. The maximum dry weight of shoot was 3.51 g from 400 ppm of AgNPs, while the highest dry weight of root was recorded from 300 ppm of AgNPs (1.08 g), superior over other varieties. The minimum plant height was noticed with the mulch cultivar. In contrast, the minimum dry weight of shoot and root (1.93 and 0.79 g, respectively) was noticed with infected control plants.
Discussion
The main core of this study was designed following the two steps. The first one is to apply an Egyptian commercial nanoproduct called LINS, which is based on AgNPs, in the agricultural domain, in a term as the antibacterial, and the second one as an antifungal agent, to overcome the problems associated with roots, namely “microbial community” and the common plant diseases, B. fabae. This study aimed to discover the best concentration of LINS (AgNPs) that can kill both the pathogenic bacteria affecting the root system and the common plant disease resulting from the presence of the dangerous fungi; B. fabae.
Bacteria are considered the predominant group of microorganisms in the environment with various types. And some are harmful, and others are helpful in the rhizosphere. The bacterial species are crucial soil components, which maintain several biotic activities of the soil ecosystem to produce it suitable for nutrient uptake and sustainable crop production (Gruyer et al., 2014). From the data, AgNPs efficiently enhanced the number of branches/plants but reduced PGPR effectiveness in producing branches. The number of leaves was increased in response to Pseudomonas putida inoculation in 100-ppm AgNPs dose.
Also, Nawaz and Bano (2020) evaluated the role of (PGPR) P. putida and AgNPs in two cucumber varieties. They reported that both varieties successfully increased shoot length at the maturity phase, while when using AgNPs, shoot length was reduced. This result concluded that P. putida were affected by various nanoparticle applications even Ag, Zn, or Fe types or their different concentrations. The toxic impacts on microbial communities depend on the kind of NP considered (Simonin and Richaume, 2015).
This study is in harmony with Xu et al. (2004), who reported that AgNPs could enhance the permeability of bacterial cell membrane, penetrate the cytoplasm, and deactivate vital respiratory enzymes and proteins responsible for RNA and DNA replication, causing bacterial death (Morones et al., 2005). In addition, the antibacterial activities of AgNPs are frequently based on the high concentration since the random physical collision of silver nanoparticles with the bacterial surface causes the penetration of silver nanoparticles into the cytoplasm (Lara et al., 2011). Usually, the antimicrobial agents target single or multiple sites in the microbial cell, and the antibacterial damage these target sites.
Various Ag NP applications, such as 0.0032, 0.032, and 0.32 mg kg–1 soil, reduced microbial biomass dose response (Hänsch and Emmerling, 2010). However, soil pollution with iron oxide magnetic NPs did not affect microbial biomass with 10 and 100 mg kg–1 soil (Vittori Antisari et al., 2013). Also, Simonin et al. (2015) found no significant impact of TiO2 NPs on bacterial abundances in the six tested soils.
It was well recognized that AgNPs exhibit great antibacterial activity because of their well-developed surface, which provides maximum contact with the environment. Here, the antibacterial effect of AgNPs was studied using the total count of bacterial numbers as described in the in vitro experiment with different concentrations of AgNPs and commercial Zn and Fe NPs. P. fluorescence was reported to increase plant height and the number of flowers of tomato plants and crop growth (Abdelsalam et al., 2022; Choudhary et al., 2022) because it plays a vital role in the growth and metabolism of the plant (Somers et al., 2004).
Our results are similar to Laware and Raskar (2014) and Abbas et al. (2022) reported that onion plants treated with different concentrations of ZnO NPs showed no significant increase in plant height, but the leaf number was significantly increased in all treatments. The highest number, that is, 23.14, was displayed in plants treated with 30-μg ml–1 ZnO NPs compared to control plants.
Based stimulation could trigger advantageous effects of PGPRs and can increase crop yield (Karunakaran et al., 2013). Using ZnNPs (2,000 ppm) and FeNPs (2,500 ppm) decreased the plant growth, as found in Table 2. These results agree with Timmusk et al. (2017) who found that wheat biomass did not significantly increase with nano-Titania (TNPs) treatment associated with PGPR. Moreover, these PGPRs stimulate plant growth and improve plant biomass (Adesemoye et al., 2009; Ahmad et al., 2022). Differences in the chlorophyll concentration in the plant could be due to several interactions of allelochemicals present in the plant extracts (Talukder et al., 2015).
