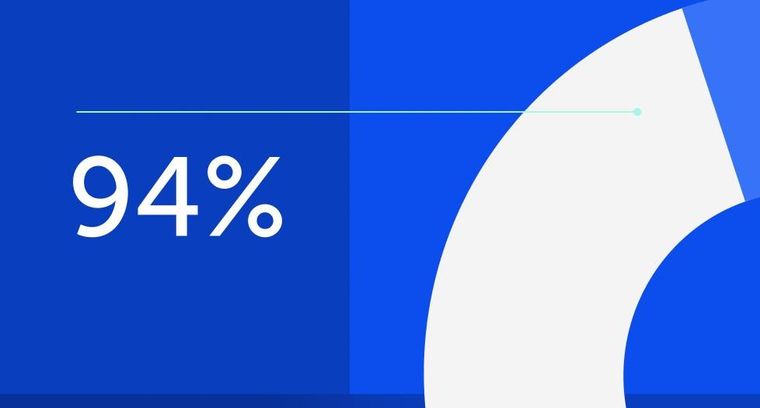
94% of researchers rate our articles as excellent or good
Learn more about the work of our research integrity team to safeguard the quality of each article we publish.
Find out more
REVIEW article
Front. Microbiol., 10 August 2022
Sec. Infectious Agents and Disease
Volume 13 - 2022 | https://doi.org/10.3389/fmicb.2022.932408
This article is part of the Research TopicRising Stars in Infectious Agents and Disease: 2021View all 5 articles
The fight against infectious diseases calls for the development of safe and effective vaccines that generate long-lasting protective immunity. In a few situations, vaccine-mediated immune responses may have led to exacerbated pathology upon subsequent infection with the pathogen targeted by the vaccine. Such vaccine-associated enhanced disease (VAED) has been reported, or at least suspected, in animal models, and in a few instances in humans, for vaccine candidates against the respiratory syncytial virus (RSV), measles virus (MV), dengue virus (DENV), HIV-1, simian immunodeficiency virus (SIV), feline immunodeficiency virus (FIV), severe acute respiratory syndrome coronavirus 1 (SARS-CoV-1), and the Middle East respiratory syndrome coronavirus (MERS-CoV). Although alleviated by clinical and epidemiological evidence, a number of concerns were also initially raised concerning the short- and long-term safety of vaccines against severe acute respiratory syndrome coronavirus 2 (SARS-CoV-2), which is causing the ongoing COVID-19 pandemic. Although the mechanisms leading to this phenomenon are not yet completely understood, the individual and/or collective role of antibody-dependent enhancement (ADE), complement-dependent enhancement, and cell-dependent enhancement have been highlighted. Here, we review mechanisms that may be associated with the risk of VAED, which are important to take into consideration, both in the assessment of vaccine safety and in finding ways to define models and immunization strategies that can alleviate such concerns.
Vaccination is considered to be one of the most-cost effective medical interventions of the 20th century (Préaud et al., 2015; Ozawa et al., 2016; Leidner et al., 2019): The significant decrease in global morbidity and mortality associated with a number of infections (Greenwood, 1645; Rappuoli et al., 2019) has synergized with the economic benefits in healthcare costs brought by the implementation of such preventive measures. Today, the goals of modern vaccinology in the context of infectious diseases are to: (A) create vaccines against (re)emerging diseases; (B) handle refractory pathogens for which efficient vaccines have proven difficult to develop, and (C) improve vaccination strategies, including vaccine accessibility (Greenwood, 1645; Centlivre and Combadière, 2015; Pollard and Bijker, 2020; Excler et al., 2021). Understanding and controlling the immune mechanisms elicited by such vaccines is crucial in moving forward in this quest. Beyond the fear of developing an ineffective vaccine, a concern (Graham, 2020) in the field of vaccinology is the formulation of vaccines that, upon subsequent natural infection, may intensify the severity and worsen the prognosis of the pathology they were designed to protect against; a process known as a vaccine-associated enhanced disease (VAED) (Huisman et al., 2009; Jamrozik et al., 2021a; Munoz et al., 2021). More specifically, VAED is defined in this review as the immune-mediated aggravation of the clinical course of infection following immunization relative to that in the absence of previous vaccination. VAED varies between vaccine platforms and may be caused by distinct mechanisms that are not yet completely understood or have not been entirely explored. Furthermore, to date, no factor has been shown to consistently predict the occurrence of VAED or even differentiate it from severe natural infection. Also, it is likely that no single factor will be able to predict VAED risk, as it might be pathogen-dependent.
In this article, we review the pathophysiological mechanisms that may lead to immune-mediated exacerbation of the disease. Then, we describe the preclinical and clinical cases in which VAED has been observed with vaccine candidates against diverse human and animal pathogens. Furthermore, we address the questions that were initially raised concerning the risk of VAED with anti-SARS-CoV-2 vaccines, as well as the extent to which it should be monitored. Finally, we discuss the use of preclinical and clinical models and how, in addition to vaccine formulation, they can be improved to better address safety in vaccine development.
The purpose of vaccination is to induce a long-lasting immune response and/or immune memory that can be rapidly unleashed during a future encounter with the pathogen being vaccinated against (Pollard and Bijker, 2020). In certain circumstances, the immune responses triggered by exposure to antigens can, individually or through their association, favor exacerbated disease upon subsequent exposure to that same pathogen. Although this process has been described to occur naturally in the case of dengue virus (DENV) after sequential exposition to different serotypes (Halstead, 2007; Guzman et al., 2013), vaccination against most infectious diseases has resulted in only very rare cases of VAED (Huisman et al., 2009; Jamrozik et al., 2021a; Munoz et al., 2021). In the few reported cases, the enhanced disease was due to failed efforts of the immune system to control the infection and subsequently manifested with symptoms related to the organ(s) targeted by the virus. Thus, VAED generally presents as an exacerbation of the pathology seen in natural infection and addressing VAED requires a proper understanding of the course of such an infection and the host-pathogen interactions that take place.
With the exception of the anti-DENV vaccine developed by Sanofi Pasteur and the FI-MV vaccine, which were withdrawn from the market, the occurrence of VAED has always been detected during preclinical and clinical experimental phases (i.e., before licensing) (Jamrozik et al., 2021a). However, continued safety monitoring is carried out even after marketing to ensure the benefits of the vaccines. While identifying VAED is crucial for the development of future safe vaccines, we still lack a clear understanding of the exact associated mechanisms that lead to enhanced pathogenesis.
Various vaccines use antibody titers as correlates of protection, i.e., reaching a specific antibody level following immunization is statistically associated with protection from infection and/or disease (Plotkin, 2010). Neutralizing antibodies attach to the receptor-binding domain (RBD) of proteins at the surface of viruses and prevent binding to the cellular receptor or blocking fusion mechanisms of the viral envelope with target cell membranes (Taylor et al., 2015). Neutralization follows a widely-accepted model, in which it depends on the stoichiometric threshold of neutralization, antibody affinity (and avidity), and, importantly, epitope accessibility (Pierson et al., 2008; Dowd et al., 2011). Both neutralizing and non-neutralizing antibodies can have additional antiviral properties by recruiting other components of the immune system, providing a direct link between innate and adaptive immune responses (Lu et al., 2017; Sedova et al., 2019; Van Erp et al., 2019). These additional effector functions require simultaneous binding to the target antigen on the surface of the pathogen or infected cell via their antigen-binding fragments (Fab region), which forms an immune complex, and the Fc receptor (FcR) on the surface of immune effector cells via their Fc-region (Lu et al., 2017), which triggers a cascade of effector functions. Antibody-dependent cellular cytotoxicity (ADCC) is a type of cell-mediated immunity directed against infected cells opsonized by antibodies, resulting in their lysis, and has been characterized as a potent antiviral response for a number of infections. It generally involves cytotoxic cells, such as NK cells and CD8+ T lymphocytes, and can be enhanced via activation of the complement system. Antibody-dependent cellular phagocytosis (ADCP) provides for the clearance of virus-infected cells by recruiting phagocytes (mainly macrophages and neutrophils) (Tay et al., 2019; Bournazos et al., 2020).
Non-neutralizing, sub-neutralizing, and/or suboptimal concentrations of otherwise potent antibodies can have disproportionate Fc-mediated functions, through receptors for the Fc-region of antibodies (FcRs), which can alter immune responses (Nimmerjahn and Ravetch, 2007). First, they can, instead of blocking viral entry, lead to increased binding to and internalization into immune cells expressing FcRs, such as monocytes, macrophages, and dendritic cells (DCs) (Tirado and Yoon, 2003; Pierson et al., 2008; Dowd et al., 2011; Wang et al., 2014; Diamond and Pierson, 2015; Taylor et al., 2015; Smatti et al., 2018). In most infections, for example with coronaviruses (Tseng et al., 2005; Yilla et al., 2005; Jaume et al., 2011; Wang et al., 2020; Yang J. et al., 2020; Zheng et al., 2021; Junqueira et al., 2022), this is not necessarily indicative of ADE, as multiplication of the pathogen is mostly abortive in these cells, meaning that there is no production or dissemination of infectious particles. In the case of DENV, however, FcR-mediated uptake leads to productive replication during natural infection (Flipse et al., 2016; Wan et al., 2018; Narayan and Tripathi, 2020), a process that has been mimicked with vaccination. During infection with DENV, pre-existing cross-reactive antibodies targeting a serotype of the pathogen other than the infecting one, or even another closely related flavivirus (Katzelnick et al., 2021), can attach with lower affinity to the newly-infecting pathogen. A loss of neutralization capacity can ensue, as well as facilitation of infection of susceptible immune cells (Figure 1A; Halstead and O’Rourke, 1977; Halstead, 1979; Dejnirattisai et al., 2010; Katzelnick et al., 2017). This abnormal process leads to enhanced infection of susceptible cells with the pathogen, enhancing the infected cell mass, which in turn results in both an increased rate of infection and exacerbated pathology (Flipse et al., 2016). Importantly, only a small proportion of antibodies leads to ADE, whereas most contribute to overall protection conferred by ADCP (Bournazos et al., 2020). Furthermore, the accumulation and deposition of immune complexes, which have, for example, been observed in the lungs of children and animal models challenged following immunization with feline immunodeficiency-respiratory syncytial virus and -measles virus (FI-RSV and FI-MV) vaccines (Polack et al., 1999, 2002b,1999), can lead to overabundant Fc-mediated recruitment of immune cells, inflammation, Th2-biased responses and increase antibody production (Anderson and Mosser, 2002), subsequently enhancing the disease (Figure 1B).
Figure 1. Proposed mechanisms leading to vaccine-associated enhancement of disease. (A) Antibody-dependent enhancement (ADE), in which cross-reactive vaccine-elicited antibodies fail to neutralize the dengue virus and instead promote viral replication in FcR-bearing cells. (B) Immune complex accumulation and deposition in the lungs, promote (1) an inflammatory environment, (2) the production of antibodies, and (3) activation of the complement cascade. (C) Skewing of the immune response toward a Th2 phenotype, followed by eosinophil infiltration and accumulation in the lungs. Created with BioRender.com.
Studies have also shown that another mechanism can result in persistent ADE-mediated productive infection of macrophages for prolonged time periods generated by innate immune suppression (Linn et al., 1996; Halstead et al., 2010; Ubol and Halstead, 2010). This phenomenon is called intrinsic ADE. It involves the modulation of innate immune effectors by internalized virus-immune complexes to favor increased replication and release of the virus from infected cells. In the case of ADE mediated by DENV infection, it has been shown that viral entry via FcγR-antibody complexes leads to the inhibition of pro-inflammatory cytokine production and causes a Th2 biased immune response and increased intracellular viral replication (Narayan and Tripathi, 2020).
Immune complex-mediated enhancement in the context of ADE can be further enhanced by complement receptors (CRs) which can expand the number of cells that are permissive to the virus to additional immune and non-immune cells (Takada et al., 2007). If viral replication is initiated in these target cells, the immunopathological response may be further amplified. This has been mostly reported after viral challenge in the case of VAERD for the FI-RSV) (Polack et al., 2002b,2003) and FI-MV vaccines, as well as DENV infection (Jane Cardosa et al., 1983; Taylor et al., 2015). This complement-mediated ADE process has also been demonstrated in vitro for several pathogens (Jane Cardosa et al., 1983; Robinson, 2006).
Finally, cell-mediated mechanisms have also been reported, mainly for FI-RSV and FI-MV vaccines. The preclinical and clinical evaluation suggests the initiation of vigorous CD4 T-cell responses and, in many instances, the skewing of cellular immune responses toward an apparently pathogenic phenotype. Animal models have highlighted a mechanism of Th2-mediated eosinophilic hypersensitivity responses in the lungs (Polack et al., 1999, 2002a; de Swart et al., 2002; Knudson et al., 2015) following vaccination with the FI-RSV and FI-MV vaccines (Figure 1C). Furthermore, lung eosinophilia has been linked to the absence of cytotoxic T-lymphocyte priming during vaccination, both for FI-RSV (Srikiatkhachorn and Braciale, 1997) and SARS-CoV-1 vaccines (Channappanavar et al., 2014). However, the role of eosinophils in the resulting pathogenicity is highly debated, as other studies have shown that distinct CD4+ T-cell responses account for specific parameters of the pathological response (Knudson et al., 2015). In the case of an FIV animal model, a mechanism of lymphocyte activation-mediated enhancement of viral replication has been characterized, in which vaccination leads to enhanced viral replication due to the recruitment of immune cells that simultaneously act as target cells for the virus (Richardson et al., 1997, 2002).
The “original antigenic sin” phenomenon refers to the propensity of the immune system to preferentially use immunological memory built from a previous encounter with an antigen (through infection or vaccination), at the expense of protective primary responses when confronting a slightly different version of the antigen (Fazekas de St Groth and Webster, 1966; Klenerman and Zinkernagel, 1998; Kim et al., 2009). By soliciting immune memory, this process is intended to induce an efficient and rapid response (Veiga-Fernandes et al., 2000), but, instead, skews the immune system toward the first response is made (i.e., the “original antigen”). This is highly detrimental, as it prevents the construction of a potentially more effective immune response targeted against the current immunizing epitopes, which can in turn hamper the control of infection and hence lead to the exacerbation of disease (Fazekas de St Groth and Webster, 1966; Klenerman and Zinkernagel, 1998; Kim et al., 2009). This has been particularly observed in the context of the binding of non-neutralizing antibodies to non-protective epitopes, subsequently preventing the attachment of neutralizing antibodies to protective epitopes, as described for DENV infections with different serotypes (de Alwis et al., 2014; Halstead, 2014). In addition to the risk of ADE due to non-neutralizing cross-reactive antibody responses, Mongkolsapaya et al. showed ineffective cytotoxic T-cell responses that may contribute to severe Dengue disease. The recruitment of T cells with a relatively low affinity for the current infecting virus relative to likely previously-encountered strains was observed in dengue-infected Thai children (Mongkolsapaya et al., 2003), leading to impaired viral clearance and more severe disease. T-cell-mediated recall of primary responses to the detriment of de novo responses to a new antigen has been described for other viruses (Klenerman and Zinkernagel, 1998). Although initial observations were not confirmed in subsequent studies, in the context of coronaviruses, it was suggested that previous exposure to non-pandemic seasonal coronaviruses (HKU1, NL63, OC43, and 229E) that cause common cold-like symptoms or other pandemic coronaviruses (SARS-CoV-1, MERS-CoV) may lead to the exacerbation of COVID-19 upon subsequent exposure to SARS-CoV-2 (Focosi et al., 2021; McNaughton et al., 2022). Extension of this notion led to the early presupposed idea that COVID-19 disease might be more severe in adults than in children due to magnified exposure to coronaviruses throughout their lifespan and associated pre-existing cross-reactive non-neutralizing antibodies (Fierz and Walz, 2020). However, a number of studies suggest that cross-reactive antibodies may have a beneficial role in vaccination against coronaviruses that cause infections in humans (Tan et al., 2021).
Infections with RSV are one of the main causes of acute lower respiratory infections (ALRI) in children and the elderly worldwide, with an estimated 33.1 million cases and 3.2 million hospital admissions of children under five years of age in 2015 (Shi et al., 2017). Despite the urgent need to produce a safe and effective vaccine against RSV (Shaw et al., 2013a), none are currently available on the market. An unfortunate turn of events in the United States in the 1960s (Kim et al., 1969; Smatti et al., 2018) with an FI-RSV vaccine considerably slowed subsequent research in humans. Approximately 80% of the vaccinated children in the trial had to be hospitalized because of VAED, two of whom died, which was significantly more than the 5% in the control group (Kim et al., 1969). Other than abnormal non-protective antibody responses (Murphy et al., 1986), several mechanisms are thought to have contributed to this process, namely complement deposition, the absence of cellular cytotoxic responses, and a bias toward a Th2 cellular response (Delgado and Polack, 2004). Bronchiolar pathology was observed in the hospitalized patients, and histological findings in the lungs highlighted peribronchiolar monocytic infiltration, with excessive levels of eosinophils (Kim et al., 1969). The mechanisms involved in the immunopathology following FI-RSV vaccination have been extensively studied to better understand the root causes associated with such adverse events and in the hope of producing an effective vaccine to meet the medical needs associated with this disease. In a BALB/c mouse model, FI-RSV vaccination followed by RSV challenge was associated with enhanced pulmonary eosinophilia and an increase in the total number of cells (eosinophils, granulocytes, CD4 T cells), mimicking that of children vaccinated with FI-RSV, probably due to an exaggerated Th2 memory response (Waris et al., 1996; Castilow et al., 2007). Interference with Th2 pathways, for example, depletion of IL-4 or IL-13, led to decreased pulmonary eosinophilia and lung pathology (Connors et al., 1994; Johnson and Graham, 1999; Castilow et al., 2007). Conversely, although their potential immunomodulatory role has been suggested by many studies (Hussell et al., 1997; Suzuki et al., 2002; Castilow et al., 2007), CD8+ T-cell numbers were shown to plummet.
The same phenomenon occurred after the licensing of an FI-MV vaccine, causing atypical measles in vaccinated individuals (Fulginiti et al., 1967; Nader et al., 1968; Martin et al., 1979; Smatti et al., 2018), characterized by fever, head, and abdominal pain, rash, and severe pneumonitis. The vaccine was removed from use in 1967, after 1.8 million people, mainly children had been vaccinated (Fulginiti et al., 1967; Martin et al., 1979). The lung histopathology observed with FI-MV was highly similar to that documented following immunization with the FI-RSV vaccine, as well as many immunological and clinical manifestations (Polack, 2007). Rhesus macaques vaccinated with FI-MV developed atypical measles, similar to that described for vaccinated infants, characterized by eosinophil infiltration and immune complex deposition in the lungs (Polack et al., 1999). As for FI-RSV, exposure to MV after FI-MV vaccination led to a Th2-biased immune response, characterized by a rapid reduction in IL-12 production and a subsequent increase in pulmonary eosinophilia and the production of IL-4 (Polack et al., 2002a).
