- 1College of Resources and Environmental Sciences, Henan Agricultural University, Zhengzhou, China
- 2College of Life Sciences, Henan Agricultural University, Zhengzhou, China
Vertisols are clayey soils with a high potential for improving production. Therefore, understanding the impact of tillage and fertilization on soil physicochemical properties and microbial community is essential for improving the vertisols with a high montmorillonite and smectite clay content. A 3-year field experiment was conducted to compare the effects of different tillage and fertilization practices at three depths of the vertisol under the wheat–maize cropping system in the North China Plain. The experimental treatments included rotary tillage without fertilization (R-CK), rotary tillage with chemical nitrogen (N), phosphorus (P), and potassium (K) fertilization (R-NPK), R-NPK plus biochar (R-NPKB), deep tillage without fertilization (D-CK), deep tillage with chemical N, P, and K fertilization (D-NPK), and D-NPK plus biochar (D-NPKB). The results showed that D-NPKB significantly improved winter wheat and summer maize yields by 14.4 and 3.8%, respectively, compared with R-NPK. The nitrate (NO3––N) content of the deeper soil layer in D-NPKB was significantly higher than that in D-NPK. Meanwhile, biochar application increased the pH in the three layers. Compared with R-NPK, D-NPKB significantly increased the average content of available phosphorus (AP), soil organic carbon (SOC), and total nitrogen (TN) by 73.7, 18.5, and 19.0%, respectively. Meanwhile, Gaiellale, Sphingomonadaceae, and Nocardioidaceae were the predominant bacteria at the family level across all treatments, with a total relative proportion ranging from 14.1 to 23.6%. In addition, the abundance of Bacillaceae in deep tillage was 9.4% higher in the 20–30-cm soil layer than that in rotary tillage. Furthermore, the correlation analysis revealed a significant positive correlation between crop yield and chemical factors such as NO3––N and the abundances of Gaiellalea, Sphingomonadaceae, and Nocardioidaceae. The findings collectively indicated that deep tillage combined with biochar application could increase the soil nutrients and modify the bacterial structure in the deeper soil layer and therefore will be beneficial for improving the productivity of the vertisols.
Introduction
A vertisol is a heavy clay soil dominated by smectite minerals, especially montmorillonite (Wu et al., 2015); it is characterized by high soil bulk density and strength, low water permeability, and significant shrinkage and swelling capacity (Novelli et al., 2020; Xiong et al., 2020). It is the most widely distributed and important agricultural soil, mainly in Australia, India, Africa, and China (Ahmad, 1996). In China, the vertisol, located in the southern part of the North China Plain with approximately 4 million hectares, is one of the main soil types for low crop production. Additionally, long-term rotary tillage further leads to a shallow plowing layer and a thick plow pan, which restricts crop growth and yield in this soil (Wang et al., 2021). The vertisols have a high productivity potential due to a high montmorillonite and smectite clay fraction (DeCarlo and Caylor, 2020). And, therefore we need to explore a measure to overcome the physical barriers and nutrition limits in deeper soil, so as to improve crop yield and help in sustainable soil development. Therefore, it is necessary to improve the physical structure and chemical properties of the vertisols.
Tillage is a widely recognized effective practice for improving soil physical quality and crop yield (Yu et al., 2020; Wang et al., 2021). Previous studies have shown that reasonable tillage methods, such as subsoiling, deep plowing, and deep mixing of soil profile combined with rotary cultivation, improve soil fertility and slow down soil degradation and are conducive to a virtuous cycle of farmland ecosystems and efficiency (Aziz et al., 2013; Wang et al., 2020; Lopes et al., 2021). Moreover, mechanical soil profile modifications, commonly called deep tillage, can alleviate subsoil strength and facilitate deeper plant rooting (Schneider et al., 2017). Deep tillage could effectively lower the soil bulk density and improve crop yield and soil properties, especially the soil organic carbon (SOC) content, in the agricultural fields (Feng et al., 2020; Hu et al., 2021; Wang et al., 2021). Biochar is an important soil amendment, with a large specific surface area, well-developed pore structure, strong adsorption capacity, and high nutrient content, and is effective in increasing soil pH in acid soils (Obia et al., 2015; Hussain et al., 2020; Pan et al., 2021). Recent studies have shown that biochar application significantly improved soil fertility and changed the soil microbial structure and diversity (Liu et al., 2017; Xu et al., 2020).
Tillage and biochar play an important role in agricultural production. These methods directly affect the soil microbes via the input of nutrients and indirectly by altering the soil properties (Abujabhah et al., 2016; Chen et al., 2021). Recent studies have suggested that deep tillage and biochar application significantly reduced the bulk density of the vertisols and increased the soil air capacity in the topsoil layer and the content of SOC and available phosphorus (AP), subsequently improving crop yield (Fang et al., 2019; Liu et al., 2021; Wang et al., 2021). Therefore, deep tillage combined with biochar application can be a potential measure to overcome the shortcomings of shallow plowing layers and poor nutrient supply, thereby improving clay vertisols. These studies on improving clay soils with poor soil structure and shallow plowing layers mostly focused on improving the mixed soil layer (Chen et al., 2020; Lopes et al., 2021; Wang et al., 2021). However, the comparison study of physicochemical and microbial properties of different soil layers along the depth of different tillage systems has not been characterized. Besides, knowledge on improving the soil physicochemical properties and bacterial community of the separated soil layers is poor.
In this study, a consecutive field comparative experiment was conducted with contrasting tillage and fertilization practices carried out on a vertisol soil of Southern Henan Province, North China. This study aimed to investigate the effects of tillage and biochar on the soil physicochemical properties, microbial structure along various soil depths, and Spearman’s correlations among crop yield, soil physicochemical properties, and the bacterial community structure. We hypothesized that deep tillage combined with biochar application could improve soil physicochemical properties and microbial diversity of the deeper soil layer, subsequently enhancing crop yield. The study will provide a valuable theoretical basis for planning fertilization and tillage practices for the sustainable development of the vertisols.
Study area
The experiment was conducted in a field in Baimiao Village, Xiping County, Henan Province, China (33°25′39″N, 113°49′23″E). The experimental area had a humid continental monsoon climate with four distinct seasons, with a mean annual precipitation of 852 mm, an air temperature of 14.8°C, and an average yearly frost-free period of 221 days. The primary cropping system of this area included summer maize (June–October) and winter wheat (October–next June). The soil type of the experimental area was classified as vertisol (FAO-UNESCO-ISRIC, 1988), with the contents of clay 50%, silt 37%, and sand 13% and with the following soil profile structure characteristics: A surface layer (0–20 cm) was heavy loam or light clay, with a granular structure and no calcareous nodules; a core layer (30–50 cm) was heavy soil or light sticky and a few are heavy sticky, with a fragmentary structure and a lot of ferromanganese nodules; and a bottom layer (>50 cm) was light sticky or heavy clay, with a block structure and a lot of calcareous nodules. The soil properties (0–20 cm) at the start of the experiment were as follows: pH 5.78; SOC 10.81 g kg–1; total soil nitrogen (TN) 1.26 g kg–1; AP 19.46 mg kg–1; and available potassium (AK) 171.6 mg kg–1. In this region, the field had been mainly cultivated by rotary tillage with 20 cm depth, which was by a four-wheel tractor with a hydraulic system.
Materials and methods
Experimental design
The tillage and fertilization trial was established in 2018 using a split-plot design with six blocks, including tillage as the main plot and fertilization as the split plot. Six treatments were carried out in the experimental plots as follows: (1) rotary tillage without fertilization (R-CK); (2) rotary tillage with chemical N, P, and K fertilization (R-NPK); (3) R-NPK plus biochar (R-NPKB); (4) deep tillage without fertilization (D-CK), (5) deep tillage with chemical N, P, and K fertilization (D-NPK); and (6) D-NPK plus biochar (D-NPKB). Each treatment was designed with three replicates; the area of each plot was 36 m2 (6 m × 6 m), with neighboring plots separated by a buffer strip of about 1 m. The local fertilization, with pure N, 180 kg hm–2, P2O5, 90 kg hm–2, and K2O, 90 kg hm–2 per season, was carried out in all treatments, except R-CK and D-CK. The P and K fertilizers were applied at the time of sowing as basal dressing, while 60% of N was applied as basal fertilizer and the remaining 40% as topdressing at the wheat jointing period and the maize big trumpet period, respectively. Maize straw biochar with a C/N ratio of 58.0 and a pH of 8.3 was obtained via pyrolysis at 500°C under anaerobic conditions from Hubei Jinri Eco-Energy Co., Ltd. (Anlu City, China). The biochar was applied at a 7500 kg ha–1 rate into the soil by an adjustable rotary tiller at 0–15 cm depth and 0–30 cm depth before sowing winter wheat. In the tillage system, a four-wheel tractor was used to pull the rotary tiller, with the operating depth adjusted using the tractor hydraulic system. And the burial depths of the rotary and deep tillage were 20 and 30 cm, respectively. A C-type blade attached to the rotary tiller destructed the deeper soil structure, especially in the deep tillage system; it broke the obstacles and promoted the intensive mixing of subsoil and topsoil. Moreover, the two wheels at the back of the rotary tiller were used to maintain the same operating depth. Following the local tillage regimes, summer maize seeds were directly sown after wheat harvest without any tillage.
Grain yield and soil sampling
We collected three winter wheat plots of 1 m2 per treatment to determine the wheat grain yield under different treatments. Meanwhile, to determine the summer maize yield, we harvested 18 plants from the middle rows and acquired the seeds by a corn thresher, weighed the seeds after drying at 75°C, and calculated the yield for the total plants per hectare.
Soil samples were collected from three soil layers (0–10, 10–20, and 20–30 cm) from three replicate plots of each treatment after wheat and maize harvest in 2019 and 2020 and at the maize filling stage in 2020. Five soil samples were randomly collected from each plot, placed in labeled plastic bags, and immediately taken to the laboratory for further processing. In total, 252 samples from five periods and three soil layers were collected for physicochemical property analysis and 45 samples from three soil layers at the maize filling stage in 2020 for bacterial community analysis. The soil samples were sieved through a 2-mm sieve and divided into two subsamples: They were stored at 4°C for NH4+/NO3––N (Li et al., 2020) and air-dried for chemical analysis. In particular, we additionally separated a part of soil samples from three soil layers collected at the maize filling stage in 2020 and stored at −80°C for soil microbial and molecular analysis.
Soil physicochemical properties
The soil moisture was measured by oven-drying the samples at 105°C to a constant mass. The soil ammonium nitrogen (NH4+–N) and nitrate (NO3––N) were extracted from the moist field soil stored at 4°C with 2 M KCL and estimated with a flow injection analyzer (SAN++, Sklar, Promega, Netherlands). The air-dried soil samples were sieved through a 100-mesh sieve and used to analyze the TN and SOC. The soil pH was determined at a soil-to-water ratio of 1:2.5 using a pH meter (Sartorius, PB-10), and SOC was determined following the classical potassium dichromate oxidation–ferrous sulfate titration method (Nelson and Sommers, 1982). Meanwhile, the soil samples were boiled with concentrated hydrogen peroxide and sulfuric acid, and the TN was determined by the Kjeldahl method (Kjeldahl, 1883). The AP was extracted using 0.5 M NaHCO3 and measured using the Olsen method (Olsen et al., 1954). The soil AK was extracted using 1 M NH4OAc and analyzed by flame emission spectrometry (Bao, 2000).