P. putida increased the chlorophyll a in Poinsett 76, whereas P. stutzeri increased the chlorophyll b content in Poinsett 76 (Nawaz and Bano, 2020). The results recorded the same trend. The combined impact of P. putida and AgNPs was more efficient in Sialkot selection. Hänsch and Emmerling (2010) found that the lesser concentrations of AgNPs did not show any variations in the enzymatic activities in the soil. Using soluble Ag ions as a positive control, these studies showed that AgNPs were responsible for the negative impacts on soil microorganisms (Shin et al., 2012; Gomaa et al., 2021).
The results showed that the impact of AgNPs and PGPRs differs with nanoparticle’s types and the treatment dose. P. putida enhanced the shoot and fresh root weights (Table 2), whereas it was inhibitory in Zn and Fe NPs. PGPR inoculation increased the rate of root development and root and shoot weight (Krishnaraj et al., 2012a). The effects of AgNPs on the root system demonstrated both positive and negative impacts based on plant species (Lamsa et al., 2011). PGPR improved the growth of the plant and seed germination (Lamsa et al., 2011). Shoot fresh weight was very much higher at 80 ppm of AgNPs, while P. putida did not improve the fresh weight of the shoot (Table 2). AgNP application inhibited PGPR for growth factors (Nawaz and Bano, 2020).
The AgNPs used this strategy to decrease the fresh shoot weight at the expense of an increase in root length and weight (Nawaz and Bano, 2020). AgNPs increased the fresh weight and length of roots and shoots in some crops (Abdelsalam, 2014; Abdelsalam et al., 2019a; Zhao et al., 2019; Fouda et al., 2020b). Also, Waqas et al. (2015) tested the influence of AgNPs (NM-300 K; < 20 nm) on soil microorganisms and obtained a decrease of 39.9–73.2% in microbial biomass after 7 days. Waqas et al. (2015) obtained a higher reduction of 92.9–94.6% in bacterial numbers in sewage following AgNP application compared to our results. In addition, our results are consistent with Arvanitidou et al. (2005). Based on our findings, we hypothesize that the sensitivity of various microorganisms to AgNPs may be linked to environmental properties (protective or enhancing AgNPs effectiveness).
During this research, we tried protecting beneficial flora by using novel strategies and alternative approaches to fungicides by using AgNPs of different concentrations to detect the identical dose of B. fabae and compared this nanomaterial with other commercial products. The current data showed a high effect for AgNPs when using all different concentrations with different responses on B. fabae. These results agree with Akther and Hemalatha (2019) who used some non-fungicides as alternative approaches to fungicides and promising alternatives to preserve the biota and control the plant pathogenic fungi. They reviewed the importance of metal nanoparticles as potential fungicides rather than conventional chemical fungicides.
Also, they reported that AgNPs showed high effectiveness in controlling plant pathogens and showed a broad-spectrum antifungal activity by inhibiting the radial growth. Also, using nanofungicides was reported by Arciniegas-Grijalba et al. (2017). They detected the antifungal capacity in vitro of ZnO NPs and examined their action on E. salmonicolor fungi causal of a pink disease. Also, our results agree with the previous studies (Kanhed et al., 2014; Tabussam et al., 2022), who reported the significant antifungal activity of copper nanoparticles on some selected crop pathogenic fungi. Other studies were conducted by Bramhanwade et al. (2016). They found that copper nanoparticles (CuNPs) showed the highest activity against Fusarium equiseti with a 25 mm zone of inhibition, followed by Fusarium oxysporum (20 mm) and F. culmorum (19 mm). Also, Kim et al. (2012) used AgNPs solution as fungicidal properties for various plant pathogens.
These results agree with Ouda (2014), who investigated the antifungal activity of AgNPs, CuNPs, and Ag/Cu NPs against two plant pathogenic fungi A. alternata and B. cinerea. Their results revealed that nanoparticles had a damaging effect on fungal hyphae and conidia. Earlier reports confirmed that the antimicrobial activity of AgNPs was different depending on microbial species (Ouda, 2014). AgNPs can significantly delay mycelial growth in a dose-dependent manner in vitro (Aguilar-Méndez et al., 2011; Alam et al., 2022). AgNPs may directly attach to and penetrate the cell membrane to kill spores, while the penetration of AgNPs into microbial cell membranes is not clearly understood (Hwang et al., 2008).