Many studies have highlighted eosinophil infiltration as being the hallmark for VAED in animal models and humans vaccinated with FI-RSV or FI-MV and afterward infected with the target virus, but the subsequent role of eosinophil infiltration in the resulting pathology is yet to be deciphered. Experiments suggest that vaccine-induced pulmonary eosinophilia following vaccination with FI-RSV may have two roles: (1) contributing to pulmonary pathogenesis but also (2) reducing the viral load (Su et al., 2015). While IL-5 and eotaxin were shown to be mediators of leukocyte recruitment, mucus production, and, overall, FI-RSV-induced inflammation, the passive transfer of eosinophils in knockout mice accelerated viral clearance. Further evidence of the dual pathogenic pro-inflammatory and protective anti-viral role of eosinophils was provided by mouse models in response to infection with the pneumonia virus of mice (Percopo et al., 2014). Other studies somewhat exonerated the pathogenic role of eosinophils in VAED by showing that the various parameters of immunopathology associated with vaccine-enhanced RSV disease in mice are mediated by distinct CD4 T cells rather than eosinophils alone (Knudson et al., 2015).
Furthermore, vaccination with both FI-RSV and FI-MV was shown to elicit suboptimal humoral responses relative to natural infection with RSV and MV: less neutralizing antibody was elicited in vaccinated infants and children (Kim et al., 1969; Polack et al., 2002b,2003; Polack, 2007; Acosta et al., 2016) than those of comparable age who were naturally-infected. Before RSV infection, the vaccinated children showed an elevated ratio of binding antibodies versus neutralizing antibodies against the RSV compared to that detected in children who were naturally infected (Murphy et al., 1986). This was speculated to have contributed to the pathogenesis by delaying viral clearance. The immune complex deposition was also observed, probably favored by the presence of large amounts of non-neutralizing antibodies (Polack et al., 2002b,2003). Poor stimulation of T cells and subsequent improper affinity maturation of B cells in germinal centers has been postulated. In addition, in animal models, the complement cascade has been shown to play an important role in VAED following FI-RSV immunization and further RSV challenge (Polack et al., 2002b; Melendi et al., 2007). In younger children, the observed immunopathology may be associated with the immaturity of their immune system characterized by weak stimulation of TLRs (Delgado et al., 2009) and less prominent Th1 responses able to tackle viral infections (Bottazzi et al., 2020). Furthermore, the pathological immune responses leading to atypical measles and VAED following immunization with FI-MV and FI-RSV, respectively, could also be due to formalin-associated disruption of critical epitopes (Murphy et al., 1986; Castilow et al., 2007). Indeed, the generation of carbonyl groups during formalin-mediated inactivation (Castilow et al., 2007) may be a contributing factor, as pulmonary eosinophilia and Th2-associated cytokines were shown to diminish when carbonyl groups were reduced (Moghaddam et al., 2006). However, VAED was not described for the formalin-inactivated vaccines against parainfluenza (Kim et al., 1969), polio (Melnick, 1996), or hepatitis A (Werzberger et al., 1992). Thus, formalin-mediated inactivation cannot be the sole mechanism involved. Lastly, it was also established that, contrary to native RSV, which presents the F glycoprotein in its pre-fusion and post-fusion form, the FI-RSV vaccine primarily presents it in the post-fusion conformation (Killikelly et al., 2016). Hence, a vaccine with the protein stabilized in the pre-fusion state through a limited number of mutations was able to elicit high levels of neutralizing antibodies and protect cotton rats against RSV challenge (Krarup et al., 2015). A number of clinical trials are currently being held to test the immunogenicity, safety, and protective efficiency of various vaccine formulations with this pre-F protein (Beran et al., 2018; DeVincenzo et al., 2019).
Dengue fever is a mosquito-borne disease caused by the dengue virus (DENV), a member of the Flavivirus genus. There are four different serotypes of the virus (DENV 1-4), each able to cause an acute systemic viral disease with a range of severity, going from mild to death by shock. With an estimated 390 million cases a year (Bhatt et al., 2013), subtropical and tropical regions are the most highly affected. Increasing travel and trade, as well as increased urbanization, are gradually increasing the territorial reach of the mosquito and hence the virus (Bhatt et al., 2013; Diamond and Pierson, 2015), resulting in massive public health and economic burden. Dengue has been extensively investigated in the context of ADE. During natural infection, antibodies induced by a first infection are generally highly neutralizing for the specific DENV serotype, thus preventing re-infection with the homologous strain in most cases. However, they appear to have poor avidity and less neutralizing capacity against heterologous DENV strains. Such natural pre-existing immunity is the main risk factor associated with severe diseases, namely dengue hemorrhagic fever (DHF) and dengue shock syndrome (DSS) (Halstead, 2007; Guzman et al., 2013).
In the 1960s, severe disease (DHF/DSS) was reported from clinical and physiological studies to be the result of a second infection by one of the other four types of DENV (reviewed in Halstead and Cohen (2015)). Studies with the rhesus macaque animal model have shown that ADE of DENV infections was observed in the presence of anti-DENV antibodies, whether actively raised (Halstead et al., 1973) or passively acquired (Halstead, 1979). In vitro, monocytes were cultured in the presence of DENV and the addition of anti-DENV antibodies resulted in enhanced infections (Halstead and O’Rourke, 1977). Finally, enhanced viremia during secondary dengue infections in monotypic immune humans was shown to be statistically associated with severe disease (Vaughn et al., 2000).
Studies in tissue culture have demonstrated that cross-reactive antibodies targeting the DENV envelope glycoproteins that either does not neutralize a DENV serotype or do not reach an adequate concentration to effect potent neutralization can lead to increased infection of host target cells via a mechanism based on increased binding and internalization of antibody-coated infectious virions by Fc receptors (Halstead, 2014; Gan et al., 2017).
Although severe disease associated with secondary infection with a heterologous strain is quite infrequent, it becomes a major problem upon primary infection of infants born from DENV-immune mothers whose DENV-specific IgG has crossed the placental barrier (Halstead et al., 2002; Diamond and Pierson, 2015). In this process, cross-reactive antibodies may augment the entry of the virus into cells in an FcγR-mediated fashion (Moi et al., 2011; Taylor et al., 2015), thus increasing viremia and exacerbating the severity of the disease in these children (Halstead, 2007; Diamond and Pierson, 2015). Complement receptors have also been shown to be involved in enhancing viral replication in a larger spectrum of cells through complement-dependent-ADE (Jane Cardosa et al., 1983; Taylor et al., 2015). These immune interactions have been recently extended to the closely related Zika virus (ZIKV). The risk of symptomatic DENV2 infection and severe disease is increased by prior ZIKV infection in children (Katzelnick et al., 2020a,b).
As for natural infection, vaccine-elicited antibodies against a single serotype can elicit cross-reactive antibodies that could become harmful if there is a subsequent infection with another serotype. Given the risks associated with pre-existing cross-reactive antibodies, it appears quite obvious that developing a safe vaccine against dengue involves the induction of protective antibodies against all four serotypes with equivalent efficacy. With this in mind, many strategies to develop effective and safe vaccines against DENV have been initiated (Durbin and Whitehead, 2011). The live-attenuated chimeric yellow fever tetravalent dengue vaccine (CYD-TDV or Dengvaxia) from Sanofi Pasteur was licensed in 2015 and has received endorsement by the WHO for targeted use in DENV-endemic countries. The phase III clinical trial demonstrated moderate efficacy for symptomatic dengue depending on the serotype (pooled efficacy of 60.3% 25 months after the third dose of vaccine across the various clinical trials conducted in endemic areas). The vaccine efficacy against hospitalization due to severe dengue forms (DSS or DHF) was much higher, reaching approximately 80% (Hadinegoro et al., 2015; Villar et al., 2015). However, the vaccine faced a number of issues, with increased rates of hospitalization of vaccinated seronegative children relative to the control group. Although ADE was speculated, it was shown that the sero-status of vaccinated individuals was critical: individuals that had no previous immunity to DENV had higher rates of hospitalization following infection with DENV than seropositive individuals. The WHO thus recommended using the vaccine in seropositive individuals between 9 and 45 years of age, for whom exacerbation of the disease was less observed (Halstead, 2018). In several countries, the vaccine is provided together with a serological test. A more recent re-evaluation of the results of phase III clinical trial (Sridhar et al., 2018) reinforced the hypothesis that, in the absence of previous dengue exposure, the CYD-TDV vaccine partially mimics primary infection. However, the increased risk of severe dengue during subsequent infection is still highly debated (Halstead et al., 2020; Salje et al., 2021). As for natural infection, the vaccine induces cross-reactive but non-neutralizing antibodies (Taylor et al., 2015). Potential mechanisms have been deciphered, in which antibodies may enhance cellular infection by increasing the fusion potential of the virus in macrophages, or, on the contrary, by increasing the binding efficiency of DENV-containing immune complexes in macrophage-like P338DI cells (Flipse et al., 2016; Gan et al., 2017).
According to the WHO, the HIV pandemic has claimed 36.3 million lives since its discovery in the 1980s (Barré-Sinoussi et al., 1983), and 37.7 million people are currently living with HIV (HIV/AIDS, 2021). HIV infection leads to a severe acute infection in which the virus actively replicates in the lymph nodes and blood. Macrophages are thought to act as major reservoirs of HIV infection and facilitate viral dissemination throughout the body (Naif, 2013). HIV infections are accompanied by the massive destruction of CD4+ T cells, followed by a gradual decay in immune system regulation and responsiveness. This subsequently leads to slow progression towards AIDS for the vast majority of HIV-infected individuals (Picker and Watkins, 2005; Naif, 2013). The main receptor for HIV is thought to be the CD4 protein (Douek et al., 2002) (which explains its massive entry into CD4+ T cells) and cell entry usually requires binding to co-receptors, mainly the chemokine receptors CCR5 and/or CXCR4 (Moore et al., 2004; Naif, 2013). Entry into target cells is mediated by the envelope protein, composed of trimers of gp120 and gp41 subunits, involved in receptor binding and membrane fusion, respectively (Wilen et al., 2012).
Despite the existence of efficient anti-retroviral therapy (INSIGHT START Study Group et al., 2015), there are still many obstacles to achieving a cure for HIV with complete remission (Pitman et al., 2018). The HIV pandemic continues to spread and the development of efficient vaccines, as difficult and demanding as it has been up to the present, appears to be crucial. Of note, anti-HIV vaccines have been primarily designed as prophylactic treatments but candidate therapeutic vaccines with the aim of boosting immune responses in infected patients are gaining interest (Pitman et al., 2018). Despite many attempts (Huisman et al., 2009), several obstacles have been encountered that prevents the development of a successful vaccine against what is considered by many to be one of the most challenging pathogens known to date. The observation that vaccine candidates can lead to VAED in certain preclinical and clinical models is one of them.
The earliest report of disease enhancement post-vaccination and challenge with this type of virus dates back to 1992 and involved cats vaccinated against the feline immunodeficiency virus (FIV) with different ISCOM-based vaccines (Hosie et al., 1992). Despite the development of neutralizing antibodies in some groups, the vaccinated cats were not protected from infection, and all became viremic earlier than that in the unvaccinated control, thus highlighting VAED through mechanisms other than the simple lack of the neutralizing ability of antibodies (Hosie et al., 1992). In further studies, the vaccination of cats with various vaccines including the FIV envelope led to accelerated viremia and enhancement of infectivity. Although the enhancement was again transferable to previously-naïve cats by plasma transfer (Siebelink et al., 1995; Osterhaus et al., 1996), the correlation with the presence or absence of neutralizing antibodies has never been clearly established (Richardson et al., 1997)(Karlas et al., 1999; Giannecchini et al., 2002). Of note, other than pre-existing vaccine-mediated humoral responses, it was suggested that an increase in FIV-target cells following immunization could also partially contribute to enhanced cell-mediated pathogenesis due to increased opportunities for viral replication in the early phases of infection (Richardson et al., 2002). This included the expansion of specific CD4+ T cells (Schwartz, 1994), CD134+ cells (Willett et al., 2006), which is the primary receptor for FIV (Shimojima et al., 2004; Reggeti et al., 2008), and CXCR4+ cells (de Parseval et al., 2004).
As hypothesized for a number of anti-FIV vaccines in cats, a mechanism of lymphocyte activation-mediated enhancement of disease has been suggested for anti-simian immunodeficiency virus (SIV) vaccines, in which vaccine-mediated priming of CD4+ T cells against a CD4+ T cell-tropic virus (Douek et al., 2002) led to enhanced viral replication. Rhesus macaques vaccinated with a chimeric DNA vaccine showed only partial control of viral replication after the SIV challenge relative to controls. Vaccinated macaques showed SIV-specific CD4+ T cells, as well as IFNγ-producing CD8+ T cells, in the early phases of infection. However, control over viremia failed to be maintained by a number of animals during the chronic phase of infection, despite the preservation of IFNγ-producing CD8+ T-cell levels, resulting in increased viral loads, the loss of CD4+ T cells, and rapid progression to AIDS (Lun et al., 2004). Thus, although the decline in SIV-specific CD4+ T-cell numbers was not explained, it was speculated that these cells may have had a role in supporting SIV replication (Lun et al., 2004). This has been confirmed in another study with a vaccine candidate which induced non-neutralizing anti-SIV envelope antibodies and weak anti-SIV CD8+ T-cell responses. After the heterologous SIV challenge, vaccinated macaques showed greater and earlier viremia than control macaques, as well as a faster decline in CD4+ T cell number and accelerated AIDS progression (Staprans et al., 2004). In this approach, SIV-specific CD8+ T-cell levels were inadequate, whereas CD4+ T-cell levels increased early after the challenge, subsequently correlating with enhanced SIV replication. SIV-specific CD4+ T cells were shown to be proliferating memory cells that were primed by vaccination and rapidly mobilized upon infection (Staprans et al., 2004).
Over the years, many attempts have been made to develop anti-HIV vaccines, most of which resulted in the induction of broadly neutralizing antibodies (bNAbs) as an overarching goal. Although most candidates were removed from the clinical pipeline due to poor efficacy (Sahay et al., 2017; Pitman et al., 2018), the adenovirus type 5 (Ad5)-vectored vaccine expressing HIV gag/pol/nef proteins, a vaccine aimed to induce cell-mediated immunity, was halted due to the risk of VAED. The vaccine initially showed promising results in preclinical evaluations in NHP models in terms of attenuating the infection in the acute phase, the maintenance and recovery of CD4+ T cells, and the control of viremia during the chronic phase of infection (Shiver et al., 2002). A phase IIb test-of-concept randomized double-blinded clinical trial (STEP) was conducted in high-risk groups in North America, South America, the Caribbean, and Australia to investigate the prevention of HIV acquisition and/or reduction of viral load post-HIV infection. Preliminary results showed no efficacy and higher rates of infection in the vaccinated group (27% of recipients) compared to the control group (3% of recipients) (Cohen, 2007; Buchbinder et al., 2008; McElrath et al., 2008; Moore et al., 2008; Duerr et al., 2012). Although enhanced replication of SIV in NHP models mainly supported a pathogenic role for anti-envelope antibodies (Siebelink et al., 1995; Osterhaus et al., 1996), it is unlikely that this mechanism is involved here, as the env gene was absent from the adenovirus vector construct. Although none were truly validated, several factors, most of them not associated with HIV-specific immune responses, were hypothesized to be associated with this observation (Sahay et al., 2017). One factor was prior humoral immunity to the Ad5 vector. A higher number of infections occurred among recipients that had elevated titers of pre-existing Ad5-specific antibodies, with a 2- to 3-fold higher rate of infections among vaccinated patients than in the placebo group. No differences were observed in terms of increased susceptibility to infection between the two groups among recipients with no pre-existing immunity to Ad5. This dose-effect relationship may indicate, but does not prove, the correlation between prior immunity to the Ad5 virus and susceptibility to HIV infection. In preclinical evaluation, the SIV-Ad5 prototype construct was used with various vaccine candidates and led to the control of viremia, with no evidence of VAED (Shiver et al., 2002; Casimiro et al., 2005; Liang et al., 2005; Wilson et al., 2006). In addition to the circumcision status and sexual behaviors of males involved in the clinical trial (Koblin et al., 2012), both “immune capacity” and host genetics were suggested to have played a role in the outcome of the clinical trial (Moore et al., 2008). Furthermore, the non-specific release of IFNγby CD4+ T cells, rather than HIV-specific responses, was also suspected (Huang et al., 2014).
Although the study outcomes may never be fully deciphered, the STEP study raised fundamental scientific and ethical questions that need to be addressed before moving forward in the quest for an anti-HIV vaccine, and the extent to which vaccination induces cellular immunity could be the answer. The pathological mechanisms that lead to AIDS and host-pathogen interactions (Überla, 2008) continue to be studied to better understand how to defeat what is considered to be one of the most challenging pathogens known to date. Many promising strategies are being evaluated worldwide (Barouch and Korber, 2010; Burton, 2019).
Coronaviruses have been known to infect a wide range of species, with low-pathogenic coronaviruses infecting the upper-respiratory tract, commonly causing seasonal cold-like respiratory diseases in humans. There are other highly pathogenic strains that pose a considerable threat to public health. They infect the lower-respiratory tract and induce a hyper-inflammatory disease, potentially leading to pneumonia and, in the most severe cases, acute lung injury (ALI) and acute respiratory distress syndrome (ARDS) (Channappanavar and Perlman, 2017). The first epidemic was recorded in 2002-2003 with SARS-CoV-1. It started in in the southeast of China before reaching a total of 29 countries (WHO, 2002) and infecting 8,000 people (Peiris et al., 2004). The mortality rate was approximately 10%, reaching up to 50% in the elderly (SARS, 2003). A second epidemic took place in 2012-2013 in the Middle East, where MERS-CoV infected people in a total of 27 countries and caused 858 known deaths (MERS-CoV, 2019). Infection with SARS-CoV-1 is initiated by binding of the receptor binding domain (RBD) of the Spike protein to the angiotensin-converting enzyme 2 (ACE2) receptor on host cells (Du et al., 2009; Li W. et al., 2003; Davidson et al., 2020), followed by viral fusion via the heptad repeat 2 (HR2) domain (Du et al., 2009). Of note, the ACE2 protein is expressed in many tissues (Davidson et al., 2020), including in the immune system, mainly by monocytes and macrophages, making them targets for infection (Nicholls et al., 2003; Boumaza et al., 2021; Junqueira et al., 2022). Following natural infection with SARS-CoV-1, anti-Spike and anti-nucleocapsid (N) antibodies appear to be the dominant humoral response in serum (Chang et al., 2004; Xu and Gao, 2004; Du et al., 2009), along with cellular responses (Xu and Gao, 2004; Du et al., 2009). They are predictive of disease outcome early following infection (Zhang et al., 2006) and persist for a long time (Li et al., 2006). Due to its role in viral attachment and entry, and as one of the main targets of antibodies, the Spike protein is the main target for anti-SARS vaccines and treatments (Du et al., 2009). Although many vaccine candidates, using different technologies, were initiated, safety alerts were reported after the observation of potential VAED both in vitro and in vivo.
