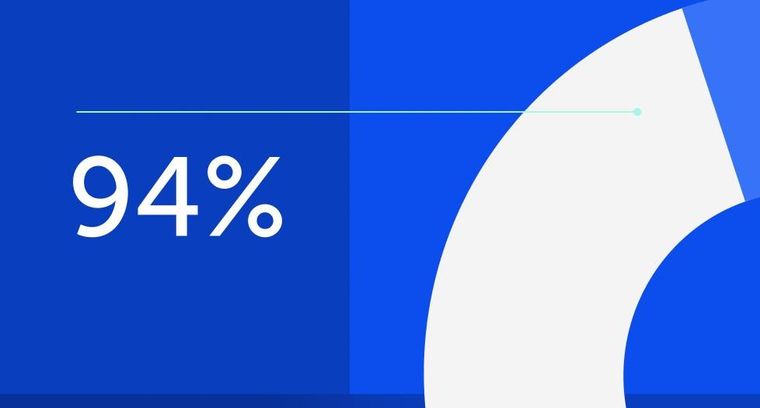
94% of researchers rate our articles as excellent or good
Learn more about the work of our research integrity team to safeguard the quality of each article we publish.
Find out more
ORIGINAL RESEARCH article
Front. Microbiol., 03 October 2022
Sec. Microorganisms in Vertebrate Digestive Systems
Volume 13 - 2022 | https://doi.org/10.3389/fmicb.2022.932495
This article is part of the Research TopicThe Origin and Establishment Process of Gut Microbiota in Early LifeView all 18 articles
Increasing studies have found breast milk (BM) contains its own microbiota. However, the route through which microbes enter the BM is still unclear. In order to verify the entero-mammary pathway of BM, we designed a rigorous study that prevented oral bacteria from contaminating the breast and nipple skin (NS) during baby nursing. Thirty-one healthy, postpartum mothers living in southern China who were immediately separated from their newborn after delivery were enrolled in this study. Using an aseptic protocol for sampling, sterile water was used to wash the NS and was then collected. Then the first drop of BM was discarded and colostrum was collected manually. Amplicon sequencing was performed targeting the V3–V4 region of the bacterial 16S rRNA gene, and the differences between the microbiota of the colostrum and NS were analyzed. Additionally, the effects of environmental factors, such as the delivery mode and intrapartum antibiotic exposure, on the diversity of the colostrum microbiota were also analyzed. We found significant differences in the α diversity and richness between the BM and NS as evidenced by richness, Chao1, and Simpson indices. There were 170 operational taxonomic units (OTUs) shared by colostrum and NS, while 111 and 87 OTUs were unique, respectively, as well as a clear distinction in OTUs was observed by unifrac binary analysis between them. Linear discriminant analysis effect size analysis found that anaerobes, such as Bifidobacterium and Pantoea at the genus level and enterobacteria including Enterobacteriaceae at the family level, were predominant in the colostrum, while the predominant bacteria on the NS were Bacteroides, Staphylococcus, and Parabacteroides at the genus level. BM is colonized by bacteria prior to baby suckling, and the diversity of the colostrum microbiota differs from that of the NS. The predominant microbiota taxa in BM indicated that they were likely to be transferred to the breast through the intestinal tract. Our study provides direct evidence for the revolutionary active migration hypothesis. Additionally, factors like intrapartum antibiotic exposure did not significantly affect the diversity of the microbiota in the BM. Therefore, it is suggested that mothers continue to provide BM for their newborns during separation.
Human breast milk (BM) is the best nutrition for infants, as it provides essential nutrients, immune cells, and bioactive components. Breastfeeding is associated with considerable health benefits for infants, including protection from diarrhea (Duijts et al., 2010), necrotizing enterocolitis (Herrmann and Carroll, 2014), respiratory infections (Lanari et al., 2013; Tromp et al., 2017), as well as obesity, type 2 diabetes, and cardiovascular disease (Arenz et al., 2004). These benefits are, especially, relevant to infants with increased susceptibility to infections, such as preterm or sick infants. Additionally, increasing evidence has shown that BM contains its own microbiota, which is important for maintaining both mammary and infant health (Zimmermann and Curtis, 2020). Breastfeeding was considered to be a smart investment in people and in economies (Hansen, 2016), thus World Health Organization (WHO) currently recommends an exclusively breastfed diet until 6 months of age, with solid food and breast milk continuing thereafter (WHO, 2001).
In recent years, the study of the BM microbiota has become increasingly extensive. BM is recognized as a source of commensal and potentially probiotic bacteria (Jost et al., 2014) and may also influence both neonatal gut colonization (Gueimonde et al., 2007) and the maturation of the immune system (Jost et al., 2014). In the past, bacteria isolated from BM were thought to be a contaminant from the infant’s oral cavity, mother’s skin, or due to incorrect handling or processing (Heikkila and Saris, 2003). One study estimated that average consumption of 800 ml of BM per day correlates to an infant ingesting approximately 8 × 107 to 1010 bacteria per day (Zimmermann and Curtis, 2020). Traditional culture and isolation techniques and culture-independent molecular methods, such as quantitative PCR, cloning and sequencing of bacterial 16S rRNA gene fragments (Cabrera-Rubio et al., 2012, 2016; Jost et al., 2013; Sakwinska et al., 2016; Ruiz et al., 2019), and metagenomics shotgun sequencing (Ward et al., 2013; Jimenez et al., 2015), have revealed the diversity and complexity of bacteria in BM.
The origin of the BM microbiota is the subject of much debate. There are currently two hypotheses regarding the source of the BM microbiota: the traditional pollution theory and the active migration theory. According to pollution theory, the microorganisms in breast milk come from the mother’s nipple and areola and the baby’s mouth. During the process of maternal feeding, the negative pressure of sucking generated by the infant allows the microorganisms colonizing the skin surrounding the nipple and areola to enter the mammary gland along the breast tube, or the newborn’s mouth is contaminated by microorganisms from the mother’s intestinal tract and vagina during delivery, which enter the mammary gland during feeding. Other studies have suggested that the microbiota in the mother’s intestines and vagina can naturally “migrate” to the newborn’s intestines during delivery (Martin et al., 2007a; Makino et al., 2011; Sanz, 2011).
The other is the hypothesis that maternal bacteria translocate through the intestinal epithelial barrier, migrate to the mammary glands via an endogenous cellular route, such as a bacterial entero-mammary pathway, and subsequently colonize the gut of the breastfed neonate (Martin et al., 2007b; Jost et al., 2014).
In order to test the hypothesis regarding an entero-mammary pathway of microbiota translocation in breast milk, we washed the skin surrounding the nipple and collected the cleaning fluid, in addition to the colostrum, from 31 mothers who were separated from their infants immediately after delivery. We used 16S rRNA gene sequencing to detect and analyze differences between the microbiota profile of the BM and nipple skin. We also investigated the impact of newborn gender, delivery mode, parity, gestational age, and intrapartum antibiotic exposure on the microbiota composition of colostrum.
The study was conducted from January to February 2021 in the Luohu Maternal and Child Hospital in Shenzhen, China. Thirty-one healthy volunteers who met the inclusion and exclusion criteria were included in the study. The inclusion criteria were as follows: 20–45 years old, healthy during pregnancy, without any common pregnancy complications, did not use probiotics during pregnancy, settled in Shenzhen, the Han nationality, and normal lactating woman. Exclusion criteria included pregnant women with gestational diabetes, pregnancy-induced hypertension syndrome, acute communicable diseases, and ethnic minorities. After birth, the newborns were immediately transferred to the neonatal intensive care unit (NICU) for monitoring or treatment due to premature rupture of membranes or other reasons, resulting in the separation of mother and baby. Thus, the newborn did not nurse from the mother’s breast immediately after delivery. Further, BM samples were collected only from mothers who are yet to nurse their newborn babies. The postpartum mothers were usually hospitalized for 3−5 days and then discharged with their baby or discharged before the baby. In order to ensure the samples were all in the same ward environment, we collected BM from lactating mothers during their hospitalization. Since we collected BM within 5 days after delivery, the milk collected was colostrum. Additionally, mothers generally did not pump BM before delivery.