DNA extraction and PCR amplification
Microbial genomic DNA was extracted from the soil samples using the FastDNA® Spin Kit for Soil (MP Biomedicals, Santa Ana, CA, USA) according to the manufacturer’s instructions. The DNA extract was assessed on 1% agarose gel, and the DNA concentration and purity were determined with a NanoDrop 2000 UV–Vis spectrophotometer (Thermo Scientific, Wilmington, NC, USA). The hypervariable regions V3–V4 of the bacterial 16S rRNA gene were amplified using the primer pairs 338F (5′-ACTCCTACGGGAGGCAGCAG-3′) and 806R (5′-GGACTACHVGGGTWTCTAAT-3′) (Xu et al., 2016) on an ABI GeneAmp® 9700 PCR thermocycler (ABI, Los Angeles, CA, USA). The PCR amplification of the 16S rRNA gene was performed as follows: initial denaturation at 95°C for 3 min, followed by 27 cycles of denaturing at 95°C for 30 s, annealing at 55°C for 30 s, and extension at 72°C for 45 s, and final extension at 72°C for 10 min. The PCR mixture contained 4 μL of 5 × TransStart FastPfu buffer, 2 μL of 2.5 mM dNTPs, 0.8 μL of each of the forward (5 μM) and reverse primers (5 μM), 0.4 μL of TransStart FastPfu DNA polymerase, and 10 ng of template DNA, made up to a final volume of 20 μL with ddH2O. PCR of each sample was performed in triplicate. The PCR product was purified from 2% agarose gel using the AxyPrep DNA Gel Extraction Kit (Axygen Biosciences, Union City, CA, USA) according to the manufacturer’s instructions and quantified using a Quantus™ fluorometer (Promega, USA).
Illumina MiSeq sequencing
The purified amplicons were pooled in equimolar concentrations and paired-end sequenced (2 × 300) on an Illumina MiSeq platform (Illumina, San Diego, CA, USA) according to the standard protocols by Majorbio Bio-Pharm Technology Co., Ltd. (Shanghai, China). The raw reads were deposited into the NCBI Sequence Read Archive (SRA) database.
Processing of sequencing data
The raw 16S rRNA gene sequencing reads were demultiplexed, quality-filtered by Fastp (version 0.19.6), and merged using FLASH (version 1.2.7) with the following criteria: (i) 300-bp reads were truncated at any site receiving an average quality score of <20 over a 50-bp sliding window, the truncated reads shorter than 50 bp were discarded, and the reads containing ambiguous characters were also discarded; (ii) only overlapping sequences longer than 10 bp were assembled according to their overlapped sequence. The maximum mismatch ratio of the overlap region allowed was 0.2. The reads that could not be assembled were discarded; and (iii) the samples were distinguished according to the barcode and primers, and the sequence direction was adjusted, with exact barcode matching and two nucleotide mismatches in primer matching.
Operational taxonomic units (OTUs) at a 97% similarity cutoff (Tipton et al., 2021) were clustered using UPARSE (version 7.1),1 and the chimeric sequences were identified and removed. The taxonomy of each OTU representative sequence was analyzed by RDP Classifier2 against the 16S rDNA database (Silva SSU128) using a confidence threshold of 0.7 (Wang et al., 2007).
Data analysis
Data were represented as mean ± standard error for all the assessed parameters. Statistical analysis was performed using Excel 2016 and SPSS 23.0 software (IBM Co., Armonk, NY, USA). The differences in crop yield, soil moisture, NH4+–N, NO3––N, pH, SOC, TN, AP, and AK between the different treatments at the same depth were analyzed using one-way analysis of variance (ANOVA) and Duncan’s multiple range test (DMRT) at a 5% significance level. Repeated measure analyses of variance (RMANOVA) with crop yield and soil properties (physicochemical properties and bacterial alpha diversity at the genus level) were performed to probe the effect of tillage, fertilization, and season. Interaction (tillage × fertilization, tillage × season, fertilization × season, and tillage × fertilization × season) and main effect (tillage, fertilization, and season) were tested. The vegan package in R language software (version 3.5.1) was used to conduct a redundancy analysis (RDA) based on the microbe and the soil physicochemical properties. Besides, the structural equation modeling (SEM) was performed to examine the direct and indirect effects of soil physicochemical properties on the bacterial richness and yield using IBM SPSS Statistics (version 23) and IBM SPSS Amos Graphics (version 21.0.0) (Build 1178) (P < 0.05). We selected bacteria at the family level with a mean relative abundance greater than 0.05% within each soil layer and OTUs with a relative abundance greater than 0.05% for Spearman’s correlation analysis. Then, the taxon–taxon co-existing network and the taxon–environment network were constructed to examine the association between various taxa and between taxa and the environment within the three soil layers using the R language software (version 4.1.0) with “psych” package and Gephi (version 0.9.2).
Results
Soil physicochemical analysis
Effect of tillage and biochar on crop yield
The trends of wheat and maize yields to tillage and biochar treatments for three consecutive years from 2018 to 2020 are given in Table 1. Varying degrees of differences in crop yields were observed among the different tillage and fertilization treatments. In the first crop year (2018), the winter wheat and summer maize yields in the R-NPKB treatment significantly increased by 8.5 and 9.2%, respectively, compared with those in the R-NPK treatment (P < 0.05). Meanwhile, the wheat and maize yields in the D-NPKB treatment in 2019 significantly increased by 8.4 and 8.9%, respectively, compared with those in the D-NPK treatment and by 9.9 and 26.8%, respectively, compared with those in the R-NPK treatment. The summer maize yield in the R-NPKB treatment was 10,635 kg ha–1, higher than in the R-NPK treatment in 2018. Deep tillage significantly increased the summer maize yield (5,792 kg ha–1) in the NPKB treatment compared with the yield under rotary tillage (4,538 kg ha–1) following the same fertilization in 2019. Thus, the average winter wheat and summer maize yields in the 3 years were 14.4 and 3.8%, respectively, higher in the D-NPKB treatment than those in the R-NPK treatment. In general, deep tillage combined with biochar application increased the crop yield compared with the other treatments in the 3 years, except for the summer maize in 2018. The analysis of the interaction revealed that deep tillage significantly increased the maize yield (P < 0.01), while the impact on wheat yield was significant only in 2020. Besides, fertilization and biochar application significantly improved the winter wheat and summer maize yields compared with the CK treatments (P < 0.001).
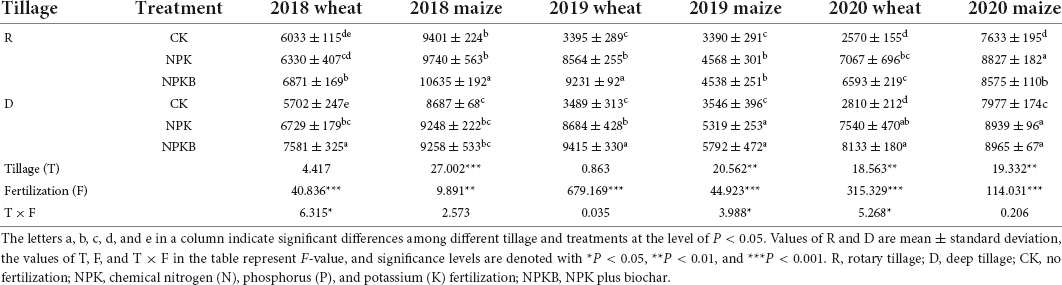
Table 1. Responses of the grain yield (kg ha–1) to different tillage and fertilization practices in the wheat–maize rotation system throughout 2018–2020 cropping years.
Effect of tillage and biochar on soil physical properties
Table 2 shows the bulk density of soil at different depths during the winter wheat and summer maize seasons in 2019 and maize in 2020. The NPK treatment significantly increased the average soil bulk density by 4.9% compared with the CK treatment, while the NPKB treatment significantly decreased by 4.2% compared with the NPK treatment in the 0–10- and 10–20-cm soil layers during the wheat and maize seasons in 2019. Meanwhile, the NPKB treatment lowered the soil bulk density (P < 0.01) in the 0–10-cm and 20–30-cm soil layers compared with the NPK treatment for most crop seasons. Under the same fertilization, deep tillage had the soil bulk density values of 1.51 g cm–3 (D-CK and D-NPKB) and 1.57 g cm–3 (D-CK) in the 10–20- and 20–30-cm soil layers for the 2019 summer maize. Besides, soil bulk density significantly increased with the treatment time in the 0–10-cm soil layer and significantly decreased in the 20–30-cm soil layer (P < 0.001) from 2019 to 2020. Interactive analysis revealed that deep tillage significantly lowered the soil bulk density in the 10–20- and 20–30-cm soil layers (P < 0.01) compared with rotary tillage, but no significant change was detected in the 0–10-cm soil layer.
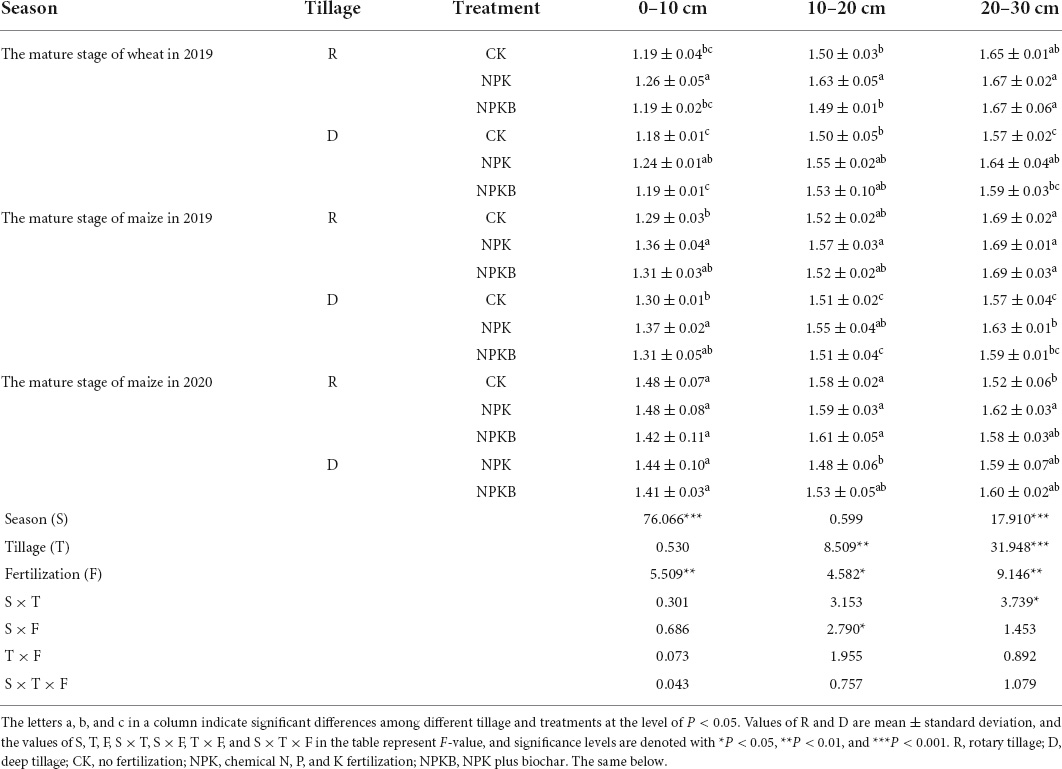
Table 2. Responses of the soil bulk density (g cm–3) to different tillage and fertilization practices in the wheat–maize rotation system throughout 2019–2020 cropping years.
The soil moisture under different treatments from 2019 to 2020 is given in Table 3. In our research, the soil moisture in the summer maize season increased from the 0–10-cm soil layer to the 20–30-cm soil layer in 2019, while it decreased along with the soil profile of each treatment in 2020. The soil moisture significantly differed among the seasons (P < 0.05), with no significant differences between treatments in 2019.