In vitro, SARS-CoV-1 has been found to infect and replicate circulating immune cells in the absence of ACE2 (Li L. et al., 2003). In a study using ACE2 and FcRII-bearing HL-CZ human promonocyte cells, anti-Spike antibodies were associated both with efficient neutralization or ADE in a concentration-dependent manner; sera with diluted anti-Spike-antibodies tended to enhance infection, whereas that with concentrated anti-Spike-antibodies promoted protection through neutralization (Wang et al., 2014). Immunization of mice and golden Syrian hamsters with a full-length recombinant trimeric Spike protein (triSpike) elicited strong, efficient, and long-lasting neutralizing immune responses against SARS-CoV-1. However, they were also shown to promote viral entry into human B cells using FcγRII-dependent and ACE2-independent pathways, but not mouse macrophages, despite the presence of these FcRs (Kam et al., 2007). The sera of mice immunized with a recombinant SARS-CoV-1 Spike protein adjuvanted-vaccine showed antibody-dependent infection of human macrophages via the same mechanism (Yip et al., 2014). However, it was demonstrated that human macrophages do not support productive replication. Jaume et al. also showed non-productive infection of SARS-CoV-1 in in vitro models, (Jaume et al., 2011). Indeed, cooperation between phagocytic cells (especially monocyte-derived infiltrating macrophages and alveolar macrophages) and anti-viral antibodies have been found to be essential for the clearance of SARS-CoV-1 in mouse models (Yasui et al., 2014).
The mouse models of SARS-CoV-1 infection have shown that several types of vaccine can induce the exacerbation of eosinophilia and the induction of Th2-mediated inflammatory pulmonary pathology independently of protection (Bolles et al., 2011; Tseng et al., 2012). The formulation of recombinant Spike protein vaccines with an alum adjuvant was associated with decreased immunopathology (Tseng et al., 2012; Chen W. H. et al., 2020). Of note, the protective role of vaccine-induced memory CD8 T cells, both in terms of immunity and preventing immunopathology, was highlighted in the mouse model following vaccination with a recombinant vaccinia virus (Channappanavar et al., 2014). Nevertheless, vaccinating macaques with a recombinant MVA virus encoding full-length SARS-CoV-1 glycoprotein (ADS-MVA) induced infiltration of the lungs by monocytes and macrophages and, overall, enhanced Spike-specific ALI (Liu et al., 2019). Anti-Spike antibodies may promote phenotypic and functional alterations of wound-healing macrophages to become pro-inflammatory, without acting on already inflammatory macrophages, leading to unrestrained inflammation and lung damage (Liu et al., 2019). Importantly, this happened despite the presence of high titers of neutralizing antibodies. FcγR-blockade reduced this process by diminishing the production of pro-inflammatory cytokines (Liu et al., 2019). Increased levels of IL-8 and IL-6 (Liu et al., 2019) also suggest a potential role of Th17-associated cytokines in SARS-CoV-1 vaccine-mediated immunopathology (Hotez et al., 2020). Moreover, Th17 is involved in eosinophil infiltration and activation (Bolles et al., 2011). Furthermore, several studies highlighted decreased pathology when vaccines (subunit or whole-inactivated) were associated with an insulin-based adjuvant (Honda-Okubo et al., 2015). Wang et al. immunized rhesus macaques with various peptide-based vaccines containing B cell-epitopes of the Spike protein (Wang et al., 2016) identified from the plasma of convalescence SARS patients. Immunization with some epitopes successfully protected the animals from infection post-challenge, whereas immunization with others lead to enhanced pathology. This study highlights a convenient strategy that should be considered for the development of safe vaccines, which consists of selecting protective epitopes for immunization and removing those that may induce VAED (Wang et al., 2016). Importantly, the authors produced monoclonal antibodies against these immunodominant epitopes and showed, in vitro in Vero E6 cells, which lack FcRs, that antibodies against “protective” epitopes block viral entry, whereas two antibodies against the “pathogenic” epitopes elicited enhanced infection (Wang et al., 2016). Nonetheless, the histopathological data published by Tseng et al. (2012); Liu et al. (2019), and Wang et al. (2016) have been criticized due to their poor quality, difficulties in interpretation, and the absence of a histopathological scoring system, which may have biased the interpretation (reviewed in Gartlan et al. (2022)). In contrast to previous results, although protective neutralizing immune responses were elicited (Zhou et al., 2005), Luo et al. showed that immunization of rhesus macaques with an inactivated SARS-CoV-1 vaccine led to protection rather than enhanced infection, even in the context of low levels of neutralizing antibodies (Luo et al., 2018). Qin et al. showed similar results with another inactivated vaccine in cynomolgus macaques (Qin et al., 2006).
Concerning MERS-CoV, immunization of mice with a whole-inactivated virus vaccine, either adjuvanted or non-adjuvanted, led to increased infiltration of the lungs by eosinophils, elevated IL-15 and IL-13 levels, and overall lung injury (Agrawal et al., 2016). However, the MERS-CoV challenge of NHPs following vaccination with a DNA vaccine (Wang et al., 2015) or Spike RBD domain subunit vaccine (Lan et al., 2015; Wang et al., 2015) with the RIBI adjuvant did not lead to immunopathology or VAED.
Severe acute respiratory syndrome-coronavirus 2 emerged from Wuhan (China) at the end of 2019 and led to the ongoing COVID-19 pandemic, which was declared by the WHO in March 2020 after having spread to more than 110 countries. As of the end of April 2022, more than 512 million COVID-19 cases had been registered worldwide, with nearly 6.13 million deaths (Worldometer, 2022). The emergence of a number of variants-of-concern after a long period of genetic stability has led to numerous rebounds of the pandemic, both in terms of altered transmission and mortality rates, as well as in terms of sanitary restrictions (Acuti Martellucci et al., 2020; Fontanet et al., 2021). The case fatality rate depends on the period (and hence the variant in question and the sanitary restrictions put in place) and localization, as well as the age and risk factors of individuals. Although the host-pathogen interactions are still being investigated, much remains to be discovered to fully understand the pathogenesis associated with the infection. Two receptors, ACE2 and TMPRSS2, have been shown to enable viral entry into target cells (Davidson et al., 2020; Beyerstedt et al., 2021; Koch et al., 2021). Infection with SARS-CoV-2 can lead to a wide spectrum of clinical manifestations. Most people experience an asymptomatic form of infection, whereas some require hospitalization after developing the severe pulmonary disease (Xu et al., 2020) with acute respiratory distress syndrome (ARDS). Over-activation of the immune system, with predominant local inflammation in the alveolar space (Martines et al., 2020), followed by systemic inflammation and the development of a cytokine storm is thought to be responsible for severe cases (Gao Y. et al., 2020; Huang et al., 2020; Jafarzadeh et al., 2020; Ragab et al., 2020; Wang et al., 2020). Aberrant activation and accumulation of macrophages in tissues are thought to account for the bulk of inflammation (Wang et al., 2020). There may also be a positive feedback circuit between infected macrophages and T cells in the contribution to alveolar inflammation and pneumonia (Grant et al., 2021).
Within a few months, dozens of vaccines entered the preclinical and clinical development pipeline, with many different technologies being evaluated (WHO, 2020), in an unprecedented race against the COVID-19 pandemic. A few were approved for an emergency, limited, and then general use within an extraordinarily short space of time due to accelerated preclinical and clinical evaluation (Graham, 2020; Haynes et al., 2020; Feraoun et al., 2021). Among other factors, an outstanding international coalition, enormous funding from both the private and public sectors, tremendous advances in technology, and previous research on SARS-CoV-1 and MERS-CoV made this incredible achievement possible. As of the end of April 2022, several vaccines against SARS-CoV-2 had been approved, with more than 150 have already reached the clinical phase and another 200 under pre-clinical evaluation (WHO, 2020; Covid-19 Vaccine, 2022). These vaccine candidates and approved vaccines are based on different platforms and formulations, but most target the Spike protein (WHO, 2020; Covid-19 Vaccine, 2022).
There were initial concerns about the possibility that the new SARS-Cov-2 vaccines may carry a risk of VAERD (Arvin et al., 2020; Halstead, 2021; Jamrozik et al., 2021a; Munoz et al., 2021; Gartlan et al., 2022), but two years of epidemiological data in clinical trials and widespread administration of these vaccines in the general population have made it possible to alleviate them. These concerns were based on several observations, a number of which are mentioned below:
A) The genetic proximity between SARS-CoV-2 and the other pandemic coronaviruses (SARS-CoV-1, MERS-CoV) for which VAED has been described.
B) As for vaccines against other pandemic coronaviruses, the production of anti-Spike antibodies, which was partially associated with VAED in a few studies, is also the main aim of many vaccines against SARS-CoV-2.
C) Early suspicions arose that the induction of high antibody titers the following infection with SARS-CoV-1 and SARS-CoV-2 may be associated with a worse clinical outcome (Hsueh et al., 2003; Zhao et al., 2020).
D) The lack of cross-neutralization of antibodies in serum from patients infected with SARS-CoV-1 against SARS-CoV-2 (Anderson et al., 2020). However, Tan et al. demonstrated that immunization of SARS survivors with the BNT162b2 mRNA vaccine (Pfizer – BioNTech vaccine (Polack et al., 2020)) induced a broad spectrum of pan-sarbecovirus neutralizing antibodies in humans (Tan et al., 2021). The ability to generate such cross-neutralizing antibodies with vaccines was already shown in macaques (Saunders et al., 2021) and mice (Martinez et al., 2021).
E) Antibodies that enhance infection in cellular models in vitro have been isolated from SARS-CoV-1 and SARS-CoV-2 patients, although these did not cause immunopathology in vivo in NHP and mouse models (Li et al., 2021) and did not lead to aberrant cytokine production (Maemura et al., 2021).
F) Phagocytic cells, such as monocytes and monocyte-derived macrophages (MDMs), are susceptible to SARS-CoV-2 infection, both in vitro and in vivo, through antibody-mediated pathways (Wan et al., 2020). These immune cells were indeed shown to be permissive to infection, but not to viral replication, meaning that the replication, production, and dissemination of infectious particles do not take place (Wang et al., 2020; Yang D. et al., 2020; Zheng et al., 2021; Junqueira et al., 2022). However, although abortive, the infection of monocytes and macrophages causes aberrant cytokine production (the “cytokine storm”) and massive lung and subsequent systemic inflammation, which may contribute to the overall pathogenesis and severity of COVID-19 (Wang et al., 2020; Yang D. et al., 2020; Zheng et al., 2021; Junqueira et al., 2022). Indeed, in a humanized-mouse model, SARS-CoV-2-infected macrophages are involved in the inflammatory pathogenesis of COVID-19 through the activation of inflammasomes and the induction of pyroptosis (Sefik et al., 2022b). Other groups have suggested that although productive replication in these cells does not take place, infectivity is preserved and they can disseminate throughout the body and act as “trojan horses” by enabling the virus to invade surrounding replication-permissive cells (Percivalle et al., 2021). Such transmission was shown to be hindered by human neutralizing antibodies and anti-Spike monoclonal antibodies (Percivalle et al., 2021). Although these observations were all made in the context of antibodies generated through natural infection, Junqueira et al. suggested that virus uptake and, hence, disease severity, did not increase in the presence of plasma from mRNA-vaccinated recipients (Junqueira et al., 2022).
There is a substantial body of preclinical and clinical data that highlights the benefits of anti-SARS-CoV-2 vaccination relative to the risk of enhanced disease. Indeed, many anti-SARS-CoV-2 candidate vaccines have been tested in NHP models and, contrary to what was observed for SARS-CoV-1 and MERS-CoV, no evidence of VAED has been observed. Protection generally correlates with high titers of species-specific neutralizing antibodies. Overall, no lung-associated immunopathology or signs of VAERD have been described after the challenge of various animal models with SARS-CoV-2 subsequent to vaccination with an mRNA vaccine (Corbett et al., 2020), recombinant adenovirus vaccine (Chen Y. et al., 2020), recombinant Spike protein vaccine (Yang J. et al., 2020), two-component Spike nanoparticle vaccine (Brouwer et al., 2021), adjuvanted-subunit vaccine (Tian et al., 2021), subunit vaccine targeting CD40-expressing APCs (Marlin, 2021), or DNA vaccine (Yu et al., 2020). Safety in animal models has also been reported with different inactivated vaccines. The PiCoVacc inactivated vaccine-induced neutralizing antibodies in mice, rats, and rhesus macaque models and overall protective immunity against SARS-CoV-2 challenge in rhesus macaques (Gao Q. et al., 2020). No ADE was observed, even in the animals that received a medium dose of vaccine and produced only limited titers of neutralizing antibodies (Gao Q. et al., 2020). Bewley et al. challenged ferrets and NHPs with SARS-CoV-2 following vaccination with a formalin-inactivated vaccine (Bewley et al., 2021). Among ferrets, two animals showed a transient slight enhanced-disease by day 7, characterized by bronchiolitis, eosinophilic infiltrate, and perivascular cuffing. This phenomenon was not observed in macaques, in which vaccinated animals were protected from the lung pathology associated with SARS-CoV-2 infection relative to control non-vaccinated groups (Bewley et al., 2021). Yoshikawa et al. have developed a lethal model using BALB/c mice that can be used to provide new insights on the potential occurrence of VAED following mouse-passaged SARS-CoV-2 infection (Iwata-Yoshikawa et al., 2022). This model could be a practical way for the preclinical evaluation of vaccines. The authors obtained Th1- or Th2-shifted immune responses with adjuvant-driven vaccines. Similar to mouse models of SARS-CoV-1 and MERS-CoV, they showed that an imbalance in Th1 and Th2 responses (Th1 < Th2) contributes to transient immunopathology mediated by eosinophil infiltrates in the pulmonary tract after challenge with mouse-adapted SARS-CoV-2. However, the level of neutralizing antibodies had no impact on the severity of the disease.
Another global concern is the emergence and spread of variants and the cross-protection conferred by the anti-SARS-CoV-2 vaccines, which target the initial/prototypic “Wuhan strain” (Hacisuleyman et al., 2021; Naaber et al., 2021; Ricke, 2021; Thomas et al., 2021; Covid World Vaccination Tracker, 2022). NHPs were vaccinated with three doses of SARS-CoV-2 inactivated vaccine and then challenged with different variants (prototypic SARS-CoV-2, Alpha (B.1.1.7), Beta (B.1.351), Delta (B.1.617.2), and Omicron (B.1.1.592 variant) (Krause et al., 2021). The vaccinated animals experienced a reduction in viral shedding and replication, as well as improved pathology in the lungs, relative to unvaccinated control animals inoculated with the SARS-CoV-2 variants. Importantly, they developed neutralizing antibodies against the variants, hence demonstrating cross-protection and the absence of VAED. Limitations of this study included the lack of statistical analysis due to the small number of animals in each group. The fact that the antibodies elicited by vaccination against the initial Wuhan strain are cross-protective against SARS-CoV-2 variants and are not associated with a risk of VEAD has also been demonstrated in the clinic (Arora et al., 2021; Cele et al., 2021; Karim, 2021; Reynolds et al., 2022; Xie et al., 2022).
Hence, contrary to what was hypothesized in the months following the marketing of SARS-Cov-2 vaccines (Mileto et al., 2021), there is today overwhelming evidence to reasonably disregard any potential risk of VAED associated with anti-SARS-CoV-2 vaccine-elicited immune responses. Millions of doses of vaccines, based on different immunization strategies, have been administered in the past months (Wolz et al., 2022) without reports of VAED. Finally, no epidemiological or clinical evidence has been documented that suggests that the waning of vaccine-induced antibodies, which has been observed in the months following vaccination (Groß et al., 2022; Nemet et al., 2022), might promote enhanced-pathology.
Although occurring rarely, VAED is still a threat to vaccine development, and, by extension, to public health as a whole. Hence, there is an undeniable necessity to further assess the safety of future vaccine candidates, the extent to which they might lead to VAED, and how this can be minimized. However, scientists are faced with evident technical difficulties and knowledge gaps in this field, which highlights the continued efforts that are required to ensure the harmlessness of future vaccines.
One of the main challenges is that, despite the knowledge that VAED has occurred in certain circumstances, no biomarkers, immunological findings, or clinical manifestations have been shown to separate VAED from severe infection. In addition, the rarity of VAED, the time between vaccination and the potential occurrence of VAED, as well as the lack of a common causative mechanism, considerably impede the prediction, surveillance, and monitoring of VAED. Given the urgent need to rapidly develop safe anti-SARS-CoV-2 vaccines and the lack of a definition for VAED and the availability of specific guidelines on how it can be assessed during preclinical and clinical trials, a Brighton Collaboration VAED group was formed in March 2020. During this consensus conference, various experts in the biomedical and public health fields were brought together by the Coalition for Epidemic Preparedness Innovations (CEPI) to discuss, among other things, current knowledge concerning the assessment of VAED in the accelerated development of anti-COVID-19 vaccines (Lambert et al., 2020; Munoz et al., 2021). A definition for VAED was established, as well as defined levels of certainty for VAED evaluation (Munoz et al., 2021).
To reduce the knowledge gap and better characterize VAED, efforts must be primarily made to better understand the immune mechanisms associated with vaccine-mediated protection and pathology. For example, the yellow fever vaccine is considered to be one of the safest and most-effective vaccines ever developed, but despite having been used for decades, the mechanisms involved in its success are not known and studies are now accumulating to decipher them (Querec et al., 2009; Wieten et al., 2016; Staples et al., 2020). In the same way that a growing number of scientists are focusing on describing immune signatures and early biomarkers associated with protective immunity following vaccination (Van Tilbeurgh et al., 2021), those potentially associated with pathogenic immune responses need to also be brought to light. A Vaccines and Related Biological Products Advisory Committee (VRBPAC) meeting took place in 2017 to discuss the immunopathogenesis of VAED and the preclinical and clinical considerations and data required to support the development of vaccines intended for RSV-naïve children (Browne et al., 2020). It was established that certain interdependent responses might be predictive of VAED or at least lead to the suspicion of VAERD; namely, dominant Th2 immune responses, lung eosinophilia, high titers of low-avidity/non-neutralizing antibodies, and the absence of specific CD8+ T cells (Browne et al., 2020). Of note, the findings concerning VAED are pathogen-specific, and even epitope-specific, meaning that although the mechanisms may be somewhat similar, current knowledge does not allow extrapolation to other vaccines.