The participants completed a questionnaire that included basic maternal information (name, age, telephone number, and address), pregnancy and childbirth information (number of pregnancies, parity, the number of gestational weeks at delivery, education level, pre-pregnancy weight, pre-partum weight, breast changes during pregnancy, diet, delivery method, and prophylactic use of antibiotics before or after delivery), and information about the infant, including gender, height, and weight, as well as the reason for separation from the mother at birth (Table 1 and Supplementary Table S1).
During hospitalization, colostrum was collected from all 31 lactating mothers within 5 days after delivery. The first BM and NS samples of the mother were collected on 5 January 2021, and the last samples were collected on 25 January 2021. Colostrum was collected by an International Board Certified Lactation Consultant (IBCLC) in our study group, and her assistant using an aseptic protocol. Briefly, 8–10 ml of sterile water was used to wash one nipple and the surrounding nipple skin (NS). The sterilized water for rinsing the nipple skin was collected in an enzyme-free, aseptic centrifugal tube. The first drop of breast milk was discarded with an aseptic yarn block, and the colostrum (3–5 ml) was manually collected into an enzyme-free, aseptic centrifugal tube by researchers with sterile gloves. After sealing the tube with sealing film, the BM and NS samples were quickly frozen in liquid nitrogen and then transferred to a −80°C freezer for storage. The total genomic DNA was extracted on 19th February 2021. These processes prevented the microbiota from replicating.
The bacterial DNA was extracted from the BM and lotion samples using the TGuide S96 Magnetic Soil/Stool DNA Kit [Tiangen Biotech (Beijing) Co., Ltd., China], and PCR amplification was conducted with barcoded-specific bacterial primers targeting the variable region 3–4 (V3–V4) of the 16S rRNA gene. The primers used were 335F: 5′-CADACTCCTACGGGAGGC-3′ and 769R: 5′-ATCCTGTTTGMTMCCCVCRC-3′.
The PCR was performed in a total reaction volume of 10 μl: DNA template (5−50 ng), *Vn F (10 μM, 0.3 μl), *Vn R (10 μM, 0.3 μl), KOD FX Neo Buffer (5 μl), dNTP (2 mM, 2 μl), KOD FX Neo (0.2 μl), and ddH2O (up to 10 μl). The amplification conditions were as follows: an initial denaturation at 95°C for 5 min, followed by 25 cycles of 95°C for 30 s, 50°C for 20 s, and 72°C for 40 s, and a final extension at 72°C for 7 min. The PCR amplified products were mixed and purified by an Omega DNA purification column (Norcross, GA, United States). The mixed PCR amplified products were then purified and recovered using 1.8% agarose gel electrophoresis.
Construction of sequencing libraries and paired-end sequencing was performed on an Illumina NovaSeq6000 platform at Biomarker Technologies Co., Ltd. (Beijing, China) according to standard protocols. Paired-end reads were merged using FLASH v1.2.7 (Yang et al., 2020), and tags with more than six mismatches were discarded. The merged tags with an average quality score < 20 in a 50-bp sliding window were determined using Trimmomatic (Sheng et al., 2019), and those shorter than 350 bps were removed. Possible chimeras were further removed, and the denoised sequences were clustered into operational taxonomic units (OTUs) with 97% similarity using USEARCH (version 10.0) (Edgar, 2010), and the OTUs with reabundance < 0.005% were filtered. Taxonomy was assigned to all OTUs by searching against the Silva databases (Release128) using USEARCH software (version 10.0). We used Decontam (Version 0.0.1) to minimize the sources of probable external contamination.
The statistical analyses were performed in R (Version 4.0.1). Microbiota profiles were included in the estimation of alpha diversity (referring to diversity within a particular region or ecosystem) and beta diversity (comparing the similarity of species diversity among different samples) analyses as described by Liu et al. (2021). We obtained the richness, chao1, and Shannon indices of alpha diversity and compared these differences between BM and NS by LSD t-test.
Dissimilarities between BM and NS were estimated with the Bray–Curtis dissimilarity index and Unifrac indices (Lozupone et al., 2007) and analyzed with principal coordinate analysis (PCoA). Moreover, permutational multivariate analysis of variance was used to describe the strength and significance that a categorical factor has in determining the variation of ecological distances. The differential OTU abundance and taxa were analyzed by Wilcoxon rank-sum tests in R version 4.0.1 (FDR < 0.05, the mean relative abundance > 0.1%).
Furthermore, we employed linear discriminant analysis (LDA) effect size (LEfSe; Segata et al., 2011) to identify the significant taxonomic difference among influencing groups, with edgeR (Robinson et al., 2010) for verification if necessary. A logarithmic LDA score of 2.0 was set as the threshold for discriminative features. Adobe Illustrator was used to plot the results.
This study was conducted according to the guidelines set forth in the Declaration of Helsinki. The experiments in this study were approved by the Ethics Committee of the Luohu Maternal and Child Health Hospital (No. LL2022051337). Written consent was obtained from each volunteer.
The study was conducted on samples collected from 31 healthy mothers (aged 21–42 years) who were separated from their infants immediately after delivery without skin-to-skin contact with babies. The causes of mother-infant immediate separation after delivery included neonatal factors, such as pulmonary sequestration, premature birth, and amniotic fluid inhalation, and maternal factors, such as premature rupture of membranes, placental abruption, and extended vaginal delivery. Details are presented in Table 1 and Supplementary Table S1. The average age of mothers was 29.3 ± 4.6 years. Nearly, 68% of mothers gave birth to their first child, 23% for the second baby, and less than 9% for the third. Among the 31 newborns, 19 were male and 12 were female, and 17 were delivered vaginally and 14 were born by cesarean section (C-section). The average birth weight of babies was 3.0 ± 0.6 Kg. Twenty-two mothers received intrapartum antibiotic prophylaxis (IAP) through intravenous infusion, including 14 who delivered via C-section and 7 who delivered vaginally. The main antibiotics were cefazolin sodium, which is a first-generation cephalosporin, and cefuroxime sodium, which is a second-generation cephalosporin. All 31 normal lactating mothers lived in Shenzhen, south of China, and experienced anxiety after being separated from their newborns.
The quantity of microbiota in the BM and NS samples collected via an aseptic protocol was sufficient for microbiota profiling based on 16S rRNA gene sequencing. All 62 samples, which included 31 BM and 31 NS samples, yielded quantifiable PCR products and were sequenced. The analysis resulted in the generation of a total of 4,646,066 clean reads, representing an average of 74,937 clean reads per sample. The average reads were 78,158 per sample in the BM samples and 71,714 reads per sample in the NS samples. Reads were clustered at the similarity level of 97.0%, and OTUs were obtained. Totally, 11,190 OTUs were retained for diversity analyses, with an average OUT abundance of 243 in BM and 212 in NS.
A dilution curve (Wang et al., 2012) (rarefaction curve) randomly takes a certain number of sequences from a sample, counts the number of species represented by the sequences, and constructs a curve based on the number of sequences and species. This curve is then used to verify whether the amount of sequencing data is sufficient to reflect the species diversity in the sample, and indirectly reflects the species richness in the sample. The rarefaction curves of the OTUs from the BM and NS samples approached the plateau phase with more than 40,000 sequences per sample, indicating that these values were saturated and there was no need to determine more sequences (Figure 1A). The α diversity of the microbiota from the BM and NS was estimated through sample richness (Figure 1C), Chao1 (Figure 1B), and Shannon index (Figure 1D). The BM samples displayed higher α diversity values than the NS samples, as determined via the three indices mentioned above (LSD t-test p = 0.000, 0.001, and 0.001, respectively) (Figures 1B–D). In short, the microbiota in BM and NS detected by 16S rRNA gene sequencing was diverse. Moreover, the microbial abundance in BM was higher than that in the NS.