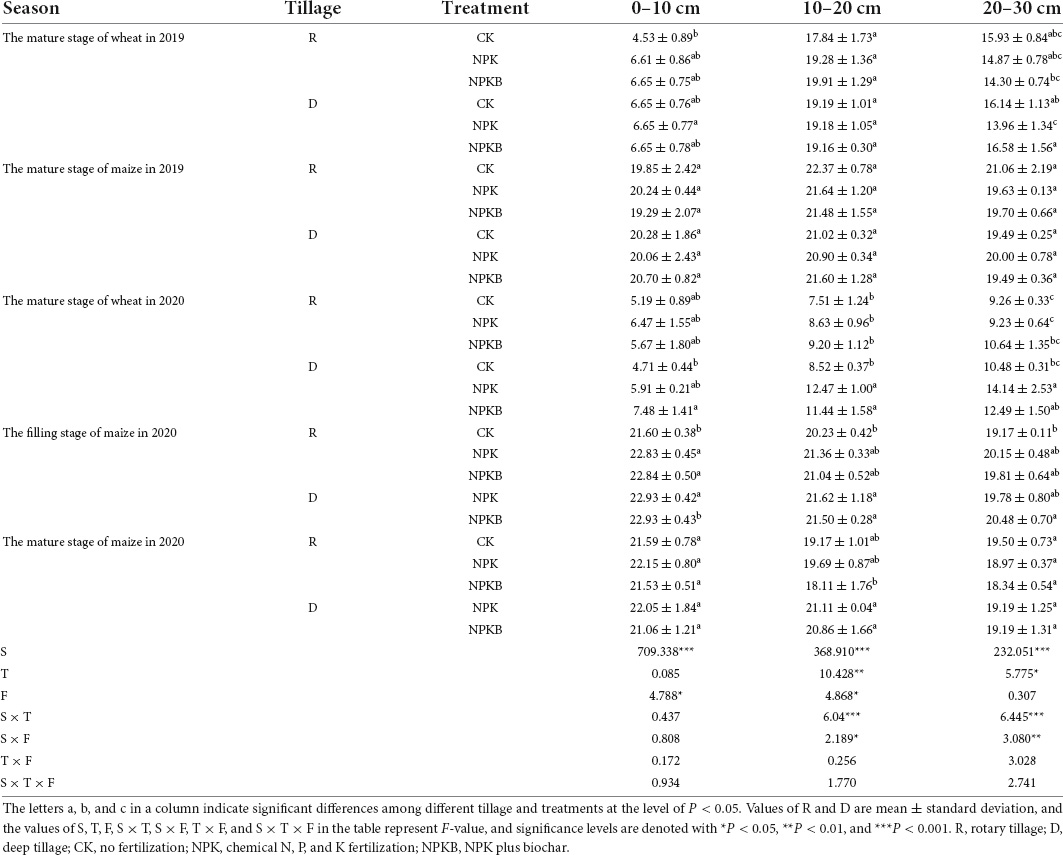
Table 3. Responses of the soil moisture content (%) to different tillage and fertilization practices in the wheat–maize rotation system in 2019–2020.
Effect of tillage and biochar on soil pH, available phosphorus, available potassium, soil organic carbon, and total nitrogen
Soil chemical properties, including pH, AP, AK, SOC, and TN, from 2019 to 2020 are listed in Supplementary Table. In the 10–20- and 20–30-cm soil layers at the winter wheat mature stage in 2019, the soil pH in the D-NPKB treatment was higher than that in the D-NPK treatment, which was 5.9 and 7.36, respectively. During the summer maize mature stage in 2019, the soil pH values ranged from 5.12 to 5.18 in the NPK and NPKB treatments under deep tillage, significantly lower than that in the CK treatment (6.19) in the topsoil layer. The soil pH in the R-NPKB treatment increased by 1.3 and 4.3% in the 10–20- and 20–30-cm soil layers, respectively, during the winter wheat season in 2020 compared with that in the R-NPK treatment. Under the same fertilization, the pH values of NPK and NPKB treatments under deep tillage were 5.58 and 5.77, respectively, which were higher than the corresponding treatments under rotary tillage (5.21 and 5.47, respectively) in the 0–10-cm soil layer.
During the mature stages of wheat and maize in 2019–2020, the AP, AK, SOC, and TN in the NPKB treatment increased to varying degrees compared with those in the NPK treatment in the 10–20- and 20–30-cm soil layers. The NPKB treatment significantly increased the average content of soil AP, AK, SOC, and TN in the three soil layers at the summer maize mature stage in 2020 by 9.7, 11.4, 11.7, and 3.9%, respectively, compared with the NPK treatment. The soil SOC levels in the NPKB treatment were 13.78 and 14.16 g kg–1 under rotary and deep tillage, respectively, which were higher than those in the NPK treatment (12.09 and 12.38 g kg–1) in the 10–20-cm soil layer at the mature maize stage in 2020. Under the same fertilization and season, deep tillage increased soil AP, TN, AK, and SOC content in the 20–30-cm soil layer for most crop seasons, and significant changes were detected in AP and TN content in both wheat and maize seasons in 2019 (P < 0.05). The D-NPKB treatment significantly increased the average content of AP, SOC, and TN in the 20–30-cm soil layer by 73.7, 18.5, and 19.0%, respectively, compared with the R-NPK treatment in the 2 years.
The interactive analysis revealed season and fertilization as the significant factors affecting the soil pH, AP, SOC, and TN. Fertilization combined with biochar application increased the soil AK, SOC, and TN content in the 20–30-cm soil layer.
Effect of tillage and biochar on soil NH4+–N and NO3––N
The values of the soil NH4+–N and NO3––N contents in each soil layer during the summer maize filling and mature stages in 2020 are given in Table 4. No significant difference was detected in the soil NH4+–N at the same stage among the treatments (P > 0.05). The soil NO3––N content in the 0–30-cm soil layer decreased along with the soil profile of each treatment. The soil NO3––N content in the NPK and NPKB treatments was significantly higher than that in the CK treatment in the 0–10-cm soil layer. In the summer maize filling stage, the soil NO3––N content in the D-NPKB treatment was significantly higher than that in the D-NPK treatment in the 10–20-cm soil layer (P < 0.05). The soil NO3––N content in the D-NPKB treatment was 21.05 mg kg–1, significantly higher than that in the D-NPK treatment (14.11 mg kg–1) in the 10–20-cm soil layer. In the summer maize mature stage, the NO3––N content in the NPK and NPKB treatments under deep tillage increased by 21.9 and 76.3%, respectively, compared with the corresponding treatments under rotary tillage in the 10–20-cm soil layer. The interactive analysis showed that soil NH4+–N and NO3––N contents changed with the growth of summer maize. The soil NH4+–N content in the mature stage was significantly lower (P < 0.001) than that in the maize filling stage in each soil layer, while the soil NO3––N content showed an opposite trend (P < 0.05) in the 0–10- and 10–20-cm soil layers. The D-NPKB treatment significantly increased the soil NO3––N content by 49.2 and 41.9% in the 10–20- and 20–30-cm soil layers, respectively, compared with the D-NPK treatment in the summer maize filling stage.
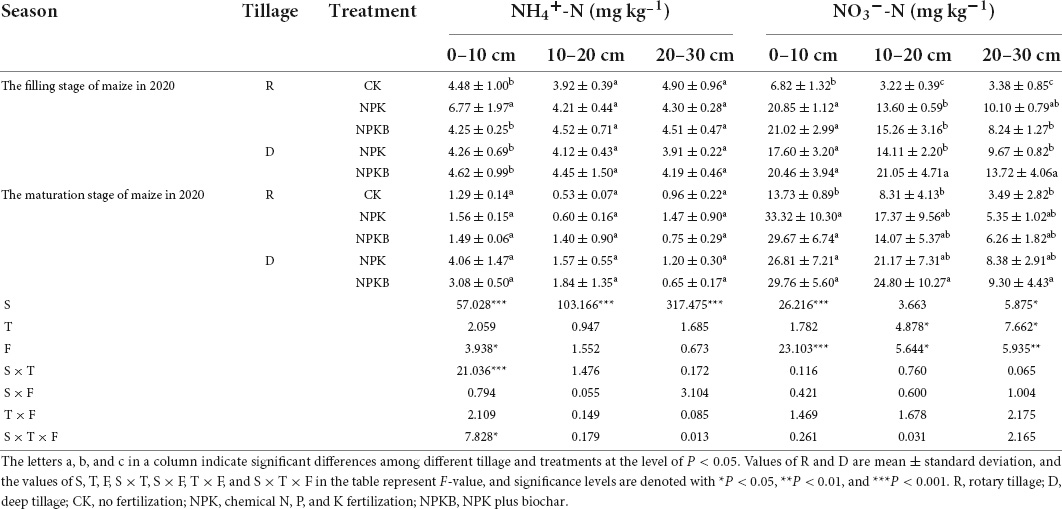
Table 4. Responses of the contents of NH4+–N and NO3––N (mg kg–1) to different tillage and fertilization practices in the wheat–maize rotation system during the maize stages in 2020.
Composition of the microbial community at different soil depths
The top 10 bacterial phyla and 15 bacterial families with a relative abundance were selected to analyze the differences in the microbial composition under different treatments and at different soil layers (Figure 1). At the phylum level, the dominant bacterial phyla were Actinobacteria, Proteobacteria, Chloroflexi, and Acidobacteria, and the total relative abundance accounted for 79.6–84.5% (Figure 1A). The abundance of Methylomirabilota in the 20–30-cm soil layer was the highest in the three soil layers. The families Gaiellale, Sphingomonadaceae, and Nocardioidaceae were predominant under different treatments and at different soil layers with a total relative proportion ranging from 14.1 to 23.6% (Figure 1B). The Sphingomonadaceae abundance decreased with the increasing soil depth under each treatment, and the highest abundance in the 10–20- and 20–30-cm soil layers was under the D-NPKB treatment (Figure 2). The Micrococcaceae abundance under deep tillage increased by 25.8 and 17.7% in the 0–10- and 10–20-cm soil layers, respectively, compared with that under rotary tillage except for the R-CK treatment; however, the abundance lowered by 10.3% in the 20–30-cm soil layer. In the 0–10- and 10–20-cm soil layers, the Bacillaceae abundance under rotary tillage was higher than that under deep tillage except for the R-CK treatment, whereas an opposite trend was observed in the 20–30-cm soil layer. The Bacillaceae abundance in the 20–30-cm soil layer improved by 9.4% under deep tillage compared with that under rotary tillage except for the R-CK treatment, and the highest abundance was detected in D-NPKB treatment. Meanwhile, Vicinamibacterales abundance was higher in the R-CK treatment than that in the other treatments.