Investigation of the possibility of VAED is crucial during clinical trials for decision-making on vaccine licensing by regulatory agencies. To minimize risks for the participants, compromises have to be made between the scientific interests of the trial and the safety of the participants (Jamrozik et al., 2021a). For example, Phase III clinical trials that test for efficacy should involve a rigorous selection of participants in areas with a higher probability of encountering the virus to reduce the required time and hence the cost of the trial, but for whom the outcome of potential VAED would not be as severe. In the case of anti-RSV vaccines, it was established that clinical studies must first be conducted in a healthy adult population and RSV-experienced children so that safety is demonstrated before being tested on RSV-naïve children (Browne et al., 2020). In the majority of cases, the risk of VAED is brought to light during preclinical stages or early clinical trial phases. Nonetheless, VAED is quite infrequent and generally occurs a long time post-immunization, as it manifests when (and only if) vaccine recipients are exposed to the pathogen and infected subsequent to vaccine administration. In this sense, VAED can sometimes only be detected in the later phases of clinical trials, as was the case for the HIV-Ad5-vectored vaccine candidate (Cohen, 2007; Buchbinder et al., 2008; McElrath et al., 2008; Moore et al., 2008; Duerr et al., 2012), or even post-license, as was the case for the Dengvaxia vaccine (Hadinegoro et al., 2015; Villar et al., 2015; Sridhar et al., 2018) and the FI-MV vaccine (Fulginiti et al., 1967; Nader et al., 1968; Martin et al., 1979; Smatti et al., 2018). Hence, vigorous surveillance of VAED is necessary for the pharmacovigilance phases post-license.
One solution to reduce the time of clinical trials could be the implementation of human-challenge trials (also called controlled human infection model (CHIM) studies), which involve the intentional infection of healthy and consenting individuals in a monitored environment. CHIM studies have received increased consideration in biomedical research, given the invaluable insight they can provide in terms of host-pathogen interactions, immune correlates of protection, accelerated vaccine development, etc. (Sekhar and Kang, 2020), even more so during the COVID-19 sanitary crisis. Despite the WHO guidance (WHO, 2017), and the strict ethical frame and thorough supervision, the deliberate exposition of humans to an infectious disease poses many ethical concerns, and is highly criticized in many countries. Despite much debate due to obvious ethical issues (Baay and Neels, 2020; Edwards and Neuzil, 2022), the idea of implementing CHIM studies in the context of the ongoing COVID-19 pandemic was considered (Baay and Neels, 2020; Deming et al., 2020; Jamrozik et al., 2021b; Edwards and Neuzil, 2022) to respond to the emergency of developing safe and effective vaccines. The first trial was conducted on young adults in the UK early in February 2021 (Oxford Vaccine Group, 2020), the first results having been published recently (Killingley et al., 2022). Young and healthy adults previously immunized by natural infection or vaccination were infected with SARS-CoV-2 in a controlled manner, with no safety issues having been declared. In the context of VAED, the trial would need to be expanded to the investigation of the impact of vaccination prior to SARS-CoV-2-challenge. The main limitations are mainly the small number of participants and the fact that participants are carefully screened to ensure that they are perfectly healthy and do not have any risk factors for the disease. Indeed, due to their rareness, VAED events may not be detectable, if they exist, or if the participants are limited in numbers. In addition, the observations made on these participants may not be extrapolatable to the general population, which is much more heterogeneous and includes people with specific attributes that could influence disease severity independently of vaccination. In addition, CHIM studies to evaluate the risks of VAED may require enrolling SARS-CoV-2 naïve participants to study the effect of prior immunity to pandemic coronaviruses on VAED occurrence, as this has been an issue in the past with DENV serotypes (Halstead, 2018).
According to the definition established by the Brighton Collaboration (Munoz et al., 2021), the possibility of VAED includes an “increased frequency of severe outcomes (including severe disease, hospitalization, and mortality) when compared to a non-vaccinated population (control group or background rates)”. Notably, the large-scale clinical experience with a variety of SARS-CoV-2 vaccines in humans, both before and after licensing, has provided no evidence of breakthrough enhanced disease (AAAS, 2021) that could justify delaying or interrupting SARS-CoV-2 vaccine accessibility and administration. On the contrary, it has highlighted the beneficial role of vaccinations (Suthar et al., 2022). Naturally, in the context of VAED and given the proximity between the viruses, this observation frames an important research opportunity to understand why laboratory animals are given SARS-CoV-1 and MERS-CoV vaccines experienced more-or-less abnormal responses to a wild virus challenge, whereas the current generation of SARS-CoV-2 vaccines has not sensitized humans nor animal models.
For ethical and safety reasons, only limited studies can be conducted on humans, with a very strict framework and legislation (European Commission, 2014; FDA, 2015). Animal models are imperfect but highly useful tools to bridge in vitro proof-of-concept studies and human clinical trials. Although preclinical testing is not a prerequisite for clinical trials, it can unquestionably provide valuable information on the safety and immunogenicity of many vaccine candidates (Kiros et al., 2012), when appropriately designed and conducted. Animal models often provide key information to decide whether to pursue vaccine development or not, as has been the case for vaccines against numerous infectious agents, including anti-SARS-CoV-2 vaccines (Chen Y. et al., 2020; Corbett et al., 2020; Yang J. et al., 2020; Yu et al., 2020; Brouwer et al., 2021; Marlin, 2021; Tian et al., 2021; Gao Q. et al., 2020; Bewley et al., 2021; Krause et al., 2021). Such studies can be systematically initiated to focus on early events or biomarkers that may indicate the risk of VAED, or at least provide mechanistic insights on the associated pathology. Another major advantage of preclinical models is the possibility of conducting extensive longitudinal studies and the availability of a greater number of samples, in terms of both sampling frequency and sample diversity. Nonetheless, it is crucial to keep in mind that, due to physiological differences between animals and humans, studies in animal models are used to enlighten scientists on certain mechanisms but are not able to replace studies in humans (Cohen, 2006). Consequently, signs can occur in preclinical studies that raise doubts on safety parameters but that will not be observed in humans and, hence, do not predict VAED. Conversely, a vaccine without any safety concerns in animal models can still induce VAED in humans. By extension, potential mechanisms or biomarkers may be identified but they are generally not sufficiently understood to be efficiently translated into the design of the vaccine.
For optimal results, animal models need to be adequately fit-for-purpose. Indeed, each animal model comes with physiological and technical advantages and disadvantages (Kiros et al., 2012), and the most relevant for a specific question needs to be used. In addition, differences in immune responses between species need to be taken into consideration. For example, antibodies and complements can have very different properties depending on the model and, as they are the main actors in VAED, may falsely influence protective/deleterious observations made concerning VAED. On the one hand, mice are the most used: they are less expensive, easier to handle and they have well-characterized immune responses and defined genetic background. On the other hand, despite ethical and practical difficulties, larger animals are more biologically and immunologically relevant models to study human infections and vaccine immune responses (Kiros et al., 2012), NHPs being the most compatible models (Estes et al., 2018). Indeed, NHPs reproduce human disease after infection with most human pathogens or closely-related ones, and they have similar immune responses. This is crucial to be able to evaluate the efficacy of a vaccine, but also to investigate risks for VAED since detection and monitoring of its occurrence appears challenging if the model does not display symptoms. NHP models are at the forefront of efforts to study HIV transmission and prevention, as well as SARS-CoV-2 (Chen Y. et al., 2020; Yu et al., 2020; Brouwer et al., 2021; Marlin, 2021; Tian et al., 2021) and many other infectious diseases (reviewed in Estes et al. (2018)).
When modeling on NHPs is not an option, scientists have turned to the use of more sophisticated models to reduce the lack of translatability from animal to human, examples of which include surrogate models. These are “artificial models” which can be infected with pathogens of interest only under experimental conditions (Kiros et al., 2012). The use of transgenic and knockout mouse models is highly relevant to studying the role of a particular individual gene in specific immune responses. The design of single and double knockout mice has made it possible to understand the role of IL-5 and eotaxin in the recruitment of eosinophils, which appears to be a hallmark of VAED of FI-RSV and FI-MV vaccines (Su et al., 2015). “Humanized mouse models”, in which specific human cells or tissues are engrafted (Allen et al., 2019), have also received great interest to mimic, as closely as technically-possible, the clinical experience and enhance translatability to human biomedical research (Wiles et al., 2006; Denayer et al., 2014; Fujiwara, 2018). Mouse models with human immune systems have been developed to gain insights into the function of the immune system in health and disease, host-pathogen interactions, prophylactic and therapeutic treatments against infectious diseases, including SARS-CoV-2 (Dinnon et al., 2020; Charles River, 2021; Sefik et al., 2021, 2022a,2022b), and many other research areas (Allen et al., 2019). Challenges of such models include the complexity of set-up, the risk of graft-versus-host disease, incomplete immune functions, etc., as well as the lack of adaptability of the models, which are designed for a specific research purpose and, hence, cannot support a broad array of research (Allen et al., 2019). Literature on the use of humanized mouse models in the context of VAED is not extensive. Sefik et al. used the MISTRG6-hACE2 humanized mouse model to show that SARS-CoV-2-infected macrophages contribute to the inflammatory pathogenesis of COVID-19 through the activation of inflammasomes and the induction of pyroptosis (Sefik et al., 2022b).
Despite evident and substantial similarities between a number of animal species, mainly mammals and humans, there are also physiological and genetic differences that can prevent the translation of results obtained during in vivo investigations to clinical application (Feinberg and Moore, 2002; Sabroe et al., 2007; Barré-Sinoussi and Montagutelli, 2015). One way to take advantage of animal models to better address scientific questions is to design studies that take into consideration both targeted subpopulations with specific attributes and the epidemiological context. Indeed, clinical trials for vaccine development are often conducted on healthy adults and rarely include populations at risks, such as infants, pregnant women, or the elderly. Targeted studies on these populations can be performed in preclinical studies. The occurrence of VAED has mostly been detected in the younger population, as the target population of most vaccines is children. Second, in the case of VAED, the possibility of setting up reinfection models could be informative, mainly in the case of DENV, for which the observed pathology could be due to repeated exposure to the antigen, both through infection and vaccination.
Lastly, closing the gap between preclinical and clinical data should involve making preclinical setups as close to that in clinical trials as possible. Standardizing technical and biological parameters between preclinical and clinical trials, by using similar scoring systems and endpoints, for instance, could be the first step. Additionally, using the same tools and techniques to measure immune and biological parameters is necessary. The reagents and other preparations used for clinical studies fall under Good Manufacturing Practices (GMP)-standards, whereas, for cost reasons, this is not necessary for preclinical studies, which could be a source of the issue. For example, Shaw et al. used a cotton rat model of VAERD following vaccination with the FI-RSV vaccine (Shaw et al., 2013b) to show that the presence of non-viral cell-culture-derived contaminants such as bovine serum albumin drives a bulk of the inflammation following challenge with RSV, while it is not the case following natural infection of children (Shaw et al., 2013b).
The observations made in vitro and in vivo during the preclinical evaluation of vaccines are used to inform decisions that will not only involve a lot of money but also affect thousands of volunteers in subsequent clinical trials. Therefore, despite being quite limited in terms of predictive power and requiring constant improvements to be more reliable and informative, animal models as they are appreciated today, are still essential in biomedical research. Concerning VAED, they are valuable in assessing the risks carried by vaccine candidates by providing information on pathological mechanisms that need to be monitored during subsequent clinical studies in humans. Although they may not be able to dissect potential pathological mechanisms per se, they might at least provide early signs to be taken into consideration before evaluation in humans.
Lastly, vaccine formulation strategies may be a way forward to minimize the risk of VAED. The design (Shukla et al., 2020), type, dose, formulation, site of administration (Rosenbaum et al., 2021), and administration schedule (Palgen et al., 2021) of a vaccine have a considerable impact on the vaccine-induced immune response and subsequent protective immunity (Zimmermann and Curtis, 2019). Hence, modulating these parameters may result in altered immune responses, some of which might be less prone to causing VAED. Clearly, with the many observations brought to light by past VAED experiences, avoiding vaccine strategies that generate non-neutralizing or suboptimal concentrations of neutralizing antibodies could help prevent the occurrence of VAED, as well as favor Th1-type over Th2-type responses. Consistent with these objectives, many safe vaccines that we know today have been formulated with adjuvants, which play a role in enhancing the magnitude and durability of immune responses to vaccines through the activation of innate immunity (Awate et al., 2013). The importance of early innate immune responses in setting the scene for ensuing adaptive responses is now well known (Iwasaki and Medzhitov, 2010). Thus, the proper use of adjuvants could be an option to condition and skew vaccine-specific humoral and cellular immune responses toward those that are functional, safe, and efficient (O’Hagan et al., 2017). Finally, from a theoretical point of view, the risk of VAED is greater for whole-inactivated vaccines, which aim to elicit a humoral response. Indeed, they expose the immune system to epitopes that might constitute non-neutralizing targets or be presented in non-neutralizing conformations, thus inducing suboptimal immune responses that could override those that are protective. Due to their intrinsic mechanisms, live-attenuated vaccines, vectored-vaccines, and nucleic-acid and subunit vaccines carry less risk of such immunopathology. Live-attenuated vaccines mimic natural infections and, to date, have not been shown to induce VAED (Graham, 2011; Browne et al., 2020). However, these observations are still theoretical, as in practice, VAED is not restricted to inactivated vaccines and many inactivated vaccines that have been commercialized have not caused any safety issues in terms of VAED.
Overall, predicting VAED is highly challenging in practice, certainly due to the fact that the tools that we have at our disposal are not yet sufficient and/or well-enough understood to perfectly mimic the vaccine-elicited immune responses that occur in humans. This subsequently restricts our ability to prevent their occurrence in future vaccine designs. Nonetheless, we have highlighted various research areas, in terms of model translatability and vaccine design and formulation, which can be improved to better understand VAED and overcome these limitations. Furthermore, in addition to finding ways to better predict and detect VAED in future vaccines, transparency and communication concerning VAED need to be improved by health policies to encourage public trust in vaccines.
JB: analysis and writing with the guidance of RL, PM, and FM. JB, RL, PM, and FM: conceptualization and reviewing, and editing. All authors contributed to the article and approved the submitted version.
This work was supported by the IDMIT infrastructure and funded by the ANR via grant No: ANR-11-INBS-0008.
We would like to express our gratitude to Caroline Manet for her very useful comments, mainly in the discussion section.
The authors declare that the research was conducted in the absence of any commercial or financial relationships that could be construed as a potential conflict of interest.
All claims expressed in this article are solely those of the authors and do not necessarily represent those of their affiliated organizations, or those of the publisher, the editors and the reviewers. Any product that may be evaluated in this article, or claim that may be made by its manufacturer, is not guaranteed or endorsed by the publisher.
ACE2, angiotensin-converting enzyme 2; ADE, antibodydependent enhancement; Ad5, adenovirus type 5; AIDS, acute immunodeficiency syndrome; ALI, acute lung injury; COVID-19, coronavirus-induced disease 2019; CR, complement receptor; DENV, Dengue Virus; DHF, dengue hemorrhagic fever; DSS, dengue shock syndrome; FcR, Fc receptor; FI, formalin-inactivated; FIV, feline immunodeficiency virus; HIV-1, human immunodeficiency virus 1; MERS-CoV, Middle-Eastern respiratory syndrome coronavirus; MV, measles virus; NHP, non-human primate; RSV, respiratory syncytial virus; SARS-CoV, severe acute respiratory syndrome coronavirus 1; SARSCoV-2, severe acute respiratory syndrome coronavirus 2; SIV, simian immunodeficiency virus; VAED, vaccine-associated enhanced disease.
Acosta, P. L., Caballero, M. T., and Polack, F. P. (2016). Brief history and characterization of enhanced respiratory syncytial virus disease. Clin. Vaccine Immunol. 23, 189–195. doi: 10.1128/CVI.00609-15
Acuti Martellucci, C., Flacco, M. E., Cappadona, R., Bravi, F., Mantovani, L., and Manzoli, L. (2020). SARS-CoV-2 pandemic: an overview. Adv. Biol. Regul. 77:100736. doi: 10.1016/j.jbior.2020.100736
Agrawal, A. S., Tao, X., Algaissi, A., Garron, T., Narayanan, K., Peng, B.-H., et al. (2016). Immunization with inactivated Middle East Respiratory Syndrome coronavirus vaccine leads to lung immunopathology on challenge with live virus. Hum. Vaccine Immunother. 12, 2351–2356. doi: 10.1080/21645515.2016.1177688
Allen, T. M., Brehm, M. A., Bridges, S., Ferguson, S., Kumar, P., Mirochnitchenko, O., et al. (2019). Humanized immune system mouse models: progress, challenges and opportunities. Nat. Immunol. 20, 770–774. doi: 10.1038/s41590-019-0416-z
Anderson, C. F., and Mosser, D. M. (2002). Cutting edge: biasing immune responses by directing antigen to macrophage Fc gamma receptors. J. Immunol. 168, 3697–3701. doi: 10.4049/jimmunol.168.8.3697
Anderson, D. E., Tan, C. W., Chia, W. N., Young, B. E., Linster, M., Low, J. G. H., et al. (2020). Lack of cross-neutralization by SARS patient sera towards SARS-CoV-2. Emerg. Microbes Infect. 9, 900–902. doi: 10.1080/22221751.2020.1761267
Arora, P., Rocha, C., Kempf, A., Nehlmeier, I., Graichen, L., Winkler, M. S., et al. (2021). The spike protein of SARS-CoV-2 variant A.30 is heavily mutated and evades vaccine-induced antibodies with high efficiency. Cell Mol. Immunol. 18, 2673–2675. doi: 10.1038/s41423-021-00779-5
Arvin, A. M., Fink, K., Schmid, M. A., Cathcart, A., Spreafico, R., Havenar-Daughton, C., et al. (2020). A perspective on potential antibody-dependent enhancement of SARS-CoV-2. Nature 584, 353–363. doi: 10.1038/s41586-020-2538-8
Awate, S., Babiuk, L. A., and Mutwiri, G. (2013). Mechanisms of action of adjuvants. Front Immunol. 4:114. doi: 10.3389/fimmu.2013.00114
Baay, M., and Neels, P. (2020). SARS-CoV-2 controlled human infection models: ethics, challenge agent production and regulatory issues. Biologicals 67, 69–74. doi: 10.1016/j.biologicals.2020.08.006
Barouch, D. H., and Korber, B. (2010). HIV-1 Vaccine Development After STEP. Annu. Rev. Med. 61:153. doi: 10.1146/annurev.med.042508.093728
Barré-Sinoussi, F., Chermann, J. C., Rey, F., Nugeyre, M. T., Chamaret, S., Gruest, J., et al. (1983). Isolation of a T-lymphotropic retrovirus from a patient at risk for acquired immune deficiency syndrome (AIDS). Science 220, 868–871. doi: 10.1126/science.6189183
Barré-Sinoussi, F., and Montagutelli, X. (2015). Animal models are essential to biological research: issues and perspectives. Future Sci. OA 1:FSO63.