Figure 1. Measures of α diversity for BM and NS. (A) A rarefaction curve was used to evaluate the sequencing saturation of the samples, and it showed the mean value and standard error of the sequencing depth and the quantity of OTUs. The smoothing of the curves indicated that the sequencing was saturated. (B–D) Box plots of three different α diversity measures: Chao1, richness, and Shannon. The three indices were based on OTUs clustered at 97% similarity for the BM and NS samples (BM, n = 31 vs. NS, n = 31). There were significant differences in Chao1, richness, and Shannon between BM and NS groups (a and b above boxes represent statistical differences) with FDR-adjusted p = 0.000, 0.001, and 0.001 with LSD t-test, respectively. Each scatter represents a sample. The horizontal bars within boxes represent the median, and the tops and bottoms of the boxes represent the 75th and 25th quartiles, respectively. ***p ≤ 0.001.
Focusing on the differences and similarities of specific phylum between the BM and NS microbiotas (Figure 2A), we found that Firmicutes was the dominant phylum in both sample types, followed by Bacteroidetes, Proteobacteria, Actinobacteria, and Fusobacteria. These phyla were detected across all the samples analyzed. Analysis at the genus level (the microbial relative abundance was above 0.1% and the top 10 were displayed) (Figure 2B) found that the common genera in both the BM and NS microbiotas were Bacteroides, Faecalibacterium, Pantoea, uncultured_bacterium_f_Lachnospiraceae, Prevotella_9, Roseburia, Lachnospira, Agathobacter, Staphylococcus, Parabacteroides, and others. On the other hand, there were also differences in the abundance of some genera between the BM and NS samples. Faecalibacterium (9.39% vs. 9.11%) and Pantoea (9.4% vs. 0.51%) were dramatically higher in the BM microbiota when compared to the NS microbiota, while Lachnospira (2.04% vs. 3.48%), Staphylococcus (1.52% vs. 3.03%), and Parabacteroides (1.30% vs. 3.12%) were dramatically lower in the BM microbiota when compared to the NS microbiota.
Figure 2. The common (A,B) and unique (C,D) relative abundances of predominant microbiota taxa in BM and NS. The common microbiota at the panels (A) phylum level and (B) genus level. The unique microbiota at the genus level of panels (C) BM and (D) NS. The proportion of microbial abundance was more than 0.1%, and the top 10 were displayed.
According to the microbial abundance top10 statistics, the unique bacterial genera of the BM samples included Pantoea, Pseudomonas, Bifidobacterium, Klebsiella, and Escherichia-Shigella (Figure 2C), which were higher than 0.1%. The unique bacterial genera in the NS samples were Lachnospira, Parabacteroides, Staphylococcus, Agathobacter, and Lachnoclostridium (Figure 2D). Additionally, the shared genera between the BM and NS microbiotas were Bacteroides, Faecalibacterium, uncultured_bacterium_f_Lachnospiraceae, Prevotella_9, Roseburia, and others (Figures 2C,D). Additionally, high variability in the microbiota composition of both BM and NS was observed among the 31 mothers. Thus, the bacterial communities were generally complex and showed individual-specific profiles.
We examined differences in the microbiota of BM and NS at the OTU level. First, a Venn diagram that showed 170 overlapped OTUs between BM and NS was constructed. The unique OTUs were 111 for BM and 87 for NS (Supplementary Figure S1A). Through differential abundance analysis, 77 OTUs were depleted and 107 were enriched in the BM when compared with the NS (Supplementary Figure S1B). We found that the composition of the bacterial microbiota of BM was different from that of NS. Unifrac binary analysis (PCoA) of Bray–Curtis distance revealed that the microbiota of BM and NS formed two distinct clusters, which separated along the first coordinate axis (Figure 3A), indicating that the BM microbiota differed from the NS microbiota.
Figure 3. Differences in the microbiota between BM (colostrum) and NS. (A) Unconstrained principal coordinate analysis with Bray–Curtis distance showing that the BM microbiota separated from those of NS in the first axis (21.49% of variance explained, P < 0.001, permutational multivariate analysis of variance (PERMANOVA) by Adonis; n = 31 in each group). Ellipses cover 68% of the data for BM and NS samples. The percentage of variation indicated in each axis corresponds to the fraction of the total variance explained by the projection. (B) Taxonomic cladogram from linear discriminant analysis (LDA) effect size (LefSe) showing microbiota differences in BM and NS taxa. Dot size is proportional to the abundance of the taxon. The yellow circles represent the classification with no significant difference. P, phylum; c, class; f, family; o, order; g, genus.
We then analyzed the enrichment of OTUs according to their taxonomy using Manhattan plots (Supplementary Figure S1C). Compared with the NS, OTUs enriched in the BM belonged to the genera Prevotella and Erwinia, while OTUs depleted in the BM were commonly in the genera Bacteroides, Phocaeicola, Staphylococcus, Agathobacter, and Kineothrix (FDR < 0.05, Wilcoxon rank-sum test; Supplementary Figure S1C). Additionally, the LEfSe analysis including taxonomic cladogram (Figure 3B) and linear discriminant analysis (LDA) scores (LDA values higher than 4.0, p = 0.05) (Supplementary Figure S1D) also determined microbiota unique to the BM, including Bifidobacterium at the genus level, Enterobacteriaceae at the family level, Lactobacillales and Pseudomonadales at the order level, Actinobacteria and Gammaproteobacteria at the class level, and Proteobacteria at the phylum level, which was totally different from the NS. The unique NS microbiota included Bacteroides, Staphylococcus, and Parabacteroides at the genus level, Lachnospiraceae, Bacteroidaceae, and Porphyromonadaceae at the family level, and Bacillales at the order level.
Possible factors, such as the parity, delivery method, IAP, gestational age, and gender of the newborn, that might affect the microbiota diversity were further explored. On the other hand, we tried to apply LEfSe to analyze the characteristic microbiota taxa for different influencing factors and verify them using edgeR for subsequent studies on the characteristic microbiota. Only taxa with LDA values higher than 3.0 were presented (Supplementary Figure S2), and the LDA value for each lineage was listed. The gender of the newborn had no significant effect on the α and β diversity values of the BM microbiota (Figures 4A,B). The LEfSe analysis showed that the BM of female newborns was rich in Streptococcaceae at the family level, while the BM of male newborns was rich in Roseburia and Alcaligenaceae (Figure 4A). In addition, the edgeR verified that Roseburia was enriched at the genus level (data not shown). Parity had no significant effect on the α and β diversity values of the BM microbiota (Figures 4E,F). Also, the delivery mode had no significant effect on the α and β diversity of the BM microbiota (Figures 4C,D). However, LEfSe analysis showed that the BM microbiota from mothers who delivered via CS was rich in Bifidobacterium (Figure 4B), which was supported by edgeR (data not shown). IAP during delivery also had no significant effect on the α and β diversity of the BM microbiota. Moreover, the two antibiotics administered to mothers (cefazolin sodium and cefuroxime sodium) had no significant effect on the microbiota diversity of BM (Figures 4G,H). However, LEfSe analysis found that mothers who did receive IAP had BM that was rich in Lachnospiraceae at the genus level, which was verified by edgeR. Those who did not receive IAP were rich in Lactobacillus in BM (Supplementary Figure S2C). Gestational age also had no significant effect on the α and β diversity of the BM microbiota (Figures 4I,J). However, mothers who delivered a full-term infant(s) had BM that was rich in Bifidobacteria, as verified by LEfSe and edgeR (Supplementary Figure S2D).