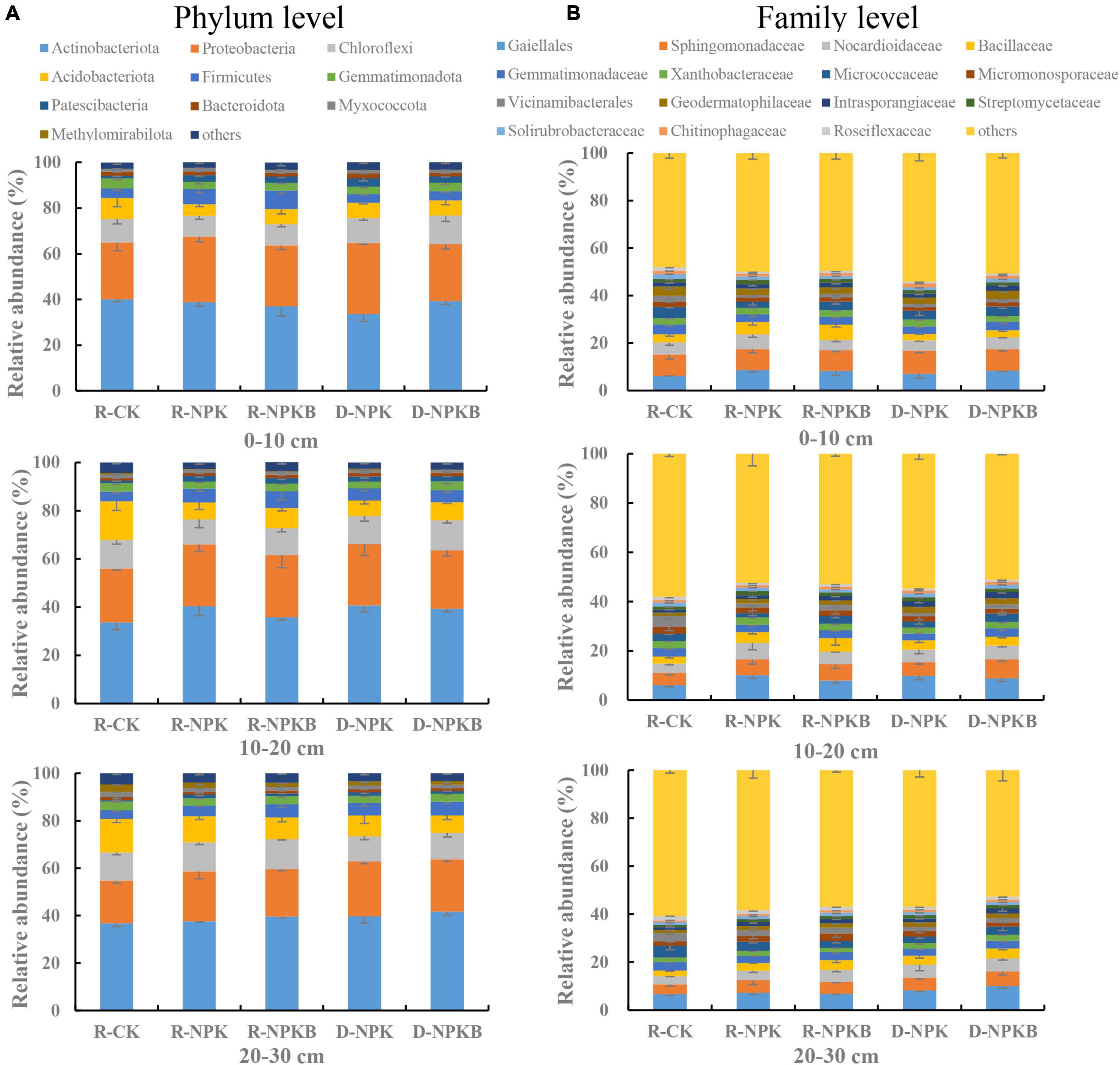
Figure 1. The differences in bacterial community composition among different treatments at the phylum and family levels in 0–10-cm, 10–20-cm, and 20–30-cm soil layers. (A) Actinobacteriota, proteobacteria, chloroflexi, acidobacteriota, firmicutes, gemmatimonadota, patescibacteria, bacteroidota, myxococcota, methylomirabilota, and others. (B) Gaiellales, sphingomonadaceae, nocardioidaceae, bacillaceae, gemmatimonadaceae, xanthobacteraceae, micrococcaceae, miceomonosporaceae, vicinamibacterales, geodermatophilaceae, intrasporangiaceae, streptomycelaceae, solirubrobacteraceae, chitinophagaceae, roseiflexaceae, and others. R-CK, rotary tillage without fertilization. R-NPK, rotary tillage with chemical nitrogen (N), phosphorus (P), and potassium (K) fertilization. R-NPKB, R-NPK plus biochar. D-NPK, deep tillage with chemical N, P, and K fertilization. D-NPKB, D-NPK plus biochar. The different colors represent the different bacterial species at the levels of phylum and family, which are arranged in descending order according to species abundance. The same below.
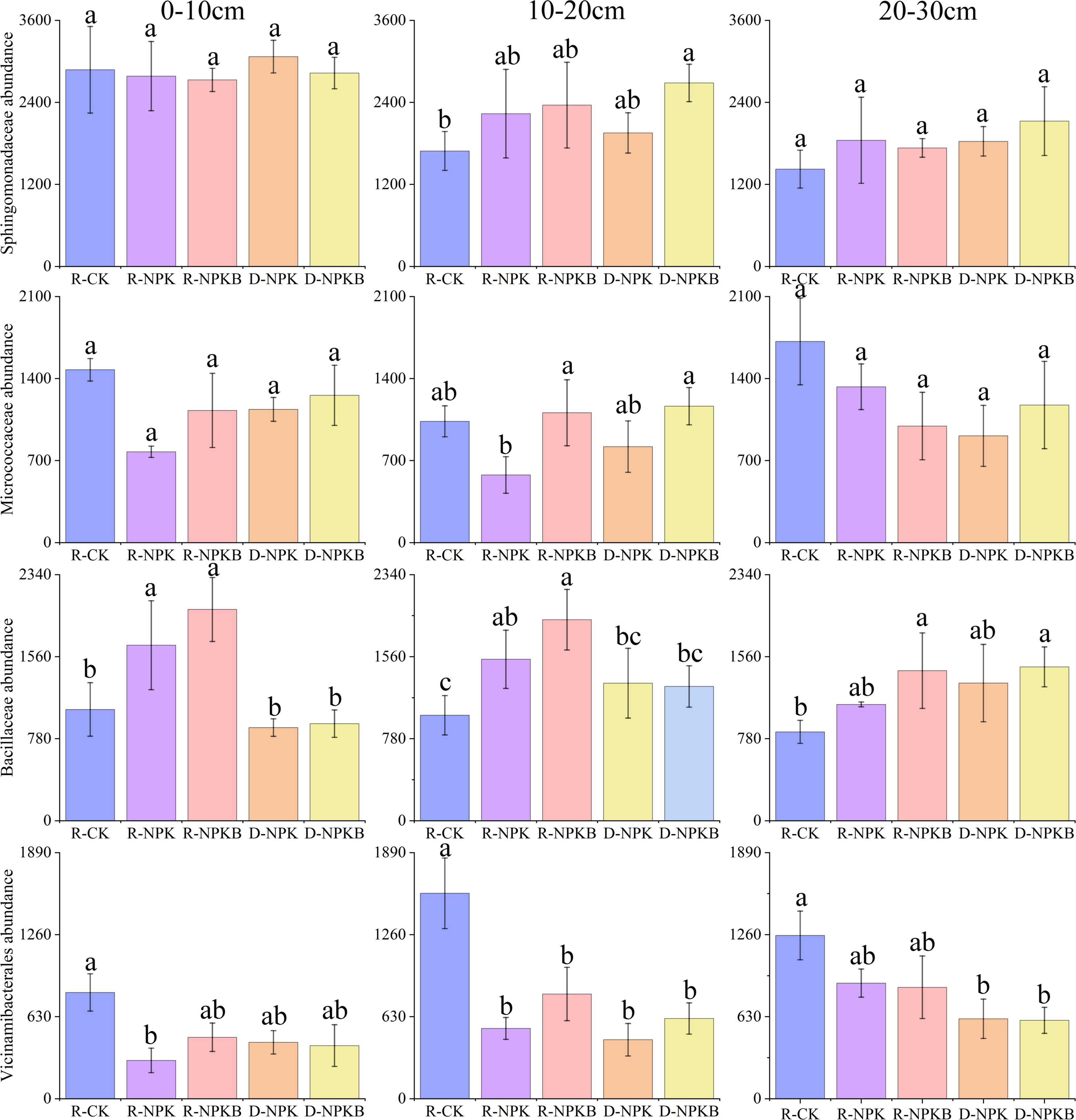
Figure 2. The differences in the abundances of Sphingomonadaceae, Micrococcaceae, Bacillaceae, and Vicinamibacterales at the family level among different treatments in the 0–10-cm, 10–20-cm, and 20–30-cm soil layers. The letters a, b, and c in each subgraph indicate significant differences among different tillage and treatments at the level of P < 0.05.
Redundancy analysis of microbial community structure and soil properties
The differences in the correlations between soil physiochemical and bacterial community among the treatments were analyzed by RDA (Figure 3). The structure of bacterial communities at the family level showed obvious differences among different treatments; significant differences in bacterial community composition were detected between the tillage and fertilization treatments. In the 10–30-cm soil layers, the R-CK treatment appeared clearly separated from the other treatments (R-NPK, R-NPKB, D-NPK, and D-NPKB) (Figures 3B,C), revealing a clear difference in the bacterial community with tillage and biochar application. Besides, in the 10–20-cm soil layer, the R-NPKB and D-NPKB treatments appeared largely separated from the R-NPK and D-NPK treatments (Figure 3B), revealing a significant change in the bacterial community with biochar application. The total variation in the 0– 10-, 10– 20-, and 20–30-cm soil layers was 70.7, 66.7, and 55.4%, respectively, which reflected the influence of soil environmental factors on the structure of the soil bacterial community.
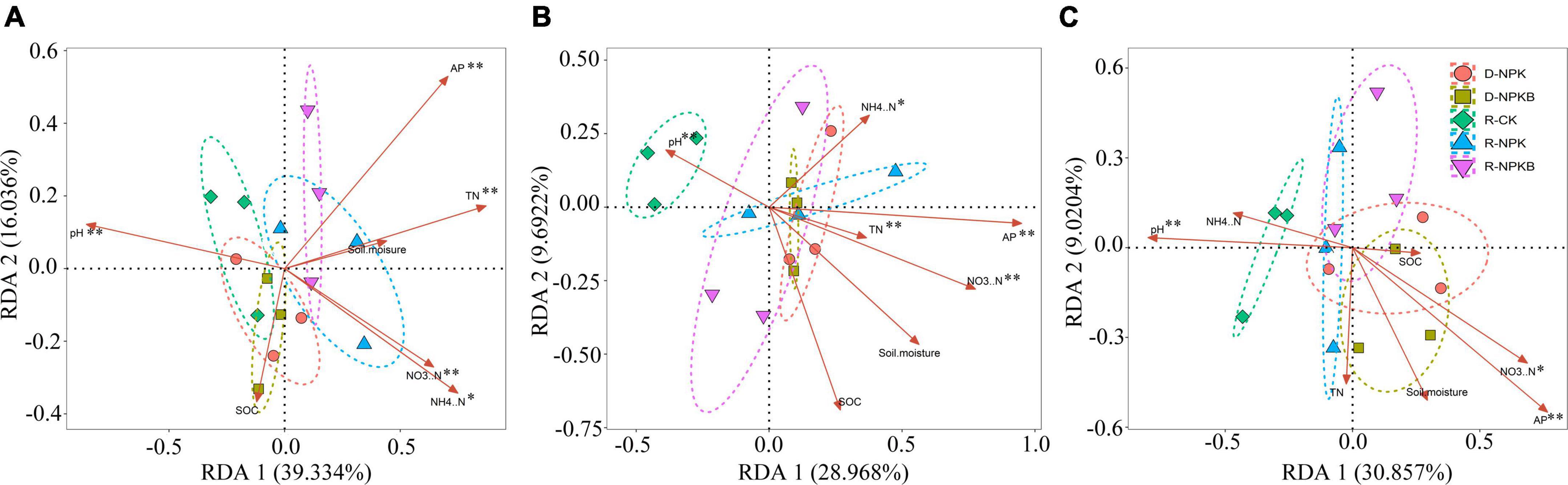
Figure 3. Redundancy analysis between soil physicochemical properties and the soil bacteria at the family level under different tillage methods and fertilizations in the 0–10-cm (A), 10–20-cm (B), and 20–30-cm (C) soil layers, and significant levels are denoted with *P < 0.05 and **P < 0.01. R-CK, rotary tillage without fertilization; R-NPK, rotary tillage with chemical N, P, and K fertilization; R-NPKB, R-NPK plus biochar; D-CK, deep tillage without fertilization; D-NPK, deep tillage with chemical N, P, and K fertilization; D-NPKB, D-NPK plus biochar; AP, available phosphorus; SOC, soil organic carbon; TN, total nitrogen; NH4+–N, ammonium nitrogen; NO3––N, nitrate.