Beran, J., Lickliter, J. D., Schwarz, T. F., Johnson, C., Chu, L., Domachowske, J. B., et al. (2018). Safety and immunogenicity of 3 formulations of an investigational respiratory syncytial virus vaccine in nonpregnant women: results from 2 Phase 2 Trials. J. Infect. Dis. 217, 1616–1625. doi: 10.1093/infdis/jiy065
Bewley, K. R., Gooch, K., Thomas, K. M., Longet, S., Wiblin, N., Hunter, L., et al. (2021). Immunological and pathological outcomes of SARS-CoV-2 challenge following formalin-inactivated vaccine in ferrets and rhesus macaques. Sci. Adv. 7:eabg7996.
Beyerstedt, S., Casaro, E. B., and Rangel, ÉB. (2021). COVID-19: angiotensin-converting enzyme 2 (ACE2) expression and tissue susceptibility to SARS-CoV-2 infection. Eur. J. Clin. Microbiol. Infect. Dis. 40, 905–919. doi: 10.1007/s10096-020-04138-6
Bhatt, S., Gething, P. W., Brady, O. J., Messina, J. P., Farlow, A. W., Moyes, C. L., et al. (2013). The global distribution and burden of dengue. Nature 496, 504–507. doi: 10.1038/nature12060
Bolles, M., Deming, D., Long, K., Agnihothram, S., Whitmore, A., Ferris, M., et al. (2011). A Double-inactivated severe acute respiratory syndrome coronavirus vaccine provides incomplete protection in mice and induces increased eosinophilic proinflammatory pulmonary response upon challenge. J. Virol. 85, 12201–12215. doi: 10.1128/JVI.06048-11
Bottazzi, M. E., Strych, U., Hotez, P. J., and Corry, D. B. (2020). Coronavirus vaccine-associated lung immunopathology-what is the significance? Microbes Infect. 22, 403–404.
Boumaza, A., Gay, L., Mezouar, S., Bestion, E., Diallo, A. B., Michel, M., et al. (2021). Monocytes and macrophages, targets of severe acute respiratory syndrome coronavirus 2: the clue for coronavirus disease 2019 Immunoparalysis. J. Infect. Dis. 224, 395–406. doi: 10.1093/infdis/jiab044
Bournazos, S., Gupta, A., and Ravetch, J. V. (2020). The role of IgG Fc receptors in antibody-dependent enhancement. Nat. Rev. Immunol. 20, 633–643. doi: 10.1038/s41577-020-00410-0
Brouwer, P. J. M., Brinkkemper, M., Maisonnasse, P., Dereuddre-Bosquet, N., Grobben, M., Claireaux, M., et al. (2021). Two-component spike nanoparticle vaccine protects macaques from SARS-CoV-2 infection. Cell 184, 1188–1200.e19. doi: 10.1016/j.cell.2021.01.035
Browne, S. K., Beeler, J. A., and Roberts, J. N. (2020). Summary of the vaccines and related biological products advisory committee meeting held to consider evaluation of vaccine candidates for the prevention of respiratory syncytial virus disease in RSV-naïve infants. Vaccine. 38, 101–106. doi: 10.1016/j.vaccine.2019.10.048
Buchbinder, S. P., Mehrotra, D. V., Duerr, A., Fitzgerald, D. W., Mogg, R., Li, D., et al. (2008). Efficacy assessment of a cell-mediated immunity HIV-1 vaccine (the Step Study): a double-blind, randomised, placebo-controlled, test-of-concept trial. Lancet 372, 1881–1893. doi: 10.1016/S0140-6736(08)61591-3
Burton, D. R. (2019). Advancing an HIV vaccine; advancing vaccinology. Nat. Rev. Immunol. 19:77. doi: 10.1038/s41577-018-0103-6
Casimiro, D. R., Wang, F., Schleif, W. A., Liang, X., Zhang, Z. Q., Tobery, T. W., et al. (2005). Attenuation of simian immunodeficiency virus SIVmac239 infection by prophylactic immunization with DNA and recombinant adenoviral vaccine vectors expressing Gag. J. Virol. 79, 15547–15555. doi: 10.1128/JVI.79.24.15547-15555.2005
Castilow, E. M., Olson, M. R., and Varga, S. M. (2007). Understanding respiratory syncytial virus (RSV) vaccine-enhanced disease. Immunol. Res. 39, 225–239. doi: 10.1007/s12026-007-0071-6
Cele, S., Jackson, L., Khoury, D. S., Khan, K., Moyo-Gwete, T., Tegally, H., et al. (2021). Omicron extensively but incompletely escapes Pfizer BNT162b2 neutralization. Nature 602, 654–656. doi: 10.1038/s41586-021-04387-1
Centlivre, M., and Combadière, B. (2015). New challenges in modern vaccinology. BMC Immunol. 16:18. doi: 10.1186/s12865-015-0075-2
Chang, M. S., Lu, Y. T., Ho, S. T., Wu, C. C., Wei, T. Y., Chen, C. J., et al. (2004). Antibody detection of SARS-CoV spike and nucleocapsid protein. Biochem. Biophys. Res. Commun. 314, 931–936. doi: 10.1016/j.bbrc.2003.12.195
Channappanavar, R., Fett, C., Zhao, J., Meyerholz, D. K., and Perlman, S. (2014). Virus-specific memory CD8 T cells provide substantial protection from lethal severe acute respiratory syndrome coronavirus Infection. J. Virol. 88, 11034–11044. doi: 10.1128/JVI.01505-14
Channappanavar, R., and Perlman, S. (2017). Pathogenic human coronavirus infections: causes and consequences of cytokine storm and immunopathology. Semin. Immunopathol. 39, 529–539. doi: 10.1007/s00281-017-0629-x
Charles River (2021). Humanized Mice Join Other Animal Models in the COVID-19 Fight. Wilmington, MA: Charles River.
Chen, W. H., Tao, X., Agrawal, A. S., Algaissi, A., Peng, B. H., Pollet, J., et al. (2020). Yeast-expressed SARS-CoV recombinant receptor-binding domain (RBD219-N1) formulated with aluminum hydroxide induces protective immunity and reduces immune enhancement. Vaccine 38, 7533–7541. doi: 10.1016/j.vaccine.2020.09.061
Chen, Y., Wei, Q., Li, R., Gao, H., Zhu, H., Deng, W., et al. (2020). Protection of Rhesus Macaque from SARS-Coronavirus challenge by recombinant adenovirus vaccine. bioRxiv [Preprint]. doi: 10.1101/2020.02.17.951939
Cohen, J. (2006). Immunology. differences in immune cell “brakes” may explain chimp-human split on AIDS. Science 312, 672–673. doi: 10.1126/science.312.5774.672b
Connors, M., Giese, N. A., Kulkarni, A. B., Firestone, C. Y., Morse, H. C., and Murphy, B. R. (1994). Enhanced pulmonary histopathology induced by respiratory syncytial virus (RSV) challenge of formalin-inactivated RSV-immunized BALB/c mice is abrogated by depletion of interleukin-4 (IL-4) and IL-10. J. Virol. 68, 5321–5325. doi: 10.1128/jvi.68.8.5321-5325.1994
Corbett, K. S., Flynn, B., Foulds, K. E., Francica, J. R., Boyoglu-Barnum, S., Werner, A. P., et al. (2020). Evaluation of the mRNA-1273 Vaccine against SARS-CoV-2 in Nonhuman Primates. N. Engl. J. Med. 383, 1544–1555. doi: 10.1056/NEJMoa2024671
Covid World Vaccination Tracker (2022). Covid World Vaccination Tracker. New York, NY: The New York Times.
Davidson, A. M., Wysocki, J., and Batlle, D. (2020). Interaction of SARS-CoV-2 and Other Coronavirus With ACE (Angiotensin-Converting Enzyme)-2 as Their Main Receptor. Hypertension 76, 1339–1349. doi: 10.1161/HYPERTENSIONAHA.120.15256
de Alwis, R., Williams, K. L., Schmid, M. A., Lai, C.-Y., Patel, B., Smith, S. A., et al. (2014). Dengue viruses are enhanced by distinct populations of serotype cross-reactive antibodies in human immune sera. PLoS Pathog. 10:e1004386. doi: 10.1371/journal.ppat.1004386
de Parseval, A., Ngo, S., Sun, P., and Elder, J. H. (2004). Factors that increase the effective concentration of CXCR4 dictate feline immunodeficiency virus tropism and kinetics of replication. J. Virol. 78, 9132–9143. doi: 10.1128/JVI.78.17.9132-9143.2004
de Swart, R. L., Kuiken, T., Timmerman, H. H., Amerongen, G. V., van den Hoogen, B. G., Vos, H. W., et al. (2002). Immunization of macaques with formalin-inactivated respiratory syncytial virus (RSV) induces interleukin-13-associated hypersensitivity to subsequent RSV infection. J. Virol. 76, 11561–11569. doi: 10.1128/JVI.76.22.11561-11569.2002
Dejnirattisai, W., Jumnainsong, A., Onsirisakul, N., Fitton, P., Vasanawathana, S., Limpitikul, W., et al. (2010). Cross-reacting antibodies enhance dengue virus infection in humans. Science 328, 745–748. doi: 10.1126/science.1185181
Delgado, M. F., Coviello, S., Monsalvo, A. C., Melendi, G. A., Hernandez, J. Z., Batalle, J. P., et al. (2009). Lack of antibody affinity maturation due to poor Toll-like receptor stimulation leads to enhanced respiratory syncytial virus disease. Nat. Med. 15, 34–41. doi: 10.1038/nm.1894
Delgado, M. F., and Polack, F. P. (2004). Involvement of antibody, complement and cellular immunity in the pathogenesis of enhanced respiratory syncytial virus disease. Expert. Rev. Vaccines 3, 693–700. doi: 10.1586/14760584.3.6.693
Deming, M. E., Michael, N. L., Robb, M., Cohen, M. S., and Neuzil, K. M. (2020). Accelerating development of SARS-CoV-2 Vaccines — the role for controlled human infection models. N. Engl. J. Med. 383:e63. doi: 10.1056/NEJMp2020076
Denayer, T., Stöhr, T., and Roy, M. V. (2014). Animal models in translational medicine: validation and prediction. Eur. J. Mol. Clin. Med. 2:5. doi: 10.1016/j.nhtm.2014.08.001
DeVincenzo, J., Gymnopoulou, E., De Paepe, E., Murray, B., Rosemary Bastian, A., Haazen, W., et al. (2019). A Randomized, double-blind, placebo-controlled study to evaluate the efficacy of a single immunization of Ad26.RSV.preF against RSV infection in a viral challenge model in healthy adults. Open Forum Infect. Dis. 6, S27–S28.
Diamond, M. S., and Pierson, T. C. (2015). Molecular insight into dengue virus pathogenesis and its implications for disease control. Cell 162, 488–492. doi: 10.1016/j.cell.2015.07.005
Dinnon, K. H., Leist, S. R., Schäfer, A., Edwards, C. E., Martinez, D. R., Montgomery, S. A., et al. (2020). A mouse-adapted model of SARS-CoV-2 to test COVID-19 countermeasures. Nature 586, 560–566. doi: 10.1038/s41586-020-2708-8
Douek, D. C., Brenchley, J. M., Betts, M. R., Ambrozak, D. R., Hill, B. J., Okamoto, Y., et al. (2002). HIV preferentially infects HIV-specific CD4+ T cells. Nature 417, 95–98. doi: 10.1038/417095a
Dowd, K. A., Jost, C. A., Durbin, A. P., Whitehead, S. S., and Pierson, T. C. (2011). A dynamic landscape for antibody binding modulates antibody-mediated neutralization of West Nile virus. PLoS Pathog. 7:1002111. doi: 10.1371/journal.ppat.1002111
Du, L., He, Y., Zhou, Y., Liu, S., Zheng, B. J., and Jiang, S. (2009). The spike protein of SARS-CoV - a target for vaccine and therapeutic development. Nat. Rev. Microbiol. 7, 226–236. doi: 10.1038/nrmicro2090
Duerr, A., Huang, Y., Buchbinder, S., Coombs, R. W., Sanchez, J., Del Rio, C., et al. (2012). Extended Follow-up confirms early vaccine-enhanced risk of HIV acquisition and demonstrates waning effect over time among participants in a randomized trial of recombinant adenovirus HIV Vaccine (Step Study). J. Infect. Dis. 206, 258–266. doi: 10.1093/infdis/jis342
Durbin, A. P., and Whitehead, S. S. (2011). Next-generation dengue vaccines: novel strategies currently under development. Viruses 3, 1800–1814. doi: 10.3390/v3101800
Edwards, K. M., and Neuzil, K. M. (2022). Understanding COVID-19 through human challenge models. Nat. Med. 28, 903–904. doi: 10.1038/s41591-022-01778-3
Estes, J. D., Wong, S. W., and Brenchley, J. M. (2018). Nonhuman primate models of human viral infections. Nat. Rev. Immunol. 18, 390–404. doi: 10.1038/s41577-018-0005-7
European Commission (2014). Clinical trials. Regulation EU No 536/2014. Brussels: European Commission.
Excler, J. L., Saville, M., Berkley, S., and Kim, J. H. (2021). Vaccine development for emerging infectious diseases. Nat. Med. 27, 591–600. doi: 10.1038/s41591-021-01301-0
Fazekas de St Groth, B., and Webster, R. G. (1966). Disquisitions of Original Antigenic Sin. I. Evidence in man. J. Exp. Med. 124, 331–345. doi: 10.1084/jem.124.3.331
Feinberg, M. B., and Moore, J. P. (2002). AIDS vaccine models: challenging challenge viruses. Nat. Med. 8, 207–210. doi: 10.1038/nm0302-207
Feraoun, Y., Maisonnasse, P., Le Grand, R., and Beignon, A. S. (2021). COVID-19, des vaccins à la vitesse de l’éclair. Méd. Sci. 37, 759–772. doi: 10.1051/medsci/2021094
Fierz, W., and Walz, B. (2020). Antibody dependent enhancement due to original antigenic sin and the development of SARS. Front. Immunol. 11:1120. doi: 10.3389/fimmu.2020.01120
Flipse, J., Diosa-Toro, M. A., Hoornweg, T. E., Van De Pol, D. P. I., Urcuqui-Inchima, S., and Smit, J. M. (2016). Antibody-dependent enhancement of dengue virus infection in primary human macrophages; balancing higher fusion against antiviral responses. Sci. Rep. 6:29201. doi: 10.1038/srep29201
Focosi, D., Genoni, A., Lucenteforte, E., Tillati, S., Tamborini, A., Spezia, P. G., et al. (2021). Previous Humoral immunity to the endemic seasonal alphacoronaviruses NL63 and 229E is associated with worse clinical outcome in COVID-19 and suggests original antigenic sin. Life 11:298. doi: 10.3390/life11040298
Fontanet, A., Autran, B., Lina, B., Kieny, M. P., Karim, S. S. A., and Sridhar, D. (2021). SARS-CoV-2 variants and ending the COVID-19 pandemic. Lancet 397, 952–954. doi: 10.1016/S0140-6736(21)00370-6
Fujiwara, S. (2018). Humanized mice: a brief overview on their diverse applications in biomedical research. J. Cell Physiol. 233, 2889–2901. doi: 10.1002/jcp.26022
Fulginiti, V. A., Eller, J. J., Downie, A. W., and Kempe, C. H. (1967). altered reactivity to measles virus: atypical measles in children previously immunized with inactivated measles virus Vaccines. JAMA 202, 1075–1080. doi: 10.1001/jama.1967.03130250057008
Gan, E. S., Ting, D. H. R., and Chan, K. R. (2017). The mechanistic role of antibodies to dengue virus in protection and disease pathogenesis. Expert. Rev. Anti. Infect. Ther. 15, 111–119. doi: 10.1080/14787210.2017.1254550
Gao, Q., Bao, L., Mao, H., Wang, L., Xu, K., Yang, M., et al. (2020). Development of an inactivated vaccine candidate for SARS-CoV-2. Science 369, 77–81. doi: 10.1126/science.abc1932
Gao, Y., Li, T., Han, M., Li, X., Wu, D., Xu, Y., et al. (2020). Diagnostic utility of clinical laboratory data determinations for patients with the severe COVID-19. J. Med. Virol. 92, 791–796. doi: 10.1002/jmv.25770
Gartlan, C., Tipton, T., Salguero, F. J., Sattentau, Q., Gorringe, A., and Carroll, M. W. (2022). Vaccine-Associated enhanced disease and pathogenic human coronaviruses. Front. Immunol. 13:882972. doi: 10.3389/fimmu.2022.882972
Giannecchini, S., Isola, P., Sichi, O., Matteucci, D., Pistello, M., Zaccaro, L., et al. (2002). AIDS vaccination studies using an ex vivo feline immunodeficiency virus model: failure to protect and possible enhancement of challenge infection by four cell-based vaccines prepared with autologous lymphoblasts. J. Virol. 76, 6882–6892. doi: 10.1128/JVI.76.14.6882-6892.2002
Graham, B. S. (2011). Biological challenges and technological opportunities for respiratory syncytial virus vaccine development. Immunol. Rev. 239, 149–166. doi: 10.1111/j.1600-065X.2010.00972.x
Graham, B. S. (2020). Rapid COVID-19 vaccine development. Science 368, 945–946. doi: 10.1126/science.abb8923
Grant, R. A., Morales-Nebreda, L., Markov, N. S., Swaminathan, S., Querrey, M., Guzman, E. R., et al. (2021). Circuits between infected macrophages and T cells in SARS-CoV-2 pneumonia. Nature 590, 635–641. doi: 10.1038/s41586-020-03148-w
Greenwood, B. (1645). The contribution of vaccination to global health: past, present and future. Philos. Trans. R Soc. B Biol. Sci. 2014:369.