Previous studies reported AgNPs to control plant pathogenic fungi, including Botrytis, with efficient inhibitory activities observed in vitro (Krishnaraj et al., 2012a; Fouda et al., 2020b). Nevertheless, the mechanism of action of nanoparticles is still unknown (Krishnaraj et al., 2012b; Alghuthaymi et al., 2015). A similar result was observed by Salama (2012), who reported that AgNPs had a stimulating effect on the growth of the common bean and corn plants. Also, Latif et al. (2017) demonstrated that various concentrations of AgNPs as foliar application increased the growth parameters of the wheat plant. Furthermore, Nghia et al. (2017) verified this positive effect of AgNPs.
Data Availability Statement
The raw data supporting the conclusions of this article will be made available by the authors, without undue reservation.
Author Contributions
NA, MS, and AH: data curation. MS and AH: investigation and methodology. MS, AH, BA, SJ, and CC-J: resources. RG: software. MM and NA: supervision. NA, RG, MM, and ME-S: writing—original draft, editing, and reviewing. All authors contributed to the article and approved the submitted version.
Funding
We are thankful for the financial support provided by the Fundação de Amparo à Pesquisa do Estado do Rio de Janeiro (FAPERJ) Brazil—(grant no. E-26/200.891/2021), and the Conselho Nacional de Desenvolvimento Científico e Tecnológico (CNPq)—(grant no. 313119/2020-1).
Conflict of Interest
The authors declare that the research was conducted in the absence of any commercial or financial relationships that could be construed as a potential conflict of interest.
Publisher’s Note
All claims expressed in this article are solely those of the authors and do not necessarily represent those of their affiliated organizations, or those of the publisher, the editors and the reviewers. Any product that may be evaluated in this article, or claim that may be made by its manufacturer, is not guaranteed or endorsed by the publisher.
Acknowledgments
We extend their appreciation to Alexandria university. We thank their respective institutions for their support. K.A. El-Tarabily would also like to thank the library at Murdoch University, Australia, for the valuable online resources and comprehensive databases.
References
Abbas, A., Shah, A. N., Shah, A. A., Nadeem, M. A., Alsaleh, A., Javed, T., et al. (2022). Genome-Wide analysis of invertase Gene family, and expression profiling under abiotic stress conditions in potato. Biology 11:539. doi: 10.3390/biology11040539
Abdel-Aziz, M. S., Shaheen, M. S., El-Nekeety, A. A., and Abdel-Wahhab, M. A. (2014). Antioxidant and antibacterial activity of silver nanoparticles biosynthesized using Chenopodium murale leaf extract. J. Saudi Chem. Soc. 18, 356–363.
Abdelsalam, N. R. (2014). Marker assisted-selection of major traits in egyptian bread wheat (Triticum aestivum l.) and wild wheat (Aegilops ventricosa tausch). Plant Cell Biotechnol. Mol. Biol. 15, 67–74.
Abdelsalam, N. R., Abdel-Megeed, A., Ghareeb, R. Y., Ali, H. M., Salem, M. Z. M., Akrami, M., et al. (2022). Genotoxicity assessment of amino zinc nanoparticles in wheat (Triticum aestivum L.) as cytogenetical perspective. Saudi J. Biol. Sci. 29, 2306–2313. doi: 10.1016/j.sjbs.2021.11.059
Abdelsalam, N. R., Fouda, M. M. G., Abdel-Megeed, A., Ajarem, J., Allam, A. A., and El-Naggar, M. E. (2019b). Assessment of silver nanoparticles decorated starch and commercial zinc nanoparticles with respect to their genotoxicity on onion. Int. J. Biol. Macromol. 133, 1008–1018. doi: 10.1016/j.ijbiomac.2019.04.134
Abdelsalam, N. R., Botros, W. A., Khaled, A. E., Ghonema, M. A., Hussein, S. G., Ali, H. M., et al. (2019a). Comparison of uridine diphosphate-glycosyltransferase UGT76G1 genes from some varieties of Stevia rebaudiana Bertoni. Sci. Rep. 9:8559. doi: 10.1038/s41598-019-44989-4
Adesemoye, A., Torbert, H., and Kloepper, J. (2009). Plant growth-promoting rhizobacteria allow reduced application rates of chemical fertilizers. Microb. Ecol. 58, 921–929. doi: 10.1007/s00248-009-9531-y
Adesemoye, A. O., and Egamberdieva, D. (2013). “Beneficial Effects of Plant Growth-Promoting Rhizobacteria on Improved Crop Production: Prospects for Developing Economies,” in Bacteria in Agrobiology: crop Productivity, eds D. K. Maheshwari, M. Saraf, and A. Aeron (Berlin: Springer), 45–63.