Further, a correlation analysis was performed to explore the effect of soil physicochemical properties on soil bacterial community (Figure 3). The soil pH, AP, and NO3––N were positively related to the community structure in the three soil layers (P < 0.05), and soil NH4+–N and TN were positively related to the bacterial community in the 0–10-cm and 10–20-cm soil layers (P < 0.05). The soil moisture and SOC did not significantly affect the bacterial community in the three soil layers (P > 0.05).
Alpha diversity indices of soil bacteria at the genus level
The bacterial diversity of 45 soil samples under rotary and deep tillage is given in Table 5. The coverage of all samples (coverage index) was over 99%, which indicated that the sequencing data were reliable and reflected the real situation of the soil bacterial community. Different tillage treatments significantly affected the evenness (ACE index; P < 0.05), but not the Shannon diversity index (P > 0.05) in the 0–10-cm soil layer. The ACE index in all treatments under deep tillage was higher than that under rotary tillage in the 0–10- and 10–20-cm soil layers. The richness (Chao’s index) based on the genus level in the NPK and NPKB treatments was significantly more than that in the CK treatment (P < 0.05) in the 0–10-cm soil layer. These findings indicate that the effect of different fertilization treatments was significant (P < 0.05) in the 0–10-cm soil layer. In the 20–30-cm soil layer, tillage significantly affected the Simpson index (P < 0.05); the Simpson index under deep tillage was higher than that under rotary tillage. However, no significant differences in alpha diversity were observed among the tillage and fertilization treatments in the 10–20-cm soil layer.
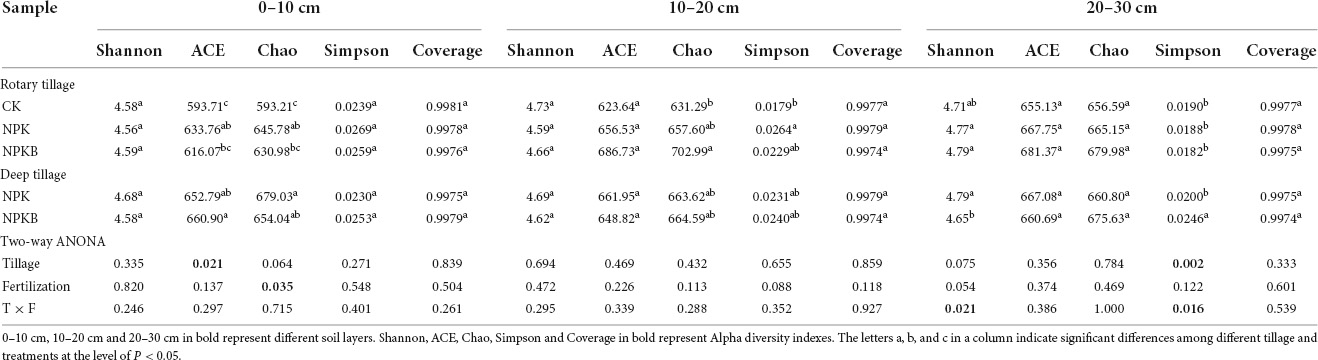
Table 5. Diversity indices of soil bacterial communities based at the genus level of different tillage and fertilization practices.
Structural equation modeling analysis
In this study, the SEM was set up to further clarify the effect of soil physicochemical properties on the richness of bacterial species and crop yield in the three layers of the vertisol (Figure 4). The model explained 6, 7, and 34% of the variation in soil bacterial species richness and 76, 71, and 88% of the variation in yield in the 0– 10-, 10– 20-, and 20–30-cm soil layers, respectively. Besides, the path coefficient was used in the model to estimate the magnitude of the effect of the independent variable on the corresponding dependent variable and compare its relative importance. Deep tillage significantly decreased the soil physicochemical properties of the 0–10-cm soil layer (P < 0.001), while it significantly improved the physicochemical properties of the 10–20-cm and 20–30-cm soil layers (P < 0.05). In addition, biochar application significantly improved the soil physicochemical properties of the 10–20-cm soil layer (P < 0.05). Deep tillage and biochar application directly or indirectly via affecting soil physicochemical properties significantly improved the crop yield (P < 0.05). Deep tillage had a significant negative effect on bacterial richness only in the 20–30-cm soil layer (P < 0.01), but showed no effect in the 0–10- and 10–20-cm soil layers (P > 0.05). Biochar application showed no effect on bacterial richness in the 10–20-cm soil layer (P > 0.05).
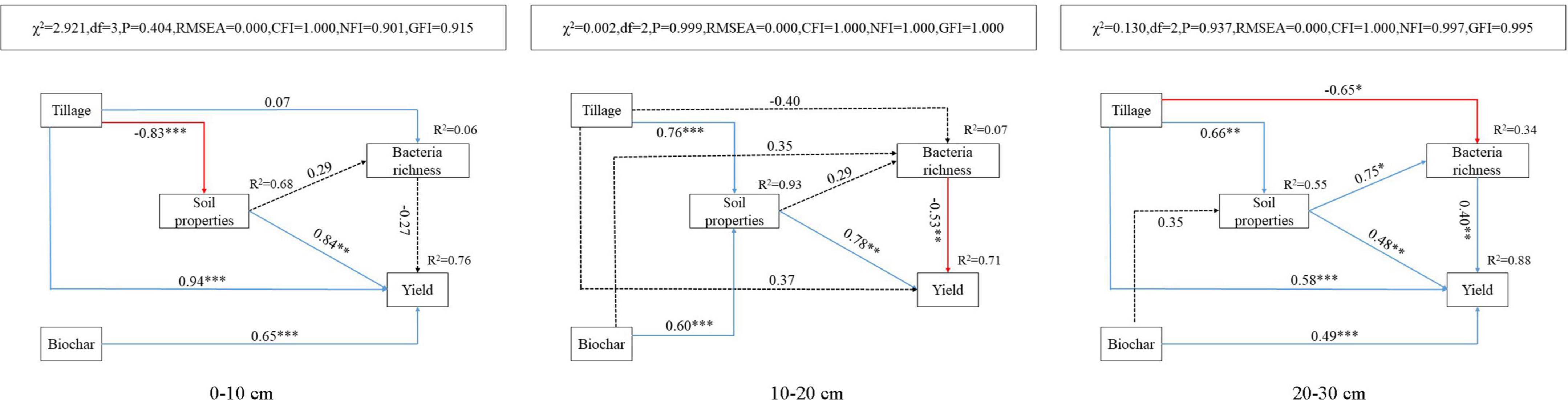
Figure 4. Analysis of factors affecting soil bacterial species richness with the structural equation modeling (SEM) in 0–10-cm, 10–20-cm, and 20–30-cm soil layers. The arrow and the number on the arrow represent the path coefficient, high or low; solid and dashed arrows represent the significant (*P < 0.05, **P < 0.01, and ***P < 0.001) and non-significant effects, respectively; and blue and red arrows represent the positive and negative correlation, respectively.
Network analysis under various soil depths
The average degree and clustering coefficient first decreased and then increased with the soil depth, indicating that the taxon–taxon co-existing network was simple in the 10–20-cm soil layer and complex in the 20–30-cm soil layer; the taxons were more interconnected in the 20–30-cm soil layer (Figures 5A,C). The study also found that compared with the relatively isolated deeper soil layer, the 0–10-cm soil layer was more exposed to environmental interference, corresponding to higher modularity in the soil bacterial community, which was vital for maintaining the stability of the soil bacterial composition (Figure 5C). TN and tillage method were the most important factors strongly associated with soil bacterial taxa in the three soil layers. However, pH was strongly associated with taxa in the 0–10- and 10–20-cm soil layers (Figure 5B).
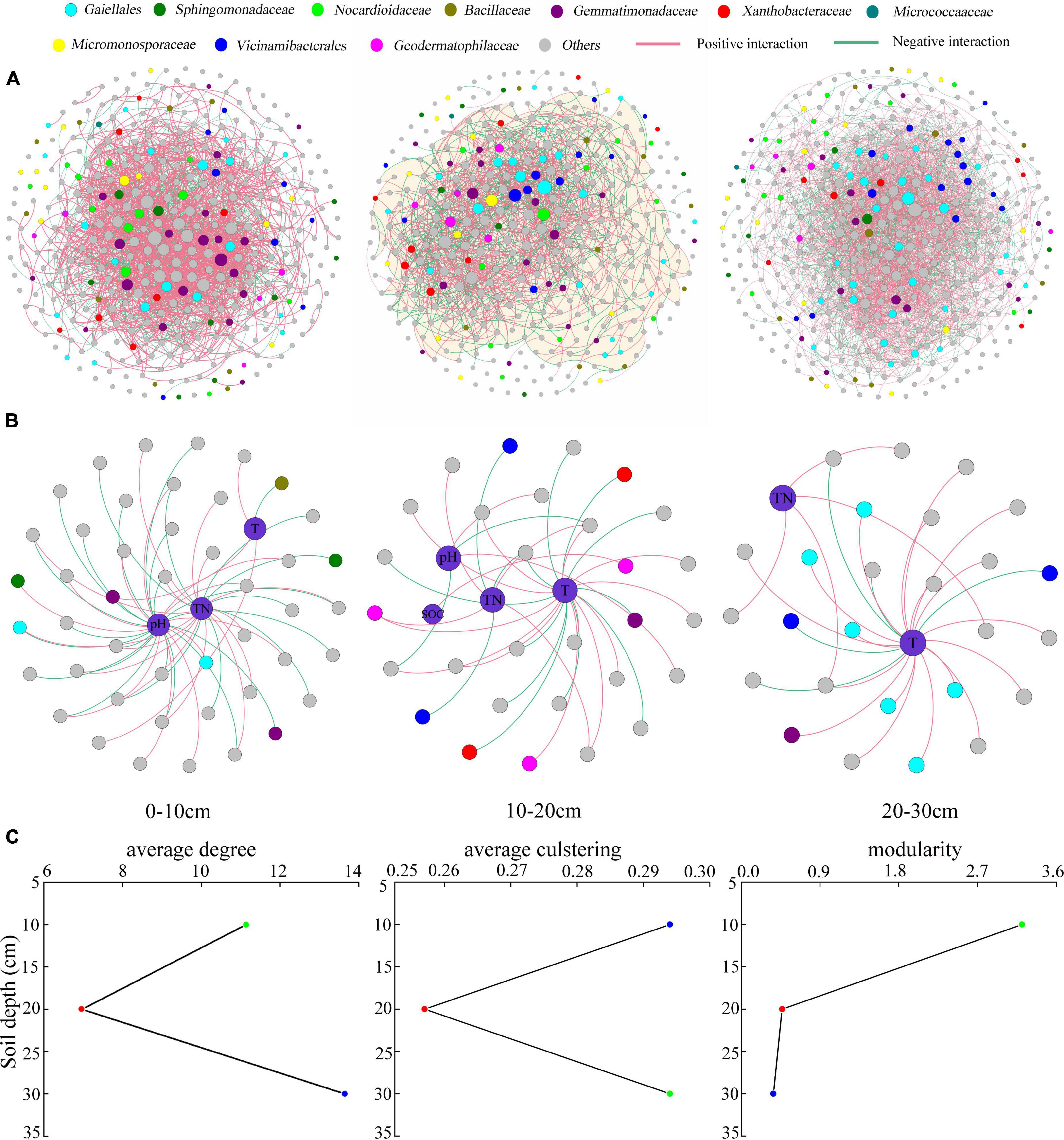
Figure 5. Taxon–taxon networks (A), taxon–environment networks (B), and statistics for taxon–taxon networks (C) in the three soil layers. In panels (A,B), the connection indicates a strong and significant (P < 0.01) correlation; the nodes represent unique sequences in the datasets; the size of each node is proportional to the relative abundance. In panel (C), the green, red, and blue circles in each subplot represent the soil layers 0–10, 10–20, and 20–30 cm, respectively. TN, total nitrogen; T, tillage; SOC, soil organic matter.