Groß, R., Zanoni, M., Seidel, A., Conzelmann, C., Gilg, A., Krnavek, D., et al. (2022). Heterologous ChAdOx1 nCoV-19 and BNT162b2 prime-boost vaccination elicits potent neutralizing antibody responses and T cell reactivity against prevalent SARS-CoV-2 variants. EBioMedicine 75:103761. doi: 10.1016/j.ebiom.2021.103761
Guzman, M. G., Alvarez, M., and Halstead, S. B. (2013). Secondary infection as a risk factor for dengue hemorrhagic fever/dengue shock syndrome: An historical perspective and role of antibody-dependent enhancement of infection. Arch. Virol. 158, 1445–1459. doi: 10.1007/s00705-013-1645-3
Hacisuleyman, E., Hale, C., Saito, Y., Blachere, N. E., Bergh, M., Conlon, E. G., et al. (2021). Vaccine Breakthrough Infections with SARS-CoV-2 Variants. N. Engl. J. Med. 384, 2212–2218. doi: 10.1056/NEJMoa2105000
Hadinegoro, S. R., Arredondo-García, J. L., Capeding, M. R., Deseda, C., Chotpitayasunondh, T., Dietze, R., et al. (2015). Efficacy and long-term safety of a dengue vaccine in regions of endemic disease. N. Engl. J. Med. 373, 1195–1206. doi: 10.1056/NEJMoa1506223
Halstead, S. B. (1979). In vivo enhancement of dengue virus infection in rhesus monkeys by passively transferred antibody. J. Infect. Dis. 140, 527–533. doi: 10.1093/infdis/140.4.527
Halstead, S. B. (2014). Dengue antibody-dependent enhancement: knowns and unknowns. Microbiol. Spectr. 2:6. doi: 10.1128/microbiolspec.AID-0022-2014
Halstead, S. B. (2018). Safety issues from a Phase 3 clinical trial of a live-attenuated chimeric yellow fever tetravalent dengue vaccine. Hum. Vaccin. Immunother. 14, 2158–2162. doi: 10.1080/21645515.2018.1445448
Halstead, S. B. (2021). Vaccine-associated enhanced viral disease: implications for viral vaccine Development. Biodrugs 35:1. doi: 10.1007/s40259-021-00495-6
Halstead, S. B., and Cohen, S. N. (2015). Dengue hemorrhagic fever at 60 years: early evolution of concepts of causation and treatment. Microbiol. Mol. Biol. Rev. 79:281. doi: 10.1128/MMBR.00009-15
Halstead, S. B., Katzelnick, L. C., Russell, P. K., Markoff, L., Aguiar, M., Dans, L. R., et al. (2020). Ethics of a partially effective dengue vaccine: lessons from the Philippines. Vaccine 38, 5572–5576. doi: 10.1016/j.vaccine.2020.06.079
Halstead, S. B., Lan, N. T., Myint, T. T., Shwe, T. N., Nisalak, A., Kalyanarooj, S., et al. (2002). Dengue hemorrhagic fever in infants: research opportunities ignored. Emerg. Infect. Dis.. 8, 1474–1479. doi: 10.3201/eid0812.020170
Halstead, S. B., Mahalingam, S., Marovich, M. A., Ubol, S., and Mosser, D. M. (2010). Intrinsic antibody-dependent enhancement of microbial infection in macrophages: disease regulation by immune complexes. Lancet Infect. Dis. 10, 712–722. doi: 10.1016/S1473-3099(10)70166-3
Halstead, S. B., and O’Rourke, E. J. (1977). Antibody-enhanced dengue virus infection in primate leukocytes. Nature 265, 739–741. doi: 10.1038/265739a0
Halstead, S. B., Shotwell, H., and Casals, J. (1973). Studies on the pathogenesis of dengue infection in monkeys, II. clinical laboratory responses to heterologous infection. J. Infect. Dis. 128, 15–22. doi: 10.1093/infdis/128.1.15
Haynes, B. F., Corey, L., Fernandes, P., Gilbert, P. B., Hotez, P. J., Rao, S., et al. (2020). Prospects for a safe COVID-19 vaccine. Sci. Transl. Med. 12:948.
Honda-Okubo, Y., Barnard, D., Ong, C. H., Peng, B.-H., Tseng, C.-T. K., and Petrovsky, N. (2015). Severe acute respiratory syndrome-associated coronavirus vaccines formulated with delta inulin adjuvants provide enhanced protection while ameliorating lung eosinophilic immunopathology. J. Virol. 89, 2995–3007. doi: 10.1128/JVI.02980-14
Hosie, M. J., Osborne, R., Reid, G., Neil, J. C., and Jarrett, O. (1992). Enhancement after feline immunodeficiency virus vaccination. Vet Immunol. Immunopathol. 35, 191–197. doi: 10.1016/0165-2427(92)90131-9
Hotez, P. J., Bottazzi, M. E., and Corry, D. B. (2020). The potential role of Th17 immune responses in coronavirus immunopathology and vaccine-induced immune enhancement. Microbes Infect. 22, 165–167. doi: 10.1016/j.micinf.2020.04.005
Hsueh, P. R., Hsiao, C. H., Yeh, S. H., Wang, W. K., Chen, P. J., Wang, J. T., et al. (2003). Microbiologic Characteristics, serologic responses, and clinical manifestations in severe acute respiratory syndrome, taiwan. Emerg. Infect. Dis. 9:1163.
Huang, C., Wang, Y., Li, X., Ren, L., Zhao, J., Hu, Y., et al. (2020). Clinical features of patients infected with 2019 novel coronavirus in Wuhan, China. Lancet 395, 497–506. doi: 10.1016/S0140-6736(20)30183-5
Huang, Y., Duerr, A., Frahm, N., Zhang, L., Moodie, Z., De Rosa, S., et al. (2014). Immune-Correlates Analysis of an HIV-1 Vaccine efficacy trial reveals an association of nonspecific interferon-γ secretion with increased hiv-1 infection risk: a cohort-based modeling study. PLoS One 9:e108631. doi: 10.1371/journal.pone.0108631
Huisman, W., Martina, B. E. E., Rimmelzwaan, G. F., Gruters, R. A., and Osterhaus, A. D. M. E. (2009). Vaccine-induced enhancement of viral infections. Vaccine 27, 505–512. doi: 10.1016/j.vaccine.2008.10.087
Hussell, T., Baldwin, C. J., O’Garra, A., and Openshaw, P. J. M. (1997). CD8+ T cells control Th2-driven pathology during pulmonary respiratory syncytial virus infection. Eur. J. Immunol. 27, 3341–3349. doi: 10.1002/eji.1830271233
INSIGHT START Study Group, J. D., Babiker, AG., Gordin, F., Emery, S., and Grund, B. (2015). Initiation of Antiretroviral Therapy in Early Asymptomatic HIV Infection. N. Engl. J. Med. 373, 795–807. doi: 10.1056/NEJMoa1506816
Iwasaki, A., and Medzhitov, R. (2010). Regulation of adaptive immunity by the innate immune system. Science 327, 291–295. doi: 10.1126/science.1183021
Iwata-Yoshikawa, N., Shiwa, N., Sekizuka, T., Sano, K., Ainai, A., Hemmi, T., et al. (2022). A lethal mouse model for evaluating vaccine-associated enhanced respiratory disease during SARS-CoV-2 infection. Sci. Adv. 8:3827.
Jafarzadeh, A., Chauhan, P., Saha, B., Jafarzadeh, S., and Nemati, M. (2020). Contribution of monocytes and macrophages to the local tissue inflammation and cytokine storm in COVID-19: Lessons from SARS and MERS, and potential therapeutic interventions. Life Sci. 257:118102. doi: 10.1016/j.lfs.2020.118102
Jamrozik, E., Heriot, G., Bull, S., and Parker, M. (2021a). Vaccine-enhanced disease: case studies and ethical implications for research and public health. Wellcome Open Res. 6:154.
Jamrozik, E., Littler, K., Bull, S., Emerson, C., Kang, G., Kapulu, M., et al. (2021b). Key criteria for the ethical acceptability of COVID-19 human challenge studies. Vaccine 39, 633–640.
Jane Cardosa, M., Porterfield, J. S., and Gordon, S. (1983). Complement receptor mediates enhanced flavivirus replication in macrophages. J. Exp. Med. 158, 258–263. doi: 10.1084/jem.158.1.258
Jaume, M., Yip, M. S., Cheung, C. Y., Leung, H. L., Li, P. H., Kien, F., et al. (2011). Anti-Severe Acute respiratory syndrome coronavirus spike antibodies trigger infection of human immune cells via a pH- and cysteine protease-independent Fc R Pathway. J. Virol. 85, 10582–10597. doi: 10.1128/JVI.00671-11
Johnson, T. R., and Graham, B. S. (1999). Secreted respiratory syncytial virus G glycoprotein induces interleukin-5 (IL-5), IL-13, and Eosinophilia by an IL-4-Independent Mechanism. J. Virol. 73, 8485–8495. doi: 10.1128/JVI.73.10.8485-8495.1999
Junqueira, C., Crespo, Â, Ranjbar, S., de Lacerda, L. B., Lewandrowski, M., Ingber, J., et al. (2022). FcγR-mediated SARS-CoV-2 infection of monocytes activates inflammation. Nature 606, 576–584. doi: 10.1038/s41586-022-04702-4
Kam, Y. W., Kien, F., Roberts, A., Cheung, Y. C., Lamirande, E. W., Vogel, L., et al. (2007). Antibodies against trimeric S glycoprotein protect hamsters against SARS-CoV challenge despite their capacity to mediate FcγRII-dependent entry into B cells in vitro. Vaccine 25, 729–740. doi: 10.1016/j.vaccine.2006.08.011
Karim, S. S. A. (2021). Vaccines and SARS-CoV-2 variants: the urgent need for a correlate of protection. Lancet 397, 1263–1264. doi: 10.1016/S0140-6736(21)00468-2
Karlas, J. A., Siebelink, K. H. J., Peer, M. A. V., Huisman, W., Cuisinier, A. M., Rimmelzwaan, G. F., et al. (1999). Vaccination with experimental feline immunodeficiency virus vaccines, based on autologous infected cells, elicits enhancement of homologous challenge infection. J. Gen. Virol. 80, 761–765. doi: 10.1099/0022-1317-80-3-761
Katzelnick, L. C., Narvaez, C., Arguello, S., Mercado, B. L., Collado, D., Ampie, O., et al. (2020b). Zika virus infection enhances future risk of severe dengue disease. Science 369, 1123–1128.
Katzelnick, L. C., Bos, S., and Harris, E. (2020a). Protective and enhancing interactions among dengue viruses 1-4 and Zika virus. Curr. Opin. Virol. 43, 59–70.
Katzelnick, L. C., Gresh, L., Halloran, M. E., Mercado, J. C., Kuan, G., Gordon, A., et al. (2017). Antibody-dependent enhancement of severe dengue disease in humans. Science 358, 929–932. doi: 10.1126/science.aan6836
Katzelnick, L. C., Zambrana, J. V., Elizondo, D., Collado, D., Garcia, N., Arguello, S., et al. (2021). Dengue and Zika virus infection in children elicit cross-reactive protective and enhancing antibodies that persist long term. Sci. Transl. Med. 13:eabg9478.
Killikelly, A. M., Kanekiyo, M., and Graham, B. S. (2016). Pre-fusion F is absent on the surface of formalin-inactivated respiratory syncytial virus. Sci. Rep. 6:34108.
Killingley, B., Mann, A. J., Kalinova, M., Boyers, A., Goonawardane, N., Zhou, J., et al. (2022). Safety, tolerability and viral kinetics during SARS-CoV-2 human challenge in young adults. Nat. Med. 28, 1031–1041. doi: 10.1038/s41591-022-01780-9
Kim, H. W., Canchola, J. G., Brandt, C. D., Pyles, G., Chanock, R. M., Jensen, K., et al. (1969). Respiratory syncytial virus disease in infants despite prior administration of antigenic inactivated vaccine12. Am. J. Epidemiol. 89, 422–434. doi: 10.1093/oxfordjournals.aje.a120955
Kim, J. H., Skountzou, I., Compans, R., and Jacob, J. (2009). Original antigenic sin responses to influenza Viruses. J. Immunol. 183, 3294. doi: 10.4049/jimmunol.0900398
Kiros, T. G., Levast, B., Auray, G., Strom, S., Van Kessel, J., and Gerdts, V. (2012). The importance of animal models in the development of vaccines. Innov. Vaccinol. 13, 251–264. doi: 10.1007/978-94-007-4543-8_11
Klenerman, P., and Zinkernagel, R. M. (1998). Original antigenic sin impairs cytotoxic T lymphocyte responses to viruses bearing variant epitopes. Nature 394, 482–485. doi: 10.1038/28860
Knudson, C. J., Hartwig, S. M., Meyerholz, D. K., and Varga, S. M. (2015). RSV Vaccine-Enhanced Disease Is Orchestrated by the Combined Actions of Distinct CD4 T Cell Subsets. PLoS Pathog. 11:e1004757. doi: 10.1371/journal.ppat.1004757
Koblin, B. A., Mayer, K. H., Noonan, E., Wang, C. Y., Marmor, M., Sanchez, J., et al. (2012). Sexual risk behaviors, circumcision status and pre-existing immunity to adenovirus type 5 among men who have sex with men participating in a randomized HIV-1 vaccine efficacy trial: Step Study. J. Acquir. Immune Defic. Syndr. 60:405. doi: 10.1097/QAI.0b013e31825325aa
Koch, J., Uckeley, Z. M., Doldan, P., Stanifer, M., Boulant, S., and Lozach, P. (2021). TMPRSS2 expression dictates the entry route used by SARS-CoV-2 to infect host cells. EMBO J. 40:e107821. doi: 10.15252/embj.2021107821
Krarup, A., Truan, D., Furmanova-Hollenstein, P., Bogaert, L., Bouchier, P., Bisschop, I. J. M., et al. (2015). A highly stable prefusion RSV F vaccine derived from structural analysis of the fusion mechanism. Nat. Commun. 6: 8143. doi: 10.1038/ncomms9143
Krause, P. R., Fleming, T. R., Longini, I. M., Peto, R., Briand, S., Heymann, D. L., et al. (2021). SARS-CoV-2 Variants and Vaccines. N. Engl. J. Med. 385, 179–186. doi: 10.1056/NEJMsr2105280
Lambert, P. H., Ambrosino, D. M., Andersen, S. R., Baric, R. S., Black, S. B., Chen, R. T., et al. (2020). Consensus summary report for CEPI/BC March 12-13, 2020 meeting: Assessment of risk of disease enhancement with COVID-19 vaccines. Vaccine 38, 4783–4791. doi: 10.1016/j.vaccine.2020.05.064
Lan, J., Yao, Y., Deng, Y., Chen, H., Lu, G., Wang, W., et al. (2015). Recombinant Receptor Binding Domain Protein Induces Partial Protective Immunity in Rhesus Macaques Against Middle East Respiratory Syndrome Coronavirus Challenge. EBioMedicine. 2, 1438–1446. doi: 10.1016/j.ebiom.2015.08.031
Leidner, A. J., Murthy, N., Chesson, H. W., Biggerstaff, M., Stoecker, C., Harris, A. M., et al. (2019). Cost-effectiveness of adult vaccinations: a systematic review. Vaccine 37, 226–234. doi: 10.1016/j.vaccine.2018.11.056
Li, D., Edwards, R. J., Manne, K., Martinez, D. R., Schäfer, A., Alam, S. M., et al. (2021). In vitro and in vivo functions of SARS-CoV-2 infection-enhancing and neutralizing antibodies. Cell 184:4203. doi: 10.1016/j.cell.2021.06.021
Li, L., Wo, J., Shao, J., Zhu, H., Wu, N., Li, M., et al. (2003). SARS-coronavirus replicates in mononuclear cells of peripheral blood (PBMCs) from SARS patients. J. Clin. Virol. 28, 239–244. doi: 10.1016/S1386-6532(03)00195-1
Li, W., Moore, M. J., Vasllieva, N., Sui, J., Wong, S. K., Berne, M. A., et al. (2003). Angiotensin-converting enzyme 2 is a functional receptor for the SARS coronavirus. Nature 426, 450–454. doi: 10.1038/nature02145
Li, T., Xie, J., He, Y., Fan, H., Baril, L., Qiu, Z., et al. (2006). Long-term persistence of robust antibody and cytotoxic T cell responses in recovered patients infected with SARS coronavirus. PLoS One 1:e24. doi: 10.1371/journal.pone.0000024
Liang, X., Casimiro, D. R., Schleif, W. A., Wang, F., Davies, M.-E., Zhang, Z.-Q., et al. (2005). Vectored Gag and Env but Not Tat Show Efficacy against Simian-Human Immunodeficiency Virus 89.6P Challenge in Mamu-A * 01-Negative Rhesus Monkeys. J. Virol. 79, 12321–12331. doi: 10.1128/JVI.79.19.12321-12331.2005
Linn, M. L., Aaskov, J. G., and Suhrbier, A. (1996). Antibody-dependent enhancement and persistence in macrophages of an arbovirus associated with arthritis. J. Gen. Virol. 77, 407–411. doi: 10.1099/0022-1317-77-3-407
Liu, L., Wei, Q., Lin, Q., Fang, J., Wang, H., Kwok, H., et al. (2019). Anti-spike IgG causes severe acute lung injury by skewing macrophage responses during acute SARS-CoV infection. JCI Insight 1:4. doi: 10.1172/jci.insight.123158
Lu, L. L., Suscovich, T. J., Fortune, S. M., and Alter, G. (2017). Beyond binding: antibody effector functions in infectious diseases. Nat. Rev. Immunol. 18, 46–61. doi: 10.1038/nri.2017.106
Lun, W. H., Takeda, A., Nakamura, H., Kano, M., Mori, K., Sata, T., et al. (2004). Loss of virus-specific CD4+ T cells with increases in viral loads in the chronic phase after vaccine-based partial control of primary simian immunodeficiency virus replication in macaques. J. Gen. Virol. 85, 1955–1963. doi: 10.1099/vir.0.79890-0
Luo, F., Liao, F. L., Wang, H., Tang, H. B., Yang, Z. Q., and Hou, W. (2018). Evaluation of Antibody-Dependent Enhancement of SARS-CoV Infection in Rhesus Macaques Immunized with an Inactivated SARS-CoV Vaccine. Virol. Sin. 33, 201–204. doi: 10.1007/s12250-018-0009-2
Maemura, T., Kuroda, M., Armbrust, T., Yamayoshi, S., Halfmann, P. J., and Kawaoka, Y. (2021). Antibody-Dependent Enhancement of SARS-CoV-2 Infection Is Mediated by the IgG Receptors FcγRIIA and FcγRIIIA but Does Not Contribute to Aberrant Cytokine Production by Macrophages. mBio 12:e0198721. doi: 10.1128/mBio.01987-21
Marlin, R. (2021). Targeting SARS-CoV-2 receptor-binding domain to cells expressing CD40 improves protection to infection in convalescent macaques. Nat. Commun. 12:5215.