Figure 4. Different factors had no influence on the α and β diversity of the microbiota of BM. (A) The α diversity of newborn gender (Female and Male). (B) The β diversity of newborn gender (Female and Male). (C) The α diversity of the delivery mode (Cesarean and Delivery). (D) The β diversity of the delivery mode (Cesarean and Delivery). (E) The α diversity of parity (Parturient and Primipara), and (F) the β diversity of parity (Parturient and Primipara). (G) The α diversity of intrapartum antibiotic prophylaxis (Cefazolin sodium, Cefuroxime sodium, and Non-antibiotics), and (H) the β diversity of intrapartum antibiotics prophylaxis (Cefazolin sodium, Cefuroxime sodium, and Non-antibiotics). (I) The α diversity of gestational age (Full-term and Non-full-term). (J) The β diversity of gestational age (Full-term and Non-full-term).
The ideal and most common state for mother and newborn is to be together, that is, the infant is immediately placed on the mother’s skin after birth and encouraged to nurse. This allows the newborn to consume BM, which provides a variety of nutrients that promote healthy programming (Cortes-Macias et al., 2021), as well as a multitude of immunomodulating components, such as soluble immunoglobulin A and growth factors that can reduce the risk of infectious diseases (Chi et al., 2021). However, not all mothers and newborns can be together after birth due to various factors, such as premature birth and amniotic fluid inhalation. Once the mother and baby are separated due to neonatal diseases or some other reasons in China, some mothers struggle to maintain and/or produce BM, resulting in a low supply of BM, which may affect the health of the newborn. The participants in this study were mothers who were separated from their newborns immediately after delivery. Their BM was sampled for studying the microbiota and exploring the origin of microbiota. The aim of this study was to provide a basis for continuous lactation in mothers separated from their newborns and encourage the mothers to provide BM to their newborns during separation.
Through breastfeeding, newborns and infants are exposed to microorganisms in BM, which stimulate the intestinal immune function of newborns and infants. The BM microbiota aids the development and functional maturity of the intestinal immune system and reduces the susceptibility to disease (Gollwitzer and Marsland, 2015). Thus, BM is recognized as a major force that shapes the infant gut microbiome in early life. Currently, little is known about the composition of the BM microbiota, and even less is known about the factors that determine it (Zimmermann and Curtis, 2020). To our knowledge, this is the first study to explore the source and composition of the colostrum (first stage of BM) microbiota in a large cohort of Southern Chinese mothers immediately separated from their newborns after delivery. We carefully designed and adopted an aseptic sampling protocol and sample preservation, as well as the elimination of the first drip, which included a few microliters to milliliters of BM, to avoid external contamination and ensure the accuracy and reliability of the results.
Colostrum, generally produced within the fifth day after delivery, is the first stage of BM during lactation. It is rich in proteins and minerals, and contains many immune-active substances, such as antibodies and complement factors (Ballard and Morrow, 2013). It has been reported that colostrum is also rich in microorganisms. Our study analyzed the microbiota of colostrum and NS from mothers who were immediately separated from their newborns after delivery. The rarefaction curves demonstrated that all BM and NS samples reached standard quality requirements for sequencing, and all samples were sequenced sufficiently (Figure 1A). Moreover, α diversity of the BM microbiota was assessed via richness, Chao1, and Shannon indices (Figures 1B–D). The dominant phyla in colostrum (BM) were Firmicutes and Proteobacteria, which was consistent with previous studies (Urbaniak et al., 2016; Biagi et al., 2017; Murphy et al., 2017; Pannaraj et al., 2017; Simpson et al., 2018; Hermansson et al., 2019; Moossavi et al., 2019). However, the next most abundant phyla were Bacteroidetes, Actinobacteria, and Fusobacteria (Figure 2A), which differed from previous reports.
At the genus level, the bacteria unique to the colostrum (BM) were Pantoea, Pseudomonas, Bifidobacterium, Klebsiella, and Escherichia-Shigella (Figure 2C vs. Figure 2D). The microbiota shared with the NS was Bacteroide, Faecalibacterium, uncultured_bacterium_f_Lachnospiraceae, Prevotella_9, and Roseburia (Figure 2B). These results differed from full-term newborns who routinely suck their mother’s breast after birth, which had higher relative abundances of Weisella, Leuconostoc, Streptococcus (Urbaniak et al., 2016), and Lactococcus (Cabrera-Rubio et al., 2012). Notably, the genus Staphylococcus has been reported to have higher relative abundances during the first 10 days of life (Cabrera-Rubio et al., 2012; Sakwinska et al., 2016; Simpson et al., 2018), in addition to being universally observed in mature BM (Solis et al., 2010; Jost et al., 2013) by pyrosequencing. However, in our study, Staphylococcus was found to be predominant in the NS microbiota (3.03%). Staphylococcus was also found in the colostrum, although at a much lower abundance (1.52%), and was particularly depleted in BM compared with NS (Supplementary Figure S1C). Thus, the origin and types of Staphylococcus in BM and NS are worthy of further study.
The composition of the microbiota in reports varies widely, suggesting that the purpose of the studies, geographical location (Kumar et al., 2016; Li et al., 2017; Ding et al., 2019), ethnic differences, sampling volunteers (Ruiz et al., 2019), collection methods, and methodological differences (Sakwinska et al., 2016) may influence the results.
The microbiota of BM has been hypothesized to originate from commensal and potential probiotic bacteria colonized in the intestine of newborns. Through temperature gradient gel electrophoresis (TGGE) (Perez et al., 2007), pyrosequencing (Jost et al., 2012, 2014), and culture methods (Solis et al., 2010; Jost et al., 2012), Bifidobacterium strains, which are gut-associated obligate anaerobes, were found to be shared between maternal feces, BM, and neonatal feces, especially during the first week. Accordingly, our results also found that the relative abundance of Bifidobacterium at the genus level in colostrum was high (Figures 2C, 4B and Supplementary Figure S1D). Thus, our results support that mothers separated from their newborns should maintain lactation and feed colostrum to their babies in the NICU in order to promote the establishment of normal intestinal microbiota.
Currently, it is still unclear how microbes reach the BM. Most previous studies have suggested the traditional contamination hypothesis (such as the mother’s skin and infant’s oral cavity). One suggested that mammary ducts become colonized by the infant oral microbiota during suckling, as the retrograde flow of BM into mammary ducts has been documented (Ramsay et al., 2004). However, other studies arrived at different conclusions. For example, buccal administration of colostrum to low-birth-weight newborns in the NICU changed their oral microbiota when compared to infants who were given standard care, suggesting that the BM might be responsible for colonizing the infant’s mouth (Sohn et al., 2016). Additionally, precolostrum already contains bacteria before suckling has occurred (Ruiz et al., 2019). Other studies have also suggested that bacteria from the skin (such as Corynebacterium, Cutibacterium, and Staphylococcus) colonize the mammary ducts. However, studies in recent years have suggested the revolutionary active migration hypothesis, which supports the existence of an entero-mammary pathway.
One of the main purposes of this study was to provide a practical and reliable basis for determining the source of bacteria in the BM. In order to avoid contamination by the infant’s oral cavity, we enrolled healthy, lactating women who were immediately separated from their newborn(s) after delivery in this study. The newborns did not suck the mother’s breast. To avoid the influence of skin microbiota, we washed the surrounding NS with sterile water, collected cleaning water, and then collected the BM after squeezing out the first drop of colostrum. Both the NS and BM samples were analyzed by 16S rRNA gene sequencing targeting the V3–V4 region to compare the composition differences between the microbiota.