Discussion
Effect of deep tillage combined with biochar application on soil physicochemical properties
Agricultural soil bulk density increased along with the soil depth. It was because that surface soil was easily disturbed by human activities and biological factors, such as the input of organic matter, intercropping of crop roots, and activities of soil animals, especially in an agricultural intensive area, which could loose soil and make the surface soil bulk density significantly lower than that of subsoil (Li et al., 2019). Numerous studies have shown that SOC was the main factor affecting the bulk density, and there was a significantly negative correlation between the two (Wang et al., 2014; Yang et al., 2016). Therefore, in our study, increasing SOC in the subsoil through deep tillage combined with biochar application reduced the bulk density.
Deep tillage inverted and completely mixed the surface and deeper soil and turned the surface nutrients into the deeper soil layer, improving the shallow plowing layer in the clay vertisol, and therefore, the soil nutrients in the 20–30-cm soil layer under deep tillage were higher than those under rotary tillage (Qi et al., 2021). Furthermore, biochar, with high pH and an alkalizing effect, increased the soil pH, consistent with the previous studies (Obia et al., 2015; Hussain et al., 2020). In addition, the biochar, with a highly porous structure and large surface area, had a strong adsorption effect on the nutrients. Due to its specific surface characteristics, it reduced the leaching of soil nutrient elements, maintained the soil fertility at a high level, and significantly affected the soil physicochemical properties (Gao and Deluca, 2020; Hussain et al., 2020; Pan et al., 2021). Meanwhile, the primary raw material of biochar is biomass, which contains various nutrients such as N and P. Consistent with several previous studies (Liu et al., 2017; Gao and Deluca, 2020), this study also showed that biochar application increased soil nutrients and productivity. It is worth noting that the soil pH of the CK treatments was significantly higher than that of the NPK treatments in all periods, especially in the 2019 winter wheat maturity stage, and the soil pH of the CK treatments was significantly higher than that of the NPK treatment by about 1 pH unit, probably due to rainfall. The low rainfall during the sampling period led to poor mobility and a high accumulation of base cations in the surface soil (Tuo et al., 2020); therefore, the soil pH treated by CK treatment was high.
Further, deep tillage combined with biochar application lowered the soil bulk density, while it significantly increased the soil AP, SOC, TN, and NO3––N content of the 20–30-cm soil layer, similar to the previous reports in various soil texture types (Gao and Deluca, 2020; Hu et al., 2021). Although the soil physicochemical properties were different, there interactions were displayed. For example, high SOC content could lower the soil bulk density by changing the soil texture and increase N and P contents in the soil by promoting N and P biochemical cycles (Huang et al., 2005; Adhikari and Bhattacharyya, 2015). In this study, the soil pH in the 20–30-cm soil layer was much higher than that in the 0–10-cm soil layer, which showed serious acidification occurred on the soil surface. Because the fertilizer was applied to the topsoil and a little fertilizer seeped into the deeper layers by rainwater and floodwater (Savin et al., 2021), the soil nutrient content in the 20–30-cm soil layer was lower than those in the topsoil. Thus, deep tillage and biochar application not only improved soil chemical nutrients, but also alleviated acidification of the vertisols.
Effects of soil physical properties on the structure of soil bacterial community
Deep tillage and biochar indirectly affect bacterial abundance via affecting the flow of water and air, which are the main factors needed for microbial growth (Abujabhah et al., 2016; Wang et al., 2020). The soil bacterial community composition varies with soil depth, and oxygen in different soil depths causes this difference. For example, the abundance of aerobic bacteria, such as Sphingomonadaceae and Bacillaceae, was higher in the topsoil than that in the subsoil. On the contrary, the abundance of Methylomirabilota, a type of anaerobic bacteria, was significantly higher in the subsoil than that in the topsoil. Consistent with the results of previous related studies, it was observed in the current study that deep tillage promoted the activation of aerobic microorganisms, such as Gaiellale and Bacillaceae, by improving the soil permeability and decreasing the soil bulk density (Wang et al., 2020, 2021).
In this study, although the soil bacterial communities varied in the different layers, no significant change in the dominant species of the community structure was observed. The similarity in the vertical distribution of the dominant soil bacterial species might be due to their strong diffusion effect, distribution range, or strong adaptability to the environmental changes in these bacterial species, which easily resulted in the random and extensive distribution characteristics (He and Ge, 2008). Besides, biochar application increased the abundances of Sphingomonadaceae and Micrococcaceae, mainly due to an increase in the soil bacterial community and activity (Fang et al., 2020; Sun et al., 2020). The decreased soil bulk density under deep tillage and with biochar additions implied the increased soil air permeability. As reported in several previous studies, due to the porous structure and large surface area (Hussain et al., 2020; Pan et al., 2021), the biochar application created a suitable living environment for soil bacteria to grow and breed by improving the soil porosity (Abujabhah et al., 2016; Hussain et al., 2020). In addition, biochar could provide safe niches for the soil bacteria to protect against predation by the protozoa (Azeem et al., 2021). Moisture content is the main factor affecting the biological activity; however, no significant effects of soil moisture on soil bacterial composition or diversity were detected in this study, probably due to the subtle difference between soil layers in the clay soils.
Effects of soil chemical properties on the bacterial community
Previous studies have demonstrated that the soil chemical properties are the important factors affecting the structure of soil bacterial communities (Wei et al., 2019; Sun et al., 2020). This study’s taxon–environment network analysis showed that the soil pH, TN, and SOC were strongly correlated with bacterial taxa in the 0–30-cm soil layers (Figure 5B), consistent with the studies of Qiu et al. (2020) and Wan et al. (2020). We found that pH and SOC significantly affected the bacterial community only in the 0–10- and 10–20-cm soil layers and were the major factors driving the bacterial community. The soil layers in 0–10 and 10–20 cm depth had an acidic environment that bacteria preferred. Meanwhile, SOC, a vital source in the microbially driven carbon cycle, was mainly distributed in the topsoil, and therefore, the changes in pH and SOC are more associated with the soil bacteria (Puissant et al., 2015; Cao et al., 2016). The studies have identified the soil pH and tillage as important factors determining the distribution pattern of microorganisms (Xia et al., 2020; Shanmugam et al., 2021). The soil pH was an important factor that influenced the structure and diversity of soil metabolism and bacterial community (Siles and Margesin, 2016; Cheng et al., 2020). Sun et al. (2015) found that the structure of the soil bacterial community in soils with similar pH had similar characteristics, consistent with the conclusion of this study.
Different tillage operations lead to the breaking up and incorporation of crop residues into different soil depths and accelerate the decomposition and mineralization processes as the microbial decomposition acts on the available nitrogen, carbon, and other nutrients (Le et al., 2019). Deep tillage provided the nutrients for soil microorganisms to grow by turning the last crop stalks into deeper soil, which impacted the activity and the abundance of soil microorganisms (Gregorutti and Caviglia, 2019; Lou et al., 2021). Thus, the bacterial community composition of the bottom soil also changed. On the contrary, tillage might have reduced the habitat heterogeneity, favoring few bacteria and helping them outgrow other competitors; this eventually changed the bacterial community (Shanmugam et al., 2021), by altering the chemical properties.
Effects of soil physicochemical properties and bacterial community structure on crop yield
Soil physicochemical properties directly affect crop yield by influencing nutrient input or indirectly by altering soil microorganisms (Lei et al., 2019; Chen et al., 2020). Soil physicochemical properties such as N, P, and K were essential for crop growth and maintenance and improvement in soil quality, which resulted in high crop yield (Belay et al., 2002; Zhao et al., 2013; Eo and Park, 2016). Moreover, soil bacterial community composition was sensitive to variations in the environmental features such as soil properties, and these soil bacteria played a key role in determining crop yield (Berg, 2009; Vasconcellos et al., 2013; Sun et al., 2015). Deep tillage broke the soil obstacles and improved the aeration, which benefited crop root growth (Muche et al., 2017; Schneider et al., 2017). In this study, deep tillage in the vertisol soil increased the winter wheat yield significantly (P < 0.05) compared with the traditional rotary tillage. However, the increase in maize yield under deep tillage was insignificant, contradicting the yield increase under deep tillage (Schneider et al., 2017; Wang et al., 2021). This inconsistency may be due to the soil environment or seasonal climate differences. We also found that the soil NO3––N content was significantly and positively associated with crop yield in the three soil layers (Table 6A). In acid soil, the soil pH of the 0–10- and 10–20-cm soil layers was positively associated with crop yield, while an opposite association was observed in the 20–30-cm soil layer. Pan et al. (2020) reported that low soil pH reduced N uptake and inhibited maize root in the topsoil, but a neutral pH in the subsoil promoted N absorption by the crop. Meanwhile, the NH4+–N and TN content of the 20–30-cm soil layer was negatively associated with the crop yield, probably because high N inhibited biological N fixation (Ding et al., 2020).

Table 6. (A) Correlation of soil properties, soil bacterial species richness, and grain yields (N = 5).
Besides, few soil bacteria might have altered the effect on the soil nutrient availability (Zhang et al., 2020). Only the abundances of Gaiellalea, Sphingomonadaceae, and Nocardioidaceae were positively correlated with the crop yield (r = 0.866, P < 0.05; r = 0.883, P < 0.05; and r = 0.810, P < 0.05, respectively), while the abundance of Vicinamibacterales was negatively correlated with the crop yield (r = −0.945, P < 0.05) (Table 6B). In the soil, bacteria belonging to Sphingomonadaceae were noted for secreting acidic exopolysaccharides, and it could protect the rhizosphere and promote the rooting by biodegrading a variety of complex xenobiotics (Johnsen et al., 2000; Benson et al., 2004). This study found that deep tillage combined with biochar increased subsoil’s bacteria abundance and bacteria diversity indexes. As the bacterial abundance improved, it increased solubilization of AP. Increased uptake of AP might enhance root growth that ultimately enhanced the uptake of nutrients, eventually resulting in better yield (Siddiq et al., 2018; Azeem et al., 2021). Bacterial diversity increased the soil quality fertility and the soil fertility, which were important for nutrient cycling and improving the plant health. Thus, the soil physicochemical properties and bacteria had a significant effect on the crop yield. Meanwhile, deep tillage combined with biochar indirectly increased crop yield by improving soil physicochemical properties and altering the bacterial community.