Martin, D. B., Weiner, L. B., Nieburg, P. I., and Blair, D. C. (1979). Atypical measles in adolescents and young adults. Ann. Intern. Med. 90, 877–881. doi: 10.7326/0003-4819-90-6-877
Martines, R. B., Ritter, J. M., Matkovic, E., Gary, J., Bollweg, B. C., Bullock, H., et al. (2020). Pathology and Pathogenesis of SARS-CoV-2 Associated with Fatal Coronavirus Disease, United. Emerg. Infect. Dis. 26, 2005–2015. doi: 10.3201/eid2609.202095
Martinez, D. R., Schäfer, A., Leist, S. R., De la Cruz, G., West, A., Atochina-Vasserman, E. N., et al. (2021). Chimeric spike mRNA vaccines protect against Sarbecovirus challenge in mice. Science 373, 991–998. doi: 10.1126/science.abi4506
McElrath, M. J., De Rosa, S. C., Moodie, Z., Dubey, S., Kierstead, L., Janes, H., et al. (2008). HIV-1 vaccine-induced immunity in the test-of-concept Step Study: a case–cohort analysis. Lancet 372, 1894–1905. doi: 10.1016/S0140-6736(08)61592-5
McNaughton, A. L., Paton, R. S., Edmans, M., Youngs, J. C. W., Wellens, J., Phalora, P., et al. (2022). Fatal COVID-19 outcomes are associated with an antibody response targeting epitopes shared with endemic coronaviruses. JCI Insight 7:e156372. doi: 10.1172/jci.insight.156372
Melendi, G. A., Hoffman, S. J., Karron, R. A., Irusta, P. M., Laham, F. R., Humbles, A., et al. (2007). C5 Modulates airway hyperreactivity and pulmonary eosinophilia during enhanced respiratory syncytial virus disease by decreasing C3a Receptor Expression. J. Virol. 81, 991–999. doi: 10.1128/JVI.01783-06
Melnick, J. L. (1996). Current status of poliovirus infections. Clin. Microbiol. Rev. 9, 293–300. doi: 10.1128/CMR.9.3.293
MERS-CoV (2019). Middle East respiratory syndrome coronavirus (MERS-CoV). Geneva: World Health Organization.
Mileto, D., Fenizia, C., Cutrera, M., Gagliardi, G., Gigantiello, A., De Silvestri, A., et al. (2021). SARS-CoV-2 mRNA vaccine BNT162b2 triggers a consistent cross-variant humoral and cellular response. Emerg. Microbes Infect. 10, 2235–2243. doi: 10.1080/22221751.2021.2004866
Moghaddam, A., Olszewska, W., Wang, B., Tregoning, J. S., Helson, R., Sattentau, Q. J., et al. (2006). A potential molecular mechanism for hypersensitivity caused by formalin-inactivated vaccines. Nat. Med. 12, 905–907. doi: 10.1038/nm1456
Moi, M. L., Lim, C.-K., Kotaki, A., Takasaki, T., and Kurane, I. (2011). Detection of Higher Levels of Dengue Viremia Using FcγR-Expressing BHK-21 Cells Than FcγR-Negative Cells in Secondary Infection but Not in Primary Infection. J. Infect. Dis. 203, 1405–1414. doi: 10.1093/infdis/jir053
Mongkolsapaya, J., Dejnirattisai, W., Xu, X. N., Vasanawathana, S., Tangthawornchaikul, N., Chairunsri, A., et al. (2003). Original antigenic sin and apoptosis in the pathogenesis of dengue hemorrhagic fever. Nat. Med. 9, 921–927. doi: 10.1038/nm887
Moore, J. P., Kitchen, S. G., Pugach, P., and Zack, J. A. (2004). The CCR5 and CXCR4 coreceptors–central to understanding the transmission and pathogenesis of human immunodeficiency virus type 1 infection. AIDS Res. Hum. Retrovir. 20, 111–126. doi: 10.1089/088922204322749567
Moore, J. P., Klasse, P. J., Dolan, M. J., and Ahuja, S. K. (2008). AIDS/HIV. A STEP into darkness or light? Science 320, 753–755.
Munoz, F. M., Cramer, J. P., Dekker, C. L., Dudley, M. Z., Graham, B. S., Gurwith, M., et al. (2021). Vaccine-associated enhanced disease: case definition and guidelines for data collection, analysis, and presentation of immunization safety data. Vaccine 39:3053. doi: 10.1016/j.vaccine.2021.01.055
Murphy, B. R., Prince, G. A., Walsh, E. E., Kim, H. W., Parrott, R. H., Hemming, V. G., et al. (1986). Dissociation between serum neutralizing and glycoprotein antibody responses of infants and children who received inactivated respiratory syncytial virus vaccine. J Clin Microbiol. 24, 197–202. doi: 10.1128/jcm.24.2.197-202.1986
Naaber, P., Tserel, L., Kangro, K., Sepp, E., Jürjenson, V., Adamson, A., et al. (2021). Dynamics of antibody response to BNT162b2 vaccine after six months: a longitudinal prospective study. Lancet Reg. Health Eur. 10:100208. doi: 10.1016/j.lanepe.2021.100208
Nader, P. R., Horwitz, M. S., and Rousseau, J. (1968). Atypical exanthem following exposure to natural measles: Eleven cases in children previously inoculated with killed vaccine. J. Pediatr. 72, 22–28. doi: 10.1016/S0022-3476(68)80396-8
Naif, H. M. (2013). Pathogenesis of HIV Infection. Infect. Dis. Rep. 5, (Suppl. 1), 26–30. doi: 10.4081/idr.2013.s1.e6
Narayan, R., and Tripathi, S. (2020). Intrinsic ADE: The Dark Side of Antibody Dependent Enhancement During Dengue Infection. Front. Cell Infect. Microbiol. 10:548. doi: 10.3389/fcimb.2020.580096
Nemet, I., Kliker, L., Lustig, Y., Zuckerman, N., Erster, O., Cohen, C., et al. (2022). Third BNT162b2 Vaccination Neutralization of SARS-CoV-2 Omicron Infection. N. Engl. J. Med. 386, 492–494. doi: 10.1056/NEJMc2119358
Nicholls, J. M., Poon, L. L. M., Lee, K. C., Ng, W. F., Lai, S. T., Leung, C. Y., et al. (2003). Lung pathology of fatal severe acute respiratory syndrome. Lancet 361, 1773–1778. doi: 10.1016/S0140-6736(03)13413-7
Nimmerjahn, F., and Ravetch, J. V. (2007). Fcγ receptors as regulators of immune responses. Nat. Rev. Immunol. 8, 34–47. doi: 10.1038/nri2206
O’Hagan, D. T., Friedland, L. R., Hanon, E., and Didierlaurent, A. M. (2017). Towards an evidence based approach for the development of adjuvanted vaccines. Curr. Opin. Immunol. 47, 93–102. doi: 10.1016/j.coi.2017.07.010
Osterhaus, A. D. M. E., Tijhaar, E., Huisman, R. C., Huisman, W., Darby, I. H., Francis, M. J., et al. (1996). Accelerated viremia in cats vaccinated with recombinant vaccinia virus expressing envelope glycoprotein of feline immunodeficiency virus the similarities between feline immunodeficiency virus. AIDS Res. Hum. Retroviruses 12, 437–441. doi: 10.1089/aid.1996.12.437
Ozawa, S., Clark, S., Portnoy, A., Grewal, S., Brenzel, L., and Walker, D. G. (2016). Return on investment from childhood immunization in low- and middle-income countries, 2011-20. Health Aff. 35, 199–207. doi: 10.1377/hlthaff.2015.1086
Palgen, J.-L., Feraoun, Y., Dzangué-Tchoupou, G., Joly, C., Martinon, F., Le Grand, R., et al. (2021). Optimize Prime/Boost Vaccine Strategies: Trained Immunity as a New Player in the Game. Front. Immunol. 12:612747. doi: 10.3389/fimmu.2021.612747
Peiris, J. S. M., Guan, Y., and Yuen, K. Y. (2004). Severe acute respiratory syndrome. Nat. Med. 10, S88–S97. doi: 10.1038/nm1143
Percivalle, E., Sammartino, J. C., Cassaniti, I., Arbustini, E., Urtis, M., Smirnova, A., et al. (2021). Macrophages and Monocytes: “Trojan Horses” in COVID-19. Viruses 13:2178. doi: 10.3390/v13112178
Percopo, C. M., Dyer, K. D., Ochkur, S. I., Luo, J. L., Fischer, E. R., Lee, J. J., et al. (2014). Activated mouse eosinophils protect against lethal respiratory virus infection. Blood 123, 743–752. doi: 10.1182/blood-2013-05-502443
Picker, L. J., and Watkins, D. I. (2005). HIV pathogenesis: the first cut is the deepest. Nat. Immunol. 6, 430–432. doi: 10.1038/ni0505-430
Pierson, T. C., Fremont, D. H., Kuhn, R. J., and Diamond, M. S. (2008). Structural Insights into the Mechanisms of Antibody-Mediated Neutralization of Flavivirus Infection: Implications for Vaccine Development. Cell Host Microbe 4, 229–238. doi: 10.1016/j.chom.2008.08.004
Pitman, M. C., Lau, J. S. Y., McMahon, J. H., and Lewin, S. R. (2018). Barriers and strategies to achieve a cure for HIV. Lancet HIV 5:e317. doi: 10.1016/S2352-3018(18)30039-0
Plotkin, S. A. (2010). Correlates of Protection Induced by Vaccination. Clin. Vaccine Immunol. 17, 1055–1065. doi: 10.1128/CVI.00131-10
Polack, F. P. (2007). Atypical measles and enhanced respiratory syncytial virus disease (ERD) made simple. Pediatric Res. 62, 111–115. doi: 10.1203/PDR.0b013e3180686ce0
Polack, F. P., Auwaerter, P. G., Lee, S. H., Nousari, H. C., Valsamakis, A., Leiferman, K. M., et al. (1999). Production of atypical measles in rhesus macaques: Evidence for disease mediated by immune complex formation and eosinophils in the presence of fusion-inhibiting antibody. Nat. Med. 5, 629–634. doi: 10.1038/9473
Polack, F. P., Hoffman, S. J., Crujeiras, G., and Griffin, D. E. (2003). A role for nonprotective complement-fixing antibodies with low avidity for measles virus in atypical measles. Nat. Med. 9, 1209–1213. doi: 10.1038/nm918
Polack, F. P., Teng, M. N., Collins, P. L., Prince, G. A., Exner, M., Regele, H., et al. (2002b). A role for immune complexes in enhanced respiratory syncytial virus disease. J. Exp. Med. 196, 859–865.
Polack, F. P., Hoffman, S. J., Moss, W. J., and Griffin, D. E. (2002a). Altered synthesis of interleukin-12 and type 1 and type 2 cytokines in rhesus macaques during measles and atypical measles. J. Infect. Dis. 185, 13–19.
Polack, F. P., Thomas, S. J., Kitchin, N., Absalon, J., Gurtman, A., Lockhart, S., et al. (2020). Safety and Efficacy of the BNT162b2 mRNA Covid-19 Vaccine. N. Engl. J. Med. 383, 2603–2615. doi: 10.1056/NEJMoa2034577
Pollard, A. J., and Bijker, E. M. (2020). A guide to vaccinology: from basic principles to new developments. Nat. Rev. Immunol. 21, 83–100. doi: 10.1038/s41577-020-00479-7
Préaud, E., Uhart, M., Böhm, K., Aidelsburger, P., Anger, D., Bianic, F., et al. (2015). Cost-effectiveness analysis of a vaccination program for the prevention of herpes zoster and post-herpetic neuralgia in adults aged 50 and over in Germany. Hum. Vaccin Immunother. 11:884.γhttps://doi.org/10.1080/21645515.2015.1011561 doi: 10.1080/21645515.2015.1011561
Qin, E., Shi, H., Tang, L., Wang, C., Chang, G., Ding, Z., et al. (2006). Immunogenicity and protective efficacy in monkeys of purified inactivated Vero-cell SARS vaccine. Vaccine 24, 1028–1034. doi: 10.1016/j.vaccine.2005.06.038
Querec, T. D., Akondy, R. S., Lee, E. K., Cao, W., Nakaya, H. I., Teuwen, D., et al. (2009). Systems biology approach predicts immunogenicity of the yellow fever vaccine in humans. Nat. Immunol. 10, 116–125. doi: 10.1038/ni.1688
Ragab, D., Salah Eldin, H., Taeimah, M., Khattab, R., and Salem, R. (2020). The COVID-19 Cytokine Storm, What We Know So Far. Front. Immunol. 11:1446. doi: 10.3389/fimmu.2020.01446
Rappuoli, R., Santoni, A., and Mantovani, A. (2019). Vaccines: An achievement of civilization, a human right, our health insurance for the future. J. Exp. Med. 216, 7–9. doi: 10.1084/jem.20182160
Reggeti, F., Ackerley, C., and Bienzle, D. (2008). CD134 and CXCR4 expression corresponds to feline immunodeficiency virus infection of lymphocytes, macrophages and dendritic cells. J. Gen. Virol. 89(Pt 1), 277–287. doi: 10.1099/vir.0.83161-0
Reynolds, C. J., Gibbons, J. M., Pade, C., Lin, K. M., Sandoval, D. M., Pieper, F., et al. (2022). Heterologous infection and vaccination shapes immunity against SARS-CoV-2 variants. Science 375, 183–192. doi: 10.1126/science.abm0811
Richardson, J., Broche, S., Baud, S., Leste-Lasserre, T., Féménia, F., Levy, D., et al. (2002). Lymphoid activation: a confounding factor in AIDS vaccine development? J. Gen. Virol. 83(Pt 10), 2515–2521. doi: 10.1099/0022-1317-83-10-2515
Richardson, J., Moraillon, A., Baud, S., Cuisinier, A. M., Sonigo, P., and Pancino, G. (1997). Enhancement of feline immunodeficiency virus (FIV) infection after DNA vaccination with the FIV envelope. J. Virol. 71, 9640–9649. doi: 10.1128/jvi.71.12.9640-9649.1997
Ricke, D. O. (2021). Two Different Antibody-Dependent Enhancement (ADE) Risks for SARS-CoV-2 Antibodies. Front. Immunol. 12:640093. doi: 10.3389/fimmu.2021.640093
Robinson, W. E. (2006). Mechanism for complement-mediated, antibody-dependent enhancement of human immunodeficiency virus type 1 infection in MT2 cells is enhanced entry through CD4, CD21, and CXCR4 chemokine receptors. Viral Immunol. 19, 434–447. doi: 10.1089/vim.2006.19.434
Rosenbaum, P., Tchitchek, N., Joly, C., Rodriguez Pozo, A., Stimmer, L., Langlois, S., et al. (2021). Vaccine inoculation route modulates early immunity and consequently antigen-specific immune response. Front. Immunol. 12:1362. doi: 10.3389/fimmu.2021.645210
Sabroe, I., Dockrell, D. H., Vogel, S. N., Renshaw, S. A., Whyte, M. K. B., and Dower, S. K. (2007). Identifying and hurdling obstacles to translational research. Nat. Rev. Immunol. 7, 77–82. doi: 10.1038/nri1999
Sahay, B., Nguyen, C. Q., and Yamamoto, J. K. (2017). Conserved HIV Epitopes for an Effective HIV Vaccine. J. Clin. Cell Immunol. 8:518. doi: 10.4172/2155-9899.1000518
Salje, H., Alera, M. T., Chua, M. N., Hunsawong, T., Ellison, D., Srikiatkhachorn, A., et al. (2021). Evaluation of extended efficacy of Dengvaxia vaccine against symptomatic and subclinical dengue infection. Nat. Med. 27:1395. doi: 10.1038/s41591-021-01392-9
Saunders, K. O., Lee, E., Parks, R., Martinez, D. R., Li, D., Chen, H., et al. (2021). Neutralizing antibody vaccine for pandemic and pre-emergent coronaviruses. Nature 594, 553–559. doi: 10.1038/s41586-021-03594-0
Schwartz, D. H. (1994). Potential pitfalls on the road to an effective HIV vaccine. Immunol. Today 15, 54–57. doi: 10.1016/0167-5699(94)90133-3
Sedova, E. S., Scherbinin, D. N., Lysenko, A. A., Alekseeva, S. V., Artemova, E. A., and Shmarov, M. M. (2019). Non-neutralizing antibodies directed at conservative influenza antigens. Acta Nat. 11, 22–32. doi: 10.32607/20758251-2019-11-4-22-32
Sefik, E., Qu, R., Junqueira, C., Kaffe, E., Mirza, H., Zhao, J., et al. (2022b). Inflammasome activation in infected macrophages drives COVID-19 pathology. Nature 606, 585–593.
Sefik, E., Israelow, B., Mirza, H., Zhao, J., Qu, R., Kaffe, E., et al. (2022a). A humanized mouse model of chronic COVID-19. Nat. Biotechnol. 40, 906–920.
Sefik, E., Israelow, B., Zhao, J., Qu, R., Song, E., Mirza, H., et al. (2021). A humanized mouse model of chronic COVID-19 to evaluate disease mechanisms and treatment options. Res Sq. [Preprint]. doi: 10.21203/rs.3.rs-279341/v1
Sekhar, A., and Kang, G. (2020). Human challenge trials in vaccine development. Semin. Immunol. 50:101429. doi: 10.1016/j.smim.2020.101429
Shaw, C. A., Ciarlet, M., Cooper, B. W., Dionigi, L., Keith, P., O’Brien, K. B., et al. (2013a). The path to an RSV vaccine. Curr. Opin. Virol. 3, 332–342.
Shaw, C. A., Galarneau, J. R., Bowenkamp, K. E., Swanson, K. A., Palmer, G. A., Palladino, G., et al. (2013b). The role of non-viral antigens in the cotton rat model of respiratory syncytial virus vaccine-enhanced disease. Vaccine 31, 306–312.