Our results showed that there were shared microorganisms between the BM and NS microbiota. As shown in the Venn diagram, there were 170 overlapping OTUs (Supplementary Figure S1A) and no significant OTUs in the Volcanic map (Supplementary Figure S1B), as well as no significant bacterial classification level in the Manhattan plot (Supplementary Figure S1C). However, there were also significant differences in the microbiota diversity between BM and NS. The Venn diagram displayed 111 OTUs unique to BM and 87 unique to NS, while 77 OUTs were depleted and 107 were enriched in BM compared with NS through a Volcanic map (Supplementary Figure S1B) for advanced differential abundance analysis. PCoA for the β diversity demonstrated two different populations, BM and NS, in the principal coordinate PCo1, explaining 21.49% of the variance (Figure 3A). The unique microbiota in BM was screened by LEfSe analysis. Anaerobic bacteria found in the colostrum (BM), which are not found on the skin (Aakko et al., 2017; Damaceno et al., 2017; Williams et al., 2017; Parnanen et al., 2018), include Bifidobacterium at the genus level, Enterobacteriaceae at the family level, Lactobacillales and Pseudomonadales at the order level, Actinobacteria and Gammaproteobacteria at the class level, and Proteobacteria at the phylum level. Similar to our results regarding Lactobacillales at the order level in the BM, a study displayed that Lactobacillus present in BM are genotypically different from those detected on the skin within individuals (Martin et al., 2007b). While in NS, the predominance of Staphylococcus at the genus level was universally observed in other studies (Solis et al., 2010; Jost et al., 2013).
Excluding neonatal oral contamination, breast skin contamination, and microbiota shared by the NS, the source of bacteria in the breast is quite amazing. As many of the bacteria found in BM can also be found in the intestine, it is plausible that an entero-mammary pathway exists, that is, intestinal organisms, or their DNA, can be transferred from the intestine to the mammary ducts (Zimmermann and Curtis, 2020). Gut-associated obligate anaerobic genera, like Bifidobacterium and Lactobacillales, have been identified by pyrosequencing to be shared among maternal feces, BM, and neonatal feces (Jost et al., 2014; Cortes-Macias et al., 2021).
Therefore, the entero-mammary pathway hypothesis of BM microbiota might be able to explain this. Our results of unique taxa enriched in the BM, including Bifidobacterium, Enterobacteriaceae, Lactobacillales, and Pseudomonadales, as well as Gammaproteobacteria and even Proteobacteria, were likely transferred from the intestine to the breast through the intestinal tract.
Previous studies have verified that when Lactobacillus is administered as a probiotic to women, the same strain can be identified in BM (Jimenez et al., 2008; Abrahamsson et al., 2009). Translocation of bacteria from the intestine to the mammary glands is thought to mainly occur through gut-associated lymphoid tissues (Vazquez-Torres et al., 1999; Qutaishat et al., 2003) and involves dendritic cells and macrophages (Vazquez-Torres et al., 1999; Rescigno et al., 2001; Qutaishat et al., 2003). Moreover, the translocation of the microbiota has been reported to increase in pregnant or lactating women (Perez et al., 2007). A previous study reported the transmission of Salmonella enterica to infants through the mother’s BM. It was proposed that there is a biologically feasible mechanism of transport of Salmonella from the gastrointestinal tract to BM. Salmonella is resistant to the acidic environment of the stomach and usually invades or is phagocytosed by cells lining the Peyer’s patches in the small intestine. Specialized epithelial M cells overlying the lymphoid follicles of Peyer’s patches provide a portal of entry for Salmonella enterica Typhimurium. The pathogenicity island 1 of S. enterica Typhimurium was required to penetrate these intestinal epithelial M cells. S. enterica Typhimurium is transported from the gastrointestinal tract to the bloodstream by CD18-expressing phagocytic leukocytes and may use macrophages and dendritic cells as a conduit to deeper tissue (Qutaishat et al., 2003).
For non-pathogenic microorganisms, a previous study reported that dendritic cells may express tight junction proteins, which open the tight junctions between epithelial cells, allowing them to penetrate the gut epithelial monolayers to sample bacteria (Rescigno et al., 2001). In future studies, we will conduct a series of animal experiments to follow non-pathogenic bacteria in maternal rats to explore their effects on the BM microbiota, intestinal-breast pathway, establishment of intestinal microbiota, and intestinal immunity.
In this study, various factors, including the newborn gender, delivery mode, parity, gestational age, and intrapartum antibiotics exposure (Supplementary Table S1), were considered to influence the microbiota diversity of colostrum (BM). Concerning the impact of the newborn gender, a study reported a lower diversity and richness in the microbiota of 3–4 months postpartum BM (mature milk) among mothers of male infants. We found no differences in the α and β indices of colostrum microbiota (Figures 4A,B). This may be due to the fact that BM has different lactation stages. The mothers of female newborns had a higher relative abundance of Streptococcaceae at the family level, which was the opposite result of another study which reported that mothers of male infants had a higher relative abundance of Streptococcus in mature milk (Williams et al., 2017). Mothers of male newborns in our study had a higher relative abundance of Roseburia at the genus level than those of female newborns (Supplementary Figure S2A). Gamez-Valdez et al. (2021) found that the BM microbiota was influenced by the infant gender in the obesity mother subgroup, with phylogenetic diversity of the female newborns more diverse than in males. Thus, the complex relationship between women’s obesity and gestational diabetes mellitus and the influence of newborn gender on the microbiota composition may be worthy of deep study.
We concluded that the delivery mode did not affect the α and β indices of the BM microbiota diversity (Figures 4C,D), which was similar to another study (Li et al., 2017). Furthermore, we found that women who delivered by CS had a higher relative abundance of Bifidobacterium and E. coli-Shigella in colostrum (BM) (Figure 4B). Thus, it could be suggested that CS might lead to increased intestinal permeability, enhanced bacterial translocation in the maternal gut, and consequently, a higher transfer of bacteria to the BM.
The conclusion of the studies showed that the effect of parity on the microbiota of BM included two aspects. One aspect was that the diversity in the BM microbiota was lower in infants who were first born as compared with those who had one or more siblings (Moossavi et al., 2019). The second aspect was that parity had no effect on the diversity of the BM microbiota. Our results were in favor of the latter (Figures 4E,F), and all microbiota were shared by parturient and primipara mothers.
It has been reported that women who receive IAP had a higher α diversity and richness in their BM microbiota, Bifidobacterium was not found (Hermansson et al., 2019), and a lower relative abundance of Lactobacilli was found (Soto et al., 2014). We found that IAP (cefazolin sodium was given intravenously to 14 mothers and cefuroxime sodium to 8 mothers) had no effect on the microbiota diversity of colostrum (BM) (Figures 4G,H). Though there was no Bifidobacterium in non-antibiotics BM as assessed by LDA scores, the BM was rich in Lactobacillus at the genus level (Supplementary Figure S2C). According to the American College of Obstetricians and Gynecologists (ACOG), IAP is recommended for all women undergoing C-section, and the first generation of cephalosporin, such as cefazolin sodium, is preferred. Fourteen mothers undergoing C-section in this study were given cephalosporin, including cefazolin sodium and cefuroxime sodium. The other eight mothers received cephalosporin prophylactically because of maternal Streptococcus B infection or premature rupture of membranes. They accepted antibiotics according to the guidelines. The half-life of cephalosporins is short, so the effect of these antibiotics on the BM microbiota was minimal by the time we collected the mothers’ BM on the third day after delivery (the average sampling days were 3 after delivery). Moreover, the type or level of antibiotics did not significantly affect the diversity of the colostrum microbiota. Additionally, Streptococcus was not found in the colostrum of mothers infected with Streptococcus B, indicating that this pathogenic bacterium did not enter the BM, or at least, was not found in the colostrum after the administration of antibiotics.