Aguilar-Méndez, M. A., San Martín-Martínez, E., Ortega-Arroyo, L., Cobián-Portillo, G., and Sánchez-Espíndola, E. (2011). Synthesis and characterization of silver nanoparticles: effect on phytopathogen Colletotrichum gloesporioides. J. Nanoparticle Res. 13, 2525–2532.
Ahmad, H., Zahid, M., Rehan, Z. A., Rashid, A., Akram, S., Aljohani, M. M. H., et al. (2022). Preparation of polyvinylidene fluoride nano-filtration membranes modified with functionalized graphene oxide for textile dye removal. Membranes 12:224. doi: 10.3390/membranes12020224
Akther, T., and Hemalatha, S. (2019). Mycosilver nanoparticles: synthesis, characterization and its efficacy against plant pathogenic fungi. BioNanoScience 9, 296–301.
Alam, M. S., Kong, J., Tao, R., Ahmed, T., Alamin, M., Alotaibi, S. S., et al. (2022). CRISPR/Cas9 mediated knockout of the OsbHLH024 transcription factor improves salt stress resistance in rice (Oryza sativa L.). Plants 11:1184. doi: 10.3390/plants11091184
Alghuthaymi, M. A., Almoammar, H., Rai, M., Said-Galiev, E., and Abd-Elsalam, K. A. (2015). Myconanoparticles: synthesis and their role in phytopathogens management. Biotechnol. Biotechnol. Equip. 29, 221–236. doi: 10.1080/13102818.2015.1008194
Alsalem, H. S., Keshk, A. A., Ghareeb, R. Y., Ibrahim, A. A., Abdelsalam, N. R., Taher, M. M., et al. (2022). Physico-chemical and biological responses for hydroxyapatite/ZnO/graphene oxide nanocomposite for biomedical utilization. Mater. Chem. Phys. 283:125988.
Arciniegas-Grijalba, P. A., Patiño-Portela, M. C., Mosquera-Sánchez, L. P., Guerrero-Vargas, J. A., and Rodríguez-Páez, J. E. (2017). ZnO nanoparticles (ZnO-NPs) and their antifungal activity against coffee fungus Erythricium salmonicolor. Appl. Nanosci. 7, 225–241.
Arvanitidou, M., Kanellou, K., and Vagiona, D. G. (2005). Diversity of Salmonella spp. and fungi in northern Greek rivers and their correlation to fecal pollution indicators. Environ. Res. 99, 278–284. doi: 10.1016/j.envres.2005.01.002
Ben-Moshe, T., Frenk, S., Dror, I., Minz, D., and Berkowitz, B. (2013). Effects of metal oxide nanoparticles on soil properties. Chemosphere 90, 640–646. doi: 10.1016/j.chemosphere.2012.09.018
Bramhanwade, K., Shende, S., Bonde, S., Gade, A., and Rai, M. (2016). Fungicidal activity of Cu nanoparticles against Fusarium causing crop diseases. Environ. Chem. Lett. 14, 229–235.
Cao, J., Feng, Y., Lin, X., and Wang, J. (2016). Arbuscular mycorrhizal fungi alleviate the negative effects of iron oxide nanoparticles on bacterial community in rhizospheric soils. Front. Environ. Sci. 4:10. doi: 10.3389/fenvs.2016.00010
Chai, H., Yao, J., Sun, J., Zhang, C., Liu, W., Zhu, M., et al. (2015). The effect of metal oxide nanoparticles on functional bacteria and metabolic profiles in agricultural soil. Bull. Environ. Contam. Toxicol. 94, 490–495. doi: 10.1007/s00128-015-1485-9
Chiang, K., Liu, H., and Bock, C. (2017). A discussion on disease severity index values. Part I: warning on inherent errors and suggestions to maximise accuracy. Ann. Appl. Biol. 171, 139–154.