Shi, T., Mcallister, D. A., O’brien, K. L., Simoes, E. A. F., Madhi, S. A., Gessner, B. D., et al. (2017). Global, regional, and national disease burden estimates of acute lower respiratory infections due to respiratory syncytial virus in young children in 2015: a systematic review and modelling study. Lancet 390, 946–958. doi: 10.1016/S0140-6736(17)30938-8
Shimojima, M., Miyazawa, T., Ikeda, Y., McMonagle, E. L., Haining, H., Akashi, H., et al. (2004). Use of CD134 as a primary receptor by the feline immunodeficiency virus. Science 303, 1192–1195. doi: 10.1126/science.1092124
Shiver, J. W., Fu, T. M., Chen, L., Casimiro, D. R., Davies, M. E., Evans, R. K., et al. (2002). Replication-incompetent adenoviral vaccine vector elicits effective anti-immunodeficiency-virus immunity. Nature 415, 331–335. doi: 10.1038/415331a
Shukla, R., Ramasamy, V., Shanmugam, R. K., Ahuja, R., and Khanna, N. (2020). Antibody-Dependent Enhancement: A Challenge for Developing a Safe Dengue Vaccine. Front. Cell Infect. Microbiol. 10:572681. doi: 10.3389/fcimb.2020.572681
Siebelink, K. H. J., Tijhaar, E., Huisman, R. C., Huisman, W., De Ronde, A., Darby, I. H., et al. (1995). Enhancement of feline immunodeficiency virus infection after immunization with envelope glycoprotein subunit vaccines. J. Virol. 69:3704. doi: 10.1128/jvi.69.6.3704-3711.1995
Smatti, M. K., Al Thani, A. A., and Yassine, H. M. (2018). Viral-induced enhanced disease illness. Front. Microbiol. 9:2991. doi: 10.3389/fmicb.2018.02991
Sridhar, S., Luedtke, A., Langevin, E., Zhu, M., Bonaparte, M., Machabert, T., et al. (2018). Effect of dengue serostatus on dengue vaccine safety and efficacy. N. Engl. J. Med. 379, 327–340. doi: 10.1056/NEJMoa1800820
Srikiatkhachorn, A., and Braciale, T. J. (1997). Virus-specific CD8+ T Lymphocytes Downregulate T Helper Cell Type 2 Cytokine Secretion and Pulmonary Eosinophilia during Experimental Murine Respiratory Syncytial Virus Infection. J. Exp. Med. 186, 421–432. doi: 10.1084/jem.186.3.421
Staples, J. E., Barrett, A. D. T., Wilder-Smith, A., and Hombach, J. (2020). Review of data and knowledge gaps regarding yellow fever vaccine-induced immunity and duration of protection. NPJ Vaccines 5, 1–7. doi: 10.1038/s41541-020-0205-6
Staprans, S. I., Barry, A. P., Silvestri, G., Safrit, J. T., Kozyr, N., Sumpter, B., et al. (2004). Enhanced SIV replication and accelerated progression to AIDS in macaques primed to mount a CD4 T cell response to the SIV envelope protein. Proc. Natl. Acad. Sci. U.S.A. 101, 13026–13031. doi: 10.1073/pnas.0404739101
Su, Y.-C., Townsend, D., Herrero, L. J., Zaid, A., Rolph, M. S., Gahan, M. E., et al. (2015). Dual Proinflammatory and Antiviral Properties of Pulmonary Eosinophils in Respiratory Syncytial Virus Vaccine-Enhanced Disease. J. Virol. 89, 1564–1578. doi: 10.1128/JVI.01536-14
Suthar, A. B., Wang, J., Seffren, V., Wiegand, R. E., Griffing, S., and Zell, E. (2022). Public health impact of covid-19 vaccines in the US: observational study. BMJ 377:e069317. doi: 10.1136/bmj-2021-069317
Suzuki, M., Maghni, K., Molet, S., Shimbara, A., Hamid, Q. A., and Martin, J. G. (2002). IFN-γ secretion by CD8+T cells inhibits allergen-induced airway eosinophilia but not late airway responses. J. Allergy Clin. Immunol. 109, 803–809. doi: 10.1067/mai.2002.123233
Takada, A., Ebihara, H., Feldmann, H., Geisbert, T. W., and Kawaoka, Y. (2007). Epitopes Required for Antibody-Dependent Enhancement of Ebola Virus Infection. J. Infect. Dis. 196, S347–S356. doi: 10.1086/520581
Tan, C.-W., Chia, W.-N., Young, B. E., Zhu, F., Lim, B.-L., Sia, W.-R., et al. (2021). Pan-sarbecovirus neutralizing antibodies in BNT162b2-Immunized SARS-CoV-1 Survivors. N. Engl. J. Med. 385, 1401–1406. doi: 10.1056/NEJMoa2108453
Tay, M. Z., Wiehe, K., and Pollara, J. (2019). Antibody dependent cellular phagocytosis in antiviral immune responses. Front. Immunol. 10:332. doi: 10.3389/fimmu.2019.00332
Taylor, A., Foo, S.-S., Bruzzone, R., Vu Dinh, L., King, N. J. C., and Mahalingam, S. (2015). Fc receptors in antibody-dependent enhancement of viral infections. Immunol. Rev. 268, 340–364. doi: 10.1111/imr.12367
Thomas, S. J., Moreira, Kitchin, N., Absalon, J., Gurtman, A., Lockhart, S., et al. (2021). Safety and Efficacy of the BNT162b2 mRNA Covid-19 Vaccine through 6 Months. N. Engl. J. Med. 385, 1761–1773. doi: 10.1056/NEJMoa2110345
Tian, J. H., Patel, N., Haupt, R., Zhou, H., Weston, S., Hammond, H., et al. (2021). SARS-CoV-2 spike glycoprotein vaccine candidate NVX-CoV2373 immunogenicity in baboons and protection in mice. Nat. Commun. 12:372. doi: 10.1038/s41467-020-20653-8
Tirado, S. M. C., and Yoon, K. J. (2003). Antibody-dependent enhancement of virus infection and disease. Viral Immunol. 16, 69–86. doi: 10.1089/088282403763635465
Tseng, C.-T., Sbrana, E., Iwata-Yoshikawa, N., Newman, P. C., Garron, T., Atmar, R. L., et al. (2012). Immunization with SARS coronavirus vaccines leads to pulmonary immunopathology on challenge with the SARS Virus. PLoS One. 7:e35421. doi: 10.1371/journal.pone.0035421
Tseng, C.-T. K., Perrone, L. A., Zhu, H., Makino, S., and Peters, C. J. (2005). Severe acute respiratory syndrome and the innate immune responses: modulation of effector cell function without Productive Infection. J. Immunol. 174, 7977–7985. doi: 10.4049/jimmunol.174.12.7977
Überla, K. (2008). HIV Vaccine development in the aftermath of the STEP study: re-focus on occult HIV Infection? PLoS Pathog. 4:e1000114. doi: 10.1371/journal.ppat.1000114
Ubol, S., and Halstead, S. B. (2010). How innate immune mechanisms contribute to antibody-enhanced viral infections. Clin. Vaccine Immunol. 17, 1829–1835. doi: 10.1128/CVI.00316-10
Van Erp, E. A., Luytjes, W., Ferwerda, G., and Van Kasteren, P. B. (2019). Fc-Mediated antibody effector functions during respiratory syncytial virus infection and disease. Front. Immunol. 10:548. doi: 10.3389/fimmu.2019.00548
Van Tilbeurgh, M., Lemdani, K., Beignon, A. S., Chapon, C., Tchitchek, N., Cheraitia, L., et al. (2021). Predictive markers of immunogenicity and efficacy for human vaccines. Vaccines 9:579. doi: 10.3390/vaccines9060579
Vaughn, D. W., Green, S., Kalayanarooj, S., Innis, B. L., Nimmannitya, S., Suntayakorn, S., et al. (2000). Dengue viremia titer, antibody response pattern, and virus serotype correlate with disease severity. J. Infect. Dis. 181, 2–9. doi: 10.1086/315215
Veiga-Fernandes, H., Walter, U., Bourgeois, C., McLean, A., and Rocha, B. (2000). Response of naïve and memory CD8+ T cells to antigen stimulation in vivo. Nat. Immunol. 1, 47–53. doi: 10.1038/76907
Villar, L., Dayan, G. H., Arredondo-García, J. L., Rivera, D. M., Cunha, R., Deseda, C., et al. (2015). Efficacy of a tetravalent dengue vaccine in children in Latin America. N. Engl. J. Med. 372, 113–123. doi: 10.1056/NEJMoa1411037
Wan, S. W., Wu-Hsieh, B. A., Lin, Y. S., Chen, W. Y., Huang, Y., and Anderson, R. (2018). The monocyte-macrophage-mast cell axis in dengue pathogenesis. J. Biomed. Sci. 25:77. doi: 10.1186/s12929-018-0482-9
Wan, Y., Shang, J., Sun, S., Tai, W., Chen, J., Geng, Q., et al. (2020). Molecular mechanism for antibody-dependent enhancement of coronavirus entry. J. Virol. 94, 2015–2034. doi: 10.1128/JVI.02015-19
Wang, C., Xie, J., Zhao, L., Fei, X., Zhang, H., Tan, Y., et al. (2020). Alveolar macrophage dysfunction and cytokine storm in the pathogenesis of two severe COVID-19 patients. EBioMedicine 57:102833. 10.1016/j.ebiom.2020.102833 doi: 10.1016/j.ebiom.2020.102833
Wang, L., Shi, W., Joyce, M. G., Modjarrad, K., Zhang, Y., Leung, K., et al. (2015). Evaluation of candidate vaccine approaches for MERS-CoV. Nat. Commun. 6:7712. doi: 10.1038/ncomms8712
Wang, Q., Zhang, L., Kuwahara, K., Li, L., Liu, Z., Li, T., et al. (2016). Immunodominant SARS coronavirus epitopes in humans elicited both enhancing and neutralizing effects on infection in non-human primates. ACS Infect. Dis. 2, 361–376. doi: 10.1021/acsinfecdis.6b00006
Wang, S. F., Tseng, S. P., Yen, C. H., Yang, J. Y., Tsao, C. H., Shen, C. W., et al. (2014). Antibody-dependent SARS coronavirus infection is mediated by antibodies against spike proteins. Biochem. Biophys. Res. Commun. 451, 208–214. 10.1016/j.bbrc.2014.07.090 doi: 10.1016/j.bbrc.2014.07.090
Waris, M. E., Tsou, C., Erdman, D. D., Zaki, S. R., and Anderson, L. J. (1996). Respiratory synctial virus infection in BALB/c mice previously immunized with formalin-inactivated virus induces enhanced pulmonary inflammatory response with a predominant Th2-like cytokine pattern. J. Virol. 70, 2852–2860. doi: 10.1128/jvi.70.5.2852-2860.1996
Werzberger, A., Mensch, B., Kuter, B., Brown, L., Lewis, J., Sitrin, R., et al. (1992). A controlled Trial of a formalin-inactivated hepatitis a vaccine in healthy children. N. Engl. J. Med. 327, 453–457. doi: 10.1056/NEJM199208133270702
WHO (2002). Summary of probable SARS cases with onset of illness from 1 November 2002. Geneva: World Health Organisation.
WHO (2017). Expert Committee on Biological Standardization Sixty-seventh report. Geneva: World Health Organisation.
WHO (2020). Draft landscape and tracker of COVID-19 candidate vaccines. Geneva: World Health Organisation.
Wieten, R. W., Jonker, E. F. F., van Leeuwen, E. M. M., Remmerswaal, E. B. M., Ten Berge, I. J. M., de Visser, A. W., et al. (2016). A Single 17D Yellow Fever Vaccination Provides Lifelong Immunity; Characterization of Yellow-Fever-Specific Neutralizing Antibody and T-Cell Responses after Vaccination. PLoS One 11:e0149871. doi: 10.1371/journal.pone.0149871
Wilen, C. B., Tilton, J. C., and Doms, R. W. (2012). Molecular Mechanisms of HIV Entry. Adv. Exp. Med. Biol. 726, 223–242. doi: 10.1007/978-1-4614-0980-9_10
Wiles, S., Hanage, W. P., Frankel, G., and Robertson, B. (2006). Modelling infectious disease - time to think outside the box? Nat. Rev. Microbiol. 4, 307–312.
Willett, B. J., Mcmonagle, E. L., and Hosie, M. (2006). Differential Utilization of CD134 as a Functional Receptor by Diverse Strains of Feline Immunodeficiency Virus Create new project “Duration of antibody response following vaccination against feline immunodeficiency virus”. J. Virol. 80, 3386–3394. doi: 10.1128/JVI.80.7.3386-3394.2006
Wilson, N. A., Reed, J., Napoe, G. S., Piaskowski, S., Szymanski, A., Furlott, J., et al. (2006). Vaccine-induced cellular immune responses reduce plasma viral concentrations after repeated low-dose challenge with pathogenic simian immunodeficiency virus SIVmac239. J. Virol. 80, 5875–5885. doi: 10.1128/JVI.00171-06
Wolz, O.-O., Kays, S.-K., Junker, H., Koch, S. D., Mann, P., Quintini, G., et al. (2022). A Third Dose of the COVID-19 Vaccine, CVnCoV, Increased the Neutralizing Activity against the SARS-CoV-2 Wild-Type and Delta Variant. Vaccines 10:508. doi: 10.3390/vaccines10040508
Worldometer (2022). COVID Live Update: 254,743,840 Cases and 5,125,779 Deaths from the Coronavirus. Geneva: World Health Organisation.
Xie, T., Lu, S., He, Z., Liu, H., Wang, J., Tang, C., et al. (2022). Three doses of prototypic SARS-CoV-2 inactivated vaccine induce cross-protection against its variants of concern. Signal Transduct. Target Ther. 7, doi: 10.1038/s41392-022-00920-4
Xu, X., and Gao, X.-M. (2004). Immunological Responses against SARS-Coronavirus Infection in Humans. Cell. Mol. Immunol. 1, 119–122.
Xu, Z., Shi, L., Wang, Y., Zhang, J., Huang, L., Zhang, C., et al. (2020). Pathological findings of COVID-19 associated with acute respiratory distress syndrome. Lancet Respir. Med. 8, 420–422. doi: 10.1016/S2213-2600(20)30076-X
Yang, D., Chu, H., Hou, Y., Chai, Y., Shuai, H., Lee, A. C. Y., et al. (2020). Attenuated Interferon and Proinflammatory Response in SARS-CoV-2–Infected Human Dendritic Cells Is Associated With Viral Antagonism of STAT1 Phosphorylation. J. Infect. Dis. 222, 734–745. doi: 10.1093/infdis/jiaa356
Yang, J., Wang, W., Chen, Z., Lu, S., Yang, F., Bi, Z., et al. (2020). A vaccine targeting the RBD of the S protein of SARS-CoV-2 induces protective immunity. Nature 586, 572–577. doi: 10.1038/s41586-020-2599-8
Yasui, F., Kohara, M., Kitabatake, M., Nishiwaki, T., Fujii, H., Tateno, C., et al. (2014). Phagocytic cells contribute to the antibody-mediated elimination of pulmonary-infected SARS coronavirus. Virology 454–455, 157–168. doi: 10.1016/j.virol.2014.02.005
Yilla, M., Harcourt, B. H., Hickman, C. J., McGrew, M., Tamin, A., Goldsmith, C. S., et al. (2005). SARS-coronavirus replication in human peripheral monocytes/macrophages. Virus Res. 107, 93–101. doi: 10.1016/j.virusres.2004.09.004
Yip, M. S., Leung, N. H. L., Cheung, C. Y., Li, P. H., Lee, H. H. Y., Daëron, M., et al. (2014). Antibody-dependent infection of human macrophages by severe acute respiratory syndrome coronavirus. Virol. J. 11, doi: 10.1186/1743-422X-11-82
Yu, J., Tostanosk, L. H., Peter, L., Mercad, N. B., McMahan, K., Mahrokhia, S. H., et al. (2020). DNA vaccine protection against SARS-CoV-2 in rhesus macaques. Science 369, 806–811. doi: 10.1126/science.abc6284
Zhang, L., Zhang, F., Yu, W., He, T., Yu, J., Yi, C. E., et al. (2006). Antibody responses against SARS coronavirus are correlated with disease outcome of infected individuals. J. Med. Virol. 78, 1–8. doi: 10.1002/jmv.20499
Zhao, J., Yuan, Q., Wang, H., Liu, W., Liao, X., Su, Y., et al. (2020). Antibody Responses to SARS-CoV-2 in Patients with Novel Coronavirus Disease 2019. Clin. Infect. Dis. 71, 2027–2034. doi: 10.1093/cid/ciaa344
Zheng, J., Wang, Y., Li, K., Meyerholz, D. K., Allamargot, C., and Perlman, S. (2021). Severe Acute Respiratory Syndrome Coronavirus 2-Induced Immune Activation and Death of Monocyte-Derived Human Macrophages and Dendritic Cells. J. Infect. Dis. 223, 785–795. doi: 10.1093/infdis/jiaa753
Zhou, J., Wang, W., Zhong, Q., Hou, W., Yang, Z., Xiao, S. Y., et al. (2005). Immunogenicity, safety, and protective efficacy of an inactivated SARS-associated coronavirus vaccine in rhesus monkeys. Vaccine 23, 3202–3209. doi: 10.1016/j.vaccine.2004.11.075
Keywords: vaccine, vaccine-associated enhanced disease, immunology, infectious diseases, immune response
Citation: Bigay J, Le Grand R, Martinon F and Maisonnasse P (2022) Vaccine-associated enhanced disease in humans and animal models: Lessons and challenges for vaccine development. Front. Microbiol. 13:932408. doi: 10.3389/fmicb.2022.932408
Received: 29 April 2022; Accepted: 19 July 2022;
Published: 10 August 2022.
Edited by:
Axel Cloeckaert, Institut National de Recherche pour l’Agriculture, l’Alimentation et l’Environnement (INRAE), FranceReviewed by:
Miles Carroll, University of Oxford, United KingdomCopyright © 2022 Bigay, Le Grand, Martinon and Maisonnasse. This is an open-access article distributed under the terms of the Creative Commons Attribution License (CC BY). The use, distribution or reproduction in other forums is permitted, provided the original author(s) and the copyright owner(s) are credited and that the original publication in this journal is cited, in accordance with accepted academic practice. No use, distribution or reproduction is permitted which does not comply with these terms.
*Correspondence: Frédéric Martinon, ZnJlZGVyaWMubWFydGlub25AY2VhLmZy
Disclaimer: All claims expressed in this article are solely those of the authors and do not necessarily represent those of their affiliated organizations, or those of the publisher, the editors and the reviewers. Any product that may be evaluated in this article or claim that may be made by its manufacturer is not guaranteed or endorsed by the publisher.
Research integrity at Frontiers
Learn more about the work of our research integrity team to safeguard the quality of each article we publish.