A few studies have explored the influence of gestational age on the BM microbiota (Khodayar-Pardo et al., 2014; Urbaniak et al., 2016). One study investigated a higher relative and absolute abundance of Bifidobacterium in women who delivered at full-term (Khodayar-Pardo et al., 2014), which was the same as our results in colostrum (BM) (Supplementary Figure S2D). Otherwise, we found no effect of gestational age on the microbiota composition of colostrum (BM) (Figures 4I,J), which was consistent with another study (Urbaniak et al., 2016).
As the first attempt to explore the microbiota of BM, we only analyzed the colostrum and NS microbiota in this study, without the collection and analysis of stool from mothers. Therefore, we will expand the sample number and continuously explore the microbiota changes in colostrum, transitional milk, and mature BM compared to the maternal stool to provide further evidence regarding the origin of the BM microbiota. Overall, illuminating the potential factors affecting the differential bacterial taxa of the BM microbiota, such as delivery mode, gestational age, and IAP, was meaningful and allows for in-depth research in the future.
Our study further substantiates the presence and diversity of a microbiota specific to colostrum (BM) among mothers separated from their newborns. Furthermore, the comparison of the microbiota diversity between BM and NS provides reliable evidence for verifying the entero-mammary bacterial translocation hypothesis. According to our results of probiotics, such as Bifidobacterium in colostrum, and no effect of intrapartum antibiotics on microbiota abundance, we support mothers to maintain lactation and pump BM for newborns in NICU.
However, our results need to be further studied for confirmation. The impact of the BM microbiota on neonatal gut microbiota establishment, immunity function, and health consequences are worthy of further study.
The datasets presented in this study can be found in online repositories. The names of the repository/repositories and accession number(s) can be found below: NCBI—PRJNA848210.
The studies involving human participants were reviewed and approved by the Shenzhen Luohu Maternity and Child Healthcare Hospital (No. LL2022051337). The patients/participants provided their written informed consent to participate in this study. Written informed consent was obtained from the individual(s) for the publication of any potentially identifiable images or data included in this article.
YD, ZHH, and RX performed the conception and design of the work and drafted the article. YD, QQ, and LW collected the samples and the data. ZOH and XW performed the data analysis and interpretation. ZOH and GJ performed the critical revision of the article. XW and GJ performed the final approval of the version to be published. All authors contributed to the article and approved the submitted version.
This work was supported by the National Natural Science Foundation of China (No. 81902022), Shenzhen Polytechnic Funds (Nos. 6021310006K, 6019260143K, and 601826K27119), and Science and Technology Foundation of Shenzhen City (No. JCYJ20210324102814038).
We would like to thank the participants who volunteered for this study. Minghua Li and Meijun Yin in the Central Laboratory of Peking University Shenzhen Hospital supported the sample collection and storage. We thank LetPub (www.letpub.com) for linguistic assistance and pre-submission expert review.
The authors declare that the research was conducted in the absence of any commercial or financial relationships that could be construed as a potential conflict of interest.
All claims expressed in this article are solely those of the authors and do not necessarily represent those of their affiliated organizations, or those of the publisher, the editors and the reviewers. Any product that may be evaluated in this article, or claim that may be made by its manufacturer, is not guaranteed or endorsed by the publisher.
The Supplementary Material for this article can be found online at: https://www.frontiersin.org/articles/10.3389/fmicb.2022.932495/full#supplementary-material
Supplementary Figure S1 | Supplementary methods to describe the differences in the microbiota between BM (colostrum) and (A) A Venn diagram representing the overlapping and unique OTUs between BM and NS. (B) Taxonomic and functional characteristics of differential bacteria between the BM and NS microbiota. (C) Manhattan plot showing OTUs enriched in the BM as compared to NS. Each triangle represents a sing out OTU. OTUs enriched in BM or NS are represented by filled or empty triangles, respectively (FDR < 0.005, Wilcoxon rank-sum test). OTUs are arranged in taxonomic order and colored according to the genus. The size of the triangle represents the abundance of OTUs. (D) Linear discriminant analysis (LDA) scores computed for differentially abundant taxa in the microbiomes of NS (blue) and BM (red). Length indicates effect size associated with a taxon; P = 0.05 for the Kruskal–Wallis H-test; LDA score = 4.
Supplementary Figure S2 | Differential bacterial taxa in the microbiome of NS (green) and BM (red) in different groups by linear discriminant analysis (LDA) scores. The length indicates the effect size associated with a taxon; p = 0.05 for the Kruskal–Wallis H-test; LDA score = 3.
Supplementary Table S1 | The demographics of the participants.
Aakko, J., Kumar, H., Rautava, S., Wise, A., Autran, C., Bode, L., et al. (2017). Human milk oligosaccharide categories define the microbiota composition in human colostrum. Benef. Microbes 8, 563–567. doi: 10.3920/BM2016.0185
Abrahamsson, T. R., Sinkiewicz, G., Jakobsson, T., Fredrikson, M., and Bjorksten, B. (2009). Probiotic lactobacilli in breast milk and infant stool in relation to oral intake during the first year of life. J. Pediatr. Gastroenterol. Nutr. 49, 349–354. doi: 10.1097/MPG.0b013e31818f091b
Arenz, S., Ruckerl, R., Koletzko, B., and von Kries, R. (2004). Breast-feeding and childhood obesity–a systematic review. Int. J. Obes. Relat. Metab. Disord. 28, 1247–1256. doi: 10.1038/sj.ijo.0802758
Ballard, O., and Morrow, A. L. (2013). Human milk composition: Nutrients and bioactive factors. Pediatr. Clin. North Am. 60, 49–74. doi: 10.1016/j.pcl.2012.10.002
Biagi, E., Quercia, S., Aceti, A., Beghetti, I., Rampelli, S., Turroni, S., et al. (2017). The bacterial ecosystem of mother’s milk and infant’s mouth and gut. Front. Microbiol. 8:1214. doi: 10.3389/fmicb.2017.01214
Cabrera-Rubio, R., Collado, M. C., Laitinen, K., Salminen, S., Isolauri, E., and Mira, A. (2012). The human milk microbiome changes over lactation and is shaped by maternal weight and mode of delivery. Am. J. Clin. Nutr. 96, 544–551. doi: 10.3945/ajcn.112.037382
Cabrera-Rubio, R., Mira-Pascual, L., Mira, A., and Collado, M. C. (2016). Impact of mode of delivery on the milk microbiota composition of healthy women. J. Dev. Orig. Health Dis. 7, 54–60. doi: 10.1017/S2040174415001397
Chi, C., Fan, Y., Li, C., Li, Y., Guo, S., Li, T., et al. (2021). Early gut microbiota colonisation of premature infants fed with breastmilk or formula with or without probiotics: A cohort study. Nutrients 13:4068. doi: 10.3390/nu13114068