Choudhary, R. C., Bairwa, H. L., Kumar, U., Javed, T., Asad, M., Lal, K., et al. (2022). Influence of organic manures on soil nutrient content, microbial population, yield and quality parameters of pomegranate (Punica granatum L.) cv. Bhagwa. PLoS One 17:e0266675. doi: 10.1371/journal.pone.0266675
Elkobrosy, D. H., Aseel, D. G., Hafez, E. E., El-Saedy, M. A., Al-Huqail, A. A., Ali, H. M., et al. (2022). Quantitative detection of induced systemic resistance genes of potato roots upon ethylene treatment and cyst nematode, Globodera rostochiensis, infection during plant–nematode interactions. Saudi J. Biol. Sci. 29, 3617–3625.
Fouda, M. M., Abdelsalam, N. R., El-Naggar, M. E., Zaitoun, A. F., Salim, B. M., Bin-Jumah, M., et al. (2020a). Impact of high throughput green synthesized silver nanoparticles on agronomic traits of onion. Int. J. Biol. Macromol. 149, 1304–1317. doi: 10.1016/j.ijbiomac.2020.02.004
Fouda, M. M. G., Abdelsalam, N. R., Gohar, I. M. A., Hanfy, A. E. M., Othman, S. I., Zaitoun, A. F., et al. (2020b). Utilization of High throughput microcrystalline cellulose decorated silver nanoparticles as an eco-nematicide on root-knot nematodes. Colloids Surf. B 188:110805. doi: 10.1016/j.colsurfb.2020.110805
Ghareeb, R. Y., Alfy, H., Fahmy, A. A., Ali, H. M., and Abdelsalam, N. R. (2020). Utilization of Cladophora glomerata extract nanoparticles as eco-nematicide and enhancing the defense responses of tomato plants infected by Meloidogyne javanica. Sci. Rep. 10:19968. doi: 10.1038/s41598-020-77005-1
Gomaa, M., Kandil, E. E., El-Dein, A. A. Z., Abou-Donia, M. E., Ali, H. M., and Abdelsalam, N. R. (2021). Increase maize productivity and water use efficiency through application of potassium silicate under water stress. Sci. Rep. 11:224. doi: 10.1038/s41598-020-80656-9
Gruyer, N., Dorais, M., Bastien, C., Dassylva, N., and Triffault-Bouchet, G. (2014). “Interaction between silver nanoparticles and plant growth,” in International Symposium on New Technologies for Environment Control, Energy-Saving and Crop Production in Greenhouse and Plant, Vol. 1037, 795–800.
Hänsch, M., and Emmerling, C. (2010). Effects of silver nanoparticles on the microbiota and enzyme activity in soil. J. Plant. Nutr. Soil Sci. 173, 554–558.
Hartmann, A., Rothballer, M., and Schmid, M. (2008). Lorenz Hiltner, a pioneer in rhizosphere microbial ecology and soil bacteriology research. Plant Soil 312, 7–14.
Hassan, M. U., Ghareeb, R. Y., Nawaz, M., Mahmood, A., Shah, A. N., Abdel-Megeed, A., et al. (2022). Melatonin: A vital pro-tectant for crops against heat stress: Mechanisms and prospects. Agronomy 12:1116.
Hiltner, L. (1904). Uber nevere Erfahrungen und Probleme auf dem Gebiet der Boden Bakteriologie und unter besonderer Beurchsichtigung der Grundungung und Broche. Arbeit. Deut. Landw. Ges. Berlin 98, 59–78.
Hwang, E. T., Lee, J. H., Chae, Y. J., Kim, Y. S., Kim, B. C., Sang, B.-I., et al. (2008). Analysis of the toxic mode of action of silver nanoparticles using stress-specific bioluminescent bacteria. Small 4, 746–750. doi: 10.1002/smll.200700954
Kandil, E. E., Abdelsalam, N. R., Aziz, A. A. A. E., Ali, H. M., and Siddiqui, M. H. (2020). Efficacy of nanofertilizer, fulvic acid and boron fertilizer on sugar beet (Beta vulgaris L.) yield and quality. Sugar Tech. 22, 782–791.
Kanhed, P., Birla, S., Gaikwad, S., Gade, A., Seabra, A. B., Rubilar, O., et al. (2014). In vitro antifungal efficacy of copper nanoparticles against selected crop pathogenic fungi. Mater. Lett. 115, 13–17.