Conclusion
This study found deep tillage combined with biochar application as a reliable agricultural practice for soils with high clay content, superficial topsoil, and soils with poor nutrient content. This agricultural practice broke the physical obstacles in the clay vertisols of the North China Plain, improving the clay vertisol texture and shallow plowing layer. At the same time, deep tillage combined with biochar application enhanced the nutrient content (NO3––N, AP, SOC, and TN) in the subsoil and subsequently improved the crop yield. However, this study carried out deep tillage and sampling only at the 0–30-cm soil layer. Further studies involving long-term experiments are needed for a better understanding of the effects of deeper tillage at other soil depths to improve the vertisol. This study provides evidence for increasing grain production and improving the vertisol via deep tillage combined with biochar application.
Data availability statement
The datasets presented in this study can be found in online repositories. The names of the repository/repositories and accession number(s) can be found below: https://www.ncbi.nlm.nih.gov/, PRJNA827575.
Author contributions
PL designed the study. YH and WC performed the field experiments and conducted the fieldwork. WC, FL, and JX conducted the laboratory work. WC and PL analyzed the data. WC wrote the manuscript. All authors read and approved the final manuscript.
Funding
This work was financially supported by the National Key Research and Development Program of China (2018YFD0200606 and 2017YFD0301103) and Young Elite Scientists Sponsorship Program by Henan Association for Science and Technology (2022HYTP036).
Conflict of interest
The authors declare that the research was conducted in the absence of any commercial or financial relationships that could be construed as a potential conflict of interest.
Publisher’s note
All claims expressed in this article are solely those of the authors and do not necessarily represent those of their affiliated organizations, or those of the publisher, the editors and the reviewers. Any product that may be evaluated in this article, or claim that may be made by its manufacturer, is not guaranteed or endorsed by the publisher.
Supplementary material
The Supplementary Material for this article can be found online at: https://www.frontiersin.org/articles/10.3389/fmicb.2022.929725/full#supplementary-material
Footnotes
References
Abujabhah, I. S., Bound, S. A., Doyle, R., and Bowman, J. P. (2016). Effects of biochar and compost amendments on soil physico-chemical properties and the total community within a temperate agricultural soil. Appl. Soil Ecol. 98, 243–253. doi: 10.1016/j.apsoil.2015.10.021
Adhikari, G., and Bhattacharyya, K. G. (2015). Correlation of soil organic carbon and nutrients (NPK) to soil mineralogy, texture, aggregation, and land use pattern. Environ. Monit. Assess. 187, 1–18. doi: 10.1007/s10661-015-4932-5
Ahmad, N. (1996). Occurrence and distribution of vertisols. Dev. Soil Sci. 24, 1–41. doi: 10.1016/S0166-2481(96)80003-1
Azeem, M., Hassan, T. U., Tahir, M. I., Ali, A., Jeyasundar, P. G. S. A., Hussain, Q., et al. (2021). Tea leaves biochar as a carrier of Bacillus cereus improves the soil function and crop productivity. Appl. Soil Ecol. 157:103732. doi: 10.1016/j.apsoil.2020.103732
Aziz, I., Mahmood, T., and Islam, K. R. (2013). Effect of long term no-till and conventional tillage practices on soil quality. Soil Tillage Res. 131, 28–22. doi: 10.1016/j.still.2013.03.002
Belay, A., Claassens, A., and Wehner, F. (2002). Effect of direct nitrogen and potassium and residual phosphorus fertilizers on soil chemical properties, microbial components and maize yield under long-term crop rotation. Biol. Fert. Soils 35, 420–427. doi: 10.1007/s00374-002-0489-x
Benson, M. J., Gawronski, J. D., Eveleigh, D. E., and Benson, D. R. (2004). Intracellular symbionts and other bacteria associated with deer ticks (Ixodes scapularis) from nantucket and wellfleet, cape cod, Massachusetts. Appl. Environ. Microb. 70, 616–620. doi: 10.1128/AEM.70.1.616-620.2004
Berg, G. (2009). Plant–microbe interactions promoting plant growth and health: Perspectives for controlled use of microorganisms in agriculture. Appl. Microbiol. Biot. 84, 11–18. doi: 10.1007/s00253-009-2092-7
Cao, H. C., Chen, R. R., Wang, L. B., Jiang, L. L., Yang, F., Zheng, S. X., et al. (2016). Soil pH, total phosphorus, climate and distance are the major factors influencing microbial activity at a regional spatial scale. Sci. Rep. 6:25815. doi: 10.1038/srep25815
Chen, H., Zheng, C. Y., Qiao, Y. Q., Du, S. Z., Li, W., Zhang, X. Q., et al. (2021). Long-term organic and inorganic fertilization alters the diazotrophic abundance, community structure, and co-occurrence patterns in a vertisol. Sci. Total Environ. 766:142441. doi: 10.1016/j.scitotenv.2020.142441
Chen, M. Q., Xu, J. S., Li, Z. Q., Zhao, B. Z., and Zhang, J. B. (2020). Soil physicochemical properties and bacterial community composition jointly affect crop yield. Agron. J. 112, 4358–4372. doi: 10.1002/agj2.20358
Cheng, J. M., Zhao, M. X., Cong, J., Qi, Q., Xiao, Y., Cong, W., et al. (2020). Soil pH exerts stronger impacts than vegetation type and plant diversity on soil bacterial community composition in subtropical broad-leaved forests. Plant Soil 450, 273–286. doi: 10.1007/s11104-020-04507-2
DeCarlo, K. F., and Caylor, K. K. (2020). Effects of crack morphology on soil carbon flux dynamics in a dryland vertisol. Geoderma 375:B4. doi: 10.1016/j.geoderma.2020.114478
Ding, Y. Q., Jin, Y. L., He, K. Z., Yi, Z. L., Tan, L., Liu, L. S., et al. (2020). Low nitrogen fertilization alter rhizosphere microorganism community and improve sweetpotato yield in a nitrogen-deficient rocky soil. Front. Microbiol. 11:678. doi: 10.3389/fmicb.2020.00678
Eo, J., and Park, K. C. (2016). Long-term effects of imbalanced fertilization on the composition and diversity of soil bacterial community. Agr. Ecosyst. Environ. 231, 176–182. doi: 10.1016/j.agee.2016.06.039
Fang, W. S., Song, Z. X., Tao, S., Zhang, D. Q., Huang, B., Ren, L. R., et al. (2020). Biochar mitigates the negative effect of chloropicrin fumigation on beneficial soil microorganisms. Sci. Total Environ. 738:139880. doi: 10.1016/j.scitotenv.2020.139880
Fang, Y. Y., Singh, B. P., Nazaries, L., Keith, A., Tavakkoli, E., Wilson, N., et al. (2019). Interactive carbon priming, microbial response and biochar persistence in a Vertisol with varied inputs of biochar and labile organic matter. Eur. J. Soil Sci. 70, 960–974. doi: 10.1111/ejss.12808
FAO-UNESCO-ISRIC. (1988). FAO-UNESCO soil map of the world: Revised legend. World soil resources report. Rome: FAO, 119.
Feng, Q., An, C. J., Chen, Z., and Wang, Z. (2020). Can deep tillage enhance carbon sequestration in soils? A meta-analysis towards GHG mitigation and sustainable agricultural management. Renew. Sust. Energ. Rev. 133:110293. doi: 10.1016/j.rser.2020.110293
Gao, S., and Deluca, T. H. (2020). Biochar alters nitrogen and phosphorus dynamics in a western rangeland ecosystem. Soil Biol. Biochem. 148:107868. doi: 10.1016/j.soilbio.2020.107868
Gregorutti, V. C., and Caviglia, O. P. (2019). Impact of crop aerial and root biomass inputs on soil nitrifiers and cellulolytic microorganisms. Soil Tillage Res. 191, 85–97. doi: 10.1016/j.still.2019.03.018
He, J. Z., and Ge, Y. (2008). Recent advances in soil microbial biogeography. Acta Ecol. Sin. 11, 5571–5582. doi: 10.3321/j.issn:1000-0933.2008.11.041
Hu, R. W., Liu, Y. J., Chen, T., Zheng, Z. Y., Peng, G. J., Zou, Y. D., et al. (2021). Responses of soil aggregates, organic carbon, and crop yield to short-term intermittent deep tillage in Southern China. J. Clean. Prod. 298:126767. doi: 10.1016/J.JCLEPRO.2021.126767
Huang, P. M., Wang, M. K., and Chiu, C. Y. (2005). Soil mineral–organic matter–microbe interactions: Impacts on biogeochemical processes and biodiversity in soils. Pedobiologia 49, 609–635. doi: 10.1016/j.pedobi.2005.06.006
Hussain, R., Ravi, K., and Garg, A. (2020). Influence of biochar on the soil water retention characteristics (SWRC): Potential application in geotechnical engineering structures. Soil Tillage Res. 204:104713. doi: 10.1016/j.still.2020.104713
Johnsen, A. R., Hausner, M., Schnell, A., and Wuertz, S. (2000). Evaluation of fluorescently labeled lectins for noninvasive localization of extracellular polymeric substances in Sphingomonas biofilms. Appl. Environ. Microb. 66, 3487–3491. doi: 10.1016/j.nimb.2004.12.071
Kjeldahl, J. (1883). New method for the determination of nitrogen. Chem. Eng. News 48, 101–102. doi: 10.1038/scientificamerican10061883-6470
Le, G. C., Chemidlin, P. N., Karimi, B., Akkal-Corfini, N., Dequiedt, S., Nowak, V., et al. (2019). Tillage intensity and pasture in rotation effectively shape soil microbial communities at a landscape scale. Microbiologyopen 8:e00676. doi: 10.1002/mbo3.676
Lei, N., Han, J. C., Mu, X. M., Sun, Z. H., and Wang, H. Y. (2019). Effects of improved materials on reclamation of soil properties and crop yield in hollow villages in China. J. Soils Sediments 19, 2374–2380. doi: 10.1007/s11368-019-02246-1
Li, P. P., Chen, W. J., Han, Y. L., Wang, D. C., Zhang, Y. T., and Wu, C. F. (2020). Effects of straw and its biochar applications on the abundance and community structure of CO2-fixing bacteria in a sandy agricultural soil. J. Soils Sediments 20, 2225–2235. doi: 10.1007/s11368-020-02584-5
Liu, L., Wang, Y. F., Yan, X. W., Li, J. W., Jiao, N. Y., and Hu, S. J. (2017). Biochar amendments increase the yield advantage of legume-based intercropping systems over monoculture. Agr. Ecosyst. Environ. 237, 16–23. doi: 10.1016/j.agee.2016.12.026
Liu, Z., Cao, S. L., Sun, Z. H., Wang, H. Y., Qu, S. D., Lei, N., et al. (2021). Tillage effects on soil properties and crop yield after land reclamation. Sci. Rep. 11:4611. doi: 10.1038/s41598-021-84191-z
Lopes, L. D., Junior, R. C. F., Pacheco, E. P., and Fernandes, M. F. (2021). Shifts in microbial and physicochemical parameters associated with increasing soil quality in a tropical Ultisol under high seasonal variation. Soil Tillage Res. 206:104819. doi: 10.1016/j.still.2020.104819
Lou, S. Y., He, J., Li, H. W., Wang, Q. J., Lu, C. Y., Liu, W. Z., et al. (2021). Current knowledge and future directions for improving subsoiling quality and reducing energy consumption in conservation fields. Agriculture 11:575. doi: 10.3390/AGRICULTURE11070575
Muche, H., Abdela, M., Schmitter, P., and Nakawuka, P. (2017). Application of deep tillage and Berken Maresha for hardpan sites to improve infiltration and crop productivity. Bahir Dar: ICAST.