Cortes-Macias, E., Selma-Royo, M., Martinez-Costa, C., and Collado, M. C. (2021). Breastfeeding practices influence the breast milk microbiota depending on pre-gestational maternal BMI and weight gain over pregnancy. Nutrients 13:1518. doi: 10.3390/nu13051518
Damaceno, Q. S., Souza, J. P., Nicoli, J. R., Paula, R. L., Assis, G. B., Figueiredo, H. C., et al. (2017). Evaluation of potential probiotics isolated from human milk and colostrum. Probiotics Antimicrob. Proteins 9, 371–379. doi: 10.1007/s12602-017-9270-1
Ding, M., Qi, C., Yang, Z., Jiang, S., Bi, Y., Lai, J., et al. (2019). Geographical location specific composition of cultured microbiota and Lactobacillus occurrence in human breast milk in China. Food Funct. 10, 554–564. doi: 10.1039/c8fo02182a
Duijts, L., Jaddoe, V. W., Hofman, A., and Moll, H. A. (2010). Prolonged and exclusive breastfeeding reduces the risk of infectious diseases in infancy. Pediatrics 126, e18–e25. doi: 10.1542/peds.2008-3256
Edgar, R. C. (2010). Search and clustering orders of magnitude faster than BLAST. Bioinformatics 26, 2460–2461. doi: 10.1093/bioinformatics/btq461
Gamez-Valdez, J. S., Garcia-Mazcorro, J. F., Montoya-Rincon, A. H., Rodriguez-Reyes, D. L., Jimenez-Blanco, G., Rodriguez, M. T. A., et al. (2021). Differential analysis of the bacterial community in colostrum samples from women with gestational diabetes mellitus and obesity. Sci. Rep. 11:24373. doi: 10.1038/s41598-021-03779-7
Gollwitzer, E. S., and Marsland, B. J. (2015). Impact of early-life exposures on immune maturation and susceptibility to disease. Trends Immunol. 36, 684–696. doi: 10.1016/j.it.2015.09.009
Gueimonde, M., Laitinen, K., Salminen, S., and Isolauri, E. (2007). Breast milk: A source of bifidobacteria for infant gut development and maturation? Neonatology 92, 64–66. doi: 10.1159/000100088
Hansen, K. (2016). Breastfeeding: A smart investment in people and in economies. Lancet 387:416. doi: 10.1016/S0140-6736(16)00012-X
Heikkila, M. P., and Saris, P. E. (2003). Inhibition of Staphylococcus aureus by the commensal bacteria of human milk. J. Appl. Microbiol. 95, 471–478. doi: 10.1046/j.1365-2672.2003.02002.x
Hermansson, H., Kumar, H., Collado, M. C., Salminen, S., Isolauri, E., and Rautava, S. (2019). Breast milk microbiota is shaped by mode of delivery and intrapartum antibiotic exposure. Front. Nutr. 6:4. doi: 10.3389/fnut.2019.00004
Herrmann, K., and Carroll, K. (2014). An exclusively human milk diet reduces necrotizing enterocolitis. Breastfeed Med. 9, 184–190. doi: 10.1089/bfm.2013.0121
Jimenez, E., de Andres, J., Manrique, M., Pareja-Tobes, P., Tobes, R., Martinez-Blanch, J. F., et al. (2015). Metagenomic analysis of milk of healthy and mastitis-suffering women. J. Hum. Lact. 31, 406–415. doi: 10.1177/0890334415585078
Jimenez, E., Fernandez, L., Maldonado, A., Martin, R., Olivares, M., Xaus, J., et al. (2008). Oral administration of Lactobacillus strains isolated from breast milk as an alternative for the treatment of infectious mastitis during lactation. Appl. Environ. Microbiol. 74, 4650–4655. doi: 10.1128/AEM.02599-07
Jost, T., Lacroix, C., Braegger, C. P., and Chassard, C. (2012). New insights in gut microbiota establishment in healthy breast fed neonates. PLoS One 7:e44595. doi: 10.1371/journal.pone.0044595
Jost, T., Lacroix, C., Braegger, C. P., Rochat, F., and Chassard, C. (2014). Vertical mother-neonate transfer of maternal gut bacteria via breastfeeding. Environ. Microbiol. 16, 2891–2904. doi: 10.1111/1462-2920.12238
Jost, T., Lacroix, C., Braegger, C., and Chassard, C. (2013). Assessment of bacterial diversity in breast milk using culture-dependent and culture-independent approaches. Br. J. Nutr. 110, 1253–1262. doi: 10.1017/S0007114513000597
Khodayar-Pardo, P., Mira-Pascual, L., Collado, M. C., and Martinez-Costa, C. (2014). Impact of lactation stage, gestational age and mode of delivery on breast milk microbiota. J. Perinatol. 34, 599–605. doi: 10.1038/jp.2014.47
Kumar, H., du Toit, E., Kulkarni, A., Aakko, J., Linderborg, K. M., Zhang, Y., et al. (2016). Distinct patterns in human milk microbiota and fatty acid profiles across specific geographic locations. Front. Microbiol. 7:1619. doi: 10.3389/fmicb.2016.01619
Lanari, M., Prinelli, F., Adorni, F., Di Santo, S., Faldella, G., Silvestri, M., et al. (2013). Maternal milk protects infants against bronchiolitis during the first year of life. Results from an Italian cohort of newborns. Early Hum. Dev. 89 Suppl 1, S51–S57. doi: 10.1016/S0378-3782(13)70016-1
Li, S. W., Watanabe, K., Hsu, C. C., Chao, S. H., Yang, Z. H., Lin, Y. J., et al. (2017). Bacterial Composition and diversity in breast milk samples from mothers living in Taiwan and Mainland China. Front. Microbiol. 8:965. doi: 10.3389/fmicb.2017.00965
Liu, Y. X., Qin, Y., Chen, T., Lu, M., Qian, X., Guo, X., et al. (2021). A practical guide to amplicon and metagenomic analysis of microbiome data. Protein Cell 12, 315–330. doi: 10.1007/s13238-020-00724-8
Lozupone, C. A., Hamady, M., Kelley, S. T., and Knight, R. (2007). Quantitative and qualitative beta diversity measures lead to different insights into factors that structure microbial communities. Appl. Environ. Microbiol. 73, 1576–1585. doi: 10.1128/AEM.01996-06
Makino, H., Kushiro, A., Ishikawa, E., Muylaert, D., Kubota, H., Sakai, T., et al. (2011). Transmission of intestinal Bifidobacterium longum subsp. longum strains from mother to infant, determined by multilocus sequencing typing and amplified fragment length polymorphism. Appl. Environ. Microbiol. 77, 6788–6793. doi: 10.1128/AEM.05346-11
Martin, R., Heilig, G. H., Zoetendal, E. G., Smidt, H., and Rodriguez, J. M. (2007a). Diversity of the Lactobacillus group in breast milk and vagina of healthy women and potential role in the colonization of the infant gut. J. Appl. Microbiol. 103, 2638–2644. doi: 10.1111/j.1365-2672.2007.03497.x
Martin, R., Heilig, H. G., Zoetendal, E. G., Jimenez, E., Fernandez, L., Smidt, H., et al. (2007b). Cultivation-independent assessment of the bacterial diversity of breast milk among healthy women. Res. Microbiol. 158, 31–37. doi: 10.1016/j.resmic.2006.11.004
Moossavi, S., Sepehri, S., Robertson, B., Bode, L., Goruk, S., Field, C. J., et al. (2019). Composition and variation of the human milk microbiota are influenced by maternal and early-life factors. Cell Host Microbe 25, 324–335.e4. doi: 10.1016/j.chom.2019.01.011
Murphy, K., Curley, D., O’Callaghan, T. F., O’Shea, C. A., Dempsey, E. M., O’Toole, P. W., et al. (2017). The composition of human milk and infant faecal microbiota over the first three months of life: A pilot study. Sci. Rep. 7:40597. doi: 10.1038/srep40597
Pannaraj, P. S., Li, F., Cerini, C., Bender, J. M., Yang, S., Rollie, A., et al. (2017). Association between breast milk bacterial communities and establishment and development of the infant gut microbiome. JAMA Pediatr. 171, 647–654. doi: 10.1001/jamapediatrics.2017.0378
Parnanen, K., Karkman, A., Hultman, J., Lyra, C., Bengtsson-Palme, J., Larsson, D. G. J., et al. (2018). Maternal gut and breast milk microbiota affect infant gut antibiotic resistome and mobile genetic elements. Nat. Commun. 9:3891. doi: 10.1038/s41467-018-06393-w