Karunakaran, G., Suriyaprabha, R., Manivasakan, P., Yuvakkumar, R., Rajendran, V., Prabu, P., et al. (2013). Effect of nanosilica and silicon sources on plant growth promoting rhizobacteria, soil nutrients and maize seed germination. IET Nanobiotechnol. 7, 70–77. doi: 10.1049/iet-nbt.2012.0048
Khan, N., Zandi, P., Ali, S., Mehmood, A., Adnan Shahid, M., and Yang, J. (2018). Impact of salicylic acid and PGPR on the drought tolerance and phytoremediation potential of Helianthus annus. Front. Microbiol. 9:2507. doi: 10.3389/fmicb.2018.02507
Kim, S. W., Jung, J. H., Lamsal, K., Kim, Y. S., Min, J. S., and Lee, Y. S. (2012). Antifungal effects of silver nanoparticles (AgNPs) against various plant pathogenic fungi. Mycobiology 40, 53–58. doi: 10.5941/MYCO.2012.40.1.053
Klaine, S. J., Alvarez, P. J., Batley, G. E., Fernandes, T. F., Handy, R. D., Lyon, D. Y., et al. (2008). Nanomaterials in the environment: behavior, fate, bioavailability, and effects. Environ. Toxicol. Chem. 27, 1825–1851.
Krishnaraj, C., Jagan, E. G., Ramachandran, R., Abirami, S. M., Mohan, N., and Kalaichelvan, P. T. (2012a). Effect of biologically synthesized silver nanoparticles on Bacopa monnieri (Linn.) Wettst. plant growth metabolism. Process Biochem. 47, 651–658.
Krishnaraj, C., Ramachandran, R., Mohan, K., and Kalaichelvan, P. T. (2012b). Optimization for rapid synthesis of silver nanoparticles and its effect on phytopathogenic fungi. Spectrochimica Acta Part A: Mol. Biomol. Spectrosc. 93, 95–99. doi: 10.1016/j.saa.2012.03.002
Kumar, N., Shah, V., and Walker, V. K. (2012). Influence of a nanoparticle mixture on an arctic soil community. Environ. Toxicol. Chem. 31, 131–135. doi: 10.1002/etc.721
Lakshmipathy, M., and Nanda, A. (2013). Biocatalysts of silver nanoparticles synthesized using an environmental isolate, Serratia marcescens S01. Int. J. Chemtech. Res. 5, 1162–1168.
Lamsa, K., Kim, S.-W., Jung, J. H., Kim, Y. S., Kim, K. S., and Lee, Y. S. (2011). Inhibition effects of silver nanoparticles against powdery mildews on cucumber and pumpkin. Mycobiology 39, 26–32. doi: 10.4489/MYCO.2011.39.1.026
Lara, H. H., Garza-Treviño, E. N., Ixtepan-Turrent, L., and Singh, D. K. (2011). Silver nanoparticles are broad-spectrum bactericidal and virucidal compounds. J.Nanobiotechnol. 9:30. doi: 10.1186/1477-3155-9-30
Latif, H. H., Ghareib, M., and Tahon, M. A. (2017). Phytosynthesis of silver nanoparticles using leaf extracts from Ocimum basilicum and Mangifira indica and their effect on some biochemical attributes of Triticum aestivum. Gesunde Pflanzen 69, 39–46.
Laware, S., and Raskar, S. (2014). Influence of zinc oxide nanoparticles on growth, flowering and seed productivity in onion. Int. J. Curr. Microbiol. Sci. 3, 874–881.
Lovern, S. B., and Klaper, R. (2006). Daphnia magna mortality when exposed to titanium dioxide and fullerene (C60) nanoparticles. Environ. Toxicol. Chem. 25, 1132–1137. doi: 10.1897/05-278r.1
Majeed, S., Nanda, A., and Thirunavukarasu, K. (2014). Evaluation of antimicrobial activity of biologically synthesized silver nanoparticles from filamentous fungi. Int. J. PharmTech Res. 6, 1049–1053.
Maliszewska, I. (2016). Effects of the biogenic gold nanoparticles on microbial community structure and activities. Ann. Microbiol. 66, 785–794.
Mishra, V. K., and Kumar, A. (2009). Impact of metal nanoparticles on the plant growth promoting rhizobacteria. Dig. J. Nanomater. Biostruct. 4, 587–592.
Morones, J. R., Elechiguerra, J. L., Camacho, A., Holt, K., Kouri, J. B., Ramírez, J. T., et al. (2005). The bactericidal effect of silver nanoparticles. Nanotechnology 16, 2346–2353.