Nelson, D. W., and Sommers, L. E. (1982). Dry combustion method using medium temperature resistance furnace. Chem. Microbiol. Properties 2, 539–579.
Novelli, L. E., Hass, W. L., Benintende, S. M., and Caviglia, O. P. (2020). Microbial activity effect on aggregate stability after residue addition in a mollisol and a vertisol in the Pampas, Argentina. Geoderma Reg. 23:e00346. doi: 10.1016/j.geodrs.2020.e00346
Obia, A., Cornelissen, G., Mulder, J., and Dörsch, P. (2015). Effect of soil pH increase by biochar on NO, N2O and N2 production during denitrification in acid soils. PLoS One 10:e0138781. doi: 10.1371/journal.pone.0138781
Olsen, S. R., Cole, C. V., Watanabe, F. S., and Dean, L. A. (1954). Estimation of available phosphorus in soils by extraction with sodium bicarbonate. USDA Cricular 939. Washington DC: U.S. Government Printing Office.
Pan, X. Q., Gu, Z. P., Chen, W. M., and Li, Q. B. (2021). Preparation of biochar and biochar composites and their application in a Fenton-like process for wastewater decontamination: A review. Sci. Total Environ. 754:142104. doi: 10.1016/j.scitotenv.2020.142104
Pan, X. Y., Baquy, M. A., Guan, P., Yan, J., Wang, R. H., Xu, R. K., et al. (2020). Effect of soil acidification on the growth and nitrogen use efficiency of maize in Ultisols. J. Soils Sediments 20, 1435–1445. doi: 10.1007/s11368-019-02515-z
Puissant, J., Cécillon, L., Mills, R. T. E., Robroek, B. J. M., Gavazov, K., Danieli, S. D., et al. (2015). Seasonal influence of climate manipulation on microbial community structure and function in mountain soils. Soil Biol. Biochem. 80, 296–305. doi: 10.1016/j.soilbio.2014.10.013
Qi, J. Y., Zhao, X., He, C., Virk, A. L., Jing, Z. H., Liu, Q. Y., et al. (2021). Effects of long-term tillage regimes on the vertical distribution of soil iron/aluminum oxides and carbon decomposition in rice paddies. Sci. Total Environ. 776:145797. doi: 10.1016/J.SCITOTENV.2021.145797
Qiu, J. M., Cao, J. H., Lan, G. Y., Liang, Y. M., Wang, H., and Li, Q. (2020). The influence of land use patterns on soil bacterial community structure in the karst graben basin of Yunnan province. China Forests 11:51. doi: 10.3390/f11010051
Savin, M. C., Daigh, A. L. M., Brye, K. R., Norman, R., and Miller, D. (2021). Vertical distribution of fertilizer nitrogen from surface water flooding of a silt loam and clay soil used for rice production. Soil Use Manage. 37, 406–417. doi: 10.1111/sum.12599
Schneider, F., Don, A., Hennings, I., Schmittmann, O., and Seidel, S. J. (2017). The effect of deep tillage on crop yield–What do we really know? Soil Tillage Res. 174, 193–204. doi: 10.1016/j.still.2017.07.005
Li, Shan, Li, Q. Q., Wang, C. Q., Li, B., Gao, X. S., Li, Y. D., et al. (2019). Spatial variability of soil bulk density and its controlling factors in an agricultural intensive area of chengdu plain, Southwest China. J. Integr. Agr. 18, 290–300. doi: 10.1016/S2095-3119(18)61930-6
Shanmugam, S. G., Buehring, N. W., Prevost, J. D., and Kingery, W. L. (2021). Soil bacterial community diversity and composition as affected by tillage intensity treatments in corn-soybean production systems. Microbiol. Res. 12, 157–172. doi: 10.3390/MICROBIOLRES12010012
Siddiq, S., Saleem, U., Ahmad, K., Anayat, A., Affan, Q. M., Anwar, M. F., et al. (2018). Comparison of conventional and non-conventional carriers for bacterial survival and plant growth. Int. J. Agric. Inn. Res. 6, 126–129. doi: 10.13140/RG.2.2.26668.56962
Siles, J. A., and Margesin, R. (2016). Abundance and diversity of bacterial, archaeal, and fungal communities along an altitudinal gradient in alpine forest soils: What are the driving factors? Microb. Ecol. 72, 207–220. doi: 10.1007/s00248-016-0748-2
Sun, R. B., Zhang, X. X., Guo, X. S., Wang, D. Z., and Chu, H. Y. (2015). Bacterial diversity in soils subjected to long-term chemical fertilization can be more stably maintained with the addition of livestock manure than wheat straw. Soil Biol. Biochem. 88, 9–18. doi: 10.1016/j.soilbio.2015.05.007
Sun, Y. T., Luo, C. L., Jiang, L. F., Song, M. K., Zhang, D. Y., Li, J., et al. (2020). Land-use changes alter soil bacterial composition and diversity in tropical forest soil in China. Sci. Total Environ. 712:136526. doi: 10.1016/j.scitotenv.2020.136526
Tipton, L., Zahn, G. L., Darcy, J. L., Amend, A. S., and Hynson, N. A. (2021). Hawaiian fungal amplicon sequence variants reveal otherwise hidden biogeography. Microb. Ecol. 83, 48–57. doi: 10.1007/S00248-021-01730-X
Tuo, Y. F., Shen, F. Y., Yang, C. P., Ma, J. M., Guo, S. N., Zhang, D., et al. (2020). Effects of rainfall on phosphrous, organic matter and pH in different land use type in middle Yunnan plateau. J. Ecol. Environ. 29, 942–950. doi: 10.16258/j.cnki.1674-5906.2020.05.010
Vasconcellos, R. L. F., Bonfim, J. A., Andreote, F. D., Mendes, L. W., Baretta, D., and Cardoso, E. J. B. N. (2013). Microbiological indicators of soil quality in a riparian forest recovery gradient. Ecol. Eng. 53, 313–320. doi: 10.1016/j.ecoleng.2012.12.067
Wan, W. J., Tan, J. D., Wang, Y., Qin, Y., He, H. M., Wu, H. Q., et al. (2020). Responses of the rhizosphere bacterial community in acidic crop soil to pH: Changes in diversity, composition, interaction, and function. Sci. Total Environ. 700:134418. doi: 10.1016/j.scitotenv.2019.134418
Wang, Q., Garrity, G. M., Tiedje, J. M., and Cole, J. R. (2007). Naive Bayesian classifier for rapid assignment of rRNA sequences into the new bacterial taxonomy. Appl. Environ. Microb. 73, 5261–5267. doi: 10.1128/AEM.00062-07
Wang, Y. K., Zhang, Z. B., Jiang, F. H., Guo, Z. C., and Peng, X. H. (2021). Evaluating soil physical quality indicators of a Vertisol as affected by different tillage practices under wheat-maize system in the North China Plain. Soil Tillage Res. 209:104970. doi: 10.1016/j.still.2021.104970
Wang, Y. Q., Shao, M. A., Liu, Z. P., and Zhang, C. C. (2014). Prediction of bulk density of soils in the Loess Plateau region of China. Surv. Geophys. 35, 395–413. doi: 10.1007/s10712-013-9249-8
Wang, Z. T., Li, T., Li, Y. Z., Zhao, D. Q., Han, J., Liu, Y., et al. (2020). Relationship between the microbial community and catabolic diversity in response to conservation tillage. Soil Tillage Res. 196:104431. doi: 10.1016/j.still.2019.104431
Wei, Z. W., Hao, Z. K., Li, X. H., Guan, Z. B., Cai, Y. J., and Liao, X. R. (2019). The effects of phytoremediation on soil bacterial communities in an abandoned mine site of rare earth elements. Sci. Total Environ. 670, 950–960. doi: 10.1016/j.scitotenv.2019.03.118
Wu, Y. H., Si, Y. B., Zhou, D. M., and Gao, J. (2015). Adsorption of diethyl phthalate ester to clay minerals. Chemosphere 119, 690–696. doi: 10.1016/j.chemosphere.2014.07.063
Xia, Q., Rufty, T., and Shi, W. (2020). Soil microbial diversity and composition: Links to soil texture and associated properties. Soil Biol. Biochem. 149:107953. doi: 10.1016/j.soilbio.2020.107953
Xiong, P., Zhang, Z. B., Hallett, P. D., and Peng, X. H. (2020). Variable responses of maize root architecture in elite cultivars due to soil compaction and moisture. Plant Soil 455, 1–2. doi: 10.1007/s11104-020-04673-3
Xu, M., Hao, X. L., Xiong, Z. Q., Liao, H., Wang, L., Zhang, T. Y., et al. (2020). Soil amendments change bacterial functional genes more than taxonomic structure in a cadmium-contaminated soil. Soil Biol. Biochem. 154:108126. doi: 10.1016/J.SOILBIO.2020.108126
Xu, N., Tan, G. C., Wang, H. Y., and Gai, X. P. (2016). Effect of biochar additions to soil on nitrogen leaching, microbial biomass and bacterial community structure. Eur. J. Soil Biol. 74, 1–8. doi: 10.1016/j.ejsobi.2016.02.004
Yang, Q. Y., Luo, W. Q., Jiang, Z. C., Li, W. J., and Yuan, D. X. (2016). Improve the prediction of soil bulk density by corkiging with predicted soil water content as auxiliary variable. J. Soil Sediment 16, 77–84. doi: 10.1007/s11368-015-1193-4
Yu, Q., Wang, H., Wen, P. F., Wang, S. L., Li, J., Wang, R., et al. (2020). A suitable rotational conservation tillage system ameliorates soil physical properties and wheat yield: An 11-year in-situ study in a semi-arid agroecosystem. Soil Tillage Res. 199:104600. doi: 10.1016/j.still.2020.104600
Zhang, D., Ge, S. F., Wang, C., Jiang, Y. M., Li, X. L., Xia, S. J., et al. (2020). The relationship between soil bacteria and metal nutrient availability for uptake of apple trees in Chinese orchards. Plant Growth Regul. 92, 181–193. doi: 10.1007/s10725-020-00629-w
Keywords: deep tillage, biochar, clayey soil, physicochemical properties, bacterial community structure, redundancy analysis, structural equation modeling
Citation: Chen W, Li P, Li F, Xi J and Han Y (2022) Effects of tillage and biochar on soil physiochemical and microbial properties and its linkage with crop yield. Front. Microbiol. 13:929725. doi: 10.3389/fmicb.2022.929725
Received: 27 April 2022; Accepted: 10 August 2022;
Published: 20 September 2022.
Edited by:
Jorge Paz-Ferreiro, RMIT University, AustraliaReviewed by:
Rocío Barros, University of Burgos, SpainTeodor Rusu, University of Agricultural Sciences and Veterinary Medicine of Cluj-Napoca, Romania
Juntao Wang, Western Sydney University, Australia
Tong Li, Zhengzhou University, China
Copyright © 2022 Chen, Li, Li, Xi and Han. This is an open-access article distributed under the terms of the Creative Commons Attribution License (CC BY). The use, distribution or reproduction in other forums is permitted, provided the original author(s) and the copyright owner(s) are credited and that the original publication in this journal is cited, in accordance with accepted academic practice. No use, distribution or reproduction is permitted which does not comply with these terms.
*Correspondence: Peipei Li, bGlwZWlwZWlAaGVuYXUuZWR1LmNu