Perez, P. F., Dore, J., Leclerc, M., Levenez, F., Benyacoub, J., Serrant, P., et al. (2007). Bacterial imprinting of the neonatal immune system: Lessons from maternal cells? Pediatrics 119, e724–e732. doi: 10.1542/peds.2006-1649
Qutaishat, S. S., Stemper, M. E., Spencer, S. K., Borchardt, M. A., Opitz, J. C., Monson, T. A., et al. (2003). Transmission of Salmonella enterica serotype typhimurium DT104 to infants through mother’s breast milk. Pediatrics 111(6 Pt 1), 1442–1446. doi: 10.1542/peds.111.6.1442
Ramsay, D. T., Kent, J. C., Owens, R. A., and Hartmann, P. E. (2004). Ultrasound imaging of milk ejection in the breast of lactating women. Pediatrics 113, 361–367. doi: 10.1542/peds.113.2.361
Rescigno, M., Urbano, M., Valzasina, B., Francolini, M., Rotta, G., Bonasio, R., et al. (2001). Dendritic cells express tight junction proteins and penetrate gut epithelial monolayers to sample bacteria. Nat. Immunol. 2, 361–367. doi: 10.1038/86373
Robinson, M. D., McCarthy, D. J., and Smyth, G. K. (2010). edgeR: A Bioconductor package for differential expression analysis of digital gene expression data. Bioinformatics 26, 139–140. doi: 10.1093/bioinformatics/btp616
Ruiz, L., Bacigalupe, R., Garcia-Carral, C., Boix-Amoros, A., Arguello, H., Silva, C. B., et al. (2019). Microbiota of human precolostrum and its potential role as a source of bacteria to the infant mouth. Sci. Rep. 9:8435. doi: 10.1038/s41598-019-42514-1
Sakwinska, O., Moine, D., Delley, M., Combremont, S., Rezzonico, E., Descombes, P., et al. (2016). Microbiota in breast milk of chinese lactating mothers. PLoS One 11:e0160856. doi: 10.1371/journal.pone.0160856
Sanz, Y. (2011). Gut microbiota and probiotics in maternal and infant health. Am. J. Clin. Nutr. 94(6 Suppl), 2000S–2005S. doi: 10.3945/ajcn.110.001172
Segata, N., Izard, J., Waldron, L., Gevers, D., Miropolsky, L., Garrett, W. S., et al. (2011). Metagenomic biomarker discovery and explanation. Genome Biol. 12:R60. doi: 10.1186/gb-2011-12-6-r60
Sheng, D., Zhao, S., Gao, L., Zheng, H., Liu, W., Hou, J., et al. (2019). BabaoDan attenuates high-fat diet-induced non-alcoholic fatty liver disease via activation of AMPK signaling. Cell Biosci. 9:77. doi: 10.1186/s13578-019-0339-2
Simpson, M. R., Avershina, E., Storro, O., Johnsen, R., Rudi, K., and Oien, T. (2018). Breastfeeding-associated microbiota in human milk following supplementation with Lactobacillus rhamnosus GG, Lactobacillus acidophilus La-5, and Bifidobacterium animalis ssp. Lactis Bb-12. J. Dairy Sci. 101, 889–899. doi: 10.3168/jds.2017-13411
Sohn, K., Kalanetra, K. M., Mills, D. A., and Underwood, M. A. (2016). Buccal administration of human colostrum: Impact on the oral microbiota of premature infants. J. Perinatol. 36, 106–111. doi: 10.1038/jp.2015.157
Solis, G., de Los Reyes-Gavilan, C. G., Fernandez, N., Margolles, A., and Gueimonde, M. (2010). Establishment and development of lactic acid bacteria and bifidobacteria microbiota in breast-milk and the infant gut. Anaerobe 16, 307–310. doi: 10.1016/j.anaerobe.2010.02.004
Soto, A., Martin, V., Jimenez, E., Mader, I., Rodriguez, J. M., and Fernandez, L. (2014). Lactobacilli and bifidobacteria in human breast milk: Influence of antibiotherapy and other host and clinical factors. J. Pediatr. Gastroenterol. Nutr. 59, 78–88. doi: 10.1097/MPG.0000000000000347
Tromp, I., Kiefte-de Jong, J., Raat, H., Jaddoe, V., Franco, O., Hofman, A., et al. (2017). Breastfeeding and the risk of respiratory tract infections after infancy: The generation R study. PLoS One 12:e0172763. doi: 10.1371/journal.pone.0172763
Urbaniak, C., Angelini, M., Gloor, G. B., and Reid, G. (2016). Human milk microbiota profiles in relation to birthing method, gestation and infant gender. Microbiome 4:1. doi: 10.1186/s40168-015-0145-y
Vazquez-Torres, A., Jones-Carson, J., Baumler, A. J., Falkow, S., Valdivia, R., Brown, W., et al. (1999). Extraintestinal dissemination of Salmonella by CD18-expressing phagocytes. Nature 401, 804–808. doi: 10.1038/44593
Wang, Y., Sheng, H. F., He, Y., Wu, J. Y., Jiang, Y. X., Tam, N. F., et al. (2012). Comparison of the levels of bacterial diversity in freshwater, intertidal wetland, and marine sediments by using millions of illumina tags. Appl. Environ. Microbiol. 78, 8264–8271. doi: 10.1128/AEM.01821-12
Ward, T. L., Hosid, S., Ioshikhes, I., and Altosaar, I. (2013). Human milk metagenome: A functional capacity analysis. BMC Microbiol. 13:116. doi: 10.1186/1471-2180-13-116
WHO (2001). The optimal duration of exclusive breastfeeding. Report of an expert consultation. Available online at: http://apps.who.int/iris/bitstream/10665/67219/1/WHO_NHD_01.09.pdf
Williams, J. E., Carrothers, J. M., Lackey, K. A., Beatty, N. F., York, M. A., Brooker, S. L., et al. (2017). Human milk microbial community structure is relatively stable and related to variations in macronutrient and micronutrient intakes in healthy lactating women. J. Nutr. 147, 1739–1748. doi: 10.3945/jn.117.248864
Yang, C., Xu, Z., Deng, Q., Huang, Q., Wang, X., and Huang, F. (2020). Beneficial effects of flaxseed polysaccharides on metabolic syndrome via gut microbiota in high-fat diet fed mice. Food Res. Int. 131:108994. doi: 10.1016/j.foodres.2020.108994
Keywords: microbiota, diversity, colostrum, nipple skin, mother–infant separation
Citation: Du Y, Qiu Q, Cheng J, Huang Z, Xie R, Wang L, Wang X, Han Z and Jin G (2022) Comparative study on the microbiota of colostrum and nipple skin from lactating mothers separated from their newborn at birth in China. Front. Microbiol. 13:932495. doi: 10.3389/fmicb.2022.932495
Received: 29 April 2022; Accepted: 02 September 2022;
Published: 03 October 2022.
Edited by:
Ekaterina Avershina, Oslo University Hospital, NorwayReviewed by:
Jaime Parra-Suescún, National University of Colombia, Medellin, ColombiaCopyright © 2022 Du, Qiu, Cheng, Huang, Xie, Wang, Wang, Han and Jin. This is an open-access article distributed under the terms of the Creative Commons Attribution License (CC BY). The use, distribution or reproduction in other forums is permitted, provided the original author(s) and the copyright owner(s) are credited and that the original publication in this journal is cited, in accordance with accepted academic practice. No use, distribution or reproduction is permitted which does not comply with these terms.
*Correspondence: Zongli Han, aGFuemwwMDdAMTYzLmNvbQ==; Xiangyu Wang, eGlhbmd5dXdhbmdzekAxNjMuY29t; Gang Jin, amluZ2FuZ0BzenB0LmRldS5jbg==
†These authors have contributed equally to this work and share first authorship
Disclaimer: All claims expressed in this article are solely those of the authors and do not necessarily represent those of their affiliated organizations, or those of the publisher, the editors and the reviewers. Any product that may be evaluated in this article or claim that may be made by its manufacturer is not guaranteed or endorsed by the publisher.
Research integrity at Frontiers
Learn more about the work of our research integrity team to safeguard the quality of each article we publish.