Nawaz, S., and Bano, A. (2020). Effects of PGPR (Pseudomonas sp.) and Ag-nanoparticles on enzymatic activity and physiology of cucumber. Recent Pat. food, Nutr. Agric. 11, 124–136. doi: 10.2174/2212798410666190716162340
Nayak, B. K., Bhat, M. A., and Nanda, A. (2013). Implementation of Penicillium sp. as raw material for synthesizing metal nanoparticles for antibiosis. Mater. Sci. Forum 760, 33–38.
Nayak, B. K., Nanda, A., and Behera, N. (1998). Airborne fungal spores in an industrial area: seasonal and diurnal periodicity. Aerobiologia 14, 59–67.
Nayak, B. K, Chitra, N., and Nanda, A. (2014). Efficacy of biosynthesized AgNPs from Alternaria chlamydospora isolated from indoor air of vegetable market. Int. J. PharmTech. Res. 6, 1309–1314.
Nghia, L. T., Tung, H. T., Huy, N. P., Luan, V. Q., and Nhut, D. T. (2017). The effects of silver nanoparticles on growth of Chrysanthemum morifolium Ramat. cv.” JIMBA” in different cultural systems. Vietnam. J. Sci. Technol. 55:503.
Ouda, S. M. (2014). Antifungal activity of silver and copper nanoparticles on two plant pathogens, Alternaria alternata and Botrytis cinerea. Res. J.Microbiol. 9:34.
Salama, H. M. (2012). Effects of silver nanoparticles in some crop plants, common bean (Phaseolus vulgaris L.) and corn (Zea mays L.). Int. Res. J. Biotechnol. 3, 190–197.
Shams, G., Ranjbar, M., and Amiri, A. (2013). Effect of silver nanoparticles on concentration of silver heavy element and growth indexes in cucumber (Cucumis sativus L. negeen). J. Nanoparticle Res. 15, 1–12.
Shin, Y.-J., Kwak, J. I., and An, Y.-J. (2012). Evidence for the inhibitory effects of silver nanoparticles on the activities of soil exoenzymes. Chemosphere 88, 524–529. doi: 10.1016/j.chemosphere.2012.03.010
Simonin, M., Guyonnet, J. P., Martins, J. M. F., Ginot, M., and Richaume, A. (2015). Influence of soil properties on the toxicity of TiO2 nanoparticles on carbon mineralization and bacterial abundance. J. Hazard. Mater. 283, 529–535. doi: 10.1016/j.jhazmat.2014.10.004
Simonin, M., and Richaume, A. (2015). Impact of engineered nanoparticles on the activity, abundance, and diversity of soil microbial communities: a review. Environ. Sci. Pollut. Res. 22, 13710–13723.
Somers, E., Vanderleyden, J., and Srinivasan, M. (2004). Rhizosphere bacterial signalling: a love parade beneath our feet. Crit. Rev. Microbiol. 30, 205–240. doi: 10.1080/10408410490468786
Sompornpailin, D., Siripattanakul-Ratpukdi, S., and Vangnai, A. S. (2014). Diethyl phthalate degradation by the freeze-dried, entrapped Bacillus subtilis strain 3C3. Int. Biodeterior. Biodegradation 91, 138–147.
Sondi, I., and Salopek-Sondi, B. (2004). Silver nanoparticles as antimicrobial agent: a case study on E. coli as a model for Gram-negative bacteria. J. Colloid Interface Sci. 275, 177–182.
Tabussam, N., Rana, R. M., Wattoo, F. M., Khan, A. I., Amir, R. M., Javed, T., et al. (2022). Single nucleotide polymorphism based assessment of genetic diversity in local and exotic onion genotypes. Mol. Biol. Rep. [Epub ahead of print]. doi: 10.1007/s11033-022-07431-z
Talukder, M. R. M., Roy, B., and Saha, K. C. (2015). Effects of herbal plant extracts on germination and seedling growth of some vegetables. Int. J. Nat. Sci. 6, 421–425.
Thuesombat, P., Hannongbua, S., Akasit, S., and Chadchawan, S. (2014). Effect of silver nanoparticles on rice (Oryza sativa L. cv. KDML 105) seed germination and seedling growth. Ecotoxicol. Environ. Saf. 104, 302–309. doi: 10.1016/j.ecoenv.2014.03.022
Timmusk, S., Behers, L., Muthoni, J., Muraya, A., and Aronsson, A.-C. (2017). Perspectives and challenges of microbial application for crop improvement. Front. Plant Sci. 8:49. doi: 10.3389/fpls.2017.00049
Venkataraju, J. L., Sharath, R., Chandraprabha, M., Neelufar, E., Hazra, A., and Patra, M. (2014). Synthesis, characterization and evaluation of antimicrobial activity of zinc oxide nanoparticles. J. Biochem. Technol. 3, 151–154.
Vittori Antisari, L., Carbone, S., Gatti, A., Vianello, G., and Nannipieri, P. (2013). Toxicity of metal oxide (CeO2, Fe3O4, SnO2) engineered nanoparticles on soil microbial biomass and their distribution in soil. Soil Biol. Biochem. 60, 87–94.
Waqas, M., Khan, A. L., Hamayun, M., Shahzad, R., Kang, S.-M., Kim, J.-G., et al. (2015). Endophytic fungi promote plant growth and mitigate the adverse effects of stem rot: an example of Penicillium citrinum and Aspergillus terreus. J. Plant Interact. 10, 280–287.
Xu, X.-H. N., Brownlow, W. J., Kyriacou, S. V., Wan, Q., and Viola, J. J. (2004). Real-Time probing of membrane transport in living microbial cells using single nanoparticle optics and living cell imaging. Biochemistry 43, 10400–10413. doi: 10.1021/bi036231a
Yang, J., Kloepper, J. W., and Ryu, C.-M. (2009). Rhizosphere bacteria help plants tolerate abiotic stress. Trends Plant Sci. 14, 1–4. doi: 10.1016/j.tplants.2008.10.004
Youssef, N. H., Al-Huqail, A. A., Ali, H. M., Abdelsalam, N. R., and Sabra, M. A. (2020). The role of Serendipita indica and Lactobacilli mixtures on mitigating mycotoxins and heavy metals’ risks of contaminated sewage sludge and its composts. Sci. Rep. 10:15159. doi: 10.1038/s41598-020-71917-8
Zarina, A., and Nanda, A. (2014). Combined efficacy of antibiotics and biosynthesised silver nanoparticles from Streptomyces albaduncus. Int. J. Pharm. Technol. Res. 6, 1862–1869.
Zhao, J., Abdelsalam, N. R., Khalaf, L., Chuang, W.-P., Zhao, L., Smith, C. M., et al. (2019). Development of single nucleotide polymorphism markers for the wheat curl mite resistance gene Cmc4. Crop Sci. 59, 1567–1575. doi: 10.1007/s00122-020-03737-3
Keywords: microbial community, fungi, pathogens, nanoparticles, fungicide, rhizosphere
Citation: Sabra MA, Alaidaroos BA, Jastaniah SD, Heflish AI, Ghareeb RY, Mackled MI, El-Saadony MT, Abdelsalam NR and Conte-Junior CA (2022) Comparative Effect of Commercially Available Nanoparticles on Soil Bacterial Community and “Botrytis fabae” Caused Brown Spot: In vitro and in vivo Experiment. Front. Microbiol. 13:934031. doi: 10.3389/fmicb.2022.934031
Received: 02 May 2022; Accepted: 30 May 2022;
Published: 22 June 2022.
Edited by:
Decai Jin, Research Center for Eco-Environmental Sciences (CAS), ChinaReviewed by:
Anima Nanda, Sathyabama Institute of Science and Technology, IndiaAmit Kishore Singh, Tilka Manjhi Bhagalpur University, India
Copyright © 2022 Sabra, Alaidaroos, Jastaniah, Heflish, Ghareeb, Mackled, El-Saadony, Abdelsalam and Conte-Junior. This is an open-access article distributed under the terms of the Creative Commons Attribution License (CC BY). The use, distribution or reproduction in other forums is permitted, provided the original author(s) and the copyright owner(s) are credited and that the original publication in this journal is cited, in accordance with accepted academic practice. No use, distribution or reproduction is permitted which does not comply with these terms.
*Correspondence: Mohamed T. El-Saadony, bS50YWxhYXRlbHNhZG9ueUBnbWFpbC5jb20=; Nader R. Abdelsalam, bmFkZXIud2hlYXRAYWxleHUuZWR1LmVn